- 1Foodborne and Waterborne Diseases Research Center, Research Institute for Gastroenterology and Liver Diseases, Shahid Beheshti University of Medical Sciences, Tehran, Iran
- 2Regulatory Department, Production and Research Complex, Pasteur Institute of Iran, Tehran, Iran
- 3Department of Microbiology, Faculty of Medicine, Tehran Medical Sciences, Islamic Azad University, Tehran, Iran
- 4Gastroenterology and Liver Diseases Research Center, Research Institute for Gastroenterology and Liver Diseases, Shahid Beheshti University of Medical Sciences, Tehran, Iran
Toxoplasma gondii is a zoonotic intracellular protozoan with worldwide distribution. Acute and severe toxoplasmosis are commonly reported in patients who suffer from acquired/congenital immune deficiency. This study aimed to synthesize mannosylated paromomycin-loaded solid lipid nanoparticles (PM-SLN-M) and to evaluate them on acute toxoplasmosis. SLN was synthesized and then loaded by 7 mg/mL paromomycin sodium. Mannose coating was performed, and after washing, the size, zeta potential, and loading percentage were calculated. To evaluate the cell toxicity, an MTT assay was performed on Vero cells by different concentrations (log 10−1) of SLN, PM-SLN-M, and PM-SLN. In addition, the anti-Toxoplasma effects were also evaluated using trypan-blue staining and scanning electron microscopy (SEM). An MTT assay was also employed to evaluate the effects of PM and PM-SLN-M on intracellular Toxoplasma. A 6-month stability test of PM-SLN and PM-SLN-M represented that the characteristics all remained constant. The cell viability assay demonstrated that PM-SLN-M had lower cell toxicity (<20%) compared to PM-SLN (<30%) and PM (<40%). Statistical analysis showed that PM-SLN-M significantly killed ~97.555 ± 0.629 (95% CI: 91.901 to 103.209; P < 0.05) of T. gondii tachyzoites. More than 50% of Toxoplasma-infected Vero cells remained viable in concentrations more than 0.07 μg/mL and 7 μg/mL of PM and PM-SLN-M, respectively. SEM analysis showed that T. gondii tachyzoites were changed in both size and morphology facing with PM-SLN-M. Our findings indicated that synthesized PM-SLN-M had anti-Toxoplasma activity without significant host cell toxicity at the highest concentration. Our study demonstrated that PM was able to kill intracellular Toxoplasma in lower concentration in comparison to PM-SLN-M, although PM-SLN-M showed lower cytotoxic effects on Vero cells.
Introduction
Toxoplasma gondii is a zoonotic obligate intracellular protozoan parasite that infects a broad range of warm-blooded animals from birds to humans. Global estimation shows 30–50% of the world's population are serology positive for toxoplasmosis (Robert-Gangneux and Darde, 2012; Flegr et al., 2014; Aguirre et al., 2019). The prevalence rates 0–100% were reported for toxoplasmosis regarding the methods of detection and geographical regions (Robert-Gangneux and Darde, 2012; Liu et al., 2015; Rostami et al., 2018).
T. gondii has three main stages during its life cycle, including the tachyzoite, tissue cyst (bradyzoite), and oocyst phases, of which tachyzoite is responsible for cell invasion, and acute phase of the disease (Kato, 2018). Although ingestion of mature oocyst via food and water is the main route of infection (Hill and Dubey, 2016), consumption of raw meat containing cysts, needle injection (particularly in research laboratory), congenital infection, blood transfusion (particularly white blood cells), and organ transplantation from an infected person are the other potential routes of transmission (Montoya and Liesenfeld, 2004).
Pathogenesis of T. gondii may differ based on its genotypes/strains; however, it seems that toxoplasmosis is asymptomatic in more than 80% of immunocompetent subjects (Montoya and Liesenfeld, 2004). Toxoplasmosis in immunocompromised patients who do not properly respond to the infection is the main challenge. Accordingly, acute infection due to toxoplasmosis ranks high on the list of fatal diseases in HIV/AIDS patients (Hill and Dubey, 2002; Ahmadpour et al., 2019). Indeed, acute and severe toxoplasmosis are commonly reported from transplant recipients (Martina et al., 2011; Paccoud et al., 2019; Schmidt-Hieber et al., 2019), cancer patients (Fox et al., 2017; Bajnok et al., 2019; Melchor and Ewald, 2019), and patients who suffer from acquired/congenital immunodeficiency (Vijaykumar et al., 2018; McDermott et al., 2019). Taken together with the complications due to acute toxoplasmosis in immunocompromised patients, more recently, the potential role of activated toxoplasmosis in response to the immunomodulatory drugs during inflammatory bowel diseases (IBD) and its pathogenesis was suggested (Mirjalali et al., 2019). Therefore, prescription of a standard drug regimen with low side effects is an important issue during acute toxoplasmosis.
Pyrimethamine and sulfadiazine are standard drugs of choice in the case of acute toxoplasmosis. However, because of their adverse and side effects, the need for a new treatment plan has been highlighted (Martins-Duarte et al., 2006; Alday and Doggett, 2017).
Paromomycin (aminosidine; PM) is an aminoglycoside–aminocyclitol antibiotic with a broad-spectrum toxicity against bacteria, as well as some parasites such as Giardia, Leishmania, and Entamoeba histolytica (Kappagoda et al., 2011). This drug faces many challenges, such as quick renal excretion and short half-life in the blood circulation. Indeed, this drug is hydrophilic and has a high molecular weight that makes its uptake difficult (Ghadiri et al., 2012; Afzal et al., 2019). However, PM can be a good option for treatment of parasitic disease due to its safety, low cost, and short course therapy.
Over the years, drug delivery systems have been experienced as a way to enhance the effectiveness of some drugs such as PM (Ghadiri et al., 2012; Gaspar et al., 2015). So far, liposomes have been used as a drug delivery system in many studies where the results indicated improved penetration properties and therapeutic effects of PM (Gaspar et al., 2015; Heidari-Kharaji et al., 2016a). The current study aimed to evaluate the cell toxicity and anti-Toxoplasma potency of PM-SLN-M, in vitro.
Methods
Preparation of Uncoated SLNs Loaded by PM (PM-SLN)
SLN was prepared by solvent injection method according to the procedure reported by Schubert and Muller-Goymann with slight modifications (Schubert and Muller-Goymann, 2003). Briefly, tristearin (1% w/v) and soya lecithin (PC; 0.3% w/v) were dissolved in a 10-mL mixture of acetone and ethanol (1:1 v/v) at 70°C. Then, stearyl amine (SA) was added into the mixture with the ratio of 10 mol % of soya lecithin. Temperature was kept at 70°C during the process. The melt was rapidly injected through a syringe at a flow rate of 5 mL/min into a stirred aqueous phase (with 0.2% w/v Tween 80) containing PM (0.1% w/v) maintained at the same temperature. The suspension was stirred at 4,000 rpm for 1 h, sonicated for 5 min, and then was filtered through membrane filter (0.45 μm) to remove any excess lipid. After this process, the PM-SLNs were ready for coating with mannose.
Preparation of Mannosylated SLNs Loaded by PM (PM-SLN-M)
Mannose coating was carried out in accordance with the previously reported method (Kumar et al., 2006). D-mannose (8 μM) was dissolved in sodium acetate buffer (pH 4.0; 0.1 M) and was added to uncoated SLNs. The mixture was continuously stirred using magnetic stirrer at room temperature for 3 days. Mannosylated nanoparticles were then subjected to extensive dialysis (dialysis bag; MWCO 12–14 kDa, Himedia, India) against double distilled water (DDW) for 30 min to remove uncoated mannose and other impurities (Ghadiri et al., 2011). Figure 1 schematically overviews the preparation of PM-SLN-M (Figure 1).
Drug Content Determination
Photon correlation spectroscopy was employed to measure the size, pumulates mean size (z-potential), and the polydispersity index (PDI) of the particles (Zetasizer Nano Series, Malvern, UK) at 25°C. The amount of PM in supernatant was determined by high performance liquid chromatography (HPLC) as mentioned elsewhere (Pick et al., 1997).
Cell Culture
In the present study, African green monkey kidney cells (Vero) were used for in vitro assay. Accordingly, Vero cells were grown in Dulbecco's Modified Eagle Medium (DMEM; Gibco BRL, USA) supplemented with 10% fetal bovine serum (FBS; Gibco BRL) and 1% penicillin/streptomycin (Gibco BRL) at pH 7.2, 37°C, and 5% CO2.
Parasite
Tachyzoites of T. gondii (RH strain), which had been passaged in BALB/c mice (8–10-week-old, 20–25 g weight) using intraperitoneal (IP) injection, were washed with sterile phosphate-buffered saline (PBS; pH 7.4). After counting by hemocytometer slide, 1.5 × 106 tachyzoites per mL was incubated with ~105 Vero cells [multiplicity of infection (MOI) = 10], cultivated in DMEM supplemented with 10% FBS and 1% penicillin/streptomycin for mass cultivation. Every 3–5 days, the cells were checked to investigate the Toxoplasma growth. After maximum cell rupture and releasing tachyzoites, the parasites were harvested for further analyses.
Cell Cytotoxicity Assay
To evaluate the toxicity effects of the synthesized nanocomponents, the viability rate of Vero cell against six log−10 concentrations (7 mg/mL−70 ng/mL) of SLN, PM-SLN, and PM-SLN-M was evaluated using MTT assay. Briefly, 2.38 × 105 Vero cells in the exponential growth stage were seeded in a 96-well cell plate containing DMEM supplemented with 10% FBS for 48 h without any antibiotics. Then, the cells were treated with SLN, PM-SLN, PM-SLN-M, and PM sodium (log−10 from 7 mg/mL to 70 ng/mL). A well of Vero cells without treatment was considered as negative control. After 48 h incubation, the culture supernatant was removed and 15% (v/v) of MTT solution (5 mg/mL) was directly added to the wells, and the plate was incubated for 4 h at 37°C in 5% CO2. Formazan extraction was performed by incubation with Me2SO (150 μL/well) at 37°C for 10 min, and the absorbance of the plates at 570 nm was read with ELISA reader (LX800; Biotec, Winooski, VA, USA).
Toxicity effects of the defined concentrations were calculated according to the following equation. The minimum concentrations that inhibited an average of 50% of the parasites and host cells were considered as inhibitory concentration (IC50) and cytotoxic concentration (CC50), respectively.
Viable microorganisms % = [(AT – AB) / (AC – AB)] × 100
Nonviable microorganisms % = 100 – Viable microorganisms %
AT is the OD of treated well, AC is the OD of negative control, and AB is the OD of the blank well. A well of Vero cells without treatment and a well with only the media were considered as negative control and blank, respectively. Mean ± SD of the experiments was reported for each sample.
Parasite Toxicity Assay
To study the anti-Toxoplasma activity of PM-SLN-M, six concentrations of PM (as positive control) and PM-SLN-M (log−10 from 7 mg/mL to 70 ng/mL) were added to 105 parasites per well of a 96-well cell culture plate containing DMEM supplemented with 10% FBS without antibiotic. After 2 h incubation in 37°C and 5% CO2, the number of alive Toxoplasma tachyzoites was counted using vital staining (trypan-blue) and hemocytometer slide.
Viability and Non-viability data was calculated using the following equation:
Nonviable microorganisms % = (PT / NT) × 100
Viable microorganisms % = 100 – Nonviable microorganisms %
*PT is the number of parasite in each test well; NT is the number of parasite in a well without treatments. Mean ± SD of the experiments was reported for each sample.
Ratio
To determine the best concentration of PM-SLN-M and compare the results to PM, the anti-Toxoplasma effects and the cell viability percentage were calculated using the following formula. The concentration closer to 1 was considered as the best dosage.
Intracellular Anti-Toxoplasma Activity
In order to investigate the effects of PM-SLN-M on the intracellular Toxoplamsa, 105 Vero cells were seeded in a 96-well cell culture plate containing DMEM supplemented with 10% FBS without antibiotics. After ~70–80% confluency, 105 tachyzoites of Toxoplasma (MOI = 1) were inoculated in each well, and the plate was incubated at 37°C and 5% CO2 for 24 h. Then, the wells were checked by inverted light microscope to confirm the maximum cellular invasion by the parasites. Afterwards, the culture supernatant was removed, and wells were washed twice by sterile PBS to remove cell debris, dead cells, and extracellular tachyzoite. The cells were then treated with PM-SLN-M and PM sodium (log−10 from 7 mg/mL to 70 ng/mL), together with DMEM supplemented with 10% FBS for 48 h. Finally, the culture supernatant was removed and MTT assay was performed.
Scanning Electron Microscopy (SEM)
To evaluate the anti-Toxoplasma effects of PM-SLN-M formulation, after 2-h exposure of 105 Toxoplasma tachyzoites with the nanodrug, tachyzoites were attached on a slide, fixed with 2% paraformaldehyde and 2.5% glutaraldehyde in 0.1 M sodium cacodylate buffer (pH 7.4) and washed in cacodylate buffer. Then, the slide was post-fixed for 2–4 h using 1–2% osmium tetroxide in 0.1 M phosphate buffer (pH 7.2) at room temperature and dehydrated in graded ethanol dilutions (70, 80, 90, and 100%). Cells were dried using critical point method, mounted on stubs, coated with gold (20–30 nm), and then observed using SEM.
Statistical Analysis
One-sample t-test incorporated in GraphPad Prism software (version 8.0.2) was employed to calculate mean ± SD, confidence interval, and statistical correlation between concentration and components. P < 0.05 was considered as statistically significant.
Results
PM-SLN-M Characterization and Properties
Specific properties of prepared PM-SLN-M such as size, polydispersity index, percentage of PM loading, and zeta potential of the particles are summarized in Table 1. The average size of the particles was 246 ± 32 nm, and the stability of the formulation (accelerated, 6 months), which was evaluated based on protocol ISCH-Q6 and stored in the refrigerator, showed good results (Figure 2). The release of drug from the PM-SLN-M formulation was slow and lasted for 24 h in the aqueous media. Indeed, the average entrapment efficiency of PM-SLN-M was 47–48%.
Nanocomponent Toxicity Assay
Toxicity assay of nanoformulations showed that more than 78.96 ± 0.07% (95% CI: 78.325–79.595%) of Vero cells remained viable at the highest concentration (7 mg/mL) of PM-SLN-M (CC50 > 7 mg/mL) while almost 56.85 ± 0.255% (95% CI: 54.563–59.137%) of the cells dead using the same concentration of PM (P < 0.05; CC50 > 7 mg/mL). Comparison of cytotoxicity effects of PM-SLN with PM-SLN-M showed that mannosylation reduced the cell toxicity of the nanodrug from ~30 to 20% of Vero cells at the highest concentration. Indeed, toxicity effects of only SLN was evaluated that showed this component was not toxic for Vero cell, even at the highest concentration (Figure 3; Table 2).
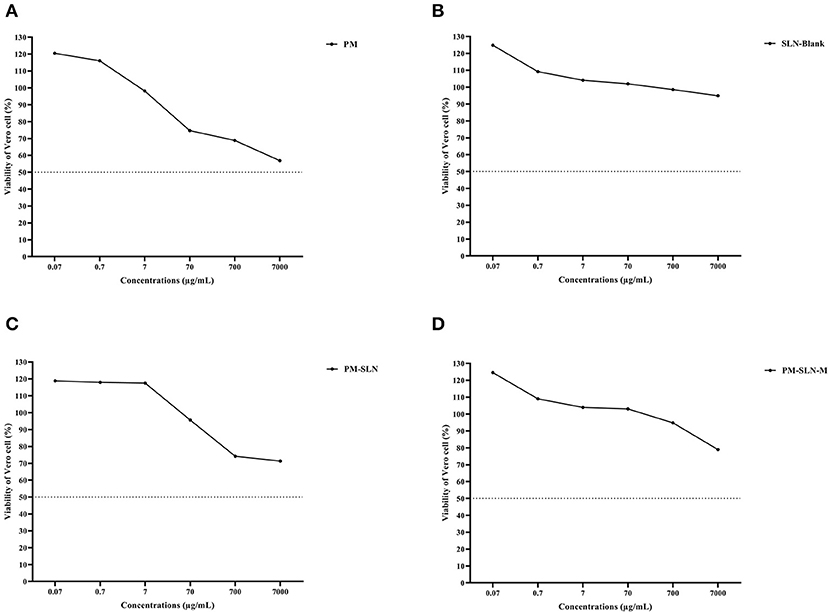
Figure 3. Cell toxicity assay of (A) PM, (B) SLN-BLANK, (C) PM-SLN, and (D) PM-SLN-M on Vero cell line.
Anti-Toxoplasma Effects of PM-SLN-M
The results of vital staining showed that at the highest concentration, PM-SLN-M was able to kill 97.555 ± 0.629% (95% CI: 91.901–103.209%) of tachyzoites, in comparison to PM (92.065 ± 1.322% [95% CI: 80.185–103.945%]; IC50 > 0.07 μg/mL). In addition, at the lowest concentration (0.07 μg/mL), PM-SLN-M killed 85.895 ± 0.148% (95% CI: 84.561–87.229%), while PM was toxic for 79.55 ± 0.636% (95% CI: 73.832–85.268%) of Toxoplasma tachyzoites (IC50 > 0.07 μg/mL) (Figure 4; Table 3).
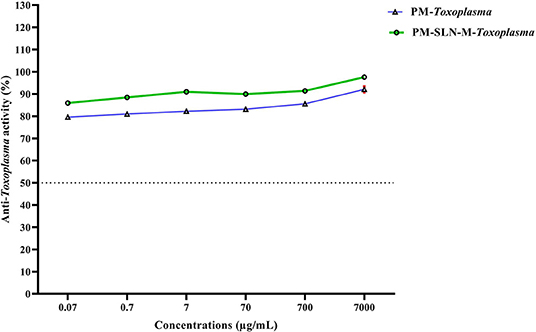
Figure 4. Comparison of anti-Toxoplasma activity of PM and PM-SLN-M according to different concentrations.
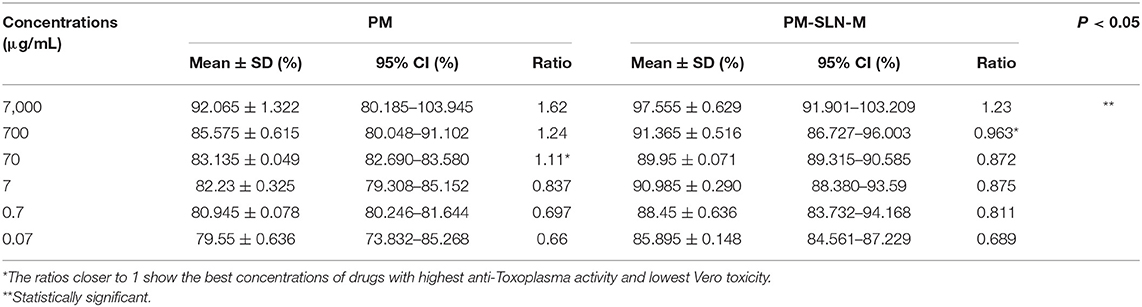
Table 3. Anti-Toxoplasma activity of different concentrations of PM and PM-SLN-M and the ratio value of anti-parasite activity per Vero cell viability.
Anti-intracellular Toxoplasma Effects of PM-SLN-M
The results represented that at least 50% of the infected Vero cells treated by the concentration higher than 7 μg/mL of PM-SLN-M remained viable (IC 50 > 7 μg/mL). Moreover, PM was able to kill Toxoplasma in the infected Vero cells by concentrations more than 0.07 μg/mL (IC 50 > 0.07 μg/mL; Figure 5; Table 4).
Ratio Analysis
The comparison of the efficacy to the safety of PM-SLN-M and PM, ratio analysis was performed that the results showed PM-SLN-M at the concentration 700 μg/mL and ratio 0.963 had the highest and lowest toxicity for Toxoplasma tachyzoites and Vero cells, respectively. Indeed, PM showed the highest and lowest toxicity for Toxoplasma tachyzoites and Vero cells, respectively, at the concentration 70 μg/mL with ratio 1.11 (Figure 6).
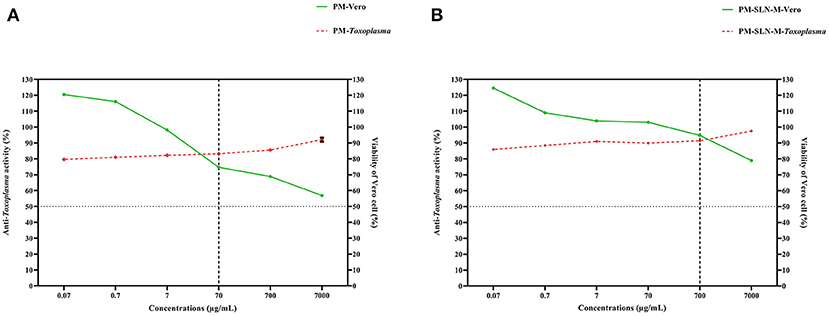
Figure 6. Ratio analysis of (A) PM and (B) PM-SLN-M shows that PM-SLN-M at the higher concentration than that in PM (700 vs. 70 μg/mL) revealed highest and lowest anti-Toxoplasma activity and Vero cell toxicity, respectively.
Scanning Electron Microscopy
Morphological analysis of T. gondii tachyzoites treated with PM-SLN-M represented a non-crescent shape with abnormal surface, while tachyzoites in control group remained normal in shape and size with a smooth surface (Figure 7).
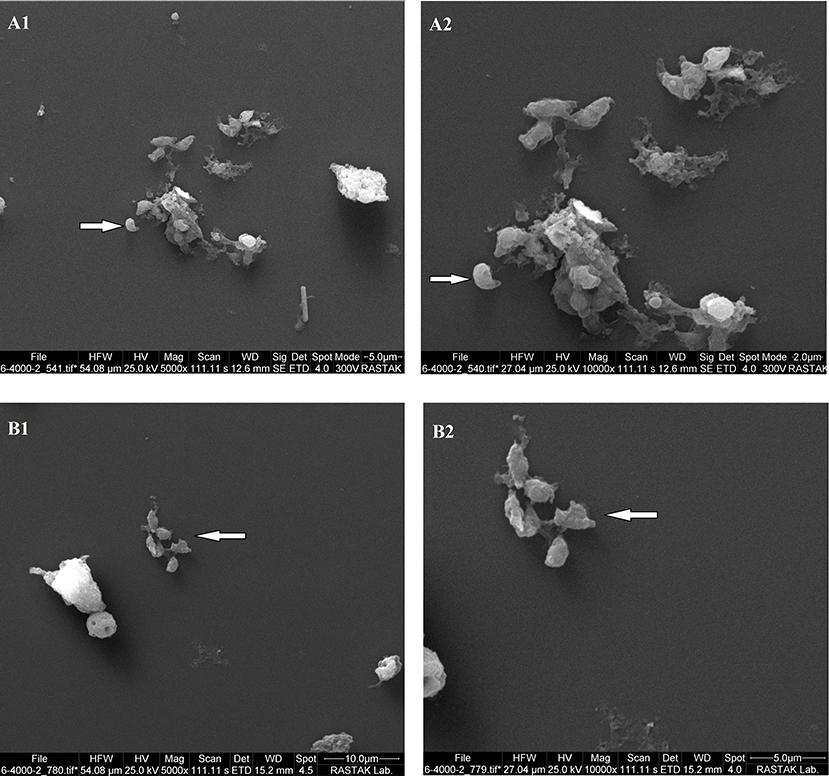
Figure 7. The results of SEM analysis reveals changes in size and morphology of Toxoplasma tachyzoites in two magnifications. (A1,A2) show untreated tachyzoites, and (B1,B2) show PM-SLN-M treated tachyzoites.
Discussion
Acute severe toxoplasmosis in immunocompromised patients and women who are infected by T. gondii during the first pregnancy is the main challenge of physicians (Montoya and Liesenfeld, 2004). Although there are single or combined drug regimens facing with acute toxoplasmosis, most of the recommended drugs are not effective enough or may lead to side effects (Alday and Doggett, 2017). However, sulfadiazine-pyrimethamine, spiramycin, clindamycin, and atovaquone are known the drugs of choice, despite being of different efficacy and probable side effects (Alday and Doggett, 2017; Montazeri et al., 2017). It was claimed that the combination of sulfadiazine and pyrimethamine, recommended drugs for acute toxoplasmosis, may lead to immunosuppression and anemia due to clampdown of bone marrow production despite of its good efficacy (Crespo et al., 2000; Faucher et al., 2011). Indeed, although parasitocidal effects of atovaquone on protozoan parasites have been demonstrated, the need for high dosage due to low bioavailability is the main challenge in oral prescription of this drug (Pentewar et al., 2015; Darade et al., 2018).
Notably, different nanoformulations and nanomaterials have been used in practice and the results are promising. In addition, it was strongly claimed that utilizing nanomaterials in either combination or formulation of the conventional drugs not only decreases the probable side effects, but also increases their efficiency (Barabadi et al., 2019).
Although many nanoformulations of available drugs were tested either in vivo or in vitro, there is no data representing efficiency of PM and its nanoformulations on toxoplasmosis. Gaafar et al. (2014) worked on chitosan and silver nanoparticles either singly or in a component against Toxoplasma and reported significant reduction in the parasite count in the infected mice. Anti-Toxoplasma activity of different molecular weights and concentrations of chitosan was then evaluated by Teimouri et al. (2018), who reported significant anti-Toxoplasma toxicity of all molecular weights and concentrations. Azami et al. (2018a) assessed nanoemulsion of atovaquon for treatment of both chronic and acute toxoplasmosis and revealed that this component was toxic for both tachyzoites and brain cysts of Toxoplasma. At the same time, Azami et al. (2018b) evaluated curcumin nanoemulsion (CR-NE) on both chronic and acute toxoplasmosis and demonstrated that CR-NE can be considered a promising compound for the treatment of toxoplasmosis. However, in these studies, host cell toxicity was not assessed on the cell lines to reveal a ratio of anti-Toxoplasma effect to host cell toxicity. In the current study, PM-SLN-M showed highest anti-Toxoplasma activity and lowest host cell toxicity at the concentration 700 μg/mL, in comparison to PM, which showed highest anti-Toxoplasma activity and lowest host cell toxicity at the concentration 70 μg/mL. This finding suggests that SLN mannosylation not only increases anti-Toxoplasma activity, but also decrease host cell toxicity of PM.
Our results confirmed the previous studies showing non-toxicity of SLN-PM either in vitro or in vitro. PM is a broad-spectrum, well-known antibiotic whose bactericidal and parasiticidal properties are shown satisfactory. Although the hydrophilic structure of this drug makes penetration of PM to the target cells difficult, there are a number of studies that reported satisfactory outcomes that resulted from the nanoformulation of PM. Up to now, nanoformulation of PM was mostly practiced on different types of leishmaniasis. Heidari-Kharaji et al. (2016a,b) showed that PM-SLN was a non-toxic compound that increased the anti-Leishmania toxicity of PM.
Notably, short half-life and specific drug delivery of PM are the main challenges during clinical practices. It was demonstrated that SLN carriers can increase the half-life of PM through controlling the drug release. Ghadiri et al. (2012) examined entrapment efficiency and release profile of PM-loaded SLN using statistical modeling and showed that SLN led to gradually efficient prolonged release of PM. Interestingly, in immunocompromised patients, chronic cysts of Toxoplasma may rupture due to insufficient immune responses; thus, activated tachyzoites can lead to lethal complications. Therefore, since SLN controls efficient prolonged release of PM, it seems PM-SLN can remain effective for a long time.
Additionally, macrophages are the first line of defense during acute toxoplasmosis (Kato, 2018; Lima and Lodoen, 2019); therefore, increasing the drug uptake by macrophages is the most important challenge during treatment of acute toxoplasmosis. Mannose receptor, known as CD206, is mostly expressed by macrophages and dendritic cells. This receptor binds with mannose that usually covers outer surface of microbes and leads to scavenging pathogens (Azad et al., 2014). Notably, macrophages are the central host cells which are infected by intracellular pathogens such as Leishmania and Toxoplasma, and it was well-established that mannosylated PM-SLN not only increase the bioavailability of PM, but also mannosylation enhance uptaking PM by macrophages (Frenz et al., 2015). In the study conducted by Afzal et al. (2019), the maximum uptake was observed in macrophages that were treated by mannosylated thiolated chitosan-coated PM-loaded PLGA nanoparticles.
As a result, PM was able to kill intracellular Toxoplasma in lower concentration than PM-SLN-M. Nonetheless, regarding the lower cell toxicity of PM-SLN-M in comparison to PM, it seems that PM-SLN-M could be a better choice particularly in long-term therapy.
In summary, our findings demonstrate that although PM is usually used for therapy of giardiasis, leishmaniosis, and amoebiasis, nanoformulation of this drug may make it a useful drug for acute toxoplasmosis. Indeed, PM-SLN-M showed higher anti-Toxoplasma toxicity in comparison to conventional PM and mannosylation may increase up taking the drug by macrophages. Taken together, it seems that nanoformulation not only increases efficiency of available commercial drugs, but also decreases the cytotoxicity of them against host's cell.
Data Availability Statement
All datasets generated for this study are included in the article/supplementary files.
Ethics Statement
All procedures performed in this study were in accordance with the ethical standards (IR.SBMU.RIGLD.REC.1398.034) released by the Ethical Review Committee of the Research Institute for Gastroenterology and Liver Diseases, Shahid Beheshti University of Medical Sciences, Tehran, Iran.
Author Contributions
HM designed the study. DD synthesized nanoparticles. MK, HMR, and HM contributed in performing the experiments and analyzing the generated data. MK, EM, and HM contributed in writing the manuscript. MZ supported and supervised the study.
Funding
This project was financially supported by the Research Institute for Gastroenterology and Liver Diseases, Shahid Beheshti University of Medical Sciences, Tehran, Iran with grant number: RIGLD 1058.
Conflict of Interest
The authors declare that the research was conducted in the absence of any commercial or financial relationships that could be construed as a potential conflict of interest.
Acknowledgments
The authors thank all members of Foodborne and Waterborne Diseases Research Center for their collaborations.
References
Afzal, I., Sarwar, H.S., Sohail, M. F., Varikuti, S., Jahan, S., Akhtar, S., et al. (2019). Mannosylated thiolated paromomycin-loaded PLGA nanoparticles for the oral therapy of visceral leishmaniasis. Nanomedicine 14, 387–406. doi: 10.2217/nnm-2018-0038
Aguirre, A. A., Longcore, T., Barbieri, M., Dabritz, H., Hill, D., Klein, P. N., et al. (2019). The one health approach to toxoplasmosis: epidemiology, control, and prevention strategies. EcoHealth 16, 378–390. doi: 10.1007/s10393-019-01405-7
Ahmadpour, E., Pishkarie-Asl, R., Spotin, A., Samadi Kafil, H., Didarlu, H., Azadi, Y., et al. (2019). Sero-molecular evaluation of Toxoplasma gondii infection among HIV-positive patients. Trans. R. Soc. Trop. Med. Hyg. 113, 771–775. doi: 10.1093/trstmh/trz082
Alday, P. H., and Doggett, J. S. (2017). Drugs in development for toxoplasmosis: advances, challenges, and current status. Drug Des. Dev. Ther. 11, 273–293. doi: 10.2147/DDDT.S60973
Azad, A. K., Rajaram, M. V., and Schlesinger, L. S. (2014). Exploitation of the macrophage mannose receptor (CD206) in infectious disease diagnostics and therapeutics. J. Cytol. Mol. Biol. 1:1000003. doi: 10.13188/2325-4653.1000003
Azami, S. J., Amani, A., Keshavarz, H., Najafi-Taher, R., Mohebali, M., Faramarzi, M. A., et al. (2018a). Nanoemulsion of atovaquone as a promising approach for treatment of acute and chronic toxoplasmosis. Eur. J. Pharm. Sci. 117, 138–146. doi: 10.1016/j.ejps.2018.02.018
Azami, S. J., Teimouri, A., Keshavarz, H., Amani, A., Esmaeili, F., Hasanpour, H., et al. (2018b). Curcumin nanoemulsion as a novel chemical for the treatment of acute and chronic toxoplasmosis in mice. Int. J. Nanomed. 13, 7363–7374. doi: 10.2147/IJN.S181896
Bajnok, J., Tarabulsi, M., Carlin, H., Bown, K., Southworth, T., Dungwa, J., et al. (2019). High frequency of infection of lung cancer patients with the parasite Toxoplasma gondii. ERJ Open Res. 5:00143-2018. doi: 10.1183/23120541.00143-2018
Barabadi, H., Alizadeh, Z., Rahimi, M. T., Barac, A., Maraolo, A. E., Robertson, L. J., et al. (2019). Nanobiotechnology as an emerging approach to combat malaria: a systematic review. Nanomed. Nanotech. Biol. Med. 18, 221–233. doi: 10.1016/j.nano.2019.02.017
Crespo, M., Quereda, C., Pascual, J., Rivera, M., Clemente, L., and Cano, T. (2000). Patterns of sulfadiazine acute nephrotoxicity. Clin. Nephrol. 54, 68–72.
Darade, A., Pathak, S., Sharma, S., and Patravale, V. (2018). Atovaquone oral bioavailability enhancement using electrospraying technology. Eur. J. Pharm. Sci. 111, 195–204. doi: 10.1016/j.ejps.2017.09.051
Faucher, B., Moreau, J., Zaegel, O., Franck, J., and Piarroux, R. (2011). Failure of conventional treatment with pyrimethamine and sulfadiazine for secondary prophylaxis of cerebral toxoplasmosis in a patient with AIDS. J. Antimicrob. Chemother. 66, 1654–1656. doi: 10.1093/jac/dkr147
Flegr, J., Prandota, J., Sovickova, M., and Israili, Z. H. (2014). Toxoplasmosis–a global threat. Correlation of latent toxoplasmosis with specific disease burden in a set of 88 countries. PLoS ONE 9:e90203. doi: 10.1371/journal.pone.0090203
Fox, B. A., Butler, K. L., Guevara, R. B., and Bzik, D. J. (2017). Cancer therapy in a microbial bottle: uncorking the novel biology of the protozoan Toxoplasma gondii. PLoS Pathog. 13:e1006523. doi: 10.1371/journal.ppat.1006523
Frenz, T., Grabski, E., Duran, V., Hozsa, C., Stepczynska, A., Furch, M., et al. (2015). Antigen presenting cell-selective drug delivery by glycan-decorated nanocarriers. Eur. J. Pharm. Biopharm. 95(Pt A), 13–17. doi: 10.1016/j.ejpb.2015.02.008
Gaafar, M. R., Mady, R. F., Diab, R. G., and Shalaby, T. I. (2014). Chitosan and silver nanoparticles: promising anti-toxoplasma agents. Exp. Parasitol. 143, 30–38. doi: 10.1016/j.exppara.2014.05.005
Gaspar, M. M., Calado, S., Pereira, J., Ferronha, H., Correia, I., Castro, H., et al. (2015). Targeted delivery of paromomycin in murine infectious diseases through association to nano lipid systems. Nanomedicine 11, 1851–1860. doi: 10.1016/j.nano.2015.06.008
Ghadiri, M., Fatemi, S., Vatanara, A., Doroud, D., Najafabadi, A. R., Darabi, M., et al. (2012). Loading hydrophilic drug in solid lipid media as nanoparticles: statistical modeling of entrapment efficiency and particle size. Int. J. Pharm. 424, 128–137. doi: 10.1016/j.ijpharm.2011.12.037
Ghadiri, M., Vatanara, A., Doroud, D., and Roholamini Najafabadi, A. (2011). Paromomycin loaded solid lipid nanoparticles: characterization of production parameters. Biotech. Biopro. Eng. 16, 617–623. doi: 10.1007/s12257-010-0331-5
Heidari-Kharaji, M., Taheri, T., Doroud, D., Habibzadeh, S., Badirzadeh, A., and Rafati, S. (2016a). Enhanced paromomycin efficacy by solid lipid nanoparticle formulation against Leishmania in mice model. Parasite Immunol. 38, 599–608. doi: 10.1111/pim.12340
Heidari-Kharaji, M., Taheri, T., Doroud, D., Habibzadeh, S., and Rafati, S. (2016b). Solid lipid nanoparticle loaded with paromomycin: in vivo efficacy against Leishmania tropica infection in BALB/c mice model. Appl. Microbiol. Biotechnol. 100, 7051–7060. doi: 10.1007/s00253-016-7422-y
Hill, D., and Dubey, J. P. (2002). Toxoplasma gondii: transmission, diagnosis and prevention. Clin. Microbiol. Infect. 8, 634–640. doi: 10.1046/j.1469-0691.2002.00485.x
Hill, D. E., and Dubey, J. P. (2016). Toxoplasma gondii as a parasite in food: analysis and control. Microbiol. Spectr. 4, 1–17. doi: 10.1128/microbiolspec.PFS-0011-2015
Kappagoda, S., Singh, U., and Blackburn, B. G. (2011). Antiparasitic therapy. Mayo Clin. Proc. 86, 561–583. doi: 10.4065/mcp.2011.0203
Kato, K. (2018). How does Toxoplama gondii invade host cells? J. Vet. Med. Sci. 80, 1702–1706. doi: 10.1292/jvms.18-0344
Kumar, P. V., Asthana, A., Dutta, T., and Jain, N. K. (2006). Intracellular macrophage uptake of rifampicin loaded mannosylated dendrimers. J. Drug Target 14, 546–556. doi: 10.1080/10611860600825159
Lima, T. S., and Lodoen, M. B. (2019). Mechanisms of human innate immune evasion by Toxoplasma gondii. Front. Cell Infect. Microbiol. 9:103. doi: 10.3389/fcimb.2019.00103
Liu, Q., Wang, Z. D., Huang, S. Y., and Zhu, X. Q. (2015). Diagnosis of toxoplasmosis and typing of Toxoplasma gondii. Parasit Vectors 8:292. doi: 10.1186/s13071-015-0902-6
Martina, M. N., Cervera, C., Esforzado, N., Linares, L., Torregrosa, V., Sanclemente, G., et al. (2011). Toxoplasma gondii primary infection in renal transplant recipients. Two case reports and literature review. Transplant. Int. 24, e6–12. doi: 10.1111/j.1432-2277.2010.01173.x
Martins-Duarte, E. S., Urbina, J. A., de Souza, W., and Vommaro, R. C. (2006). Antiproliferative activities of two novel quinuclidine inhibitors against Toxoplasma gondii tachyzoites in vitro. J. Antimicrob. Chemother. 58, 59–65. doi: 10.1093/jac/dkl180
McDermott, D. H., Heusinkveld, L. E., Zein, W. M., Sen, H. N., Marquesen, M. M., Parta, M., et al. (2019). Case report: ocular toxoplasmosis in a WHIM syndrome immunodeficiency patient. F1000Res. 8:2. doi: 10.12688/f1000research.16825.1
Melchor, S. J., and Ewald, S. E. (2019). Disease tolerance in Toxoplasma infection. Front. Cell. Infect. Microbiol. 9:185. doi: 10.3389/fcimb.2019.00185
Mirjalali, H., Shahrokh, S., Asadzadeh Aghdaei, H., and Zali, M. R. (2019). Letter to the editor: activated Toxoplasma may attenuate the effect of azathioprine and deteriorate the symptoms in ibd patients via mTORC1manipulation. Inflamm. Bowel Dis. 25:e137. doi: 10.1093/ibd/izz161
Montazeri, M., Sharif, M., Sarvi, S., Mehrzadi, S., Ahmadpour, E., and Daryani, A. (2017). A systematic review of in vitro and in vivo activities of anti-Toxoplasma drugs and compounds (2006-2016). Front. Microbiol. 8:25. doi: 10.3389/fmicb.2017.00025
Montoya, J. G., and Liesenfeld, O. (2004). Toxoplasmosis. Lancet. 363, 1965–1976. doi: 10.1016/S0140-6736(04)16412-X
Paccoud, O., Guitard, J., Labopin, M., Surgers, L., Malard, F., Battipaglia, G., et al. (2019). Features of Toxoplasma gondii reactivation after allogeneic hematopoietic stem-cell transplantation in a high seroprevalence setting. Bone. Marrow. Transplant. 55, 93–99. doi: 10.1038/s41409-019-0641-y
Pentewar, R., Shaikh, N., Bhalerao, A., Bharti, R., and Pulgamwar, V. (2015). Solubility enhancement technique for an anti-malarial drug. Der. Pharm. Sinica 6, 36–54.
Pick, J., Olson, L. L., Ellis, W. Y., and Lim, P. (1997). Development and validation of a method to extract and quantitate paromomycin and gentamicin from an Aquaphilic cream formulation. J. Pharm. Biomed. Anal. 16, 131–137. doi: 10.1016/S0731-7085(96)02044-4
Robert-Gangneux, F., and Darde, M. L. (2012). Epidemiology of and diagnostic strategies for toxoplasmosis. Clin. Microbiol. Rev. 25, 264–296. doi: 10.1128/CMR.05013-11
Rostami, A., Karanis, P., and Fallahi, S. (2018). Advances in serological, imaging techniques and molecular diagnosis of Toxoplasma gondii infection. Infection 46, 303–315. doi: 10.1007/s15010-017-1111-3
Schmidt-Hieber, M., Engelhard, D., Ullmann, A., Ljungman, P., Maertens, J., Martino, R., et al. (2019). Central nervous system disorders after hematopoietic stem cell transplantation: a prospective study of the infectious diseases working party of EBMT. J. Neurol. 267, 430–439. doi: 10.1007/s00415-019-09578-5
Schubert, M. A., and Muller-Goymann, C. C. (2003). Solvent injection as a new approach for manufacturing lipid nanoparticles–evaluation of the method and process parameters. Eur. J. Pharm. Biopharm. 55, 125–131. doi: 10.1016/S0939-6411(02)00130-3
Teimouri, A., Azami, S. J., Keshavarz, H., Esmaeili, F., Alimi, R., Mavi, S. A., et al. (2018). Anti-Toxoplasma activity of various molecular weights and concentrations of chitosan nanoparticles on tachyzoites of RH strain. Int. J. Nanomed. 13, 1341–1351. doi: 10.2147/IJN.S158736
Vijaykumar, B. R., Kant, R. S., Rajendran, C., Lekshmi, S. U., Keerthana, S., Mahadevan, A., et al. (2018). Restriction fragment length polymorphism-based genotyping of Toxoplasma gondii from autopsy-proven cases of acquired immunodeficiency syndrome-associated cerebral toxoplasmosis. Ann. Indian Acad. Neurol. 21, 250–255. doi: 10.4103/aian.AIAN_358_17
Keywords: Toxoplasma gondii, acute toxoplasmosis, paromomycin, mannosylation, solid lipid nanoparticles
Citation: Khosravi M, Mohammad Rahimi H, Doroud D, Mirsamadi ES, Mirjalali H and Zali MR (2020) In vitro Evaluation of Mannosylated Paromomycin-Loaded Solid Lipid Nanoparticles on Acute Toxoplasmosis. Front. Cell. Infect. Microbiol. 10:33. doi: 10.3389/fcimb.2020.00033
Received: 23 November 2019; Accepted: 17 January 2020;
Published: 13 February 2020.
Edited by:
Ehsan Ahmadpour, Tabriz University of Medical Sciences, IranReviewed by:
Wanderley De Souza, Federal University of Rio de Janeiro, BrazilMaria M. Corvi, National Council for Scientific and Technical Research (CONICET), Argentina
Copyright © 2020 Khosravi, Mohammad Rahimi, Doroud, Mirsamadi, Mirjalali and Zali. This is an open-access article distributed under the terms of the Creative Commons Attribution License (CC BY). The use, distribution or reproduction in other forums is permitted, provided the original author(s) and the copyright owner(s) are credited and that the original publication in this journal is cited, in accordance with accepted academic practice. No use, distribution or reproduction is permitted which does not comply with these terms.
*Correspondence: Hamed Mirjalali, aGFtZWRfbWlyamFsYWxpQGhvdG1haWwuY29t; aGFtZWRtaXJqYWxhbGlAc2JtdS5hYy5pcg==