Modulation of a rapid neurotransmitter receptor-ion channel by membrane lipids
- Biomedical Research Institute (BIOMED), Catholic University of Argentina (UCA)–National Scientific and Technical Research Council (CONICET), Buenos Aires, Argentina
Membrane lipids modulate the proteins embedded in the bilayer matrix by two non-exclusive mechanisms: direct or indirect. The latter comprise those effects mediated by the physicochemical state of the membrane bilayer, whereas direct modulation entails the more specific regulatory effects transduced via recognition sites on the target membrane protein. The nicotinic acetylcholine receptor (nAChR), the paradigm member of the pentameric ligand-gated ion channel (pLGIC) superfamily of rapid neurotransmitter receptors, is modulated by both mechanisms. Reciprocally, the nAChR protein exerts influence on its surrounding interstitial lipids. Folding, conformational equilibria, ligand binding, ion permeation, topography, and diffusion of the nAChR are modulated by membrane lipids. The knowledge gained from biophysical studies of this prototypic membrane protein can be applied to other neurotransmitter receptors and most other integral membrane proteins.
1 Introduction
Chemical signaling via hormones or neurotransmitters at the plasmalemma was acquired early in phylogeny. Though electrical signaling via low resistance intercellular gap junctions and extracellular electrical fields generated by the electrical activity of neurons are also operative mechanisms (Faber and Pereda, 2018), chemical signaling provided specificity to the messages conveyed by the coding molecules. Such an evolutionary event prompted the emergence of cell-surface receptors approximately 4,000 million years ago. One such group of membrane-associated receptors is the pentameric-gated ion channel (pLGIC) superfamily initially in prokaryotes and subsequently in eukaryotes (Ortells et al., 1992; Barrantes, 2015). The pLGIC superfamily includes the following neurotransmitter receptor families: the nicotinic acetylcholine receptors (nAChRs), the γ-aminobutyric acid (type A and C) receptors, the glycine receptors, subtype 3 of the serotonin receptor family, and the glutamate-gated chloride channel (Zoli et al., 2018). pLGICs are built following a common architecture: five subunits in a pseudo-symmetric layout surrounding an ion-permeation pathway, the ionic pore. The structural similitude is related to the evolutionary history and conservation of these membrane-bound proteins (Stroud and Finer-Moore, 1985; Le Novère and Changeux, 1995; Ortells, 1998; Barrantes, 2015; Tessier et al., 2017).
The adult neuromuscular junction is an exceptionally large synapse with ca. 107 nAChR molecules. Moreover, this exceptionally high number of receptors is arranged at densities of ∼10,000–20,000 μm-2 (Albuquerque et al., 1974; Fertuck and Salpeter, 1976; Land et al., 1977). These two characteristic features make the neuromuscular synapse a unique case, in clear contrast with the distribution and density of cholinergic receptors in brain (Borroni and Barrantes, 2021). Brain nicotinic receptors are found in the soma of neurons as well as in pre- post- and extra-synaptic regions. Of the multiple possible subunit combinations, the α4β2 and the α7 nAChRs are the most abundant oligomeric forms. Other subunit combinations and/or stoichiometries are less represented, usually in smaller quantities, and with limited regional representation (Dani and Bertrand, 2007; Taly et al., 2009).
Membrane protein topography is indivisibly paired to the host membrane composition and lateral/transversal organization of its lipid constituents. Membrane spatial organization is in turn a key modulator of membrane protein function. The tight correspondence between structure and function was finely optimized during the evolution of receptors for chemical neurotransmitters and hormones, membrane macromolecules for which efficacious signaling is closely linked to their topography and dynamics. The nAChR is a particularly well-studied case that has therefore served as a paradigmatic example of the pLGIC superfamily. This review focuses on the mechanisms of nAChR modulation by membrane lipids, organized considering the notion that the latter operate via both indirect and direct effects on membrane-embedded proteins. The nAChR fulfills the general criteria of a typical membrane-bound protein, though its abundance, density, and distinct functional properties dictate some singular modes of crosstalk with its membrane milieu.
2 Mechanisms of receptor protein modulation by membrane lipids
As mentioned, membrane lipids can modulate the proteins embedded in the bilayer by two non-exclusive mechanisms: direct or indirect.
2.1 Indirect modulation
Indirect effects of the lipid milieu on membrane-bound proteins are those exerted by general physical properties of the bulk bilayer, such as fluidity (Cooper, 1978; Mély-Goubert and Freedman, 1980; Pottel et al., 1983; Golfetto et al., 2013; de Mendoza and Pilon, 2019), elasticity (Needham and Nunn, 1990; Zeman et al., 1990; Strey et al., 1995; Chen and Rand, 1997; Bruno et al., 2007; Yersin et al., 2007), membrane curvature (Marsh, 1996b; Lundbaek et al., 1997), hydrostatic (Behan et al., 1992; Barshtein et al., 1997) and/or lateral pressure (Marsh, 1996a; Cantor, 1998; Laan et al., 2004; Heerklotz and Tsamaloukas, 2006). These physical properties, in turn, can be influenced by external factors, including drugs (Goldstein, 1984; Lehr et al., 1989), e.g., general anesthetics (Dilger et al., 1993; Franks and Lieb, 1994; Balasubramanian et al., 2002) or insecticides that alter membrane fluidity (Wolff and Bull, 1982; Moya-Quiles et al., 1996) and/or disease conditions like cancer (Schroeder, 1978; Pavel et al., 2020).
The nAChR is also a target of many of the above general, indirect modulatory factors operating via the general physical properties of the membrane bilayer. General anesthetics (Lin et al., 1991; Raines and McClure, 1997; Salord et al., 1997; Violet et al., 1997; Flood and Role, 1998; Raines and Zachariah, 1999) and alcohols are the most widely studied drugs in this regard. Straight-chain alcohols, from ethanol to octanol, inhibit the nAChR-induced current responses in a dose-dependent manner and reduce the apparent dissociation constant of the endogenous neurotransmitter, acetylcholine, by mechanisms presumably affecting membrane fluidity (Bradley et al., 1984). Patch-clamp recordings showed that both the conductance and the single-channel mean open time are reduced upon application of benzyl alcohol to muscle-type nAChRs (Bouzat and Barrantes, 1991). Ethanol is of particular relevance, since nicotine addiction is usually associated with alcohol consumption, and both drugs target the neuronal type α4β2 nAChR (Godden and Dunwiddie, 2002).
Shelby and Veatch have analyzed the indirect influences of the membrane physical state from a thermodynamic perspective and proposed that “plasma membranes have a high compositional susceptibility, arising from their thermodynamic state in a single phase that is close to a miscibility phase transition” (Shelby and Veatch, 2023). The single phase is purported to display coexisting compositional fluctuations and long-lived structures consisting of induced domains. The compositional fluctuations would provide the compositional diversity required for the multiplicity of biological functions of the plasmalemma. The model draws from the classical fluid-mosaic model of Singer and Nicolson, who proposed in 1972 that the biological membrane is a simple 2-dimensional fluid at equilibrium in which proteins and lipids are randomly distributed over distances larger than those dictated by direct interactions (Singer and Nicolson, 1972). In such a theoretical construct the lipid bilayer has been envisaged as a homogeneous phase that provides the substratum for solvating membrane-embedded proteins. The initial conceptualization has evolved over the course of decades (see the 40-year (Nicolson, 2014) and the more recent 50-year historical perspectives (Bechinger, 2023; Nicolson and Ferreira de Mattos, 2023)). Veatch and coworkers have also discussed the bilayer thermodynamic status as a fundamental property for tuning the composition of the membrane in response to external stimuli (Veatch et al., 2023).
The lipid composition of the host plasmalemma is a key, albeit indirect determinant of the conformational transitions and other functional properties of the nAChR. Early studies pointed to the lipid requirements for maintaining the receptor protein in an activatable conformational state that could eventually become unresponsive, i.e., desensitized, in the sustained presence of the agonist, (Epstein and Racker, 1978). Phospholipids were reported to stabilize the structure of the affinity purified receptor (Chang and Bock, 1979). The essential need to include cholesterol in reconstitution experiments was also an early finding (Criado et al., 1982a). Fatty acyl chain length and headgroup of the phospholipids were found to impact on the conformational equilibria of the receptor (Criado et al., 1984). Neutral and negatively charged phospholipids were analyzed in their capacity to stabilize receptor structure (Sunshine and McNamee, 1992). This led to the identification of phosphatidic acid (PA) as an anionic phospholipid playing a major role in the modulation of the nAChR (Bhushan and Mcnamee, 1993; Baenziger et al., 2000; Poveda et al., 2002; daCosta et al., 2002; Hamouda et al., 2006b; Cheng et al., 2007; Wenz and Barrantes, 2005).
Twenty years after the inception of the Singer and Nicolson model, Simons and Ikonen postulated a major challenge to the idea that the lipid bilayer constituted a homogeneous phase (Simons and Ikonen, 1997). These authors proposed the existence of lateral discontinuities in the membrane bilayer, thus introducing the concept of discrete domains whose composition differed from the rest of the membrane. They coined the term “raft” for such domains, enriched in cholesterol and sphingolipids, and drew important inferences about their functional implications, proposing that lipid rafts served as platforms for the attachment and sorting of proteins and were involved in signal transduction. Subsequent work from Simons’ laboratory explored the dynamics of glycosylphosphatidylinositol (GPI)-anchored and transmembrane protein-containing domains using single particle tracking, demonstrating the minute-long stability of discrete domains 26 ± 13 nm in diameter (Pralle et al., 2000). The degree of clustering and the dimensions of the GPI-anchored protein nanoclusters have been reported to be regulated by the lipid content -cholesterol in particular of these domains (Sharma et al., 2004).
Raft domains have been postulated to exhibit some of the characteristics of liquid-ordered (Lo) gels produced by synthetic lipid systems (Samsonov et al., 2001). Lo domains in biological membranes are characterized by their relative enrichment in cholesterol, sphingolipids and glycerophospholipids with saturated acyl chains (relative to the rest of the bilayer lipid) (see review in (Pike, 2006)). The initial characterization of “raft” domains, and the techniques employed to operationally define them, were of a biochemical nature. Homogenization of the tissue, ultracentrifugation and non-ionic detergent extraction led to the categorization of a detergent-insoluble fraction. This fraction was purported to be equivalent to the Lo-like domains in synthetic lipid systems and to occur in biological membranes.
In a recent review (Barrantes, 2023), I have critically analyzed the flaws of these biochemically-based criteria as applied to the nAChR-rich membrane domains. Using lipid mixtures with the composition of a Lo phase, no preferensolubility criteria, too broad to adequately assess the partitioning of the receptor into different lipid phases (Lichtenberg et al., 2005; Barrantes, 2007) or to be extrapolated to the conditions present in the living cell. Other direct high resolution co-localization studies are more suitable to resolve this important issue.
Indeed, Förster’s resonance energy transfer (FRET) microscopy was instrumental in providing one of the most solid experimental pieces of evidence of the nano-scale character of lipid domain structures, their active maintenance by the cell (Rao and Mayor, 2005), and their highly dynamic nature (Mayor and Rao, 2004; Sezgin et al., 2017). The “membrane domain” concept has been further expanded by experimental approaches demonstrating the effect of the underlying actin cytoskeleton on the compartmentalization and stability of lipid domains, and the various modalities (passive, neutral, active) under which the actin meshwork interacts with the membrane (Goswami et al., 2008; Raghupathy et al., 2015; Köster et al., 2016; Köster and Mayor, 2016). In its current, more complex version, the membrane domain concept encompasses a collection of lipid domain subtypes defined not only by the spatial compartmentalization produced by the lateral segregation of lipid species and their distinct chemical composition but more importantly by the signaling mechanisms they subserve (Anderson and Jacobson, 2002; Dietrich et al., 2002; Jacobson et al., 2019). This emphasis on the functional aspects was consolidated in the Keystone Symposium consensus definition of rafts as “small (10–200 nm), heterogeneous, highly dynamic sterol- and sphingolipid-enriched domains that compartmentalize cellular processes” (reviewed by (Pike, 2006)). Thus, despite their size and compositional heterogeneity, lipid domains can be defined by their ability to indirectly modulate membrane protein function, especially signaling.
In the field of the nAChR, raft-type lipid domains have been reported to be necessary for the stability of the α7-type nAChR in neurons (Brusés et al., 2001). Similarly, ordered lipid domains appear to be required for the clustering of nAChRs in muscle cells (Stetzkowski-Marden et al., 2006; Willmann et al., 2006; Zhu et al., 2006). The receptor-aggregating protein agrin triggers the initial steps leading to receptor aggregation in these discrete lateral lipid domains (Moransard et al., 2003; Mittaud et al., 2004). Purified nAChR reconstituted in lipid mixtures of varying composition, complexity, and morphology (single-to multi-layer vesicles, planar bilayers, giant unilamellar vesicles) (Popot et al., 1977; Anholt et al., 1980; Dalziel et al., 1980; Boheim et al., 1981; Wu and Raftery, 1981; Martinez et al., 2002) has proved to be an efficient experimental paradigm to test indirect membrane effects on membrane protein function. The purified nAChR did not show any preferential partitioning into Lo-type lipid mixtures (1:1:1 cholesterol:palmitoyloleoylphosphatidylcholine:sphingomyelin) (Bermudez et al., 2010). However, the asymmetry of the reconstituted lipid membrane resulting from inclusion of brain sphyngomyelins in the lipid mixture that modified the distribution of lipids across the reconstituted membrane induced favored nAChR partitioning in the Lo phase (Perillo et al., 2016).
Research on lipid domains in membranes has extended to the search for optimal lipid mixtures that fulfill two criteria: i) retain functional properties of the membrane protein upon affinity purification and reconstitution and ii) preserve the structure as close as possible to that of the native conformation. There has been a long search for the optimization of mild detergents and amphiphiles (Popot, 2010; Le Bon et al., 2021) to undertake structural cryo-electron microscopy studies on membrane proteins, inherently difficult to crystalize in 3 dimensions. Early attempts in this direction applied to the nAChR met with moderate success (Hertling-Jaweed et al., 1988; Schürholz et al., 1989). The more recent achievements of high-resolution studies of the nAChR by X-ray and cryo-electron microscopy (cryo-EM) methods are analyzed in Section 5.
2.2 Direct modulation
Direct modulation takes place when lipids exert their action upon binding to sites predominantly located on lipid-exposed domains of the protein. Direct modulation does not necessarily imply high affinity binding of specific lipids to the membrane protein (both low- and high-affinity binding modalities are found in Nature), though the degree of stereoselectivity is, in general, higher than that of lipids exerting indirect modulation of the membrane-embedded protein.
It was early realized that indirect effects could be targeted to the transmembrane domains of the nAChR protein (Fong and McNamee, 1986; White and Cohen, 1988; Blanton and Cohen, 1992; Fernandez-Ballester et al., 1994; Addona et al., 1998; Baenziger et al., 2000)). Exceptions to this rule can be found in the effect of ethanol on the homomeric neuronal-type α7 nAChR, which exhibits direct inhibition upon binding of the alcohol to its N-terminal extra-membranous domain (Yu et al., 1996). Using a physical technique (electron spin resonance (ESR) spectroscopy) we found that in native postsynaptic membranes from Torpedo marmorata electric tissue, a fraction of the lipids was relatively immobile (Marsh and Barrantes, 1978). Subsequent ESR and fluorescence experiments refined this picture (Marsh et al., 1981; Arias et al., 1990; Horvath et al., 1990) and provided information on the stoichiometry of cholesterol sites on the nAChR. About 15 cholesterol molecules could be accommodated around the transmembrane perimeter of the nAChR (Mantipragada et al., 2003). More recent work identified a discrete number of motifs with the expected amino acid residues in linear sequences favourable to the binding of cholesterol molecules. These linear motifs are found predominantly in crevices on the lipid-exposed surface of the nAChR transmembrane peptides (see Section 4.3 below).
The ESR experiments provided an additional -and unexpected-piece of information on the lipid-nAChR protein interactions: the proportion of immobilized lipid was higher than that calculated for a single boundary layer around the protein. In fact, immobilization extended to the totality of the interstitial lipid located between adjacent protein molecules in native receptor-rich postsynaptic membranes from T. marmorata (Marsh and Barrantes, 1978). Subsequent fluorescence quenching experiments defined a class of phospholipid sites readily exchangeable with the bulk lipid, in contradistinction to non-annular sites, accessible only to cholesterol and not to phospholipids (Jones and McNamee, 1988a). The interpretation of the fluorescence experiments in terms of “annular/non-annular” lipids was based on the additional intrinsic fluorescence quenching produced by dibromo-cholesterol when the receptor was reconstituted (and hence already partially quenched) in dibromo-dioleoyl-phosphatidylcholine liposomes. The additional quenching was assumed to unveil the presence of cholesterol sites inaccessible to phospholipids. This issue has been recently revisited, and the notion of annular vs. non-annular lipids in the context of membrane protein-associated lipid has been qualified as a myth (Gómez-Fernández and Goñi, 2022).
The early ESR experimental data on native receptor-rich membranes bear direct relevance to the physical status of the lipid in the actual neuromuscular synapse. As emphasized in the Introduction, the nAChR is present at extremely high densities in quasi-crystalline 2-dimensional arrays in the neuromuscular junction (Heuser and Salpeter, 1979), as is the case with the electromotor synapse of electric fish. Hence, in these two postsynaptic membranes interstitial lipid occupies the remaining available volume between adjacent receptor macromolecules: the emerging picture is not a sea of lipids with isolated “iceberg-resembling” receptor macromolecules but rather a large 2-D “picket” of nAChRs and lipid filling in the gaps (Barrantes, 2004). This implies that each receptor cylinder, ∼8 nm in diameter, exerts a gradient of influence that extends for only a few lipid layers and is shared within a few nanometers with equivalent influences from neighboring receptors (Figure 1).
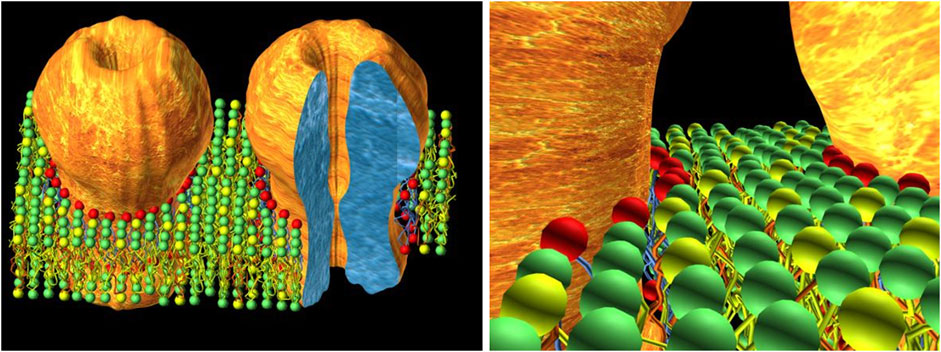
FIGURE 1. The concept of interstitial lipid. On the left, a schematic cartoon depicting the close proximity of nAChR molecules in the postsynaptic membrane, typical of the adult neuromuscular junction (and the electromotor synapse), where nAChR densities can reach values of 10,000–20,000/μm2 (Heuser and Salpeter, 1979). The tightly-packed 2D array of nAChRs (only two molecules are shown for the sake of clarity) leaves only a few concentric layers of lipid molecules filling the interstices between receptor macromolecules. Colored spheres represent the polar head groups of different phospholipids. The red spheres correspond to the first-layer lipid, exchanging rapidly with other layers and likely involved in direct modulation of the nAChR. The second and third layers (green and yellow spheres) beyond the first-layer lipid of a given nAChR molecule are shared without boundaries with the homologous layers of vicinal lipids surrounding adjacent neighboring receptors. The cartoon on the right shows this in more detail. This interstitial lipid thus consists of only a very few layers, with little if any “bulk” lipid in the neuromuscular junction; it is a common territory shared by neighboring receptors. The experimental basis of this concept relies on early ESR (Marsh and Barrantes, 1978; Marsh et al., 1981) and electron microscopy (Fertuck and Salpeter, 1976; Heuser and Salpeter, 1979; Salpeter and Loring, 1985). The cartoon on the right is taken from Barrantes, (2004) with permission from Elsevier.
The lipid-protein interactions at the peripheral adult muscle-type and electromotor synapses may thus be unique and current attempts to understand them using single-molecule reconstituted systems may fall short of providing a realistic depiction of the conditions present at the uniquely large and densely crowded peripheral synapse. The valuable inferences on lipid-protein interactions drawn from the cryo-electron microscopy (see Section 5 below) and in silico (this Section) studies using single nAChR molecules in lipid nanodiscs are more pertinent to the conditions met at the less populated central nervous system synapses and at early ontogenetic neurodevelopmental stages of the neuromuscular junction. In the latter scenario, between embryonic day 13 and 14, individual muscle-type nAChRs diffuse in the plane of the membrane (see Section 6 on receptor dynamics below) and begin to associate (Sanes and Lichtman, 2001), in a process that I have figuratively called “receptor socialization”, leading to the formation of clusters of nanoscopic dimensions (Barrantes, 2022), different from the micron-sized clusters found in postnatal life (Figure 2). The nAChR nanoclusters likely constitute the intermediate supramolecular organization leading to the meso-scale “microaggregates” observed during the embryonic stages of neuromuscular development ((Olek et al., 1986a; Olek et al., 1986b) reviewed in (Sanes and Lichtman, 2001)) as well as in mammalian cells heterologously expressing nAChRs (Olson et al., 1983; Borroni et al., 2007).
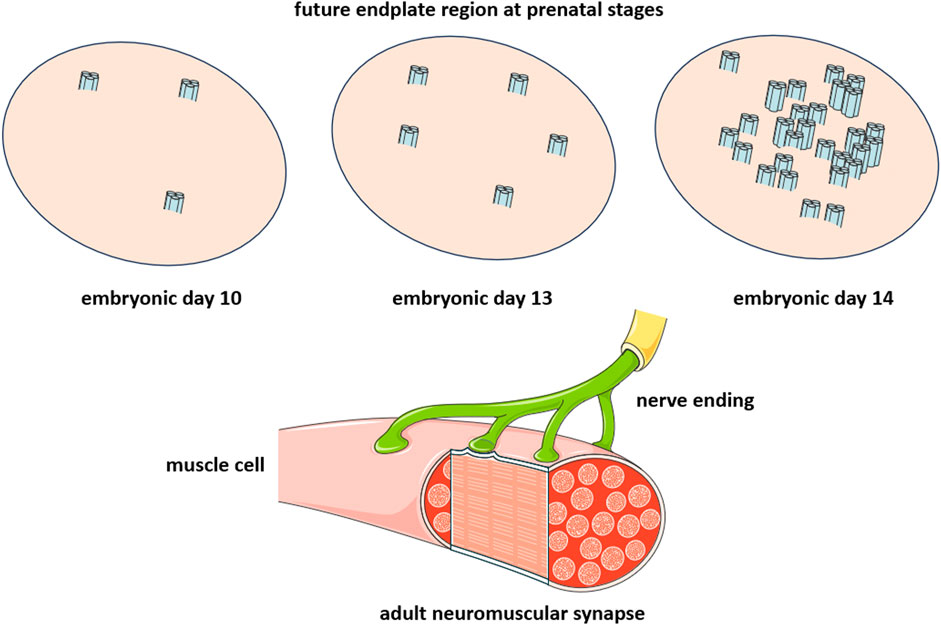
FIGURE 2. Critical developmental stage of the peripheral cholinergic synapse, the neuromuscular junction. The diagram is intended to emphasize the change occurring within a time window of 24 h between embryonic day 13 (E13) and embryonic day 14 (E14), during which individual nAChR molecules associate to form nanoclusters consisting of a few nAChR molecules. The nerve endings arrive during postnatal life (lower diagram), leading to the establishment of the neuromuscular junction (see (Sanes and Lichtman, 2001) for a review).
Jonathan Cohen and Michael Blanton pioneered the use of photoaffinity labeling techniques to identify lipid sites on the nAChR molecule (Blanton and Cohen, 1992; Blanton and Cohen, 1994; Blanton et al., 2001; Hamouda et al., 2006a; Hamouda et al., 2007; Hamouda et al., 2008). Miller and coworkers employed similar approaches to characterize anesthetic sites at the lipid-nAChR interface (Forman et al., 2015). Currently there are more than 100 crystal structures of pLGICs in the Protein Data Bank (PDB). Searching for putative drug sites for general anesthetics, anxiolytics, smoking cessation drugs, and antiemetics on the transmembrane regions of the different members of the superfamily revealed the presence of recognition sites, some of which may be unique to individual receptors, while others were found to be broadly conserved among pLGIC members (Koniuszewski et al., 2022). Most recently, insecticides targeting pLGICs have been described as “structural mimetics of neurotransmitters that manipulate and deregulate” these channel proteins (Raisch and Raunser, 2023).
A minimal number of 45 lipid molecules per nAChR molecule were reported to be required to sustain ion permeation (Jones et al., 1988). Jones and McNamee found 5 to 10 “non-annular lipid” sites per nAChR monomer (Jones and McNamee, 1988b). The sterol, androstanol, was found to display a higher selectivity for the nAChR (relative to PC). Thirty-eight sterol sites per nAChR were reported by the group of McNamee (Ellena et al., 1983). ESR studies documented the presence of nAChR-immobilized PA (Ellena et al., 1983). A recent coarse-grained molecular dynamics study using a host bilayer including 30 different lipids (Sharp and Brannigan, 2021) was used to categorize lipid sites in a neuronal-type nAChR. Two classes of sites were identified: an inter-subunit site deep inside the receptor molecule, flanked by M1-M2 in a given subunit and M2-M3 of the adjacent subunit, and a peripheral, lipid-exposed site on the M4 transmembrane domain (Brannigan et al., 2008). Cholesterol was found to exhibit higher affinity for the external hemilayer. Phosphatidylinositol (PI), phosphatidylserine (PS), and phosphatidylcholines (PC) had stronger affinities for these sites than the phosphoinositides PIP1, PIP2, PIP3. The latter exhibited higher affinity than PA. From strongest to weakest, the affinities of the phospholipids for the inner hemilayer sites follow the order: PE > PI ∼ PS ∼ PC ≫ PIP1 ∼ PIP2. In the case of the M4 site, the order of affinities, from strongest to weakest, was: PE > PI > PS > PC ≫ PIP1 ∼ PIP2 ∼ PIP3 ∼ PA (Sharp and Brannigan, 2021).
The combination of biochemical and biophysical studies has contributed to establishing the important modulatory role of cholesterol in nAChR function (reviewed in (Barrantes, 1992; Barrantes, 2004). One area that still demands attention is the identification of specific sites for the sterol. An in silico search disclosed the occurrence of linear amino acid sequences compatible with recognition motifs CRAC and/or CARC (Baier et al., 2011). The amino acid consensus sequence termed ‘‘CRAC’’ (cholesterol recognition/interaction amino acid consensus) is usually found in the juxtamembrane region of the nAChR, with the pattern–L/V–(X) (1–5)–Y–(X) (1–5)–R/K–, where (X) (1–5) represents between one and five residues of any amino acid (Baier et al., 2011). The individual energetic contribution of the motifs was found to vary among the different transmembrane segments. The combination of mutagenesis and lipid monolayer studies established the role of key amino acid residues in determining cholesterol affinity. Recent cryo-EM studies reviewed in Section 4 below have provided experimental confirmation of these motifs in the nAChR transmembrane region (see also review in (Barrantes, 2023).
3 Physical state of the nAChR-vicinal lipid
Comparison of the general polarization (GP) of the fluorescent probe Laurdan and single-channel patch-clamp data obtained on two different clonal cell lines disclosed a correlation between these two parameters, pointing to the differential influence of cell-specific lipid compositions on the functional (ion permeation) properties of the receptor (Zanello et al., 1996). The inception of the FRET technique in the Laurdan GP field enabled us to examine the physical state of the interstitial lipid, i.e., the lipid microenvironment in close proximity to the receptor. The FRET pair used was the tryptophan fluorescence of the receptor (donor) and the Laurdan molecule (acceptor). Native T. marmorata nAChR-rich membranes and reconstituted liposomes made up of synthetic phosphatidylcholines were compared (Antollini et al., 1996). FRET-GP within donor-acceptor distances of 14 ± 1 Å served as a sensor of interstitial lipid in very close contact with the receptor protein. In coincidence with the relative lipid immobilization reported in ESR experiments (Marsh and Barrantes, 1978), the FRET-GP experiments revealed a lower polarity and an increased solvent dipolar relaxation at the hydrophilic/hydrophobic interface in native membranes relative to diluted nAChR-containing reconstituted systems (Antollini et al., 1996). In model lipid membranes, incorporation of the nAChR increases the proportion of non-hydrogen bonded lipid ester carbonyl groups (daCosta et al., 2002).
Pyrene, an organic planar molecule characterized by its extremely long fluorescence lifetime, has been used to covalently tag lipids, resulting in fluorescent probes that incorporate readily into membranes because of the highly hydrophobic fluorophore moiety. These probes form excimers When a pyrene molecule in the excited state collides with another pyrene molecule in the ground state, an excimer is formed. Successful collisions depend on both concentration and lateral diffusion of the probe (Galla and Hartmann, 1980; Holopainen et al., 1997; Somerharju, 2002). Pyrene phospholipid derivatives partition preferentially in lipid-fluid phases (Somerharju et al., 1985; Jones and Lentz, 1986; Koivusalo et al., 2004), and form probe-enriched domains (“patches”) laterally segregated from lipids in the gel state (Holopainen et al., 1997; Holopainen et al., 1998; Holopainen et al., 2001). Excimer/monomer ratiometric measurements revealed the appearance of distinct lateral heterogeneities produced by addition of the receptor protein to pure DPPC/DOPC liposomes or the same supplemented by saturated lipid (palmitoyl-oleoyl-PC or palmitoyl-oleoyl-PA). Complementary measurements of FRET from the protein to the probe diphenylhexatriene (DPH) further showed that the nAChR partitioned preferentially into POPC- or POPA-enriched, relatively more rigid domains (Wenz and Barrantes, 2005).
4 Cryo-EM structures reveal lipid sites on the nAChR surface
The successful combination of lipid-mimicking detergents and lipid mixtures known to support nAChR function led to the obtention of nanodiscs apt for high-resolution structural methods (Padilla-Morales et al., 2011; Quesada et al., 2016; Delgado-Vélez et al., 2021). The 3.94 Å resolution obtained by X-ray diffraction studies of the neuronal-type α4β2 nAChR provided one of the first glimpses at the atomic structure of a complete nAChR molecule (Morales-Perez et al., 2016). This was followed by studies of the other most abundant homomeric neuronal-type α7 nAChR (PDB: 7KOQ) (Noviello et al., 2021).
4.1 Phospholipid sites revealed by cryo-EM on the nAChR transmembrane surface
The atomic structure of the muscle-type nAChR and the localization of lipid sites was tackled more recently with cryo-EM techniques using the Torpedo receptor reconstituted in lipid nanodiscs. Densities attributable to phosphoglyceride sites could be resolved (Rahman et al., 2020; Rahman et al., 2022; Zarkadas et al., 2022). In the Zarkadas and coworkers study, (PDB: 7QL5), phospholipid sites (6–11) on the receptor transmembrane region could be identified, distinguishing whether the receptor was reconstituted in nanodiscs in the presence or absence of cholinergic ligands. PC sites were apparent at i) the inner, cytoplasmic facing hemilayer, sandwiched between the M3 helix of the principal subunit, and another charged residue (Lys or Arg) in the complementary M4 domain and ii) at the outer leaflet, in a shallow cavity flanked by M3, the M2-M3 loop, and the Cys loop from the principal subunit, and the M1 from the complementary subunit (Zarkadas et al., 2022). A detailed account of the nAChR-lipid structural data has recently been published (Barrantes, 2023).
4.2 Cholesteryl ester sites revealed in the neuronal nAChR transmembrane domains
The structure of another neuronal-type nAChR, the ganglionic α3β4 receptor, could be elucidated (PDB: 6PV7) (Gharpure et al., 2019) upon reconstitution into nanodiscs that included ion channel function-supporting lipids, supplemented with cholesteryl hemisuccinate, a water-soluble cholesteryl ester (Criado et al., 1982a), and PA. Cryo-EM densities interpreted as cholesteryl ester sites were identified on the α3β4 nAChR at the M4-M1 and the M4-M3 transmembrane interfaces of each subunit (Gharpure et al., 2019). Hibbs and coworkers had previously observed two “bowl-shaped” cholesterol sites per receptor subunit on the inner hemilayer transmembrane region in the α4β2 nAChR (Walsh et al., 2018). Each cholesterol molecule established contacts almost exclusively with a given subunit and with another adjacent cholesterol molecule in a pairwise fashion, a motif identified by Unwin in the Torpedo electromotor nAChR (Unwin, 2020). Cryo-EM of the detergent-solubilized nAChR protein from Torpedo californica in reconstituted soybean lipid-saposin nanodiscs at 2.7 Å resolution (PDB: 6UWZ) (Rahman et al., 2020) identified densities “consistent with a bound lipid” at the base of the a subunit M4 transmembrane domain. The possible role of cholesterol in receptor desensitization was addressed in a subsequent work (Zhuang et al., 2022).
4.3 Low- and high-affinity cholesterol sites revealed by cryo-EM on the Torpedo nAChR surface
A cryo-EM study from Hibbs´ group reported the structure at 2.51 Å resolution of the “apo”-conformer of the Torpedo nAChR in the absence of ligands (PDB: 7SMM) in a soybean lipid mixture (PDB: 7SMM) (Rahman et al., 2022). Comparison of the apo-conformer with the structure of the receptor in the same lipid mixture but with added cholesterol (PDB: 7SMQ), permitted Hibbs and coworkers to distinguish cholesterol sites from phospholipid sites, and identify cholesterol sites of high- and low-affinity on the nAChR surface at the outer and inner lipid hemilayers, respectively (Figure 3). In the apo-nAChR without exogenously added cholesterol, 4 to 5 cholesterol molecules remained bound to the nAChR upon elution from affinity chromatography, and the corresponding densities identified in the inner, cytoplasmic-facing hemilayer were therefore assumed to correspond to tightly-bound endogenous cholesterols (3 per nAChR monomer). These high affinity sites are hydrophobic pockets formed by the M4, M3 and MX transmembrane domains on the principal face of the two a subunits and the single ß subunit. About 25 bound cholesterol molecules were calculated to be present in the nanodisc in samples with exogenously added cholesterol, depicting cholesterol densities at the extracellular-facing hemilayer. These outer leaflet-facing cholesterol molecules were interpreted as low-affinity cholesterol binding sites.
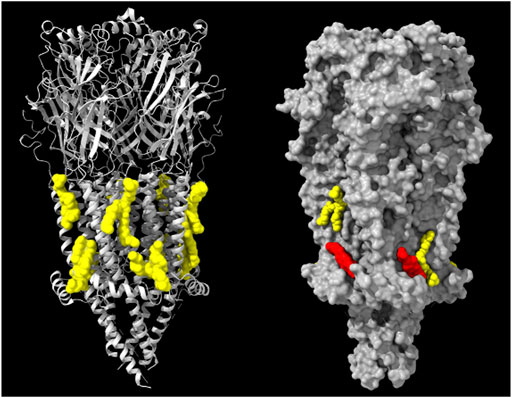
FIGURE 3. Left: Model derived from the cryo-EM data of the Torpedo (Tetronarce) californica electric organ muscle-type nAChR (grey ribbon rendering) with 8 phospholipid (phosphatidyloleoyl-PC, POPC) molecules (yellow) on both hemilayers of the receptor transmembrane region (PDB: 7QL5) (Zarkadas et al., 2022). Right: Model derived from the cryo-EM data of the Torpedo californica electric organ muscle-type nAChR (grey surface rendering) with 2 phospholipid molecules (yellow) on both hemilayers and two cholesterol molecules (red) present only in the cytoplasmic-facing hemilayer of the receptor transmembrane region (PDB: 7SMT). From ref. (Zarkadas et al., 2022). Molecular graphics performed with UCSF ChimeraX (Pettersen et al., 2004).
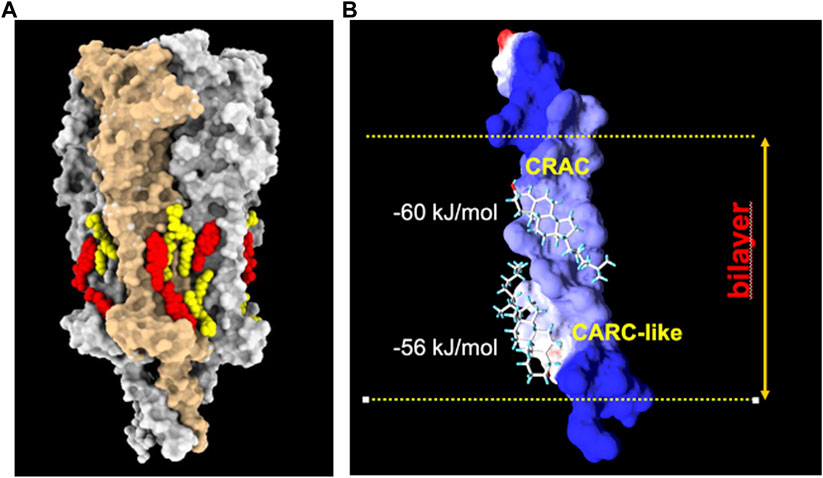
FIGURE 4. (A): Model derived from the cryo-EM data of the Torpedo californica electric organ muscle-type nAChR (grey surface rendering) reconstituted in nanodisc with exogenously added soybean lipids. Under these experimental conditions, phospholipid (yellow) and cholesterol (red) molecules are observed on both hemilayers of the receptor transmembrane region (PDB: 7SMQ). Notice the preference of lipids for cavities or crevices between adjacent subunits, and the end-to-end cholesterol doublets on the inner and outer segments of the same transmembrane helices. From ref. (Rahman et al., 2022). Molecular graphics performed with UCSF ChimeraX (Pettersen et al., 2004). (B): Cholesterol doublets in the γM4 transmembrane domain of the human nAChR, with the calculated free energy of interaction with the CRAC and CARC-like linear cholesterol consensus domains at the exofacial (top)- and cytoplasmic-facing hemilayers, respectively (reviewed in ref (Fantini et al., 2019).). Reproduced from Barrantes, 2023, an open access article distributed under the terms of the Common Access CC BY licence.
An interesting additional feature emerged from the cryo-EM atomic structures: the presence of cholesterol “doublets” on the same transmembrane segment of the nAChR (Rahman et al., 2022). This observation provided experimental confirmation of the “mirror code” motif disclosed by molecular dynamics calculations for the nAChR and a great variety of membrane-embedded proteins, including pLGICs and G-protein coupled receptors (Fantini et al., 2016). The mirror code consists of two end-to-end or tail-to-tail cholesterol recognition linear consensus domains (Figure 4). The Supplementary Information video provides a complementary dynamic 3D depiction of the Torpedo electric organ (muscle-type) nAChR (PDB 7QKO, (Zarkadas et al., 2022)) and cholesterol molecules on its surface (Figure 3).
5 Fluorescence microscopy to study the nanoscale and mesoscale dynamics of nAChRs in the plasmalemma
Learning about the modulation of nAChR dynamics by the lipid environment is particularly important for understanding the neurodevelopmental aspect of synaptogenesis. As discussed under Section 3.2 above, the motions of the receptor at embryonic stages play a fundamental role in redistributing the molecules at the muscle sarcolemma prior to the arrival of the peripheral motor nerve terminal. The nanoclusters formed at early embryonic stages precede the arrival of the nerve endings (which occurs in postnatal life). The process of receptor nanocluster assembly is fundamentally driven by lateral diffusion of individual molecules on the muscle cell membrane, thus leading to encounters with other receptor macromolecules and eventually to their aggregation. Nanoaggregates coalesce into patches of micrometric dimensions, still prior to the arrival of the nerve endings. The embryonic stages find the striated muscle cells covered with large patches (20–60 μm) that precede the fully developed muscle endplate. Translational diffusion, i.e., lateral motion, is therefore a major motor in the ontogenetic development of the neuromuscular junction, as is the case with the lateral motion of receptors from non-synaptic areas to the synaptic region in brain synapses.
Daniel Axelrod (Axelrod et al., 1976) pioneered the use of the fluorescence recovery after photobleaching (FRAP) technique for the study of nAChR translational dynamics. In postnatal developing myoblasts, the majority of the nAChRs in 20–60 μm patches are immobile; their average lateral diffusion coefficient (D) is <10−4 μm2 s-1. nAChRs in non-patched regions are diffusely distributed on the plasma membrane and display D values ∼0.5 × 10−2 μm2 s-1. FRAP was also applied to learn about lateral diffusion of purified nAChR monomers and dimers reconstituted into different lipid systems (Criado et al., 1982b). We compared the behavior of the two oligomeric forms of the receptor in pure dimyristoyl-phosphatidylcholine (DMPC) versus DMPC-cholesteryl hemisuccinate mixtures or soybean phospholipids and observed, as expected, a marked drop in diffusion coefficient as the temperature was reduced from 37°C to 14°C.
Immobilization of nAChRs is also observed in CHO-K1/A5 mammalian clonal cells expressing muscle-type nAChR but devoid of non-receptor proteins that cluster receptor molecules (Roccamo et al., 1999). FRAP combined with fluorescence correlation spectroscopy (FCS) (Baier et al., 2010) showed that ca. 55% of the receptors did not exhibit lateral motion. Cholesterol depletion reduced the fraction of mobile receptors even further (from 55% to 20%) and reduced the diffusion of the mobile receptor fraction, an observation that was subsequently interpreted as resulting from an increase in the size of the nAChR nanoclusters observed with STED nanoscopy (Kellner et al., 2007). These experiments provided evidence that nAChR nanocluster formation is strongly driven by receptor-receptor interactions and is a cholesterol-dependent phenomenon.
A subsequent series of experiments employed total internal reflection fluorescence (TIRF) microscopy to study receptor diffusion in cells tagged with fluorescent α-bungarotoxin or monoclonal anti-receptor antibodies (Almarza et al., 2014). Fluorescent α-bungarotoxin revealed a wide range of receptor mobilities including a highly mobile population (16%), a freely moving Brownian component, and a main component with restricted motion, amounting to ∼50% of the total. The rest (44%) of the nAChR molecules were considered immobile The TIRF experiments confirmed the previous FRAP/FCS results (Baier et al., 2010) showing that a large proportion of the receptors at the plasma membrane are immobile, while the mobile nAChR population is quite heterogeneous, displaying a complex spectrum of diffusional modalities, modulated by cholesterol content.
More recent experiments showed that the “highly mobile” population corresponds to superdiffusing molecules, whereas the predominant restricted motion populations correspond to various subdiffusive subpopulations (Mosqueira et al., 2018). A similar study using monoclonal antibodies (Mosqueira et al., 2020) addressed the in vitro effect of mAb35, an antibody that mimics the effects observed in vivo in the rare and severe autoimmune disease, myasthenia gravis (Tzartos and Lindstrom, 1980; Tzartos et al., 1981). Upon application of mAb35 to the CHO-K1/A5 muscle-type model cells, larger nAChR nanoclusters and slower lateral diffusion were observed (Mosqueira et al., 2020), in contrast with receptors labeled with fluorescent α-bungarotoxin, which does not crosslink the nAChR (Mosqueira et al., 2018). These biophysical studies bear relevance to the pathogenesis of myasthenia gravis: circulating anti-nAChR autoantibodies in myasthenic patients crosslink nAChR molecules and trigger their internalization (Drachman et al., 1978). Moreover, with Satyajit Mayor in Bangalore, we could reproduce the increased rate of receptor internalization upon mAb35 binding to developing myoblasts and CHO-K1/A5 cells (Kumari et al., 2008).
The combined SPT-SMLM study further showed that mAb35-induced crosslinking results in increases in the percentage of immobile nAChR molecules (∼80%) (Mosqueira et al., 2020). Moreover, antibody-tagged nAChRs interrupt their motion for periods of confinement lasting for 340–440 ms (Mosqueira et al., 2020). α-bungarotoxin tagged nAChRs also make stopovers, but for much shorter periods (135–257 ms) (Mosqueira et al., 2018).
6 Concluding remarks
The decades-long studies on nAChR-lipid interactions have provided a great deal of information on the modulation of the protein by membrane lipids. This review has highlighted the exceptional properties of the neuromuscular synapse, both in terms of absolute numbers and density of its receptor dotation, which dictate unique modes of lipid modulation of the protein and more importantly, underscore the significant influence of the nAChR on its interstitial lipid microenvironment. Contemporary atomistic structural studies are increasingly contributing to the identification and characterization of sites on the nAChR transmembrane domains that fulfill the requirements for lipid binding sites of low- and high-affinity and, importantly, to confirming many of the properties of these sites previously characterized by biochemical and biophysical approaches.
Author contributions
FB: Conceptualization, Project administration, Visualization, Writing–original draft, Writing–review and editing.
Funding
The author declares that no financial support was received for the research, authorship, and/or publication of this article.
Acknowledgments
I thank Ana Sofía Vallés for help with Figure 2, Carlos Javier Baier for providing Supplementary Video S1, and Phyllis Johnson for critical reading of the manuscript. ChimeraX (Pettersen et al., 2004) from the University of California at San Francisco was used to produce the molecular models of the nAChR-lipids derived from cryo-EM data.
Conflict of interest
The author declares that the research was conducted in the absence of any commercial or financial relationships that could be construed as a potential conflict of interest.
Publisher’s note
All claims expressed in this article are solely those of the authors and do not necessarily represent those of their affiliated organizations, or those of the publisher, the editors and the reviewers. Any product that may be evaluated in this article, or claim that may be made by its manufacturer, is not guaranteed or endorsed by the publisher.
Supplementary material
The Supplementary Material for this article can be found online at: https://www.frontiersin.org/articles/10.3389/fcell.2023.1328875/full#supplementary-material
References
Addona, G. H., Sandermann, H., Kloczewiak, M. A., Husian, S. S., and Miller, K. W. (1998). Where does cholesterol act during activation of the nicotinic acetylcholine receptor? Biochim. Biophys. Acta. 1370, 299–309. doi:10.1016/s0005-2736(97)00280-0
Albuquerque, E. X., Barnard, E. A., Porter, C. W., and Warnick, J. E. (1974). The density of acetylcholine receptors and their sensitivity in the postsynaptic membrane of muscle endplates. Proc. Natl. Acad. Sci. U.S.A. 71, 2818–2822. doi:10.1073/pnas.71.7.2818
Almarza, G., Sanchez, F., and Barrantes, F. J. (2014). Transient cholesterol effects on nicotinic acetylcholine receptor cell-surface mobility. PLoS One 9, e100346. doi:10.1371/journal.pone.0100346
Anderson, R. G., and Jacobson, K. (2002). A role for lipid shells in targeting proteins to caveolae, rafts, and other lipid domains. Science 296, 1821–1825. doi:10.1126/science.1068886
Anholt, R., Lindstrom, J., and Montal, M. (1980). Functional equivalence of monomeric and dimeric forms of purified acetylcholine receptors from Torpedo californica in reconstituted lipid vesicles. Eur. J. Biochem. 109, 481–487. doi:10.1111/j.1432-1033.1980.tb04819.x
Antollini, S. S., Soto, M. A., Bonini de Romanelli, I. C., Gutierrez-Merino, C., Sotomayor, P., and Barrantes, F. J. (1996). Physical state of bulk and protein-associated lipid in nicotinic acetylcholine receptor-rich membrane studied by laurdan generalized polarization and fluorescence energy transfer. Biophys. J. 70, 1275–1284. doi:10.1016/S0006-3495(96)79684-4
Arias, H. R., Sankaram, M. B., Marsh, D., and Barrantes, F. J. (1990). Effect of local anaesthetics on steroid-nicotinic acetylcholine receptor interactions in native membranes of Torpedo marmorata electric organ. Biochim. Biophys. Acta 1027, 287–294. doi:10.1016/0005-2736(90)90320-n
Axelrod, D., Koppel, D. E., Schlessinger, J., Elson, E., and Webb, W. W. (1976). Mobility measurement by analysis of fluorescence photobleaching recovery kinetics. Biophys. J. 16, 1055–1069. doi:10.1016/S0006-3495(76)85755-4
Baenziger, J. E., Morris, M. L., Darsaut, T. E., and Ryan, S. E. (2000). Effect of membrane lipid composition on the conformational equilibria of the nicotinic acetylcholine receptor. J. Biol. Chem. 275, 777–784. doi:10.1074/jbc.275.2.777
Baier, C. J., Fantini, J., and Barrantes, F. J. (2011). Disclosure of cholesterol recognition motifs in transmembrane domains of the human nicotinic acetylcholine receptor. Sci. Rep. 1, 69. doi:10.1038/srep00069
Baier, C. J., Gallegos, C. E., Levi, V., and Barrantes, F. J. (2010). Cholesterol modulation of nicotinic acetylcholine receptor surface mobility. Eur. Biophys. J. 39, 213–227. doi:10.1007/s00249-009-0521-2
Balasubramanian, S. V., Campbell, R. B., and Straubinger, R. M. (2002). Propofol, a general anesthetic, promotes the formation of fluid phase domains in model membranes. Chem. Phys. Lipids 114, 35–44. doi:10.1016/s0009-3084(01)00199-2
Barrantes, F. J. (1992). Structural and functional crosstalk between acetylcholine receptor and its membrane environment. Mol. Neurobiol. 6, 463–482. doi:10.1007/BF02757947
Barrantes, F. J. (2004). Structural basis for lipid modulation of nicotinic acetylcholine receptor function. Brain Res.Brain res.rev. 47, 71–95. doi:10.1016/j.brainresrev.2004.06.008
Barrantes, F. J. (2007). Cholesterol effects on nicotinic acetylcholine receptor. J. Neurochem. 103 (Suppl. 1), 72–80. doi:10.1111/j.1471-4159.2007.04719.x
Barrantes, F. J. (2015). Phylogenetic conservation of protein–lipid motifs in pentameric ligand-gated ion channels. Biochimica Biophysica Acta (BBA) - Biomembr. 1848, 1796–1805. doi:10.1016/j.bbamem.2015.03.028
Barrantes, F. J. (2022). Fluorescence microscopy imaging of a neurotransmitter receptor and its cell membrane lipid milieu. Front. Mol. Biosci. 9, 1014659. doi:10.3389/fmolb.2022.1014659
Barrantes, F. J. (2023). Structure and function meet at the nicotinic acetylcholine receptor-lipid interface. Pharmacol. Res. 190, 106729. doi:10.1016/j.phrs.2023.106729
Barshtein, G., Bergelson, L., Dagan, A., Gratton, E., and Yedgar, S. (1997). Membrane lipid order of human red blood cells is altered by physiological levels of hydrostatic pressure. Am.J.Physiol.Heart Circ.Physiol. 272, H538–H543. doi:10.1152/ajpheart.1997.272.1.H538
Bechinger, B. (2023). Special issue: 50 years of the fluid mosaic model for cell membranes. Biochimica Biophysica Acta (BBA) - Biomembr. 1865, 184181. doi:10.1016/j.bbamem.2023.184181
Behan, M. K., Macdonald, A. G., Jones, G. R., and Cossins, A. R. (1992). Homeoviscous adaptation under pressure: the pressure dependence of membrane order in brain myelin membranes of deep-sea fish. Biochim.Biophys.Acta Bio-Membr. 1103, 317–323. doi:10.1016/0005-2736(92)90102-r
Bermudez, V., Antollini, S. S., Fernandez Nievas, G. A., Aveldano, M. I., and Barrantes, F. J. (2010). Partition profile of the nicotinic acetylcholine receptor in lipid domains upon reconstitution. J. Lipid Res. 51, 2629–2641. doi:10.1194/jlr.M005132
Bhushan, A., and Mcnamee, M. G. (1993). Correlation of phospholipid structure with functional effects on the nicotinic acetylcholine receptor. A modulatory role for phosphatidic acid. Biophys. J. 64, 716–723. doi:10.1016/S0006-3495(93)81431-0
Blanton, M. P., and Cohen, J. B. (1992). Mapping the lipid-exposed regions in the Torpedo californica nicotinic acetylcholine receptor. Biochemistry 31, 3738–3750. doi:10.1021/bi00130a003
Blanton, M. P., and Cohen, J. B. (1994). Identifying the lipid-protein interface of the Torpedo nicotinic acetylcholine receptor: secondary structure implications. Biochemistry 33, 2859–2872. doi:10.1021/bi00176a016
Blanton, M. P., Lala, A. K., and Cohen, J. B. (2001). Identification and characterization of membrane-associated polypeptides in Torpedo nicotinic acetylcholine receptor-rich membranes by hydrophobic photolabeling. Biochim. Biophys. Acta. 1512, 215–224. doi:10.1016/s0005-2736(01)00321-2
Boheim, G., Hanke, W., Barrantes, F. J., Eibl, H., Sakmann, B., Fels, G., et al. (1981). Agonist-activated ionic channels in acetylcholine receptor reconstituted into planar lipid bilayers. Proc. Natl. Acad. Sci. U. S. A. 78, 3586–3590. doi:10.1073/pnas.78.6.3586
Borroni, V., Baier, C. J., Lang, T., Bonini, I., White, M. M., Garbus, I., et al. (2007). Cholesterol depletion activates rapid internalization of submicron-sized acetylcholine receptor domains at the cell membrane. Mol. Membr. Biol. 24, 1–15. doi:10.1080/09687860600903387
Borroni, V., and Barrantes, F. J. (2021). Homomeric and heteromeric α7 nicotinic acetylcholine receptors in health and some central nervous system diseases. Membranes 11, 664. doi:10.3390/membranes11090664
Bouzat, C., and Barrantes, F. J. (1991). Acetylcholine receptor channel properties are modified by benzyl alcohol. NeuroReport 2, 681–684. doi:10.1097/00001756-199111000-00012
Bradley, R. J., Sterz, R., and Peper, K. (1984). The effects of alcohols and diols at the nicotinic acetylcholine receptor of the neuromuscular junction. Brain Res. 295, 101–112. doi:10.1016/0006-8993(84)90820-5
Brannigan, G., Henin, J., Law, R., Eckenhoff, R., and Klein, M. L. (2008). Embedded cholesterol in the nicotinic acetylcholine receptor. Proc. Natl. Acad. Sci. U. S. A. 105, 14418–14423. doi:10.1073/pnas.0803029105
Bruno, M. J., Koeppe, R. E., and Andersen, O. S. (2007). Docosahexaenoic acid alters bilayer elastic properties. Proc. Natl. Acad. Sci. U.S.A 104, 9638–9643. doi:10.1073/pnas.0701015104
Brusés, J. L., Chauvet, N., and Rutishauser, U. (2001). Membrane lipid rafts are necessary for the maintenance of the (alpha)7 nicotinic acetylcholine receptor in somatic spines of ciliary neurons. J. Neurosci. 21, 504–512. doi:10.1523/JNEUROSCI.21-02-00504.2001
Cantor, R. S. (1998). The lateral pressure profile in membranes: a physical mechanism of general anesthesia. Toxicol. Lett. 100-101, 451–458. doi:10.1016/s0378-4274(98)00220-3
Chang, H. W., and Bock, E. (1979). Structural stabilization of isolated acetylcholine receptor: specific interaction with phospholipids. Biochemistry 18, 172–179. doi:10.1021/bi00568a026
Cheng, M. H., Liu, L. T., Saladino, A. C., Xu, Y., and Tang, P. (2007). Molecular dynamics simulations of ternary membrane mixture: phosphatidylcholine, phosphatidic acid, and cholesterol. J. Phys.Chem.B 111, 14186–14192. doi:10.1021/jp075467b
Chen, Z., and Rand, R. P. (1997). The influence of cholesterol on phospholipid membrane curvature and bending elasticity. Biophys. J. 73, 267–276. doi:10.1016/S0006-3495(97)78067-6
Cooper, R. A. (1978). Influence of increased membrane cholesterol on membrane fluidity and cell function in human red blood cells. J. Supramol. Struct. 8, 413–430. doi:10.1002/jss.400080404
Criado, M., Eibl, H., and Barrantes, F. J. (1982a). Effects of lipids on acetylcholine receptor. Essential need of cholesterol for maintenance of agonist-induced state transitions in lipid vesicles. Biochemistry 21, 3622–3629. doi:10.1021/bi00258a015
Criado, M., Eibl, H., and Barrantes, F. J. (1984). Functional properties of the acetylcholine receptor incorporated in model lipid membranes. Differential effects of chain length and head group of phospholipids on receptor affinity states and receptor-mediated ion translocation. J. Biol. Chem. 259, 9188–9198. doi:10.1016/s0021-9258(17)47283-8
Criado, M., Vaz, W. L., Barrantes, F. J., and Jovin, T. M. (1982b). Translational diffusion of acetylcholine receptor (monomeric and dimeric forms) of Torpedo marmorata reconstituted into phospholipid bilayers studied by fluorescence recovery after photobleaching. Biochemistry 21, 5750–5755. doi:10.1021/bi00266a004
Dacosta, C. J., Ogrel, A. A., Mccardy, E. A., Blanton, M. P., and Baenziger, J. E. (2002). Lipid-protein interactions at the nicotinic acetylcholine receptor. A functional coupling between nicotinic receptors and phosphatidic acid-containing lipid bilayers. J. Biol.Chem. 277, 201–208. doi:10.1074/jbc.M108341200
Dalziel, A. W., Rollins, E. S., and Mcnamee, M. G. (1980). The effect of cholesterol on agonist-induced flux in reconstituted acetylcholine receptor vesicles. FEBS Lett. 122, 193–196. doi:10.1016/0014-5793(80)80435-2
Delgado-Vélez, M., Quesada, O., Villalobos-Santos, J. C., Maldonado-Hernández, R., Asmar-Rovira, G., Stevens, R. C., et al. (2021). Pursuing high-resolution structures of nicotinic acetylcholine receptors: lessons learned from five decades. Molecules 26, 5753. doi:10.3390/molecules26195753
de Mendoza, D., and Pilon, M. (2019). Control of membrane lipid homeostasis by lipid-bilayer associated sensors: a mechanism conserved from bacteria to humans. Prog. Lipid Res. 76, 100996. doi:10.1016/j.plipres.2019.100996
Dietrich, C., Yang, B., Fujiwara, T., Kusumi, A., and Jacobson, K. (2002). Relationship of lipid rafts to transient confinement zones detected by single particle tracking. Biophys. J. 82, 274–284. doi:10.1016/S0006-3495(02)75393-9
Dilger, J. P., Vidal, A. M., Mody, H. I., and Liu, Y. (1993). Evidence for direct actions of general anesthetics on an ion channel protein: a new look at a unified mechanism of action. Anesthesiology 81, 431–442. doi:10.1097/00000542-199408000-00022
Drachman, D. B., Angus, C. W., Adams, R. N., Michelson, J. D., and Hoffman, G. J. (1978). Myasthenic antibodies cross-link acetylcholine receptors to accelerate degradation. N. Engl. J. Med. 298, 1116–1122. doi:10.1056/NEJM197805182982004
Ellena, J. F., Blazing, M. A., and Mcnamee, M. G. (1983). Lipid-protein interactions in reconstituted membranes containing acetylcholine receptor. Biochemistry 22, 5523–5535. doi:10.1021/bi00293a012
Epstein, M., and Racker, E. (1978). Reconstitution of carbamylcholine-dependent sodium ion flux and desensitization of the acetylcholine receptor from Torpedo californica. J. Biol. Chem. 253, 6660–6662. doi:10.1016/s0021-9258(17)37967-x
Faber, D. S., and Pereda, A. E. (2018). Two forms of electrical transmission between neurons. Front. Mol. Neurosci. 11, 427. doi:10.3389/fnmol.2018.00427
Fantini, J., di Scala, C., Evans, L. S., Williamson, P. T. F., and Barrantes, F. J. (2016). A mirror code for protein-cholesterol interactions in the two leaflets of biological membranes. Sci. Rep. 6, 21907. doi:10.1038/srep21907
Fantini, J., Epand, R. M., and Barrantes, F. J. (2019). Cholesterol-recognition motifs in membrane proteins. Adv. Exp. Med. Biol. 1135, 3–25. doi:10.1007/978-3-030-14265-0_1
Fernandez-Ballester, G., Castresana, J., Fernandez, A. M., Arrondo, J.-L. R., Ferragut, J. A., and Gonzalez-Ros, J. M. (1994). A role for cholesterol as a structural effector of the nicotinic acetylcholine receptor. Biochemistry 33, 4065–4071. doi:10.1021/bi00179a035
Fertuck, H. C., and Salpeter, M. M. (1976). Quantitation of junctional and extrajunctional acetylcholine receptors by electron microscope autoradiography after 125I-a- bungarotoxin binding at mouse neuromuscular junctions. J. Cell. Biol. 69, 144–158. doi:10.1083/jcb.69.1.144
Flood, P., and Role, L. W. (1998). Neuronal nicotinic acetylcholine receptor modulation by general anesthetics. Toxicol. Lett. 101, 149–153. doi:10.1016/s0378-4274(98)00179-9
Fong, T. M., and Mcnamee, M. G. (1986). Correlation between acetylcholine receptor function and structural properties of membranes. Biochemistry 25, 830–840. doi:10.1021/bi00352a015
Forman, S. A., Chiara, D. C., and Miller, K. W. (2015). Anesthetics target interfacial transmembrane sites in nicotinic acetylcholine receptors. Neuropharmacology 96, 169–177. Part B. doi:10.1016/j.neuropharm.2014.10.002
Franks, N. P., and Lieb, W. R. (1994). Molecular and cellular mechanisms of general anaesthesia. Nature 367, 607–614. doi:10.1038/367607a0
Galla, H. J., and Hartmann, W. (1980). Excimer-forming lipids in membrane research. Chem. Phys. Lipids. 27, 199–219. doi:10.1016/0009-3084(80)90036-5
Gharpure, A., Teng, J., Zhuang, Y., Noviello, C. M., Walsh, R. M., Cabuco, R., et al. (2019). Agonist selectivity and ion permeation in the α3β4 ganglionic nicotinic receptor. Neuron 104, 501–511. doi:10.1016/j.neuron.2019.07.030
Godden, E. L., and Dunwiddie, T. V. (2002). Structural requirements of alkanol interaction sites on human alpha 2 beta 4 neuronal nicotinic acetylcholine receptors expressed in Xenopus oocytes: effects of linear and branched-chain alkanols. Alcohol Clin. Exp. Res. 26, 8–18. doi:10.1097/00000374-200201000-00003
Goldstein, D. B. (1984). The effects of drugs on membrane fluidity. Ann. Rev. Pharmacol. Toxicol. 24, 43–64. doi:10.1146/annurev.pa.24.040184.000355
Golfetto, O., Hinde, E., and Gratton, E. (2013). Laurdan fluorescence lifetime discriminates cholesterol content from changes in fluidity in living cell membranes. Biophys. J. 104, 1238–1247. doi:10.1016/j.bpj.2012.12.057
Gómez-Fernández, J. C., and Goñi, F. M. (2022). The myth of the annular lipids. Biomedicines 10, 2672. doi:10.3390/biomedicines10112672
Goswami, D., Gowrishankar, K., Bilgrami, S., Ghosh, S., Raghupathy, R., Chadda, R., et al. (2008). Nanoclusters of GPI-anchored proteins are formed by cortical actin-driven activity. Cell. 135, 1085–1097. doi:10.1016/j.cell.2008.11.032
Hamouda, A. K., Chiara, D. C., Blanton, M. P., and Cohen, J. B. (2008). Probing the structure of the affinity-purified and lipid-reconstituted torpedo nicotinic acetylcholine receptor. Biochemistry 47, 12787–12794. doi:10.1021/bi801476j
Hamouda, A. K., Chiara, D. C., Sauls, D., Cohen, J. B., and Blanton, M. P. (2006a). Cholesterol interacts with transmembrane alpha-helices M1, M3, and M4 of the Torpedo nicotinic acetylcholine receptor: photolabeling studies using [3H]Azicholesterol. Biochemistry 45, 976–986. doi:10.1021/bi051978h
Hamouda, A. K., Sanghvi, M., Chiara, D. C., Cohen, J. B., and Blanton, M. P. (2007). Identifying the lipid-protein interface of the alpha4beta2 neuronal nicotinic acetylcholine receptor: hydrophobic photolabeling studies with 3-(trifluoromethyl)-3-(m-[125I]iodophenyl)diazirine. Biochemistry 46, 13837–13846. doi:10.1021/bi701705r
Hamouda, A. K., Sanghvi, M., Sauls, D., Machu, T. K., and Blanton, M. P. (2006b). Assessing the lipid requirements of the Torpedo californica nicotinic acetylcholine receptor. Biochemistry 45, 4327–4337. doi:10.1021/bi052281z
Heerklotz, H., and Tsamaloukas, A. (2006). Gradual change or phase transition: characterizing fluid lipid-cholesterol membranes on the basis of thermal volume changes. Biophys. J. 91, 600–607. doi:10.1529/biophysj.106.082669
Hertling-Jaweed, S., Bandini, G., Müller-Fahrnow, A., Dommes, V., and Hucho, F. (1988). Rapid preparation of the nicotinic acetylcholine receptor for crystallization in detergent solution. FEBS Lett. 241, 29–32. doi:10.1016/0014-5793(88)81024-x
Heuser, J. E., and Salpeter, S. R. (1979). Organization of acetylcholine receptors in quick-frozen, deep-etched, and rotary-replicated Torpedo postsynaptic membrane. J. Cell. Biol. 82, 150–173. doi:10.1083/jcb.82.1.150
Holopainen, J. M., Brockman, H. L., Brown, R. E., and Kinnunen, P. K. (2001). Interfacial interactions of ceramide with dimyristoylphosphatidylcholine: impact of the N-acyl chain. Biophys. J. 80, 765–775. doi:10.1016/S0006-3495(01)76056-0
Holopainen, J. M., Lehtonen, J. Y., and Kinnunen, P. K. (1997). Lipid microdomains in dimyristoylphosphatidylcholine-ceramide liposomes. Chem. Phys. Lipids. 88, 1–13. doi:10.1016/s0009-3084(97)00040-6
Holopainen, J. M., Subramanian, M., and Kinnunen, P. K. (1998). Sphingomyelinase induces lipid microdomain formation in a fluid phosphatidylcholine/sphingomyelin membrane. Biochemistry 37, 17562–17570. doi:10.1021/bi980915e
Horvath, L. I., Arias, H. R., Hankovszky, H. O., Hideg, K., Barrantes, F. J., and Marsh, D. (1990). Association of spin-labeled local anesthetics at the hydrophobic surface of acetylcholine receptor in native membranes from Torpedo marmorata. Biochemistry 29, 8707–8713. doi:10.1021/bi00489a029
Jacobson, K., Liu, P., and Lagerholm, B. C. (2019). The lateral organization and mobility of plasma membrane components. Cell. 177, 806–819. doi:10.1016/j.cell.2019.04.018
Jones, M. E., and Lentz, B. R. (1986). Phospholipid lateral organization in synthetic membranes as monitored by pyrene-labeled phospholipids: effects of temperature and prothrombin fragment 1 binding. Biochemistry 25, 567–574. doi:10.1021/bi00351a009
Jones, O. T., Eubanks, J. H., Earnest, J. P., and Mcnamee, M. G. (1988). A minimum number of lipids are required to support the functional properties of the nicotinic acetylcholine receptor. Biochemistry 27, 3733–3742. doi:10.1021/bi00410a032
Jones, O. T., and Mcnamee, M. G. (1988a). Annular and nonannular binding sites for cholesterol associated with the nicotinic acetylcholine receptor. Biochemistry 27, 2364–2374. doi:10.1021/bi00407a018
Jones, O. T., and Mcnamee, M. G. (1988b). Annular and nonannular binding sites for cholesterol associated with the nicotinic acetylcholine receptor. Biochemistry 27, 2364–2374. doi:10.1021/bi00407a018
Kellner, R. R., Baier, C. J., Willig, K. I., Hell, S. W., and Barrantes, F. J. (2007). Nanoscale organization of nicotinic acetylcholine receptors revealed by stimulated emission depletion microscopy. Neuroscience 144, 135–143. doi:10.1016/j.neuroscience.2006.08.071
Koivusalo, M., Alvesalo, J., Virtanen, J. A., and Somerharju, P. (2004). Partitioning of pyrene-labeled phospho- and sphingolipids between ordered and disordered bilayer domains. Biophys. J. 86, 923–935. doi:10.1016/S0006-3495(04)74168-5
Koniuszewski, F., Vogel, F. D., Bampali, K., Fabjan, J., Seidel, T., Scholze, P., et al. (2022). Molecular mingling: multimodal predictions of ligand promiscuity in pentameric ligand-gated ion channels. Front. Mol. Biosci. 9, 860246. doi:10.3389/fmolb.2022.860246
Köster, D. V., Husain, K., Iljazi, E., Bhat, A., Bieling, P., Mullins, R. D., et al. (2016). Actomyosin dynamics drive local membrane component organization in an in vitro active composite layer. Proc. Natl. Acad. Sci. U. S. A. 113, E1645–E1654. doi:10.1073/pnas.1514030113
Köster, D. V., and Mayor, S. (2016). Cortical actin and the plasma membrane: inextricably intertwined. Curr. Opin. Cell. Biol. 38, 81–89. doi:10.1016/j.ceb.2016.02.021
Kumari, S., Borroni, V., Chaudhry, A., Chanda, B., Massol, R., Mayor, S., et al. (2008). Nicotinic acetylcholine receptor is internalized via a Rac-dependent, dynamin-independent endocytic pathway. J. Cell. Biol. 181, 1179–1193. doi:10.1083/jcb.200709086
Laan, V. D. B.-V. D., Chupin, V., Killian, J. A., and de Kruijff, B. (2004). Small alcohols destabilize the KcsA tetramer via their effect on the membrane lateral pressure. Biochemistry 43, 5937–5942. doi:10.1021/bi0496079
Land, B. R., Podleski, T. R., Salpeter, E. E., and Salpeter, M. M. (1977). Acetylcholine receptor distribution on myotubes in culture correlated to acetylcholine sensitivity. J.Physiol (Lond) 269, 155–176. doi:10.1113/jphysiol.1977.sp011897
Le Bon, C., Michon, B., Popot, J.-L., and Zoonens, M. (2021). Amphipathic environments for determining the structure of membrane proteins by single-particle electron cryo-microscopy. Q. Rev. Biophysics 54, e6–e62. doi:10.1017/S0033583521000044
Lehr, H. A., Zimmer, J. P., Hübner, C., Reisinger, E. C., Kohlschütter, A., Claussen, M., et al. (1989). Decreased plasma membrane fluidity of peripheral blood lymphocytes after diethyldithiocarbamate (DTC) therapy in HIV- infected patients. Eur. J. Clin. Pharmacol. 37, 521–523. doi:10.1007/BF00558135
Le Novère, N., and Changeux, J.-P. (1995). Molecular evolution of the nicotinic acetylcholine receptor: an example of multigene family in excitable cells. J. Mol. Evol. 40, 155–172. doi:10.1007/BF00167110
Lichtenberg, D., Goni, F. M., and Heerklotz, H. (2005). Detergent-resistant membranes should not be identified with membrane rafts. Trends biochem. Sci. 30, 430–436. doi:10.1016/j.tibs.2005.06.004
Lin, L., Koblin, D. D., and Wang, H. H. (1991). Saturable binding of anesthetics to nicotinic acetylcholine receptors: a possible mechanism of anesthetic action. Ann. NY Acad. Sci. 625, 628–644. doi:10.1111/j.1749-6632.1991.tb33897.x
Lundbaek, J. A., Maer, A. M., and Andersen, O. S. (1997). Lipid bilayer electrostatic energy, curvature stress, and assembly of gramicidin channels. Biochemistry 36, 5695–5701. doi:10.1021/bi9619841
Mantipragada, S. B., Horvath, L. I., Arias, H. R., Schwarzmann, G., Sandhoff, K., Barrantes, F. J., et al. (2003). Lipid-protein interactions and effect of local anesthetics in acetylcholine receptor-rich membranes from Torpedo marmorata electric organ. Biochemistry 42, 9167–9175. doi:10.1021/bi034485q
Marsh, D. (1996a). Components of the lateral pressure in lipid bilayers deduced from HII phases dimensions. Biochim.Biophys.Acta Bio-Membr. 11279, 119–123. doi:10.1016/0005-2736(95)00296-0
Marsh, D. (1996b). Intrinsic curvature in normal and inverted lipid structures and in membranes. Biophys. J. 70, 2248–2255. doi:10.1016/S0006-3495(96)79790-4
Marsh, D., and Barrantes, F. J. (1978). Immobilized lipid in acetylcholine receptor-rich membranes from Torpedo marmorata. Proc. Natl. Acad. Sci. U.S.A 75, 4329–4333. doi:10.1073/pnas.75.9.4329
Marsh, D., Watts, A., and Barrantes, F. J. (1981). Phospholipid chain immobilization and steroid rotational immobilization in acetylcholine receptor-rich membranes from Torpedo marmorata. Biochim. Biophys. Acta 645, 97–101. doi:10.1016/0005-2736(81)90516-2
Martinez, K. L., Gohon, Y., Corringer, P. J., Tribet, C., Merola, F., Changeux, J.-P., et al. (2002). Allosteric transitions of Torpedo acetylcholine receptor in lipids, detergent and amphipols: molecular interactions vs. physical constraints. FEBS Lett. 528, 251–256. doi:10.1016/s0014-5793(02)03306-9
Mayor, S., and Rao, M. (2004). Rafts: scale-dependent, active lipid organization at the cell surface. Traffic 5, 231–240. doi:10.1111/j.1600-0854.2004.00172.x
Mély-Goubert, B., and Freedman, M. H. (1980). Lipid fluidity and membrane protein monitoring using 1,6- diphenyl-1,3,5-hexatriene. Biochim. Biophys. Acta. 601, 315–327. doi:10.1016/0005-2736(80)90536-2
Mittaud, P., Camilleri, A. A., Willmann, R., Erb-Vogtli, S., Burden, S. J., and Fuhrer, C. (2004). A single pulse of agrin triggers a pathway that acts to cluster acetylcholine receptors. Mol.Cell Biol. 24, 7841–7854. doi:10.1128/MCB.24.18.7841-7854.2004
Morales-Perez, C. L., Noviello, C. M., and Hibbs, R. E. (2016). X-ray structure of the human α4β2 nicotinic receptor. Nat. Adv. online Publ. 538, 411–415. doi:10.1038/nature19785
Moransard, M., Borges, L. S., Willmann, R., Marangi, P. A., Brenner, H. R., Ferns, M. J., et al. (2003). Agrin regulates rapsyn interaction with surface acetylcholine receptors, and this underlies cytoskeletal anchoring and clustering. J. Biol. Chem. 278, 7350–7359. doi:10.1074/jbc.M210865200
Mosqueira, A., Camino, P. A., and Barrantes, F. J. (2018). Cholesterol modulates acetylcholine receptor diffusion by tuning confinement sojourns and nanocluster stability. Sci. Rep. 8, 11974. doi:10.1038/s41598-018-30384-y
Mosqueira, A., Camino, P. A., and Barrantes, F. J. (2020). Antibody-induced crosslinking and cholesterol-sensitive, anomalous diffusion of nicotinic acetylcholine receptors. J. Neurochem. 152, 663–674. doi:10.1111/jnc.14905
Moya-Quiles, M. R., Muñoz-Delgado, E., and Vidal, C. J. (1996). Effects of the pyrethroid insecticide permethrin on membrane fluidity. Chem. Phys. Lipids 79, 21–28. doi:10.1016/0009-3084(95)02503-0
Needham, D., and Nunn, R. S. (1990). Elastic deformation and failure of lipid bilayer membranes containing cholesterol. Biophys. J. 58, 997–1009. doi:10.1016/S0006-3495(90)82444-9
Nicolson, G. L. (2014). The Fluid—mosaic Model of Membrane Structure: still relevant to understanding the structure, function and dynamics of biological membranes after more than 40 years. Biochimica Biophysica Acta (BBA) - Biomembr. 1838, 1451–1466. doi:10.1016/j.bbamem.2013.10.019
Nicolson, G. L., and Ferreira de Mattos, G. (2023). The Fluid–Mosaic model of cell membranes: a brief introduction, historical features, some general principles, and its adaptation to current information. Biochimica Biophysica Acta (BBA) - Biomembr. 1865, 184135. doi:10.1016/j.bbamem.2023.184135
Noviello, C. M., Gharpure, A., Mukhtasimova, N., Cabuco, R., Baxter, L., Borek, D., et al. (2021). Structure and gating mechanism of the α7 nicotinic acetylcholine receptor. Cell. 184, 21–2134. doi:10.1016/j.cell.2021.02.049
Olek, A. J., Krikorian, J. G., and Daniels, M. P. (1986a). Early stages in the formation and stabilization of acetylcholine receptor aggregates on cultured myotubes: sensitivity to temperature and azide. Dev. Biol. 117, 24–34. doi:10.1016/0012-1606(86)90344-1
Olek, A. J., Ling, A., and Daniels, M. P. (1986b). Development of ultrastructural specializations during the formation of acetylcholine receptor aggregates on cultured myotubes. J. Neurosci. 6, 487–497. doi:10.1523/JNEUROSCI.06-02-00487.1986
Olson, E. N., Glaser, L., Merlie, J. P., Sebanne, R., and Lindstrom, J. (1983). Regulation of surface expression of acetylcholine receptors in response to serum and cell growth in the BC3H1 muscle cell line. J. Biol. Chem. 258, 13946–13953. doi:10.1016/s0021-9258(17)44008-7
Ortells, M. O. (1998). “Evolution of the AChR and ligand-gated ion channels,” in The Nicotinic acetylcholine Receptor: current views and future trends. Editor F. J. BARRANTES (Austin, Texas: Landes Bioscience).
Ortells, M. O., Cockcroft, V. B., Lunt, G. G., Marsh, D., and Barrantes, F. J. (1992). “The nicotinic acetylcholine receptor and its lipid microenvironment,” in Membrane proteins: structures, interactions and models. Editors A. PULLMAN, J. JORTNER, and B. PULLMAN (Dordrecht/Boston/London: Kluwer Academic Publishers).
Padilla-Morales, L. F., Morales-Perez, C. L., de La Cruz-Rivera, P. C., Asmar-Rovira, G., Baez-Pagan, C. A., Quesada, O., et al. (2011). Effects of lipid-analog detergent solubilization on the functionality and lipidic cubic phase mobility of the Torpedo californica nicotinic acetylcholine receptor. J. Membr. Biol. 243, 47–58. doi:10.1007/s00232-011-9392-4
Pavel, M. A., Petersen, E. N., Wang, H., Lerner, R. A., and Hansen, S. B. (2020). Studies on the mechanism of general anesthesia. Proc. Natl. Acad. Sci. 117, 13757–13766. doi:10.1073/pnas.2004259117
Perillo, V. L., Penalva, D. A., Vitale, A. J., Barrantes, F. J., and Antollini, S. S. (2016). Transbilayer asymmetry and sphingomyelin composition modulate the preferential membrane partitioning of the nicotinic acetylcholine receptor in Lo domains. Arch. Biochem. Biophys. 591, 76–86. doi:10.1016/j.abb.2015.12.003
Pettersen, E. F., Goddard, T. D., Huang, C. C., Couch, G. S., Greenblatt, D. M., Meng, E. C., et al. (2004). UCSF Chimera–a visualization system for exploratory research and analysis. J. Comput. Chem. 2513, 1605–1612. doi:10.1002/jcc.20084
Pike, L. J. (2006). Rafts defined: a report on the Keystone Symposium on lipid rafts and cell function. J. Lipid Res. 47, 1597–1598. doi:10.1194/jlr.E600002-JLR200
Popot, J. L. (2010). Amphipols, nanodiscs, and fluorinated surfactants: three nonconventional approaches to studying membrane proteins in aqueous solutions. Annu.Rev Biochem. 79, 737–775. doi:10.1146/annurev.biochem.052208.114057
Popot, J. L., Demel, R. A., Sobel, A., van Deenen, L. L., and Changeux, J. P. (1977). Preferential affinity of acetylcholine receptor protein for certain lipids studied using monolayer cultures. C R. Acad. Sci. Hebd. Seances Acad. Sci. D. 285, 1005–1008.
Pottel, H., van der Meer, W., and Herreman, W. (1983). Correlation between the order parameter and the steady-state fluorescence anisotropy of 1,6-diphenyl-1,3,5-hexatriene and an evaluation of membrane fluidity. Biochim. Biophys. Acta. 730, 181–186. doi:10.1016/0005-2736(83)90331-0
Poveda, J. A., Encinar, J. A., Fernandez, A. M., Mateo, C. R., Ferragut, J. A., and Gonzalez-Ros, J. M. (2002). Segregation of phosphatidic Acid-rich domains in reconstituted acetylcholine receptor membranes. Biochemistry 41, 12253–12262. doi:10.1021/bi0200099
Pralle, A., Keller, P., Florin, E. L., Simons, K., and Horber, J. K. (2000). Sphingolipid-cholesterol rafts diffuse as small entities in the plasma membrane of mammalian cells. J. Cell. Biol. 148, 997–1008. doi:10.1083/jcb.148.5.997
Quesada, O., González -Freire, C., Ferrer, M. C., Colón -Sáez, J. O., Fernández-García, E., Mercado, J., et al. (2016). Uncovering the lipidic basis for the preparation of functional nicotinic acetylcholine receptor detergent complexes for structural studies. Sci. Rep. 6, 32766. doi:10.1038/srep32766
Raghupathy, R., Anilkumar, A. A., Polley, A., Singh, P. P., Yadav, M., Johnson, C., et al. (2015). Transbilayer lipid interactions mediate nanoclustering of lipid-anchored proteins. Cell. 161, 581–594. doi:10.1016/j.cell.2015.03.048
Rahman, M. M., Basta, T., Teng, J., Lee, M., Worrell, B. T., Stowell, M. H. B., et al. (2022). Structural mechanism of muscle nicotinic receptor desensitization and block by curare. Nat. Struct. Mol. Biol. 29, 386–394. doi:10.1038/s41594-022-00737-3
Rahman, M. M., Teng, J., Worrell, B. T., Noviello, C. M., Lee, M., Karlin, A., et al. (2020). Structure of the native muscle-type nicotinic receptor and inhibition by snake venom toxins. Neuron 106, 952–962. doi:10.1016/j.neuron.2020.03.012
Raines, D. E., and Mcclure, K. B. (1997). Halothane interactions with nicotinic acetylcholine receptor membranes - steady-state and kinetic studies of intrinsic fluorescence quenching. Anesthesiology 86, 476–486. doi:10.1097/00000542-199702000-00023
Raines, D. E., and Zachariah, V. T. (1999). Isoflurane increases the apparent agonist affinity of the nicotinic acetylcholine receptor. Anesthesiology 90, 135–146. doi:10.1097/00000542-199901000-00019
Raisch, T., and Raunser, S. (2023). The modes of action of ion-channel-targeting neurotoxic insecticides: lessons from structural biology. Nat. Struct. Mol. Biol. 30, 1411–1427. doi:10.1038/s41594-023-01113-5
Rao, M., and Mayor, S. (2005). Use of Forster's resonance energy transfer microscopy to study lipid rafts. Biochim. Biophys. Acta. 1746, 221–233. doi:10.1016/j.bbamcr.2005.08.002
Roccamo, A. M., Pediconi, M. F., Aztiria, E., Zanello, L., Wolstenholme, A., and Barrantes, F. J. (1999). Cells defective in sphingolipids biosynthesis express low amounts of muscle nicotinic acetylcholine receptor. Eur. J. Neurosci. 11, 1615–1623. doi:10.1046/j.1460-9568.1999.00574.x
Salord, F., Keita, H., Lecharny, J. B., Henzel, D., Desmonts, J. M., and Mantz, J. (1997). Halothane and isoflurane differentially affect the regulation of dopamine and gamma-aminobutyric acid release mediated by presynaptic acetylcholine receptors in the rat striatum. Anesthesiology 86, 632–641. doi:10.1097/00000542-199703000-00016
Salpeter, M. M., and Loring, R. H. (1985). Nicotinic acetylcholine receptors in vertebrate muscle: properties, distribution and neural control. Prog. Neurobiol. 25, 297–325. doi:10.1016/0301-0082(85)90018-8
Samsonov, A. V., Mihalyov, I., and Cohen, F. S. (2001). Characterization of cholesterol-sphingomyelin domains and their dynamics in bilayer membranes. Biophys. J. 81, 1486–1500. doi:10.1016/S0006-3495(01)75803-1
Sanes, J. R., and Lichtman, J. W. (2001). Induction, assembly, maturation and maintenance of a postsynaptic apparatus. Nat. Rev. Neurosci. 2, 791–805. doi:10.1038/35097557
Schroeder, F. (1978). Differences in fluidity between bilayer halves of tumour cell plasma membranes. Nature 276, 528–530. doi:10.1038/276528a0
Schürholz, T., Gieselmann, A., and Neumann, E. (1989). Miscibility of octyl glucoside-phosphatidylcholine micellar solutions. Partition of the nicotinic acetylcholine receptor into the surfactant-rich phase. Biochim. Biophys. Acta. 986, 225–233. doi:10.1016/0005-2736(89)90471-9
Sezgin, E., Levental, I., Mayor, S., and Eggeling, C. (2017). The mystery of membrane organization: composition, regulation and roles of lipid rafts. Nat. Rev. Mol. Cell. Biol. 18, 361–374. doi:10.1038/nrm.2017.16
Sharma, P., Varma, R., Sarasij, R. C., Ira, Gousset, K., Krishnamoorthy, G., Rao, M., et al. (2004). Nanoscale organization of multiple GPI-anchored proteins in living cell membranes. Cell. 116, 577–589. doi:10.1016/s0092-8674(04)00167-9
Sharp, L., and Brannigan, G. (2021). Spontaneous lipid binding to the nicotinic acetylcholine receptor in a native membrane. J. Chem. Phys. 154, 185102. doi:10.1063/5.0046333
Shelby, S. A., and Veatch, S. L. (2023). The membrane phase transition gives rise to responsive plasma membrane structure and function. Cold Spring Harb. Perspect. Biol. 15, a041395. doi:10.1101/cshperspect.a041395
Simons, K., and Ikonen, E. (1997). Functional rafts in cell membranes. Nature 387, 569–572. doi:10.1038/42408
Singer, S. J., and Nicolson, G. L. (1972). The fluid mosaic model of the structure of cell membranes. Science 175, 720–731. doi:10.1126/science.175.4023.720
Somerharju, P. J., Virtanen, J. A., Eklund, K. K., Vainio, P., and Kinnunen, P. K. (1985). 1-Palmitoyl-2-pyrenedecanoyl glycerophospholipids as membrane probes: evidence for regular distribution in liquid-crystalline phosphatidylcholine bilayers. Biochemistry 24, 2773–2781. doi:10.1021/bi00332a027
Somerharju, P. (2002). Pyrene-labeled lipids as tools in membrane biophysics and cell biology. Chem. Phys. Lipids. 116, 57–74. doi:10.1016/s0009-3084(02)00020-8
Stetzkowski-Marden, F., Recouvreur, M., Camus, G., Cartaud, A., Marchand, S., and Cartaud, J. (2006). Rafts are required for acetylcholine receptor clustering. J. Mol.Neurosci. 30, 37–38. doi:10.1385/JMN:30:1:37
Strey, H., Peterson, M., and Sackmann, E. (1995). Measurement of erythrocyte membrane elasticity by flicker eigenmode decomposition. Biophys. J. 69, 478–488. doi:10.1016/S0006-3495(95)79921-0
Stroud, R. M., and Finer-Moore, J. (1985). Acetylcholine receptor structure, function, and evolution. Ann. Rev. Cell. Biol. 1, 317–351. doi:10.1146/annurev.cb.01.110185.001533
Sunshine, C., and Mcnamee, M. G. (1992). Lipid modulation of nicotinic acetylcholine receptor function: the role of neutral and negatively charged lipids. Biochim.Biophys.Acta Bio-Membr. 1108, 240–246. doi:10.1016/0005-2736(92)90031-g
Tessier, C. J. G., Emlaw, J. R., Cao, Z. Q., Javier Perez-Areales, F., Salameh, J. J., Prinston, J. E., et al. (2017). Back to the future: rational maps for exploring acetylcholine receptor space and time. Biochim. Biophys. Acta 1865, 1522–1528. doi:10.1016/j.bbapap.2017.08.006
Tzartos, S. J., and Lindstrom, J. M. (1980). Monoclonal antibodies used to probe acetylcholine receptor structure: localization of the main immunogenic region and detection of similarities between subunits. Proc. Natl. Acad. Sci. U. S. A. 77, 755–759. doi:10.1073/pnas.77.2.755
Tzartos, S. J., Rand, D. E., Einarson, B. L., and Lindstrom, J. M. (1981). Mapping of surface structures of electrophorus acetylcholine receptor using monoclonal antibodies. J. Biol. Chem. 256, 8635–8645. doi:10.1016/s0021-9258(19)68891-5
Unwin, N. (2020). Protein-lipid architecture of a cholinergic postsynaptic membrane. IUCrJ 7, 852–859. doi:10.1107/S2052252520009446
Veatch, S. L., Rogers, N., Decker, A., and Shelby, S. A. (2023). The plasma membrane as an adaptable fluid mosaic. Biochimica Biophysica Acta (BBA) - Biomembr. 1865, 184114. doi:10.1016/j.bbamem.2022.184114
Villar, V. A., Cuevas, S., Zheng, X., and Jose, P. A. (2016). Localization and signaling of GPCRs in lipid rafts. Methods Cell. Biol. 132, 3–23. doi:10.1016/bs.mcb.2015.11.008
Violet, J. M., Downie, D. L., Nakisa, R. C., Lieb, W. R., and Franks, N. P. (1997). Differential sensitivities of mammalian neuronal and muscle nicotinic acetylcholine receptors to general anesthetics. Anesthesiology 86, 866–874. doi:10.1097/00000542-199704000-00017
Walsh, R. M., Roh, S. H., Gharpure, A., Morales-Perez, C. L., Teng, J., and Hibbs, R. E. (2018). Structural principles of distinct assemblies of the human α4β2 nicotinic receptor. Nature 557, 261–265. doi:10.1038/s41586-018-0081-7
Wenz, J. J., and Barrantes, F. J. (2005). Nicotinic acetylcholine receptor induces lateral segregation of phosphatidic acid and phosphatidylcholine in reconstituted membranes. Biochemistry 44, 398–410. doi:10.1021/bi048026g
White, B. H., and Cohen, J. B. (1988). Photolabeling of membrane-bound Torpedo nicotinic acetylcholine receptor with the hydrophobic probe 3- trifluoromethyl-3-(m-[125I]iodophenyl)diazirine. Biochemistry 27, 8741–8751. doi:10.1021/bi00424a009
Willmann, R., Pun, S., Stallmach, L., Sadasivam, G., Santos, A. F., Caroni, P., et al. (2006). Cholesterol and lipid microdomains stabilize the postsynapse at the neuromuscular junction. EMBO J. 25, 4050–4060. doi:10.1038/sj.emboj.7601288
Wolff, D., and Bull, R. (1982). Modification on ion transport in lipid bilayer membranes by the insecticides DDT and DDE. Biochim. Biophys. Acta. 688, 138–144. doi:10.1016/0005-2736(82)90588-0
Wu, W. C., and Raftery, M. A. (1981). Functional properties of acetylcholine receptor monomeric and dimeric forms in reconstituted membranes. Biochem. Biophys. Res. Commun. 99, 436–444. doi:10.1016/0006-291x(81)91764-2
Yeagle, P. L. (1990). Frontiers of membrane research: lipid-protein complexes in membranes; membrane fusion. Horizons in Membrane Biotechnology. China: Wiley-Liss, Inc.
Yersin, A., Hirling, H., Kasas, S., Roduit, C., Kulangara, K., Dietler, G., et al. (2007). Elastic properties of the cell surface and trafficking of single AMPA receptors in living hippocampal neurons. Biophys. J. 92, 4482–4489. doi:10.1529/biophysj.106.092742
Yu, D. H., Zhang, L., Eiselé, J. L., Bertrand, D., Changeux, J.-P., and Weight, F. F. (1996). Ethanol inhibition of nicotinic acetylcholine type alpha 7 receptors involves the amino-terminal domain of the receptor. Mol. Pharmacol. 50, 1010–1016.
Zanello, L. P., Aztiria, E., Antollini, S., and Barrantes, F. J. (1996). Nicotinic acetylcholine receptor channels are influenced by the physical state of their membrane environment. Biophys. J. 70, 2155–2164. doi:10.1016/S0006-3495(96)79781-3
Zarkadas, E., Pebay-Peyroula, E., Thompson, M. J., Schoehn, G., Uchański, T., Steyaert, J., et al. (2022). Conformational transitions and ligand-binding to a muscle-type nicotinic acetylcholine receptor. Neuron 110, 1358–1370.e5. doi:10.1016/j.neuron.2022.01.013
Zeman, K., Engelhard, H., and Sackmann, E. (1990). Bending undulations and elasticity of the erythrocyte membrane: effects of cell shape and membrane organization. Eur. Biophys. J. 18, 203–219. doi:10.1007/BF00183373
Zhuang, Y., Noviello, C. M., Hibbs, R. E., Howard, R. J., and Lindahl, E. (2022). Differential interactions of resting, activated, and desensitized states of the α7 nicotinic acetylcholine receptor with lipidic modulators. Proc. Natl. Acad. Sci. 119, e2208081119. doi:10.1073/pnas.2208081119
Zhu, D., Xiong, W. C., and Mei, L. (2006). Lipid rafts serve as a signaling platform for nicotinic acetylcholine receptor clustering. J. Neurosci. 26, 4841–4851. doi:10.1523/JNEUROSCI.2807-05.2006
Keywords: plasma membrane, plasmalemma, membrane lipids, lipid influence on membrane proteins, cholesterol, nicotinic acetylcholine receptor
Citation: Barrantes FJ (2024) Modulation of a rapid neurotransmitter receptor-ion channel by membrane lipids. Front. Cell Dev. Biol. 11:1328875. doi: 10.3389/fcell.2023.1328875
Received: 27 October 2023; Accepted: 26 December 2023;
Published: 11 January 2024.
Edited by:
Florina Zakany, University of Debrecen, HungaryReviewed by:
Wen Xiong, Baylor College of Medicine, United StatesAvia Rosenhouse-Dantsker, University of Illinois Chicago, United States
Copyright © 2024 Barrantes. This is an open-access article distributed under the terms of the Creative Commons Attribution License (CC BY). The use, distribution or reproduction in other forums is permitted, provided the original author(s) and the copyright owner(s) are credited and that the original publication in this journal is cited, in accordance with accepted academic practice. No use, distribution or reproduction is permitted which does not comply with these terms.
*Correspondence: Francisco J. Barrantes, francisco_barrantes@uca.edu.ar