Gut Microbiota Approach—A New Strategy to Treat Parkinson’s Disease
- 1Department of Microbiology and Immunity, The College of Medical Technology, Shanghai University of Medicine & Health Sciences, Shanghai, China
- 2Microbial Pharmacology Laboratory, Shanghai University of Medicine & Health Sciences, Shanghai, China
- 3State Key Laboratory of New Drug and Pharmaceutical Process, Shanghai Institute of Pharmaceutical Industry, Shanghai, China
Parkinson’s disease (PD) is a progressive neurodegenerative disorder characterized by neuronal loss and dysfunction of dopaminergic neurons located in the substantia nigra, which contain a variety of misfolded α-synuclein (α-syn). Medications that increase or substitute for dopamine can be used for the treatment of PD. Recently, numerous studies have shown gut microbiota plays a crucial role in regulating and maintaining multiple aspects of host physiology including host metabolism and neurodevelopment. In this review article, the role of gut microbiota in the etiological mechanism of PD will be reviewed. Furthermore, we discussed current pharmaceutical medicine-based methods to prevent and treat PD, followed by describing specific strains that affect the host brain function through the gut-brain axis. We explained in detail how gut microbiota directly produces neurotransmitters or regulate the host biosynthesis of neurotransmitters. The neurotransmitters secreted by the intestinal lumen bacteria may induce epithelial cells to release molecules that, in turn, can regulate neural signaling in the enteric nervous system and subsequently control brain function and behavior through the brain-gut axis. Finally, we proved that the microbial regulation of the host neuronal system. Endogenous α-syn can be transmitted long distance and bidirectional between ENS and brain through the circulatory system which gives us a new option that the possibility of altering the community of gut microbiota in completely new medication option for treating PD.
Introduction
Parkinson’s disease (PD), also known as tremor paralysis, is a common neurological degenerative disease in the elderly, characterized by the lesions of substantia nigra and striatum (Liepelt-Scarfone et al., 2013). Tremor, muscle rigidity, bradykinesia, and unstable posture are the main clinical symptoms of this disease. PD is associated with a variety of factors, including cerebrovascular disease (Haugarvoll et al., 2005), cerebral arteriosclerosis (Kummer et al., 2019), infections (Limphaibool et al., 2019), poisoning (Taba, 2017; Li et al., 2018), trauma, medications (Höllerhage, 2019), and genetic defects (Vila and Przedborski, 2004; Manzoni and Lewis, 2013). PD is the second most common neurodegenerative condition after Alzheimer’s disease which affects patients in the world. With the acceleration of the population aging process and decrease of physical functions of senile patients, the incidence and prevalence of PD have shown an increasing trend year by year (Nussbaum and Ellis, 2003; Hirsch et al., 2016). Approximately between 4.1 million and 4.6 million people are affected by PD in 2005 totaled, and it has been estimated that number will more than double by 2030 to between 8.7 million and 9.3 million in the most populous nations (Dorsey et al., 2007; Wang et al., 2020). The majority of people who get PD are over the age of 60, the incidence of PD among people over 60 is about one percent, men are more susceptible to PD than women at a ratio of about 3:2 (De Lau and Breteler, 2006; Dorsey and Bloem, 2018; Cerri et al., 2019).
The deposition of alpha-synuclein (α-syn) in neuronal cells could contribute to the development of PD. The α-syn is the most abundant protein constituent of Lewy bodies(LBs), which are generally described as round lamellar eosinophilic cytoplasmic inclusions (Braak et al., 2003a). LBs are the hallmark pathologic features of PD. Whether LBs are cytotoxic or cytoprotective to neuronal cells remains debatable. It could potentially be toxic since the number of cortical LBs positively correlated with the severity of symptoms of dementia in PD (Hurtig et al., 2000). However, in some cases, Lewy pathology is also found but without present parkinsonism (Parkkinen et al., 2008; Adler et al., 2010; Milber et al., 2012). Some studies have also suggested that α-syn aggregates might be protective (da Costa et al., 2000; Tanaka et al., 2004), while oligomers and pre-fibrillar α-syn are the toxic species responsible for neurodegeneration (Chen et al., 2009). The excessive accumulation of a-syn can enhance its toxicity and lead to the degeneration of DA (dopamine) neurons in the substantia nigra of the midbrain in PD, and the loss of neurons is associated with motor symptoms (Dickson et al., 2009). It is also possible rather than the neuronal loss, the presynaptic terminal failure may be the more critical pathogenic factor for motor symptoms of PD (Schulz-Schaeffer, 2010). Besides motor symptoms, PD is also associated with non-motor symptoms. In the early stage of PD, non-motor symptoms such as insomnia, impairment of smell (Shah et al., 2009) as well as gastrointestinal (GI) dysfunction (nausea, abnormal salivation, constipation, prolonged intestinal transit time etc.) (Cersosimo et al., 2013; Mulak and Bonaz, 2015) can be found. The typical movement-related symptoms, such as tremor, rigidity, bradykinesia, and postural instability are reported in the second stage of PD. In the final stage, the severe psychotic symptoms such as motor disorders and neuropsychiatric disturbances which including depression (Marsh, 2013; Parashar and Udayabanu, 2017; Guo et al., 2020; Park et al., 2020), dementia (Tsuang et al., 2013) can be observed in PD patients. The interesting phenomenon is that PD patients who suffer from GI symptoms can occur several years ahead of classic motor symptoms (Chen et al., 2015). The GI dysfunction caused by gut microbiota disorder which can initiate α-syn accumulation in the enteric nerve cell, causing concurrent mucosal inflammation and oxidative stress (Chen et al., 2019). So scientists give the hypothesis that PD may begin in the gastrointestinal tract and transfer to the brain through the gut-brain axis (Hawkes et al., 2007).
It has been shown that the intestinal microbiota and its metabolites can be involved in modulating a lot of GI functions, such as intestinal permeability (Frazier et al., 2011), mucosal immune function (Simrén et al., 2013), the motility (Cani et al., 2013) and sensitivity of the intestine (Valdez-Morales et al., 2013), as well as the activity in the ENS(enteric nervous system) (Forsythe and Kunze, 2013). The microbiota and its metabolites are also likely to modulate behaviors and brain processes, including stress responsiveness (Dinan and Cryan, 2012), emotional behavior (Foster and Neufeld, 2013), pain modulation, ingestive behavior (Cryan and Dinan, 2012), and brain biochemistry (Stilling et al., 2014). Therefore, altering the community of gut microbiota through prebiotics and antibiotics or fecal transplantation can give a new approach to treat PD due to gut microbiotas play a significant role in the neuropathogenesis of CNS (central nervous system) disorders.
The Etiological Mechanism of PD
Aging is the most important risk factor for PD, and the biochemical changes caused by aging exacerbate these abnormalities in the brain of PD (Reeve et al., 2014). Dysfunction of DA neurons will cause a neuronal loss in the substantia nigra, which ultimately leads to inhibition of motor cortex neuron activation and function (Sulzer, 2007). In PD patients, motor symptoms are mainly related to the loss of DA neurons in the substantia nigra (Yamada et al., 2004). Moreover, neuropathological changes could be found in the autonomic nervous system, olfactory structures, the lower brainstem, and cerebral cortex (Dickson, 2012; Rey et al., 2016). Extrapyramidal pathology is associated with a wide range of non-motor symptoms, which is considered as an important feature of PD (Lim and Lang, 2010). It is reported that about 80% of PD patients have gastrointestinal dysfunction especially constipation (Frazzitta et al., 2019) and the GI dysfunction can occur several years before the onset of motor symptoms. Idiopathic constipation is one of the most substantial risk factors for PD (Poirier et al., 2016). For many years, people have understood that the environmental and genetic factors can cause loss of DA neurons in the substantia nigra, which has been dramatically expanded the understanding of the etiology of PD (Schapira and Jenner, 2011). Based on lots of experiment investigations, people have reached a consensus on the mechanism of cell death induced by toxins (Anselmi et al., 2018), while how the genetic defects lead to the loss of the neurons in PD is not clear. The neuronal cell death could be caused by apoptosis or autophagy. Mitochondrial dysfunction, oxidative stress, altered protein handling, and inflammatory could be involved in the neuronal cell death (Lin and Beal, 2006; Schapira and Jenner, 2011). Mitochondria play a critical role in cellular energy metabolism, mitochondrial dysfunction and LBs formation are vital to the pathogenesis of PD (Golpich et al., 2017).
As shown before, the formation of LBs is very important for the understanding of pathogenesis in PD. Lewy neurites are the elongated structures in dendritic or axonal compartments that are in the central and peripheral nervous systems (Volpicelli-Daley et al., 2014). Both LBs and Lewy neurites are mainly composed of filaments of misfolded α-syn protein (Spillantini et al., 1998; Goedert et al., 2013) The native conformation of α-syn is a soluble monomer that serves a pivotal role in synaptic transmission and enhances the transmitter release from the presynaptic vesicle (Burré, 2015). The α-syn protein is generally expressed in the CNS with a function of modifying the supply and release of DA to regulate neurotransmission in the brain (Longhena et al., 2019). The intermediate oligomeric protofibrillar form of α-syn has been suggested to be the most toxic species (Goldberg and Lansbury, 2000). Their accumulation at presynaptic terminals will affect the pivotal steps of neurotransmitter release (Bridi and Hirth, 2018). In PD patients, the progressive degeneration of DA neurons in the dense substantia nigra of the midbrain is the main pathological change of PD (Lindvall and Kokaia, 2009).
Damage in synaptic activity by α-syn microaggregation plays a key role in DA neurons degeneration (Calo et al., 2016). In healthy conditions, the correct organization of synaptic vesicle pools in a dopaminergic striatal terminal can be observed in the brain, monomer α-syn by regulating DA transporters can control preserved DA release and reuptake (Burré, 2015). In the prodromal phases of PD, high levels of α-syn microaggregate at synaptic terminals, this will alter the size of synaptic vesicle pools. The trafficking between the reserve and readily releasable pools will be impaired (Bridi and Hirth, 2018). Misfold α-syn can misregulate or redistribute proteins of the presynaptic Soluble NSF Attachment Protein Receptor (SNARE) complex, synaptic vesicles cluster, and their recycling was attenuated (Wang et al., 2014; Bellucci et al., 2017). Furthermore, α-syn overexpression reduces dopamine transporter (DAT) membrane content and reduces DA release (Vaughan and Foster, 2013). The presynaptic alterations impair neurotransmitter exocytosis and neuronal communication. Terminal loss and axonal or cell body degeneration not yet happen during this stage. In the early stages of PD, loss of neuronal connections at terminals could trigger axonal damage synaptic and axonal loss, the onset of symptoms is related to these changes (Caminiti et al., 2017). DAT binding decreased and partial Nigrosome-1 degenerated (Wang et al., 2017). Finally, in the advanced phases of PD, broad synaptic, axonal, and cell body degeneration can be detected concomitantly participate in disease progression. The degeneration of DA neurons in PD can be mediated by apoptosis. Two particular proteins have an essential function in the process of apoptosis: DRP1 promotes mitochondrial cytochrome C release, while the OPA1 inhibits cytochrome C release (Estaquier and Arnoult, 2007; Sheridan and Martin, 2010). When the balance between the two proteins is broken, a large number of cytochrome C are released, the cell death process will happen (Suen et al., 2008). Once apoptosis is activated, amoeba changes, cell membranes blister, cytoskeleton collapse, and cytoplasm condense will also happen. These will cause nuclear agglutination, chromosome agglutination or fragmentation, plasma membrane bleb and apoptotic body formation (Elmore, 2007). Apoptotic signals are transmitted to the mitochondria, causing the release of cytochrome C, which is located in the intermembrane of mitochondria, where it acts as an electron shuttle function in the respiratory chain (Li et al., 2000). Cytochrome C binds to APAF-1 and activates caspase 9, causing protein hydrolysis and eventually leading to neuronal apoptosis (Woo et al., 2003). Under normal physiological conditions, neuronal cells have a highly resistant ability to apoptosis in the late stage of mitosis. However, pathologic apoptosis can occur in nerve cells under some stimulus. In recent years, more and more researchers have realized the importance of abnormal apoptotic pathways in the pathogenesis of PD (Lama et al., 2020).
The researchers have found that α-syn not only can regulate the neurotransmission in the brain and also it can regulate the GI function. The α-syn forms and diffuse from the intestinal tract to the brain, supporting the hypothesis that PD pathogenesis may primarily function through the gut intestine, as shown in Figure 1 (Holmqvist et al., 2014). It is reported that during the early stage of PD, the internal and external innervation of the GI tract, the dorsal motor nucleus of the vagus nerve (DMV) and the ENS of the vagus nerve were affected to various degrees by the intestine, suggesting that the PD pathogenesis observed in the gut were even earlier than the substantia nigra (Braak et al., 2003b). It has been proven that an unknown neurotropic pathogen initially damaged and disrupted the innervation of the GI tract and led to Lewy pathology of the intestine. The intestinal α-syn forms (including monomers, oligomers, and fibrils) reach the DMV through vagal innervation and eventually damage the substantia nigra, which lead to the appearance of the clinical symptoms of PD (Braak et al., 2006; Recasens and Dehay, 2014; Longhena et al., 2017) (Figure 1). According to this hyperthesis, the clinical pathology of PD can be found in the following three stages. In the early stage of PD, initial pathological α-syn appears in the olfactory bulb and DMV (Braak et al., 2003a; Forsyth et al., 2011). In the second stage of PD, substantia nigra be positive for immunoreactive α-syn inclusions (Repovš and Baddeley, 2006; Rey et al., 2016). In the final stage, as LBs reach the striatum and cerebral cortex, the severe psychotic symptoms of PD can be observed. It is reported that gut-initiated pathological processes in PD not only can be caused by a PD pathogen or environmental toxin, it also can be directly caused by gut microbiota disorder. Holmqvist proved that α-syn could be retrogradely transported from the intestinal wall to the brain by the experiment that the injection of α-syn into the intestinal wall of rats and track the transfer route (Holmqvist et al., 2014). Some researchers also found that α-syn can be transmitted via endocytosis to neighboring neurons by using in vitro and in vivo experiments (Hansen et al., 2011; Angot et al., 2012; Kim et al., 2019).
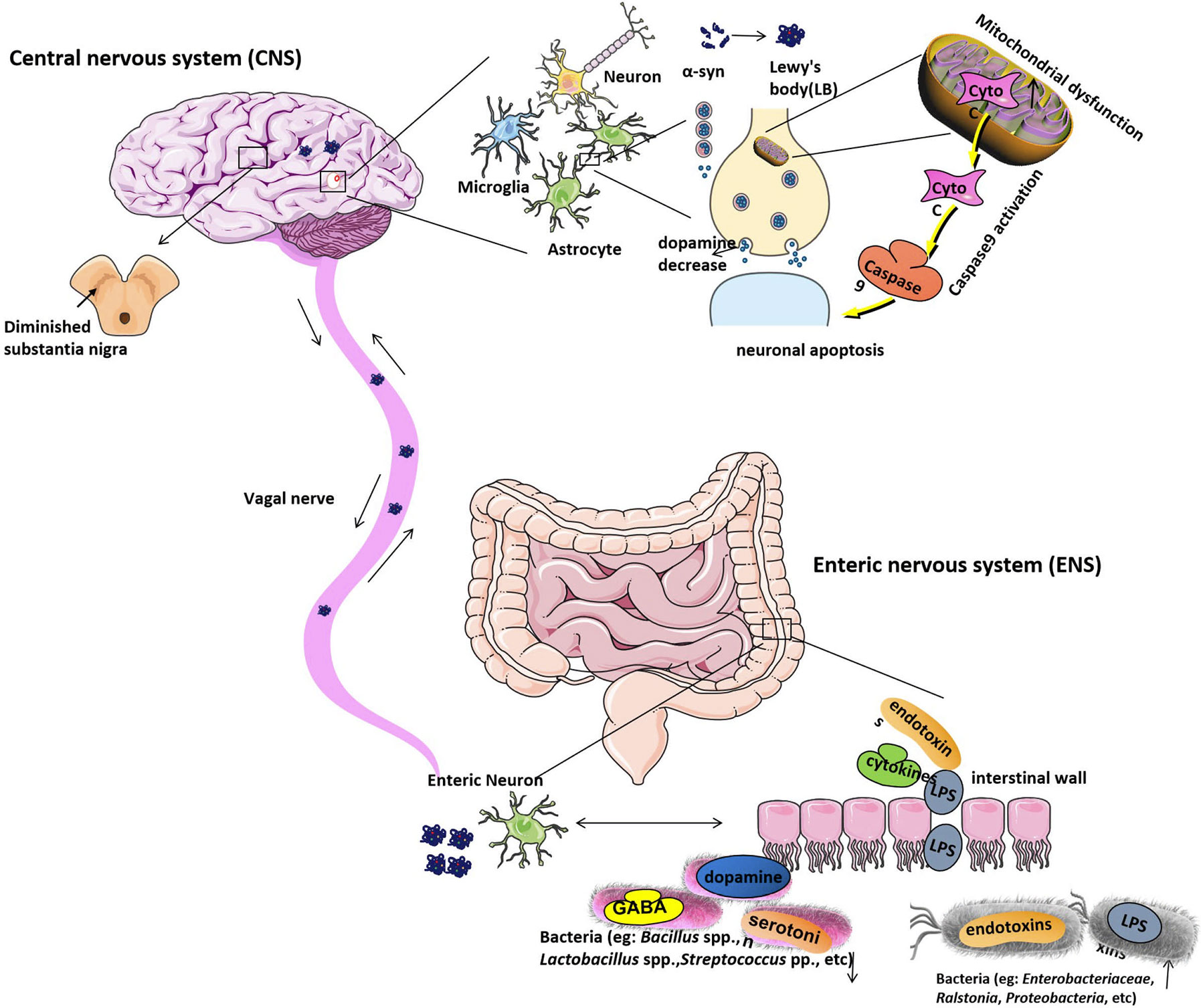
Figure 1 The etiological mechanism of Parkinson’s disease. Gut microbiota dysbiosis leads to increased intestinal permeability and systemic exposure of bacterial endotoxins, thereby initiating excess α-syn expression and supporting its misfolding to form LBs. The intestinal LBs from ENS will reach the CNS through the vagal nerve and eventually move to and damage the substantia nigra, which will lead to the appearance of the clinical symptoms of PD. The α-syn protein is generally expressed in the CNS with a function of modifying the supply and release of dopamine to regulate neurotransmission in the brain, while, in PD patients’ brains, α-syn protein is overexpressed and forms the LBs will cause dopamine release decreased. Moreover, LBs are the most toxic species in the brain. Mitochondrial dysfunction can be found under pathological conditions. Apoptotic signals are transmitted to the mitochondria, causing the release of Cyto C, which located in the intermembrane of mitochondria. Cyto C activates caspase9, causing protein hydrolysis and eventually leading to neuronal apoptosis. Under normal physiological conditions, neuronal cells have a highly resistant ability to apoptosis. However, when the conditions are pathological, their auto-apoptosis can occur abnormally and cause DA neurons degeneration. Bacteria, including Enterobacteriaceae, Ralstonia, Proteobacteria, etc. are increased in PD stool samples, which will raise the serum lipopolysaccharide (LPS) and other endotoxins concentration. And bacteria like Bacillus spp., Lactobacillus spp., Streptococcus spp. that can produce neurotransmitters such as gamma-aminobutyric acid (GABA), serotonin, and dopamine separately are decreased in PD stool samples. LB, Lewy’s body; CNS, central nervous system; ENS, Enteric nervous system; PD, Parkinson’s disease; Cyto C, Cytochrome C; DA, dopamine.
Since α-syn can be transmitted between brain and intestine which gives us a new option that we can modify the gut microbiota to alter the system of the intestine and reduce concurrent mucosal inflammation, eventually reduce the symptoms of PD. Nowadays, it is clear that certain bacteria are strain-specific that they can produce different essential neurotransmitters and specific neuromodulators. It is reported that several neurotransmitters such as gamma-aminobutyric acid (GABA), noradrenaline, serotonin, dopamine, and acetylcholine are produced by gut microbiota in human beings. For example, researchers reported that Lactobacillus spp., Bifidobacterium spp. (Y2) and Streptococcus salivarius subsp. thermophilus can produce GABA (Barrett et al., 2012; Pokusaeva et al., 2017). Escherichia spp., Saccharomyces spp., and Bacillus spp. can produce noradrenaline (Shishov et al., 2009; Rogers et al., 2016); Streptococcus pp., Candida spp., Enterococcus spp., and Escherichia spp. produce serotonin (Özoğul, 2004; Shishov et al., 2009; Özoğul et al., 2012); Bacillus spp.(Bacillus cereus, Bacillus mycoides, Bacillus subtilis), Escherichia coli (K-12), Hafnia alvei (NCIMB, 11999), Klebsiella pneumoniae (NCIMB, 673), Morganella morganii (NCIMB, 10466), Proteus vulgaris, Serratia marcescens, and Staphylococcus aureus can produce dopamine (Özoğul, 2004; Shishov et al., 2009; Rogers et al., 2016); and Lactobacillus spp. produce acetylcholine (Rogers et al., 2016). The neurotransmitters secreted by the intestinal lumen bacteria may induce epithelial cells to release molecules that, in turn, can regulate neural signaling in the ENS and subsequently control brain function and behavior through the brain-gut axis. In some animal studies, various bacterial strains also mediate behavioral effects through the vagus nerve (Reid, 2019). Other important molecules produced in the colon by microbial fermentation of dietary fiber are short-chain fatty acids (SCFAs), such as butyrate, acetate, and propionate. SCFAs can regulate intestinal inflammation and immune function; they can be detected in the blood circulation system and also across the blood-brain barrier (BBB) via monocarboxylate transporters to affect the CNS system. SCFAs can promote microglia-mediated neuroinflammation (Sampson et al., 2016). For example, the administration of high doses of propionate in rats can cause neurasthenia (den Besten et al., 2013). Therefore, intestinal microorganisms and their products play important roles in improving the symptoms and pathogenesis of PD.
The Current Treatments for PD
Current treatments for PD either increase/replace DA, or prevent the breakdown of DA, or prolong the action of levodopa to help control tremors (Isaacson and Hauser, 2009). Medications and surgery have been used to treat PD, but both have moderate side effects and often produce disappointing results. We will cover the current treatments related to PD and also discuss the side effects of different treatments (Table 1).
Medications Increase or Substitute for Dopamine
Since DA cannot enter into the brain, it cannot be given directly to treat PD. Medications increase or substitute for DA can be used to treat the PD.
Carbidopa-Levodopa
Levodopa can pass into the brain and be converted to DA to treat PD (Haddad et al., 2018) and carbidopa can prevent levodopa breakdown. Side effects may happen, including lightheadedness (orthostatic hypotension) (Lau et al., 2018) or nausea (Kell et al., 2017). As the disease progresses after years, the effect of levodopa becomes less stable, and a tendency to wane and dyskinesia may appear in PD (Tran et al., 2018).
Duopa Therapy
Duopa is a form of carbidopa/levodopa delivered in gel form. It delivers the medication directly to the small intestine in the gel form through a feeding tube (LeWitt, 2016). A pump slowly and consistently delivers Duopa to the intestine through the tube. This procedure allows the medicine to be absorbed smoothly and reduces motion fluctuations and movement disorders (Chaudhuri et al., 2016). The Duopa therapy has the risks that the tube may fall out or infections may happen at the infusion site or a blockage occurring in the tube.
Dopamine Agonists
DA agonists mimic DA effects in the brain, the effective time is longer than levodopa (Grall-Bronnec et al., 2018). Short-acting injectable DA agonists such as Requip, Mirapex, and Neupro can be used for quick relief in PD treatment (Pahwa and Lyons, 2009; Kulisevsky and Pagonabarraga, 2010; Yu and Fernandez, 2017). Apomorphine is a DA agonist that can be delivered by intermittent subcutaneous injections to treat the fluctuations in motor symptoms of PD (Antonini and Nitu, 2018). Side effects including hallucinations, sleepiness, and compulsive behaviors can be found (Patel et al, 2017).
MAO B Inhibitors
The brain enzyme monoamine oxidase B (MAO B) metabolizes the brain DA (Tabakman et al., 2004). MAO B inhibitors can prevent the breakdown of brain DA by inhibiting MAO B enzyme activities (Finberg, 2019). These MAO B inhibitors include rasagiline, safinamide, and selegiline (Deshwal et al., 2017; Dezsi and Vecsei, 2017; Binde et al., 2018; Szökő et al., 2018). Side effects including nausea or insomnia may happen (Dezsi and Vecsei, 2017).
Catechol O-methyltransferase (COMT) Inhibitors
COMT inhibitors mildly prolong the effect of levodopa by blocking an enzyme that can break down DA (Schlesinger and Korczyn, 2016; Katsaiti and Nixon, 2018). The medication from this class mainly includes Comtan and Tasmar (Olanow and Watkins, 2007; Lees, 2008). This medicine has a risk of serious liver damage and liver failure, other side effects include diarrhea or increased risk of dyskinesia (Silva et al., 2020).
Anticholinergics
Anticholinergics including Cogentin and trihexyphenidyl were used to control the tremor associated with PD (Olanow et al., 2001; Nishtala et al., 2016; Mishima et al., 2018). It is reported that they work better on tremors than on other PD characteristics (Lang and Lees, 2002). They are common side effects such as impaired memory, hallucinations, confusion, constipation, dry mouth, and impaired urination (Morrow et al., 2018; Hong et al., 2019).
Amantadine
It can provide short-term relief of mild symptoms. It can be used during the later stages of PD by giving together with carbidopa-levodopa therapy to control involuntary movements (Wolf et al., 2010). Side effects of amantadine may include ankle swelling, skin purple mottling, or hallucinations (Kim et al., 2018).
Creatine
Creatine is an energy compound that exerts neuroprotective effects in animal models of PD (Duarte‐Silva et al., 2018; Marques and Wyse, 2019). It also acts as an antioxidant protected against the loss of both Nissl and tyrosine hydroxylase in the substantia nigra (Xiao et al., 2014). Weight gain is the most common side effect of creatine, impairment of renal function can also be found (Bender et al., 2008).
Surgical Procedures
Deep Brain Stimulation
DBS is offered to people with advanced PD (Lee et al., 2018). DBS stabilizes medication fluctuations, reduces or prevents dyskinesias, reduces tremors and stiffness, and improves movement slowness. In deep brain stimulation (DBS), a surgeon first implants electrodes into a specific part of the patients’ brain. Then the electrodes are connected to a generator implanted in the chest near the patient’s clavicle (Follett et al., 2010). Risks such as infections, brain hemorrhage, or stroke may happen.
Gene Therapy
A lot of PD gene therapy clinical trials aim to alter local neurotransmitters or neurotrophic factors in the basal. Although these trials show that gene therapy can be safely delivered to the brain and induce specific neuronal protein expression, the clinical results have been less encouraging (Bartus et al., 2014).
Immunotherapy
Immunotherapy targeted mainly using antibodies against misfolded α-syn (George and Brundin, 2015). Previous studies have tried to remove α-syn from extracellular space, thereby reducing the progressive deposition of α-syn aggregates throughout the brain (Masliah et al., 2005; Masliah et al., 2011). A possible side effect of immunotherapy is Th17 cell-mediated inflammatory autoimmunity involving in neurodegenerative neuritis (Reynolds et al., 2010).
Cell Transplantation
Introducing new DA cells into the brain may help replace what is lost in PD. To date, there have been cell transplantation clinical trials using autologous and nonautologous cells, including the use of the human embryonic stem cells (ESCs) and induced pluripotent stem cells (iPS) (Parmar et al., 2020). The Japanese scientists have injected dopaminergic progenitor cells directly into an area of the brain associated with neural degeneration in PD in 2018 (Normile, 2018). The main challenge has been unacceptable graft-induced dyskinesia (Piquet et al., 2012).
Microbiota-Targeted Intervention Strategies to Manage PD
Nowadays, it has been estimated that the human intestinal tract harbors a diverse and complex microbial community which plays an important role in many aspects of host physiology, including nervous system development and human neurodegenerative diseases (Thomas et al., 2017; Ceppa et al., 2020). The intestinal flora is currently considered a key regulator of a smooth two-way dialogue between the intestine and the brain (gut-brain axis). This fact provides a promising opportunity for preventing or treating neuropsychiatric conditions in PD. The relationship between gut flora and the brain can be traced back to brain development. After the fetus is born, the microorganisms obtained from the mother and the environment colonize the fetus’s intestine and play a critical role in brain development (Codagnone et al., 2019). The functions of gut microbiota include participation in the synthesis of multiple vitamins and fatty acids, and regulation of brain-derived neurotrophic factor (BDNF), synaptophysin, post-synaptic density protein 95 (PSD-95) (Sudo et al., 2004). A recent study has shown that injections of LBs from PD into the striatum of baboons or the intestine could induce the damage of the nigrostriatal pathway and the pathological changes of the ENS. No pathological damage of α-syn was detected in the vagus nerve and the DMV, suggesting that DMV may not be the pathologic transmission route of α-syn. The levels of α-syn in the blood of baboons injected were increased, which was positively correlated with the levels of α-syn in ENS. Endogenous α-syn may be transmitted long-distance and bidirectional between ENS and the brain through the circulatory system (Arotcarena et al., 2020).
It has been found in sterile animal research that the intestinal flora is necessary for the healthy development of the nervous system, and the nervous system function is challenging to mature sterile animals because of the lack of intestinal flora (Luczynski et al., 2016). It was found that compared with normal mice, the expression of BDNF in the cerebral cortex and hippocampus of sterile mice was significantly reduced (Arentsen et al., 2015). And sterile mice were more likely to show anxiety and less activity performance. After transplanting the healthy intestinal flora to sterile mice, it was showed increased activity and decreased anxiety in mice, and the 5-HT content of norepinephrine, DA, and terminal brain striatum also significantly increased (De Theije et al., 2014). The anxious behaviors and activities of SPF mice indicated that the colonization rate of the GI flora during colonization could affect the corresponding excitatory neuron cell signaling mechanism to some extent.
The Association between Gut Microbiota Alteration and PD
There are associations between the composition of gut microbiota alteration and multiple prodromal markers of PD. Several studies have proven that certain bacterial taxa can be used as biomarkers or even drug targets for PD. A study showed that gut microbiota dysbiosis was observed in the PD compared to the healthy group. OTUs include Proteus sp., Bilophila sp., and Roseburia sp., were increased with PD microbiomes and members of families Lachnospiraceae, Rikenellaceae, and Peptostreptococcaceae, as well as Butyricicoccus sp. were decreased (Scheperjans et al., 2015). Another study was supporting that compared to the healthy control, the levels of Lactobacillus, Prevotellaceae, Peptostreptococcus, and Butyricicoccus spp. are lower and the levels of Proteus and Enterobacter spp. are higher (Sampson et al., 2016). A recent clinical trial including 666 elderly subjects was done to analyze the association between PD risk factors and prodromal symptoms markers with the composition of gut microbiota. The physical activity, occupation-related solvent exposure, and constipation were associated with the α-diversity of gut microbiota, and the physical activity, gender, constipation, REM sleep behavior disorder (RBD), as well as smoking, are associated with β-diversity of gut microbiota, the age and uric acid-lowering drugs are associated with both α- and β-diversity of gut microbiota. Physical inactivity and constipation in individuals were highest common with Firmicutes-enriched enterotype, while constipation is the least common among individuals with Prevotella-enriched enterotype (Heinzel et al., 2020). Another study showed Ralstonia, Proteobacteria, Enteococcaceae concentration in the mucosa of PD patients increased. These bacteria have pro-inflammatory cytokine producing function. Anti-inflammatory bacteria including Blautia, Coprococcus, Roseburia, and Faecalibacterium in the stool samples of PD patients decreased (Keshavarzian et al., 2015) The LPS (lipopolysaccharide) biosynthesis genes were also reported significantly increased in the PD fecal samples (Keshavarzian et al., 2015). Helicobacter pylori infection is also related to trigger the pathogenesis in PD (Çamcı and Oğuz, 2016). A two-year following study showed that low counts of Bacteroides fragilis were related to worsening of motivation/activeness and Bifidobacterium was associated with hallucinations/delusions (Minato et al., 2017).
A study used the microbiome-wide association study (MWAS) in two large datasets to specify the gut microbiota alteration in PD. Cluster 1 which was composed of opportunistic pathogens including Porphyromonas, Corynebacterium, Prevotella, Porphyromonas, and Corynebacterium were increased in PD. Genera in Cluster 2 including (Oscillospira, Lachnospiraceae_UCG-004, Lachnospiraceae_ND3007_group) and (Agathobacter, Butyricicoccus, Blautia, Faecalibacterium, Lachnospira, Fusicatenibacter, Roseburia) were reduced in PD. Most increased groups belong to Ruminococcaceae and Lachnospiraceae families which are already known as SCFAs producing bacteria. Lactobacillus and Bifidobacteria increased in PD in cluster 3. The genera in cluster 3 were probiotics with carbohydrate-metabolizing and possible of becoming opportunistic pathogens and immunogenic (Wallen et al., 2020). Nishiwaki et al. use a meta-analysis method compared 223 PD patients with 137 health controls and give a conclusion that genera Akkermansia, Catabacter, and families Akkermansiaceae were elevated, while Roseburia, Faecalibacterium, and Lachnospiraceae ND3007 group were decreased in PD (Nishiwaki et al., 2020). When the dietary fibers defected, Akkermansia muciniphila can degrade the gut mucus layer and enhance enteric pathogen infection risk (Desai et al., 2016). Abundance Akkermansia can increase the permeability of intestine which exposes the intestinal neural plexus to an oxidative or toxic environment, and this may lead to α-syn fibrils aggregate in the intestine. Faecalibacterium and Roseburia decreased in PD may provoke intestinal inflammatory, these two genera are butyrate-producing bacteria and butyrate belongs to SCFAs can induce anti-inflammatory cytokines gene expression by inhibiting histone deacetylase (Sokol et al., 2008; Canani et al., 2011). Cirstea also proved the intestinal function of PD is related to gut microbiota composition and metabolism (Cirstea et al., 2020). The microbiota composition of fecal samples as well as serum metabolomics were analyzed from 197 PD patients and 103 controls. There is a higher abundance of Christensenellaceae, Desulfovibrionaceae, Bifidobacterium, Bilophila, Collinsella, Akkermansia and lower abundance of Lachnospiraceae, Roseburia, Faecalibacterium in PD. The microbiota in PD showed reduced carbohydrate fermentation and low butyrate synthesis capacity, while the proteolytic fermentation and deleterious amino acid metabolites (p-cresol and phenylacetylglutamine) production were increased. The interesting phenomenon is that butyrate-producing bacteria were negatively associated with stool firmness since butyrate can regulate intestinal serotonin biosynthesis and improve the motility of colonic (Vincent et al., 2018; Cirstea et al., 2020). The SCFAs concentrations were significantly reduced in PD fecal samples. The Bacteroidetes (phylum) and Prevotellaceae (family) were reduced, and Enterobacteriaceae increased in PD. SCFAs may induce ENS alterations and dysmotility of gastrointestinal in PD (Unger et al., 2016). From all these studies, we could conclude that the opportunistic pathogens were increased, while potential benefit bacteria were reduced: Prevotellace decreased and Enterobacteriaceae increased in PD. The microbiome changed in PD is shown in Table 2.
The interplay between α‐syn and gut microbiota attracts a lot of researchers’ interest. A previous study has already confirmed that increased expression of α‐syn in the substantia nigra can cause pathology of CNS, including motor and cognitive functions impaired (Crowley et al., 2018). Nigral overexpression of α‐syn reduced neuronal number in myenteric submucosal plexus, increased glial expression in the myenteric plexus, modulated myenteric and submucosal TH (tyrosine hydroxylase) intensity, alter gut microbiota as well as bile acid composition (O’Donovan et al., 2020). Plexus neuronal loss can affect epithelial barrier integrity, secretomotor functions and immune cell migration, which can increase the permeability of intestine and GI inflammation. Potentially beneficial bacteria Faecalibacterium prausnitzii, Prevotellaceae, and Lactobacillaceae were reduced in PD, the abundance of Enterobacteriaceae was increased. Nigral overexpression of α‐syn increased the level of fecal free bile acids. The distributions of bile acid indicate the liver synthesis increased or transporter deficiencies and reabsorption of bile acid in the small intestine also reduced. CA (cholic acid) and DCA (deoxycholic acid) have a role in cognitive decline (MahmoudianDehkordi et al., 2019). Increased DCA levels can inhibit the motility of GI. The DCA level were significant positive correlations with Ruminococcaceae and significantly negatively with Lactobacillus (O’Donovan et al., 2020). Gorecki used a Thy1-αSyn PD mice model, and found LPS can induce inflammation and alter the distribution of tight junction proteins. The mucin-degrading Verrucomicrobiae and LPS-producing Gammaproteobacteria were increased in PD patients. LPS administration leads to the increasing of intestinal permeability, motor impairment, nigral α‐syn aggregation, dopaminergic neuronal loss and reduction in striatal dopamine. So LPS-producing bacteria increasing can change the gut environment and trigger the pathogenesis of PD by α‐syn aggregation (Gorecki et al., 2019). LPS or inflammatory endotoxin modulate α‐syn amyloidogenesis by the formation of intermediate nucleating species. LPS-binding structural motif interacts with soluble monomer stabilizes the α-helical intermediates in the α-syn aggregation pathway. By saturation, transfer LPS can mediate the nucleation probe. Finally, the nucleating intermediates mediated by LPS mature into divergent fibrillary forms. LPS-induced can alter the backbone motility of α‐syn, modulate α-syn aggregation, and increase LPS-α‐syn fibril formation which is toxic in PD (Bhattacharyya et al., 2019). Thus gut microbiota plays an important role in the pathology of PD.
Microbial Regulation of Host Neuronal System
The Prevotellaceace family members are important mediators of host nutrition. They can ferment complex polysaccharides to product SCFAs and modify bile acids through dietary metabolism. (Arumugam et al., 2011). The SCFAs receptors 2 (FFAR2) and 3 (FFAR3) were found expressed in the ENS, portal nerve and sensory ganglia system. The microbiota metabolites can directly function to sensory neurons or can signal to neurons via intermediate interactions with enteroendocrine or epithelial cells and regulate the host behavior (Egerod et al., 2018). The Prevotellaceae decreased which lead to intestinal permeability increased and bacterial endotoxins exposure (Figure 1), thereby initiating or retaining excess α-syn expression in the colon and supporting its misfolding (Sarkar and Banerjee, 2019). The increased Enterobacteriaceae in PD can raise the serum LPS concentration and the relative abundance of the Enterobacter spp. is also positively correlated with the severity of posture instability and gait difficulties of PD patients (Lin et al., 2019). LPS is derived from the gram-negative bacteria cell walls and crosses the intestinal wall then enter into the bloodstream and result in intestinal epithelial barrier disruption (Guo et al., 2013). LPS in the bloodstream may induce systemic inflammation (Tufekci et al., 2011), LPS as well as inflammatory cytokines like tumor necrosis factor (TNF-α), interleukin (IL)-1β, and IL-6 can disrupt BBB and promotes α-syn misfolding (Block et al., 2007), which lead to the destruction of DA neurons in the substantia nigra (Rite et al., 2007). Thus the overgrowth of Enterobacter spp. are correlated with the progression of PD (Mulak and Bonaz, 2015; Nair et al., 2018).
The approach that gut microbial interventions can be used to treat PD is supported by the fact that the gut microbiota can directly produce neurotransmitters or regulate the host biosynthesis of neurotransmitters (Bested et al., 2013), as shown in Table 3. But what are the functions of the neurotransmitters in gut microbiota and how they function on the host neuronal system is still not clear. A recent study shows that the Bacteroides fragilis can synthesis GABA and supported the KLE1738 growth, which means the GABA may be served as the growth substrate for KLE1738 (Strandwitz et al., 2019). Another study showed the 5-HT can increase the colonization rate of Turicibacter sanguinis, suggesting the role of neurotransmitter modulate bacterial colonization in the gut (Fung et al., 2019). Almost half of the host dopamine is produced by gut microbiota and up to 60% of colonic and blood 5-HT levels are biosynthesis by gut microbiota (Yano et al., 2015). Microbiota modulates the 5-HT activates in the intestine and increase the motility of GI. Microbiota also can regulate the local 5-HT to impact the central nervous system, an increasing number of researches report that the microbiome affects the host neuronal system (Sgritta et al., 2019). In the future, the microbiota specific functions on the neurological disorders and use the microbiota as the potential medical treatment for PD are needed to assess.
Gut Microbiota Approach to Treat PD
Antibiotics
Koutzoumis et al. test broad-spectrum antibiotics function on oxidopamine injected rat PD model and found 90% of microbial richness was reduced. The level of Firmicutes was reduced, while Proteobacteria, Verrucomicrobia, Bacteroidetes, and Cyanobacteria increased. Antibiotics treatment can decrease striatum IL-1b and TNF-α levels, protect dopaminergic neuron cell loss and alleviate motor deficits in the PD rodent model (Pu et al., 2019; Koutzoumis et al., 2020).
Probiotics
Probiotics treatment has been proven to be a useful method to improve the PD. Probiotics strain bifidobacteria and lactobacilli have been reported to reverse PD conditions. The regular intake of fermented milk beverages containing the probiotic Lactobacillus casei shirota has been shown to improve the bowel movement and inhibit staphylococci growth in PD patients (Cassani et al., 2011). Probiotic bacterium Bacillus spp. can convert L-tyrosine to L-DOPA, which can supply the lost dopamine of PD (Surwase and Jadhav, 2011). Some bacteria in the gut can convert levodopa to dopamine through tyrosine decarboxylases(TDC). TDC has been identified in the genome of more than 50 Enterococcus strains, several Lactobacillus and Staphylococcus, which are potential probiotics of the small intestine (Zoetendal et al., 2012; van Kessel et al., 2019). Mediterranean diet (MeDi) which contains a large quantity of Lactobacilli is shown have effective in preventing Alzheimer’s disease, several clinical studies also show that higher MeDi adherence was associated with reduced odds for PD (Alcalay et al., 2012). A study showed that pretreatment with a probiotic mixture containing B. animalis lactis, L. rhamnosus GG, and L. acidophilus has neuroprotective effects in PD models. Possibility because of butyrate can induce the BDNF and glial cell line-derived neurotrophic factor (GDNF) upregulated, and monoamine-oxidase was inhibited in the brain. Furthermore, probiotics mixture pretreatment can reduce DA neurons loss, increase the level of DA and reduce the activity of inflammatory cells of brain (Srivastav et al., 2019).
Prebiotics
Butyrate produced from bacteria is likely an interesting candidate for PD treatment. Butyrate can induce Atg5- and PI3K/Akt/mTOR-related autophagy way to cause α-syn degradation in a pesticide-induced PD rat model. The abundance of butyrate-producing bacteria elevated in the gut can prevent intestinal barrier dysfunction and increase striatal DA levels (Qiao et al., 2020).
Fecal Microbiota Transplantation (FMT)
FMT has a 1700-year history and was proposed to treat human GI diseases (Zhang et al., 2012). Currently, there are multiple ways to modulate gut microbiota, including antibiotics, probiotics, and prebiotics. Moreover, FMT remains an effective method to restore the gut microbiota ecosystem. FMT including screening for a specific microbial population, homogenizing, filtering, and resuspending stool samples, followed by colonoscopy, enema, orogastric tube, or oral delivery in the form of capsules containing lyophilized material (Biagi et al., 2013). Besides PD, FMT has been used to treat various diseases, such as Irritable bowel syndrome (IBS), type 2 diabetes, ulcerative colitis, and neurodegenerative diseases (Glass et al., 2010). Patients with PD often suffer from changes in GI motility. For example, chronic or idiopathic constipation is often found as a co-comorbid condition in PD patients and is associated with colonic and anorectal dyskinesias (Abbott et al., 2007; Mertsalmi et al., 2017; Yu et al., 2018). Several studies have shown that FMT is beneficial for the treatment of constipation in PD and can also significantly improve the non-GI symptoms of patients with neurological diseases (Sun et al., 2018; Huang et al., 2019). The discovery of the gut microbiota regulatory mechanism of PD pathogenesis has been highly valued (Borody and Khoruts, 2012; Cryan and Dinan, 2012). The proposed approach to evaluate FMT as a potential treatment for PD is primarily to assess direct communication of the vagus nerve, changes in neurotransmitter metabolites, activation of immune responses, and production of neuroactive metabolites as well as neurotoxins (Aroniadis and Brandt, 2013). A recent study using a PD mouse model as a recipient found that fecal transplantation from PD patients exacerbates dyskinesias and is associated with a decrease in Lachnospiraceae and Ruminococceae, which is a genus significantly reduced from PD patients’ stool samples (Keshavarzian et al., 2015). Besides, compared with healthy controls, FMT from PD patients may exacerbate α-syn-related motor dysfunction in α-syn overexpressing mice (Sampson et al., 2016). When transferring the gut microbiota from PD mice to normal mice, striatal neurotransmitter decreasing and motor impairment can be observed in normal mice. In fecal samples of PD, several changes can be observed: fecal SCFAs concentrations were significantly increased, the number of bacteria Proteobacteria, Turicibacterales and Enterobacteriales increased, while Firmicutes and Clostridiales decreased. FMT can suppress the TLR4/TNF-α signaling pathway which is involved in inflammation of the gut and brain. Finally, FMT administration can improve the gut dysbiosis, decrease fecal SCFAs concentration, increase DA and 5-HT levels, reduce activation of microglia and astrocytes in the substantia nigra, restore motor impairment of PD (Sun et al., 2018). Several clinical cases of PD have shown that FMT treatment can reduce symptoms of co-morbid GI, including bowel disorders, constipation, and ulcerative colitis. Compared to the traditional PD treatment methods mentioned in table 1, FMT has fewer side effects. In future, FMT treatment may also help relieve several non-GI comorbid disorders and provide additional support for the association between gut microbiota and PD. Possible microbiota-targeted intervention strategies can improve health status and prevent PD in the near future.
Conclusion
The most possible conclusion about the connection between gut microbiota and PD is that: The GI dysfunction could be found in the early stage of PD, α-syn was found in both the gut and brain. The gut disorder exacerbates α-syn deposition and will aggravate neurodegeneration. α-syn deposition may start in the ENS of PD, then accumulate and transfer to the CNS via a trans-synaptic cell-to-cell transmission (Lionnet et al., 2018). The imbalance of the gut tract shows a pro-inflammatory environment, the number of the pathogen was elevated, the permeability of the intestinal epithelial barrier also increased. The inflammatory signals could be transferred to the brain through the gut-brain axis and cause brain & behavior dysfunction.
Gut microbiota has been shown as the potential modulator of human health. They play an important role in the intestine system and brain function. Current studies indicate that modify gut microbiota composition can affect brain neurochemistry via neural, immune and endocrine. Through antibiotics, probiotics, prebiotics or FMT approach could restore the gut ecosystem and improve brain functions. In the future, more new GI biomarkers need to discovery and the mechanism of specific bacteria through which pathway effect on the host system needs to be clarified.
Author Contributions
JL contributed in manuscript writing. FX and ZN critically reviewed the manuscript. LS supervised the whole process and reviewed the manuscript. All authors contributed to the article and approved the submitted version.
Funding
This research was financially supported by the National Natural Science Foundation of China (81773616), Shanghai Excellent Technology Leader Program (17XD1423200), Shanghai Education Development Project: Industry-University-Research Practice (A3-2601-20-311001-11), and the Natural Science Foundation of Shanghai (20ZR1424600).
Conflict of Interest
The authors declare that the research was conducted in the absence of any commercial or financial relationships that could be construed as a potential conflict of interest.
References
Abbott R. D., Ross G. W., Petrovitch H., Tanner C. M., Davis D. G., Masaki K. H., et al. (2007). Bowel movement frequency in late-life and incidental Lewy bodies. Mov. Disord. 22, 1581–1586. doi: 10.1002/mds.21560
Adler C. H., Connor D. J., Hentz J. G., Sabbagh M. N., Caviness J. N., Shill H. A., et al. (2010). Incidental Lewy body disease: clinical comparison to a control cohort. Mov. Disord. 25, 642–646. doi: 10.1002/mds.22971
Alcalay R. N., Gu Y., Mejia-Santana H., Cote L., Marder K. S., Scarmeas N. (2012). The association between Mediterranean diet adherence and Parkinson’s disease. Mov. Disord. 27, 771–774. doi: 10.1002/mds.24918
Angot E., Steiner J. A., Tomé C. M. L., Ekström P., Mattsson B., Björklund A., et al. (2012). Alpha-synuclein cell-to-cell transfer and seeding in grafted dopaminergic neurons in vivo. PLoS One 7, e39465. doi: 10.1371/journal.pone.0039465
Anselmi L., Bove C., Coleman F., Le K., Subramanian M., Venkiteswaran K., et al. (2018). Ingestion of subthreshold doses of environmental toxins induces ascending Parkinsonism in the rat. NPJ Parkinson’s Dis. 4, 1–10. doi: 10.1038/s41531-018-0066-0
Antonini A., Nitu B. (2018). Apomorphine and levodopa infusion for motor fluctuations and dyskinesia in advanced Parkinson disease. J. Neural Transm. 125, 1131–1135. doi: 10.1007/s00702-018-1906-0
Arentsen T., Raith H., Qian Y., Forssberg H., Heijtz R. D. (2015). Host microbiota modulates development of social preference in mice. Microbial. Ecol. Health Dis. 26, 29719. doi: 10.3402/mehd.v26.29719
Aroniadis O. C., Brandt L. J. (2013). Fecal microbiota transplantation: past, present and future. Curr. Opin. Gastroenterol. 29, 79–84. doi: 10.1097/MOG.0b013e32835a4b3e
Arotcarena M. L., Dovero S., Prigent A., Bourdenx M., Camus S., Porras G., et al. (2020). Bidirectional gut-to-brain and brain-to-gut propagation of synucleinopathy in non-human primates. Brain 143, 1462–1475. doi: 10.1093/brain/awaa096
Arumugam M., Raes J., Pelletier E., Le Paslier D., Yamada T., Mende D. R., et al. (2011). Enterotypes of the human gut microbiome. Nature 473, 174–180. doi: 10.1038/nature09944
Barrett E., Ross R., O’toole P., Fitzgerald G., Stanton C. (2012). γ-Aminobutyric acid production by culturable bacteria from the human intestine. J. Appl. Microbiol. 113, 411–417. doi: 10.1111/j.1365-2672.2012.05344.x
Bartus R. T., Weinberg M. S., Samulski R. J. (2014). Parkinson’s disease gene therapy: success by design meets failure by efficacy. Mol. Ther. 22, 487–497. doi: 10.1038/mt.2013.281
Bellucci A., Antonini A., Pizzi M., Spano P. (2017). The end is the beginning: Parkinson’s disease in the light of brain imaging. Front. Aging Neurosci. 9, 330. doi: 10.3389/fnagi.2017.00330
Bender A., Samtleben W., Elstner M., Klopstock T. (2008). Long-term creatine supplementation is safe in aged patients with Parkinson disease. Nutr. Res. 28, 172–178. doi: 10.1016/j.nutres.2008.01.001
Bested A. C., Logan A. C., Selhub E. M. (2013). Intestinal microbiota, probiotics and mental health: from Metchnikoff to modern advances: Part I–autointoxication revisited. Gut Pathog. 5, 5. doi: 10.1186/1757-4749-5-5
Bhattacharyya D., Mohite G. M., Krishnamoorthy J., Gayen N., Mehra S., Navalkar A., et al. (2019). Lipopolysaccharide from gut microbiota modulates α-synuclein aggregation and alters its biological function. ACS Chem. Neurosci. 10, 2229–2236. doi: 10.1021/acschemneuro.8b00733
Biagi E., Candela M., Turroni S., Garagnani P., Franceschi C., Brigidi P. (2013). Ageing and gut microbes: perspectives for health maintenance and longevity. Pharmacol. Res. 69, 11–20. doi: 10.1016/j.phrs.2012.10.005
Binde C. D., Tvete I. F., Gåsemyr J., Natvig B., Klemp M. (2018). A multiple treatment comparison meta-analysis of monoamine oxidase type B inhibitors for Parkinson’s disease. Br. J. Clin. Pharmacol. 84, 1917–1927. doi: 10.1111/bcp.13651
Block M. L., Zecca L., Hong J.-S. (2007). Microglia-mediated neurotoxicity: uncovering the molecular mechanisms. Nat. Rev. Neurosci. 8, 57–69. doi: 10.1038/nrn2038
Borody T. J., Khoruts A. (2012). Fecal microbiota transplantation and emerging applications. Nat. Rev. Gastroenterol. Hepatol. 9, 88. doi: 10.1038/nrgastro.2011.244
Braak H., Del Tredici K., Rüb U., De Vos R. A., Steur E. N. J., Braak E. (2003a). Staging of brain pathology related to sporadic Parkinson’s disease. Neurobiol. Aging 24, 197–211. doi: 10.1016/S0197-4580(02)00065-9
Braak H., Rüb U., Gai W., Del Tredici K. (2003b). Idiopathic Parkinson’s disease: possible routes by which vulnerable neuronal types may be subject to neuroinvasion by an unknown pathogen. J. Neural Transm. 110, 517–536. doi: 10.1007/s00702-002-0808-2
Braak H., De Vos R. A., Bohl J., Del Tredici K. (2006). Gastric α-synuclein immunoreactive inclusions in Meissner’s and Auerbach’s plexuses in cases staged for Parkinson’s disease-related brain pathology. Neurosci. Lett. 396, 67–72. doi: 10.1016/j.neulet.2005.11.012
Bridi J. C., Hirth F. (2018). Mechanisms of α-synuclein induced synaptopathy in Parkinson’s disease. Front. Neurosci. 12, 80. doi: 10.3389/fnins.2018.00080
Burré J. (2015). The synaptic function of α-synuclein. J. Parkinson’s Dis. 5, 699–713. doi: 10.3233/JPD-150642
Calo L., Wegrzynowicz M., Santivañez-Perez J., Grazia Spillantini M. (2016). Synaptic failure and α-synuclein. Mov. Disord. 31, 169–177. doi: 10.1002/mds.26479
Çamcı G., Oğuz S. (2016). Association between Parkinson’s disease and Helicobacter pylori. J. Clin. Neurol. 12, 147–150. doi: 10.3988/jcn.2016.12.2.147
Caminiti S. P., Presotto L., Baroncini D., Garibotto V., Moresco R. M., Gianolli L., et al. (2017). Axonal damage and loss of connectivity in nigrostriatal and mesolimbic dopamine pathways in early Parkinson’s disease. NeuroImage: Clin. 14, 734–740. doi: 10.1016/j.nicl.2017.03.011
Canani R. B., Di Costanzo M., Leone L., Pedata M., Meli R., Calignano A. (2011). Potential beneficial effects of butyrate in intestinal and extraintestinal diseases. World J. Gastroenterol.: WJG 17, 1519. doi: 10.3748/wjg.v17.i12.1519
Cani P. D., Everard A., Duparc T. (2013). Gut microbiota, enteroendocrine functions and metabolism. Curr. Opin. Pharmacol. 13, 935–940. doi: 10.1016/j.coph.2013.09.008
Cassani E., Privitera G., Pezzoli G., Pusani C., Madio C., Iorio L., et al. (2011). Use of probiotics for the treatment of constipation in Parkinson’s disease patients. Minerva gastroenterologica e Dietologica 57, 117–121. doi: 10.1109/IFOST.2011.6021087
Ceppa F. A., Izzo L., Sardelli L., Raimondi I., Tunesi M., Albani D., et al. (2020). Human Gut-Microbiota Interaction in Neurodegenerative Disorders and Current Engineered Tools for Its Modeling. Front. Cell. Infect. Microbiol. 10, 297–314. doi: 10.3389/fcimb.2020.00297
Cerri S., Mus L., Blandini F. (2019). Parkinson’s Disease in Women and Men: What’s the Difference?. J. Parkinsons Dis. 9 (3), 501–515. doi: 10.3233/JPD-191683
Cersosimo M. G., Raina G. B., Pecci C., Pellene A., Calandra C. R., Gutiérrez C., et al. (2013). Gastrointestinal manifestations in Parkinson’s disease: prevalence and occurrence before motor symptoms. J. Neurol. 260, 1332–1338. doi: 10.1007/s00415-012-6801-2
Chaudhuri K. R., Qamar M. A., Rajah T., Loehrer P., Sauerbier A., Odin P., et al. (2016). Non-oral dopaminergic therapies for Parkinson’s disease: current treatments and the future. NPJ Parkinson’s Dis. 2, 1–7. doi: 10.1038/npjparkd.2016.23
Chen L., Periquet M., Wang X., Negro A., Mclean P. J., Hyman B. T., et al. (2009). Tyrosine and serine phosphorylation of α-synuclein have opposing effects on neurotoxicity and soluble oligomer formation. J. Clin. Invest. 119, 3257–3265. doi: 10.1172/JCI39088
Chen H., Zhao E. J., Zhang W., Lu Y., Liu R., Huang X., et al. (2015). Meta-analyses on prevalence of selected Parkinson’s nonmotor symptoms before and after diagnosis. Trans. Neurodegeneration 4, 1. doi: 10.1186/2047-9158-4-1
Chen Q.-Q., Haikal C., Li W., Li J.-Y. (2019). Gut inflammation in association with pathogenesis of Parkinson’s disease. Front. Mol. Neurosci. 12, 218. doi: 10.3389/fnmol.2019.00218
Cirstea M. S., Yu A. C., Golz E., Sundvick K., Kliger D., Radisavljevic N., et al. (2020). Microbiota Composition and Metabolism Are Associated With Gut Function in Parkinson’s Disease. Mov. Disord. 35, 1208–1271. doi: 10.1002/mds.28052
Codagnone M. G., Stanton C., O’mahony S. M., Dinan T. G., Cryan J. F. (2019). Microbiota and neurodevelopmental trajectories: role of maternal and early-life nutrition. Ann. Nutr. Metab. 74, 16–27. doi: 10.1159/000499144
Crowley E. K., Nolan Y. M., Sullivan A. M. (2018). Neuroprotective effects of voluntary running on cognitive dysfunction in an α-synuclein rat model of Parkinson’s disease. Neurobiol. Aging 65, 60–68. doi: 10.1016/j.neurobiolaging.2018.01.011
Cryan J. F., Dinan T. G. (2012). Mind-altering microorganisms: the impact of the gut microbiota on brain and behaviour. Nat. Rev. Neurosci. 13, 701–712. doi: 10.1038/nrn3346
da Costa C. A., Ancolio K., Checler F. (2000). Wild-type but not Parkinson’s disease-related ala-53→ Thr mutant α-synuclein protects neuronal cells from apoptotic stimuli. J. Biol. Chem. 275, 24065–24069. doi: 10.1074/jbc.M002413200
De Lau L. M., Breteler M. M. (2006). Epidemiology of Parkinson’s disease. Lancet Neurol. 5, 525–535. doi: 10.1016/S1474-4422(06)70471-9
De Theije C. G., Wopereis H., Ramadan M., Van Eijndthoven T., Lambert J., Knol J., et al. (2014). Altered gut microbiota and activity in a murine model of autism spectrum disorders. Brain Behav. Immun. 37, 197–206. doi: 10.1016/j.bbi.2013.12.005
den Besten G., Van Eunen K., Groen A. K., Venema K., Reijngoud D.-J., Bakker B. M. (2013). The role of short-chain fatty acids in the interplay between diet, gut microbiota, and host energy metabolism. J. Lipid Res. 54, 2325–2340. doi: 10.1194/jlr.R036012
Desai M. S., Seekatz A. M., Koropatkin N. M., Kamada N., Hickey C. A., Wolter M., et al. (2016). A dietary fiber-deprived gut microbiota degrades the colonic mucus barrier and enhances pathogen susceptibility. Cell 167, 1339–1353. doi: 10.1016/j.cell.2016.10.043
Deshwal S., Di Sante M., Di Lisa F., Kaludercic N. (2017). Emerging role of monoamine oxidase as a therapeutic target for cardiovascular disease. Curr. Opin. Pharmacol. 33, 64–69. doi: 10.1016/j.coph.2017.04.003
Dezsi L., Vecsei L. (2017). Monoamine oxidase B inhibitors in Parkinson’s disease. CNS Neurol. Disorders-Drug Targets (Formerly Curr. Drug Targets-CNS Neurol. Disorders) 16, 425–439. doi: 10.2174/1871527316666170124165222
Dickson D. W., Fujishiro H., Orr C., Delledonne A., Josephs K. A., Frigerio R., et al. (2009). Neuropathology of non-motor features of Parkinson disease. Parkinsonism related Disord. 15, S1–S5. doi: 10.1016/S1353-8020(09)70769-2
Dickson D. W. (2012). Parkinson’s disease and parkinsonism: neuropathology. Cold Spring Harbor Perspect. Med. 2, a009258. doi: 10.1101/cshperspect.a009258
Dinan T. G., Cryan J. F. (2012). Regulation of the stress response by the gut microbiota: implications for psychoneuroendocrinology. Psychoneuroendocrinology 37, 1369–1378. doi: 10.1016/j.psyneuen.2012.03.007
Dorsey E. R., Bloem B. R. (2018). The Parkinson pandemic—a call to action. JAMA Neurol. 75, 9–10. doi: 10.1001/jamaneurol.2017.3299
Dorsey E., Constantinescu R., Thompson J., Biglan K., Holloway R., Kieburtz K., et al. (2007). Projected number of people with Parkinson disease in the most populous nations 2005 through 2030. Neurology 68, 384–386. doi: 10.1212/01.wnl.0000247740.47667.03
Duarte-Silva S., Neves-Carvalho A., Soares-Cunha C., Silva J. M., Teixeira-Castro A., Vieira R., et al. (2018). Neuroprotective effects of creatine in the CMVMJD135 mouse model of spinocerebellar ataxia type 3. Mov. Disord. 33, 815–826. doi: 10.1002/mds.27292
Egerod K. L., Petersen N., Timshel P. N., Rekling J. C., Wang Y., Liu Q., et al. (2018). Profiling of G protein-coupled receptors in vagal afferents reveals novel gut-to-brain sensing mechanisms. Mol. Metab. 12, 62–75. doi: 10.1016/j.molmet.2018.03.016
Elmore S. (2007). Apoptosis: a review of programmed cell death. Toxicol. Pathol. 35, 495–516. doi: 10.1080/01926230701320337
Estaquier J., Arnoult D. (2007). Inhibiting Drp1-mediated mitochondrial fission selectively prevents the release of cytochrome c during apoptosis. Cell Death Differ. 14, 1086–1094. doi: 10.1038/sj.cdd.4402107
Finberg J. P. (2019). Inhibitors of MAO-B and COMT: Their effects on brain dopamine levels and uses in Parkinson’s disease. J. Neural Transm. 126, 433–448. doi: 10.1007/s00702-018-1952-7
Follett K. A., Weaver F. M., Stern M., Hur K., Harris C. L., Luo P., et al. (2010). Pallidal versus subthalamic deep-brain stimulation for Parkinson’s disease. N. Engl. J. Med. 362, 2077–2091. doi: 10.1056/NEJMoa0907083
Forsyth C. B., Shannon K. M., Kordower J. H., Voigt R. M., Shaikh M., Jaglin J. A., et al. (2011). Increased intestinal permeability correlates with sigmoid mucosa alpha-synuclein staining and endotoxin exposure markers in early Parkinson’s disease. PLoS One 6, e28032. doi: 10.1371/journal.pone.0028032
Forsythe P., Kunze W. A. (2013). Voices from within: gut microbes and the CNS. Cell. Mol. Life Sci. 70, 55–69. doi: 10.1007/s00018-012-1028-z
Foster J. A., Neufeld K.-a. (2013). Gut–brain axis: how the microbiome influences anxiety and depression. Trends Neurosci. 36, 305–312. doi: 10.1016/j.tins.2013.01.005
Frazier T. H., Dibaise J. K., Mcclain C. J. (2011). Gut microbiota, intestinal permeability, obesity-induced inflammation, and liver injury. J. Parenteral Enteral Nutr. 35, 14S–20S. doi: 10.1177/0148607111413772
Frazzitta G., Ferrazzoli D., Folini A., Palamara G., Maestri R. (2019). Severe constipation in Parkinson’s disease and in Parkinsonisms: prevalence and affecting factors. Front. Neurol. 10, 621. doi: 10.3389/fneur.2019.00621
Fung T. C., Vuong H. E., Luna C. D., Pronovost G. N., Aleksandrova A. A., Riley N. G., et al. (2019). Intestinal serotonin and fluoxetine exposure modulate bacterial colonization in the gut. Nat. Microbiol. 4, 2064–2073. doi: 10.1038/s41564-019-0540-4
George S., Brundin P. (2015). Immunotherapy in Parkinson’s disease: micromanaging alpha-synuclein aggregation. J. Parkinson’s Dis. 5, 413–424. doi: 10.3233/JPD-150630
Glass C. K., Saijo K., Winner B., Marchetto M. C., Gage F. H. (2010). Mechanisms underlying inflammation in neurodegeneration. Cell 140, 918–934. doi: 10.1016/j.cell.2010.02.016
Goedert M., Spillantini M. G., Del Tredici K., Braak H. (2013). 100 years of Lewy pathology. Nat. Rev. Neurol. 9, 13. doi: 10.1038/nrneurol.2012.242
Goldberg M. S., Lansbury P. T. Jr. (2000). Is there a cause-and-effect relationship between α-synuclein fibrillization and Parkinson’s disease? Nat. Cell Biol. 2, E115–E119. doi: 10.1038/35017124
Golpich M., Amini E., Mohamed Z., Azman Ali R., Mohamed Ibrahim N., Ahmadiani A. (2017). Mitochondrial dysfunction and biogenesis in neurodegenerative diseases: pathogenesis and treatment. CNS Neurosci. Ther. 23, 5–22. doi: 10.1111/cns.12655
Gorecki A. M., Preskey L., Bakeberg M. C., Kenna J. E., Gildenhuys C., Macdougall G., et al. (2019). Altered gut microbiome in Parkinson’s disease and the influence of lipopolysaccharide in a human α-synuclein over-expressing mouse model. Front. Neurosci. 13, 839. doi: 10.3389/fnins.2019.00839
Grall-Bronnec M., Victorri-Vigneau C., Donnio Y., Leboucher J., Rousselet M., Thiabaud E., et al. (2018). Dopamine agonists and impulse control disorders: a complex association. Drug Saf. 41, 19–75. doi: 10.1007/s40264-017-0590-6
Guo S., Al-Sadi R., Said H. M., Ma T. Y. (2013). Lipopolysaccharide causes an increase in intestinal tight junction permeability in vitro and in vivo by inducing enterocyte membrane expression and localization of TLR-4 and CD14. Am. J. Pathol. 182, 375–387. doi: 10.1016/j.ajpath.2012.10.014
Guo T., Guan X., Zhou C., Gao T., Wu J., Song Z., et al. (2020). Clinically relevant connectivity features define three subtypes of Parkinson’s disease patients. Hum. Brain Mapp. 41, 4077–4092. doi: 10.1002/hbm.25110
Haddad F., Sawalha M., Khawaja Y., Najjar A., Karaman R. (2018). Dopamine and levodopa prodrugs for the treatment of Parkinson’s disease. Molecules 23, 40. doi: 10.3390/molecules23010040
Hansen C., Angot E., Bergström A.-L., Steiner J. A., Pieri L., Paul G., et al. (2011). α-Synuclein propagates from mouse brain to grafted dopaminergic neurons and seeds aggregation in cultured human cells. J. Clin. Invest. 121, 715–725. doi: 10.1172/JCI43366
Haugarvoll K., Aarsland D., Wentzel-Larsen T., Larsen J. (2005). The influence of cerebrovascular risk factors on incident dementia in patients with Parkinson’s disease. Acta Neurol. Scand. 112, 386–390. doi: 10.1111/j.1600-0404.2005.00389.x
Hawkes C. H., Del Tredici K., Braak H. (2007). Parkinson’s disease: a dual-hit hypothesis. Neuropathol. Appl. Neurobiol. 33, 599–614. doi: 10.1111/j.1365-2990.2007.00874.x
Heinzel S., Aho V. T. E., Suenkel U., Von Thaler A. K., Schulte C., Deuschle C., et al. (2020). Gut microbiome signatures of risk and prodromal markers of Parkinson’s disease. Ann. Neurol. 88, 320–331. doi: 10.1002/ana.25788
Hirsch L., Jette N., Frolkis A., Steeves T., Pringsheim T. (2016). The incidence of Parkinson’s disease: a systematic review and meta-analysis. Neuroepidemiology 46, 292–300. doi: 10.1159/000445751
Höllerhage M. (2019). “Secondary parkinsonism due to drugs, vascular lesions, tumors, trauma, and other insults,” in International review of neurobiology. (Elsevier Academic Press), 377–418.
Holmqvist S., Chutna O., Bousset L., Aldrin-Kirk P., Li W., Björklund T., et al. (2014). Direct evidence of Parkinson pathology spread from the gastrointestinal tract to the brain in rats. Acta Neuropathol 128, 805–820. doi: 10.1007/s00401-014-1343-6
Hong C.-T., Chan L., Wu D., Chen W.-T., Chien L.-N. (2019). Antiparkinsonism anticholinergics increase dementia risk in patients with Parkinson’s disease. Parkinsonism Related Disord. 65, 224–229. doi: 10.1016/j.parkreldis.2019.06.022
Huang H., Xu H., Luo Q., He J., Li M., Chen H., et al. (2019). Fecal microbiota transplantation to treat Parkinson’s disease with constipation: A case report. Medicine 98, 129. doi: 10.1097/MD.0000000000016163
Hurtig H., Trojanowski J., Galvin J., Ewbank D., Schmidt M., Lee V.-Y., et al. (2000). Alpha-synuclein cortical Lewy bodies correlate with dementia in Parkinson’s disease, (2001):: Treatment Guidelines. Neurology 54, 1916–1921. doi: 10.1212/WNL.54.10.1916
Isaacson S. H., Hauser R. A. (2009). Improving symptom control in early Parkinson’s disease. Ther. Adv. Neurol. Disord. 2, 393–400. doi: 10.1177/1756285609339383
Katsaiti I., Nixon J. (2018). Are There Benefits in Adding Catechol-O Methyltransferase Inhibitors in the Pharmacotherapy of Parkinson’s Disease Patients? A Systematic Review. J. Parkinson’s Dis. 8, 217–231. doi: 10.3233/JPD-171225
Kell S., Khanna S., Rustay N., Gupta S. (2017). Adverse event reports in PD patients receiving extended-release carbidopa-levodopa: Effects of age. Innov Aging 1, 865. doi: 10.1093/geroni/igx004.3113
Keshavarzian A., Green S. J., Engen P. A., Voigt R. M., Naqib A., Forsyth C. B., et al. (2015). Colonic bacterial composition in Parkinson’s disease. Mov. Disord. 30, 1351–1360. doi: 10.1002/mds.26307
Kim A., Kim Y. E., Yun J. Y., Kim H.-J., Yang H.-J., Lee W.-W., et al. (2018). Amantadine and the risk of dyskinesia in patients with early Parkinson’s disease: an open-label, pragmatic trial. J. Mov. Disord. 11, 65. doi: 10.14802/jmd.18005
Kim S., Kwon S.-H., Kam T.-I., Panicker N., Karuppagounder S. S., Lee S., et al. (2019). Transneuronal propagation of pathologic α-synuclein from the gut to the brain models Parkinson’s disease. Neuron 103, 627–641. e627. doi: 10.1016/j.neuron.2019.05.035
Koutzoumis D. N., Vergara M., Pino J., Buddendorff J., Khoshbouei H., Mandel R. J., et al. (2020). Alterations of the gut microbiota with antibiotics protects dopamine neuron loss and improve motor deficits in a pharmacological rodent model of Parkinson’s disease. Exp. Neurol. 325, 113159. doi: 10.1016/j.expneurol.2019.113159
Kulisevsky J., Pagonabarraga J. (2010). Tolerability and safety of ropinirole versus other dopamine agonists and levodopa in the treatment of Parkinson’s disease. Drug Saf. 33, 147–161. doi: 10.2165/11319860-000000000-00000
Kummer B. R., Diaz I., Wu X., Aaroe A. E., Chen M. L., Iadecola C., et al. (2019). Associations between cerebrovascular risk factors and parkinson disease. Ann. Neurol. 86, 572–581. doi: 10.1002/ana.25564
Lama A., Pirozzi C., Avagliano C., Annunziata C., Mollica M. P., Calignano A., et al. (2020). Nutraceuticals: an integrative approach to starve Parkinson’s disease. Brain Behav. Immunity-Health 2, 100037. doi: 10.1016/j.bbih.2020.100037
Lang A. E., Lees A. (2002). Management of Parkinson’s disease: an evidence-based review. Mov. Disord. 17, S1–S166. doi: 10.1002/mds.5554
Lau A. C., Diggle J. L., Bring P. P. (2018). Improvement in severe orthostatic hypotension following carbidopa dose reduction. Can. J. Neurol. Sci. 45, 252–253. doi: 10.1017/cjn.2017.284
Lee D. J., Dallapiazza R. F., De Vloo P., Lozano A. M. (2018). Current surgical treatments for Parkinson’s disease and potential therapeutic targets. Neural Regener. Res. 13, 1342. doi: 10.4103/1673-5374.235220
Lees A. J. (2008). Evidence-based efficacy comparison of tolcapone and entacapone as adjunctive therapy in Parkinson’s disease. CNS Neurosci. Ther. 14, 83–93. doi: 10.1111/j.1755-5949.2007.00035.x
LeWitt P. A. (2016). New levodopa therapeutic strategies. Parkinsonism Related Disord. 22, S37–S40. doi: 10.1016/j.parkreldis.2015.09.021
Li J., Chou M., Lai P. (2018). “Carbon Monoxide Poisoning-Induced Parkinsonism: A Prospective Study of Clinical and Neuroimage Correlation,” in Movement Disorders. NJ USA: Wiley, S70–S70.
Li K., Li Y., Shelton J. M., Richardson J. A., Spencer E., Chen Z. J., et al. (2000). Cytochrome c deficiency causes embryonic lethality and attenuates stress-induced apoptosis. Cell 101, 389–399. doi: 10.1016/S0092-8674(00)80849-1
Liepelt-Scarfone I., Gauss K., Maetzler W., Muller K., Bormann C., Fruhmann Berger M., et al. (2013). Evaluation of progression markers in the premotor phase of Parkinson's disease: the progression markers in the premotor phase study. Neuroepidemiology 41, 174–182. doi: 10.1159/000353560
Lim S. Y., Lang A. E. (2010). The nonmotor symptoms of Parkinson’s disease—an overview. Mov. Disord. 25, S123–S130. doi: 10.1002/mds.22786
Limphaibool N., Iwanowski P., Holstad M. J. V., Kobylarek D., Kozubski W. (2019). Infectious etiologies of Parkinsonism: pathomechanisms and clinical implications. Front. Neurol. 10, 652. doi: 10.3389/fneur.2019.00652
Lin M. T., Beal M. F. (2006). Mitochondrial dysfunction and oxidative stress in neurodegenerative diseases. Nature 443, 787–795. doi: 10.1038/nature05292
Lin C.-H., Chen C.-C., Chiang H.-L., Liou J.-M., Chang C.-M., Lu T.-P., et al. (2019). Altered gut microbiota and inflammatory cytokine responses in patients with Parkinson’s disease. J. Neuroinflamm 16, 129. doi: 10.1186/s12974-019-1528-y
Lindvall O., Kokaia Z. (2009). Prospects of stem cell therapy for replacing dopamine neurons in Parkinson’s disease. Trends Pharmacol. Sci. 30, 260–267. doi: 10.1016/j.tips.2009.03.001
Lionnet A., Leclair-Visonneau L., Neunlist M., Murayama S., Takao M., Adler C. H., et al. (2018). Does Parkinson’s disease start in the gut? Acta Neuropathol. 135, 1–12. doi: 10.1007/s00401-017-1777-8
Longhena F., Faustini G., Missale C., Pizzi M., Spano P., Bellucci A. (2017). The contribution of α-synuclein spreading to Parkinson’s disease synaptopathy. Neural Plast. 2017, 1–15. doi: 10.1155/2017/5012129
Longhena F., Faustini G., Spillantini M. G., Bellucci A. (2019). Living in promiscuity: the multiple partners of alpha-synuclein at the synapse in physiology and pathology. Int. J. Mol. Sci. 20, 141. doi: 10.3390/ijms20010141
Luczynski P., Mcvey Neufeld K.-A., Oriach C. S., Clarke G., Dinan T. G., Cryan J. F. (2016). Growing up in a bubble: using germ-free animals to assess the influence of the gut microbiota on brain and behavior. Int. J. Neuropsychopharmacol. 19, 1–17. doi: 10.1093/ijnp/pyw020
MahmoudianDehkordi S., Arnold M., Nho K., Ahmad S., Jia W., Xie G., et al. (2019). Altered bile acid profile associates with cognitive impairment in Alzheimer’s disease—An emerging role for gut microbiome. Alzheimer’s Dementia 15, 76–92. doi: 10.1016/j.jalz.2018.07.217
Manzoni C., Lewis P. A. (2013). Dysfunction of the autophagy/lysosomal degradation pathway is a shared feature of the genetic synucleinopathies. FASEB J. 27, 3424–3429. doi: 10.1096/fj.12-223842
Marques E. P., Wyse A. T. (2019). Creatine as a neuroprotector: an actor that can play many parts. Neurotoxic. Res. 36, 1–13. doi: 10.1007/s12640-019-00053-7
Marsh L. (2013). Depression and Parkinson’s disease: current knowledge. Curr. Neurol. Neurosci. Rep. 13, 409. doi: 10.1007/s11910-013-0409-5
Masliah E., Rockenstein E., Adame A., Alford M., Crews L., Hashimoto M., et al. (2005). Effects of α-synuclein immunization in a mouse model of Parkinson’s disease. Neuron 46, 857–868. doi: 10.1016/j.neuron.2005.05.010
Masliah E., Rockenstein E., Mante M., Crews L., Spencer B., Adame A., et al. (2011). Passive immunization reduces behavioral and neuropathological deficits in an alpha-synuclein transgenic model of Lewy body disease. PLoS One 6, e19338. doi: 10.1371/journal.pone.0019338
Mertsalmi T., Aho V., Pereira P., Paulin L., Pekkonen E., Auvinen P., et al. (2017). More than constipation–bowel symptoms in Parkinson’s disease and their connection to gut microbiota. Eur. J. Neurol. 24, 1375–1383. doi: 10.1111/ene.13398
Milber J. M., Noorigian J. V., Morley J. F., Petrovitch H., White L., Ross G. W., et al. (2012). Lewy pathology is not the first sign of degeneration in vulnerable neurons in Parkinson disease. Neurology 79, 2307–2314. doi: 10.1212/WNL.0b013e318278fe32
Minato T., Maeda T., Fujisawa Y., Tsuji H., Nomoto K., Ohno K., et al. (2017). Progression of Parkinson’s disease is associated with gut dysbiosis: two-year follow-up study. PLoS One 12, e0187307. doi: 10.1371/journal.pone.0187307
Mishima T., Fujioka S., Yamaguchi Y., Hayashi Y., Onozawa R., Fukae J., et al. (2018). Role of anticholinergic medications in the treatment of Parkinson’s disease. Parkinsonism Related Disord. 46, e85. doi: 10.1016/j.parkreldis.2017.11.296
Morrow S. A., Rosehart H., Sener A., Welk B. (2018). Anti-cholinergic medications for bladder dysfunction worsen cognition in persons with multiple sclerosis. J. Neurol. Sci. 385, 39–44. doi: 10.1016/j.jns.2017.11.028
Mulak A., Bonaz B. (2015). Brain-gut-microbiota axis in Parkinson’s disease. World J. Gastroenterol.: WJG 21, 10609. doi: 10.3748/wjg.v21.i37.10609
Nair A. T., Ramachandran V., Joghee N. M., Antony S., Ramalingam G. (2018). Gut microbiota dysfunction as reliable non-invasive early diagnostic biomarkers in the pathophysiology of Parkinson’s disease: a critical review. J. Neurogastroenterol. Motil. 24, 30. doi: 10.5056/jnm17105
Nishiwaki H., Ito M., Ishida T., Hamaguchi T., Maeda T., Kashihara K., et al. (2020). Meta-Analysis of Gut Dysbiosis in Parkinson’s Disease. Mov. Disord. 35, 1626–1635. doi: 10.1002/mds.28119
Nishtala P. S., Salahudeen M. S., Hilmer S. N. (2016). Anticholinergics: theoretical and clinical overview. Expert Opin. Drug Saf. 15, 753–768. doi: 10.1517/14740338.2016.1165664
Normile D. (2018). First-of-its-kind clinical trial will use reprogrammed adult stem cells to treat Parkinson’s. Science 80. doi: 10.1126/science.aau9466
Nussbaum R. L., Ellis C. E. (2003). Alzheimer’s disease and Parkinson’s disease. N. Engl. J. Med. 348, 1356–1364. doi: 10.1056/NEJM2003ra020003
Olanow C. W., Watkins P. B. (2007). Tolcapone: an efficacy and safety review, (2007). Clin. Neuropharmacol. 30, 287–294. doi: 10.1097/wnf.0b013e318038d2b6
Olanow C. W., Watts R. L., Koller W. C. (2001). An algorithm (decision tree) for the management of Parkinson’s disease, (2001)):: Treatment Guidelines. Neurology 56, S1–S88. doi: 10.1212/WNL.56.suppl_5.S1
O’Donovan S. M., Crowley E. K., Brown J. R. M., O’sullivan O., O’leary O. F., Timmons S., et al. (2020). Nigral overexpression of α-synuclein in a rat Parkinson’s disease model indicates alterations in the enteric nervous system and the gut microbiome. Neurogastroenterol. Motil. 32, e13726. doi: 10.1111/nmo.13726
Özoğul F., Kuley E., Özoğul Y., Özoğul İ. (2012). The function of lactic acid bacteria on biogenic amines production by food-borne pathogens in arginine decarboxylase broth. Food Sci. Technol. Res. 18, 795–804. doi: 10.3136/fstr.18.795
Özoğul F. (2004). Production of biogenic amines by Morganella morganii, Klebsiella pneumoniae and Hafnia alvei using a rapid HPLC method. Eur. Food Res. Technol. 219, 465–469. doi: 10.1007/s00217-004-0988-0
Pahwa R., Lyons K. E. (2009). Levodopa-related wearing-off in Parkinson’s disease: identification and management. Curr. Med. Res. Opin. 25, 841–849. doi: 10.1185/03007990902779319
Parashar A., Udayabanu M. (2017). Gut microbiota: Implications in Parkinson’s disease. Parkinsonism Related Disord. 38, 1–7. doi: 10.1016/j.parkreldis.2017.02.002
Park J. H., Lee S. H., Kim Y., Park S. W., Byeon G. H., Jang J. W., et al. (2020). Depressive symptoms are associated with worse cognitive prognosis in patients with newly diagnosed idiopathic Parkinson disease. Psychogeriatrics. doi: 10.1111/psyg.12601
Parkkinen L., Pirttilä T., Alafuzoff I. (2008). Applicability of current staging/categorization of α-synuclein pathology and their clinical relevance. Acta Neuropathol. 115, 399–407. doi: 10.1007/s00401-008-0346-6
Parmar M., Grealish S., Henchcliffe C. (2020). The future of stem cell therapies for Parkinson disease. Nat. Rev. Neurosci. 21, 1–13. doi: 10.1038/s41583-019-0257-7
Patel S., Garcia X., Mohammad M. E., Yu X. X., Vlastaris K., O'Donnell K., et al. (2017). Dopamine agonist withdrawal syndrome (DAWS) in a tertiary Parkinson disease treatment center. J Neurol Sci 379, 308–311. doi: 10.1016/j.jns.2017.06.022
Piquet A. L., Venkiteswaran K., Marupudi N. I., Berk M., Subramanian T. (2012). The immunological challenges of cell transplantation for the treatment of Parkinson’s disease. Brain Res. Bull. 88, 320–331. doi: 10.1016/j.brainresbull.2012.03.001
Poirier A.-A., Aubé B., Côté M., Morin N., Di Paolo T., Soulet D. (2016). Gastrointestinal dysfunctions in Parkinson’s disease: symptoms and treatments. Parkinson’s Dis. 2016, 1–23. doi: 10.1155/2016/6762528
Pokusaeva K., Johnson C., Luk B., Uribe G., Fu Y., Oezguen N., et al. (2017). GABA-producing Bifidobacterium dentium modulates visceral sensitivity in the intestine. Neurogastroenterol. Motil. 29, e12904. doi: 10.1111/nmo.12904
Pu Y., Chang L., Qu Y., Wang S., Zhang K., Hashimoto K. (2019). Antibiotic-induced microbiome depletion protects against MPTP-induced dopaminergic neurotoxicity in the brain. Aging (Albany NY) 11, 6915. doi: 10.18632/aging.102221
Qiao C.-M., Sun M.-F., Jia X.-B., Shi Y., Zhang B.-P., Zhou Z.-L., et al. (2020). Sodium butyrate causes α-synuclein degradation by an Atg5-dependent and PI3K/Akt/mTOR-related autophagy pathway. Exp. Cell Res. 387, 111772. doi: 10.1016/j.yexcr.2019.111772
Recasens A., Dehay B. (2014). Alpha-synuclein spreading in Parkinson’s disease. Front. Neuroanat. 8, 159. doi: 10.3389/fnana.2014.00159
Reeve A., Simcox E., Turnbull D. (2014). Ageing and Parkinson’s disease: why is advancing age the biggest risk factor? Ageing Res. Rev. 14, 19–30. doi: 10.1016/j.arr.2014.01.004
Reid G. (2019). Disentangling what we know about microbes and mental health. Front. Endocrinol. 10, 81–87. doi: 10.3389/fendo.2019.00081
Repovš G., Baddeley A. (2006). The multi-component model of working memory: Explorations in experimental cognitive psychology. Neuroscience 139, 5–21. doi: 10.1016/j.neuroscience.2005.12.061
Rey N. L., Steiner J. A., Maroof N., Luk K. C., Madaj Z., Trojanowski J. Q., et al. (2016). Widespread transneuronal propagation of α-synucleinopathy triggered in olfactory bulb mimics prodromal Parkinson’s disease. J. Exp. Med. 213, 1759–1778. doi: 10.1084/jem.20160368
Reynolds A. D., Stone D. K., Hutter J. A., Benner E. J., Mosley R. L., Gendelman H. E. (2010). Regulatory T cells attenuate Th17 cell-mediated nigrostriatal dopaminergic neurodegeneration in a model of Parkinson’s disease. J. Immunol. 184, 2261–2271. doi: 10.4049/jimmunol.0901852
Rite I., Machado A., Cano J., Venero J. L. (2007). Blood–brain barrier disruption induces in vivo degeneration of nigral dopaminergic neurons. J. Neurochem. 101, 1567–1582. doi: 10.1111/j.1471-4159.2007.04567.x
Rogers G. B., Keating D. J., Young R. L., Wong M. L., Licinio J., Wesselingh S. (2016). From gut dysbiosis to altered brain function and mental illness: mechanisms and pathways. Mol Psychiatry 21, 738–748. doi: 10.1038/mp.2016.50
Sampson T. R., Debelius J. W., Thron T., Janssen S., Shastri G. G., Ilhan Z. E., et al. (2016). Gut microbiota regulate motor deficits and neuroinflammation in a model of Parkinson’s disease. Cell 167, 1469–1480.e1412. doi: 10.1016/j.cell.2016.11.018
Sarkar S. R., Banerjee S. (2019). Gut microbiota in neurodegenerative disorders. J. Neuroimmunol. 328, 98–104. doi: 10.1016/j.jneuroim.2019.01.004
Schapira A. H., Jenner P. (2011). Etiology and pathogenesis of Parkinson’s disease. Mov. Disord. 26, 1049–1055. doi: 10.1002/mds.23732
Scheperjans F., Aho V., Pereira P. A., Koskinen K., Paulin L., Pekkonen E., et al. (2015). Gut microbiota are related to Parkinson’s disease and clinical phenotype. Mov. Disord. 30, 350–358. doi: 10.1002/mds.26069
Schlesinger I., Korczyn A. D. (2016). Catechol-O-methyltransferase inhibitors in the management of Parkinson’s disease. Parkinson’s Disease: Curr. Future Ther. Clin. Trials 76, 76–82. doi: 10.1017/CBO9781107284210.008
Schulz-Schaeffer W. J. (2010). The synaptic pathology of α-synuclein aggregation in dementia with Lewy bodies, Parkinson’s disease and Parkinson’s disease dementia. Acta Neuropathol. 120, 131–143. doi: 10.1007/s00401-010-0711-0
Sgritta M., Dooling S. W., Buffington S. A., Momin E. N., Francis M. B., Britton R. A., et al. (2019). Mechanisms underlying microbial-mediated changes in social behavior in mouse models of autism spectrum disorder. Neuron 101, 246–259. e246. doi: 10.1016/j.neuron.2018.11.018
Shah M., Deeb J., Fernando M., Noyce A., Visentin E., Findley L. J., et al. (2009). Abnormality of taste and smell in Parkinson’s disease. Parkinsonism Related Disord. 15, 232–237. doi: 10.1016/j.parkreldis.2008.05.008
Sheridan C., Martin S. J. (2010). Mitochondrial fission/fusion dynamics and apoptosis. Mitochondrion 10, 640–648. doi: 10.1016/j.mito.2010.08.005
Shishov V., Kirovskaya T., Kudrin V., Oleskin A. (2009). Amine neuromediators, their precursors, and oxidation products in the culture of Escherichia coli K-12. Appl. Biochem. Microbiol. 45, 494–497. doi: 10.1134/S0003683809050068
Silva T. B., Borges F., Serrão M. P., Soares-Da-Silva P. (2020). Liver says no: the ongoing search for safe catechol O-methyltransferase inhibitors to replace tolcapone. Drug Discov. Today. doi: 10.1016/j.drudis.2020.07.015
Simrén M., Barbara G., Flint H. J., Spiegel B. M., Spiller R. C., Vanner S., et al. (2013). Intestinal microbiota in functional bowel disorders: a Rome foundation report. Gut 62, 159–176. doi: 10.1136/gutjnl-2012-302167
Sokol H., Pigneur B., Watterlot L., Lakhdari O., Bermúdez-Humarán L. G., Gratadoux J.-J., et al. (2008). Faecalibacterium prausnitzii is an anti-inflammatory commensal bacterium identified by gut microbiota analysis of Crohn disease patients. Proc. Natl. Acad. Sci. 105, 16731–16736. doi: 10.1073/pnas.0804812105
Spillantini M. G., Crowther R. A., Jakes R., Hasegawa M., Goedert M. (1998). α-Synuclein in filamentous inclusions of Lewy bodies from Parkinson’s disease and dementia with Lewy bodies. Proc. Natl. Acad. Sci. 95, 6469–6473. doi: 10.1073/pnas.95.11.6469
Srivastav S., Neupane S., Bhurtel S., Katila N., Maharjan S., Choi H., et al. (2019). Probiotics mixture increases butyrate, and subsequently rescues the nigral dopaminergic neurons from MPTP and rotenone-induced neurotoxicity. J. Nutr. Biochem. 69, 73–86. doi: 10.1016/j.jnutbio.2019.03.021
Stilling R. M., Dinan T. G., Cryan J. F. (2014). Microbial genes, brain & behaviour–epigenetic regulation of the gut–brain axis. Genes Brain Behav. 13, 69–86. doi: 10.1111/gbb.12109
Strandwitz P., Kim K. H., Terekhova D., Liu J. K., Sharma A., Levering J., et al. (2019). GABA-modulating bacteria of the human gut microbiota. Nat. Microbiol. 4, 396–403. doi: 10.1038/s41564-018-0307-3
Sudo N., Chida Y., Aiba Y., Sonoda J., Oyama N., Yu X. N., et al. (2004). Postnatal microbial colonization programs the hypothalamic–pituitary–adrenal system for stress response in mice. J. Physiol. 558, 263–275. doi: 10.1113/jphysiol.2004.063388
Suen D.-F., Norris K. L., Youle R. J. (2008). Mitochondrial dynamics and apoptosis. Genes Dev. 22, 1577–1590. doi: 10.1101/gad.1658508
Sulzer D. (2007). Multiple hit hypotheses for dopamine neuron loss in Parkinson’s disease. Trends Neurosci. 30, 244–250. doi: 10.1016/j.tins.2007.03.009
Sun M.-F., Zhu Y.-L., Zhou Z.-L., Jia X.-B., Xu Y.-D., Yang Q., et al. (2018). Neuroprotective effects of fecal microbiota transplantation on MPTP-induced Parkinson’s disease mice: gut microbiota, glial reaction and TLR4/TNF-α signaling pathway. Brain Behav. Immun. 70, 48–60. doi: 10.1016/j.bbi.2018.02.005
Surwase S. N., Jadhav J. P. (2011). Bioconversion of L-tyrosine to L-DOPA by a novel bacterium Bacillus sp. JPJ. Amino Acids 41, 495–506. doi: 10.1007/s00726-010-0768-z
Szökő É., Tábi T., Riederer P., Vécsei L., Magyar K. (2018). Pharmacological aspects of the neuroprotective effects of irreversible MAO-B inhibitors, selegiline and rasagiline, in Parkinson’s disease. J. Neural Transm. 125, 1735–1749. doi: 10.1007/s00702-018-1853-9
Taba P. (2017). “Toxic-induced parkinsonism,” in Movement Disorders Curricula. (Springer Vienna: Springer), 225–232.
Tabakman R., Lecht S., Lazarovici P. (2004). Neuroprotection by monoamine oxidase B inhibitors: a therapeutic strategy for Parkinson’s disease? Bioessays 26, 80–90. doi: 10.1002/bies.10378
Tanaka M., Kim Y. M., Lee G., Junn E., Iwatsubo T., Mouradian M. M. (2004). Aggresomes formed by α-synuclein and synphilin-1 are cytoprotective. J. Biol. Chem. 279, 4625–4631. doi: 10.1074/jbc.M310994200
Thomas S., Izard J., Walsh E., Batich K., Chongsathidkiet P., Clarke G., et al. (2017). The host microbiome regulates and maintains human health: a primer and perspective for non-microbiologists. Cancer Res. 77, 1783–1812. doi: 10.1158/0008-5472.CAN-16-2929
Tran T. N., Vo T. N., Frei K., Truong D. D. (2018). Levodopa-induced dyskinesia: clinical features, incidence, and risk factors. J. Neural Transm. 125, 1109–1117. doi: 10.1007/s00702-018-1900-6
Tsavkelova E., Botvinko I., Kudrin V., Oleskin A. (2000). Detection of neurotransmitter amines in microorganisms with the use of high-performance liquid chromatography. Doklady Biochem.: Proc. Acad. Sci. USSR Biochem. Section 372, 115. doi: 10.1038/mp.2016.50
Tsuang D., Leverenz J. B., Lopez O. L., Hamilton R. L., Bennett D. A., Schneider J. A., et al. (2013). APOE ϵ4 increases risk for dementia in pure synucleinopathies. JAMA Neurol. 70, 223–228. doi: 10.1001/jamaneurol.2013.600
Tufekci K. U., Genc S., Genc K. (2011). The endotoxin-induced neuroinflammation model of Parkinson’s disease. Parkinson’s Dis. 2011. doi: 10.4061/2011/487450
Unger M. M., Spiegel J., Dillmann K.-U., Grundmann D., Philippeit H., Bürmann J., et al. (2016). Short chain fatty acids and gut microbiota differ between patients with Parkinson’s disease and age-matched controls. Parkinsonism Related Disord. 32, 66–72. doi: 10.1016/j.parkreldis.2016.08.019
Valdez-Morales E. E., Overington J., Guerrero-Alba R., Ochoa-Cortes F., Ibeakanma C. O., Spreadbury I., et al. (2013). Sensitization of peripheral sensory nerves by mediators from colonic biopsies of diarrhea-predominant irritable bowel syndrome patients: a role for PAR2. Am. J. Gastroenterol. 108, 1634–1643. doi: 10.1038/ajg.2013.241
van Kessel S. P., Frye A. K., El-Gendy A. O., Castejon M., Keshavarzian A., Van Dijk G., et al. (2019). Gut bacterial tyrosine decarboxylases restrict levels of levodopa in the treatment of Parkinson’s disease. Nat. Commun. 10, 1–11. doi: 10.1038/s41467-019-08294-y
Vaughan R. A., Foster J. D. (2013). Mechanisms of dopamine transporter regulation in normal and disease states. Trends Pharmacol. Sci. 34, 489–496. doi: 10.1016/j.tips.2013.07.005
Vila M., Przedborski S. (2004). Genetic clues to the pathogenesis of Parkinson’s disease. Nat. Med. 10, S58–S62. doi: 10.1038/nm1068
Vincent A. D., Wang X.-Y., Parsons S. P., Khan W. I., Huizinga J. D. (2018). Abnormal absorptive colonic motor activity in germ-free mice is rectified by butyrate, an effect possibly mediated by mucosal serotonin. Am. J. Physiology-Gastrointestinal Liver Physiol. 315, G896–G907. doi: 10.1152/ajpgi.00237.2017
Volpicelli-Daley L. A., Gamble K. L., Schultheiss C. E., Riddle D. M., West A. B., Lee V. M.-Y. (2014). Formation of α-synuclein Lewy neurite–like aggregates in axons impedes the transport of distinct endosomes. Mol. Biol. Cell 25, 4010–4023. doi: 10.1091/mbc.e14-02-0741
Wallen Z. D., Appah M., Dean M. N., Sesler C. L., Factor S. A., Molho E., et al. (2020). Characterizing dysbiosis of gut microbiome in PD: Evidence for overabundance of opportunistic pathogens. NPJ Parkinson’s Dis. 6, 1–12. doi: 10.1101/2020.01.13.905166
Wang L., Das U., Scott D. A., Tang Y., Mclean P. J., Roy S. (2014). α-synuclein multimers cluster synaptic vesicles and attenuate recycling. Curr. Biol. 24, 2319–2326. doi: 10.1016/j.cub.2014.08.027
Wang P.-S., Yeh C.-L., Lu C.-F., Wu H.-M., Soong B.-W., Wu Y.-T. (2017). The involvement of supratentorial white matter in multiple system atrophy: a diffusion tensor imaging tractography study. Acta Neurol. Belgica 117, 213–220. doi: 10.1007/s13760-016-0724-0
Wang H., Liu X., Tan C., Zhou W., Jiang J., Peng W., et al. (2020). Bacterial, viral, and fungal infection-related risk of Parkinson’s disease: Meta-analysis of cohort and case–control studies. Brain Behav. 10, e01549. doi: 10.1002/brb3.1549
Wolf E., Seppi K., Katzenschlager R., Hochschorner G., Ransmayr G., Schwingenschuh P., et al. (2010). Long-term antidyskinetic efficacy of amantadine in Parkinson’s disease. Mov. Disord. 25, 1357–1363. doi: 10.1002/mds.23034
Woo M., Hakem R., Furlonger C., Hakem A., Duncan G. S., Sasaki T., et al. (2003). Caspase-3 regulates cell cycle in B cells: a consequence of substrate specificity. Nat. Immunol. 4, 1016–1022. doi: 10.1038/ni976
Xiao Y., Luo M., Luo H., Wang J. (2014). Creatine for Parkinson’s disease. Cochrane Database Syst. Rev. CD009646. doi: 10.1002/14651858.CD009646.pub2
Yamada M., Iwatsubo T., Mizuno Y., Mochizuki H. (2004). Overexpression of α-synuclein in rat substantia nigra results in loss of dopaminergic neurons, phosphorylation of α-synuclein and activation of caspase-9: resemblance to pathogenetic changes in Parkinson’s disease. J. Neurochem. 91, 451–461. doi: 10.1111/j.1471-4159.2004.02728.x
Yano J. M., Yu K., Donaldson G. P., Shastri G. G., Ann P., Ma L., et al. (2015). Indigenous bacteria from the gut microbiota regulate host serotonin biosynthesis. Cell 161, 264–276. doi: 10.1016/j.cell.2015.02.047
Yu X. X., Fernandez H. H. (2017). Dopamine agonist withdrawal syndrome: a comprehensive review. J. Neurol. Sci. 374, 53–55. doi: 10.1016/j.jns.2016.12.070
Yu Q.-J., Yu S.-Y., Zuo L.-J., Lian T.-H., Hu Y., Wang R.-D., et al. (2018). Parkinson disease with constipation: clinical features and relevant factors. Sci. Rep. 8, 1–9. doi: 10.1038/s41598-017-16790-8
Zhang F., Luo W., Shi Y., Fan Z., Ji G. (2012). Should we standardize the 1,700-year-old fecal microbiota transplantation? Am. J. Gastroenterol. 107, 1755. doi: 10.1038/ajg.2012.251
Keywords: Parkinson’s disease , dopamine, intestinal neuromodulation, brain-gut axis, gut microbiota
Citation: Liu J, Xu F, Nie Z and Shao L (2020) Gut Microbiota Approach—A New Strategy to Treat Parkinson’s Disease. Front. Cell. Infect. Microbiol. 10:570658. doi: 10.3389/fcimb.2020.570658
Received: 10 June 2020; Accepted: 30 September 2020;
Published: 22 October 2020.
Edited by:
Robert Czajkowski, University of Gdansk, PolandReviewed by:
Francesca Longhena, University of Brescia, ItalyIrmgard Blanca Paris, Universidad Santo Tomás, Chile
Copyright © 2020 Liu, Xu, Nie and Shao. This is an open-access article distributed under the terms of the Creative Commons Attribution License (CC BY). The use, distribution or reproduction in other forums is permitted, provided the original author(s) and the copyright owner(s) are credited and that the original publication in this journal is cited, in accordance with accepted academic practice. No use, distribution or reproduction is permitted which does not comply with these terms.
*Correspondence: Lei Shao, shaolei00@gmail.com
†These authors contributed equally to this work