Extracellular Vesicles Could Carry an Evolutionary Footprint in Interkingdom Communication
- 1Center of Cellular and Molecular Biology of Diseases, Instituto de Investigaciones Cientificas y Servicios de Alta Tecnologia (INDICASAT AIP), Panama, Panama
- 2Department of Biotechnology, Acharya Nagarjuna University, Guntur, India
- 3Department of Biology, University of Massachusetts, Boston, MA, United States
Extracellular vesicles (EVs) are minute particles secreted by the cells of living organisms. Although the functional role of EVs is not yet clear, recent work has highlighted their role in intercellular communication. Here, we expand on this view by suggesting that EVs can also mediate communication among interacting organisms such as hosts, pathogens and vectors. This inter-kingdom communication via EVs is likely to have important evolutionary consequences ranging from adaptation of parasites to specialized niches in the host, to host resistance and evolution and maintenance of parasite virulence and transmissibility. A potential system to explore these consequences is the interaction among the human host, the mosquito vector and Plasmodium parasite involved in the malaria disease. Indeed, recent studies have found that EVs derived from Plasmodium infected red blood cells in humans are likely mediating the parasite's transition from the asexual to sexual stage, which might facilitate transmission to the mosquito vector. However, more work is needed to establish the adaptive consequences of this EV signaling among different taxa. We suggest that an integrative molecular approach, including a comparative phylogenetic analysis of the molecules (e.g., proteins and nucleic acids) derived from the EVs of interacting organisms (and their closely-related species) in the malaria system will prove useful for understanding interkingdom communication. Such analyses will also shed light on the evolution and persistence of host, parasite and vector interactions, with implications for the control of vector borne infectious diseases.
Introduction
The evolution of cell communication is crucial in determining how organisms respond to and interact with each other and their environment. Protozoan parasites also have the ability of releasing membrane–enclosed vesicles toward their extracellular space. These small bodies are collectively known as “extracellular vesicles” (EVs) and can vary in size, composition and origin (Lener et al., 2015). EVs can function as signal carriers for single cell-cell communication or even more complex interactions at the level of tissues and organs (Kim et al., 2015). In this review, we present an overview of what is currently known about these extracellular bodies, the findings reported on protozoarian communication through EVs, their role in pathogenesis, program cell death, communication, and interkingdom coevolution.
Historical Perspective
Despite the use of EV terminology for more than 30 years, starting with the description of exosomes from reticulocytes (Harding et al., 1984), one of the first reports of what would be later referred to as EVs came from the activity of “thromboplastic proteins of the blood” (1946) (Chargaff and West, 1946). These proteins were composed of nanometric vesicles, and were later denominated “platelet dust” (1967) (Wolf, 1967). Other studies were also performed to investigate the activity of cartilage matrix vesicles in bone mineralization in 1969 (Anderson, 1969). Not long after, in 1971, small vesicles originating from RBCs infected with P. falciparum were observed using electron microscopy (Luse and Miller, 1971). Later on, studies on the regulatory capacity of EVs from T cells on the immune response triggered a renewed interest due to the potential therapeutic uses of EVs (Raposo et al., 1996). Important advances in the understanding of the role of EVs began to emerge with the identification of key functions such as their role in horizontal genetic transfer, modulation of the immune response, and cell differentiation. This multifunctional activity of EVs stems from their capacity to transport a wide range of distinct biomolecules that can alter the biological functions of target cells (Kalra et al., 2016). Currently, the application of high throughput sequencing tools enables the acquisition of massive data on EV populations from a diverse source of cellular and tissue models, offering new opportunities for the development of novel applications.
EVs have been grouped in a variety of ways (van der Pol et al., 2012; Akers et al., 2013; Raposo and Stoorvogel, 2013; Yanez-Mo et al., 2015; Szatanek et al., 2017). Briefly, EVs originate from cell sacs that are essentially comprised of thousands of different proteins and unique lipids, and which contain not only DNA and mRNA but also small nucleolar RNA (snRNA), Y RNA, mitochondrial RNA, vault RNA and long ncRNA (non-coding RNA) (Lazaro-Ibanez et al., 2014; Kreimer et al., 2015; van Balkom et al., 2015). Thus, EVs have been classified into exosomes, microvesicles and apoptotic bodies, depending on their origin, size and molecular composition. For instance, exosomes range in size from 50 to 150 nm. They are produced by invagination of the endosomal membrane during maturation of multivesicular bodies, and are released outside the cell after fusion with the plasma membrane (Keller et al., 2006; van Niel et al., 2018). Exosome formation is associated with specific proteins located in the endosome, such as tetraspanins, chaperones, and the Rab GTPase family (Ostrowski et al., 2010). A primary component of exosomes is the endosomal sorting complex required for transport (ESCRT), which is involved in the formation of exosomes in the late endosome and in the transportation of cargo (Raposo and Stoorvogel, 2013).
On the other hand, micro vesicles (MVs) are produced after budding directly from the plasma membrane. They have often been referred to in the literature with different names, such as ectosomes, microparticles, or shedding vesicles (Meldolesi, 2018). MVs are formed in cytosolic microdomains produced by the redistribution of phospholipids of the interior side of the plasma membrane, and then released to the extracellular space after vesicle fission (Cocucci and Meldolesi, 2015). MVs have sizes that range from 0.1 to 1 μm, which overlaps with the reported size of exosomes. This indicates that size is not a reliable criterion to differentiate between EVs. In living cells, the redistribution of lipids is facilitated by translocases that allow the movement of phospholipids in both directions across the plasma membrane, such as phophatidyl serine, which induces membrane budding and generation of MVs (Leventis and Grinstein, 2010; van der Heyde et al., 2011; Mantel and Marti, 2014). Additionally, other changes in the endosome and the plasma membrane are involved in the production of MVs, such as overexpression of GTP-binding ARF factor 6 (ADP-ribosylation factor 6), the formation of the complex VPS ATPse E3 ligase, and the interaction of the tumor susceptibility gene 101 (TSG101) with arrestin domain-containing protein 1 (ARRDC1). These modifications produce contractions in the cytoskeletal arrangement and the interaction with phospholipases result in the release of MVs (Muralidharan-Chari et al., 2009; Nabhan et al., 2012).
Finally, apoptotic bodies are released only when apoptosis is triggered in a healthy cell, beginning with chromatin condensation and blebbing of the membrane, followed by proteomic degradation and releasing of apoptotic bodies to the extracellular space (Elmore, 2007). Apoptotic bodies have a larger size in comparison with other types of EVs, ranging from 50 to 5000 μm (Nawaz et al., 2014). Smaller vesicles can be embedded within apoptotic bodies, and may enclose organelles as well as fragmented nuclei, a feature that uniquely separates these particles from other types of EVs. It is still unknown how these smaller vesicles are formed (Bergsmedh et al., 2001; Akers et al., 2013), but the blebbing mechanism of apoptotic bodies has been associated with actin-myosin interaction (Coleman et al., 2001).
EV Characteristics During Pathogen Infection
Intracellular and free-living pathogen-derived EVs carry signals that may mediate pathogen-pathogen communication. These signals modulate host biological functions, and induce the production of EVs by host effector cells that have been stimulated by direct contact with the pathogens or by contact with their EVs (Mantel and Marti, 2014). Special attention has been paid to the EVs of intracellular pathogens because this type of pathogen can modify the molecular composition of EVs, changing their function upon release from an infected host cell. For example, macrophages are induced to release inflammatory cytokines after taking up exosomes that were released from other macrophages infected with Mycobacterium avium (Bhatnagar and Schorey, 2007). EVs from a wide variety of other pathogens have important activity during infections, such as those produced by Giardia, Chlamydia, Trichomonas, and Cryptococcus (Benchimol, 2004; Rodrigues et al., 2008; Frohlich et al., 2012; Twu et al., 2013). Similarly, intracellular protozoan parasites also have an impact on the host, by altering its immune response. For instance, EVs derived from Toxoplasma gondii are known to induce murine macrophages to release inflammatory proteins such as TNF-α, iNOS, and IL-10 (Silva et al., 2018).
EVs in Trypanosomatids
In infections caused by protozoan parasites, the release of EVs can be observed throughout most of the parasite's life cycle, suggesting that EVs are a fundamental component of parasitic infection. As previously reported, EVs are involved in the host–parasite interaction and in communication between parasites, inducing dysfunction in the immune responses or manipulating the physiology and metabolism of the host (Silvester et al., 2017). EVs have been identified in some of the most pathogenic protozoans, those responsible for some of the most widespread, lethal and disabling vector–borne diseases, such as Chagas disease, African trypanosomiasis, and leishmaniasis. Their causative parasites have a complex life cycle, undergoing several transformations during their asexual and sexual stages. As explained below, new findings have determined that EVs play a role in a variety of infective processes, including the immune response of the hosts. The principal findings on the parasite-derived EVs from these major vector–borne diseases are summarized below.
Trypanosoma cruzi, the etiological agent of Chagas disease, infects macrophages during its trypomastigote stage and can generate both exosomes and ectosomes during infection (de Pablos Torro et al., 2018). The parasite then invades new cells and tissues after cellular differentiation and replication. It has been shown that EVs are present in almost all stages of infection (Bayer-Santos et al., 2013). In addition, the modulating activity of EVs is determined by the phase of the disease (acute or chronic), the immune status of patients and the parasite load during infection. During T. cruzi infection, EVs can be released from parasites or host cells and can be taken up by both parasites and host cells. For instance, Ramirez et al. (2017) showed that the integration rate of EVs with THP1 monocyte cells is significantly higher upon release from human tissue–cultured trypomastigotes than from insect stage epimastigotes or metacyclic trypomastigotes. If these infected monocyte-derived EVs fuse with other trypomastigotes, they can offer them protection against lysis from the complement system action (Cestari et al., 2012; Ramirez et al., 2017). Likely, this protection originates after trypomastigotes and immune complexes interact with each other through their EVs (Diaz Lozano et al., 2017). The increased incorporation of EVs through a Ca2+-dependent mechanism, in comparison with other stages, is caused by a higher rate of redistribution of phosphatidylserine (PS) in THP-1 monocytes upon interaction with tissue-culture trypomastigotes (Ramirez et al., 2017). Therefore, similar to other cell models, the modulation of the immune response using EV-cargo signals relies on the key role played by PS in facilitating the uptake of EVs from infected host cells. Nevertheless, more studies are required to describe the precise signal cascades involved in the Ca2+ efflux and the release of EVs during T. cruzi infection. Interestingly, T. cruzi proteins are found inside host cells after their incorporation of T. cruzi EVs, and this insertion appears to be targeted at specific cells types such as fibroblasts, muscle and neuronal cells. In fact, parasite proteins have not been detected in other types of cells such as lymphocytes or erythrocytes (de Pablos Torro et al., 2018). EVs fusion with THP1 cells produce a higher expression of the genes IKBKB, NR3C1, and TIRAP, which have been associated with an increased generation of reactive oxygen species (ROS) and nitric oxide (NO) (Chowdhury et al., 2017). Additional evidence of the role of EVs in the pathogenicity of trypanosomatids was offered by Trocoli Torrecilhas et al. (2009) who demonstrated that the parasitaemia in BALB/C mice increased following the inoculation of T. cruzi EVs prior to infection. Notably, proteomic analysis of EVs from trypomastigotes (host stage) and epimastigotes (vector stage) of T. cruzi revealed that most (70%) of the identified EV proteins are present in both stages.
In a similar scenario, EVs play an important role during infection by Leishmania parasites, which are transmitted to humans by sandfly bites. Most of the research on EVs from Leishmania species has been performed using L. major and L. donovani, which have different clinical manifestations, with the former developing in the skin and the latter, viscerally. Following the transformation of promastigotes to amastigotes inside macrophages in in vitro studies, it has been shown that EVs may be released to the supernatant and present virulence factors such as zinc-metalloproteinase gp63 (Silverman et al., 2008). This protein activates the tyrosine phosphatase SHP-1, which has an inhibitory effect on the pro-inflammatory cytokine response activity of macrophages after inhibiting the host IFN-γ/Jak-STAT1 cascade and the p38-MAPK signaling pathway (Gomez et al., 2009; Mantel and Marti, 2014).
In hepatic cells, protein gp63 also inhibits the enzyme Dicer1, causing the downregulation of the miRNA miR-122, which provokes a change in the lipid metabolism of the host, contributing to the survival of the parasite (Descoteaux et al., 2013). In addition, gp63 participates in the activation of signaling proteins and transcription factors that might be involved in the up/down regulation of several genes in the target macrophage (Hassani and Olivier, 2013). It is not clear yet, however, whether this gp63 action is mediated by EV integration into hepatic cells (Ghosh et al., 2011). On the other hand, EVs from promastigotes have been shown to modify the immune response by decreasing the production of IL-8 and TNF-α, increasing IL-10 and eliminating the dendritic cell capacity to activate Th1 cells (Silverman et al., 2010). In a recent report, EVs were associated with the increased production of cytokines in macrophages and B1 cells as well as with several virulence factors in L. amazonensis (Barbosa et al., 2018). What is clear in infections by Leishmania parasites is that the EVs from both intracellular and free-living parasites are modulating the immune response of the human host.
EVs in Malaria Pathogenesis
An early report on malaria EVs focused on their capacity to induce thrombocytopenia, which is associated with cerebral malaria (CM) (Piguet et al., 2002). Later, it was shown that P. falciparum EVs were also present in the sera of infected patients, establishing the occurrence of increased level of EVs in patients ridden with this species of Plasmodium (especially associated with coma cases in CM) in comparison with P. malariae and P. ovale infections (Combes et al., 2004; Pankoui Mfonkeu et al., 2010). In the case of P. vivax infections, patients also show elevated levels of EVs derived from erythrocytes, platelets, leucocytes and monocytes, relative to healthy individuals (Campos et al., 2010). Proteomic analyses of circulating EVs in infected patients have identified proteins related to parasite metabolism, invasion and pathogenesis, such as enolase, Hsp 90, lactate dehydrogenase, ADP-ribosylation factor 1, phosphoglycerate kinase and merozoite surface protein 1 (Antwi-Baffour et al., 2016).
Animal models have also served to study the relationship between EVs and malaria pathogenesis and to gain insights into the corresponding host immune response. For example, the high levels of iRBC (infected red blood cell)-derived EVs during CM were reduced after inhibition of the ATP-binding cassette transporter ABCA1 gene, which is a known mediator of MV formation. Specifically, this ABCA1 inhibition reduced TNF-αlevels in sera and cell sequestration of leukocytes into the brain, both features of complicated malaria (Combes et al., 2005). Remarkably, it has been shown that the particular haplotypes of the ABCA1 promoter determine the levels of EV secretion during infections, thus increasing or decreasing the virulence of the parasite (Sahu et al., 2013). Mice models have been used to study the immune response, particularly the inflammatory processes related to macrophage activation in a TLR–dependent manner after P. berghei-derived EVs were inoculated into the animals (Couper et al., 2010). Recently, Shrivastava et al. (2017) reported that RBC-derived EVs could be internalized massively by astrocytes along with high levels of CXCL10, a known marker of CM, in P. berghei–infected mice (Shrivastava et al., 2017).
On the other hand, after hand in vitro studies of P. falciparum have also informed the biological function of EVs. For instance, in a model of CM, platelet–derived EVs were associated with the transference of platelet antigens into iRBCs using a PfEMP1-dependent pathway, which resulted in an increase in the binding of infected cells with the endothelium (Faille et al., 2009). In 2013, two independent studies found that P. falciparum uses EVs for parasite-parasite and host-parasite communication (Mantel et al., 2013; Regev-Rudzki et al., 2013). These studies elegantly demonstrated several PfEV activities, such as activation of neutrophils, secretion of both pro- and anti-inflammatory cytokines following EV uptake by macrophages, induction of gametocytogenesis, and incorporation of DNA plasmids, which can spread drug resistance among naïve parasites. In addition, MV markers stomatin, GTP-binding protein ARF6 and the disassembly factor VPS4 were identified as cargo of the EVs using proteomic analysis (Mantel et al., 2013). In the context of these findings, the evidence suggested that EVs could be used as indicators during P. falciparum infections to estimate parasite density, serve as signals in parasite–parasite communication, and regulate the period of transmissibility to vectors (Mantel and Marti, 2014). Interestingly, recent proteomic analysis identified several proteins that may participate in the invasion of RBCs using molecular mimicry of the host molecules. Particularly, the ring–exported protein 2 (Rex2) and the Plasmodium-exported element (PEXEL) present a high molecular similarity to the human RAC2 protein family (Barteneva et al., 2013). Recently, it was also shown that human NK cells activated by iRBC–derived EVs respond by exposing exposed MDA5, a pathogen recognition receptor (Ye et al., 2018).
The origin of P. falciparum EVs has been described in asexual stages. Maurer's clefts are important erythrocytic structures formed after invasion by the parasite, participating in protein trafficking and vesicle formation directed at the erythrocyte plasma membrane (Spycher et al., 2006). This was demonstrated when Maurer's cleft proteins were reported as components of released EVs during P. falciparum in vitro culture (Mantel et al., 2013). The molecular deletion of Maurer's cleft proteins inhibited the production of EVs, confirming the role of this host-parasite structure in vesicle biogenesis (Regev-Rudzki et al., 2013). Further findings confirmed the participation of the EV cargo in host immune modulation, host vascular function manipulation, gametocytogenesis and gene regulatory functions through small RNAs, DNA, and specific interacting components associated with severe malaria (Mantel et al., 2016; Sisquella et al., 2017; Babatunde et al., 2018). Altogether, these findings revealed that malaria parasites use EVs as an effective communication system that act like cellular “carrier pigeons” to regulate multiple functions of both the parasite population and host homeostasis.
Individual Suicide or Collective Homeostasis? the Role Of EVs
Traditionally, programed cell death (PCD) is defined as a genetically regulated mechanism that is used by multicellular organisms for homeostasis and development (Gunjan et al., 2016). However, in recent years, there has been increasing interest in death regulation in protozoan parasites, generating debate around the traditional classification of programed cell death (PCD) pathways (Proto et al., 2013). In fact, new evidence shows that intracellular parasites can suffer apoptosis (Li et al., 2015; Mandal et al., 2016; de Castro et al., 2017; Gunjan et al., 2018), indicating that PCD is not unique to multicellular organisms. Thus, understanding of the PCD mechanisms that take place during microbial infections is an exciting field of study, given that death signals might be used in therapeutic treatments. For example, Plasmodium, Trypanosoma, and Leishmania parasites present PCD features under distinct experimental conditions (Duszenko et al., 2006; Gunjan et al., 2016; Mandal et al., 2016; Basmaciyan et al., 2017; de Castro et al., 2017; Wei et al., 2018).
Similar to multicellular organisms, these parasites present classic PCD markers, such as DNA fragmentation, cell shrinkage, chromatin condensation, PS translocation and loss of the mitochondrial membrane potential (Reece et al., 2011). Furthermore, several effectors have been suggested to induce apoptosis in protozoan parasites. For example, prostaglandin D2 is involved in PCD in Trypanosoma brucei procyclic forms, by generating ROS (Duszenko et al., 2006). The description of the gene metacaspases 1 (PfMCA1) and the use of pan caspase inhibitor z-VAD-fmk confirmed the presence of PCD in P. falciparum and in P. vivax (Meslin et al., 2007; Rezanezhad et al., 2011). In addition, it has been shown that PfMCA1 is regulated by the C2 domain of the calcium-dependent membrane targeting domain and a CARD domain (Caspase Recruitment Domain) (Sow et al., 2015). In Leishmania the role of MCA has been identified as being a precursor of PCD, acting on the autophagic protein ATG8 (Casanova et al., 2015). The above evidence makes it clear that protozoan parasites do indeed have PCD mechanisms, albeit distinct from those of multicellular organisms.
Remarkably, EVs can also induce apoptosis during stress and normal development conditions by carrying Fas and TRAIL to different target cells (Janiszewski et al., 2004; Stenqvist et al., 2013). Recently, our group reported that parasite-derived EVs carrying lactate dehydrogenase can induce PCD in in vitro cultures of P. falciparum during high parasitaemia levels, showing that parasites can likely sense each other and release EVs to regulate the parasite population (Correa et al., 2019). If apoptosis induction involves the pathogen communicating with its kind through EVs, as the evidence suggests, this would represent an important evolutionary force in the adaptation of parasitism to specialized niches in the host. EVs could be involved in the communication strategies used by the parasite to manipulate its hosts and vector in order to maintain its virulence, survival and transmissibility. There is increasing evidence from various fields and approaches suggesting that the study of EVs might help understand the complexity of mammalian host-parasite-vector interactions in protozoan pathogens. We suggest that an integral molecular analysis of EVs could provide a genetic marker(s) that would help answer certain mysteries present in the study of the evolution of interkingdom communication and its role in the adaptation of parasite species and that P. falciparum could be a good model to test this hypothesis.
In the case of the malaria parasite, it has been suggested that a mechanism of self-regulation may exist to limit the intensity of the parasitaemia in in vitro cultures. Interestingly, in those conditions, the parasitaemia growth of P. falciparum can reach a maximum multiplying factor of 8, which is far below a potential factor of 16 (Deponte and Becker, 2004) under ideal conditions. Based on this behavior, it had been suggested that P. falciparum uses mechanisms of self-regulation in response to density stress (Mutai and Waitumbi, 2010), and these mechanisms might depend on EVs mediated apoptosis. For instance, recent studies have observed programmed cell death in highly parasitized in vitro cultures of Plasmodium, and another study that has identified molecules involved in the signaling of death (Totino et al., 2014; Engelbrecht and Coetzer, 2016; Chou et al., 2017; Correa et al., 2019).
The Possible Role of EVs in Evolution by Horizontal Gene Transfer
The basic biochemical composition of EVs includes a phospholipid membrane that forms a sac and can contain conserved proteins, such as enzymes, growth factors, different types of receptors and cytokines, as well as DNA, coding and non-coding RNA and several metabolites (Raposo and Stoorvogel, 2013). Since their discovery, EVs derived from red blood cells infected with P. falciparum generated interest regarding their potential role in cell signaling, intra-regulation and communication between host and pathogens (Mutai and Waitumbi, 2010; Proto et al., 2013). Recently, Sundararaman et al. (2016) suggested that EVs could play a role in DNA recombination in the asexual stage of the infection inside the erythrocytes. This indicates that the study of EVs in P. falciparum could provide an opportunity to understand host-parasite and parasite-parasite interactions. In particular, its ability to grow in laboratory cultures and its specialized life cycle confined to mosquitoes and humans, makes P. falciparum an optimal model system for the study of the evolution of inter-kingdom communication (Figure 1).
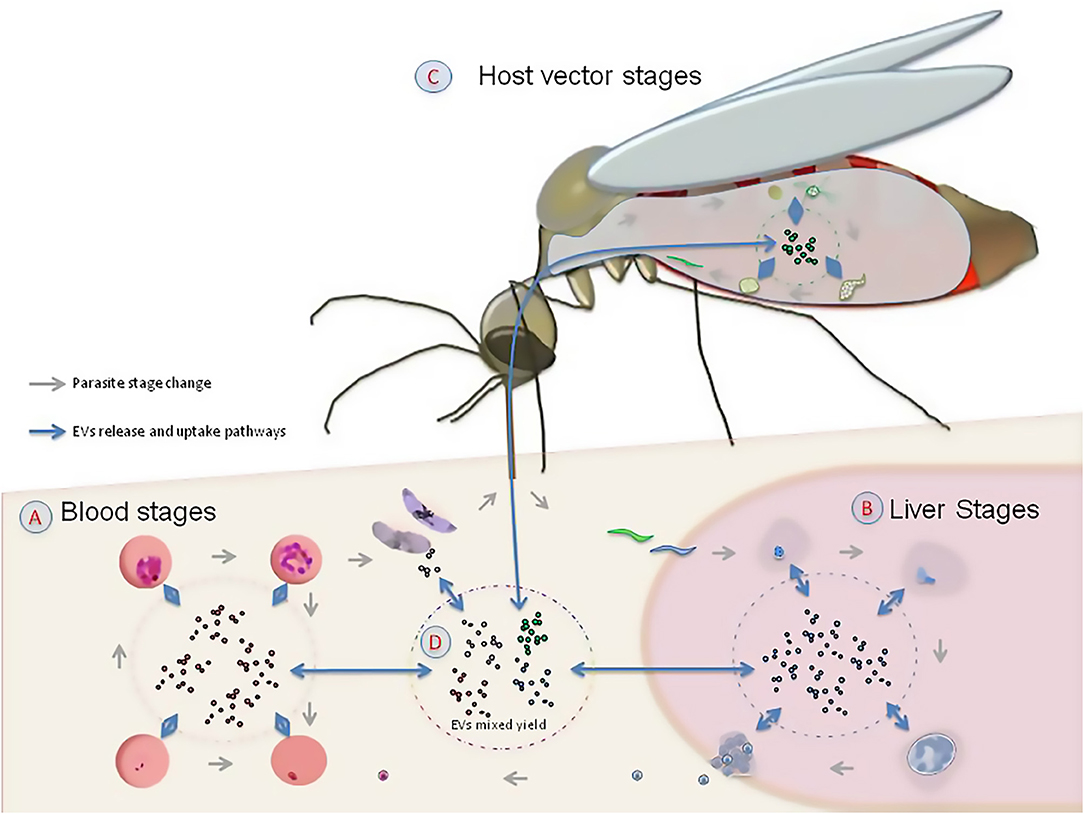
Figure 1. The role of extracellular vesicles (EVs) in the life cycle of Plasmodium falciparum. Given that EVs are produced by the parasite and the hosts in the human erythrocyte (A), the liver (B), and the mosquito vector (C), they are likely to play a signaling role in the interaction among host, parasite and vector (D).
A series of molecules derived from EVs have been involved in cell signaling and intra-regulation (Mutai and Waitumbi, 2010; Proto et al., 2013), including a variety of proteins and genetic material, and, potentially, transposons (Lefebvre et al., 2016; Preusser et al., 2018), all of which are known to mediate communication between host and pathogens (Schorey et al., 2015). For instance, recent studies on P. falciparum show that EVs carry proteins and nucleic acids potentially involved in the horizontal transfer of information parasite↔parasite and/or parasite→host (Mantel et al., 2013, 2016; Regev-Rudzki et al., 2013). In addition, the release of EVs from iRBCs has been involved in signaling the transition of the parasite from the asexual to sexual stage as well as its sequestration in micro-capillaries, both of which are transitions that would favor vector transmission (Aingaran et al., 2012; Cornet et al., 2014).
Interkingdom communication via EVs could also play a role in coevolution of interacting organisms. However, the coevolution of cell signaling between different species, such as host-pathogen interactions or mixed infection by related pathogens has not been considered so far. Mixed infections of multiple parasites within a host are common in nature (Krishna et al., 2015; Bernotiene et al., 2016; Graystock et al., 2016). For instance, great apes, and even humans, can host several species of Plasmodia parasites (Prugnolle et al., 2010). EVs could mediate communication by facilitating horizontal transfer of genetic information between different Plasmodia species (Regev-Rudzki et al., 2013; Kawamura et al., 2017; Otto et al., 2018; Ben-Hur et al., 2019; Plenderleith et al., 2019). Several lines of evidence support this possibility. Indeed, the frequency of EVs found in pathogens suggests that EVs are common in interactions between host and parasites, and are likely to modify the biological functions of the host (Mantel et al., 2016; Zhu et al., 2016). In addition, molecules derived from EVs associated with host-pathogen interactions are likely to show a footprint of the reciprocal selection between host and pathogens (Kawamura et al., 2017).
Evolutionary Consequences of Interkingdom Communication
The evolution of interkingdom communication could have several consequences. For example, information transfer in a host with mixed infections (Mantel and Marti, 2014) could facilitate the stable co-existence of multiple pathogen species. This is likely the case in mixed infections of Plasmodia spp. in apes, which could be less virulent than a solo P. falciparum infection in modern humans, as reported in van Niel et al. (2018). However, it is not yet clear how universal mild infection in apes hosting mixed infections of Plasmodia spp. is. Similar to host and parasites, EVs could also facilitate signaling between the Plasmodium parasite and the mosquito vector (Deolindo et al., 2013; Twu et al., 2013; Bayer-Santos et al., 2014; Montaner et al., 2014). This information exchange is likely to have played a key role in the initial approach between host and parasite that ended up in the establishment of the infection (Figure 1D). However, an integrative examination of EVs derived from infected host, parasite and vector is necessary to shed light on the origins of P. falciparum and the development of malaria pathogenesis.
Testing for the Role of EVs
To test for the role of EVs in the evolution of host-parasite interaction, a deeper analysis of the properties and molecular composition of EVs is necessary. Proteins derived from EVs have already been suggested to mediate communication between host and pathogens, especially in intracellular microorganisms such as Trypanosoma spp. (Matthews, 2005; Garcia-Silva et al., 2014), Leishmania spp. (Silverman and Reiner, 2011) and Plasmodium spp. (Martin-Jaular et al., 2011; Regev-Rudzki et al., 2013). In Plasmodium spp., an important number of the molecules have recently been identified (Batista et al., 2011; Correa et al., 2017). These molecules include a wide range of proteins that are involved in metabolism and immune processes. Sequence variation in these EV proteins and their interacting counterpart in hosts could be examined across tissues infected by the parasite, such as host erythrocytes and mosquito gut lining and salivary glands. Sequence data from these proteins, as well as nucleic acids (e.g., DNA, RNAs) derived from EVs, can be used to infer parallel changes associated with coevolution between parasites and hosts. Some ideas are given in Table 1. Additional analyses of these EV molecules could explore patterns of selection acting on aminoacid (or nucleotide) sequence in hosts and parasite. These analyses could be performed using cutting-edge sequencing tools in combination with readily available comparative phylogenetic methods such as parsimony, maximum likelihood, and Bayesian inference (Yang and Bielawski, 2000). From a phylogenetic perspective, comparative analyses could include EV molecules derived from host, parasite and vectors, as well as their closely-related species. These analyses could also reveal whether the communication mechanism via EVs represents a conserved strategy across plasmodia-insect-mammalian species. Finding a conserved communication strategy via EVs could explain the unusual ability of New World mosquitoes to transmit the Plasmodium parasite, even though American mosquitoes diverged from their African counterparts 95 million years ago (Molina-Cruz and Barillas-Mury, 2014).
The analyses suggested in Table 1 might exploit whole-genome sequence tools that have been used recently to study the coevolution of host-parasite interactions. In the absence of known rare genomic changes or other idiosyncratic markers, whole-genome analyses provide a useful tool to circumvent studies with single or low number of markers (Froeschke and von der Heyden, 2014). One example is the use of linkage mapping to perform genetic crosses, useful for localizing phenotypic traits in a range of organisms. Linkage mapping has been applied to P. falciparum to identify key genes related to drug resistance and cell development (Ranford-Cartwright and Mwangi, 2012). This method has made it possible to map genes, causing a given phenotype, to genome regions and to find the responsible genes and/or mutations by performing functional genomics experiments (Anderson et al., 2018). Additionally, there are a number of next-generation sequencing tools that can be used to explore the components of the EV cargo as well as the evolutionary history of host-parasite interaction mediated by EVs. This includes parallel sequencing of known (and novel) peptides and nucleic acids derived from the EVs host and parasite. These analyses can also be targeted to specific cell types (e.g., via single cell parallel sequencing), which could facilitate the functional characterization of host and parasite EVs in vitro (Turchinovich et al., 2019).
To date, most studies have focused on the interaction between mammalian host and parasites. Nevertheless, the role of parasite EVs in vector attraction to infected cells has received less attention (Figure 1). The study of these interactions could help explain the observation that infected mosquitoes can be manipulated by Plasmodium in a way that enhances vector attraction to human odor (Lacroix et al., 2005; Smallegange et al., 2013; Batista et al., 2014). In this interaction, a parasite-driven regulation of vector genes (Lu et al., 2007) might activate the receptors used by mosquitoes to detect hosts (Takken and Knols, 1999). EVs could mediate the attraction to the infected hosts by influencing both gene regulation and activation of other signals during infection (reviewed in Barteneva et al., 2013; Mantel and Marti, 2014). While understanding these signaling pathways is challenging because of the inherent difficulties of studying intracellular pathogens, the specialized nature of the P. falciparum life cycle presents a unique opportunity to explore this issue.
A number of reports that suggest coevolution between microbes and hosts can be found in literature (Chapman and Hill, 2012; Mozzi et al., 2018; Quintana-Murci, 2019). The importance of polymicrobial interactions in this occurrence has also been presented (Rioux et al., 2007). Thus, the possible role of EVs on these events would only add another piece to explain coevolution.
Conclusions
The functional role of EVs has been associated with intercellular communication. However, recent evidence indicates that EVs are also ubiquitous in the interaction among mammalian hosts, parasites and vectors. This suggests that EVs play an important role in interkingdom communication. Thus, communication through EVs is likely to have important evolutionary consequences such as the adaptation of parasitism to specialized niches in the host. EVs could also be involved in the communication strategies used by the parasite to take advantage of its mammalian host and its vector to maintain its virulence, survival and transmissibility. We suggest that the Plasmodium parasite involved in the malaria disease represents a potential model system to explore the role of EVs in the evolution of host-parasite interaction and interkingdom communication. Deciphering the cargo composition of EVs derived from parasites, hosts, and vectors will also inform the evolution of the pathogenicity of malaria and other protozoan parasites.
Author Contributions
RC conceived the idea and drafted the initial manuscript. ZC and LD wrote sections of the manuscript. RC, ZC, and CS reviewed and worked to produce the final concepts. CS put together the final draft. All authors read, revised, and approved the final manuscript before submission.
Funding
CS, ZC, and RC were partially funded by the National System of Research (SNI Panama).
Conflict of Interest
The authors declare that the research was conducted in the absence of any commercial or financial relationships that could be construed as a potential conflict of interest.
Acknowledgments
The authors would like to thank Ernst Hempelmann for critically reviewing the manuscript.
References
Aingaran, M., Zhang, R., Law, S. K., Peng, Z., Undisz, A., Meyer, E., et al. (2012). Host cell deformability is linked to transmission in the human malaria parasite Plasmodium falciparum. Cell Microbiol. 14, 983–993. doi: 10.1111/j.1462-5822.2012.01786.x
Akers, J. C., Gonda, D., Kim, R., Carter, B. S., and Chen, C. C. (2013). Biogenesis of extracellular vesicles (EV): exosomes, microvesicles, retrovirus-like vesicles, and apoptotic bodies. J. Neurooncol. 113, 1–11. doi: 10.1007/s11060-013-1084-8
Anderson, H. C. (1969). Vesicles associated with calcification in the matrix of epiphyseal cartilage. J. Cell Biol. 41, 59–72. doi: 10.1083/jcb.41.1.59
Anderson, T. J. C., LoVerde, P. T., Le Clec'h, W., and Chevalier, F. D. (2018). Genetic crosses and linkage mapping in schistosome parasites. Trends Parasitol. 34, 982–996. doi: 10.1016/j.pt.2018.08.001
Antwi-Baffour, S., Adjei, J. K., Agyemang-Yeboah, F., Annani-Akollor, M., Kyeremeh, R., Asare, G. A., et al. (2016). Proteomic analysis of microparticles isolated from malaria positive blood samples. Proteome Sci. 15:5. doi: 10.1186/s12953-017-0113-5
Babatunde, K. A., Mbagwu, S., Hernandez-Castaneda, M. A., Adapa, S. R., Walch, M., Filgueira, L., et al. (2018). Malaria infected red blood cells release small regulatory RNAs through extracellular vesicles. Sci. Rep. 8:884. doi: 10.1038/s41598-018-19149-9
Barbosa, F. M. C., Dupin, T. V., Toledo, M. D. S., Reis, N., Ribeiro, K., Cronemberger-Andrade, A., et al. (2018). Extracellular vesicles released by leishmania (Leishmania) amazonensis promote disease progression and induce the production of different cytokines in macrophages and B-1 cells. Front. Microbiol. 9:3056. doi: 10.3389/fmicb.2018.03056
Barteneva, N. S., Maltsev, N., and Vorobjev, I. A. (2013). Microvesicles and intercellular communication in the context of parasitism. Front. Cell Infect. Microbiol. 3:49. doi: 10.3389/fcimb.2013.00049
Basmaciyan, L., Azas, N., and Casanova, M. (2017). Calcein+/PI- as an early apoptotic feature in Leishmania. PLoS ONE 12:e0187756. doi: 10.1371/journal.pone.0187756
Batista, B. S., Eng, W. S., Pilobello, K. T., Hendricks-Munoz, K. D., and Mahal, L. K. (2011). Identification of a conserved glycan signature for microvesicles. J. Proteome Res. 10, 4624–4633. doi: 10.1021/pr200434y
Batista, E. P., Costa, E. F., and Silva, A. A. (2014). Anopheles darlingi (Diptera: Culicidae) displays increased attractiveness to infected individuals with Plasmodium vivax gametocytes. Parasit. Vectors 7:251. doi: 10.1186/1756-3305-7-251
Bayer-Santos, E., Aguilar-Bonavides, C., Rodrigues, S. P., Cordero, E. M., Marques, A. F., Varela-Ramirez, A., et al. (2013). Proteomic analysis of Trypanosoma cruzi secretome: characterization of two populations of extracellular vesicles and soluble proteins. J. Proteome. Res. 12, 883–897. doi: 10.1021/pr300947g
Bayer-Santos, E., Lima, F. M., Ruiz, J. C., Almeida, I. C., and da Silveira, J. F. (2014). Characterization of the small RNA content of Trypanosoma cruzi extracellular vesicles. Mol. Biochem. Parasitol. 193, 71–74. doi: 10.1016/j.molbiopara.2014.02.004
Benchimol, M. (2004). The release of secretory vesicle in encysting Giardia lamblia. FEMS Microbiol. Lett. 235, 81–87. doi: 10.1111/j.1574-6968.2004.tb09570.x
Ben-Hur, S., Biton, M., and Regev-Rudzki, N. (2019). Extracellular vesicles: a prevalent tool for microbial gene delivery? Proteomics 19:e1800170. doi: 10.1002/pmic.201800170
Bergsmedh, A., Szeles, A., Henriksson, M., Bratt, A., Folkman, M. J., Spetz, A. L., et al. (2001). Horizontal transfer of oncogenes by uptake of apoptotic bodies. Proc. Natl. Acad. Sci. U.S.A. 98, 6407–6411. doi: 10.1073/pnas.101129998
Bernotiene, R., Palinauskas, V., Iezhova, T., Murauskaite, D., and Valkiunas, G. (2016). Avian haemosporidian parasites (Haemosporida): a comparative analysis of different polymerase chain reaction assays in detection of mixed infections. Exp. Parasitol. 163, 31–37. doi: 10.1016/j.exppara.2016.01.009
Bhatnagar, S., and Schorey, J. S. (2007). Exosomes released from infected macrophages contain Mycobacterium avium glycopeptidolipids and are proinflammatory. J. Biol. Chem. 282, 25779−25789. doi: 10.1074/jbc.M702277200
Campos, F. M., Franklin, B. S., Teixeira-Carvalho, A., Filho, A. L., de Paula, S. C., Fontes, C. J., et al. (2010). Augmented plasma microparticles during acute Plasmodium vivax infection. Malar. J. 9:327. doi: 10.1186/1475-2875-9-327
Casanova, M., Gonzalez, I. J., Sprissler, C., Zalila, H., Dacher, M., Basmaciyan, L., et al. (2015). Implication of different domains of the Leishmania major metacaspase in cell death and autophagy. Cell Death Dis. 6:e1933. doi: 10.1038/cddis.2015.288
Cestari, I., Ansa-Addo, E., Deolindo, P., Inal, J. M., and Ramirez, M. I. (2012). Trypanosoma cruzi immune evasion mediated by host cell-derived microvesicles. J. Immunol. 188, 1942–1952. doi: 10.4049/jimmunol.1102053
Chapman, S. J., and Hill, A. V. (2012). Human genetic susceptibility to infectious disease. Nat. Rev. Genet. 13,175–188. doi: 10.1038/nrg3114
Chargaff, E., and West, R. (1946). The biological significance of the thromboplastic protein of blood. J. Biol. Chem. 166, 189–197.
Chou, E. S., Abidi, S. Z., Teye, M., Leliwa-Sytek, A., Rask, T. S., Cobbold, S. A., et al. (2017). A high parasite density environment induces transcriptional changes and cell death in Plasmodium falciparum blood stages. FEBS J. 285, 848–870. doi: 10.1111/febs.14370
Chowdhury, I. H., Koo, S. J., Gupta, S., Liang, L. Y., Bahar, B., Silla, L., et al. (2017). Gene expression profiling and functional characterization of macrophages in response to circulatory microparticles produced during Trypanosoma cruzi infection and chagas disease. J. Innate. Immun. 9, 203–216. doi: 10.1159/000451055
Cocucci, E., and Meldolesi, J. (2015). Ectosomes and exosomes: shedding the confusion between extracellular vesicles. Trends Cell Biol. 25, 364–372. doi: 10.1016/j.tcb.2015.01.004
Coleman, M. L., Sahai, E. A., Yeo, M., Bosch, M., Dewar, A., and Olson, M. F. (2001). Membrane blebbing during apoptosis results from caspase-mediated activation of ROCK I. Nat. Cell. Biol. 3, 339–345. doi: 10.1038/35070009
Combes, V., Coltel, N., Alibert, M., van Eck, M., Raymond, C., Juhan-Vague, I., et al. (2005). ABCA1 gene deletion protects against cerebral malaria: potential pathogenic role of microparticles in neuropathology. Am. J. Pathol. 166, 295–302. doi: 10.1016/S0002-9440(10)62253-5
Combes, V., Taylor, T. E., Juhan-Vague, I., Mege, J. L., Mwenechanya, J., Tembo, M., et al. (2004). Circulating endothelial microparticles in malawian children with severe falciparum malaria complicated with coma. JAMA 291, 2542–2544. doi: 10.1001/jama.291.21.2542-b
Cornet, S., Nicot, A., Rivero, A., and Gandon, S. (2014). Evolution of plastic transmission strategies in avian malaria. PLoS Pathog. 10:e1004308. doi: 10.1371/journal.ppat.1004308
Correa, R., Coronado, L., Caballero, Z., Faral, P., Robello, C., and Spadafora, C. (2019). Extracellular vesicles carrying lactate dehydrogenase induce suicide in increased population density of Plasmodium falciparum in vitro. Sci. Rep. 9:5042. doi: 10.1038/s41598-019-41697-x
Correa, R., Coronado, L. M., Garrido, A. C., Durant-Archibold, A. A., and Spadafora, C. (2017). Volatile organic compounds associated with Plasmodium falciparum infection in vitro. Parasit. Vectors. 10:215. doi: 10.1186/s13071-017-2157-x
Couper, K. N., Barnes, T., Hafalla, J. C., Combes, V., Ryffel, B., Secher, T., et al. (2010). Parasite-derived plasma microparticles contribute significantly to malaria infection-induced inflammation through potent macrophage stimulation. PLoS Pathog. 6:e1000744. doi: 10.1371/journal.ppat.1000744
de Castro, E., Reus, T. L., de Aguiar, A. M., Avila, A. R., and de Arruda Campos Brasil de Souza, T. (2017). Procaspase-activating compound-1 induces apoptosis in Trypanosoma cruzi. Apoptosis 22, 1564–1577. doi: 10.1007/s10495-017-1428-5
de Pablos Torro, L. M., Retana Moreira, L., and Osuna, A. (2018). Extracellular vesicles in chagas disease: a new passenger for an old disease. Front. Microbiol. 9:1190. doi: 10.3389/fmicb.2018.01190
Deolindo, P., Evans-Osses, I., and Ramirez, M. I. (2013). Microvesicles and exosomes as vehicles between protozoan and host cell communication. Biochem. Soc. Trans. 41, 252–257. doi: 10.1042/BST20120217
Deponte, M., and Becker, K. (2004). Plasmodium falciparum—do killers commit suicide? Trends Parasitol. 20, 165–169. doi: 10.1016/j.pt.2004.01.012
Descoteaux, A., Moradin, N., and Arango Duque, G. (2013). Leishmania dices away cholesterol for survival. Cell Host Microbe. 13, 245–247. doi: 10.1016/j.chom.2013.02.018
Diaz Lozano, I. M., De Pablos, L. M., Longhi, S. A., Zago, M. P., Schijman, A. G., and Osuna, A. (2017). Immune complexes in chronic Chagas disease patients are formed by exovesicles from Trypanosoma cruzi carrying the conserved MASP N-terminal region. Sci. Rep. 7:44451. doi: 10.1038/srep44451
Duszenko, M., Figarella, K., Macleod, E. T., and Welburn, S. C. (2006). Death of a trypanosome: a selfish altruism. Trends Parasitol. 22, 536–542. doi: 10.1016/j.pt.2006.08.010
Elmore, S. (2007). Apoptosis: a review of programmed cell death. Toxicol. Pathol. 35, 495–516. doi: 10.1080/01926230701320337
Engelbrecht, D., and Coetzer, T. L. (2016). Plasmodium falciparum exhibits markers of regulated cell death at high population density in vitro. Parasitol. Int. 65(6 Pt A), 715–727. doi: 10.1016/j.parint.2016.07.007
Faille, D., Combes, V., Mitchell, A. J., Fontaine, A., Juhan-Vague, I., Alessi, M. C., et al. (2009). Platelet microparticles: a new player in malaria parasite cytoadherence to human brain endothelium. FASEB J. 23, 3449–3458. doi: 10.1096/fj.09-135822
Froeschke, G., and von der Heyden, S. (2014). A review of molecular approaches for investigating patterns of coevolution in marine host-parasite relationships. Adv. Parasitol. 84, 209–252. doi: 10.1016/B978-0-12-800099-1.00004-1
Frohlich, K., Hua, Z., Wang, J., and Shen, L. (2012). Isolation of Chlamydia trachomatis and membrane vesicles derived from host and bacteria. J. Microbiol. Methods 91, 222–230. doi: 10.1016/j.mimet.2012.08.012
Garcia-Silva, M. R., das Neves, R. F., Cabrera-Cabrera, F., Sanguinetti, J., Medeiros, L. C., Robello, C., et al. (2014). Extracellular vesicles shed by Trypanosoma cruzi are linked to small RNA pathways, life cycle regulation, and susceptibility to infection of mammalian cells. Parasitol. Res. 113, 285–304. doi: 10.1007/s00436-013-3655-1
Ghosh, J., Lal, C. S., Pandey, K., Das, V. N., Das, P., Roychoudhury, K., et al. (2011). Human visceral leishmaniasis: decrease in serum cholesterol as a function of splenic parasite load. Ann. Trop. Med. Parasitol. 105, 267–271. doi: 10.1179/136485911X12899838683566
Gomez, M. A., Contreras, I., Halle, M., Tremblay, M. L., McMaster, R. W., and Olivier, M. (2009). Leishmania GP63 alters host signaling through cleavage-activated protein tyrosine phosphatases. Sci. Signal. 2:ra58. doi: 10.1126/scisignal.2000213
Graystock, P., Meeus, I., Smagghe, G., Goulson, D., and Hughes, W. O. (2016). The effects of single and mixed infections of Apicystis bombi and deformed wing virus in Bombus terrestris. Parasitology 143, 358–365. doi: 10.1017/S0031182015001614
Gunjan, S., Sharma, T., Yadav, K., Chauhan, B. S., Singh, S. K., Siddiqi, M. I., et al. (2018). Artemisinin derivatives and synthetic trioxane trigger apoptotic cell death in asexual stages of plasmodium. Front. Cell Infect. Microbiol. 8:256. doi: 10.3389/fcimb.2018.00256
Gunjan, S., Singh, S. K., Sharma, T., Dwivedi, H., Chauhan, B. S., Imran Siddiqi, M., et al. (2016). Mefloquine induces ROS mediated programmed cell death in malaria parasite: Plasmodium. Apoptosis 21, 955–964. doi: 10.1007/s10495-016-1265-y
Harding, C., Heuser, J., and Stahl, P. (1984). Endocytosis and intracellular processing of transferrin and colloidal gold-transferrin in rat reticulocytes: demonstration of a pathway for receptor shedding. Eur. J. Cell Biol. 35, 256–263.
Hassani, K., and Olivier, M. (2013). Immunomodulatory impact of leishmania-induced macrophage exosomes: a comparative proteomic and functional analysis. PLoS Negl. Trop. Dis. 7:e2185. doi: 10.1371/journal.pntd.0002185
Janiszewski, M., Do Carmo, A. O., Pedro, M. A., Silva, E., Knobel, E., and Laurindo, F. R. (2004). Platelet-derived exosomes of septic individuals possess proapoptotic NAD(P)H oxidase activity: a novel vascular redox pathway. Crit. Care Med. 32, 818–825. doi: 10.1097/01.CCM.0000114829.17746.19
Kalra, H., Drummen, G. P., and Mathivanan, S. (2016). Focus on extracellular vesicles: introducing the next small big thing. Int. J. Mol. Sci. 17:170. doi: 10.3390/ijms17020170
Kawamura, Y., Yamamoto, Y., Sato, T. A., and Ochiya, T. (2017). Extracellular vesicles as trans-genomic agents: emerging roles in disease and evolution. Cancer Sci. 108, 824–830. doi: 10.1111/cas.13222
Keller, S., Sanderson, M. P., Stoeck, A., and Altevogt, P. (2006). Exosomes: from biogenesis and secretion to biological function. Immunol. Lett. 107, 102–108. doi: 10.1016/j.imlet.2006.09.005
Kim, D. K., Lee, J., Kim, S. R., Choi, D. S., Yoon, Y. J., Kim, J. H., et al. (2015). EVpedia: a community web portal for extracellular vesicles research. Bioinformatics 31, 933–939. doi: 10.1093/bioinformatics/btu741
Kreimer, S., Belov, A. M., Ghiran, I., Murthy, S. K., Frank, D. A., and Ivanov, A. R. (2015). Mass-spectrometry-based molecular characterization of extracellular vesicles: lipidomics and proteomics. J. Proteome Res. 14, 2367–2384. doi: 10.1021/pr501279t
Krishna, S., Bharti, P. K., Chandel, H. S., Ahmad, A., Kumar, R., Singh, P. P., et al. (2015). Detection of mixed infections with Plasmodium spp. by PCR, India, 2014. Emerg. Infect. Dis. 21, 1853–1857. doi: 10.3201/eid2110.150678
Lacroix, R., Mukabana, W. R., Gouagna, L. C., and Koella, J. C. (2005). Malaria infection increases attractiveness of humans to mosquitoes. PLoS Biol. 3:e298. doi: 10.1371/journal.pbio.0030298
Lazaro-Ibanez, E., Sanz-Garcia, A., Visakorpi, T., Escobedo-Lucea, C., Siljander, P., Ayuso-Sacido, A., et al. (2014). Different gDNA content in the subpopulations of prostate cancer extracellular vesicles: apoptotic bodies, microvesicles, and exosomes. Prostate 74, 1379–1390. doi: 10.1002/pros.22853
Lefebvre, F. A., Benoit Bouvrette, L. P., Perras, L., Blanchet-Cohen, A., Garnier, D., Rak, J., et al. (2016). Comparative transcriptomic analysis of human and Drosophila extracellular vesicles. Sci. Rep. 6:27680. doi: 10.1038/srep27680
Lener, T., Gimona, M., Aigner, L., Borger, V., Buzas, E., Camussi, G., et al. (2015). Applying extracellular vesicles based therapeutics in clinical trials - an ISEV position paper. J. Extracell Vesicles 4:30087. doi: 10.3402/jev.v4.30087
Leventis, P. A., and Grinstein, S. (2010). The distribution and function of phosphatidylserine in cellular membranes. Annu. Rev. Biophys. 39, 407–427. doi: 10.1146/annurev.biophys.093008.131234
Li, M., Wang, H., Liu, J., Hao, P., Ma, L., and Liu, Q. (2015). The Apoptotic role of metacaspase in Toxoplasma gondii. Front. Microbiol. 6:1560. doi: 10.3389/fmicb.2015.01560
Lu, T., Qiu, Y. T., Wang, G., Kwon, J. Y., Rutzler, M., Kwon, H. W., et al. (2007). Odor coding in the maxillary palp of the malaria vector mosquito Anopheles gambiae. Curr. Biol. 17, 1533–1544. doi: 10.1016/j.cub.2007.07.062
Luse, S. A., and Miller, L. H. (1971). Plasmodium falciparum malaria. Ultrastructure of parasitized erythrocytes in cardiac vessels. Am. J. Trop. Med. Hyg. 20, 655–660. doi: 10.4269/ajtmh.1971.20.655
Mandal, A., Das, S., Roy, S., Ghosh, A. K., Sardar, A. H., Verma, S., et al. (2016). Deprivation of L-Arginine induces oxidative stress mediated apoptosis in leishmania donovani promastigotes: contribution of the polyamine pathway. PLoS. Negl. Trop. Dis. 10:e0004373. doi: 10.1371/journal.pntd.0004373
Mantel, P. Y., Hjelmqvist, D., Walch, M., Kharoubi-Hess, S., Nilsson, S., Ravel, D., et al. (2016). Infected erythrocyte-derived extracellular vesicles alter vascular function via regulatory Ago2-miRNA complexes in malaria. Nat. Commun. 7:12727. doi: 10.1038/ncomms12727
Mantel, P. Y., Hoang, A. N., Goldowitz, I., Potashnikova, D., Hamza, B., Vorobjev, I., et al. (2013). Malaria-infected erythrocyte-derived microvesicles mediate cellular communication within the parasite population and with the host immune system. Cell Host Microbe. 13, 521–534. doi: 10.1016/j.chom.2013.04.009
Mantel, P. Y., and Marti, M. (2014). The role of extracellular vesicles in Plasmodium and other protozoan parasites. Cell. Microbiol. 16, 344–354. doi: 10.1111/cmi.12259
Martin-Jaular, L., Nakayasu, E. S., Ferrer, M., Almeida, I. C., and Del Portillo, H. A. (2011). Exosomes from Plasmodium yoelii-infected reticulocytes protect mice from lethal infections. PLoS ONE 6:e26588. doi: 10.1371/journal.pone.0026588
Matthews, K. R. (2005). The developmental cell biology of Trypanosoma brucei. J. Cell Sci. 118(Pt 2), 283–290. doi: 10.1242/jcs.01649
Meldolesi, J. (2018). Exosomes and ectosomes in intercellular communication. Curr. Biol. 28, R435–R444. doi: 10.1016/j.cub.2018.01.059
Meslin, B., Barnadas, C., Boni, V., Latour, C., De Monbrison, F., Kaiser, K., et al. (2007). Features of apoptosis in Plasmodium falciparum erythrocytic stage through a putative role of PfMCA1 metacaspase-like protein. J. Infect Dis. 195, 1852–1859. doi: 10.1086/518253
Molina-Cruz, A., and Barillas-Mury, C. (2014). The remarkable journey of adaptation of the Plasmodium falciparum malaria parasite to New World anopheline mosquitoes. Mem. Inst. Oswaldo Cruz. 109, 662–667. doi: 10.1590/0074-0276130553
Montaner, S., Galiano, A., Trelis, M., Martin-Jaular, L., Del Portillo, H. A., Bernal, D., et al. (2014). The role of extracellular vesicles in modulating the host immune response during parasitic infections. Front. Immunol. 5:433. doi: 10.3389/fimmu.2014.00433
Mozzi, A., Pontremoli, C., and Sironi, M. (2018). Genetic susceptibility to infectious diseases: current status and future perspectives from genome-wide approaches. Infect. Genet. Evol. 66, 286–307. doi: 10.1016/j.meegid.2017.09.028
Muralidharan-Chari, V., Clancy, J., Plou, C., Romao, M., Chavrier, P., Raposo, G., et al. (2009). ARF6-regulated shedding of tumor cell-derived plasma membrane microvesicles. Curr. Biol. 19, 1875–1885. doi: 10.1016/j.cub.2009.09.059
Mutai, B. K., and Waitumbi, J. N. (2010). Apoptosis stalks Plasmodium falciparum maintained in continuous culture condition. Malar. J. 9(Suppl. 3):S6. doi: 10.1186/1475-2875-9-S3-S6
Nabhan, J. F., Hu, R., Oh, R. S., Cohen, S. N., and Lu, Q. (2012). Formation and release of arrestin domain-containing protein 1-mediated microvesicles (ARMMs) at plasma membrane by recruitment of TSG101 protein. Proc. Natl. Acad. Sci. U.S.A. 109, 4146–4151. doi: 10.1073/pnas.1200448109
Nawaz, M., Camussi, G., Valadi, H., Nazarenko, I., Ekstrom, K., Wang, X., et al. (2014). The emerging role of extracellular vesicles as biomarkers for urogenital cancers. Nat. Rev. Urol. 11, 688–701. doi: 10.1038/nrurol.2014.301
Ostrowski, M., Carmo, N. B., Krumeich, S., Fanget, I., Raposo, G., Savina, A., et al. (2010). Rab27a and Rab27b control different steps of the exosome secretion pathway. Nat. Cell Biol. 12, 19–30. doi: 10.1038/ncb2000
Otto, T. D., Gilabert, A., Crellen, T., Bohme, U., Arnathau, C., Sanders, M., et al. (2018). Genomes of all known members of a Plasmodium subgenus reveal paths to virulent human malaria. Nat. Microbiol. 3, 687–697. doi: 10.1038/s41564-018-0162-2
Pankoui Mfonkeu, J. B., Gouado, I., Fotso Kuate, H., Zambou, O., Amvam Zollo, P. H., Grau, G. E., et al. (2010). Elevated cell-specific microparticles are a biological marker for cerebral dysfunctions in human severe malaria. PLoS ONE 5:e13415. doi: 10.1371/journal.pone.0013415
Piguet, P. F., Kan, C. D., and Vesin, C. (2002). Thrombocytopenia in an animal model of malaria is associated with an increased caspase-mediated death of thrombocytes. Apoptosis 7, 91–98. doi: 10.1023/A:1014341611412
Plenderleith, L. J., Liu, W., Learn, G. H., Loy, D. E., Speede, S., Sanz, C. M., et al. (2019). Ancient introgression between two ape malaria parasite species. Genome. Biol. Evol. 11, 3269–3274. doi: 10.1093/gbe/evz244
Preusser, C., Hung, L. H., Schneider, T., Schreiner, S., Hardt, M., Moebus, A., et al. (2018). Selective release of circRNAs in platelet-derived extracellular vesicles. J. Extracell. Vesicles 7:1424473. doi: 10.1080/20013078.2018.1424473
Proto, W. R., Coombs, G. H., and Mottram, J. C. (2013). Cell death in parasitic protozoa: regulated or incidental? Nat. Rev. Microbiol. 11, 58–66. doi: 10.1038/nrmicro2929
Prugnolle, F., Durand, P., Neel, C., Ollomo, B., Ayala, F. J., Arnathau, C., et al. (2010). African great apes are natural hosts of multiple related malaria species, including Plasmodium falciparum. Proc. Natl. Acad. Sci. U.S.A. 107, 1458–1463. doi: 10.1073/pnas.0914440107
Quintana-Murci, L. (2019). Human immunology through the lens of evolutionary genetics. Cell 177,184–199. doi: 10.1016/j.cell.2019.02.033
Ramirez, M. I., Deolindo, P., de Messias-Reason, I. J., Arigi, E. A., Choi, H., Almeida, I. C., et al. (2017). Dynamic flux of microvesicles modulate parasite-host cell interaction of Trypanosoma cruzi in eukaryotic cells. Cell. Microbiol. (2017) 19:e12672. doi: 10.1111/cmi.12672
Ranford-Cartwright, L. C., and Mwangi, J. M. (2012). Analysis of malaria parasite phenotypes using experimental genetic crosses of Plasmodium falciparum. Int. J. Parasitol. 42, 529–534. doi: 10.1016/j.ijpara.2012.03.004
Raposo, G., Nijman, H. W., Stoorvogel, W., Liejendekker, R., Harding, C. V., Melief, C. J., et al. (1996). B lymphocytes secrete antigen-presenting vesicles. J. Exp. Med. 183, 1161–1172. doi: 10.1084/jem.183.3.1161
Raposo, G., and Stoorvogel, W. (2013). Extracellular vesicles: exosomes, microvesicles, and friends. J. Cell. Biol. 200, 373–383. doi: 10.1083/jcb.201211138
Reece, S. E., Pollitt, L. C., Colegrave, N., and Gardner, A. (2011). The meaning of death: evolution and ecology of apoptosis in protozoan parasites. PLoS Pathog. 7:e1002320. doi: 10.1371/journal.ppat.1002320
Regev-Rudzki, N., Wilson, D. W., Carvalho, T. G., Sisquella, X., Coleman, B. M., Rug, M., et al. (2013). Cell-cell communication between malaria-infected red blood cells via exosome-like vesicles. Cell 153, 1120–1133. doi: 10.1016/j.cell.2013.04.029
Rezanezhad, H., Menegon, M., Sarkari, B., Hatam, G. R., and Severini, C. (2011). Characterization of the metacaspase 1 gene in Plasmodium vivax field isolates from southern Iran and Italian imported cases. Acta Trop. 119, 57–60. doi: 10.1016/j.actatropica.2011.03.010
Rioux, J. D., Xavier, R. J., Taylor, K. D., Silverberg, M. S., Goyette, P., Huett, A., et al. (2007). Genome-wide association study identifies new susceptibility loci for Crohn disease and implicates autophagy in disease pathogenesis: Nat. Genet. 39, 596–604. doi: 10.1038/ng2032
Rodrigues, M. L., Nakayasu, E. S., Oliveira, D. L., Nimrichter, L., Nosanchuk, J. D., Almeida, I. C., et al. (2008). Extracellular vesicles produced by Cryptococcus neoformans contain protein components associated with virulence. Eukaryot. Cell 7, 58–67. doi: 10.1128/EC.00370-07
Sahu, U., Mohapatra, B. N., Kar, S. K., and Ranjit, M. (2013). Promoter polymorphisms in the ATP binding cassette transporter gene influence production of cell-derived microparticles and are highly associated with susceptibility to severe malaria in humans. Infect. Immun. 81, 1287–1294. doi: 10.1128/IAI.01175-12
Schorey, J. S., Cheng, Y., Singh, P. P., and Smith, V. L. (2015). Exosomes and other extracellular vesicles in host-pathogen interactions. EMBO Rep. 16, 24–43. doi: 10.15252/embr.201439363
Shrivastava, S. K., Dalko, E., Delcroix-Genete, D., Herbert, F., Cazenave, P. A., and Pied, S. (2017). Uptake of parasite-derived vesicles by astrocytes and microglial phagocytosis of infected erythrocytes may drive neuroinflammation in cerebral malaria. Glia 65, 75–92. doi: 10.1002/glia.23075
Silva, V. O., Maia, M. M., Torrecilhas, A. C., Taniwaki, N. N., Namiyama, G. M., Oliveira, K. C., et al. (2018). Extracellular vesicles isolated from Toxoplasma gondii induce host immune response. Parasite Immunol. 40:e12571. doi: 10.1111/pim.12571
Silverman, J. M., Chan, S. K., Robinson, D. P., Dwyer, D. M., Nandan, D., Foster, L. J., et al. (2008). Proteomic analysis of the secretome of Leishmania donovani. Genome. Biol. 9:R35. doi: 10.1186/gb-2008-9-2-r35
Silverman, J. M., Clos, J., Horakova, E., Wang, A. Y., Wiesgigl, M., Kelly, I., et al. (2010). Leishmania exosomes modulate innate and adaptive immune responses through effects on monocytes and dendritic cells. J. Immunol. 185, 5011–5022. doi: 10.4049/jimmunol.1000541
Silverman, J. M., and Reiner, N. E. (2011). Leishmania exosomes deliver preemptive strikes to create an environment permissive for early infection. Front. Cell Infect. Microbiol. 1:26. doi: 10.3389/fcimb.2011.00026
Silvester, E., Young, J., Ivens, A., and Matthews, K. R. (2017). Interspecies quorum sensing in co-infections can manipulate trypanosome transmission potential. Nat. Microbiol. 2, 1471–1479. doi: 10.1038/s41564-017-0014-5
Sisquella, X., Ofir-Birin, Y., Pimentel, M. A., Cheng, L., Abou Karam, P., Sampaio, N. G., et al. (2017). Malaria parasite DNA-harbouring vesicles activate cytosolic immune sensors. Nat. Commun. 8:1985. doi: 10.1038/s41467-017-02083-1
Smallegange, R. C., van Gemert, G. J., van de Vegte-Bolmer, M., Gezan, S., Takken, W., Sauerwein, R. W., et al. (2013). Malaria infected mosquitoes express enhanced attraction to human odor. PLoS ONE 8:e63602. doi: 10.1371/journal.pone.0063602
Sow, F., Nyonda, M., Bienvenu, A. L., and Picot, S. (2015). Wanted Plasmodium falciparum, dead or alive. Microb. Cell 2, 219–224. doi: 10.15698/mic2015.07.211
Spycher, C., Rug, M., Klonis, N., Ferguson, D. J., Cowman, A. F., Beck, H. P., et al. (2006). Genesis of and trafficking to the Maurer's clefts of Plasmodium falciparum-infected erythrocytes. Mol. Cell Biol. 26, 4074–4085. doi: 10.1128/MCB.00095-06
Stenqvist, A. C., Nagaeva, O., Baranov, V., and Mincheva-Nilsson, L. (2013). Exosomes secreted by human placenta carry functional Fas ligand and TRAIL molecules and convey apoptosis in activated immune cells, suggesting exosome-mediated immune privilege of the fetus. J. Immunol. 191, 5515–5523. doi: 10.4049/jimmunol.1301885
Sundararaman, S. A., Plenderleith, L. J., Liu, W., Loy, D. E., Learn, G. H., Li, Y., et al. (2016). Genomes of cryptic chimpanzee Plasmodium species reveal key evolutionary events leading to human malaria. Nat. Commun. 7:11078. doi: 10.1038/ncomms11078
Szatanek, R., Baj-Krzyworzeka, M., Zimoch, J., Lekka, M., Siedlar, M., and Baran, J. (2017). The methods of choice for extracellular vesicles (EVs) characterization. Int. J. Mol. Sci. 18:E1153. doi: 10.3390/ijms18061153
Takken, W., and Knols, B. G. (1999). Odor-mediated behavior of Afrotropical malaria mosquitoes. Annu. Rev. Entomol. 44, 131–157. doi: 10.1146/annurev.ento.44.1.131
Totino, P. R., Magalhaes, A., Alves, E. B., Costa, M. R., de Lacerda, M. V., Daniel-Ribeiro, C. T., et al. (2014). Plasmodium falciparum, but not P. vivax, can induce erythrocytic apoptosis. Parasit. Vectors 7:484. doi: 10.1186/PREACCEPT-1516774475118464
Trocoli Torrecilhas, A. C., Tonelli, R. R., Pavanelli, W. R., da Silva, J. S., Schumacher, R. I., de Souza, W., et al. (2009). Trypanosoma cruzi: parasite shed vesicles increase heart parasitism and generate an intense inflammatory response. Microbes. Infect. 11, 29–39. doi: 10.1016/j.micinf.2008.10.003
Turchinovich, A., Drapkina, O., and Tonevitsky, A. (2019). Transcriptome of extracellular vesicles: state-of-the-art. Front. Immunol. 10:202. doi: 10.3389/fimmu.2019.00202
Twu, O., de Miguel, N., Lustig, G., Stevens, G. C., Vashisht, A. A., Wohlschlegel, J. A., et al. (2013). Trichomonas vaginalis exosomes deliver cargo to host cells and mediate hostratioparasite interactions. PLoS Pathog. 9:e1003482. doi: 10.1371/journal.ppat.1003482
van Balkom, B. W., Eisele, A. S., Pegtel, D. M., Bervoets, S., and Verhaar, M. C. (2015). Quantitative and qualitative analysis of small RNAs in human endothelial cells and exosomes provides insights into localized RNA processing, degradation and sorting. J. Extracell. Vesicles 4:26760. doi: 10.3402/jev.v4.26760
van der Heyde, H. C., Gramaglia, I., Combes, V., George, T. C., and Grau, G. E. (2011). Flow cytometric analysis of microparticles. Methods Mol. Biol. 699, 337–354. doi: 10.1007/978-1-61737-950-5_16
van der Pol, E., Boing, A. N., Harrison, P., Sturk, A., and Nieuwland, R. (2012). Classification, functions, and clinical relevance of extracellular vesicles. Pharmacol. Rev. 64, 676–705. doi: 10.1124/pr.112.005983
van Niel, G., D'Angelo, G., and Raposo, G. (2018). Shedding light on the cell biology of extracellular vesicles. Nat. Rev. Mol. Cell. Biol. 19, 213–228. doi: 10.1038/nrm.2017.125
Wei, M., Lu, L., Sui, W., Liu, Y., Shi, X., and Lv, L. (2018). Inhibition of GLUTs by WZB117 mediates apoptosis in blood-stage Plasmodium parasites by breaking redox balance. Biochem. Biophys. Res. Commun. 503, 1154–1159. doi: 10.1016/j.bbrc.2018.06.134
Wolf, P. (1967). The nature and significance of platelet products in human plasma. Br. J. Haematol. 13, 269–288. doi: 10.1111/j.1365-2141.1967.tb08741.x
Yanez-Mo, M., Siljander, P. R., Andreu, Z., Zavec, A. B., Borras, F. E., Buzas, E. I., et al. (2015). Biological properties of extracellular vesicles and their physiological functions. J. Extracell. Vesicles 4:27066. doi: 10.3402/jev.v4.27066
Yang, Z., and Bielawski, J. P. (2000). Statistical methods for detecting molecular adaptation. Trends Ecol. Evol. 15, 496–503. doi: 10.1016/S0169-5347(00)01994-7
Ye, W., Chew, M., Hou, J., Lai, F., Leopold, S. J., Loo, H. L., et al. (2018). Microvesicles from malaria-infected red blood cells activate natural killer cells via MDA5 pathway. PLoS Pathog. 14:e1007298. doi: 10.1371/journal.ppat.1007298
Keywords: extracellular vesicles, Plasmodium falciparum, interkingdom, communication, evolution, parasites, host, vector
Citation: Correa R, Caballero Z, De León LF and Spadafora C (2020) Extracellular Vesicles Could Carry an Evolutionary Footprint in Interkingdom Communication. Front. Cell. Infect. Microbiol. 10:76. doi: 10.3389/fcimb.2020.00076
Received: 14 October 2019; Accepted: 14 February 2020;
Published: 03 March 2020.
Edited by:
Matthias Marti, University of Glasgow, United KingdomReviewed by:
Ana Maria Jansen, Oswaldo Cruz Foundation (Fiocruz), BrazilRobert P. Hirt, Newcastle University, United Kingdom
Copyright © 2020 Correa, Caballero, De León and Spadafora. This is an open-access article distributed under the terms of the Creative Commons Attribution License (CC BY). The use, distribution or reproduction in other forums is permitted, provided the original author(s) and the copyright owner(s) are credited and that the original publication in this journal is cited, in accordance with accepted academic practice. No use, distribution or reproduction is permitted which does not comply with these terms.
*Correspondence: Carmenza Spadafora, cspadafora@indicasat.org.pa