- 1Biological and Biomedical Sciences, Harvard Medical School, Boston, MA, United States
- 2Division of Infectious Diseases, Boston Children’s Hospital, Boston, MA, United States
- 3Department of Pediatrics, Harvard Medical School, Boston, MA, United States
The actomyosin contractile ring is a key feature of eukaryotic cytokinesis, conserved across many eukaryotic kingdoms. Recent research into the cell biology of the divergent eukaryotic clade Apicomplexa has revealed a contractile ring structure required for asexual division in the medically relevant genera Toxoplasma and Plasmodium; however, the structure of the contractile ring, known as the basal complex in these parasites, remains poorly characterized and in the absence of a myosin II homolog, it is unclear how the force required of a cytokinetic contractile ring is generated. Here, we review the literature on the basal complex in Apicomplexans, summarizing what is known about its formation and function, and attempt to provide possible answers to this question and suggest new avenues of study by comparing the Apicomplexan basal complex to well-studied, established cytokinetic contractile rings and their mechanisms in organisms such as S. cerevisiae and D. melanogaster. We also compare the basal complex to structures formed during mitochondrial and plastid division and cytokinetic mechanisms of organisms beyond the Opisthokonts, considering Apicomplexan diversity and divergence.
Introduction
The Apicomplexa are a phylum of obligate intracellular parasites, categorized within the SAR (Stramenopile, Alveolata, Rhizaria) eukaryotes (Gräf et al., 2015). Toxoplasma and Plasmodium, two genera within the Apicomplexan phylum, are responsible for a significant degree of humanity’s infectious disease burden. While much progress has been made in the control of malaria over the past fifteen years, it still takes over 400,000 lives a year, and toxoplasmosis remains one of the world’s most widespread parasitic infections, posing significant danger to the pregnant and/or immunocompromised (Furtado et al., 2011; World Malaria Report 2019). Apicomplexans are also so divergent from the organisms in which most cell biology studies are done such as Saccharomyces cerevisiae, Drosophila rerio, Caenorhabditis elegans, etc., that basic cellular processes remain poorly understood. Cell division, for example, is incredibly complex in these organisms since their life cycles involve multiple morphologically diverse stages with both sexual and asexual replication. While most eukaryotes undergo simple cytokinesis where one cell produces two daughter cells, these pathogenic Some Apicomplexans utilize a divergent process known as schizogony wherein several successive rounds of karyokinesis result in a multinucleated schizont. The final karyokinesis is paired with cytokinesis, as the cytoplasmic mass segments into many daughter cells, each containing one nucleus. We note that Toxoplasma divides by schizogony in its definitive host in the process of producing gametes, but divides by endodyogeny, a single closed mitosis process where the daughter cells are assembled internally, during asexual replication; Plasmodium divide via schizogony during the asexual intra-erythrocytic stage of its life cycle (Francia and Striepen, 2014).
The molecular mechanisms underlying schizogony and how they relate to higher eukaryote division processes remain unknown. Possible parallels to the actomyosin ring-mediated cell division that characterizes most eukaryotic cytokinesis are difficult to identify as a result of Apicomplexan divergence and unique life cycle and division processes. Among the most significant differences at the genetic level is that Apicomplexans lack a homolog to the conventional myosin involved in actomyosin-mediated cell division, myosin II, and must utilize a different, yet uncharacterized, mechanism (Frénal et al., 2020). Recent research has begun to shed light on Apicomplexan cellular division, however, and revealed an important molecular machine, the basal complex, which likely acts as a contractile ring during asexual division.
Considering the phylogenetic distance between Apicomplexans and well-studied organisms such as yeast, it is likely not possible to find direct homologs in the latter for basal complex proteins, or vice versa. However, examining contractile ring composition in these organisms in comparison to the Apicomplexan basal complex may illuminate new avenues of study. While apicomplexans lack myosin II, for example, the ‘non-canonical’ myosins in Plasmodium may take on a myosin II-like role in the basal complex. Identifying similarities between other contractile rings and the basal complex may also identify Apicomplexan proteins important for basal complex function.
The Minimal Contractile Ring
Before discussing the basal complex and how it compares to other eukaryotes’ contractile rings, it is useful to understand what a well-studied contractile ring usually consists of and how it functions in cellular division. In eukaryotes, the contractile ring is a structure that functions to generate constricting force required to separate daughter cells during cell division. It is assembled during cytokinesis and though it contains different accessory or regulatory proteins required for its assembly and function, the core proteins of the contractile ring are, in the vast majority of eukaryotes in which the contractile ring has been extensively studied, actin filaments (F-actin) and the myosin II motor protein. Its reliance on actin filaments means that actin filament stabilizing and destabilizing proteins are often important to proper formation and function, and because the contractile ring must assemble and constrict during a very specific late stage in the cell cycle, the processes underlying its assembly and function are often tightly temporally controlled by variations in mitotic kinase levels and phosphorylation/dephosphorylation signals (Miller, 2011).
The contractile ring as it exists in most eukaryotes requires both structural proteins (actin) and proteins that can generate force on actin fibers (myosin II), but even the most minimal contractile ring systems require at least a few additional components (Mangione and Gould, 2019). Detailing the minimal requirements, to the best of our current knowledge, for a contractile ring’s formation and function will provide a baseline against which in vivo contractile rings in both well-studied organisms and the more enigmatic Apicomplexan structures can be compared.
In vitro contractile rings have not yet been reconstituted from recombinant proteins alone, but functional structures approximate to a ‘minimal contractile ring’ have been purified from yeast spheroplasts. Rings isolated from S. cerevisiae cells via differential centrifugation and fractionation contained, in addition to actin and myosin, IQGAP (Iqg1p), actin, mlc2p (myosin regulatory light chain), cytokinetic F-BAR protein Hof1p, and the septin filament marker Cdc11p (Young et al., 2010). The rings retained some constrictive ability; thus this can be considered the ‘minimal’ apparatus required for S. cerevisiae ring formation and function (Young et al., 2010) (Table 1).
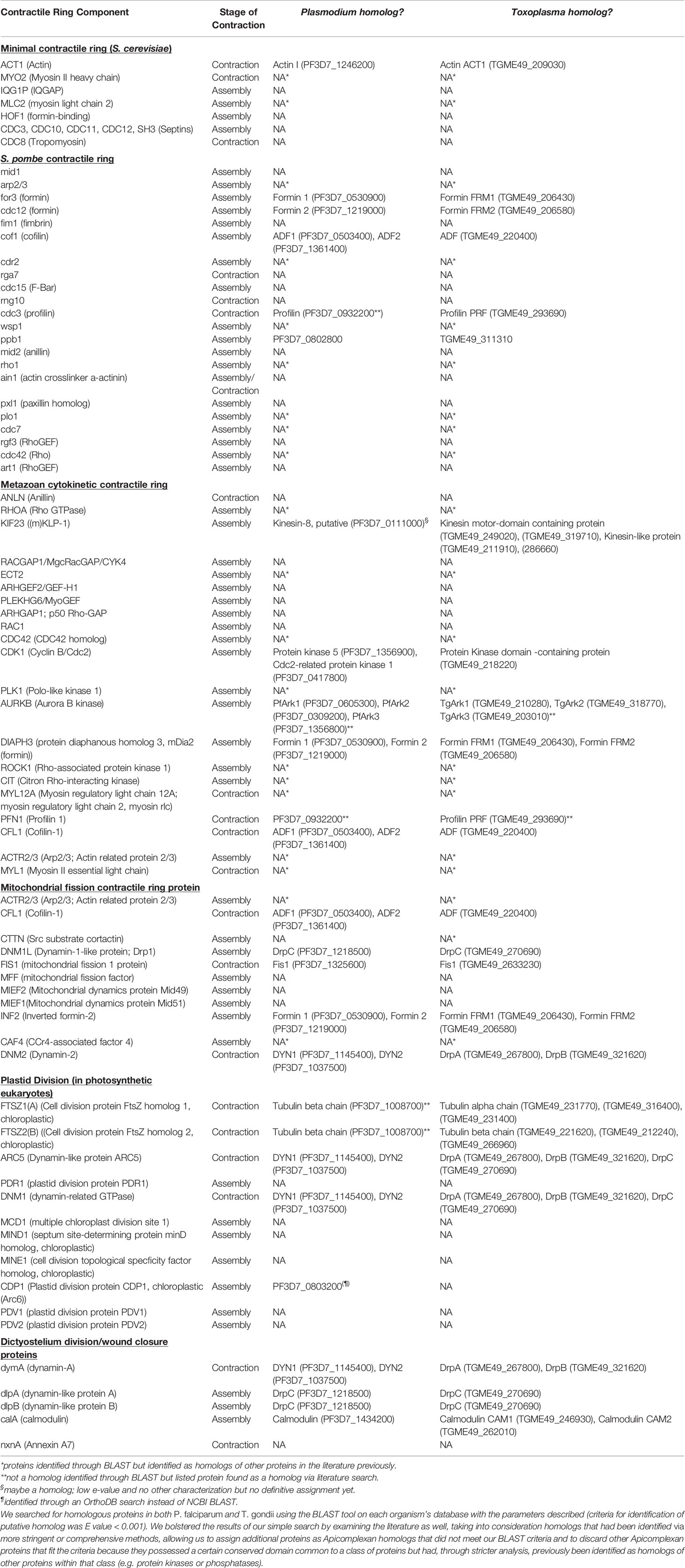
Table 1 To provide a general overview of which components of different eukaryotes’ contractile rings are conserved in Apicomplexans, we compiled a list of proteins important for the formation and function of each eukaryote’s contractile ring.
In less stringent in vitro conditions, the contractile rings of permeabilized Schizosaccharomyces pombe spheroplasts contained myosin II, actin, and tropomyosin Cdc8p (Mishra et al., 2013; Mabuchi et al., 2017). These rings too could constrict in the absence of other cytoplasmic components, provided ATP hydrolysis was uninhibited. Though mutants deficient in multiple proteins involved in the formation and function of the cytokinetic ring were made, only inhibition of ATP hydrolysis, tropomyosin, and actin crosslinking activity rendered the rings unable to form or constrict (Mishra et al., 2013). These ‘minimal’ contractile rings, therefore, required only F-actin, myosin II, ATPase activity, and actin crosslinking (Table 1).
This minimal contractile ring apparatus can generate force in the absence of accessory proteins. In one model, myosin mini-filaments are formed when the head region binds to actin and two heavy chains dimerize and interact with essential light chains and regulatory chains to form a functional hexamer unit, which can further polymerize (Schwayer et al., 2016; Pollard and O’Shaughnessy, 2019). Actin filaments are bound by these myosin ‘mini-filaments’ which pull anti-parallel actin strands together and reduce the ring diameter. A second model suggests depolymerization of actin filaments cross-linked by proteins bound to their minus ends could generate tension and drive ring constriction (Schwayer et al., 2016). In this model, myosin pulls on filaments adjacent to those depolymerizing, triggering disassembly of the cross-linked actin filaments (Zumdieck et al., 2007; Pinto et al., 2013; Lenz, 2020).
These hypothesized force generation mechanisms are unlikely to have exact parallels in Apicomplexans, considering how divergent Apicomplexans are from other eukaryotes. Plasmodium falciparum’s actin protein, for example, is only 70-80% similar to S. cerevisiae actin whereas human actin is over 90% similar (Das et al., 2017). Since Apicomplexans lack myosin II, it is difficult to speculate on the contribution of their non-canonical myosins to this process. Even if Apicomplexan myosins do not generate force during segmentation, actin depolymerization alone could potentially generate enough force to induce constriction, utilizing similar protrusive force as required for swift movement of Listeria (Mogilner et al., 2003; Ennomani et al., 2016). However, even this “elastic Brownian ratchet” mechanism requires the presence of ActA, Arp2/3, ADF, cofilin, and members of the Ena/Mena/VASP family, some of which have no Plasmodium homologues (Table 1). As previously mentioned, disrupting actin networks during division resulted in mis-localization of basal complex proteins in Toxoplasma, bolstering the idea that actin networks and actin depolymerization may play a significant role in the formation/contraction of the ring. Ultimately, more basal complex proteins need to be identified before a mechanism can confidently be proposed.
The Basal Complex: Apicomplexans’ Cytokinetic Contractile Ring
Apicomplexa possess a structure analogous to the actomyosin ring common to eukaryotic cytokinesis, the basal complex, but it is still only hypothesized to act as a contractile ring. The basal complex is novel but poorly understood. While the structural details of cell division in Apicomplexa have been thoroughly examined using transmission electron microscopy, only recently have advances in genetics and microscopy enabled the molecular and mechanistic basis of these processes to begin to be understood in these organisms (Trager et al., 1966; Sheffield and Melton, 1968; Aikawa, 1971; Striepen et al., 2007). It is clear though that the proper formation and function of the basal complex is essential for successful segmentation in Toxoplasma and Plasmodium (Hu, 2008; Rudlaff et al., 2019).
The description of the basal complex in Apicomplexan parasites requires some basic definitions and descriptions of cellular structures specific to Apicomplexa and required for cytokinesis. (Figures 1, 2). The Apicomplexan cortical cytoskeleton includes a unique, specialized membrane system immediately beneath the plasma membrane and composed of flattened vesicles or alveoli, known as the inner membrane complex. The inner membrane complex, or IMC, is made of multiple fused membrane ‘plates’ in Toxoplasma spp but in Plasmodium (aside from the transmission gametocyte stage), the IMC is made of a single vesicle (Porchet and Torpier, 1977; Bannister and Mitchell, 1995; Kono et al., 2012; Harding and Meissner, 2014). This membrane system provides structure, shape, and stability to the parasite, acts as a scaffold for proteins both within and attached to the membrane system during division, and is where the force generating complex required for invasion is located (Bergman et al., 2003; Baum et al., 2006). Beneath the flattened vesicles of the IMC, the subpellicular network (SPN) made of interwoven filaments of proteins known as alveolins, which are similar to intermediate filaments, stabilizes this system (Mann and Beckers, 2001). They also help maintain the shape and structure of the parasite and may be involved in other processes. Internal to the SPN, the Apicomplexans have one or more subpellicular microtubules that extend longitudinally down the long axis of the tachyzoite in Toxoplasma or the merozoite in Plasmodium.
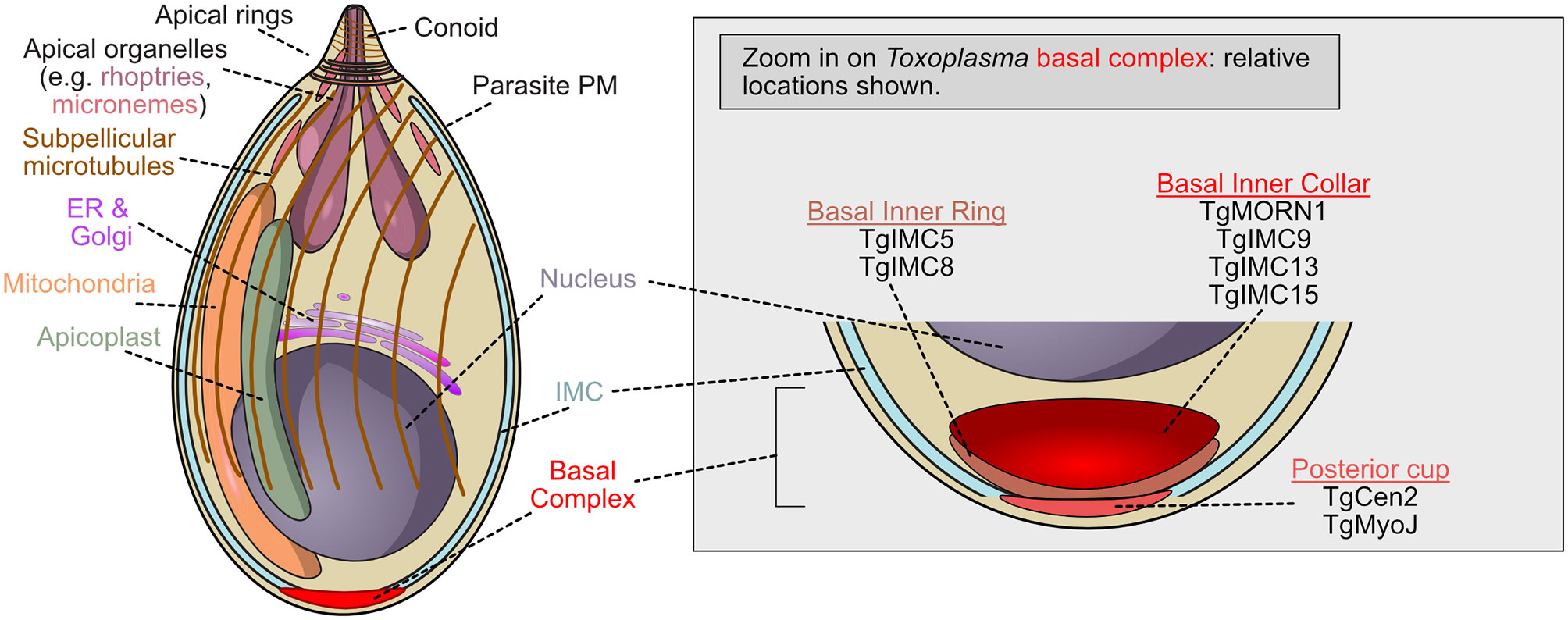
Figure 1 Schematic of T. gondii tachyzoite demonstrating the localization of basal complex proteins. Toxoplasma gondii is the Apicomplexan whose cell biology is best understood, but molecular function of the basal complex components remain poorly understood. Many basal complex proteins have been identified but little is known about their organization or function. The zoomed view of the basal complex is shown when cytokinesis is complete (or nearly complete). At this stage, TgMORN1, one of the first proteins recruited to the complex, is located with TgIMC9, TgIMC13, and TgIMC15 in the basal inner collar. The posterior cup contains two proteins which may provide contractile force, TgMyoJ and TgCentrin2. Between these two groups of proteins, TgIMC5 and TgIMC8 localize to the basal inner ring.
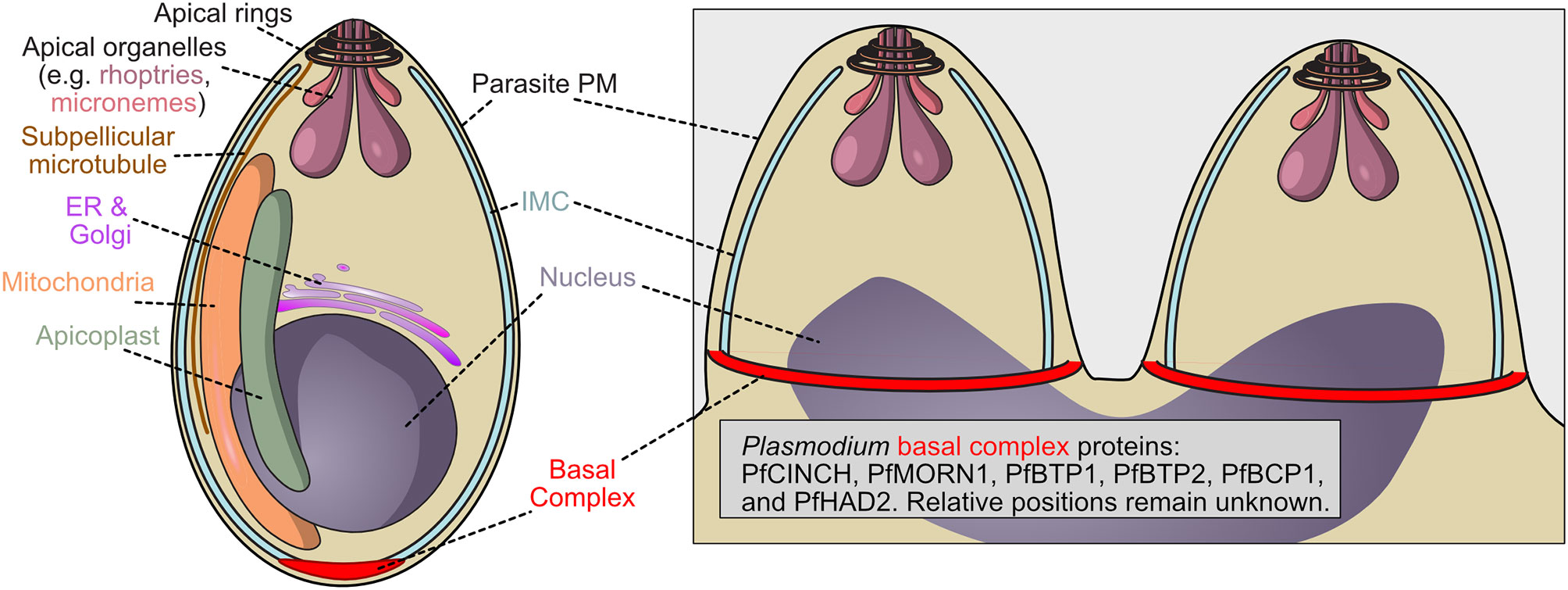
Figure 2 Schematic of P. falciparum merozoite demonstrating the localization of the basal complex. The basal complex of Plasmodium falciparum has not been as extensively characterized as that of Toxoplasma. Proteins PfMORN1, PfBTP1, PfCINCH, PfBTP2, PfBCP1, and PfHAD2 have been identified as members of the basal complex, visualized at the leading edge of the inner membrane complex, but their function, relative location within the structure, and order of assembly remain unknown.
The Toxoplasma gondii Basal Complex
Toxoplasma parasites replicate asexually in a process called ‘endodyogeny’, wherein two daughter cells are formed inside the parent (Goldman et al., 1958; Sheffield and Melton, 1968; van den Zypen and Piekarski, 1968). In the intestinal epithelium of the cat, the definitive host, Toxoplasma reproduces via schizogony, like Plasmodium, and the released merozoites begin gamete formation (Aikawa, 1971; Dubey et al., 1998). However, these stages of Toxoplasma development have been studied much less than the asexual replication of Toxoplasma tachyzoites in intermediate hosts, and much less is known about this process, so this overview will focus on that stage.
During endodyogeny, centrosome replication occurs in the late G1 and early S phase, and the replicated centrosome helps assemble microtubules, the spindle, and the daughter cytoskeleton in general (Francia et al., 2012; Morlon-Guyot et al., 2016). Immediately following centrosome replication, the mitotic spindle begins to assemble and cytokinesis begins. The daughter basal complex forms relatively early in endodyogeny, before the assembly of daughter cell microtubules and the IMC, and before the mother cell’s cytoskeleton disassembles (Hu, 2008). DNA replication proceeds during S phase until the parasite is about 1.8N, which is reached right before daughter cells emerge (Radke et al., 2001; Blader et al., 2015). Karyokinesis is finally completed when the endoplasmic reticulum has finished dividing and the subpellicular microtubules which give the new parasites shape and structure have reached far into the growing daughter cell(s) (Radke et al., 2001; Blader et al., 2015). Above the subpellicular microtubules lies the inner membrane complex, a system of flattened rectangular membranous sacs that encircle the entire parasite, providing shape and structure, with openings at the apical and basal end (Bommer et al., 1968; Anderson-White et al., 2012).
Work in Toxoplasma gondii identified the basal complex as electron dense ring-shaped structures at the basal end of nascent parasites (Gubbels et al., 2006; Hu, 2008; Anderson-White et al., 2011; Hammarton, 2019). This basal complex, which accumulates at the leading edge of the developing daughter cell as it buds, is first marked by the unique localization of TgMORN1 (TGME49_310440), which occupies the basal gap of the inner membrane complex and constricts until it reaches the basal pole (Hu, 2008; Anderson-White et al., 2012). The formation and integrity of the inner membrane complex, or IMC, is thought to be connected to the function of the basal complex as it is essential for the proper formation of parasites during endodyogeny (Anderson-White et al., 2012; Dubey et al., 2017). Though its actual function is unknown, TgMORN1 is the earliest known marker of the T. gondii basal complex, and likely one of the first cytoskeletal structures assembled. TgMORN1 forms rings around duplicated centrioles before polarization occurs, significantly contributes to the structure of the complex, and co-localizes with MyoC, a noncanonical myosin which may, in conjunction with TgMORN1, provide the force necessary for basal complex constriction (Figure 1) (Gubbels et al., 2006; Hu, 2008). TgMORN1 is not unique to the basal complex, however; it also localizes to the centrocone, a structure that helps to organize the mitotic spindle in apicomplexans (Gubbels et al., 2006; Naumov et al., 2017).
In T. gondii, MORN1 and other basal complex proteins likely remain at the growing end of the cortical cytoskeleton, driven toward the basal end of the parasite by the addition of tubulin monomers to the subpellicular microtubules (Gubbels et al., 2006). However, TgMORN1 rings can assemble without cortical cytoskeleton growth, suggesting their maintenance is independent of, yet simultaneous with, cortical cytoskeleton assembly (Gubbels et al., 2006; Hu, 2008).
A host of other proteins have been identified as members of the T. gondii basal complex: TgHAD2a, TgDLC, TgCentrin2, TgIMC5, TgIMC8, TgIMC9, TgIMC13, TgIMC15, Tg14-3-3, TgMSC1a, TgMyoC, and TgMyoJ (Delbac et al., 2001; Hu, 2008; Lorestani et al., 2010; Anderson-White et al., 2011; Engelberg et al., 2016). However, only a few have been shown to be essential and/or are likely to play a role in generating contractile force.
TgCentrin2 (TGME49_250340) is recruited to the basal complex at the end of interphase, and occupies a compartment posterior (i.e. more basal) to that containing TgMORN1 (Figure 1). TgCentrin2 is hypothesized to contribute to contraction and may respond to changes in local Ca2+ concentrations via its EF-hand domain (Hu, 2008). Since elevating intracellular Ca2+ levels can artificially induce basal complex constriction, TgCentrin2 could drive this process.
TgDLC (TGME49_223000), a dynein light chain protein, and TgMyoJ (TGME49_257470), a non-canonical myosin, also localize to the basal complex (Hu, 2008; Frénal et al., 2017). TgMyoJ colocalizes with TgCentrin2 and in its absence, TgCentrin2 localization is abrogated, and constriction fails. Basal complex constriction also fails when TgCentrin2 is knocked down, suggesting both proteins are required for the basal complex to function appropriately (Frénal et al., 2017).
Destabilization of actin networks with cytochalasin D in T. gondii prevented both constriction and TgCentrin2 localization, suggesting basal complex formation and function depends on actin dynamics (Frénal et al., 2017). Though the specific roles of both actin and centrin2 in Toxoplasma are still unclear, recent research has broadened our understanding of their role in division. In the case of actin, since the actin cytoskeleton is composed of fibers that are inherently shorter and less stable than those of other organisms, it has been difficult to visualize actin in vivo in Apicomplexans. Recent work utilizing an actin chromobody has highlighted that while actin may be involved in the process of division, it seems to not form an actin ring at the basal end of the parasite (Periz et al., 2017). Basal morphology is negatively impacted by the loss of actin, but actin localizes to first the IMC and then the residual body over the course of Toxoplasma division; therefore the function of actin in the process of contractile ring formation and function is likely distinct from its key structural role in other eukaryotic contractile ring systems (Periz et al., 2017). In the case of Centrin2, the protein localizes to multiple locations within the parasite, including the preconoidal ring at the apical end, the basal complex, the centrioles, and peripheral annuli (Arnot et al., 2011; Leung et al., 2019). While division defects that may reflect a loss of basal complex activity are present in Cen2 knockdown parasites, it is difficult to assign these defects to the basal complex specifically because of the complex localization of Cen2 (Arnot et al., 2011; Leung et al., 2019). Within the basal complex, TgCentrin2 is hypothesized to act as a light chain for TgMyoJ, but this has not yet been demonstrated (Hu, 2008; Frénal et al., 2017).
The Plasmodium falciparum Basal Complex
The basal complex and its composite proteins are less well characterized in Plasmodium than in Toxoplasma, and while both are Apicomplexans, during the asexual stage, Toxoplasma divides via endodyogeny, whereas Plasmodium asexual replication utilizes schizogony (as does Toxoplasma during the sexual stage). During schizogony, parasite nuclei divide asynchronously within the mother parasite, generating a multi-nucleated cell. The inner membrane complex, which in Plasmodium consists of a coherent vesicle rather than a series of plates, still provides shape and structure to the merozoites produced by schizogony, and the basal complex do not form until the final semi-synchronous round of nuclear division (Figure 2) (Harding and Meissner, 2014; Rudlaff et al., 2020). In this final division, the basal complex is thought to draw the inner membrane complex down around each merozoite, generating 18-32 new parasites. Both Apicomplexans, however, possess putative contractile rings that draw the inner membrane complex around the parasite and share some basal complex proteins.
Plasmodium MORN1 (PfMORN1; PF3D7_1031200), a homologue of TgMORN1, localizes to the basal complex only while TgMORN1 also localizes to the apical complex, a structure unique to and defining of the Apicomplexa which is located at the apical end of the cell and is involved in the invasion of host cells (Dubremetz et al., 1998; Ferguson et al., 2008; Katris et al., 2014) (Figure 2). PfCentrin2 (PF3D7_1446600) and PfMyoJ (PF3D7_1229800), whose homologs could be generating constrictive force in Toxoplasma, have been identified in co-immunoprecipitation experiments with basal complex proteins (Rudlaff et al., 2019). However, while TgCentrin2 localizes clearly to the basal complex, early studies demonstrate that PfCentrin2 localizes to the centrosome and is more strongly associated with the nucleus (Mahajan et al., 2008). Considering its interaction with basal complex proteins, Plasmodium Centrin 2 either indirectly assists in basal complex formation and function or further analysis or higher resolution imaging may be needed to determine the exact localization of PfCentrin2 during schizogony.
Further complicating Apicomplexan contractile ring theories is that knocking out the MyoJ ortholog PBANKA_1444500 in P. berghei does not inhibit basal complex constriction phenotype nor successful schizogony (Wall et al., 2019). Though transcriptional data suggests it to be highly expressed in schizogony, PbMyoJ-GFP protein was undetectable via immunofluorescence. We note that the mass spectrometry identification of PfMyoJ as an interacting partner of basal complex protein PfCINCH during schizogony demonstrates that in P. falciparum, MyoJ is expressed here (Rudlaff et al., 2019). In contrast to PbMyoJ, the P. berghei orthologs of MyoA, MyoF, and MyoK were all essential to asexual division, although neither are likely basal complex myosin candidates (Wall et al., 2019). The function of PfMyoA (PF3D7_1342600) as part of the motor for parasite invasion, for example, has been well-established (Baum et al., 2006; Jones et al., 2006; Bookwalter et al., 2017; Perrin et al., 2018; Robert-Paganin et al., 2019). Therefore, whether the centrin and/or myosin-based force generation hypotheses for the Toxoplasma basal complex apply to Plasmodium remains unknown.
Other identified Plasmodium basal complex proteins are specific to the genus, such as PfBCP1 (PF3D7_1436200), PfBTP1 (PF3D7_0611600) and 2 (PF3D7_0704100), PfHAD2 (PF3D7_1205200) and PfCINCH (PF3D7_0407800), which interacts or at least colocalizes with these proteins, as well as PfMORN1 (Figure 2) (Guggisberg et al., 2017). We note that there is another Plasmodium protein that has also been named HAD2 (PF3D7_1226300) that is unrelated to the basal complex (Engelberg et al., 2016). PfCINCH is essential to Plasmodium cell division and is a crucial member of the basal complex, although its molecular mechanism remains unknown (Rudlaff et al., 2019). The importance of a Plasmodium specific protein potentially suggests that basal complex assembly and constriction mechanisms differ among Apicomplexa.
As in Toxoplasma, the structure and complete protein composition of the basal complex have not yet been uncovered, but actin dynamics may play a role. The best characterized role for actomyosin in Plasmodium is in gliding motility and invasion, which uses the motor activity of MyoA (Baum et al., 2006; Robert-Paganin et al., 2019). However, there is evidence for actin and associated proteins being involved in division also. PfACT1 (PF3D7_1246200) and multiple actin binding proteins present in Plasmodium – profilin, cofilin, CAP, ADF1, ADF2, and others – are upregulated in schizogony (Gordon and Sibley, 2005; Wong et al., 2011). Actin filaments are already known to play a role in the segregation of apicoplasts, vestigial plant-like plastids that are essential for apicomplexan growth and replication (Lim and McFadden, 2010). PfACT1 and PfFRM2 (PF3D7_1219000) (actin I and formin homologs respectively) knockdown parasites fail to segment or segregate apicoplasts (Das et al., 2017). Without actin I, merozoites are malformed and conjoined, lacking individual inner membrane complexes, indicating a role for actin in separating nascent merozoites, and thus likely in basal complex formation and function (Ganter et al., 2015; Das et al., 2017). Formin 2’s nucleating activity in Plasmodium stabilizes the inherently unstable actin polymers created by the divergent PfActin, and its upregulation during and requirement for schizogony suggests actin and actin stabilizing proteins may play a role in the formation and function of the basal complex (Stortz et al., 2019).
The Most Well Characterized Contractile Rings: S. pombe and S. cerevisiae Models
The most extensive studies of the role of the cytokinetic contractile ring have occurred in yeast, specifically S. pombe and S. cerevisiae, making them an important comparand for studying the basal complex, and trying to determine how and via what mechanisms these rings function. In S. pombe, a species of yeast that divides by binary fission, contractile ring assembly and contraction have been studied in exquisite detail. First, the location for the ring is chosen by anillin-like Mid1p/Dmf1p which binds to the membrane in a series of nodes (Hachet and Simanis, 2008). This band of nodes is joined by myosin II heavy chain Myo2p, light chains Cdc4 and Rlc1, IQGAP, Rng2p, F-BAR protein Cdc15p, and formin Cdc12p (Laporte et al., 2010) (Table 1).
Though it divides by budding instead of fission, S. cerevisiae ring constriction/construction utilizes many S. pombe orthologs (Meitinger and Palani, 2016). Septins first assemble at the site of the bud neck, recruited by Rho-GTPase Cdc42, and form hetero-octameric filaments in a collar-like structure, which will surround the actomyosin ring (Wu et al., 2010; Oh and Bi, 2011). This scaffold recruits Bni5 which crosslinks the septin filaments and links with myosin light chain I, which in turn recruits myosin II with IQGAP homolog Iqg1 (Patasi et al., 2015) (Table 1). The importance of septins to contractile ring formation is species specific, however: in S. cerevisiae, Myo1p, the only type II myosin in this species, is recruited early on in formation of the contractile ring, recruited by septins (Bi et al., 1998; Lippincott and Li, 1998), whereas in S. pombe, Myo2 is recruited well before septins, which are nonessential for viability (Wu et al., 2003).
Myosin motors are involved in contractile ring assembly in both S. pombe and S. cerevisiae, but there are important differences. S. cerevisiae’s Myo1p appears at the ring quite early and before actin. In S. pombe, two class II and one class V myosin are required for assembly and contraction. Myo2, the conventional myosin-II homolog which is primarily responsible for assembly and contraction, is recruited first, before actin polymerization is initiated by Cdc12. Myo51, the class V myosin, is recruited once actin polymerization and node condensing begin, and Myp2 is recruited only once the contractile rings are fully formed, though each can form nodes and assemble the ring in the absence of the others (Laplante et al., 2015).
Initially, there are two distinct groups of parallel actin filaments of opposite directionality, arrayed in a semicircle (Kamasaki et al., 2007). After assembly, actin directionality becomes mixed, expedited by formin Cdc12p nucleation of actin filaments (Coffman et al., 2013). During contraction, populations of f-actin slide over each other, then depolymerize during contraction, which itself generates force (Kamasaki et al., 2007) (Figure 3). This action is capable of enabling ring constriction in S. cerevisae on its own, but in S. pombe, myosin 2 ATPase activity is required for the process of cytokinesis (Kitayama et al., 1997, Lord et al., 2005).
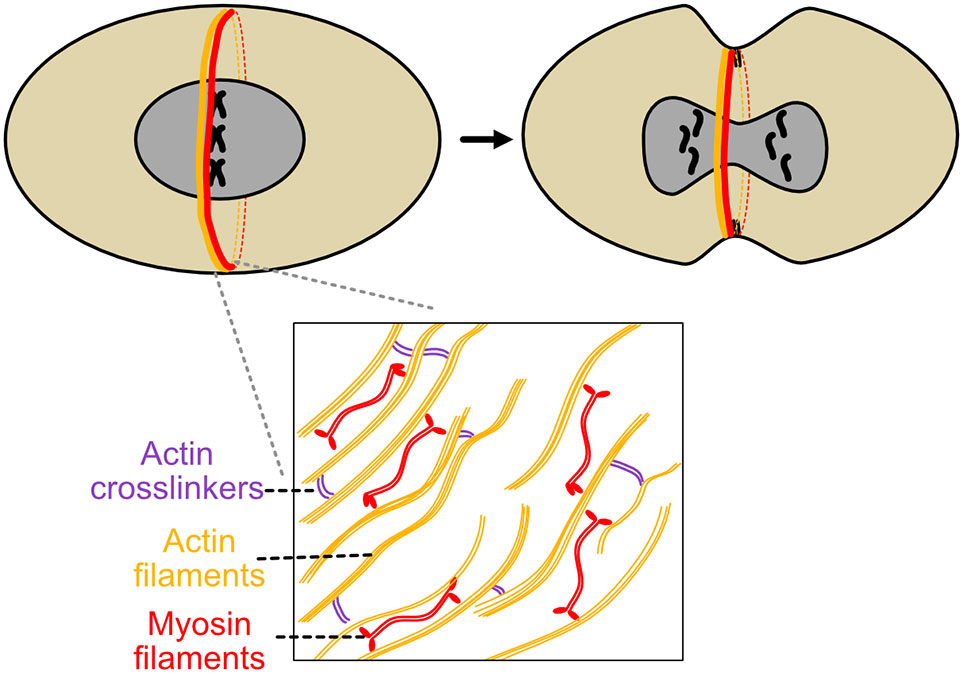
Figure 3 Actomyosin ring constriction during S. pombe cytokinesis. S. pombe’s contractile ring is perhaps the most extensively studied example of this structure. Actin and myosin are recruited to the nascent ring, actin filament directionality is modified by formins, and myosin II ATPase activity in conjunction with actin dynamics generates constrictive force. As constriction proceeds, glucan synthesis by bgs1 helps induce septum ingression and gives the nascent cell shape and structure.
Apicomplexans lack myosin II and tropomyosin. While their roles in Plasmodium are still unclear, in Toxoplasma, both MyoJ and Centrin 2 are involved in constricting the basal complex, and it is hypothesized that the EF-hand domain in TgCen2 could allow it to act as a myosin light chain for TgMyoJ and generate contractile force (Geimer and Melkonian, 2005; Frénal et al., 2017). In S. pombe, two unconventional myosins are utilized during division, Myp2p and Myo51. In particular, the fact the yeast can complete cytokinesis with Myo51 only does speak to the possibility of non-myosin II myosins being capable of providing force for cytokinetic ring constriction (Laplante et al., 2015).
Actin polymerization in S. pombe, required for contractile ring assembly and function, requires Arp2/3, Cdc12/For3, profilin, cofilin, and WASP – but not necessarily myosin II or IQGAP, as rings, albeit defective, can form even without myosin II nodes (Bathe and Chang, 2010). In S. cerevisiae, actin depolymerization may be the predominant mechanism underlying contraction (Mendes Pinto et al., 2012). Considering actin polymerization/depolymerization as a force generating mechanism; Plasmodium does have two formin proteins; one has homology to Cdc12 and the other to For3 (Baum et al., 2008). The former functions during invasion but the latter is highly expressed in late schizogony and could act in Plasmodium’s basal complex like For3 in the S. pombe contractile ring. Plasmodium also contains a cofilin homolog (PF3D7_1361400), capable of binding actin monomers and upregulated in late schizogony (Table 1). However, proteins which stabilize actin filaments (tropomyosin, a-actinin) lack apicomplexan homologs, so if the short, transient actin filaments of apicomplexans form the structure of the contractile ring, novel proteins are likely required for assembly and constriction.
Constriction speeds as anaphase concludes, and cytokinesis begins to occur in conjunction with this transition (Willet et al., 2015). Glucan (S. pombe) or chitin (S. cerevisiae) synthesizing proteins are recruited to the ring (Santos et al., 2003; Hercyk et al., 2019). These proteins induce ingression of the septum and membrane invagination, processes which are not only coupled to ring constriction, but also required for proper placement of the ring (Willet et al., 2015).
In yeast, ring constriction drives septum formation. In Apicomplexan division, while it is not known if the IMC is built up simultaneously with basal complex constriction, the basal complex assembles independently of microtubules and the IMC (Hu, 2008). The basal complex and apical complex are, however, separated as microtubules polymerize and the daughter cells grow, and in Toxoplasma, the constriction of the basal complex brings IMC plates together. During the asexual blood stage, however, the IMC in Plasmodium lacks plates and is made of a single vesicle (Bannister and Mitchell, 1995). It remains unknown if the ring drives formation of furrows between Plasmodium merozoites, for example, or the expansion of the IMC, as the cytokinetic ring drives formation of the furrow and septum ingression in S. pombe.
Contractile Rings in Metazoan Cytokinesis
While yeast cytokinesis may be the most well studied, the mechanisms of cytokinesis and the processes of contractile ring assembly can be more complicated in metazoans, if not in basic replicative cytokinesis, then in the creation of multiple cells from one predecessor during embryogenesis (Pollard, 2017). Thus, comparing Apicomplexan contractile rings to their metazoan counterparts may reveal similarities on this basis, since Apicomplexans also produce multiple progeny during both asexual and sexual division.
As in yeast, animal cell contractile rings consist of actin filaments, bipolar myosin II filaments, and septin filaments. Anillin crosslinkers can bind all three filament types, and other proteins which modulate actin dynamics play a similarly important role (D’Avino, 2009). Contractile ring assembly is controlled temporally by mitotic kinase levels. During metaphase, MKLP-1 is phosphorylated by Cdk1/Cyclin b, inhibiting its ability to bind microtubules (Kamijo et al., 2005). When anaphase begins, Cdk1 activity declines, phosphorylation cannot be maintained, and centralspindlin binds microtubules, in a process mediated by Aurora B kinase and 14-3-3 proteins (Basant et al., 2015). Centralspindlin interacts with Ect2, which enhances Rho turnover, allowing it to quickly cycle between inactive (GDP-bound) and active (GTP-bound) forms (Mishima et al, 2002). Active, GTP-bound Rho interacts with formins to polymerize actin and with myosin light chains to induce myosin II activation/filament formation at the equatorial cortex, generating the actin and myosin II filaments which form the contractile ring (Table 1) (Maupin and Pollard, 1986; Piekny et al., 2005; Makkonen et al., 2013).
Many of these kinases and phosphorylases involved in contractile ring assembly lack Apicomplexan orthologs, but both Toxoplasma and Plasmodium contain three Aurora-related Kinases (TgArk 1-3: TGME49_210280, TGME49_318770, TGME49_203010; PfArk 1-3: PF3D7_0605300, PF3D7_0309200, PF3D7_1356800) (Table 1) (Berry et al., 2016). While Aurora B kinase in animal cells helps recruit proteins to the nascent contractile ring none of the Plasmodium homologs localize to the basal complex, seemingly associated with other novel cytoskeleton development during cytokinesis (Berry et al., 2016).
Neither of the Toxoplasma Aurora kinase orthologs localize with TgMORN1 either, but if cytoskeletal assembly coordinated by the centrioles is involved in basal complex formation, it could be that Pf/TgArk plays an indirect role in basal complex formation. In the absence of TgArk3, T. gondii parasites failed to form the typical “rosette” structure of dividing tachyzoites, a morphological defect that could indicate a role for Apicomplexan aurora kinases in organizing the basal complex (Berry et al., 2016). Basal complex integrity needs to be examined in Ark-knockdown parasites to determine if Pf/TgArks are involved in its assembly and function.
Once all proteins have been recruited to the nascent metazoan actomyosin ring, assembly ends, and cytokinesis begins. Actin modulating proteins like profilin, formins, cofilin, and anillin parallel their yeast counterparts during ring constriction (Hachet and Simanis, 2008; Piekny and Glotzer, 2008). The ring contracts when bipolar myosin II filaments slide along antiparallel actin filaments. F-actin disassembly balances polymerization; both are required for contraction (Khaliullin et al., 2018; Pollard and O’Shaughnessy, 2019). Though metazoan contractile ring force generation mechanisms have not been fully elucidated, it is posited that either myosin II generates contractile stress around bundles of randomly-oriented actin filaments, or that contractile stress is generated by actin dynamics alone (Oelz et al., 2015; Pollard and O’Shaughnessy, 2019).
As mentioned, some metazoan systems utilize more complex cell division processes. For example, during Drosophila melanogaster embryonic development, the multi-nucleated syncytial blastoderm divides to form several individual cells, each with a single nucleus, in a process known as cellularization (Mazumdar and Mazumdar, 2002). Like Apicomplexan schizogony, cellularization relies on a contractile ring to draw down membranes and create individual cells from a collection of nuclei (Chougule et al., 2016; Carter et al., 2019). There are two “phases” of ring constriction during cellularization, whose contributions depend on initial ring size (Martin, 2016; Xue and Sokac, 2016). Since initial ring size determines constriction rate, the contractile ring must retain a ‘structural memory’ of its initial size, allowing it to constrict as fast as required for successful mitosis (Carvalho et al., 2009). Thus, it is thought that the ring is assembled from a number of ‘contractile units’ of fixed size and which shorten at a constant rate according to actin depolymerization (Carvalho et al., 2009). This could be compared to Apicomplexan schizogony – while each merozoite tends to be the same size, the final number of merozoites formed depends on how many merozoites the parasite ‘chose’ to make before its final division. Rings formed of contractile units could assemble in as great a number as necessary to surround each merozoite, and still constrict contemporaneously.
Contractile Action in Mitochondrial Division
Considering the divergent nature of apicomplexan biology, it isn’t surprising that there are striking similarities between apicomplexan contractile rings/division structures and those required in more ancient forms of division. Whether or not a contractile ring is involved in the process of mitochondrial fission, many of the proteins utilized in actomyosin ring – mediated cytokinesis in eukaryotic cells are also involved in mitochondrial fission, from myosin II to septins to actin modulating proteins such as Arp2/3, cofilin, and cortactin (Table 1) (Mears et al., 2011; Pagliuso et al., 2018).
The primary and best-characterized action of mitochondrial fission relies on the action of dynamin related protein Drp1 which regulates constriction and remodeling of the mitochondria via oligomerization (Mears et al., 2011). Drp1 is a GTPase; upon the hydrolysis of GTP, a conformational change induces compaction of Drp1 multimeric spirals and constriction of the mitochondrion, supporting the idea of a contractile mechanism in fission (Mears et al., 2011). Further effectors of Drp1 recruitment are ER tubules which help determine the site of fission and the presence of actin and myosin (Mears et al., 2011).
Like in cytokinesis, septins regulate fission by interacting with Drp1 and as in cellularization, transient actin polymerization coincides with mitochondrial fission (Gandre-Babbe and van der Bliek, 2008; Pagliuso et al., 2016).
Mitochondrial fission relies on the constricting force of an actin and myosin II complex- as well as spiraling Drp1 oligomers regulated by septins, although dynamin performs the terminal abscission step (Pagliuso et al., 2016; Pagliuso et al., 2018).
This could be an attractive comparand for apicomplexan division: There are two functional dynamin proteins in P. falciparum, both upregulated during schizogony and homologous to human dynamin proteins (Table 1). PfDYN2 (PF3D7_1037500) contains the GTPase and GED domains consistent with dynamins but lacks the C-terminal PRD and domains associated with “classical” dynamins, resembling human Drp1 in structure (Charneau et al., 2007). PfDYN1 (PF3D7_1145400) has a longer C-terminal domain and localizes to membrane compartments whereas PfDYN2 forms punctate structures in the cytoplasm and colocalizes with the ER/Golgi body and apicoplast (Li et al., 2004; Charneau et al., 2007). While the necessity of PfDYN2 has not been determined, knockdown of PfDYN1 hindered parasite replication, thus the dynamins are not redundant (Li et al., 2004). Examining Plasmodium dynamins in late schizogony could help determine whether dynamin/DLPs are involved in the basal complex
In Toxoplasma, more is known about how dynamin-like proteins, particularly a Drp1 homolog called DrpC, contribute to overall division (Table 1) (Breinich et al., 2009; Heredero-Bermejo et al., 2019). Like Plasmodium DYN2, TgDrpC (TGME49_270690) localizes to cytoplasmic puncta, but in dividing parasites it redistributes to the growing edge of daughter cells. TgDrpC also co-localizes with TgMORN1, an early marker of the Toxoplasma basal complex, and forms similar ring structures. Conditional knockdown of TgDrpC induced mitochondrial fragmentation, but also caused defects in apicoplast division and IMC formation which altogether halted division, suggesting a fundamental role for DrpC in endodyogeny (Heredero-Bermejo et al., 2019). Like TgMORN1, TgDrpC normally relocates from the cytoplasm to ring-like structures at the growing ends of daughter buds, but the phenotypic consequences of the TgDrpC knockdown are quite broad, so while this could suggest a role within the basal complex for DrpC, it may also be the result of broader defects in endodyogeny than a direct relationship with the basal complex.
However, there are complicating factors, namely a separate paper wherein conditional knockdown of DrpC did not impact any organelles other than the mitochondria (Melatti et al., 2019). While it is possible that TgDrpC could, like Drp1 during mitochondrial fission, recruit proteins to help form the contractile ring and generate force, conflicting information that may result from different strategies used to generate DrpC knockdown lines or the genetic modifications (ie, addition of fluorescent proteins) themselves makes it difficult to make a true prediction without more information (Heredero-Bermejo et al., 2019; Melatti et al., 2019). TgDrpC is conserved in Plasmodium as protein PF3D7_1218500, which has a similar transcriptomic expression to basal complex proteins. Further studies on this Plasmodium homolog (PfDrpC) will determine if its functions in Plasmodium mirror those in Toxoplasma.
In Toxoplasma as well, some proteins in the basal complex are also directly involved in the division of the apicoplast, but making direct conclusions is difficult considering the lack of mechanistic knowledge as yet. TgMORN1 is required for successful apicoplast segregation, and it had been hypothesized that the growing daughter cell, led at the basal end by the constricting TgMORN1-containing basal complex, has a functional role in apicoplast division and generates the force for apicoplast fission (van Dooren et al., 2009). However, while MORN1 is essential for apicoplast segregation, DrpA is likely primarily responsible for generating force, as DrpA’s knockdown did not inhibit general cell division, but did inhibit apicoplast fission (van Dooren et al., 2009). TgMORN1 does not surround the apicoplast tightly and any specific role in recruiting DrpA or constricting the apicoplast has not been determined; though it is clearly relevant to the process, it may not be directly related to its role in the basal complex, and it is likely dynamin-like protein DrpA which performs the final separation (van Dooren et al., 2009; Lorestani et al., 2010). While TgMORN1 is required, its specific role in apicoplast division, whether or not it is tied to its basal complex functions, remains to be determined.
With these caveats outlined, it is true that in TgMORN1-deficient parasites, not only did apicoplast segregation fail, but the tachyzoite daughters themselves failed to separate/constrict (Heaslip et al., 2010; Lorestani et al., 2010). The fact that, in Toxoplasma, the apicoplast and the entire parasite utilize at least one significant, shared protein between them (TgMORN1) to divide, and both division processes require dynamin-related proteins, suggests that the basal complex may function similarly to contractile ring structures in mitochondrial division, even if the proteins utilized in each process vary. However, this hypothesis illustrates the necessity of considering Apicomplexan diversity: in Plasmodium, PfMORN1localizes only to the basal complex; thus apicoplast segregation may not be connected at all to the constriction of the basal complex
The Contractile Ring in Florideophyte Red Algae
Though there may be interesting points of comparison between Apicomplexans and Opisthokontae (a clade containing both the fungi and animal kingdoms), Apicomplexans are extremely phylogenetically distant from them. Looking outside of Opisthokonta for well-studied organisms, we could compare Apicomplexan contractile rings to those of red algae, which are much more closely related to the Alveolata, which includes Apicomplexans. Indeed, red algae and alveolates are contained within the same recently- described “megagroup”, distinct from that containing fungi and animals (Burki et al., 2008).
Contractile rings for division exist in florideophyte red algae, whose division may resemble the separation of Plasmodium merozoites after multiple karyokineses (Garbary and McDonald, 1996). Florideophyte actin rings are formed early in cytokinesis, and in the Tiffaniella/Griffithsia genera, nuclei move out of the furrowing zone in preparation for the formation of the actin ring, although specific mechanisms remain unknown (Garbary and McDonald, 1996).
In C. merolae, the contractile ring used to divide the chloroplast has been studied with a significant degree of detail, and much more is known about its formation, function, and accessory proteins (Kuroiwa, 1998; Sumiya et al., 2014; Yoshida and Mogi, 2019). Plastid dividing rings contain a dynamic ‘trio’ of rings: the PD or MD ring (PDR1/MDR1), the FtsZ ring (FtsZ1/2 or A/B), and the dynamin ring (Dnm2), which spans the double membrane (Osteryoung and Pyke, 2014; Yoshida, 2018). While few of these proteins or their have direct Apicomplexan orthologs, microtubule-like FtsZ and dynamin-like DNM2 may provide intriguing comparands (Imoto et al., 2011; TerBush et al., 2013) (Table 1).
In bacteria, FtsZ forms rings at the cell midpoint. In chloroplasts, eukaryotic homologs FtsZA and B work together to form the FtsZ ring. They form protofilaments which bundle into the ring in a 1:1 ratio, similar to microtubule assembly (Yoshida et al., 2016). This outer ring in conjunction with PDR1 then recruits Dnm2, a dynamin-related protein, and helps cross-link outer PD ring filaments (Miyagishima et al., 2008). While Dnm2 is essential for plastid division, it, like Plasmodium Dyn1/Dyn2 and Toxoplasma DrpC, only has GTP binding/hydrolyzing domains, unlike conventional dynamins (Table 1). Apicomplexan dynamins could therefore be capable of playing a similar role in ring formation and contraction during schizogony (Miyagishima et al., 2008).
Mechanisms for contraction of this triple-ring system are unknown, but it is hypothesized that FtsZ and Dnm2 generate force and Dnm2’s GTP hydrolysis induces destabilization, disassembly, and release of FtsZ monomers (Yoshida et al., 2016; Yoshida, 2018). These actin and myosin- free contraction models may merit further study in the Apicomplexan context as well - Dnm2/DRP5B aligns with Plasmodium DYN1, and Dnm1 with Plasmodium DYN2, strengthening the idea that these proteins could generate constrictive force in the basal complex in the absence of myosin II (Table 1).
While many C. merolae plastid division proteins lack Apicomplexan orthologs, Arc6, a protein required for FtsZ ring assembly, has multiple orthologs in Babesia, another Apicomplexan. A search of this protein sequence in the Plasmodium database reveals PF3D7_0803200, a putative filament-former with exactly analogous timing to known basal complex proteins (Table 1). As the protein is completely uncharacterized, it would be interesting to determine if it functions similarly in the assembly of the basal complex, as its timing and homology suggests.
Cytokinetic Contractile Rings and Other Contractile Mechanisms in Dictyostelium
The slime mold Dictyostelium may be another useful model for the role of a contractile ring in Apicomplexan division. Amoebozoa are more distantly related to Apicomplexa than red algae, but Dictyostelium contains well-studied examples of myosin II-free cytokinetic division. Dictyostelium can perform 4 types of cytokinesis but only cytokinesis A involves a contractile ring. Though myosin II is required for cytokinesis A, associated proteins do have Apicomplexan homologs (Yumura, 2001). Dictyostelium has five dynamin-like proteins: DymA and DymB have a GTPase domain and a GTPase effector domain, like Plasmodium DYN1/2. DlpA, B, and C, have a GTPase domain only, like Toxoplasma DrpC (Table 1). All three of the dynamins involved in cytokinesis in Dictyostelium have GTPase domains required for cytokinesis (Rai et al., 2011; Rana et al., 2013). The role of dynamin-like proteins in cytokinesis A depends on their GTPase activity, and in Plasmodium and Toxoplasma all dynamin homologs have the GTPase domain conserved as well. DlpB and A both contribute to furrowing; they colocalize and in their absence, actin filaments fragment (Rai et al., 2011; Fujimoto et al., 2019). Reliance on stabilized actin filaments for division may seem an unlikely model for Apicomplexans where short, unstable actin filaments proliferate, but Plasmodium/Toxoplasma DrpC could perform a similarly stabilizing role (Rana et al., 2013).
Dictyostelium wound closure may also compare to Plasmodium asexual schizogony or Toxoplasma sexual schizogony in particular: in other eukaryotes, such as the D. melanogaster embryo, actomyosin ring contraction at the leading edge of the wound closes it but Dictyostelium utilizes a myosin independent ‘contractile ring’ reliant on calcium signaling (Darenfed and Mandato, 2005; Abreu-Blanco et al., 2012). Actin accumulates at the wound site filaments and forms a ring like structure, then a filled circle that grows and then decreases (Itoh and Yumura, 2007). Actin accumulation is dependent on Ca2+ signaling preceding it, just as Plasmodium schizogony relies on calcium signaling (Talukder et al., 2020). In the presence of latrunculin A, membrane pore size increases – thus therefore, actin may form a constrictive ring around the pore. This model where a contractile ring of actin leads a sheet of new membrane to fill the pore may echo how the Plasmodium basal complex draws down the IMC during schizogony (Talukder et al., 2020).
Discussion
Apicomplexan methods of cell division are profoundly distinct from those of other eukaryotes, making it difficult to elucidate their underlying mechanisms. The basal complex, present in Toxoplasma and Plasmodium, is integral to division but poorly understood as a result of Apicomplexan evolutionary divergence. By comparing what is known about the basal complex to well-studied contractile rings, it is hoped that possible comparands to the Apicomplexan basal complex could help guide research directions.
If the basal complex is a variation on an actomyosin contractile ring, one of the many non-canonical myosins in Apicomplexans will have to act as myosin II. In Toxoplasma, TgMyoJ is already hypothesized to help generate constrictive force. Plasmodium MyoJ, however, did not localize to the basal complex and is not predicted to be essential. Plasmodium MyoJ and Cen2’s relationship to other basal complex proteins should be re-examined, but there may be different methods of force generation among Apicomplexans.
Careful examination of contractile rings among eukaryotes can reveal interesting parallels such as between asexual Apicomplexan and mitochondrial division. Dynamin-like protein DrpC has already been shown to be required for successful Toxoplasma endodyogeny. Further study on the localization and function of its homolog in Plasmodium, and other dynamin like proteins identified and only partially characterized, could elucidate additional molecular details.
The biphasic constriction model identified in the process of embryo cellularization in Drosophila melanogaster can suggest mechanisms for the generation of constrictive force in the absence of canonical myosins in Apicomplexans. Apicomplexans contain multiple proteins capable of modulating actin dynamics, and a closer examination of the role of Actin I in cytokinesis could determine if actin depolymerization is largely responsible for constriction.
All of these are merely possibilities, hypothetical conclusions drawn from interesting similarities. We hope that they may serve as a rough starting point to understand basal complex function. In any case, it is clear that before the basal complex can be classified or even accurately compared with well-studied contractile rings in other systems, many more protein components of the complex must be identified. The newfound relative ease of genetic manipulation in both primary Apicomplexan genera, however, makes that task less daunting and more approachable than ever before.
Author Contributions
AM and JD wrote the manuscript. All authors contributed to the article and approved the submitted version.
Funding
The authors acknowledge funding from the National Institutes of Health to JD (R01 AI145941).
Conflict of Interest
The authors declare that the research was conducted in the absence of any commercial or financial relationships that could be construed as a potential conflict of interest.
Acknowledgments
We thank Rebecca Clements, Sreelakshmi Srinivasamurthy, and Ana Karla Cepeda Diaz for helpful comments and Bryan McGuffie for contribution to the figures.
References
Abreu-Blanco, M. T., Verboon, J. M., Liu, R., Watts, J. J., Parkhurst, S. M. (2012). Drosophila embryos close epithelial wounds using a combination of cellular protrusions and an actomyosin purse string. J. Cell Sci. 125, 5984–5997. doi: 10.1242/jcs.109066
Aikawa, M. (1971). Parasitological review. Plasmodium: the fine structure of malarial parasites. Exp. Parasitol. 30, 284–320. doi: 10.1016/0014-4894(71)90094-4
Anderson-White, B. R., Ivey, F. D., Cheng, K., Szatanek, T., Lorestani, A., Beckers, C. J., et al. (2011). A Family of Intermediate Filament-Like Proteins is Sequentially Assembled Into the Cytoskeleton of Toxoplasma gondii. Cell. Microbiol. 13, 18–31. doi: 10.1111/j.1462-5822.2010.01514.x
Anderson-White, B., Beck, J. R., Chen, C.-T., Meissner, M., Bradley, P. J., Gubbels, M.-J. (2012). Cytoskeleton Assembly in Toxoplasma gondii Cell Division. Int. Rev. Cell. Mol. Biol. 298, 1–31. doi: 10.1016/B978-0-12-394309-5.00001-8
Arnot, D. E., Ronander, E., Bengtsson, D. C. (2011). The progression of the intra-erythrocytic cell cycle of Plasmodium falciparum and the role of the centriolar plaques in asynchronous mitotic division during schizogony. Int. J. Parasitol. 41, 71–80. doi: 10.1016/j.ijpara.2010.07.012
Bannister, L. H., Mitchell, G. H. (1995). The role of the cytoskeleton in Plasmodium falciparum merozoite biology: an electron-microscopic view. Ann. Trop. Med. Parasitol. 89, 105–111. doi: 10.1080/00034983.1995.11812940
Basant, A., Lekomtsev, S., Tse, Y. C., Zhang, D., Longhini, K. M., Petronczki, M., et al. (2015). Aurora B Kinase Promotes Cytokinesis by Inducing Centralspindlin Oligomers that Associate with the Plasma Membrane. Dev. Cell. 33, 204–215. doi: 10.1016/j.devcel.2015.03.015
Bathe, M., Chang, F. (2010). Cytokinesis and the contractile ring in fission yeast: towards a systems-level understanding. Trends Microbiol. 18, 38–45. doi: 10.1016/j.tim.2009.10.002
Baum, J., Richard, D., Healer, J., Rug, M., Krnajski, Z., Gilberger, T.-W., et al. (2006). A Conserved Molecular Motor Drives Cell Invasion and Gliding Motility across Malaria Life Cycle Stages and Other Apicomplexan Parasites. J. Biol. Chem. 281, 5197–5208. doi: 10.1074/jbc.M509807200
Baum, J., Tonkin, C. J., Paul, A. S., Rug, M., Smith, B. J., Gould, S. B., et al. (2008). A Malaria Parasite Formin Regulates Actin Polymerization and Localizes to the Parasite-Erythrocyte Moving Junction during Invasion. Cell Host Microbe 3, 188–198. doi: 10.1016/j.chom.2008.02.006
Bergman, L. W., Kaiser, K., Fujioka, H., Coppens, I., Daly, T. M., Fox, S., et al. (2003). Myosin A Tail Domain Interacting Protein (MTIP) Localizes to the Inner Membrane Complex of Plasmodium sporozoites. J. Cell. Sci. 116, 39–49. doi: 10.1242/jcs.00194
Berry, L., Chen, C.-T., Reininger, L., Carvalho, T. G., El Hajj, H., Morlon-Guyot, J., et al. (2016). The conserved apicomplexan Aurora kinase TgArk3 is involved in endodyogeny, duplication rate and parasite virulence. Cell. Microbiol. 18, 1106–1120. doi: 10.1111/cmi.12571
Bi, E., Maddox, P., Lew, D. J., Salmon, E. D., McMillan, J. N., Yeh, E., et al. (1998). Involvement of an Actomyosin Contractile Ring in Saccharomyces cerevisiae Cytokinesis. J. Cell Biol. 142, 1301–1312. doi: 10.1083/jcb.142.5.1301
Blader, I., Coleman, B., Chen, C.-T., Gubbels, M.-J. (2015). The lytic cycle of Toxoplasma gondii: 15 years later. Annu. Rev. Microbiol. 69, 463–485. doi: 10.1146/annurev-micro-091014-104100
Bommer, W., Höfling, K. H., Heunert, H. H. (1968). In Vivo Observations on the Penetration of Toxoplasma Into the Host Cell. Dtsch Med. Wochenschr. 93, 2365–2367. doi: 10.1055/s-0028-1110941
Bookwalter, C. S., Tay, C. L., McCrorie, R., Previs, M. J., Lu, H., Krementsova, E. B., et al. (2017). Reconstitution of the core of the malaria parasite glideosome with recombinant Plasmodium class XIV myosin A and Plasmodium actin. J. Biol. Chem. 292, 19290–19303. doi: 10.1074/jbc.M117.813972
Breinich, M. S., Ferguson, D. J. P., Foth, B. J., van Dooren, G. G., Lebrun, M., Quon, D. V., et al. (2009). An alveolate specific dynamin is required for the biogenesis of specialised secretory organelles in Toxoplasma gondii. Curr. Biol. 19, 277–286. doi: 10.1016/j.cub.2009.01.039
Burki, F., Shalchian-Tabrizi, K., Pawlowski, J. (2008). Phylogenomics reveals a new ‘megagroup’ including most photosynthetic eukaryotes. Biol. Lett. 4, 366–369. doi: 10.1098/rsbl.2008.0224
Carter, T. Y., Gadwala, S., Chougule, A. B., Bui, A. P. N., Sanders, A. C., Chaerkady, R., et al. (2019). Actomyosin contraction during cellularization is regulated in part by Src64 control of Actin 5C protein levels. Genesis 57, e23297. doi: 10.1002/dvg.23297
Carvalho, A., Desai, A., Oegema, K. (2009). Structural Memory in the Contractile Ring Makes the Duration of Cytokinesis Independent of Cell Size. Cell 137, 926–937. doi: 10.1016/j.cell.2009.03.021
Charneau, S., Dourado Bastos, I. M., Mouray, E., Ribeiro, B. M., Santana, J. M., Grellier, P., et al. (2007). Characterization of PfDYN2, a dynamin-like protein of Plasmodium falciparum expressed in schizonts. Microbes Infect. 9, 797–805. doi: 10.1016/j.micinf.2007.02.020
Chougule, A. B., Hastert, M. C., Thomas, J. H. (2016). Drak Is Required for Actomyosin Organization During Drosophila Cellularization. G3 (Bethesda) 6, 819–828. doi: 10.1534/g3.115.026401
Coffman, V. C., Sees, J. A., Kovar, D. R., Wu, J.-Q. (2013). The formins Cdc12 and For3 cooperate during contractile ring assembly in cytokinesis. J. Cell Biol. 203, 101–114. doi: 10.1083/jcb.201305022
Darenfed, H., Mandato, C. A. (2005). Wound-induced contractile ring: a model for cytokinesis. Biochem. Cell Biol. 83, 711–720. doi: 10.1139/o05-164
Das, S., Lemgruber, L., Tay, C. L., Baum, J., Meissner, M. (2017). Multiple essential functions of Plasmodium falciparum actin-1 during malaria blood-stage development. BMC Biol. 15, 70. doi: 10.1186/s12915-017-0406-2
D’Avino, P. P. (2009). How to scaffold the contractile ring for a safe cytokinesis - lessons from Anillin-related proteins. J. Cell. Sci. 122, 1071–1079. doi: 10.1242/jcs.034785
Delbac, E, Sänger, A., Neuhaus, E. M., Stratmann, R., Ajioka, J. W., Toursel, C., et al. (2001). Toxoplasma gondii Myosins B/C. J. Cell Biol. 155, 613–624. doi: 10.1083/jcb.200012116
Dubey, J. P., Lindsay, D. S., Speer, C. A. (1998). Structures of Toxoplasma gondii Tachyzoites, Bradyzoites, and Sporozoites and Biology and Development of Tissue Cysts. Clin. Microbiol. Rev. 11, 267–299
Dubey, R., Harrison, B., Dangoudoubiyam, S., Bandini, G., Cheng, K., Kosber, A., et al. (2017). Differential Roles for Inner Membrane Complex Proteins across Toxoplasma gondii and Sarcocystis neurona Development. mSphere 2, ee00409–17. doi: 10.1128/mSphere.00409-17
Dubremetz, J. F., Garcia-Réguet, N., Conseil, V., Fourmaux, M. N. (1998). Apical organelles and host-cell invasion by Apicomplexa. Int. J. Parasitol. 28, 1007–1013. doi: 10.1016/s0020-7519(98)00076-9
Engelberg, K., Ivey, F. D., Lin, A., Kono, M., Lorestani, A., Faugno-Fusci, D., et al. (2016). A MORN1-associated HAD phosphatase in the basal complex is essential for Toxoplasma gondii daughter budding. Cell Microbiol. 18, 1153–1171. doi: 10.1111/cmi.12574
Ennomani, H., Letort, G., Guérin, C., Martiel, J.-L., Cao, W., Nédélec, F., et al. (2016). Architecture and Connectivity Govern Actin Network Contractility. Curr. Biol. 26, 616–626. doi: 10.1016/j.cub.2015.12.069
Ferguson, D. J. P., Sahoo, N., Pinches, R. A., Bumstead, J. M., Tomley, F. M., Gubbels, M.-J. (2008). MORN1 Has a Conserved Role in Asexual and Sexual Development across the Apicomplexa. Eukaryot. Cell. 7, 698–711. doi: 10.1128/EC.00021-08
Francia, M. E., Striepen, B. (2014). Cell division in apicomplexan parasites. Nat. Rev. Microbiol. 12, 125–136. doi: 10.1038/nrmicro3184
Francia, M. E., Jordan, C. N., Patel, J. D., Sheiner, L., Demerly, J. L., Fellows, J. D., et al. (2012). Cell division in Apicomplexan parasites is organized by a homolog of the striated rootlet fiber of algal flagella. PloS Biol. 10, e1001444. doi: 10.1371/journal.pbio.1001444
Frénal, K., Jacot, D., Hammoudi, P.-M., Graindorge, A., Maco, B., Soldati-Favre, D. (2017). Myosin-dependent cell-cell communication controls synchronicity of division in acute and chronic stages of Toxoplasma gondii. Nat. Commun. 8, 1–18. doi: 10.1038/ncomms15710
Frénal, K., Krishnan, A., Soldati-Favre, D. (2020). “The Actomyosin Systems in Apicomplexa,” in Myosins Advances in Experimental Medicine and Biology. Ed. Coluccio, L. M. (Cham: Springer International Publishing), 331–354. doi: 10.1007/978-3-030-38062-5_14
Fujimoto, K., Tanaka, M., Masud Rana, A. Y. K., Jahan, G. S., Itoh, G., Tsujioka, M., et al. (2019). Dynamin-Like Protein B of Dictyostelium Contributes to Cytokinesis Cooperatively with Other Dynamins. Cells 8, 781. doi: 10.3390/cells8080781
Furtado, J. M., Smith, J. R., Belfort, R., Gattey, D., Winthrop, K. L. (2011). Toxoplasmosis: A Global Threat. J. Glob. Infect. Dis. 3, 281–284. doi: 10.4103/0974-777X.83536
Gandre-Babbe, S., van der Bliek, A. M. (2008). The Novel Tail-anchored Membrane Protein Mff Controls Mitochondrial and Peroxisomal Fission in Mammalian Cells. Mol. Biol. Cell. 19, 2402–2412. doi: 10.1091/mbc.E07-12-1287
Ganter, M., Rizopoulos, Z., Schüler, H., Matuschewski, K. (2015). Pivotal and distinct role for Plasmodium actin capping protein alpha during blood infection of the malaria parasite. Mol. Microbiol. 96, 84–94. doi: 10.1111/mmi.12922
Garbary, D. J., McDonald, A. R. (1996). Actin rings in cytokinesis of apical cells in red algae. Can. J. Bot. 74, 971–974. doi: 10.1139/b96-121
Geimer, S., Melkonian, M. (2005). Centrin Scaffold in Chlamydomonas reinhardtii Revealed by Immunoelectron Microscopy. Eukaryot. Cell. 4, 1253–1263. doi: 10.1128/EC.4.7.1253-1263.2005
Goldman, M., Carver, R. K., Sulzer, A. J. (1958). Reproduction of Toxoplasma gondii by Internal Budding. J. Parasitol. 44, 161–171. doi: 10.2307/3274692
Gordon, J. L., Sibley, L. D. (2005). Comparative genome analysis reveals a conserved family of actin-like proteins in apicomplexan parasites. BMC Genomics 6:179. doi: 10.1186/1471-2164-6-179
Gräf, R., Batsios, P., Meyer, I. (2015). Evolution of centrosomes and the nuclear lamina: Amoebozoan assets. Eur. J. Cell Biol. 94, 249–256. doi: 10.1016/j.ejcb.2015.04.004
Gubbels, M.-J., Vaishnava, S., Boot, N., Dubremetz, J.-F., Striepen, B. (2006). A MORN-repeat protein is a dynamic component of the Toxoplasma gondii cell division apparatus. J. Cell. Sci. 119, 2236–2245. doi: 10.1242/jcs.02949
Guggisberg, A. M., Gandhi, A. Y., Erlinger, S. J., Merino, E. F., Cassera, M. B., John, A. R. O. (2017). Suppressor Screen Connects HAD Protein Function to Metabolic Control in Plasmodium falciparum. bioRxiv, 155523. doi: 10.1101/155523
Hachet, O., Simanis, V. (2008). Mid1p/anillin and the septation initiation network orchestrate contractile ring assembly for cytokinesis. Genes Dev. 22, 3205–3216. doi: 10.1101/gad.1697208
Hammarton, T. C. (2019). Who Needs a Contractile Actomyosin Ring? The Plethora of Alternative Ways to Divide a Protozoan Parasite. Front. Cell. Infect. Microbiol. 9, 397. doi: 10.3389/fcimb.2019.00397
Harding, C. R., Meissner, M. (2014). The Inner Membrane Complex Through Development of Toxoplasma gondii and Plasmodium. Cell. Microbiol. 16, 632–641. doi: 10.1111/cmi.12285
Heaslip, A. T., Dzierszinski, F., Stein, B., Hu, K. (2010). TgMORN1 Is a Key Organizer for the Basal Complex of Toxoplasma gondii. PloS Pathog. 6, e1000754. doi: 10.1371/journal.ppat.1000754
Hercyk, B. S., Onwubiko, U. N., Das, M. E. (2019). Coordinating septum formation and the actomyosin ring during cytokinesis in Schizosaccharomyces pombe. Mol. Microbiol. 112, 1645–1657. doi: 10.1111/mmi.14387
Heredero-Bermejo, I., Varberg, J. M., Charvat, R., Jacobs, K., Garbuz, T., Sullivan, W. J., Jr, et al. (2019). TgDrpC, an atypical dynamin-related protein in Toxoplasma gondii, is associated with vesicular transport factors and parasite division. Mol. Microbiol. 111, 46–64. doi: 10.1111/mmi.14138
Hu, K. (2008). Organizational Changes of the Daughter Basal Complex during the Parasite Replication of Toxoplasma gondii. PloS Pathog. 4, e10. doi: 10.1371/journal.ppat.0040010
Imoto, Y., Nishida, K., Yagisawa, F., Yoshida, Y., Ohnuma, M., Yoshida, M., et al. (2011). Involvement of Elongation Factor-1α in Cytokinesis without Actomyosin Contractile Ring in the Primitive Red Alga Cyanidioschyzon merolae. Cytologia 76, 431–437. doi: 10.1508/cytologia.76.431
Itoh, G., Yumura, S. (2007). A novel mitosis-specific dynamic actin structure in Dictyostelium cells. J. Cell Sci. 120, 4302–4309. doi: 10.1242/jcs.015875
Jones, M. L., Kitson, E. L., Rayner, J. C. (2006). Plasmodium falciparum erythrocyte invasion: a conserved myosin associated complex. Mol. Biochem. Parasitol. 147, 74–84. doi: 10.1016/j.molbiopara.2006.01.009
Kamasaki, T., Osumi, M., Mabuchi, I. (2007). Three-dimensional arrangement of F-actin in the contractile ring of fission yeast. J. Cell Biol. 178, 765–771. doi: 10.1083/jcb.200612018
Kamijo, K., Ohara, N., Abe, M., Uchimura, T., Hosoya, H., Lee, J.-S., et al. (2005). Dissecting the Role of Rho-mediated Signaling in Contractile Ring Formation. MBoC 17, 43–55. doi: 10.1091/mbc.e05-06-0569
Katris, N. J., Dooren, G. G., van, McMillan, P. J., Hanssen, E., Tilley, L., Waller, R. F. (2014). The Apical Complex Provides a Regulated Gateway for Secretion of Invasion Factors in Toxoplasma. PLOS Pathogens 10, e1004074. doi: 10.1371/journal.ppat.1004074
Khaliullin, R. N., Green, R. A., Shi, L. Z., Gomez-Cavazos, J. S., Berns, M. W., Desai, A., et al. (2018). A positive-feedback-based mechanism for constriction rate acceleration during cytokinesis in Caenorhabditis elegans. eLife 7, e36073. doi: 10.7554/eLife.36073
Kitayama, C., Sugimoto, A., Yamamoto, M. (1997). Type II Myosin Heavy Chain Encoded by the myo2 Gene Composes the Contractile Ring during Cytokinesis in Schizosaccharomyces pombe. J. Cell Biol. 137, 1309–1319. doi: 10.1083/jcb.137.6.1309
Kono, M., Herrmann, S., Loughran, N. B., Cabrera, A., Engelberg, K., Lehmann, C., et al. (2012). Evolution and Architecture of the Inner Membrane Complex in Asexual and Sexual Stages of the Malaria Parasite. Mol. Biol. Evol. 29, 2113–2132. doi: 10.1093/molbev/mss081
Kuroiwa, T. (1998). The primitive red algae Cyanidium caldarium and Cyanidioschyzon merolae as model system for investigating the dividing apparatus of mitochondria and plastids. BioEssays 20, 344–354. doi: 10.1002/(SICI)1521-1878(199804)20:4<344::AID-BIES11>3.0.CO;2-2
Laplante, C., Berro, J., Karatekin, E., Hernandez-Leyva, A., Lee, R., Pollard, T. D. (2015). Three myosins contribute uniquely to the assembly and constriction of the fission yeast cytokinetic contractile ring. Curr. Biol. 25, 1955–1965. doi: 10.1016/j.cub.2015.06.018
Laporte, D., Zhao, R., Wu, J.-Q. (2010). Mechanisms of Contractile-Ring Assembly in Fission Yeast and Beyond. Semin. Cell Dev. Biol. 21, 892–898. doi: 10.1016/j.semcdb.2010.08.004
Lenz, M. (2020). Reversal of contractility as a signature of self-organization in cytoskeletal bundles. eLife 9 , e51751. doi: 10.7554/eLife.51751
Leung, J. M., Liu, J., Wetzel, L. A., Hu, K. (2019). Centrin2 from the human parasite Toxoplasma gondii is required for its invasion and intracellular replication. J. Cell Sci. 132, jcs228791. doi: 10.1242/jcs.228791
Li, H., Han, Z., Lu, Y., Lin, Y., Zhang, L., Wu, Y., et al. (2004). Isolation and functional characterization of a dynamin-like gene from Plasmodium falciparum. Biochem. Biophys. Res. Commun. 320, 664–671. doi: 10.1016/j.bbrc.2004.06.010
Lim, L., McFadden, G. I. (2010). The Evolution, Metabolism and Functions of the Apicoplast. Philos. Trans. R. Soc. Lond. B Biol. Sci. 365, 749–763. doi: 10.1098/rstb.2009.0273
Lippincott, J., Li, R. (1998). Sequential assembly of myosin II, an IQGAP-like protein, and filamentous actin to a ring structure involved in budding yeast cytokinesis. J. Cell Biol. 140, 355–366. doi: 10.1083/jcb.140.2.355
Lord, M., Laves, E., Pollard, T. D. (2005). Cytokinesis Depends on the Motor Domains of Myosin-II in Fission Yeast but Not in Budding Yeast. MBoC 16, 5346–5355. doi: 10.1091/mbc.e05-07-0601
Lorestani, A., Sheiner, L., Yang, K., Robertson, S. D., Sahoo, N., Brooks, C. F., et al. (2010). A Toxoplasma MORN1 Null Mutant Undergoes Repeated Divisions but Is Defective in Basal Assembly, Apicoplast Division and Cytokinesis. PloS One 5, e12302. doi: 10.1371/journal.pone.0012302
Mabuchi, I., Kashiwazaki, J., Mishra, M. (2017). “Chapter 23 - In vitro reactivation of the cytokinetic contractile ring of fission yeast cells,” in Methods in Cell Biology Cytokinesis. Ed. Echard, A. (Academic Press), 387–394. doi: 10.1016/bs.mcb.2016.03.037
Mahajan, B., Selvapandiyan, A., Gerald, N. J., Majam, V., Zheng, H., Wickramarachchi, T., et al. (2008). Centrins, Cell Cycle Regulation Proteins in Human Malaria Parasite Plasmodium falciparum. J. Biol. Chem. 283, 31871–31883. doi: 10.1074/jbc.M800028200
Makkonen, M., Bertling, E., Chebotareva, N. A., Baum, J., Lappalainen, P. (2013). Mammalian and Malaria Parasite Cyclase-associated Proteins Catalyze Nucleotide Exchange on G-actin through a Conserved Mechanism. J. Biol. Chem. 288, 984–994. doi: 10.1074/jbc.M112.435719
Mangione, M. C., Gould, K. L. (2019). Molecular form and function of the cytokinetic ring. J. Cell. Sci. 132, jcs226928. doi: 10.1242/jcs.226928
Mann, T., Beckers, C. (2001). Characterization of the Subpellicular Network, a Filamentous Membrane Skeletal Component in the Parasite Toxoplasma gondii. Mol. Biochem. Parasitol. 115, 257–268. doi: 10.1016/s0166-6851(01)00289-4
Martin, A. C. (2016). Embryonic ring closure: Actomyosin rings do the two-step. J. Cell Biol. 215, 301–303. doi: 10.1083/jcb.201610061
Maupin, P., Pollard, T. D. (1986). Arrangement of actin filaments and myosin-like filaments in the contractile ring and of actin-like filaments in the mitotic spindle of dividing HeLa cells. J. Ultrastruct. Mol. Struct. Res. 94, 92–103. doi: 10.1016/0889-1605(86)90055-8
Mazumdar, A., Mazumdar, M. (2002). How one becomes many: Blastoderm cellularization in Drosophila melanogaster. BioEssays 24, 1012–1022. doi: 10.1002/bies.10184
Mears, J. A., Lackner, L. L., Fang, S., Ingerman, E., Nunnari, J., Hinshaw, J. E. (2011). Conformational changes in Dnm1 support a contractile mechanism for mitochondrial fission. Nat. Struct. Mol. Biol. 18, 20–26. doi: 10.1038/nsmb.1949
Meitinger, F., Palani, S. (2016). Actomyosin ring driven cytokinesis in budding yeast. Semin. Cell Dev. Biol. 53, 19–27. doi: 10.1016/j.semcdb.2016.01.043
Melatti, C., Pieperhoff, M., Lemgruber, L., Pohl, E., Sheiner, L., Meissner, M. (2019). A unique dynamin-related protein is essential for mitochondrial fission in Toxoplasma gondii. PloS Pathog. 15, e1007512. doi: 10.1371/journal.ppat.1007512
Mendes Pinto, I., Rubinstein, B., Kucharavy, A., Unruh, J. R., Li, R. (2012). Actin Depolymerization Drives Actomyosin Ring Contraction during Budding Yeast Cytokinesis. Dev. Cell. 22, 1247–1260. doi: 10.1016/j.devcel.2012.04.015
Miller, A. L. (2011). The contractile ring. Curr. Biol. 21, R976–R978. doi: 10.1016/j.cub.2011.10.044
Mishima, M., Kaitna, S., Glotzer, M. (2002). Central Spindle Assembly and Cytokinesis Require a Kinesin-like Protein/RhoGAP Complex with Microtubule Bundling Activity. Dev. Cell. 2, 41–54. doi: 10.1016/S1534-5807(01)00110-1
Mishra, M., Kashiwazaki, J., Takagi, T., Srinivasan, R., Huang, Y., Balasubramanian, M. K., et al. (2013). In vitro contraction of cytokinetic ring depends on myosin II but not on actin dynamics. Nat. Cell Biol. 15, 853–859. doi: 10.1038/ncb2781
Miyagishima, S., Kuwayama, H., Urushihara, H., Nakanishi, H. (2008). Evolutionary linkage between eukaryotic cytokinesis and chloroplast division by dynamin proteins. Proc. Natl. Acad. Sci. U. S. A. 105, 15202–15207. doi: 10.1073/pnas.0802412105
Morlon-Guyot, J., Francia, M. E., Dubremetz, J.-F., Daher, W. (2017). Towards a Molecular Architecture of the Centrosome in Toxoplasma gondii. Cytoskeleton 74, 55–71. doi: 10.1002/cm.21353
Mogilner, A., Oster, G. (2003). Force Generation by Actin Polymerization II: The Elastic Ratchet and Tethered Filaments. Biophys. J. 84, 1591–1605. doi: 10.1016/S0006-3495(03)74969-8
Naumov, A., Kratzer, S., Ting, L.-M., Kim, K., Suvorova, E. S., White, M. W. (2017). The Toxoplasma Centrocone Houses Cell Cycle Regulatory Factors. mBio 8. doi: 10.1128/mBio.00579-17
Oelz, D. B., Rubinstein, B. Y., Mogilner, A. (2015). A Combination of Actin Treadmilling and Cross-Linking Drives Contraction of Random Actomyosin Arrays. Biophys. J. 109, 1818–1829. doi: 10.1016/j.bpj.2015.09.013
Oh, Y., Bi, E. (2011). Septin structure and function in yeast and beyond. Trends Cell Biol. 21, 141–148. doi: 10.1016/j.tcb.2010.11.006
Osteryoung, K. W., Pyke, K. A. (2014). Division and dynamic morphology of plastids. Annu. Rev. Plant Biol. 65, 443–472. doi: 10.1146/annurev-arplant-050213-035748
Pagliuso, A., Tham, T. N., Stevens, J. K., Lagache, T., Persson, R., Salles, A., et al. (2016). A role for septin 2 in Drp1-mediated mitochondrial fission. EMBO Rep. 17, 858–873. doi: 10.15252/embr.201541612
Pagliuso, A., Cossart, P., Stavru, F. (2018). The Ever-Growing Complexity of the Mitochondrial Fission Machinery. Cell. Mol. Life Sci. 75, 355–374. doi: 10.1007/s00018-017-2603-0
Patasi, C., Godočíková, J., Michlíková, S., Nie, Y., Káčeriková, R., Kválová, K., et al. (2015). The Role of Bni5 in the Regulation of Septin Higher-Order Structure Formation. Biol. Chem. 396, 1325–1337. doi: 10.1515/hsz-2015-0165
Periz, J., Whitelaw, J., Harding, C., Gras, S., Del Rosario Minina, M. I., Latorre-Barragan, F., et al. (2017). Toxoplasma gondii F-actin forms an extensive filamentous network required for material exchange and parasite maturation. eLife 6, e24119. doi: 10.7554/eLife.24119
Perrin, A. J., Collins, C. R., Russell, M. R. G., Collinson, L. M., Baker, D. A., Blackman, M. J. (2018). The Actinomyosin Motor Drives Malaria Parasite Red Blood Cell Invasion but Not Egress. mBio 9. doi: 10.1128/mBio.00905-18
Piekny, A. J., Glotzer, M. (2008). Anillin Is a Scaffold Protein That Links RhoA, Actin, and Myosin during Cytokinesis. Curr. Biol. 18, 30–36. doi: 10.1016/j.cub.2007.11.068
Piekny, A., Werner, M., Glotzer, M. (2005). Cytokinesis: welcome to the Rho zone. Trends Cell Biol. 15, 651–658. doi: 10.1016/j.tcb.2005.10.006
Pinto, I. M., Rubinstein, B., Li, R. (2013). Force to Divide: Structural and Mechanical Requirements for Actomyosin Ring Contraction. Biophys. J. 105, 547–554. doi: 10.1016/j.bpj.2013.06.033
Pollard, T. D., O’Shaughnessy, B. (2019). Molecular Mechanism of Cytokinesis. Annu. Rev. Biochem. 88, 661–689. doi: 10.1146/annurev-biochem-062917-012530
Pollard, T. D. (2017). Nine unanswered questions about cytokinesis. J. Cell Biol. 216, 3007–3016. doi: 10.1083/jcb.201612068
Porchet, E., Torpier, G. (1977). [Freeze Fracture Study of Toxoplasma and Sarcocystis Infective Stages (author’s transl)]. Z Parasitenkd 54, 101–124. doi: 10.1007/BF00380795
Radke, J. R., Striepen, B., Guerini, M. N., Jerome, M. E., Roos, D. S., White, M. W. (2001). Defining the Cell Cycle for the Tachyzoite Stage of Toxoplasma gondii. Mol. Biochem. Parasitol. 115, 165–175. doi: 10.1016/s0166-6851(01)00284-5
Rai, A., Nöthe, H., Tzvetkov, N., Korenbaum, E., Manstein, D. J. (2011). Dictyostelium dynamin B modulates cytoskeletal structures and membranous organelles. Cell Mol. Life Sci. 68, 2751–2767. doi: 10.1007/s00018-010-0590-5
Rana, A. Y. K. M. M., Tsujioka, M., Miyagishima, S., Ueda, M., Yumura, S. (2013). Dynamin contributes to cytokinesis by stabilizing actin filaments in the contractile ring. Genes to Cells 18, 621–635. doi: 10.1111/gtc.12060
Robert-Paganin, J., Robblee, J. P., Auguin, D., Blake, T. C. A., Bookwalter, C. S., Krementsova, E. B., et al. (2019). Plasmodium myosin A drives parasite invasion by an atypical force generating mechanism. Nat. Commun. 10, 3286. doi: 10.1038/s41467-019-11120-0
Rudlaff, R. M., Kraemer, S., Streva, V. A., Dvorin, J. D. (2019). An essential contractile ring protein controls cell division in Plasmodium falciparum. Nat. Commun. 10, 2181. doi: 10.1038/s41467-019-10214-z
Rudlaff, R. M., Kraemer, S., Marshman, J., Dvorin, J. D. (2020). Three-Dimensional Ultrastructure of Plasmodium falciparum Throughout Cytokinesis. PLOS Pathogens 16, e1008587. doi: 10.1371/journal.ppat.1008587
Santos, B., Gutiérrez, J., Calonge, T. M., Pérez, P. (2003). Novel Rho GTPase Involved in Cytokinesis and Cell Wall Integrity in the Fission Yeast Schizosaccharomyces pombe. Eukaryot. Cell. 2, 521–533. doi: 10.1128/EC.2.3.521-533.2003
Schwayer, C., Sikora, M., Slováková, J., Kardos, R., Heisenberg, C.-P. (2016). Actin Rings of Power. Dev. Cell. 37, 493–506. doi: 10.1016/j.devcel.2016.05.024
Sheffield, H. G., Melton, M. L. (1968). The Fine Structure and Reproduction of Toxoplasma gondii. J. Parasitol. 54, 209–226. doi: 10.2307/3276925
Stortz, J. F., Del Rosario, M., Singer, M., Wilkes, J. M., Meissner, M., Das, S. (2019). Formin-2 drives polymerisation of actin filaments enabling segregation of apicoplasts and cytokinesis in Plasmodium falciparum. eLife 8, e49030. doi: 10.7554/eLife.49030
Striepen, B., Jordan, C. N., Reiff, S., Dooren, G. G. (2007). Building the Perfect Parasite: Cell Division in Apicomplexa. PloS Pathog. 3, e78. doi: 10.1371/journal.ppat.0030078
Sumiya, N., Fujiwara, T., Kobayashi, Y., Misumi, O., Miyagishima, S. (2014). Development of a heat-shock inducible gene expression system in the red alga Cyanidioschyzon merolae. PloS One 9, e111261. doi: 10.1371/journal.pone.0111261
Talukder, S. U., Pervin, S., Tanvir, I. O., Fujimoto, K., Tanaka, M., Itoh, G., et al. (2020). Ca2+–Calmodulin Dependent Wound Repair in Dictyostelium Cell Membrane. Cells 9, 1058. doi: 10.3390/cells9041058
TerBush, A. D., Yoshida, Y., Osteryoung, K. W. (2013). FtsZ in chloroplast division: structure, function and evolution. Curr. Opin. Cell Biol. 25, 461–470. doi: 10.1016/j.ceb.2013.04.006
Trager, W., Rudzinska, M. A., Bradbury, P. C. (1966). The fine structure of Plasmodium falciparum and its host erythrocytes in natural malarial infections in man. Bull. World Health Organ. 35, 883–885.
van den Zypen, E., Piekarski, G. (1968). [Ultrastructure on endodyogeny in Toxoplasma gondii]. Bol. Chil. Parasitol. 23, 90–94.
van Dooren, G. G., Reiff, S. B., Tomova, C., Meissner, M., Humbel, B. M., Striepen, B. (2009). An novel dynamin-related protein has been recruited for apicoplast fission in Toxoplasma gondii. Curr. Biol. 19, 267–276. doi: 10.1016/j.cub.2008.12.048
Wall, R. J., Zeeshan, M., Katris, N. J., Limenitakis, R., Rea, E., Stock, J., et al. (2019). Systematic analysis of Plasmodium myosins reveals differential expression, localisation, and function in invasive and proliferative parasite stages. Cell. Microbiol. 21, e13082. doi: 10.1111/cmi.13082
Willet, A. H., McDonald, N. A., Gould, K. L. (2015). Regulation of contractile ring formation and septation in Schizosaccharomyces pombe. Curr. Opin. Microbiol. 28, 46–52. doi: 10.1016/j.mib.2015.08.001
Wong, W., Skau, C. T., Marapana, D. S., Hanssen, E., Taylor, N. L., Riglar, D. T., et al. (2011). Minimal requirements for actin filament disassembly revealed by structural analysis of malaria parasite actin-depolymerizing factor 1. Proc. Natl. Acad. Sci. U. S. A. 108, 9869–9874. doi: 10.1073/pnas.1018927108
Wu, J.-Q., Kuhn, J. R., Kovar, D. R., Pollard, T. D. (2003). Spatial and Temporal Pathway for Assembly and Constriction of the Contractile Ring in Fission Yeast Cytokinesis. Dev. Cell. 5, 723–734. doi: 10.1016/S1534-5807(03)00324-1
Wu, J.-Q., Ye, Y., Wang, N., Pollard, T. D., Pringle, J. R. (2010). Cooperation Between the Septins and the Actomyosin Ring and Role of a Cell-Integrity Pathway During Cell Division in Fission Yeast. Genetics 186, 897–915. doi: 10.1534/genetics.110.119842
Xue, Z., Sokac, A. M. (2016). Back-to-back mechanisms drive actomyosin ring closure during Drosophila embryo cleavage. J. Cell Biol. 215, 335–344. doi: 10.1083/jcb.201608025
Yoshida, Y., Mogi, Y. (2019). How do plastids and mitochondria divide? Microscopy (Oxf) 68, 45–56. doi: 10.1093/jmicro/dfy132
Yoshida, Y., Mogi, Y., TerBush, A. D., Osteryoung, K. W. (2016). Chloroplast FtsZ assembles into a contractible ring via tubulin-like heteropolymerization. Nat. Plants 2, 1–10. doi: 10.1038/nplants.2016.95
Yoshida, Y. (2018). Insights into the Mechanisms of Chloroplast Division. IJMS 19, 733. doi: 10.3390/ijms19030733
Young, B. A., Buser, C., Drubin, D. G. (2010). Isolation and Partial Purification of the Saccharomyces cerevisiae Cytokinetic Apparatus. Cytoskeleton (Hoboken) 67, 13–22. doi: 10.1002/cm.20412
Yumura, S. (2001). Myosin II dynamics and cortical flow during contractile ring formation in Dictyostelium cells. J. Cell Biol. 154, 137–146. doi: 10.1083/jcb.200011013
Keywords: cytokinesis, contractile ring, basal complex, Apicomplexans, actomyosin, division, schizogony, endodyogeny
Citation: Morano AA and Dvorin JD (2021) The Ringleaders: Understanding the Apicomplexan Basal Complex Through Comparison to Established Contractile Ring Systems. Front. Cell. Infect. Microbiol. 11:656976. doi: 10.3389/fcimb.2021.656976
Received: 21 January 2021; Accepted: 19 March 2021;
Published: 19 April 2021.
Edited by:
Catherine J. Merrick, University of Cambridge, United KingdomReviewed by:
Maria E. Francia, Institut Pasteur de Montevideo, UruguayAoife Heaslip, University of Connecticut, United States
Copyright © 2021 Morano and Dvorin. This is an open-access article distributed under the terms of the Creative Commons Attribution License (CC BY). The use, distribution or reproduction in other forums is permitted, provided the original author(s) and the copyright owner(s) are credited and that the original publication in this journal is cited, in accordance with accepted academic practice. No use, distribution or reproduction is permitted which does not comply with these terms.
*Correspondence: Jeffrey D. Dvorin, amVmZnJleS5kdm9yaW5AY2hpbGRyZW5zLmhhcnZhcmQuZWR1