- 1Department of Molecular Genetics, Groningen Biomolecular Sciences and Biotechnology Institute, University of Groningen, Groningen, Netherlands
- 2Department of Bioinformatics and Biotechnology, Government College University, Faisalabad, Pakistan
- 3Department of Microbiology, Tumor and Cell Biology, Karolinska Institutet, Stockholm, Sweden
In this study, we have explored the transcriptomic response of Streptococcus pneumoniae D39 to N-acetylglucosamine (NAG). Transcriptome comparison of S. pneumoniae D39 wild-type grown in chemically defined medium (CDM) in the presence of 0.5% NAG to that grown in the presence of 0.5% glucose revealed elevated expression of many genes/operons, including nagA, nagB, manLMN, and nanP. We have further confirmed the NAG-dependent expression of nagA, nagB, manLMN, and nanP by β-galactosidase assays. nagA, nagB and glmS are putatively regulated by a transcriptional regulator NagR. We predicted the operator site of NagR (dre site) in PnagA, PnagB, and PglmS, which was further confirmed by mutating the predicted dre site in the respective promoters (nagA, nagB, and glmS). Growth comparison of ΔnagA, ΔnagB, and ΔglmS with the D39 wild-type demonstrates that nagA and nagB are essential for S. pneumoniae D39 to grow in the presence of NAG as a sole carbon source. Furthermore, deletion of ccpA shows that CcpA has no effect on the expression of nagA, nagB, and glmS in the presence of NAG in S. pneumoniae.
Introduction
Pneumonia, sepsis, meningitis, otitis media, and conjunctivitis are a few of the diseases caused by the major human pathogen Streptococcus pneumoniae that results in over a million deaths each year worldwide (Ispahani et al., 2004; O'Brien et al., 2009). S. pneumoniae relies on several nutrient sources and virulence factors to colonize in the human nasopharynx (Phillips et al., 1990; Titgemeyer and Hillen, 2002; Carvalho et al., 2011). Regulatory mechanisms of a number of carbon and nitrogen sources important for the lifestyle and virulence of S. pneumoniae have already been studied (Kloosterman et al., 2006b; Carvalho et al., 2011; Kloosterman and Kuipers, 2011; Afzal et al., 2015c,d). S. pneumoniae has been shown to metabolize 32 carbohydrates including the three-carbon molecule glycerol, nine hexoses or hexose derivatives (ascorbate, fructose, galactose, glucosamine, glucose, mannose, N-acetylglucosamine, N-acetyl-mannosamine, and N-acetyl-neuraminic acid), three α-galactosides (melibiose, raffinose, and stachyose), two β-galactosides (lactose, and lactulose), four α-glucosides (maltose, maltotriose, sucrose, and trehalose), seven β-glucosides (amygdalin, arbutin, 1-O-methyl-β-glucoside, cellobiose, gentiobiose, aesculin, and salicin) and six polysaccharides (glycogen, hyaluronate, inulin, maltodextrin, pectin, and pullulan) (Bidossi et al., 2012).
The importance of carbon sources in the life-style of S. pneumoniae can be judged from the fact that over 30% of all the transporters in the genome are presumably involved in sugar uptake (Tettelin et al., 2001; Bidossi et al., 2012), a considerably larger number than that present in the other microorganisms inhabiting the same niche (Paulsen et al., 2000; Tettelin et al., 2001). The glycoproteins lining the epithelial surfaces in the human nasopharynx might be good carbon and energy sources for pneumococcal growth. Notably, S. pneumoniae has the ability to grow on mucin as a sole carbon source (Yesilkaya et al., 2008). Mucins are constituents of the mucus that span the epithelial surfaces (Rose and Voynow, 2006). These structures are largely O-glycosylated glycoproteins and are usually composed of N-acetylglucosamine (NAG), N-acetylgalactosamine, N-acetylneuraminic acid, galactose, fucose, and sulphated sugars connected to the protein core, mostly via an N-acetylgalactosamine moiety (Rose and Voynow, 2006; Terra et al., 2010). S. pneumoniae has several extracellular glycosidases with an extensive variety of substrates specificities and can make use of the other host glycans, such as N-glycans and glycosaminoglycans (Burnaugh et al., 2008; King, 2010; Marion et al., 2012). These enzymes produce a number of free sugars that potentially can be used by the pneumococcus. The deglycosylation activity of both exo- and endoglycosidades has previously been demonstrated in S. pneumoniae (King et al., 2006; Burnaugh et al., 2008; Jeong et al., 2009; Marion et al., 2009). The ability to utilize complex glycans present at the site of colonization contributes to the successful survival and virulence of S. pneumoniae in the host (Buckwalter and King, 2012; Linke et al., 2013). Besides, the role of these enzymes in in vivo fitness is demonstrated by the findings that glycosidase mutants show attenuated capacity to colonize and to cause disease in mouse models (Tong et al., 2000; Jeong et al., 2009; Marion et al., 2009; Terra et al., 2010).
NAG is an important amino-carbon source for several bacteria due to its role as an energy resource and in peptidoglycan synthesis (Dobrogosz, 1968; Mobley et al., 1982). Several studies highlighted the importance of NAG as a preferred carbon source in bacteria (Dobrogosz, 1968; Mobley et al., 1982). The involvement of NAG for both catabolic and anabolic purposes requires proper regulatory mechanisms for its utilization, as shown in model microorganisms, such as Bacillus subtilis, Escherichia coli, Streptococcus mutans, and Streptomyces coelicolor (Plumbridge, 2001; Nothaft et al., 2010; Bertram et al., 2011; Zeng and Burne, 2015). The NAG regulon consists of nagA, nagB, and glmS in S. mutans, and is regulated by a GntR-family transcriptional regulator NagR (Moye et al., 2014). NagA is an NAG-6-phosphate deacetylase, whereas NagB is a GlcN-6-P deaminase, and GlmS is a Fru-6-P amidotransferase. NagB was upregulated in the presence of NAG while GlmS expression decreased, signifying that the regulatory mode of these enzymes depends on the concentration of environmental NAG. A glmS-inactivated mutant could not grow in the absence of NAG, whereas the growth of nagB-inactivated mutant was decreased in the presence of NAG (Kawada-Matsuo et al., 2012). nagB inactivation led to a decrease in the expression of virulence factors, including cell-surface protein antigen and glucosyltransferase, and also impeded biofilm formation and saliva-induced aggregation in S. mutans (Kawada-Matsuo et al., 2012). NagA has been shown to be important for the growth of pneumococcus in the presence of NAG as a sole carbon source (Paixão et al., 2015).
Diverse bacterial groups including streptomycetes, firmicutes, and enterobacteriaceae universally use phosphotransferase systems (PTSs) for uptake and phosphorylation of NAG (Simoni et al., 1976; Mobley et al., 1982; Alice et al., 2003; Nothaft et al., 2003, 2010). ManLMN PTS has been shown to be a glucose and mannose PTS in Streptococcus salivarius (Vadeboncoeur, 1984) and was also responsible for uptake of fructose, and NAG (Lortie et al., 2000). ManLMN transports glucose and mannose, and also shows specificity for galactose, NAG, and glucosamine in S. pneumoniae (Bidossi et al., 2012). NagP (PTS EIIBC component) is the main transporter of NAG in B. subtilis and S. mutans (Reizer et al., 1999; Saier et al., 2002; Moye et al., 2014).
Here, we demonstrate the effect of NAG on the global gene expression of S. pneumoniae and NAG-dependent expression of nagA, nagB, manLMN, and nanP. We further hypothesize that a GntR-family transcriptional regulator, NagR, might be involved in the regulation of nagA, nagB, and glmS and predict a putative operator site for NagR (dre site). We also explored the global impact of ccpA deletion on the transcriptome of S. pneumoniae in the presence of NAG, showing that ccpA has no effect on the expression of nagA, nagB, and glmS. Furthermore, we show that nagA and nagB are essential for S. pneumoniae to grow on NAG validating the previous study, where essentiality of nagA in the growth of S. pneumoniae was demonstrated (Paixão et al., 2015).
Materials and Methods
Bacterial Strains, Growth Conditions, and DNA Isolation and Manipulation
Bacterial strains and plasmids used in this study are listed in Table 1. S. pneumoniae D39 was grown as described previously (Kloosterman et al., 2006a; Afzal et al., 2014). For β-galactosidase assays, derivatives of S. pneumoniae D39 were grown in chemically defined medium (CDM) (Neves et al., 2002) supplemented either with 0.5% glucose or with 0.5% NAG. For selection on antibiotics, medium was supplemented with the following concentrations of antibiotics: 150 μg/ml spectinomycin, 15 μg/ml trimethoprim and 2.5 μg/ml tetracycline for S. pneumoniae; and 100 μg/ml ampicillin for E. coli. All bacterial strains used in this study were stored in 10% (v/v) glycerol at −80°C. For PCR amplification, chromosomal DNA of S. pneumoniae D39 (Lanie et al., 2007) was used. Primers used in this study are based on the sequence of the S. pneumoniae D39 genome and listed in Table 2.
Construction of nagA, nagB, and glmS Mutants
nagA and nagB deletion mutants were made by allelic replacement with trimethoprim- and spectinomycin-resistance cassettes, respectively. Briefly, primers nagA-1/nagA-2 and nagA-3/nagA-4 were used to generate PCR fragments of the left and right flanking regions of nagA. Similarly, primers nagB-1/nagB-2 and nagB3/nagB-4 were used to generate PCR fragments of the left and right flanking regions of nagB. PCR products of left and right flanking regions of nagA and nagB contain AscI and NotI restriction enzyme sites, respectively. The trimethoprim- and spectinomycin-resistance cassettes that are amplified by primers Spec-F/Spec-R and Trmp-F/Trmp-R, respectively from pORI38 and pKOT, also contain AscI and NotI restriction enzyme sites on their ends. Then, by restriction and ligation, the left and right flanking regions of nagA and nagB were fused to the trimethoprim- and spectinomycin-resistance genes, respectively. The resulting ligation products were transformed to S. pneumoniae D39 wild-type and selection of the mutant strains was done on appropriate concentrations of trimethoprim and spectinomycin.
A markerless glmS mutant (ΔglmS) was constructed in the S. pneumoniae D39 wild-type using the pORI280 plasmid, as described before (Kloosterman et al., 2006a). Primer pairs, glmS-1/glmS-2 and glmS-3/glmS-4, were used to generate PCR fragments of the left and right flanking regions of glmS, respectively. These PCR fragments were inserted into pORI280 using XbaI and BglII restriction sites, resulting in pMA700. All mutants were further confirmed by PCR and DNA sequencing.
Growth Analysis
For growth analysis of ΔnagA, ΔnagB, and ΔglmS, S. pneumoniae D39 wild-type and its isogenic mutants (ΔnagA, ΔnagB, and ΔglmS) were grown microaerobically at 37°C in 5 ml tubes containing 3 ml CDM supplemented either with 0.5% NAG or with 0.5% Glucose. Cultures were maintained at 37°C for 11 h and optical density at 600 nm was recorded with 1 h interval. CDM without inoculum was taken as blank. The growth of each strain was monitored from six biological replicates from at least two different days.
Construction of Promoter lacZ-fusions and β-Galactosidase Assays
Chromosomal transcriptional lacZ-fusions to the nagA, nagB, glmS, and manL promoters were constructed in the integration plasmid pPP2 (Halfmann et al., 2007) with primer pairs mentioned in Table 2, resulting in pMA701-04 respectively. Briefly, PCR products of nagA, nagB, glmS, and manL promoters were obtained using primers pairs mentioned in Table 2. These PCR fragments contain EcoRI and BamHI restriction sites at their ends. pPP2 also has EcoRI and BamHI restriction sites in its multiple cloning site (MCS). Then, by restriction and ligation, these PCR fragments were cloned into pPP2. pMA701-04 were further introduced into the S. pneumoniae D39 wild-type resulting in strains MA703-06, respectively. All plasmid constructs were checked by PCR and DNA sequencing. β-galactosidase assays were performed as described before (Israelsen et al., 1995; Kloosterman et al., 2006a) using cells that were grown in CDM with appropriate sugar mentioned in Results section and harvested in the mid-exponential phase of growth.
To study the functionality of dre site, the following promoter lacZ-fusions of nagA, nagB, and glmS with mutated dre sites were made in pPP2 (Halfmann et al., 2007) using the primer pairs mentioned in Table 2: PnagA-M (mutation in the dre site), PnagB-M (mutation in the dre site), PglmS1-M (mutation in the 1st dre site), and PglmS3-M (mutation in the 3rd dre site), resulting in plasmid pMA705-08, respectively. The mutations were incorporated into the primers used to amplify the target promoter regions containing the dre sites. These constructs were introduced into the S. pneumoniae D39 wild-type, resulting in strains MA707-10.
Microarray Analysis
For DNA microarray analysis in the presence of NAG, the transcriptome of S. pneumoniae D39 wild-type, grown in biological replicates in CDM with 0.5% NAG was compared to D39 wild-type grown in CDM with 0.5% glucose. Similarly, for DNA microarray analysis of ΔccpA, the transcriptome of S. pneumoniae D39 wild-type was compared to D39 ΔccpA, grown in biological replicates in CDM with 0.5% NAG. The cells were harvested at their respective mid-exponential growth phases. All other procedures regarding the DNA microarray experiment and data analysis were performed as previously described (Afzal et al., 2015a; Shafeeq et al., 2015). For the identification of differentially expressed genes a Bayesian p < 0.001 and a fold change cut-off ≥2 was applied. Microarray data have been submitted to GEO (Gene Expression Omnibus) database under the accession number GSE89589 and GSE89590.
Results
The Putative NAG Regulon in S. pneumoniae
The NAG regulon consists of nagA, nagB, and glmS in S. mutans and is regulated by a GntR-family transcriptional regulator NagR (Moye et al., 2014). NagA is an NAG-6-phosphate deacetylase, whereas NagB is a GlcN-6-P deaminase, and GlmS is a Fru-6-P amidotransferase. S. pneumoniae D39 also possess the genes that encode proteins putatively involved in the transport and utilization of NAG. These genes are nagA, nagB, manLMN, nanP, and glmS. In S. pneumoniae, it appears that NAG enters the cell through NanP PTS (SPD-1496) and/or ManLMN (SPD-0262-64) and is subsequently phosphorylated (Kanehisa et al., 2014). NanP (PTS) is encoded by the gene that is part of nan operon-I (spd_1488-97) of the nan gene cluster and is proposed to transport amino sugars (Bidossi et al., 2012; Afzal et al., 2015b). nanP codes for EIIBC components of the PTS and therefore, needs EIIA component of another PTS to phosphorylate the incoming NAG. The phosphorylated NAG is deacetylated to glucosamine-6-P by NagA (Kanehisa et al., 2014). NagB converts glucosamine-6-P to fructose-6-P, whereas GlmS converts fructose-6-P to glucosamine-6-P. The role of NAG on the gene expression of S. pneumoniae has not been investigated before. Therefore, we decided to explore the effect of NAG on the whole transcriptome of S. pneumoniae.
NAG-Dependent Gene Expression in S. pneumoniae
To study the transcriptomic response of S. pneumoniae D39 to NAG, microarray comparison of S. pneumoniae D39 wild-type grown in CDM with 0.5% NAG to that grown in CDM with 0.5% glucose was performed. Presence of NAG in the medium resulted in altered expression of a number of genes/operons after applying the criteria of ≥2.0-fold and p < 0.001 (Table S1). Table 3 summarizes the transcriptome changes incurred in S. pneumoniae in the presence of NAG and lists the fold-change in the expression of the putative NAG transport and utilization genes.
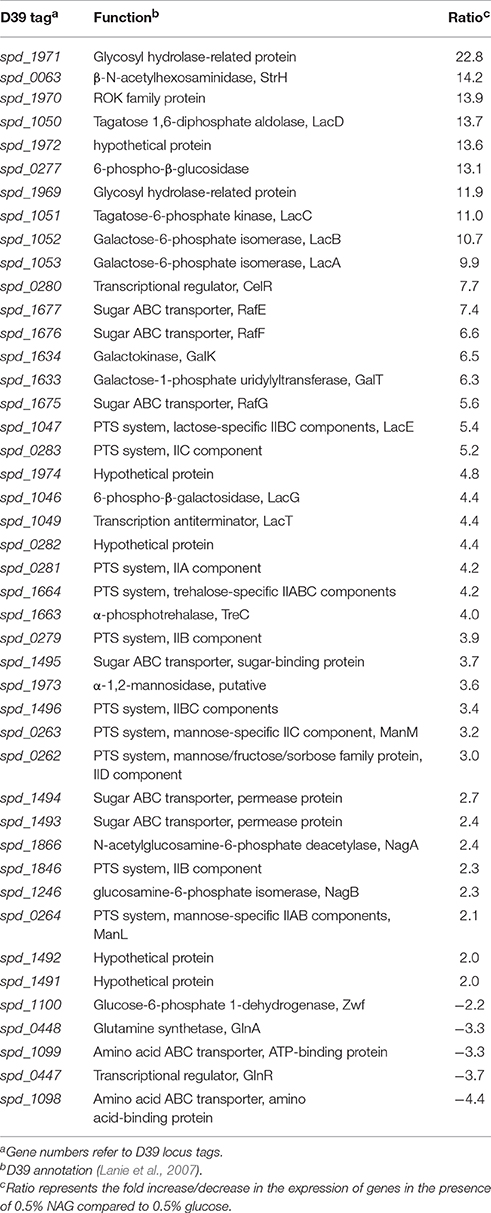
Table 3. Summary of data from Table S1 showing transcriptome comparison of S. pneumoniae D39 wild-type grown in CDM with 0.5% NAG to that grown in CDM with 0.5% glucose.
The glutamine regulon was downregulated around 4-fold in the presence of NAG. This regulon consists of genes involved in glutamine synthesis and uptake (glnA and glnPQ), glutamate synthesis (gdhA), and the gene coding for the pentose phosphate pathway enzyme Zwf, which forms an operon with glnPQ (Kloosterman et al., 2006b). The glutamine regulon is shown to be repressed in the presence of a nitrogen source (Kloosterman et al., 2006b). The presence of nitrogen in NAG might be the reason of down-regulation of the glutamine regulon. A putative operon spd_1969-72 was highly upregulated in the presence of NAG. This operon encodes proteins that are putatively involved in the utilization of carbohydrates. spd_1970 codes for a ROK-family protein (RokD), but it lacks an HTH (helix-turn-helix) domain making it a less probable candidate as a transcriptional regulator of this operon (Shafeeq et al., 2012). ROK-family proteins are a class of transcriptional regulators involved in carbohydrate-dependent transcriptional control (repressor, ORF and kinase). They also contain sugar kinases and many functionally uncharacterized proteins (Titgemeyer et al., 1994). This operon was also upregulated in the presence of cellobiose (Shafeeq et al., 2013) and some other sugars making it a candidate for the utilization of multiple sugars. strH is another gene that was highly upregulated in the presence of NAG. StrH is a β-N-acetylhexosaminidase and is an important virulence factor of S. pneumoniae. StrH is a cell-surface attached β-N-acetylglucosaminidase that is used by S. pneumoniae to process the termini of host complex N-linked glycans (Pluvinage et al., 2013). StrH and SPD-1969 are also possibly being involved in the conversion of chitobiose into NAG based presumably on bioinformatics (Kanehisa et al., 2014). Similarly, spd_1973-74 was also upregulated in our microarray analysis. Both these genes are annotated to be involved in carbohydrate metabolism, where SPD-1973 is a putative α-1,2-mannosidase and SPD-1974 is a hypothetical protein.
cel gene cluster (spd-0277-0283) putatively involved in the utilization of cellobiose is upregulated in the presence of NAG. This gene cluster is shown to be activated by transcriptional regulator CelR in the presence of cellobiose (Shafeeq et al., 2011). Tagatose pathways (lac gene cluster) and Leloir pathway genes (galK and galT) involved in the utilization of lactose and galactose (Afzal et al., 2014, 2015e) are significantly upregulated in the presence of NAG. lac gene cluster comprises of two operons in S. pneumoniae, i.e., lac operon-I (lacABCD) and lac operon-II (lacFEG) (Afzal et al., 2014). LacR, a DeoR-type regulator acts as a transcriptional repressor of lac operon-I in the absence of lactose/galactose (Afzal et al., 2014). Whereas, BglG-family transcriptional antiterminator LacT acts as a transcriptional activator of the lac operon-II in the presence of lactose (Afzal et al., 2014). Putative Raffinose uptake genes rafEFG (spd-1675-77) are highly upregulated in the presence of NAG. Glucose and sucrose are shown to inhibit raffinose uptake (Tyx et al., 2011). A putative trehalose system (spd-1663-64) is highly expressed under our tested conditions. Upregulation of these different sugar systems under our tested conditions might be due to absence of CCR in the presence of NAG as a sole carbon source and further experiments are required to explore the role of these genes in the utilization of NAG.
Expression of genes that are putatively part of NAG utilization and transport pathway was also altered in our transcriptome analysis (Table 3). Expression of manLMN is upregulated around 3-fold in the presence of NAG. We observed upregulation of the nan operon-I, which is involved in the transport and utilization of sialic acid (an amino carbon source) (Marion et al., 2011). Moreover, expression of nagA and nagB was upregulated more than two folds in the presence of NAG (Table 3). No change in the expression of glmS is observed in our transcriptome in the presence of NAG. Upregulation of nanP, manLMN, nagA, and nagB in our transcriptome supports that these genes are important in NAG utilization in S. pneumoniae and strengthens the notion that NanP and ManLMN might be very important for NAG transport. Therefore, we decided to further explore the regulation of these genes in the presence of NAG.
NAG Induces the Expression of the Genes Involved in the Putative Transport and Utilization of Amino Sugars
In order to investigate in more detail the transcriptional regulation of nanP, manLMN, nagA, and nagB in the presence of NAG and to confirm our microarray results, we made ectopic transcriptional lacZ-fusions of nanE, manL, nagA, and nagB promoters (PnagA-lacZ, PnagB-lacZ, PnanE-lacZ, and PmanL-lacZ) and performed β-galactosidase assays (Figure 1). Our β-galactosidase assays data revealed that the expression of PnagA-lacZ, PnagB-lacZ, PnanE-lacZ, and PmanL-lacZ was strikingly higher in the presence of NAG compared to glucose in CDM (Figure 1). These data further confirm our microarray data mentioned above. We did not observe any change in the expression of glmS in our microarray analysis in the presence of NAG. To further study the expression of glmS in the presence of NAG and confirm our microarray analysis, we constructed ectopic transcriptional lacZ-fusion of glmS promoter (PglmS-lacZ) and performed β-galactosidase assays. We could not see any significant change in the expression of PglmS-lacZ under our tested conditions.
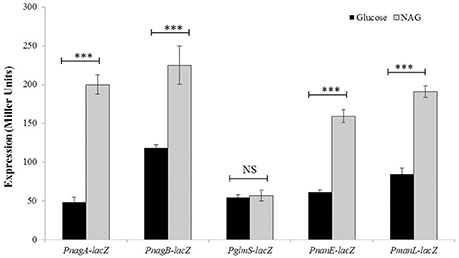
Figure 1. Expression levels (in Miller units) of PnagA-lacZ, PnagB-lacZ, PglmS-lacZ, PnanE-lacZ, and PmanL-lacZ in S. pneumoniae D39 wild-type grown in CDM either with 0.5% glucose or with 0.5% NAG. Standard deviations of three independent experiments are indicated in bars. Statistical significance of the differences in the expression levels was determined by one-way ANOVA (NS, not significant and ***P < 0.0001).
Predication and Confirmation of the dre Sites in the Promoter Regions of glmS, nagA, and nagB
Recently, NagR was characterized as a transcriptional repressor and shown to bind with specific DNA sequences named as dre sites, present in the promoter regions of the nagAB and glmS genes in S. mutans (Zeng and Burne, 2015). Blast search in S. pneumoniae D39 for NagR revealed the presence of a putative GntR-family transcriptional regulator NagR (SPD-1275) in S. pneumoniae D39. Presence of a NagR ortholog in S. pneumoniae might suggest its role in the regulation of the glmS, nagA, and nagB. We decided to delete nagR in S. pneumoniae D39 and study its role. However, we could not delete nagR in S. pneumoniae D39, suggesting the essentiality of NagR in the lifestyle of S. pneumoniae.
To explore the NagR regulated genes in S. pneumoniae D39, we decided to explore the genome of S. pneumoniae D39 for dre sites by using the Genome2D tool (Baerends et al., 2004) and a MEME motif sampler search (Bailey and Elkan, 1994). A 20-bp consensus sequence was found upstream of nagA (5′-AAATAGGTCTATACCATTTA-3′) and nagB (5′- AAATTGGTCTATACCATATA-3′) in S. pneumoniae D39 (Figure S1). We also found three dre sites in the promoter region of glmS (5′-AATTTGAACTATACCAATTT-3′, 5′-AAACAAGTATATACTGTTTT-3′ and 5′-GAATTAGACTATACCAATTT-3′). These DNA stretches may serve as dre sites in S. pneumoniae. We further checked the conservation of this dre site in other streptococcal species (Streptococcus mitis, Streptococcus agalactiae, Streptococcus dysgalactiae, Streptococcus equi, S. mutans, Streptococcus pyogenes, Streptococcus sanguinis, Streptococcus gallolyticus, Streptococcus suis, and Streptococcus uberis) and constructed weight matrix of the putative dre sites presents in different streptococci (Figure S2). We found that the dre sequence is highly conserved in the promoter regions of nagA, nagB and glmS in these streptococci as well (Figure S1).
To determine if the located stretch of DNA mediates the NagR-dependent transcriptional control of the glmS, nagA, and nagB, we made a number of transcriptional lacZ-fusions, where conserved bases in the putative dre sites were mutated in PnagA (5′-AAATAGGTCTATACCATTTA-3′ to 5′-AAATAGGTCGCTGTCATTTA-3′), PnagB (5′-AAATTGGTCTATACCATATA-3′ to 5′-AAATTGGTCGCTGTCATATA-3′) and PglmS (first site: 5′-AATTTGAACTATACCAATTT-3′ to 5′-AATTTGAACGCTGTCAATTT-3′ and third site: 5′-GAATTAGACTATACCAATTT-3′ to 5′-GAATTAGACGCGCCCAATTT-3′). We could not mutate the second dre site in PglmS as it overlaps with core promoter sequence. The expression of PnagA-M-lacZ and PnagB-M-lacZ (few conserved bases of the dre sites were mutated) compared to that of the promoters with the intact dre sites (PnagA-lacZ and PnagB-lacZ) was considerably higher in the presence of glucose and NAG (Figures 2A,B). A derepression of the expression of PglmS was observed when either of the putative dre sites in PglmS (dre site 1 and 3) was mutated. These results suggest that dre sites present in PglmS, PnagA and PnagB are active and may act as an operator site for NagR in S. pneumoniae.
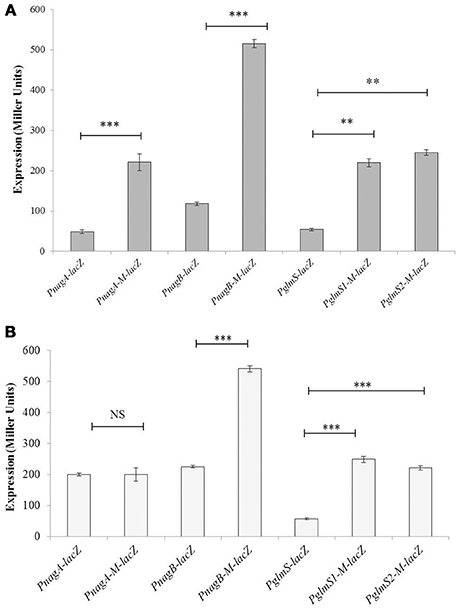
Figure 2. Verification of dre sites in PnagA, PnagB, and PglmS. Expression levels (in Miller units) of PnagA-lacZ, PnagB-lacZ, PglmS-lacZ, PnagA-M-lacZ, PnagB-M-lacZ, PglmS1-M-lacZ, and PglmS3-M-lacZ in S. pneumoniae D39 wild-type grown in CDM with 0.5% glucose (A) and 0.5% NAG (B). PnagA-M-lacZ and PnagB-M-lacZ represent promoter lacZ-fusions of nagA and nagB with mutated dre sites, whereas PglmS1-M-lacZ and PglmS3-M-lacZ represents promoter-lacZ-fusions with mutated dre site 1 and 3, respectively in PglmS. Standard deviations of three independent experiments are indicated in bars. Statistical significance of the differences in the expression levels was determined by one-way ANOVA (NS, not significant, **P < 0.001, and ***P < 0.0001).
nagA and nagB Are Essential for S. pneumoniae D39 to Grow in the Presence of NAG as a Sole Carbon Source
nagA, nagB, and glmS encode important enzymes for the metabolism of NAG in bacteria. To elucidate the significance of these genes on the growth of S. pneumoniae, we made knockout mutants of these genes (ΔnagA, ΔnagB, and ΔglmS), and explored the impact of mutation of these genes on the growth of S. pneumoniae D39 in the presence of 0.5% NAG or glucose in CDM. The genetic organization and PCR confirmation of nagA, nagB, and glmS mutants are given in the Figure 3 and Figure S3, respectively. All three mutants (ΔnagA, ΔnagB, and ΔglmS) had approximately the same growth as D39 wild-type in the presence of glucose in the medium (Figure 4). ΔglmS also showed the same growth pattern as the D39 wild-type in the presence of NAG. However, in contrast to D39 wild-type, ΔnagA and ΔnagB were not able to grow in the presence of NAG (Figure 4). These results suggest that nagA and nagB are necessary for S. pneumoniae to grow in the presence of NAG. These results are also in accordance with recently published data, where they showed that a mutant of nagA did not grow in the presence of NAG as a sole carbon source (a phenotype that could be complemented) (Paixão et al., 2015).
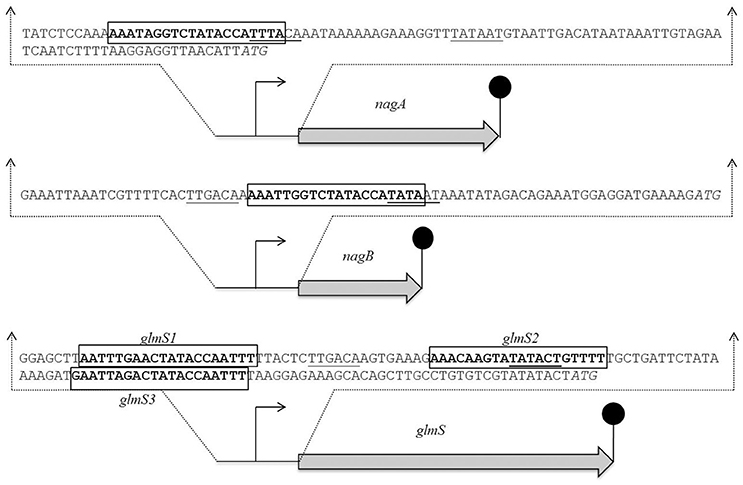
Figure 3. Identification of the dre site in the promoter regions of nagA, nagB, and glmS in S. pneumoniae D39 wild-type. Translational start sites are italicized and putative dre sites are bold and rectangle. Core promoter sequences are underlined.
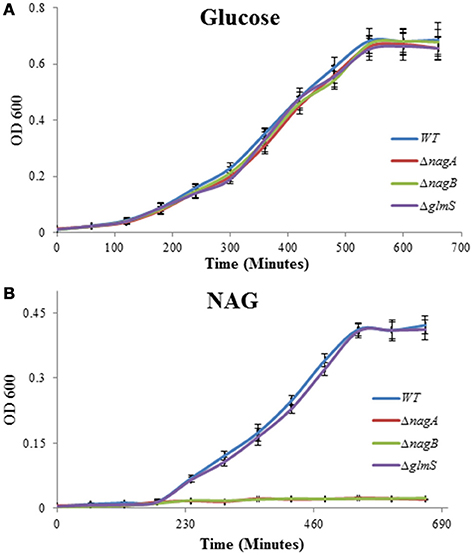
Figure 4. Growth of S. pneumoniae D39 wild-type and its isogenic mutants ΔnagA, ΔnagB, and ΔglmS grown in CDM with 0.5% glucose (A) and 0.5% NAG (B).
Role of CcpA in Regulation of nagA, nagB, nanP, glmS, and manL
CcpA is the master transcriptional regulator in S. pneumoniae that represses the expression of genes involved in the utilization of non-preferred sugars in the presence of a preferred one. To explore the effect of ccpA deletion on the transcriptome of S. pneumoniae and in NAG-dependent regulation of NAG utilization and transport genes, we performed transcriptome comparison of D39 ΔccpA to D39 wild-type in CDM with 0.5% NAG. Expression of a number of genes was altered significantly (Table S1). These genes have been categorized according to their protein function in COG categories (Table 4). We did not observe any significant change in the expression of nagA, nagB, or glmS, suggesting CcpA independent expression of these genes. However, expression of manLMN and nan operon-I was upregulated in ΔccpA, which might suggest a putative role of CcpA in regulation of the manLMN and nan operon-I. nan operon-I was already shown to be regulated by CcpA and to have a cre box (Afzal et al., 2015b). Therefore, upregulation of nan operon-I in the absence of ccpA strengthens the previous observation (Afzal et al., 2015b).
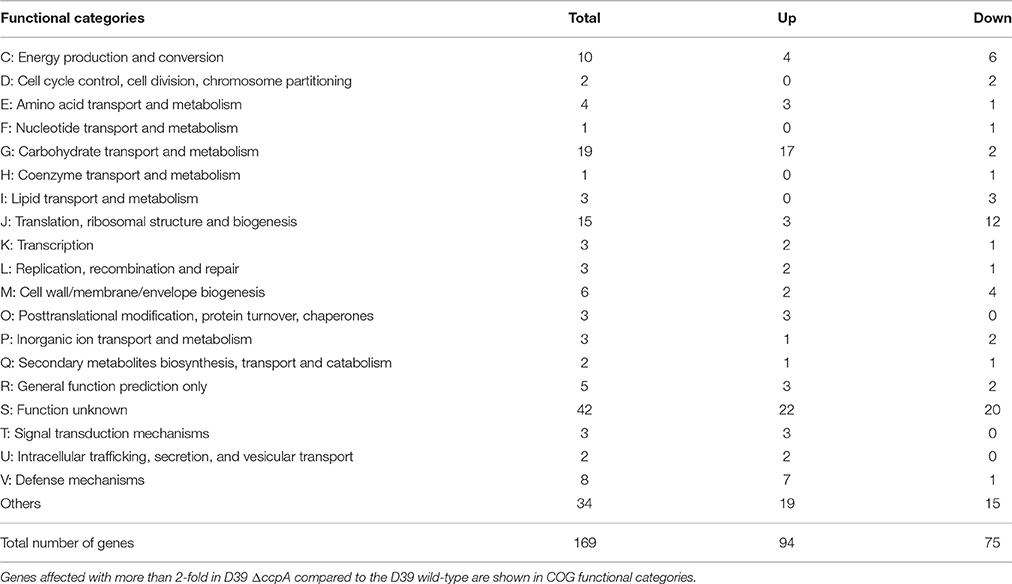
Table 4. Number of genes significantly affected in S. pneumoniae D39 ΔccpA compared to the D39 wild-type grown in CDM with 0.5% NAG.
To further confirm the role of CcpA in the regulation of nagA, nagB, glmS, and manL, we analyzed the promoter regions of these genes for the presence of cre boxes. We could not find a cre box in the promoter regions of nagA, nagB, and glmS, which might confirm the CcpA-independent regulation of the nagA, nagB, and glmS by transcriptional regulator NagR. However, we found a putative cre box (5′-ATGAAAACGGTTTATA-3′) in the promoter regions of manL, further confirming the role of CcpA in the regulation of manLMN.
Discussion and Conclusions
The existence of well-developed sugar transport mechanisms in the opportunistic respiratory human pathogen, S. pneumoniae, emphasizes the importance of carbohydrates in the lifestyle of pneumococcus and confers an extra advantage to survive in a changing nutritional environment (Tettelin et al., 2001). Glucose is the most preferred carbon source for S. pneumoniae but the presence of several other sugar-specific systems in S. pneumoniae indicates its ability to use other available sugars (Hoskins et al., 2001; Lanie et al., 2007; Bidossi et al., 2012). Extensive studies have been performed regarding regulatory mechanisms of different dedicated systems for sugars, including maltose, raffinose, cellobiose, sialic acid, and others in S. pneumoniae (Tyx et al., 2011; Shafeeq et al., 2013; Afzal et al., 2015b,f). Lack of free carbohydrates in the human airway makes modification and import of complex glycans much more critical for pneumococci to obtain the necessary carbon (Buckwalter and King, 2012). At least nine surface-associated glycosidases have been shown to modify host glycans in pneumococci, which makes bacterial survival better in the host (King et al., 2006; Burnaugh et al., 2008; Dalia et al., 2010). Data suggests that NAG may be an important carbohydrate for pneumococci (Bidossi et al., 2012). The regulatory mechanisms of genes putatively involved in NAG utilization have not been explored in S. pneumoniae. The current study sheds light on the regulatory mechanism of the nagA, nagB, and glmS in S. pneumoniae.
nagA, nagB, and glmS are annotated as a part of the amino sugar metabolism pathways in S. pneumoniae (Kanehisa et al., 2014). In our transcriptome comparison of S. pneumoniae D39 grown in CDM with 0.5% NAG to that grown in CDM with 0.5% glucose revealed increased expression of nagA, nagB, manLMN, and nanP. In S. mutans, expression of glmS is repressed in the presence of NAG compared to glucose (Zeng and Burne, 2015). This repression of glmS in the presence of NAG was relieved in nagR mutant (Zeng and Burne, 2015). However, no change in the expression of glmS is observed in our NAG-dependent transcriptome and no effect of ccpA deletion on the expression of glmS is observed. Mutating dre site 1 or 3 in the PglmS led to increase in expression of PglmS in the presence of glucose and NAG. This might indicate that NagR represses the expression of glmS in the presence of glucose and NAG.
The transport of amino-sugars has been attributed to a PTS (NanP) and manLMN in S. mutans (Moye et al., 2014). Similarly, a NAG-specific PTS (NagE) and a mannose-specific PTS ManXYZ have been shown to be involved in the NAG transport in E. coli (White, 1970; Alvarez-Añorve et al., 2009). manLMN has also been proposed to be involved in NAG transport in S. pneumoniae (Bidossi et al., 2012). Similarly, a PTS present in nan operon-I (putatively called nanP) has been suggested to play a part in the transport of glucosamine in S. pneumoniae (Kanehisa et al., 2014). manLMN and nanP are upregulated in our NAG-dependent transcriptome analysis, which is further confirmed by β-galactosidase assays. These observations confirm the findings of the previous studies and strengthen the involvement of nanP and manLMN in the transport of NAG.
NagA, NagB, and GlmS are very important for the metabolism of NAG and these three factors are associated with the synthesis of GlcN-6-P, a precursor for cell wall peptidoglycan synthesis in E. coli (Plumbridge et al., 1993; Plumbridge and Vimr, 1999). Here, we have studied the impact of nagA, nagB, and glmS deletions on the growth of S. pneumoniae in the presence of NAG. Our studies suggest that nagA and nagB are important for pneumococcus to grow on NAG as their deletion mutants failed to grow in the presence of NAG in the medium as a sole carbon source. NagA has also been shown to be essential for growth in the presence of NAG as a sole carbon source (Paixão et al., 2015). However, no impact of glmS deletion on the growth of S. pneumoniae was observed. In B. subtilis, nagB has been shown to be essential for growth in the presence of NAG (Gaugué et al., 2013). NagB and GlmS have been shown to be involved in virulence in S. mutans (Kawada-Matsuo et al., 2012). Inactivation of nagB led to a decrease in the expression of virulence factors, including cell-surface protein antigen and glucosyltransferase, and also impeded biofilm formation and saliva-induced aggregation in S. mutans (Kawada-Matsuo et al., 2012). Pneumococcal nagA mutant was tested in mouse model of colonization and of model of bronchopneumonia with bacteremia, and no difference in virulence was observed (Paixão et al., 2015). It might be still interesting to further explore the role of the nagB and glmS in virulence of S. pneumoniae.
In E. coli, a ROK-family protein (NagC) acts as a transcriptional repressor of the NAG regulon (nagE and nagBACD), which encodes genes that are involved in the uptake and metabolism of NAG. Furthermore, it has been shown that NAG binds to NagC to relieve the repression caused by NagC (Plumbridge, 1991; Titgemeyer et al., 1994). Similarly, a GntR family transcriptional regulator NagR has been shown to act as a transcriptional regulator of the genes involved in NAG utilization in B. subtilis, S. mutans and in some other bacteria (Bertram et al., 2011; Moye et al., 2014). In S. mutans, NagR has been shown to regulate the expression of glmS and nagAB by binding to the NagR operator sites called dre sites (Zeng and Burne, 2015). Our study suggests that NagR is present in S. pneumoniae and might regulate the expression of the nagA, nagB, and glmS by binding to the dre sites present in the promoter regions of these genes. We could not delete nagR, which might indicate about its essentiality or its involvement in some important cell process directly or indirectly. However, we mutated the conserved bases in the dre sites present in the promoter regions of nagA, nagB, and glmS which might suggest the importance of these bases in the regulation of these genes. To explore more putative dre sites in the D39 genome, we conducted a genome-wide search for putative pneumococcal dre sites. A dre site was only found in the promoter regions of nagA and nagB, and three dre sites were found in the promoter region of glmS. This predicted dre site was also found to be highly conserved in other streptococcal species as well (Novichkov et al., 2010), suggesting a similar function of NagR in these organisms.
The master transcriptional regulator, CcpA (Carbon catabolite protein A), was shown to be involved in the repression of non-preferred sugar metabolism genes in the presence of a preferred carbon source, and has a role in pneumococcal pathogenesis (Lulko et al., 2007; Zomer et al., 2007; Carvalho et al., 2011). A number of non-preferred sugar systems have also been shown to be regulated independently of CcpA by other transcriptional regulators, like the cel gene cluster activated by CelR in S. pneumoniae (Shafeeq et al., 2011). In this study, we elucidated the role of CcpA in the regulation of nagA, nagB, glmS, manLMN, and the nan operon-I by elaborating the impact of a ccpA deletion on the whole transcriptome of S. pneumoniae in the presence of NAG as a sole carbon source in CDM. Our transcriptome data demonstrated the CcpA-independent expression of nagA, nagB, and glmS, and CcpA-dependent expression of manLMN and the nan operon-I. We further analyzed the promoter regions of these genes for the presence of a cre box and found cre boxes only in the promoter regions of manLMN and the nan operon-I. The absence of cre boxes in the promoter regions of nagA, nagB, and glmS confirms that CcpA may not have a role in the regulation of nagA, nagB, and glmS. However, the presence of a cre box in the promoter regions of manLMN and the nan operon-I further supports the role of CcpA in the regulation of manLMN and the nan operon-I.
Author Contributions
Substantial contributions to the conception or design of the work; or the acquisition, analysis, or interpretation of data for the work: MA, SS, IM, BHN, and OPK. Drafting the work or revising it critically for important intellectual content: MA, SS, IM, BHN, and OPK. Final approval of the version to be published: MA, SS, IM, BHN, and OPK. Agreement to be accountable for all aspects of the work in ensuring that questions related to the accuracy or integrity of any part of the work are appropriately investigated and resolved: MA, SS, IM, BHN, and OPK.
Conflict of Interest Statement
The authors declare that the research was conducted in the absence of any commercial or financial relationships that could be construed as a potential conflict of interest.
Acknowledgments
MA and IM are supported by Government College University, Faisalabad, Pakistan under faculty development program of HEC Pakistan.
Supplementary Material
The Supplementary Material for this article can be found online at: http://journal.frontiersin.org/article/10.3389/fcimb.2016.00158/full#supplementary-material
References
Afzal, M., Manzoor, I., and Kuipers, O. P. (2015a). A fast and reliable pipeline for bacterial transcriptome analysis case study: serine-dependent gene regulation in Streptococcus pneumoniae. J. Vis. Exp. JoVE. e52649. doi: 10.3791/52649
Afzal, M., Shafeeq, S., Ahmed, H., and Kuipers, O. P. (2015b). Sialic acid-mediated gene expression in Streptococcus pneumoniae and role of NanR as a transcriptional activator of the nan gene cluster. Appl. Environ. Microbiol. 81, 3121–3131. doi: 10.1128/AEM.00499-15
Afzal, M., Shafeeq, S., Henriques-Normark, B., and Kuipers, O. P. (2015c). UlaR activates expression of the ula operon in Streptococcus pneumoniae in the presence of ascorbic acid. Microbiol. Read. Engl. 161, 41–49. doi: 10.1099/mic.0.083899-0
Afzal, M., Shafeeq, S., and Kuipers, O. P. (2014). LacR is a repressor of lacABCD and LacT is an activator of lacTFEG, constituting the lac-gene cluster in Streptococcus pneumoniae. Appl. Environ. Microbiol. 80, 5349–5358. doi: 10.1128/AEM.01370-14
Afzal, M., Shafeeq, S., and Kuipers, O. P. (2015d). Ascorbic acid-dependent gene expression in Streptococcus pneumoniae and the activator function of the transcriptional regulator UlaR2. Front. Microbiol. 6:72. doi: 10.3389/fmicb.2015.00072
Afzal, M., Shafeeq, S., Manzoor, I., and Kuipers, O. P. (2015e). GalR acts as a transcriptional activator of galKT in the presence of galactose in Streptococcus pneumoniae. J. Mol. Microbiol. Biotechnol. 25, 363–371. doi: 10.1159/000439429
Afzal, M., Shafeeq, S., Manzoor, I., and Kuipers, O. P. (2015f). Maltose-dependent transcriptional regulation of the mal regulon by MalR in Streptococcus pneumoniae. PLoS ONE 10:e0127579. doi: 10.1371/journal.pone.0127579
Alice, A. F., Pérez-Martínez, G., and Sánchez-Rivas, C. (2003). Phosphoenolpyruvate phosphotransferase system and N-acetylglucosamine metabolism in Bacillus sphaericus. Microbiol. Read. Engl. 149, 1687–1698. doi: 10.1099/mic.0.26231-0
Alvarez-Añorve, L. I., Bustos-Jaimes, I., Calcagno, M. L., and Plumbridge, J. (2009). Allosteric regulation of glucosamine-6-phosphate deaminase (NagB) and growth of Escherichia coli on glucosamine. J. Bacteriol. 191, 6401–6407. doi: 10.1128/JB.00633-09
Baerends, R. J. S., Smits, W. K., de Jong, A., Hamoen, L. W., Kok, J., and Kuipers, O. P. (2004). Genome2D: a visualization tool for the rapid analysis of bacterial transcriptome data. Genome Biol. 5:R37. doi: 10.1186/gb-2004-5-5-r37
Bailey, T. L., and Elkan, C. (1994). Fitting a mixture model by expectation maximization to discover motifs in biopolymers. Proc. Int. Conf. Intell. Syst. Mol. Biol. ISMB Int. Conf. Intell. Syst. Mol. Biol. 2, 28–36.
Bertram, R., Rigali, S., Wood, N., Lulko, A. T., Kuipers, O. P., and Titgemeyer, F. (2011). Regulon of the N-acetylglucosamine utilization regulator NagR in Bacillus subtilis. J. Bacteriol. 193, 3525–3536. doi: 10.1128/JB.00264-11
Bidossi, A., Mulas, L., Decorosi, F., Colomba, L., Ricci, S., Pozzi, G., et al. (2012). A functional genomics approach to establish the complement of carbohydrate transporters in Streptococcus pneumoniae. PLoS ONE 7:e33320. doi: 10.1371/journal.pone.0033320
Buckwalter, C. M., and King, S. J. (2012). Pneumococcal carbohydrate transport: food for thought. Trends Microbiol. 20, 517–522. doi: 10.1016/j.tim.2012.08.008
Burnaugh, A. M., Frantz, L. J., and King, S. J. (2008). Growth of Streptococcus pneumoniae on human glycoconjugates is dependent upon the sequential activity of bacterial exoglycosidases. J. Bacteriol. 190, 221–230. doi: 10.1128/JB.01251-07
Carvalho, S. M., Kloosterman, T. G., Kuipers, O. P., and Neves, A. R. (2011). CcpA ensures optimal metabolic fitness of Streptococcus pneumoniae. PLoS ONE 6:e26707. doi: 10.1371/journal.pone.0026707
Dalia, A. B., Standish, A. J., and Weiser, J. N. (2010). Three surface exoglycosidases from Streptococcus pneumoniae, NanA, BgaA, and StrH, promote resistance to opsonophagocytic killing by human neutrophils. Infect. Immun. 78, 2108–2116. doi: 10.1128/IAI.01125-09
Dobrogosz, W. J. (1968). Effect of amino sugars on catabolite repression in Escherichia coli. J. Bacteriol. 95, 578–584.
Gaugué, I., Oberto, J., Putzer, H., and Plumbridge, J. (2013). The use of amino sugars by Bacillus subtilis: presence of a unique operon for the catabolism of glucosamine. PLoS ONE 8:e63025. doi: 10.1371/journal.pone.0063025
Halfmann, A., Hakenbeck, R., and Bruckner, R. (2007). A new integrative reporter plasmid for Streptococcus pneumoniae. FEMS Microbiol. Lett. 268, 217–224. doi: 10.1111/j.1574-6968.2006.00584.x
Hoskins, J., Alborn, W. E. Jr., Arnold, J., Blaszczak, L. C., Burgett, S., DeHoff, B. S., et al. (2001). Genome of the bacterium Streptococcus pneumoniae strain R6. J. Bacteriol. 183, 5709–5717. doi: 10.1128/JB.183.19.5709-5717.2001
Ispahani, P., Slack, R. C. B., Donald, F. E., Weston, V. C., and Rutter, N. (2004). Twenty year surveillance of invasive pneumococcal disease in Nottingham: serogroups responsible and implications for immunisation. Arch. Dis. Child. 89, 757–762. doi: 10.1136/adc.2003.036921
Israelsen, H., Madsen, S. M., Vrang, A., Hansen, E. B., and Johansen, E. (1995). Cloning and partial characterization of regulated promoters from Lactococcus lactis Tn917-lacZ integrants with the new promoter probe vector, pAK80. Appl. Environ. Microbiol. 61, 2540–2547.
Jeong, J. K., Kwon, O., Lee, Y. M., Oh, D. B., Lee, J. M., Kim, S., et al. (2009). Characterization of the Streptococcus pneumoniae BgaC protein as a novel surface beta-galactosidase with specific hydrolysis activity for the Galbeta1-3GlcNAc moiety of oligosaccharides. J. Bacteriol. 191, 3011–3023. doi: 10.1128/JB.01601-08
Kanehisa, M., Goto, S., Sato, Y., Kawashima, M., Furumichi, M., and Tanabe, M. (2014). Data, information, knowledge and principle: back to metabolism in KEGG. Nucleic Acids Res. 42, D199–D205. doi: 10.1093/nar/gkt1076
Kawada-Matsuo, M., Mazda, Y., Oogai, Y., Kajiya, M., Kawai, T., Yamada, S., et al. (2012). GlmS and NagB regulate amino sugar metabolism in opposing directions and affect Streptococcus mutans virulence. PLoS ONE 7:e33382. doi: 10.1371/journal.pone.0033382
King, S. J. (2010). Pneumococcal modification of host sugars: a major contributor to colonization of the human airway? Mol. Oral Microbiol. 25, 15–24. doi: 10.1111/j.2041-1014.2009.00564.x
King, S. J., Hippe, K. R., and Weiser, J. N. (2006). Deglycosylation of human glycoconjugates by the sequential activities of exoglycosidases expressed by Streptococcus pneumoniae. Mol. Microbiol. 59, 961–974. doi: 10.1111/j.1365-2958.2005.04984.x
Kloosterman, T. G., Bijlsma, J. J. E., Kok, J., and Kuipers, O. P. (2006a). To have neighbour's fare: extending the molecular toolbox for Streptococcus pneumoniae. Microbiol. Read. Engl. 152, 351–359. doi: 10.1099/mic.0.28521-0
Kloosterman, T. G., Hendriksen, W. T., Bijlsma, J. J., Bootsma, H. J., van Hijum, S. A., Kok, J., et al. (2006b). Regulation of glutamine and glutamate metabolism by GlnR and GlnA in Streptococcus pneumoniae. J. Biol. Chem. 281, 25097–25109. doi: 10.1074/jbc.M601661200
Kloosterman, T. G., and Kuipers, O. P. (2011). Regulation of arginine acquisition and virulence gene expression in the human pathogen Streptococcus pneumoniae by transcription regulators ArgR1 and AhrC. J. Biol. Chem. 286, 44594–44605. doi: 10.1074/jbc.M111.295832
Lanie, J. A., Ng, W. L., Kazmierczak, K. M., Andrzejewski, T. M., Davidsen, T. M., Wayne, K. J., et al. (2007). Genome sequence of Avery's virulent serotype 2 strain D39 of Streptococcus pneumoniae and comparison with that of unencapsulated laboratory strain R6. J. Bacteriol. 189, 38–51. doi: 10.1128/JB.01148-06
Leenhouts, K., Venema, G., and Kok, J. (1998). A lactococcal pWV01 based integration toolbox for bacteria. Methods Cell Sci. 20, 35–50. doi: 10.1023/A:1009862119114
Linke, C. M., Woodiga, S. A., Meyers, D. J., Buckwalter, C. M., Salhi, H. E., and King, S. J. (2013). The ABC transporter encoded at the pneumococcal fructooligosaccharide utilization locus determines the ability to utilize long- and short-chain fructooligosaccharides. J. Bacteriol. 195, 1031–1041. doi: 10.1128/JB.01560-12
Lortie, L. A., Pelletier, M., Vadeboncoeur, C., and Frenette, M. (2000). The gene encoding IIAB(Man)L in Streptococcus salivarius is part of a tetracistronic operon encoding a phosphoenolpyruvate: mannose/glucose phosphotransferase system. Microbiol. Read. Engl. 146(Pt 3), 677–685. doi: 10.1099/00221287-146-3-677
Lulko, A. T., Buist, G., Kok, J., and Kuipers, O. P. (2007). Transcriptome analysis of temporal regulation of carbon metabolism by CcpA in Bacillus subtilis reveals additional target genes. J. Mol. Microbiol. Biotechnol. 12, 82–95. doi: 10.1159/000096463
Marion, C., Burnaugh, A. M., Woodiga, S. A., and King, S. J. (2011). Sialic acid transport contributes to pneumococcal colonization. Infect. Immun. 79, 1262–1269. doi: 10.1128/IAI.00832-10
Marion, C., Limoli, D. H., Bobulsky, G. S., Abraham, J. L., Burnaugh, A. M., and King, S. J. (2009). Identification of a pneumococcal glycosidase that modifies O-linked glycans. Infect. Immun. 77, 1389–1396. doi: 10.1128/IAI.01215-08
Marion, C., Stewart, J. M., Tazi, M. F., Burnaugh, A. M., Linke, C. M., Woodiga, S. A., et al. (2012). Streptococcus pneumoniae can utilize multiple sources of hyaluronic acid for growth. Infect. Immun. 80, 1390–1398. doi: 10.1128/IAI.05756-11
Mobley, H. L., Doyle, R. J., Streips, U. N., and Langemeier, S. O. (1982). Transport and incorporation of N-acetyl-D-glucosamine in Bacillus subtilis. J. Bacteriol. 150, 8–15.
Moye, Z. D., Burne, R. A., and Zeng, L. (2014). Uptake and metabolism of N-acetylglucosamine and glucosamine by Streptococcus mutans. Appl. Environ. Microbiol. 80, 5053–5067. doi: 10.1128/AEM.00820-14
Neves, A. R., Ventura, R., Mansour, N., Shearman, C., Gasson, M. J., Maycock, C., et al. (2002). Is the glycolytic flux in Lactococcus lactis primarily controlled by the redox charge? Kinetics of NAD(+) and NADH pools determined in vivo by 13C NMR. J. Biol. Chem. 277, 28088–28098. doi: 10.1074/jbc.M202573200
Nothaft, H., Dresel, D., Willimek, A., Mahr, K., Niederweis, M., and Titgemeyer, F. (2003). The phosphotransferase system of Streptomyces coelicolor is biased for N-acetylglucosamine metabolism. J. Bacteriol. 185, 7019–7023. doi: 10.1128/JB.185.23.7019-7023.2003
Nothaft, H., Rigali, S., Boomsma, B., Swiatek, M., McDowall, K. J., van Wezel, G. P., et al. (2010). The permease gene nagE2 is the key to N-acetylglucosamine sensing and utilization in Streptomyces coelicolor and is subject to multi-level control. Mol. Microbiol. 75, 1133–1144. doi: 10.1111/j.1365-2958.2009.07020.x
Novichkov, P. S., Laikova, O. N., Novichkova, E. S., Gelfand, M. S., Arkin, A. P., Dubchak, I., et al. (2010). RegPrecise: a database of curated genomic inferences of transcriptional regulatory interactions in prokaryotes. Nucleic Acids Res. 38, D111–D118. doi: 10.1093/nar/gkp894
O'Brien, K. L., Wolfson, L. J., Watt, J. P., Henkle, E., Deloria-Knoll, M., McCall, N., et al. (2009). Burden of disease caused by Streptococcus pneumoniae in children younger than 5 years: global estimates. Lancet 374, 893–902. doi: 10.1016/S0140-6736(09)61204-6
Paixão, L., Oliveira, J., Veríssimo, A., Vinga, S., Lourenço, E. C., Ventura, M. R., et al. (2015). Host glycan sugar-specific pathways in Streptococcus pneumoniae: galactose as a key sugar in colonisation and infection [corrected]. PLoS ONE 10:e0121042. doi: 10.1371/journal.pone.0121042
Paulsen, I. T., Nguyen, L., Sliwinski, M. K., Rabus, R., and Saier, M. H. (2000). Microbial genome analyses: comparative transport capabilities in eighteen prokaryotes. J. Mol. Biol. 301, 75–100. doi: 10.1006/jmbi.2000.3961
Phillips, N. J., John, C. M., Reinders, L. G., Gibson, B. W., Apicella, M. A., and Griffiss, J. M. (1990). Structural models for the cell surface lipooligosaccharides of Neisseria gonorrhoeae and Haemophilus influenzae. Biomed. Environ. Mass Spectrom. 19, 731–745. doi: 10.1002/bms.1200191112
Plumbridge, J. (2001). Regulation of PTS gene expression by the homologous transcriptional regulators, Mlc and NagC, in Escherichia coli (or how two similar repressors can behave differently). J. Mol. Microbiol. Biotechnol. 3, 371–380.
Plumbridge, J. A. (1991). Repression and induction of the nag regulon of Escherichia coli K-12: the roles of nagC and nagA in maintenance of the uninduced state. Mol. Microbiol. 5, 2053–2062. doi: 10.1111/j.1365-2958.1991.tb00828.x
Plumbridge, J. A., Cochet, O., Souza, J. M., Altamirano, M. M., Calcagno, M. L., and Badet, B. (1993). Coordinated regulation of amino sugar-synthesizing and -degrading enzymes in Escherichia coli K-12. J. Bacteriol. 175, 4951–4956.
Plumbridge, J., and Vimr, E. (1999). Convergent pathways for utilization of the amino sugars N-acetylglucosamine, N-acetylmannosamine, and N-acetylneuraminic acid by Escherichia coli. J. Bacteriol. 181, 47–54.
Pluvinage, B., Stubbs, K. A., Hattie, M., Vocadlo, D. J., and Boraston, A. B. (2013). Inhibition of the family 20 glycoside hydrolase catalytic modules in the Streptococcus pneumoniae exo-β-D-N-acetylglucosaminidase, StrH. Org. Biomol. Chem. 11, 7907–7915. doi: 10.1039/c3ob41579a
Reizer, J., Bachem, S., Reizer, A., Arnaud, M., Saier, M. H. Jr., and Stulke, J. (1999). Novel phosphotransferase system genes revealed by genome analysis - the complete complement of PTS proteins encoded within the genome of Bacillus subtilis. Microbiol. Read. Engl. 145(Pt 12), 3419–3429. doi: 10.1099/00221287-145-12-3419
Rose, M. C., and Voynow, J. A. (2006). Respiratory tract mucin genes and mucin glycoproteins in health and disease. Physiol. Rev. 86, 245–278. doi: 10.1152/physrev.00010.2005
Saier, M. H., Goldman, S. R., Maile, R. R., Moreno, M. S., Weyler, W., Yang, N., et al. (2002). Transport capabilities encoded within the Bacillus subtilis genome. J. Mol. Microbiol. Biotechnol. 4, 37–67.
Shafeeq, S., Afzal, M., Henriques-Normark, B., and Kuipers, O. P. (2015). Transcriptional profiling of UlaR-regulated genes in Streptococcus pneumoniae. Genomics Data 4, 57–59. doi: 10.1016/j.gdata.2015.02.004
Shafeeq, S., Kloosterman, T. G., and Kuipers, O. P. (2011). CelR-mediated activation of the cellobiose-utilization gene cluster in Streptococcus pneumoniae. Microbiol. Read. Engl. 157, 2854–2861. doi: 10.1099/mic.0.051359-0
Shafeeq, S., Kloosterman, T. G., Rajendran, V., and Kuipers, O. P. (2012). Characterization of the ROK-family transcriptional regulator RokA of Streptococcus pneumoniae D39. Microbiol. Read. Engl. 158, 2917–2926. doi: 10.1099/mic.0.062919-0
Shafeeq, S., Kuipers, O. P., and Kloosterman, T. G. (2013). Cellobiose-mediated gene expression in Streptococcus pneumoniae: a repressor function of the novel GntR-type regulator BguR. PLoS ONE 8:e57586. doi: 10.1371/journal.pone.0057586
Simoni, R. D., Roseman, S., and Saier, M. H. (1976). Sugar transport. Properties of mutant bacteria defective in proteins of the phosphoenolpyruvate: sugar phosphotransferase system. J. Biol. Chem. 251, 6584–6597.
Terra, V. S., Homer, K. A., Rao, S. G., Andrew, P. W., and Yesilkaya, H. (2010). Characterization of novel beta-galactosidase activity that contributes to glycoprotein degradation and virulence in Streptococcus pneumoniae. Infect. Immun. 78, 348–357. doi: 10.1128/IAI.00721-09
Tettelin, H., Nelson, K. E., Paulsen, I. T., Eisen, J. A., Read, T. D., Peterson, S., et al. (2001). Complete genome sequence of a virulent isolate of Streptococcus pneumoniae. Science 293, 498–506. doi: 10.1126/science.1061217
Titgemeyer, F., and Hillen, W. (2002). Global control of sugar metabolism: a gram-positive solution. Antonie Van Leeuwenhoek 82, 59–71. doi: 10.1023/A:1020628909429
Titgemeyer, F., Reizer, J., Reizer, A., and Saier, M. H. Jr. (1994). Evolutionary relationships between sugar kinases and transcriptional repressors in bacteria. Microbiol. Read. Engl. 140(Pt 9), 2349–2354. doi: 10.1099/13500872-140-9-2349
Tong, H. H., Blue, L. E., James, M. A., and DeMaria, T. F. (2000). Evaluation of the virulence of a Streptococcus pneumoniae neuraminidase-deficient mutant in nasopharyngeal colonization and development of otitis media in the chinchilla model. Infect. Immun. 68, 921–924. doi: 10.1128/IAI.68.2.921-924.2000
Tyx, R. E., Roche-Hakansson, H., and Hakansson, A. P. (2011). Role of dihydrolipoamide dehydrogenase in regulation of raffinose transport in Streptococcus pneumoniae. J. Bacteriol. 193, 3512–3524. doi: 10.1128/JB.01410-10
Vadeboncoeur, C. (1984). Structure and properties of the phosphoenolpyruvate: glucose phosphotransferase system of oral streptococci. Can. J. Microbiol. 30, 495–502. doi: 10.1139/m84-073
White, R. J. (1970). The role of the phosphoenolpyruvate phosphotransferase system in the transport of N-acetyl-D-glucosamine by Escherichia coli. Biochem. J. 118, 89–92. doi: 10.1042/bj1180089
Yesilkaya, H., Manco, S., Kadioglu, A., Terra, V. S., and Andrew, P. W. (2008). The ability to utilize mucin affects the regulation of virulence gene expression in Streptococcus pneumoniae. FEMS Microbiol. Lett. 278, 231–235. doi: 10.1111/j.1574-6968.2007.01003.x
Zeng, L., and Burne, R. A. (2015). NagR differentially regulates the expression of the glmS and nagAB genes required for amino sugar metabolism by Streptococcus mutans. J. Bacteriol. 197, 3533–3544. doi: 10.1128/JB.00606-15
Keywords: N-acetylglucosamine, NAG, NagA, NagB, GlmS, pneumococcus, CcpA
Citation: Afzal M, Shafeeq S, Manzoor I, Henriques-Normark B and Kuipers OP (2016) N-acetylglucosamine-Mediated Expression of nagA and nagB in Streptococcus pneumoniae. Front. Cell. Infect. Microbiol. 6:158. doi: 10.3389/fcimb.2016.00158
Received: 17 January 2016; Accepted: 02 November 2016;
Published: 16 November 2016.
Edited by:
Jorge Eugenio Vidal, Emory University, USAReviewed by:
Samantha J. King, Ohio State University, USAMarco Rinaldo Oggioni, University of Leicester, UK
Zehava Eichenbaum, Georgia State University, USA
Copyright © 2016 Afzal, Shafeeq, Manzoor, Henriques-Normark and Kuipers. This is an open-access article distributed under the terms of the Creative Commons Attribution License (CC BY). The use, distribution or reproduction in other forums is permitted, provided the original author(s) or licensor are credited and that the original publication in this journal is cited, in accordance with accepted academic practice. No use, distribution or reproduction is permitted which does not comply with these terms.
*Correspondence: Oscar P. Kuipers, by5wLmt1aXBlcnNAcnVnLm5s