- Tuberculosis Prevention and Control Key Laboratory/Beijing Key Laboratory of New Techniques of Tuberculosis Diagnosis and Treatment, Senior Department of Tuberculosis, The 8th Medical Center of PLA General Hospital, Beijing, China
Tuberculosis (TB) is a serious public health problem worldwide. The combination of various anti-TB drugs is mainly used to treat TB in clinical practice. Despite the availability of effective antibiotics, effective treatment regimens still require long-term use of multiple drugs, leading to toxicity, low patient compliance, and the development of drug resistance. It has been confirmed that immune recognition, immune response, and immune regulation of Mycobacterium tuberculosis (Mtb) determine the occurrence, development, and outcome of diseases after Mtb infection. The research and development of TB-specific immunotherapy agents can effectively regulate the anti-TB immune response and provide a new approach toward the combined treatment of TB, thereby preventing and intervening in populations at high risk of TB infection. These immunotherapy agents will promote satisfactory progress in anti-TB treatment, achieving the goal of “ultra-short course chemotherapy.” This review highlights the research progress in immunotherapy of TB, including immunoreactive substances, tuberculosis therapeutic vaccines, chemical agents, and cellular therapy.
1 Introduction
Tuberculosis (TB) is a leading infectious disease caused by Mycobacterium tuberculosis (Mtb), which invades the host. The World Health Organization (WHO) estimated 9.9 million new cases and 1.28 million deaths in 2020 (WHO, 2021). Despite the availability of antibiotics and effective treatment regimens, there are still many inevitable problems during chemotherapy, such as the long course of treatment, severe adverse reactions, poor compliance, and multidrug resistance. Therefore, anti-TB treatments are facing significant challenges worldwide.
It is well known that immune recognition, immune response, and immune regulation to Mtb determine the occurrence, development, and outcome of the disease. The immune response of the body to TB results from the interactions of diverse innate and adaptive immune cells and is determined by genetic and environmental factors of the bacteria and host. At the initial stage of infection, robust innate immunity plays a pivotal role in the early clearance of Mtb. Macrophages, natural killer cells, dendritic cells, γδ T cells, neutrophils, and other phagocytes together build the first-line of defense, in which macrophages are the most essential cells in resisting Mtb (Sia et al., 2015). Mtb mainly survives and proliferates in alveolar macrophages and other innate immune cells of the host. The interaction between Mtb and immunity is a dynamic game process, leading to different outcomes: (1) When the invasiveness of Mtb is weaker than the host immunity, the alveolar macrophages directly kill and eliminate Mtb (Korbel et al., 2008; Cadena et al., 2016). After that, macrophages, NK cells and other innate immune cell populations can produce “trained immunity,” and the immune system can mount a faster and more effective protective immune response after the second invasiveness of Mtb (Divangahi et al., 2021); (2) If the invasiveness of Mtb is balanced with host immunity, Mtb may turn into a dormant state, presenting immune escape and a symbiotic state with the host (Gong and Wu, 2021); (3) When the invasiveness of Mtb is stronger than the host immunity, Mtb will replicate in the granuloma, which may undergo caseous necrosis, liquefaction, and cavitation, leading to a spread of Mtb and an initiation of active TB (Khan et al., 2016; de Martino et al., 2019). Thus, adaptive immune responses play an essential role in anti-TB immune defense. Since the protective responses of the host against TB are based on the production of innate immune cells and the interaction between activated macrophages and specific T cells, enhancing protective immunity or regulating adaptive immune responses against TB may be valuable adjuvant treatments for advanced disease. Therefore, reasonable anti-TB chemotherapy combined with immune modulators will help adjust the immune status of the patient and enhance the therapeutic effect of chemical drugs on TB. In general, anti-TB immunotherapy mainly includes activating immune activity, enhancing protective immunity, and suppressing adverse immune responses and inflammatory damage. The research and development of TB-specific immunotherapy agents can effectively regulate the anti-TB immune response, provide a new way for the combined treatment of TB, prevent and intervene in populations at high risk of TB infection, which will make TB treatment achieve a significant effect, and achieve the goal of “ultra-short course chemotherapy.”
This review highlights the research progress in the immunotherapy of TB including immunoactive substances, tuberculosis therapeutic vaccines, chemical agents, and cellular therapy (Tables 1, 2). We believe that immunotherapy has a high potential to prevent the activation of latent Mtb and treat patients with active TB.
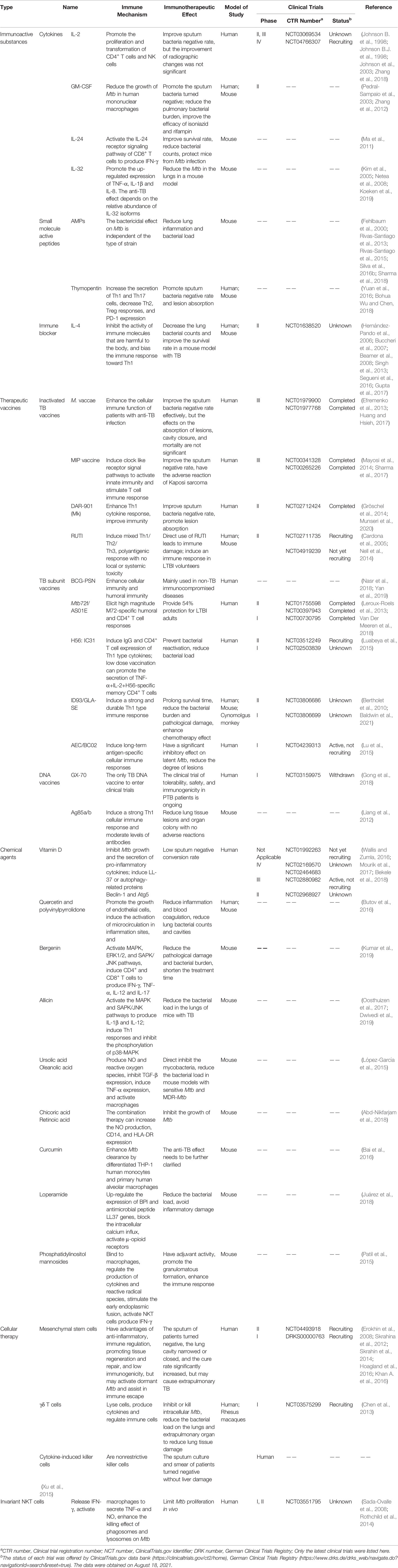
Table 1 Summary of the development of immunoactive substances, vaccines, chemical agents, and cellular therapies.
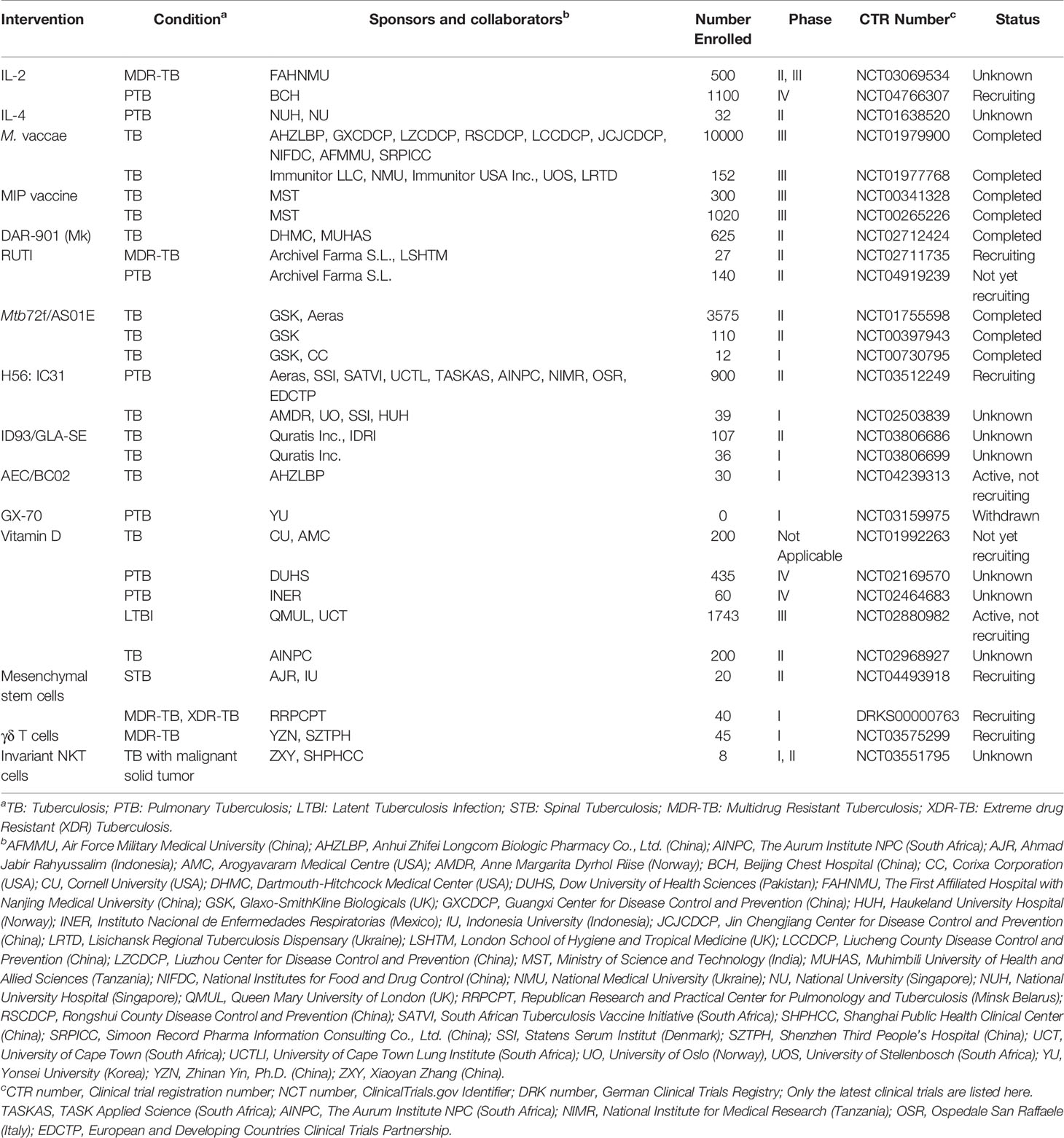
Table 2 The development of clinical trials in immunoactive substances, vaccines, chemical agents, and cellular therapies.
2 Immunoactive Substances
Immunoactive substances, produced by immune cells or other cells to exert an immune effect, mainly include cytokines (such as interferon, interleukin, and tumor necrosis factor), antibodies, lysozyme, and complement. In recent years, a growing number of studies have focused on the application of these immunoactive substances in the immunotherapy of TB.
2.1 Cytokines
Cytokines are proteins that coordinate innate and adaptive immune responses by affecting cell development, transport, and function. Currently, the cytokines used in clinical applications or clinical trials are recombinant human interferon (rhuIFN-γ), recombinant human interleukin-2 (rhuIL-2), α-tumor necrosis factor (TNF-α), and recombinant human granulocyte-macrophage colony-stimulating factor (rhuGM-CSF); however, cytokine immunotherapy has the shortcomings of short half-life and high cost.
2.1.1 Interleukin-2
IL-2, a cytokine belonging to the Th1-type immune response, functions in immune activation and regulation. Studies have shown that IL-2 can induce differential expression of genes in peripheral blood mononuclear cells (PBMCs) stimulated with Mtb, and reduce or clear sputum bacteria in about 60% of patients with MDR-TB combined with chemotherapy (Johnson B. et al., 1998; Johnson B.J. et al., 1998). However, data from a double-blind, placebo-controlled clinical trial showed that daily intradermal injection of rhuIL-2 could not enhance bacillary clearance or improve symptoms in patients with drug-susceptible TB (Johnson et al., 2003). Therefore, the clinical results of rhuIL-2 combined with chemotherapy for refractory pulmonary TB (PTB) or MDR-TB are inconsistent. In addition, a meta-analysis showed that rhuIL-2 immunoadjuvant therapy was safe for patients with PTB/MDR-TB, and could promote the proliferation and transformation of CD4+ T cells and NK cells, improving the sputum bacterium-negative rate of patients with TB, however, there was no significant improvement in radiographic changes in patients with TB (Zhang et al., 2018). A multicenter, large-sample prospective clinical study of rhuIL-2 adjuvant therapy for MDR-TB for 24 months is ongoing in China (ClinicalTrials.gov Identifier: NCT03069534).
2.1.2 Granulocyte-Macrophage Colony-Stimulating Factor
GM-CSF, a cytokine with immune activation and regulatory effects, is a monomer glycoprotein secreted by macrophages, T cells, mast cells, natural killer cells, endothelial cells, and fibroblasts. GM-CSF has been shown to reduce the growth of Mtb in human mononuclear macrophages (Denis et al., 1990). The results of a phase II clinical trial of rhuGM-CSF combined with anti-TB chemotherapy in the treatment of active PTB (APTB) showed that rhuGM-CSF adjuvant immunotherapy had better safety and tolerance in patients, and the sputum bacteria rapidly turned negative in the eighth week of treatment (Pedral-Sampaio et al., 2003). In addition, immunotherapy with IL-2 and GM-CSF in the MDR-TB mouse model could increase the survival rate of mice and decrease the bacterial loads in the lung, spleen and lung lesions, which will improve the efficacy of first-line anti-TB drugs (isoniazid and rifampin) (Zhang et al., 2012). As a new gene therapy, recombinant GM-CSF adenoviruses (AdGM-CSF) in a mouse model could also significantly reduce pulmonary bacterial burden compared to conventional chemotherapy when administered in a single dose (Francisco-Cruz et al., 2016).
2.1.3 Interleukin-24
IL-24, a novel tumor suppressor, has been designated as a member of the IL-10 cytokine family because of its conserved structure, chromosomal location, and cytokine-like properties. Mtb infection has been demonstrated to inhibit the expression of IL-24 in human PBMCs and decrease the levels of IL-24 in the sera of patients with TB, which may increase the susceptibility of TB and promote the development of chronic TB (Ma et al., 2011). IL-24 can activate the IL-24 receptor signaling pathway of CD8+ T cells to produce large amounts of interferon-γ (IFN-γ) to combat Mtb, which depends on the early involvement of neutrophils (Ma et al., 2011). The application of IL-24 in the treatment of the mouse TB model showed an anti-TB effect, suggesting that IL-24 might be a new potential immunotherapy (Ma et al., 2011).
2.1.4 Interleukin-32
IL-32, a cytokine produced mainly by T cells, NK cells, and epithelial cells, is an important secretory protein involved in innate and adaptive immune responses that induce the production of critical inflammatory factors (such as TNF-α, IL-1β, IL-6, MIP-2, and IL-8) in macrophages to eliminate Mtb (Kim et al., 2005; Netea et al., 2008). Therefore, the amplification effect of IL-32 in innate immunity against TB can upregulate TNF-α and promote cell apoptosis. Recent studies have found that stimulation of human PBMCs with heat-killed Mtb could induce the production of a large amount of IL-32 to enhance the Mtb clearance ability of human monocyte-macrophages (Li et al., 2018). The amount of lung Mtb can be significantly reduced by human IL-32γ expressed by type II alveolar epithelial cells from transgenic mice (Bai et al., 2015). In addition, after the downregulation of endogenous IL-32 expression in human THP-1 macrophages by siRNA interference, intracellular inflammatory factors, such as TNF-α, IL-1β, and significantly, and the intracellular Mtb increased (Bai et al., 2010). In the Mtb-infected PBMCs of the healthy control group, IL-32γ was downregulated while IL-32β was upregulated, suggesting that IL-32 contributes to preventing Mtb infection, and this effect may depend on the relative abundance of IL-32 isoforms (Koeken et al., 2019). Thus, IL-32 is a promising new immunotherapy for establishing protective responses and inhibiting Mtb growth.
2.2 Anti-TB Antibodies
The role of humoral immunity in TB is controversial because anti-TB is generally believed to rely mainly on the cellular immune response. However, more studies have shown that antibodies also have a protective effect on anti-TB immunity in recent years. The application of Mtb antigen-specific antibodies could potentially induce an inflammatory response, phagosome maturation, and intracellular bactericidal activity, and can block the immune damage induced by harmful molecules (Teitelbaum et al., 1998; Hamasur et al., 2004b).
A meta-analysis showed increased susceptibility to TB in some antibody-deficient hosts (Rodríguez et al., 2005). The protective function of antibodies against different Mtb epitopes varies. For example, the lower the level of anti-LAM or AM antibodies in patients with TB, the faster the progress of TB and the higher the frequency of dissemination (Costello et al., 1992); human anti-HBHA IgM antibodies could prevent Mtb from entering the epithelial cells of patients with TB (Shin et al., 2006); anti-Ag85A IgG could reduce the risk of active TB, reduce cavities, and remove sputum bacteria (Liang et al., 2018).
In addition, passive injection of poly/monoclonal antibodies or serum against Mtb antigen could improve phagocytosis, regulate CD8+ T cells, and reduce tissue damage, lung inflammation, and bacterial load in mice. For example, Mtb-infected mice inoculated intratracheally or intranasally with anti-Acr IgA antibody or pretreated with hsIgA reduced lung colony counts and improved granulomatous formation (Williams et al., 2004; Alvarez et al., 2013); Mycobacterium surface protein heparin-binding hemagglutinin adhesive (HBHA) promoted Mtb dissemination in mice, while the passive transfer of anti-HBHA IgG3 McAb 4057D2 and IgG2a McAb 3921E4, or anti-LAM IgG could significantly reduce the Mtb extrapulmonary spread (Pethe et al., 2001; Hamasur et al., 2004a).
Immunoglobulin Y (IgY), a major antibody in the blood of poultry, reptiles, and lungfish, is a potential immunoglobulin for TB immunotherapy with functions similar to IgG (Sudjarwo et al., 2017b). Similar to other immunoglobulins, IgY is a class of recognition proteins formed by the immune system in response to foreign substances. Sudjarwo et al. found that IgY could significantly increase the proliferation of rat PBMCs and secretion of IL-2 and IFN-γ in PBMCs, suggesting that the pharmacological activity of IgY against Mtb may be mediated by regulating the production of cytokines (Sudjarwo et al., 2017a).
2.3 Small Molecule Active Peptides
Small molecule active peptides, consisting of 2 to 15 amino acids with a simple structure and small molecular weight, can directly enter the cells through the skin, blood-brain, placenta, and gastrointestinal barriers to exert their biological activity.
Antimicrobial peptides (AMPs) are small, cationic, and amphiphilic peptides produced by organisms. They have antimicrobial activity as well as chemotactic, autophagic, and immunoregulatory activities (Hancock and Lehrer, 1998; Yamasaki and Gallo, 2008). Previous studies have identified many AMPs that have a potential immunotherapeutic effect on Mtb infection, and their bactericidal effect on Mtb was independent of the type of Mtb strain (e.g., whether they are resistant to drugs or not) (AlMatar et al., 2018). The essential amino acid L-isoleucine and its analogs have been shown to induce β-defensins through the activation of the transcription factor NF-κB, and using L-isoleucine to treat the mouse model with PTB could increase the expression of mβd-3 and -4 genes, as well as reduce the bacterial load and pulmonary inflammation (Fehlbaum et al., 2000); nano-encapsulated synthetic Magainin-I analog peptide could reduce the amount of viable Mtb by up to approximately 3.03-log CFU and enhance host defense mechanism by averting bacteria-induced inhibition of phagosomal-lysosome fusion and apoptosis (Sharma et al., 2018); and recombinant human neutrophil peptide-1 (HNP-1), human beta defensin-2 (HBD-2), HNP-1/HBD-2, LL-37 derived peptide HHC-10 or LLKKK18, and natural defense regulatory factors (IDR HH2 and IDR-1018) were used to treat the murine model of TB, resulting in diminished bacillary load and pulmonary inflammation (Rivas-Santiago et al., 2013; Rivas-Santiago et al., 2015; Silva et al., 2016b). In addition, several other AMPs have also shown anti-TB activity, including amphiphilic α-helical peptide D-V13 K, D-LAK analogs, bacteriocins (Bcn1–Bcn5), nisin A, lactoferrin, HBD variants, PR-39, and 1-C134mer (Silva et al., 2016a).
Thymopentin is a synthetic pentapeptide with immunological activity. In a BALB/c mouse model treated with thymopentin, Th1 and Th17 cells in the peripheral blood increased significantly, while Th2, Treg responses, and programmed cell death protein-1 (PD-1) expression decreased, with no significant side effects when compared with the control group (Yuan et al., 2016). In addition, patients with TB treated with thymopentin combined with chemotherapy showed a synergistic effect with symptom improvement, promoting sputum-negative conversion and lesion absorption (Wu et al., 2018).
2.4 Immune Blocker
Long-term chronic inflammation caused by active pulmonary TB can lead to Th1 and Th2 immune imbalance, immunosuppression, or T cell depletion. The application of immune blockers to block the action of harmful immune molecules can achieve immunotherapeutic purposes. For example, a mouse TB model treated with an anti-IL-4 antibody could block the secretion of the Th2 cytokine IL-4, shift the immune balance toward a protective Th1 response, and reduce the bacillary load in mouse spleens and lungs (Buccheri et al., 2007). In June 2012, a randomized, double-blind, placebo-controlled phase II clinical trial was performed to assess the safety and efficacy of blocking IL-4 with pascolizumab in patients with PTB receiving standard combination therapy (https://www.clinicaltrials.gov/ct2/show/NCT01638520?term=NCT01638520&cond=tuberculosis&draw=2&rank=1). The trial was scheduled to be completed in July 2017. However, the latest results have not been published (ClinicalTrials.gov identifier: NCT01638520). Recombinant β-glycan (type III TGFβ receptor) or small interfering RNA targeting TGFβ1 was used in the mouse TB model, which blocked TGFβ, enhanced the Th1 type immune response, increased the expression of IFN-γ, IL-2, NO, and iNOS, and decreased lung bacterial counts, and downregulated IL-4 (Hernández-Pando et al., 2006). Blocking the IL-10 receptor in chronic Mtb-infected mice with McAb could relieve the inhibition of IL-10 on macrophage activation and Th1 immune responses, increase T cell recruitment in the lung and IFN-γ production, decrease bacterial load in the lung, and improve mouse survival rate (Beamer et al., 2008). The treatment of a mouse TB model with anti-IL-17A mAb could block massive neutrophil recruitment and tissue damage caused by Th17 overreaction (Segueni et al., 2016). Thus, maintaining the Th1-Th17 balance during TB treatment is crucial for promoting anti-TB immunity and avoiding inflammatory tissue damage. Denileukin diftitox, a recombinant fusion protein of diphtheria toxin active domain and IL-2, induced diphtheria toxin to kill CD25 cells expressing the high-affinity IL-2 receptor through the binding of IL-2 moiety, to reduce the frequency of Treg and myeloid-derived suppressor cells (MDSCs, CD11b+GR1HI) frequency, improve pathological damage, reduce the number of viable bacteria, and inhibit the spread of Mtb in a TB mouse model (Gupta et al., 2017). In addition, blocking PD-1 decreased the number of T cells expressing PD-1 and rescued T cells producing Mtb-specific IFN-γ from apoptosis and increased their survival, which may reverse T cell depletion in patients with TB (Singh et al., 2013).
3 Tuberculosis Therapeutic Vaccines
TB therapeutic vaccines can restore immune balance, inhibit immune damage, improve immunity, and inhibit or kill Mtb by regulating or selectively inducing the potential of the immune system of Mtb-infected people. The clinical application of vaccines is simple, convenient, economical, and has few side effects.
3.1 Inactivated TB Vaccines
Among the inactivated TB vaccines prepared by heat-killed non-tuberculous mycobacteria, M. vaccae (MV), and M. phlei F.U.36 (termed Utilins) have been certified for TB treatment [MV: Certificate No, (1999) S-03; F.U.36: Approval No, S20040068]. Previously, F.U.36 was mostly used for other immunocompromised diseases. In addition, M. indicus pranii(MIP), M. kyogaense sp. Nov. (DAR-901), RUTI, M. smegmatis vaccines, and MV used for latent TB infection (LTBI) have entered clinical research, and no further research on acellular M. smegmatis inactivated vaccines has been carried out after the completion of the phase II clinical trials in China.
MV, made from inactivated M. vaccae, is an immunomodulator used in the adjuvant treatment of active TB. The MV vaccine protected against pulmonary Mtb infection in the lungs in a mouse model (Gong et al., 2020). A meta-analysis showed that MV could effectively improve the sputum bacterium-negative rate. However, the effects on the absorption of lesions, cavity closure, and mortality are inconsistent, which may be due to the disunity of the frequency and interval of the MV application. Therefore, it is necessary to determine the most effective drug delivery scheme and the long-term effects of MV (Huang and Hsieh, 2017). A phase III, randomized, double-blind clinical trial of the treatment of LTBI with MV carried out in 2013 showed a trend of reduced TB incidence with low adverse reactions. However, the data of the completed phase III trial have not been published (ClinicalTrials.gov Identifier: NCT01979900). Another phase II trial showed that Mtb in sputum smears was eliminated significantly in patients with TB after one month of treatment with an MV (V7) pill, but the long-term effects still need to be observed (ClinicalTrials.gov Identifier: NCT01380119) (Efremenko et al., 2013).
The MIP (or Mw) vaccine, which is made of heat-inactivated nonpathogenic M. indicus pranii, can induce Toll-like receptor signaling pathways to activate innate immunity and stimulate T cell immune response (Das et al., 2016). A phase III clinical trial and meta-analysis showed that the MIP vaccine could improve the sputum-negative rate of patients with TB without adverse reactions (ClinicalTrials.gov Identifier: NCT00265226) (Sharma et al., 2017). However, MIP had no significant therapeutic effect but showed serious side effects in a phase III clinical trial of TB pericarditis (two-thirds of the subjects were TB-HIV coinfection). Abscess appeared at the injection site in 15% of patients, and the incidence of Kaposi’s sarcoma in HIV-positive patients was higher (ClinicalTrials.gov Identifier: NCT00810849) (Mayosi et al., 2014).
The DAR-901 vaccine (also known as Mk) is an immunotherapeutic agent made of heat-inactivated M. kyogaense sp. nov. Phase I and II clinical trials have shown that intradermal injection of DAR-901 is prone to form a long-term scar, and 3–12 times injection of DAR-901 combined with chemotherapy could improve sputum bacterium-negative rate, promote lesion absorption, and enhance the Th1 cytokine response (von Reyn et al., 2017; Lu and Miao, 2019). In addition, a phase II clinical trial of DAR-901 tablets showed that it could increase the sputum-negative rate and had high safety when combined with chemotherapy for one month in treating patients with TB and MDR-TB (ClinicalTrials.gov Identifier: NCT01380119) (Gong et al., 2018).
RUTI is a vaccine prepared by breaking and detoxifying Mtb H37Rv cultured under hypoxic conditions, low pH, and poor nutritional conditions, and then embedded in liposomes (Cardona, 2006). It can induce a humoral immune response to multiple antigens and a Th1/Th2/Th3 mixed cellular immune response with no local or systemic toxicity (Cardona, 2006). RUTI alone is ineffective in an animal model of active TB, but may also lead to immune injury. However, infected animals treated with RUTI after chemotherapy may achieve good results (Cardona et al., 2005). The results of phase I and phase II clinical trials of RUTI combined with chemotherapy in the treatment of LTBI showed that the vaccine could effectively induce a cellular immune response in LTBI volunteers. However, the adverse reactions correlated positively with the dose, and the injection site was prone to nodules (Nell et al., 2014). As an adjuvant agent of MDR-TB chemotherapy, the safety and immunogenicity of RUTI have been validated through a phase IIa clinical trial (ClinicalTrials.gov Identifier: NCT02711735).
3.2 TB Subunit Vaccines
TB subunit vaccines, prepared from part of the cellular components of Mtb, can also be used for adjuvant treatment of patients with TB and preventive therapy for LTBI. Bacillus Calmette-Guerin (BCG) polysaccharide nucleic acid injection (BCG-PSN, trade name SIQIKANG) is the only currently licensed immunotherapeutic vaccine against TB (Approval No: S20020019), but it has been mainly used in non-TB immunocompromised diseases in recent years (Nasr et al., 2018; Yan et al., 2019). The other four recombinant protein vaccines (Mtb72f/AS01, H56/IC31, ID93/GLA-SE, and AEC/BC02) have entered phase І or phase II clinical trials.
Mtb72f/AS01E is prepared with a recombinant chimeric protein (M72) of Mtb39 and Mtb32, adjuvanted with AS01E to boost the immune response (Van Der Meeren et al., 2018). Phase I/IIa clinical trials showed that Mtb72f/AS01E was well tolerated clinically and elicited strong M72-specific humoral immune responses and CD4+ T cell responses, but weak CD8+ T cell responses (ClinicalTrials.gov Identifier: NCT00730795 and NCT00397943, respectively) (Leroux-Roels et al., 2013). Subsequently, phase II b clinical trials showed that it could provide 54% protection for HIV-negative LTBI adults to achieve low PTB incidence (ClinicalTrials.gov Identifier: NCT01755598) (Van Der Meeren et al., 2018).
H56:IC31 is a recombinant fusion protein of three antigens (Mtb Ag85B, ESAT-6, and Rv2660c) formulated in adjuvant IC31 (Luabeya et al., 2015). Studies have shown that vaccination with the H56:IC31 vaccine prevented bacterial reactivation and significantly reduced bacterial load in LTBI or active TB mice or NHP models compared with the control group (Aagaard et al., 2011). The phase І/II clinical trials showed it was safe and could induce antigen-specific IgG and CD4+ T cell response expressing Th1-type cytokines. TNF-α+IL-2+H56-specific memory CD4+ T cells can be detected by low-dose vaccination in Mtb-infected individuals (ClinicalTrials.gov Identifier: NCT01967134) (Luabeya et al., 2015). However, the therapeutic effect of H56:IC31 requires further evaluation in clinical trials. ID93/GLA-SE is a recombinant fusion protein of four antigens (Mtb Rv2608, Rv3619, Rv3620, and Rv1813) formulated with glucopyranosyl lipid adjuvant in a stable emulsion (GLA-SE, a TLR-4 activator) (Baldwin et al., 2016). This vaccine combined with chemotherapy induced a strong and durable Th1-type cellular immune response, prolonged survival time, decreased bacterial burden in organs, reduced pathological damage, and enhanced the chemotherapy effect in mouse and monkey models with TB (Bertholet et al., 2010; Baldwin et al., 2021). In addition, a stable, inhalable dry powder version of ID93/GLA-SE has already been developed as an alternative to injectable administration (Gomez et al., 2021). At present, phase I clinical trials of this vaccine is underway, however, participants have not been recruited (ClinicalTrials.gov Identifier: NCT03806686 and NCT03806699, respectively).
AEC/BC02 is also a freeze-dried recombinant fusion protein of Ag85B, ESAT6, and -CFP10 combined with adjuvant BC02 based on BCG-derived cytosine-phosphate-guanine and aluminum salt (Lu et al., 2015). This new vaccine has a significant inhibitory effect on latent Mtb and reduces the lesions in various organs in guinea pigs, and has a preventive and protective effect on the guinea pig LTBI model (Lu et al., 2015). Phase I clinical trials that focused on the tolerance of the human body to this vaccine have been completed, but the results have not yet been published (ClinicalTrials.gov Identifier: NCT03026972). Additionally, a phase Іb clinical trial to evaluate the safety and immunogenicity of AEC/BC02 in healthy adults is underway, however, participants have not been recruited (ClinicalTrials.gov Identifier: NCT04239313).
3.3 DNA Vaccines
TB DNA vaccine constructed by encoding genes of Mtb protective antigens and a eukaryotic expression vector has become a promising strategy for developing an effective vaccine against TB. It does not only efficiently induce humoral immunity and Th1-type cell immune response, but also elicits specific cytotoxic T lymphocyte response (Liang et al., 2016; Liang et al., 2017). GX-70, consisting of four Mtb antigen plasmids (specific antigens not disclosed) and a recombinant Flt3-ligand, is the only TB DNA vaccine that can be used in clinical trials. Phase І clinical trials of GX-70 will be evaluated in PTB patients with at high risk for treatment failure or recurrence (ClinicalTrials.gov Identifier: NCT03159975). However, this study was withdrawn because of unconfirmed research expenses (Gong et al., 2018).
The Ag85a/b chimeric DNA vaccine, which completed the preclinical research and pilot process could induce moderate antibody levels, enhance Th1 cellular immune responses, and reduce lung tissue lesions and organ colony number via electric induction in a mouse TB model (Liang et al., 2012). The safety evaluation did not reveal any adverse reactions and preparation for application for clinical trial approval is underway.
Many new TB therapeutic vaccines are still in the preclinical stage. They were constructed with different Mtb antigens and new vaccine delivery systems, such as LT69 (HspX, ESAT6, Ag85B, and Mtb8.4 recombinant fusion protein) (Niu et al., 2015) and LT70 (Rv2626, ESAT6, Ag85B, and Mtb8.4 recombinant fusion protein) subunit vaccines (Chen et al., 2017), hsp70/CD80 chimeric DNA vaccine (Yan et al., 2013), and peptide-based ACP and MP3RT vaccines (Gong et al., 2021a; Gong et al., 2021b). These vaccines have strong immunogenicity and can significantly reduce the bacterial load in animal experiments (Shi et al., 2004; Niu et al., 2015; Liu et al., 2016). However, the immunotherapeutic effects of these vaccines against TB need to be further validated in clinical trials. Most of the TB therapeutic vaccines were combined with chemotherapy for the immunotherapy of patients with TB, and no severe adverse reactions related to the vaccines were observed. However, attention should be given to the “Koch phenomenon” problem caused by the direct application of vaccines that may induce the release of cytokines such as TNF-α when used in the preventive treatment of LTBI populations. Therefore, the immune intervention strategies of chemotherapy followed by vaccination or a combination of vaccination and chemotherapy should be further studied.
4 Chemical Agents
Despite the availability of antibiotics, effective treatment regimens still require long-term multidrug combinations. The discovery of new-generation antibiotics may still face problems related to drug toxicity and resistance. Current chemotherapy regimens are mainly aimed at sterilization and cannot directly reduce the host pathologic inflammatory response associated with TB. In recent years, the use of chemical agents to enhance the host immune regulatory response to Mtb has become an attractive approach, as it reduces the risk of drug resistance and clinical complications. Therefore, chemical agents that enhance the antibacterial activity and accelerate the decline of inflammation in the host can be considered as adjuvant therapy to exert immunotherapeutic activity to improve the clinical therapeutic effect of TB. At present, several chemical agents have reported immunotherapeutic effects against TB. Vitamin D, quercetin, and polyvinylpyrrolidone have been used in clinical studies. Some chemical drugs used in the clinical treatment of other diseases (such as the asthma drug zileuton, the anticancer drugs imatinib, everolimus, tacrolimus, and ridaforolimus; the diabetes drug metformin) are undergoing preclinical research on anti-TB.
4.1 Vitamin D
Vitamin D and its active metabolite 1α, 25-dihydroxy-vitamin D3 [1α, 25(OH)2D3], play an important role in host immune defense against Mtb. 1α,25(OH)2D3 can inhibit Mtb growth and secretion of pro-inflammatory cytokines in PBMCs and induce LL-37 or autophagy-related proteins Beclin-1 and Atg5 to mediate killing effects (Ramos-Espinosa et al., 2018). In addition, they have chemotactic activity on multinuclear leukocytes, activate immune cells to migrate outward, and enhance the killing effect of macrophages on Mtb (Agerberth et al., 2000; Martineau et al., 2007; Yuk et al., 2009). Studies have shown that vitamin D receptor activation by vitamin D administration could inhibit Mtb-induced bone destruction (Deng et al., 2021). Mourik et al. treated the mouse TB model with immunotherapy consisting of all-trans-retinoic acid, 1,25(OH)2-vitamin D3, and α-galactosylceramide in combination with chemotherapy, which showed lower Mtb loads after 5 weeks of treatment and a significantly shortened treatment course, as well as a reduction in TB recurrence (Mourik et al., 2017). However, Bekele et al. found that the application of vitamin D3 and phenylbutyrate combined with chemotherapy in the treatment of PTB could improve clinical symptoms and reduce complications, but had no effect on bacterial clearance in sputum (Bekele et al., 2018). The meta-analysis showed that the inconsistent clinical research results of vitamin D might be due to large differences in clinical trial design, patient characteristics, dosage, time, and study endpoints. Most research results showed that vitamin D supplementation did not improve the sputum bacterium-negative rate of patients and had good safety and tolerance, but three cases had severe adverse reactions (Wallis and Zumla, 2016). At present, the effect of vitamin D combined with chemotherapy on drug-sensitive TB is unsatisfactory, but the sputum bacterium-negative rate seems to be significantly improved in a minority of patients with MDR-TB (Wallis and Zumla, 2016). Therefore, it is necessary to further clarify its effectiveness and safety by establishing strict clinical trial protocols. Currently, several clinical trials of vitamin D as an adjunct to treat or prevent TB are ongoing, but participants are yet to be recruited (ClinicalTrials.gov Identifier: NCT01992263, NCT02880982, NCT02968927, NCT02464683).
4.2 Quercetin and Polyvinylpyrrolidone
Quercetin and polyvinylpyrrolidone (QP) are capillary stabilizing agents and antioxidants with immunomodulatory activities. It can promote the growth of endothelial cells, induce the activation of microcirculation in inflammation sites, and reduce inflammation and blood coagulation (Butov et al., 2016). QP combined with chemotherapy to treat patients with APTB rapidly improved the clinical symptoms and signs, significantly reduced the sputum bacteria and pulmonary cavity, increased lesion absorption, decreased expression of IL-1β and TNF-α with a substantial increase in IL-4, as well as an increase in nitric oxide (NO) at the inflammatory site with no side effects, suggesting that QP might be a new potential immunotherapy (Butov et al., 2016). Currently, 3D printed medicated skin patches containing QP have been developed, which could provide an appropriate therapeutic drug concentration and satisfactory sustained release profiles in the treatment of PTB (Chaudhari et al., 2021).
4.3 Bergenin
Bergenin is a natural compound extracted from fresh leaves of the genus Bergenia. It activates the MAPK, ERK1/2, and SAPK/JNK pathways in Mtb-infected macrophages, selectively induces the secretion of IFN-γ, TNF-α, IL-12, IL-17 expressed from CD4+ and CD8+ T cells, and promotes NO production for the clearance of Mtb in mouse TB models (Kumar et al., 2019). Bergenin combined with isoniazid in an animal model of MDR-TB could significantly reduce the pathological damage and bacterial load, and shorten the treatment time (Kumar et al., 2019). Therefore, bergenin may be a potential immunomodulator for the treatment of TB.
4.4 Allicin
Allicin is a major biologically active component of freshly crushed garlic, which has antibacterial activity against Mtb and can significantly reduce the bacterial load in the lungs of the mouse TB model (Dwivedi et al., 2019). As a potential immunomodulator against TB, allicin can also enhance the activation of the MAPK and SAPK/JNK pathways of Mtb-infected mouse peritoneal macrophages to produce various effectors, such as IL-1β and IL-12. In addition, allicin treatment could selectively induce Th1 responses to resist the invasion of Mtb and inhibit the phosphorylation of p38-MAPK to reduce the expression of TNF-α and IL-10 (Oosthuizen et al., 2017; Dwivedi et al., 2019). Although allicin can improve cellular immunity, humoral immunity, and nonspecific immunity, its efficacy in the adjuvant treatment of TB requires further study.
4.5 Ursolic Acid and Oleanolic Acid
Ursolic acid (UA) and its isomer oleanolic acid (OA) are representative pentacyclic triterpenoids, which have a direct inhibitory effect on mycobacteria. The immunoregulatory effect against Mtb of UA and OA mainly depends on the production of NO and reactive oxygen species, inhibition of TGF-β expression, induction of TNF-α expression, and activation of macrophages (López-García et al., 2015). It is well established that UA and OA can be recognized by CD36 and TGR5, respectively, to induce the overexpression of these two cell membrane receptors and activate TLR2/1, TLR2/6, or TLR4/6 heterodimer, which leads to the inhibition of nuclear factor κB (NF-κB) activation and secretion of pro-inflammatory cytokines such as TNF-α, IL-6, and IL-1β (López-García et al., 2015). The specific mechanisms of UA and OA in Mtb-infected macrophages should be further studied.
4.6 Chicoric Acid and Retinoic Acid
Chicoric acid (CA) is a phenolic compound that stimulates phagocytosis, enhances immune function, and has anti-inflammatory and anti-oxidative effects (Wu et al., 2018). Retinoic acid (RA), a natural oxidation metabolite of vitamin A, has various stereoisomers, including all-trans RA, 13-cis RA, and 9-cis RA (Gundersen et al., 1997). They exert anti-inflammatory effects through the retinoic acid receptor system. For example, the treatment of infected U937 macrophages with 13-cis RA and CA showed a significant increase in NO production, CD14, and HLA-DR expression and also prevented the intracellular survival of Mtb (Abd-Nikfarjam et al., 2018). Although both 13-cis RA and CA had obvious inhibitory effects on the growth of Mtb, the inhibitory effect of high concentrations of CA was significantly greater than that of RA (Abd-Nikfarjam et al., 2018). Therefore, the anti-TB therapeutic effects of CA and RA requires further research in animal models.
4.7 Curcumin
Curcumin is a polyphenolic substance that enhances Mtb clearance by differentiated THP-1 human monocytes and primary human alveolar macrophages. As an inducer of caspase-3-dependent apoptosis and autophagy, curcumin mediates macrophage anti-Mtb function in part through NF-κB activation (Bai et al., 2016). Although curcumin is safe in most cases, its anti-TB effect needs to be demonstrated in animal models.
4.8 Loperamide
Loperamide, a benpiperidine derivative that targets μ-opioid receptors and calcium channels, has an immunomodulatory effect and can induce autophagy, and enhances the anti-Mtb activity of alveolar macrophages by upregulating the expression of bactericidal/permeability-increasing protein (BPI) and antimicrobial peptide LL37 genes to reduce the bacterial load significantly (Juárez et al., 2018). Moreover, loperamide reduces the production of pro-inflammatory cytokines (IL-6, TNF-α, MCP-1, and IFN-γ) in mouse macrophages by blocking intracellular calcium influx to avoid inflammatory damage. In addition, loperamide can induce the production of TNF-α and prostaglandin E2 in endothelial cells and PBMCs by activating μ-opioid receptors (Juárez et al., 2018). Therefore, loperamide has potential immunomodulatory effects, supporting its use in the treatment of TB.
4.9 Phosphatidylinositol Mannosides
Phosphatidylinositol mannosides (PIMs) are a type of Mtb cell wall-associated lipids. It is the structural basis for the synthesis of lipomannan (LM) and lipoarabinomannan (LAM) (Brennan, 2003). PIMs exert an immunomodulatory effect by binding to macrophages, regulating the production of cytokines and active radicals, and stimulating early endoplasmic fusion by binding to Toll-like receptors, C-type lectins, and DC-SIGN ligands (Torrelles et al., 2006). It can also activate NKT cells together with CD1 molecules to produce IFN-γ, interact with α5β1 on CD4+ T cells to promote granulomatous formation and promote alveolar epithelial cell apoptosis (Rojas et al., 2006). Recent research has shown that tetraacylated phosphatidylinositol hexamannoside (Ac2PIM6), which is synthesized with stearic and tuberculostearic acid as the lipid components, could increase the production of IL-4 and IFN-γ in mouse serum and have comparable adjuvant activity (Patil et al., 2015). Therefore, the immunotherapeutic effect of PIMs on TB requires further verification.
4.10 Other Chemical Agents
Zileuton, a novel selective 5-lipoxygenase antagonist, can inhibit the production of the highly active inflammatory substance leukotriene; it has tracheal protection, tracheal dilation, and anti-inflammatory effects, and is currently being evaluated for its anti-TB effects in animal models (Mayer-Barber et al., 2014). Imatinib, a tyrosine kinase inhibitor used to treat cancers such as leukemia, could inhibit Mtb survival by promoting autophagy and acidification (Napier et al., 2011). Some drugs (everolimus, temsirolimus, and ridaforolimus) that inhibit graft rejection or have anticancer effects can reduce Mtb load by inhibiting the rapamycin (mTOR) pathway to promote cell autophagy (Singh and Subbian, 2018). Metformin, a drug used to treat diabetes, can improve and control Mtb infection in mice and reduce its severity by increasing mROS production in cells and mediating acidification of mycobacterium phagosomes; clinical trials of metformin against TB are currently underway (Singhal et al., 2014; Padmapriyadarsini et al., 2019). Glucocorticoids, such as dexamethasone, are effective in the treatment of disseminated TB, such as tuberculous meningitis, and studies have found that dexamethasone can significantly reduce the levels of IL-13 and IL-1RA (Clifford et al., 2015), however, more evidence is needed to determine whether glucocorticoids have potential immunomodulatory effects.
5 Cellular Therapy
Cellular therapy, as a potential adjunct therapeutic option for TB, refers to killing Mtb and infected cells by activating and expanding autologous or allogeneic immune effector cells in vitro and then transfusing them to the patient to correct immune imbalances and improve immune function (Rao et al., 2019). Animal experiments and clinical trials have shown that cellular therapy provides a promising new immunotherapy approach for the individualized and comprehensive treatment of MDR-TB, extensively drug-resistant TB (XDR-TB), and widely disseminated TB.
5.1 Mesenchymal Stem Cells
Mesenchymal stem cells (MSCs) are multipotent differentiation stem cells that are usually derived from the bone marrow, cord blood, and placenta (Wang et al., 2013). The advantages of their anti-inflammatory, immune regulation, tissue regeneration and repair promotion, and low immunogenicity make them promising immunotherapies for immune system disorders and immune tissue damage caused by chronic Mtb infection (Parida et al., 2015). The clinical trial results of autologous bone marrow-derived MSC-assisted treatment in patients with MDR-TB and XDR-TB with no response to previous chemotherapy showed that the functional immune response of the host recovered, the sputum turned negative, the lung cavity narrowed or closed, and the cure rate significantly increased, with only a few adverse reactions occurring in a few patients (Erokhin et al., 2008; Skrahina et al., 2012; Skrahin et al., 2014). Nonetheless, it has also been found that MSCs may activate dormant Mtb and assist Mtb immune escape and long-term latency in the host, thus leaving the possibility of TB recurrence (Hoagland et al., 2016). Moreover, it may induce a Th2 immune response to produce IL-4, inhibit the production of IFN-γ, form an immunosuppressive microenvironment in infected organs, and increase the susceptibility to pathogenic microorganisms, causing extrapulmonary TB (Hoagland et al., 2016; Khan A. et al., 2016). In addition, MSCs are stromal cells, rather than typical immune cell types. They usually regulate immunity and promote regeneration by secreting cytokines or growth factors, which makes the therapeutic mechanism difficult to clarify. In recent years, no new clinical data of MSCs in the treatment of MDR-TB or XDR-TB have been reported, so more studies are needed to clarify the therapeutic effect on TB.
5.2 γδ T Cells
γδ T cells can be divided into two subgroups depending on the different expressions of the γ and δ chains. As a major human peripheral γδ T-cell subset, Vγ9Vδ2 T cells mainly participate in the immune response by lysing cells, producing cytokines, and regulating immune cells. It has the advantages of non-major histocompatibility complex (MHC) restriction (allogeneic use), easy amplification in vitro, and low cost. Thus, Vγ9Vδ2 T cells have become adoptive cells for immunotherapy (Vantourout and Hayday, 2013). Several studies in primate Mtb infection models have shown that the PBMCs were induced by phosphate antigen combined with IL-2 injection to expand Vγ9Vδ2 T cells. Expanded Vγ9Vδ2 T cells were transplanted and then accumulated in the lungs, which could significantly reduce the bacterial load in the lungs and extrapulmonary organs, prevent the spread of Mtb, significantly reduce lung tissue injury, and increase the weight of animals. Amplified Vγ9Vδ2 T cell transplantation inhibits or kills intracellular Mtb by producing IFN-γ, perforin, and granulysin (Shen et al., 2019). In addition, expanded Vγ9Vδ2 T effector cells and IL-12 enhanced the response of CD4+/CD8+ T cells in the lung, thereby enhancing the resistance of the body to Mtb infection (Chen et al., 2013). At present, clinical trials of Vγ9Vδ2 T cells in the treatment of MDR-TB are underway in China but without relevant reports.
5.3 Cytokine-Induced Killer
Cytokine-induced killer (CIK) cells are nonrestrictive killer cells derived from human PBMCs stimulated by multiple cytokines and are amplified in vitro (Introna, 2017). One case of disseminated pulmonary TB was treated with chemotherapy combined with CIK immunotherapy in China, which showed sputum culture- and smear-negative results without liver damage after one month of treatment (Xu et al., 2015). Additional clinical trial data are needed to prove its effectiveness.
5.4 Invariant NKT Cells
Invariant NKT (iNKT) cells, a conservative subset of T cells, are restricted by CD1d and are dominated by CD4-CD8- NKT cells (Nakamura et al., 2003). Mtb-infected macrophages can activate iNKT cells to release a large amount of IFN-γ, further activate macrophages to secrete TNF-α and NO, and enhance the killing effect of phagosomes and lysosomes on Mtb (Sada-Ovalle et al., 2008). Mtb infection promotes the secretion of GM-CSF by iNKT cells in a CD1d-dependent manner to inhibit Mtb proliferation (Rothchild et al., 2014). Transplantation of iNKT cells can also limit Mtb proliferation in vivo and may become a new cell therapy to treat TB.
In addition, macrophages produced by BCG-induced differentiation of mouse hematopoietic stem cells (HSCs) provide significantly better protection against virulent Mtb infection than naïve macrophages. Adoptive transplantation of Mtb-infected macrophages from the bone marrow of animals vaccinated with BCG to another animal also showed long-lasting anti-TB activity in vivo (Kaufmann et al., 2018).
6 Future Challenges and Prospects
As the key to controlling Mtb infection, the immunity of the host has made some breakthroughs in TB immunotherapy. The application of immune interventions, including immunologically active substances, therapeutic vaccines, chemical agents, and cell therapies combined with anti-TB drugs have shown satisfactory results in animal experiments or clinical trials; a few of them have been used in the clinic. However, the overall clinical treatment of TB has not changed significantly, and the goal of “ultra-short course chemotherapy” has not been achieved. The reasons may include the following four aspects: (1) The immune regulation mechanism of TB has not yet been fully elucidated. It is well known that the immune response of TB is a double-edged sword for TB treatment. Effective use of immune methods to conduct immune regulation and immune intervention to promote its physiological response and suppress its pathological effect, to seek benefits and avoid harm, is the direction for further research in the future. (2) Only a few immunotherapy preparations can be used for clinical treatment. Many new immunotherapies are still in the preclinical research stage, suggesting that there are still many hurdles to overcome in fundamental scientific discoveries to clinical application. (3) There is a lack of immunological markers that systematically reflect the biological stages of Mtb in vivo. It is necessary to develop new immunodiagnostic methods to help clinicians clearly understand the progress of TB, judge the therapeutic effects, and predict clinical outcomes, to provide experimental evidence for the application of immunotherapy. (4) The clinical application and research are not in-depth, and the application of immune preparations is not standardized. There is also a lack of in-depth studies on the selection, dosage, application timing, treatment course of immune agents, immune status of the hosts, and impact on immunity. Moreover, a standardized, safe, and effective combined therapy scheme has not yet been developed. The development of molecular biology and immunology will reveal the immune response mechanism of TB at the molecular and cellular levels. Given the different immune defects or immune abnormalities in patients, new immunotherapeutic agents and methods will be developed, and appropriate immunotherapeutic agents and methods will be reasonably selected for combined application with anti-TB chemotherapy to treat TB effectively. Thus, immunotherapy is expected to become another critical and promising breakthrough in controlling TB, especially MDR-TB, following chemotherapy.
Author Contributions
JM: wrote and revised the manuscript. YL, JQL, WPG, SYW, JXZ and ZML: consulted the literatures and contributed with writing. XQW: supervised the manuscript. All authors contributed to the article and approved the submitted version.
Funding
This work was supported by the grant from the Serious Infectious Diseases Special Foundation of China (2018ZX10731301-005) and Key Projects of Medical Innovation Engineering (18CXZ028).
Conflict of Interest
The authors declare that the research was conducted in the absence of any commercial or financial relationships that could be construed as a potential conflict of interest.
Publisher’s Note
All claims expressed in this article are solely those of the authors and do not necessarily represent those of their affiliated organizations, or those of the publisher, the editors and the reviewers. Any product that may be evaluated in this article, or claim that may be made by its manufacturer, is not guaranteed or endorsed by the publisher.
References
Aagaard, C., Hoang, T., Dietrich, J., Cardona, P. J., Izzo, A., Dolganov, G., et al. (2011). A Multistage Tuberculosis Vaccine That Confers Efficient Protection Before and After Exposure. Nat. Med. 17 (2), 189–194. doi: 10.1038/nm.2285
Abd-Nikfarjam, B., Nassiri-Asl, M., Hajiaghayi, M., Farivar, T. N. (2018). Role of Chicoric Acid and 13-Cis Retinoic Acid in Mycobacterium Tuberculosis Infection Control by Human U937 Macrophage. Archivum. Immunol. Ther. Exp. 66 (5), 399–406. doi: 10.1007/s00005-018-0511-0
Agerberth, B., Charo, J., Werr, J., Olsson, B., Idali, F., Lindbom, L., et al. (2000). The Human Antimicrobial and Chemotactic Peptides LL-37 and α-Defensins are Expressed by Specific Lymphocyte and Monocyte Populations. Blood J. Am. Soc. Hematol. 96 (9), 3086–3093. doi: 10.1182/blood.V96.9.3086
AlMatar, M., Makky, E. A., Yakici, G., Var, I., Kayar, B., Koksal, F. (2018). Antimicrobial Peptides as an Alternative to Anti-Tuberculosis Drugs. Pharmacol. Res. 128, 288–305. doi: 10.1016/j.phrs.2017.10.011
Alvarez, N., Otero, O., Camacho, F., Borrero, R., Tirado, Y., Puig, A., et al. (2013). Passive Administration of Purified Secretory IgA From Human Colostrum Induces Protection Against Mycobacterium Tuberculosis in a Murine Model of Progressive Pulmonary Infection. BMC Immunol. 14 (S1), 1–4. doi: 10.1186/1471-2172-14-S1-S3
Bai, X., Kim, S. H., Azam, T., McGibney, M. T., Huang, H., Dinarello, C. A., et al. (2010). IL-32 is a Host Protective Cytokine Against Mycobacterium Tuberculosis in Differentiated THP-1 Human Macrophages. J. Immunol. 184 (7), 3830–3840. doi: 10.4049/jimmunol.0901913
Bai, X., Oberley-Deegan, R. E., Bai, A., Ovrutsky, A. R., Kinney, W. H., Weaver, M., et al. (2016). Curcumin Enhances Human Macrophage Control of Mycobacterium Tuberculosis Infection. Respirology 21 (5), 951–957. doi: 10.1111/resp.12762
Bai, X., Shang, S., Henao-Tamayo, M., Basaraba, R. J., Ovrutsky, A. R., Matsuda, J. L., et al. (2015). Human IL-32 Expression Protects Mice Against a Hypervirulent Strain of Mycobacterium Tuberculosis. Proc. Natl. Acad. Sci. 112 (16), 5111–5116. doi: 10.1073/pnas.1424302112
Baldwin, S. L., Reese, V. A., Huang, P. W., Beebe, E. A., Podell, B. K., Reed, S. G., et al. (2016). Protection and Long-Lived Immunity Induced by the ID93/GLA-SE Vaccine Candidate Against a Clinical Mycobacterium Tuberculosis Isolate. Clin. Vaccine Immunol. 23 (2), 137–147. doi: 10.1128/cvi.00458-15
Baldwin, S. L., Reese, V. A., Larsen, S. E., Beebe, E., Guderian, J., Orr, M. T., et al. (2021). Prophylactic Efficacy Against Mycobacterium Tuberculosis Using ID93 and Lipid-Based Adjuvant Formulations in the Mouse Model. PloS One 16 (3), e0247990. doi: 10.1371/journal.pone.0247990
Beamer, G. L., Flaherty, D. K., Assogba, B. D., Stromberg, P., Gonzalez-Juarrero, M., de Waal Malefyt, R., et al. (2008). Interleukin-10 Promotes Mycobacterium Tuberculosis Disease Progression in CBA/J Mice. J. Immunol. 181 (8), 5545–5550. doi: 10.4049/jimmunol.181.8.5545
Bekele, A., Gebreselassie, N., Ashenafi, S., Kassa, E., Aseffa, G., Amogne, W., et al. (2018). Daily Adjunctive Therapy With Vitamin D 3 and Phenylbutyrate Supports Clinical Recovery From Pulmonary Tuberculosis: A Randomized Controlled Trial in Ethiopia. J. Internal Med. 284 (3), 292–306. doi: 10.1111/joim.12767
Bertholet, S., Ireton, G. C., Ordway, D. J., Windish, H. P., Pine, S. O., Kahn, M., et al. (2010). A Defined Tuberculosis Vaccine Candidate Boosts BCG and Protects Against Multidrug-Resistant Mycobacterium Tuberculosis. Sci. Trans. Med. 2 (53), 53ra74–53ra74. doi: 10.1126/scitranslmed.3001094
Bohua Wu, Y. C., Chen, B. (2018). Observation of Thymosin Combined With Antituberculosis Drugs for Retreatment of Smear Positive Pulmonary Tuberculosis. J. Modern Clin. Med. 44 (1), 58–59.
Brennan, P. J. (2003). Structure, Function, and Biogenesis of the Cell Wall of Mycobacterium Tuberculosis. Tuberc. (Edinb) 83 (1-3), 91–97. doi: 10.1016/s1472-9792(02)00089-6
Buccheri, S., Reljic, R., Caccamo, N., Ivanyi, J., Singh, M., Salerno, A., et al. (2007). IL-4 Depletion Enhances Host Resistance and Passive IgA Protection Against Tuberculosis Infection in BALB/c Mice. Eur. J. Immunol. 37 (3), 729–737. doi: 10.1002/eji.200636764
Butov, D., Zaitseva, S., Butova, T., Stepanenko, G., Pogorelova, O., Zhelezniakova, N. (2016). Efficacy and Safety of Quercetin and Polyvinylpyrrolidone in Treatment of Patients With Newly Diagnosed Destructive Pulmonary Tuberculosis in Comparison With Standard Antimycobacterial Therapy. Int. J. Mycobacteriol. 5 (4), 446–453. doi: 10.1016/j.ijmyco.2016.06.012
Cadena, A. M., Flynn, J. L., Fortune, S. M. (2016). The Importance of First Impressions: Early Events in Mycobacterium Tuberculosis Infection Influence Outcome. mBio 7 (2), e00342–e00316. doi: 10.1128/mBio.00342-16
Cardona, P.-J. (2006). RUTI: A New Chance to Shorten the Treatment of Latent Tuberculosis Infection. Tuberculosis 86 (3-4), 273–289. doi: 10.1016/j.tube.2006.01.024
Cardona, P.-J., Amat, I., Gordillo, S., Arcos, V., Guirado, E., Díaz, J., et al. (2005). Immunotherapy With Fragmented Mycobacterium Tuberculosis Cells Increases the Effectiveness of Chemotherapy Against a Chronical Infection in a Murine Model of Tuberculosis. Vaccine 23 (11), 1393–1398. doi: 10.1016/j.vaccine.2004.09.008
Chaudhari, V. S., Malakar, T. K., Murty, U. S., Banerjee, S. (2021). Extruded Filaments Derived 3d Printed Medicated Skin Patch to Mitigate Destructive Pulmonary Tuberculosis: Design to Delivery. Expert Opin. Drug Delivery 18 (2), 301–313. doi: 10.1080/17425247.2021.1845648
Chen, H., Liu, X., Ma, X., Wang, Q., Yang, G., Niu, H., et al. (2017). A New Rabbit-Skin Model to Evaluate Protective Efficacy of Tuberculosis Vaccines. Front. Microbiol. 8, 842. doi: 10.3389/fmicb.2017.00842
Chen, C. Y., Yao, S., Huang, D., Wei, H., Sicard, H., Zeng, G., et al. (2013). Phosphoantigen/IL2 Expansion and Differentiation of Vγ2vδ2 T Cells Increase Resistance to Tuberculosis in Nonhuman Primates. PloS Pathog. 9 (8), e1003501. doi: 10.1371/journal.ppat.1003501
Clifford, V., Zufferey, C., Germano, S., Ryan, N., Leslie, D., Street, A., et al. (2015). The Impact of Anti-Tuberculous Antibiotics and Corticosteroids on Cytokine Production in QuantiFERON-TB Gold In Tube Assays. Tuberc. (Edinb) 95 (3), 343–349. doi: 10.1016/j.tube.2015.02.039
Costello, A. M., Kumar, A., Narayan, V., Akbar, M. S., Ahmed, S., Abou-Zeid, C., et al. (1992). Does Antibody to Mycobacterial Antigens, Including Lipoarabinomannan, Limit Dissemination in Childhood Tuberculosis? Trans. R. Soc. Trop. Med. Hyg. 86 (6), 686–692. doi: 10.1016/0035-9203(92)90192-f
Das, S., Chowdhury, B. P., Goswami, A., Parveen, S., Jawed, J., Pal, N., et al. (2016). Mycobacterium Indicus Pranii (MIP) Mediated Host Protective Intracellular Mechanisms Against Tuberculosis Infection: Involvement of TLR-4 Mediated Signaling. Tuberculosis 101, 201–209. doi: 10.1016/j.tube.2016.09.027
de Martino, M., Lodi, L., Galli, L., Chiappini, E. (2019). Immune Response to Mycobacterium Tuberculosis: A Narrative Review. Front. Pediatr. 7, 350. doi: 10.3389/fped.2019.00350
Deng, J., Yang, Y., He, J., Xie, Z., Luo, F., Xu, J., et al. (2021). Vitamin D Receptor Activated by Vitamin D Administration Alleviates Mycobacterium Tuberculosis-Induced Bone Destruction by Inhibiting Nfκb-Mediated Aberrant Osteoclastogenesis. FASEB J. 35 (6), e21543. doi: 10.1096/fj.202100135R
Denis, M., Gregg, E. O., Ghandirian, E. (1990). Cytokine Modulation of Mycobacterium Tuberculosis Growth in Human Macrophages. Int. J. Immunopharmacol. 12 (7), 721–727. doi: 10.1016/0192-0561(90)90034-k
Divangahi, M., Aaby, P., Khader, S. A., Barreiro, L. B., Bekkering, S., Chavakis, T., et al. (2021). Trained Immunity, Tolerance, Priming and Differentiation: Distinct Immunological Processes. Nat. Immunol. 22 (1), 2–6. doi: 10.1038/s41590-020-00845-6
Dwivedi, V. P., Bhattacharya, D., Singh, M., Bhaskar, A., Kumar, S., Fatima, S., et al. (2019). Allicin Enhances Antimicrobial Activity of Macrophages During Mycobacterium Tuberculosis Infection. J. Ethnopharmacol. 243, 111634. doi: 10.1016/j.jep.2018.12.008
Efremenko, Y. V., Butov, D. A., Prihoda, N. D., Zaitzeva, S. I., Yurchenko, L. V., Sokolenko, N. I., et al. (2013). Randomized, Placebo-Controlled Phase II Trial of Heat-Killed Mycobacterium Vaccae (Longcom Batch) Formulated as an Oral Pill (V7). Hum. Vaccines Immunother. 9 (9), 1852–1856. doi: 10.4161/hv.25280
Erokhin, V. V., Vasil'Eva, I. A., Konopliannikov, A. G., Chukanov, V. I., Tsyb, A. F., Bagdasarian, T. R., et al. (2008). Systemic Transplantation of Autologous Mesenchymal Stem Cells of the Bone Marrow in the Treatment of Patients With Multidrug-Resistant Pulmonary Tuberculosis. Probl. Tuberk. Bolezn. Legk. 10), 3–6.
Fehlbaum, P., Rao, M., Zasloff, M., Anderson, G. M. (2000). An Essential Amino Acid Induces Epithelial β-Defensin Expression. Proc. Natl. Acad. Sci. 97 (23), 12723–12728. doi: 10.1073/pnas.220424597
Francisco-Cruz, A., Mata-Espinosa, D., Ramos-Espinosa, O., Marquina-Castillo, B., Estrada-Parra, S., Xing, Z., et al. (2016). Efficacy of Gene-Therapy Based on Adenovirus Encoding Granulocyte-Macrophage Colony-Stimulating Factor in Drug-Sensitive and Drug-Resistant Experimental Pulmonary Tuberculosis. Tuberculosis 100, 5–14. doi: 10.1016/j.tube.2016.05.015
Gomez, M., McCollum, J., Wang, H., Ordoubadi, M., Jar, C., Carrigy, N. B., et al. (2021). Development of a Formulation Platform for a Spray-Dried, Inhalable Tuberculosis Vaccine Candidate. Int. J. Pharm. 593, 120121. doi: 10.1016/j.ijpharm.2020.120121
Gong, W. P., Liang, Y., Ling, Y. B., Zhang, J. X., Yang, Y. R., Wang, L., et al. (2020). Effects of Mycobacterium Vaccae Vaccine in a Mouse Model of Tuberculosis: Protective Action and Differentially Expressed Genes. Mil. Med. Res. 7 (1), 25. doi: 10.1186/s40779-020-00258-4
Gong, W., Liang, Y., Mi, J., Jia, Z., Xue, Y., Wang, J., et al. (2021a). Peptides-Based Vaccine MP3RT Induced Protective Immunity Against Mycobacterium Tuberculosis Infection in a Humanized Mouse Model. Front. Immunol. 12, 666290. doi: 10.3389/fimmu.2021.666290
Gong, W., Liang, Y., Mi, J., Xue, Y., Wang, J., Wang, L. (2021b). A Peptide-Based Vaccine ACP Derived From Antigens of Mycobacterium Tuberculosis Induced Th1 Response But Failed to Enhance the Protective Efficacy of BCG in Mice. Indian J. Tuberc. doi: 10.1016/j.ijtb.2021.08.016
Gong, W., Liang, Y., Wu, X. (2018). The Current Status, Challenges, and Future Developments of New Tuberculosis Vaccines. Hum. Vaccin. Immunother. 14 (7), 1697–1716. doi: 10.1080/21645515.2018.1458806
Gong, W., Wu, X. (2021). Differential Diagnosis of Latent Tuberculosis Infection and Active Tuberculosis: A Key to a Successful Tuberculosis Control Strategy. Front. Microbiol. doi: 10.3389/fmicb.2021.745592
Gröschel, M. I., Prabowo, S. A., Cardona, P.-J., Stanford, J. L., van der Werf, T. S. (2014). Therapeutic Vaccines for Tuberculosis—A Systematic Review. Vaccine 32 (26), 3162–3168. doi: 10.1016/j.vaccine.2014.03.047
Gundersen, T. E., Lundanes, E., Blomhoff, R. (1997). Quantitative High-Performance Liquid Chromatographic Determination of Retinoids in Human Serum Using on-Line Solid-Phase Extraction and Column Switching. Determination of 9-Cis-Retinoic Acid, 13-Cis-Retinoic Acid, All-Trans-Retinoic Acid, 4-Oxo-All-Trans-Retinoicacid and 4-Oxo-13-Cis-Retinoic Acid. J. Chromatogr. B. BioMed. Sci. Appl. 691 (1), 43–58. doi: 10.1016/s0378-4347(96)00434-3
Gupta, S., Cheung, L., Pokkali, S., Winglee, K., Guo, H., Murphy, J. R., et al. (2017). Suppressor Cell–Depleting Immunotherapy With Denileukin Diftitox is an Effective Host-Directed Therapy for Tuberculosis. J. Infect. Dis. 215 (12), 1883–1887. doi: 10.1093/infdis/jix208
Hamasur, B., Haile, M., Pawlowski, A., Schröder, U., Källenius, G., Svenson, S. (2004a). A Mycobacterial Lipoarabinomannan Specific Monoclonal Antibody and its F (Ab′) 2 Fragment Prolong Survival of Mice Infected With Mycobacterium Tuberculosis. Clin. Exp. Immunol. 138 (1), 30–38. doi: 10.1111/j.1365-2249.2004.02593.x
Hamasur, B., Haile, M., Pawlowski, A., Schroder, U., Kallenius, G., Svenson, S. B. (2004b). A Mycobacterial Lipoarabinomannan Specific Monoclonal Antibody and Its F(ab') Fragment Prolong Survival of Mice Infected With Mycobacterium Tuberculosis. Clin. Exp. Immunol. 138 (1), 30–38. doi: 10.1111/j.1365-2249.2004.02593.x
Hancock, R. E., Lehrer, R. (1998). Cationic Peptides: A New Source of Antibiotics. Trends Biotechnol. 16 (2), 82–88. doi: 10.1016/s0167-7799(97)01156-6
Hernández-Pando, R., Orozco-Esteves, H., Maldonado, H., Aguilar-León, D., Vilchis-Landeros, M., Mata-Espinosa, D., et al. (2006). A Combination of a Transforming Growth Factor-β Antagonist and an Inhibitor of Cyclooxygenase Is an Effective Treatment for Murine Pulmonary Tuberculosis. Clin. Exp. Immunol. 144 (2), 264–272. doi: 10.1111/j.1365-2249.2006.03049.x
Hoagland, D., Liu, J., Lee, R. B., Lee, R. E. (2016). New Agents for the Treatment of Drug-Resistant Mycobacterium Tuberculosis. Adv. Drug Deliv. Rev. 102, 55–72. doi: 10.1016/j.addr.2016.04.026
Huang, C.-Y., Hsieh, W.-Y. (2017). Efficacy of Mycobacterium Vaccae Immunotherapy for Patients With Tuberculosis: A Systematic Review and Meta-Analysis. Hum. Vaccines Immunother. 13 (9), 1960–1971. doi: 10.1080/21645515.2017.1335374
Introna, M. (2017). CIK as Therapeutic Agents Against Tumors. J. Autoimmun. 85, 32–44. doi: 10.1016/j.jaut.2017.06.008
Johnson, B., Bekker, L. G., Ress, S., Kaplan, G. (1998). Recombinant Interleukin 2 Adjunctive Therapy in Multidrug-Resistant Tuberculosis. Novartis Found. Symp. 217 (217), 99–105. doi: 10.1002/0470846526.ch7
Johnson, B. J., Estrada, I., Shen, Z., Ress, S., Willcox, P., Colston, M. J., et al. (1998). Differential Gene Expression in Response to Adjunctive Recombinant Human Interleukin-2 Immunotherapy in Multidrug-Resistant Tuberculosis Patients. Infect. Immun. 66 (6), 2426–2433. doi: 10.1128/IAI.66.6.2426-2433.1998
Johnson, J. L., Ssekasanvu, E., Okwera, A., Mayanja, H., Hirsch, C. S., Nakibali, J. G., et al. (2003). Randomized Trial of Adjunctive Interleukin-2 in Adults With Pulmonary Tuberculosis. Am. J. Respir. Crit. Care Med. 168 (2), 185–191. doi: 10.1164/rccm.200211-1359OC
Juárez, E., Ruiz, A., Cortez, O., Sada, E., Torres, M. (2018). Antimicrobial and Immunomodulatory Activity Induced by Loperamide in Mycobacterial Infections. Int. Immunopharmacol. 65, 29–36. doi: 10.1016/j.intimp.2018.09.013
Kaufmann, E., Sanz, J., Dunn, J. L., Khan, N., Mendonça, L. E., Pacis, A., et al. (2018). BCG Educates Hematopoietic Stem Cells to Generate Protective Innate Immunity Against Tuberculosis. Cell 172 (1-2), 176–190. doi: 10.1016/j.cell.2017.12.031
Khan, A., Hunter, R. L., Jagannath, C. (2016). Emerging Role of Mesenchymal Stem Cells During Tuberculosis: The Fifth Element in Cell Mediated Immunity. Tuberculosis 101, S45–S52. doi: 10.1016/j.tube.2016.09.019
Khan, N., Vidyarthi, A., Javed, S., Agrewala, J. N. (2016). Innate Immunity Holding the Flanks Until Reinforced by Adaptive Immunity Against Mycobacterium Tuberculosis Infection. Front. Microbiol. 7, 328. doi: 10.3389/fmicb.2016.00328
Kim, S.-H., Han, S.-Y., Azam, T., Yoon, D.-Y., Dinarello, C. A. (2005). Interleukin-32: A Cytokine and Inducer of Tnfα. Immunity 22 (1), 131–142. doi: 10.1016/j.immuni.2004.12.003
Koeken, V. A., Verrall, A. J., Ardiansyah, E., Apriani, L., dos Santos, J. C., Kumar, V., et al. (2019). IL-32 and its Splice Variants are Associated With Protection Against Mycobacterium Tuberculosis Infection and Skewing of Th1/Th17 Cytokines. J. Leuk. Biol. 107 (1), 113–118. doi: 10.1002/JLB.4AB0219-071R
Korbel, D. S., Schneider, B. E., Schaible, U. E. (2008). Innate Immunity in Tuberculosis: Myths and Truth. Microbes Infect. 10 (9), 995–1004. doi: 10.1016/j.micinf.2008.07.039
Kumar, S., Sharma, C., Kaushik, S. R., Kulshreshtha, A., Chaturvedi, S., Nanda, R. K., et al. (2019). The Phytochemical Bergenin as an Adjunct Immunotherapy for Tuberculosis in Mice. J. Biol. Chem. 294 (21), 8555–8563. doi: 10.1074/jbc.RA119.008005
Leroux-Roels, I., Forgus, S., De Boever, F., Clement, F., Demoitié, M.-A., Mettens, P., et al. (2013). Improved CD4+ T Cell Responses toMycobacterium Tuberculosis in PPD-Negative Adults by M72/AS01 as Compared to the M72/AS02 and Mtb72F/AS02 Tuberculosis Candidate Vaccine Formulations: A Randomized Trial. Vaccine 31 (17), 2196–2206. doi: 10.1016/j.vaccine.2012.05.035
Liang, Y., Wu, X., Zhang, J., Xiao, L., Yang, Y., Bai, X., et al. (2012). Immunogenicity and Therapeutic Effects of Ag85A/B Chimeric DNA Vaccine in Mice Infected With Mycobacterium Tuberculosis. FEMS Immunol. Med. Microbiol. 66 (3), 419–426. doi: 10.1111/1574-695x.12008
Liang, Y., Zhang, X., Bai, X., Xiao, L., Wang, X., Zhang, J., et al. (2017). Immunogenicity and Therapeutic Effects of a Mycobacterium Tuberculosis Rv2190c DNA Vaccine in Mice. BMC Immunol. 18 (1), 11. doi: 10.1186/s12865-017-0196-x
Liang, Y., Zhang, X., Xiao, L., Bai, X., Wang, X., Yang, Y., et al. (2016). Immunogenicity and Therapeutic Effects of Pvax1- Rv1419 DNA From Mycobacterium Tuberculosis. Curr. Gene Ther. 16 (4), 249–255. doi: 10.2174/1566523216666161102170123
Liang, Y., Zhao, Y., Bai, X., Xiao, L., Yang, Y., Zhang, J., et al. (2018). Immunotherapeutic Effects of Mycobacterium Tuberculosis Rv3407 DNA Vaccine in Mice. Autoimmunity 51 (8), 417–422. doi: 10.1080/08916934.2018.1546291
Li, W., Deng, W., Xie, J. (2018). The Biology and Role of Interleukin-32 in Tuberculosis. J. Immunol. Res. 2018:1535194. doi: 10.1155/2018/1535194
Liu, X., Da, Z., Wang, Y., Niu, H., Li, R., Yu, H., et al. (2016). A Novel Liposome Adjuvant DPC Mediates Mycobacterium Tuberculosis Subunit Vaccine Well to Induce Cell-Mediated Immunity and High Protective Efficacy in Mice. Vaccine 34 (11), 1370–1378. doi: 10.1016/j.vaccine.2016.01.049
López-García, S., Castañeda-Sanchez, J. I., Jiménez-Arellanes, A., Domínguez-López, L., Castro-Mussot, M. E., Hernández-Sanchéz, J., et al. (2015). Macrophage Activation by Ursolic and Oleanolic Acids During Mycobacterial Infection. Molecules 20 (8), 14348–14364. doi: 10.3390/molecules200814348
Luabeya, A. K. K., Kagina, B. M., Tameris, M. D., Geldenhuys, H., Hoff, S. T., Shi, Z., et al. (2015). First-In-Human Trial of the Post-Exposure Tuberculosis Vaccine H56: IC31 in Mycobacterium Tuberculosis Infected and non-Infected Healthy Adults. Vaccine 33 (33), 4130–4140. doi: 10.1016/j.vaccine.2015.06.051
Lu, J.-b., Chen, B.-w., Wang, G.-z., Fu, L.-l., Shen, X.-b., Su, C., et al. (2015). Recombinant Tuberculosis Vaccine AEC/BC02 Induces Antigen-Specific Cellular Responses in Mice and Protects Guinea Pigs in a Model of Latent Infection. J. Microbiol. Immunol. Infect. 48 (6), 597–603. doi: 10.1016/j.jmii.2014.03.005
Lu, J.-b., Miao, X. (2019). Progress in Clinical Trials of Inactivated Mycobacterium Vaccines Against Tuberculosis. Prog. Microbiol. Immunol. 47 (4), 69–74. doi: 10.13309/j.cnki.pmi.2019.04.013
Ma, Y., Chen, H.-D., Wang, Y., Wang, Q., Li, Y., Zhao, Y., et al. (2011). Interleukin 24 as a Novel Potential Cytokine Immunotherapy for the Treatment of Mycobacterium Tuberculosis Infection. Microbes Infect. 13 (12-13), 1099–1110. doi: 10.1016/j.micinf.2011.06.012
Martineau, A. R., Wilkinson, K. A., Newton, S. M., Floto, R. A., Norman, A. W., Skolimowska, K., et al. (2007). IFN-γ-and TNF-Independent Vitamin D-Inducible Human Suppression of Mycobacteria: The Role of Cathelicidin LL-37. J. Immunol. 178 (11), 7190–7198. doi: 10.4049/jimmunol.178.11.7190
Mayer-Barber, K. D., Andrade, B. B., Oland, S. D., Amaral, E. P., Barber, D. L., Gonzales, J., et al. (2014). Host-Directed Therapy of Tuberculosis Based on Interleukin-1 and Type I Interferon Crosstalk. Nature 511 (7507), 99–103. doi: 10.1038/nature13489
Mayosi, B. M., Ntsekhe, M., Bosch, J., Pandie, S., Jung, H., Gumedze, F., et al. (2014). Prednisolone and Mycobacterium Indicus Pranii in Tuberculous Pericarditis. New Engl. J. Med. 371 (12), 1121–1130. doi: 10.1056/NEJMoa1407380
Mourik, B. C., Leenen, P. J., de Knegt, G. J., Huizinga, R., van der Eerden, B. C., Wang, J., et al. (2017). Immunotherapy Added to Antibiotic Treatment Reduces Relapse of Disease in a Mouse Model of Tuberculosis. Am. J. Respir. Cell Mol. Biol. 56 (2), 233–241. doi: 10.1165/rcmb.2016-0185OC
Munseri, P., Said, J., Amour, M., Magohe, A., Matee, M., Rees, C. A., et al. (2020). DAR-901 Vaccine for the Prevention of Infection With Mycobacterium Tuberculosis Among BCG-Immunized Adolescents in Tanzania: A Randomized Controlled, Double-Blind Phase 2b Trial. Vaccine 38 (46), 7239–7245. doi: 10.1016/j.vaccine.2020.09.055
Nakamura, T., Sonoda, K. H., Faunce, D. E., Gumperz, J., Yamamura, T., Miyake, S., et al. (2003). CD4+ NKT Cells, But Not Conventional CD4+ T Cells, Are Required to Generate Efferent CD8+ T Regulatory Cells Following Antigen Inoculation in an Immune-Privileged Site. J. Immunol. 171 (3), 1266–1271. doi: 10.4049/jimmunol.171.3.1266
Napier, R. J., Rafi, W., Cheruvu, M., Powell, K. R., Zaunbrecher, M. A., Bornmann, W., et al. (2011). Imatinib-Sensitive Tyrosine Kinases Regulate Mycobacterial Pathogenesis and Represent Therapeutic Targets Against Tuberculosis. Cell Host Microbe 10 (5), 475–485. doi: 10.1016/j.chom.2011.09.010
Nasr, M. M., Ebrahim, H. M., Khattab, F. M., Marei, A. M. (2018). Bacillus Calmette-Guerin, Polysaccharide Nucleic Acid in the Treatment of Cutaneous and Oral Lichen Planus. Dermatol. Ther. 31 (3), e12591. doi: 10.1111/dth.12591
Nell, A. S., D'Lom, E., Bouic, P., Sabate, M., Bosser, R., Picas, J., et al. (2014). Safety, Tolerability, and Immunogenicity of the Novel Antituberculous Vaccine RUTI: Randomized, Placebo-Controlled Phase II Clinical Trial in Patients With Latent Tuberculosis Infection. PloS One 9 (2), e89612. doi: 10.1371/journal.pone.0089612
Netea, M. G., Lewis, E. C., Azam, T., Joosten, L. A., Jaekal, J., Bae, S.-Y., et al. (2008). Interleukin-32 Induces the Differentiation of Monocytes Into Macrophage-Like Cells. Proc. Natl. Acad. Sci. 105 (9), 3515–3520. doi: 10.1073/pnas.0712381105
Niu, H., Peng, J., Bai, C., Liu, X., Hu, L., Luo, Y., et al. (2015). Multi-Stage Tuberculosis Subunit Vaccine Candidate LT69 Provides High Protection Against Mycobacterium Tuberculosis Infection in Mice. PloS One 10 (6), e0130641. doi: 10.1371/journal.pone.0130641
Oosthuizen, C., Arbach, M., Meyer, D., Hamilton, C., Lall, N. (2017). Diallyl Polysulfides From Allium Sativum as Immunomodulators, Hepatoprotectors, and Antimycobacterial Agents. J. Med. Food 20 (7), 685–690. doi: 10.1089/jmf.2016.0137
Padmapriyadarsini, C., Bhavani, P. K., Natrajan, M., Ponnuraja, C., Kumar, H., Gomathy, S. N., et al. (2019). Evaluation of Metformin in Combination With Rifampicin Containing Antituberculosis Therapy in Patients With New, Smear-Positive Pulmonary Tuberculosis (METRIF): Study Protocol for a Randomised Clinical Trial. BMJ Open 9 (3), e024363. doi: 10.1136/bmjopen-2018-024363
Parida, S. K., Madansein, R., Singh, N., Padayatchi, N., Master, I., Naidu, K., et al. (2015). Cellular Therapy in Tuberculosis. Int. J. Infect. Dis. 32, 32–38. doi: 10.1016/j.ijid.2015.01.016
Patil, P. S., Cheng, T.-J. R., Zulueta, M. M. L., Yang, S.-T., Lico, L. S., Hung, S.-C. (2015). Total Synthesis of Tetraacylated Phosphatidylinositol Hexamannoside and Evaluation of its Immunomodulatory Activity. Nat. Commun. 6 (1), 1–9. doi: 10.1038/ncomms8239
Pedral-Sampaio, D. B., Netto, E. M., Brites, C., Bandeira, A. C., Guerra, C., Barberin, M. G., et al. (2003). Use of Rhu-GM-CSF in Pulmonary Tuberculosis Patients: Results of a Randomized Clinical Trial. Braz. J. Infect. Dis. 7 (4), 245–252. doi: 10.1590/S1413-86702003000400004
Pethe, K., Alonso, S., Biet, F., Delogu, G., Brennan, M. J., Locht, C., et al. (2001). The Heparin-Binding Haemagglutinin of M. Tuberculosis is Required for Extrapulmonary Dissemination. Nature 412 (6843), 190–194. doi: 10.1038/35084083
Ramos-Espinosa, O., Islas-Weinstein, L., Peralta-Álvarez, M. P., López-Torres, M. O., Hernández-Pando, R. (2018). The Use of Immunotherapy for the Treatment of Tuberculosis. Expert Rev. Respir. Med. 12 (5), 427–440. doi: 10.1080/17476348.2018.1457439
Rao, M., Ligeiro, D., Maeurer, M. (2019). Precision Medicine in the Clinical Management of Respiratory Tract Infections Including Multidrug-Resistant Tuberculosis: Learning From Innovations in Immuno-Oncology. Curr. Opin. Pulm. Med. 25 (3), 233–241. doi: 10.1097/mcp.0000000000000575
Rivas-Santiago, B., Castañeda-Delgado, J. E., Santiago, C. E. R., Waldbrook, M., González-Curiel, I., León–Contreras, J. C., et al. (2013). Ability of Innate Defence Regulator Peptides IDR-1002, IDR-HH2 and IDR-1018 to Protect Against Mycobacterium Tuberculosis Infections in Animal Models. PloS One 8 (3), e59119. doi: 10.1371/journal.pone.0059119
Rivas-Santiago, B., Rivas-Santiago, C., Sada, E., Hernández-Pando, R. (2015). Prophylactic Potential of Defensins and L-Isoleucine in Tuberculosis Household Contacts: An Experimental Model. Immunotherapy 7 (3), 207–213. doi: 10.2217/imt.14.119
Rodríguez, A., Tjärnlund, A., Ivanji, J., Singh, M., García, I., Williams, A., et al. (2005). Role of IgA in the Defense Against Respiratory Infections: IgA Deficient Mice Exhibited Increased Susceptibility to Intranasal Infection With Mycobacterium Bovis BCG. Vaccine 23 (20), 2565–2572. doi: 10.1016/j.vaccine.2004.11.032
Rojas, R. E., Thomas, J. J., Gehring, A. J., Hill, P. J., Belisle, J. T., Harding, C. V., et al. (2006). Phosphatidylinositol Mannoside From Mycobacterium Tuberculosis Binds Alpha5beta1 Integrin (VLA-5) on CD4+ T Cells and Induces Adhesion to Fibronectin. J. Immunol. 177 (5), 2959–2968. doi: 10.4049/jimmunol.177.5.2959
Rothchild, A. C., Jayaraman, P., Nunes-Alves, C., Behar, S. M. (2014). iNKT Cell Production of GM-CSF Controls Mycobacterium Tuberculosis. PloS Pathog. 10 (1), e1003805. doi: 10.1371/journal.ppat.1003805
Sada-Ovalle, I., Chiba, A., Gonzales, A., Brenner, M. B., Behar, S. M. (2008). Innate Invariant NKT Cells Recognize Mycobacterium Tuberculosis–Infected Macrophages, Produce Interferon-γ, and Kill Intracellular Bacteria. PloS Pathog. 4 (12), e1000239. doi: 10.1371/journal.ppat.1000239
Segueni, N., Tritto, E., Bourigault, M.-L., Rose, S., Erard, F., Le Bert, M., et al. (2016). Controlled Mycobacterium Tuberculosis Infection in Mice Under Treatment With Anti-IL-17A or IL-17f Antibodies, in Contrast to Tnfα Neutralization. Sci. Rep. 6, 36923. doi: 10.1038/srep36923
Sharma, S. K., Katoch, K., Sarin, R., Balambal, R., Jain, N. K., Patel, N., et al. (2017). Efficacy and Safety of Mycobacterium Indicus Pranii as an Adjunct Therapy in Category II Pulmonary Tuberculosis in a Randomized Trial. Sci. Rep. 7 (1), 3354. doi: 10.1038/s41598-017-03514-1
Sharma, A., Vaghasiya, K., Gupta, P., Gupta, U. D., Verma, R. K. (2018). Reclaiming Hijacked Phagosomes: Hybrid Nano-In-Micro Encapsulated MIAP Peptide Ensures Host Directed Therapy by Specifically Augmenting Phagosome-Maturation and Apoptosis in TB Infected Macrophage Cells. Int. J. Pharm. 536 (1), 50–62. doi: 10.1016/j.ijpharm.2017.11.046
Shen, L., Frencher, J., Huang, D., Wang, W., Yang, E., Chen, C. Y., et al. (2019). Immunization of Vγ2vδ2 T Cells Programs Sustained Effector Memory Responses That Control Tuberculosis in Nonhuman Primates. Proc. Natl. Acad. Sci. U.S.A. 116 (13), 6371–6378. doi: 10.1073/pnas.1811380116
Shi, X., Hui, L., Zhong, S. (2004). Study on the Role of the Chimeric Hsp70/CD80 DNA Vaccine for Treating Infection of Mycobacterium Tuberculosis. Chin. J. Infect. Dis. 22 (1), 30–33.
Shin, A. R., Lee, K. S., Lee, J. S., Kim, S. Y., Song, C. H., Jung, S. B., et al. (2006). Mycobacterium Tuberculosis HBHA Protein Reacts Strongly With the Serum Immunoglobulin M of Tuberculosis Patients. Clin. Vaccine Immunol. 13 (8), 869–875. doi: 10.1128/cvi.00103-06
Sia, J. K., Georgieva, M., Rengarajan, J. (2015). Innate Immune Defenses in Human Tuberculosis: An Overview of the Interactions Between Mycobacterium Tuberculosis and Innate Immune Cells. J. Immunol. Res. 2015:747543. doi: 10.1155/2015/747543
Silva, J. P., Appelberg, R., Gama, F. M. (2016a). Antimicrobial Peptides as Novel Anti-Tuberculosis Therapeutics. Biotechnol. Adv. 34 (5), 924–940. doi: 10.1016/j.biotechadv.2016.05.007
Silva, J. P., Gonçalves, C., Costa, C., Sousa, J., Silva-Gomes, R., Castro, A. G., et al. (2016b). Delivery of LLKKK18 Loaded Into Self-Assembling Hyaluronic Acid Nanogel for Tuberculosis Treatment. J. Controlled Release 235, 112–124. doi: 10.1016/j.jconrel.2016.05.064
Singhal, A., Jie, L., Kumar, P., Hong, G. S., Leow, M. K., Paleja, B., et al. (2014). Metformin as Adjunct Antituberculosis Therapy. Sci. Transl. Med. 6 (263), 263ra159. doi: 10.1126/scitranslmed.3009885
Singh, A., Mohan, A., Dey, A. B., Mitra, D. K. (2013). Inhibiting the Programmed Death 1 Pathway Rescues Mycobacterium Tuberculosis–Specific Interferon γ–Producing T Cells From Apoptosis in Patients With Pulmonary Tuberculosis. J. Infect. Dis. 208 (4), 603–615. doi: 10.1093/infdis/jit206
Singh, P., Subbian, S. (2018). Harnessing the mTOR Pathway for Tuberculosis Treatment. Front. Microbiol. 9, 70. doi: 10.3389/fmicb.2018.00070
Skrahin, A., Ahmed, R., Ferrara, G., Rane, L., Poiret, T., Isaikina, Y., et al. (2014). Autologous Mesenchymal Stromal Cell Infusion as Adjunct Treatment in Patients With Multidrug and Extensively Drug-Resistant Tuberculosis: An Open-Label Phase 1 Safety Trial. Lancet Respir. Med. 2 (2), 108–122. doi: 10.1016/S2213-2600(13)70234-0
Skrahina, A., Hurevich, H., Zalutskaya, A., Sahalchyk, E., Astrauko, A., van Gemert, W., et al. (2012). Alarming Levels of Drug-Resistant Tuberculosis in Belarus: Results of a Survey in Minsk. Eur. Respir. J. 39 (6), 1425–1431. doi: 10.1183/09031936.00145411
Sudjarwo, S. A., Eraiko, K., Giftania Wardani Sudjarwo, K. (2017a). The Activity of Immunoglobulin Y Anti-Mycobacterium Tuberculosis on Proliferation and Cytokine Expression of Rat Peripheral Blood Mononuclear Cells. Pharmacogn. Res. 9 (S1), S5–S8. doi: 10.4103/pr.pr_66_17
Sudjarwo, S. A., Eraiko, K., Sudjarwo, G. W., Koerniasari (2017b). The Potency of Chicken Egg Yolk Immunoglobulin (IgY) Specific as Immunotherapy to Mycobacterium Tuberculosis Infection. J. Adv. Pharm. Technol. Res. 8 (3), 91–96. doi: 10.4103/japtr.JAPTR_167_16
Teitelbaum, R., Glatman-Freedman, A., Chen, B., Robbins, J. B., Unanue, E., Casadevall, A., et al. (1998). A mAb Recognizing a Surface Antigen of Mycobacterium Tuberculosis Enhances Host Survival. Proc. Natl. Acad. Sci. U.S.A. 95 (26), 15688–15693. doi: 10.1073/pnas.95.26.15688
Torrelles, J. B., Azad, A. K., Schlesinger, L. S. (2006). Fine Discrimination in the Recognition of Individual Species of Phosphatidyl-Myo-Inositol Mannosides From Mycobacterium Tuberculosis by C-Type Lectin Pattern Recognition Receptors. J. Immunol. 177 (3), 1805–1816. doi: 10.4049/jimmunol.177.3.1805
Van Der Meeren, O., Hatherill, M., Nduba, V., Wilkinson, R. J., Muyoyeta, M., Van Brakel, E., et al. (2018). Phase 2b Controlled Trial of M72/AS01E Vaccine to Prevent Tuberculosis. N. Engl. J. Med. 379 (17), 1621–1634. doi: 10.1056/NEJMoa1803484
Vantourout, P., Hayday, A. (2013). Six-Of-The-Best: Unique Contributions of γδ T Cells to Immunology. Nat. Rev. Immunol. 13 (2), 88. doi: 10.1038/nri3384
Von Reyn, C. F., Lahey, T., Arbeit, R. D., Landry, B., Kailani, L., Adams, L. V., et al. (2017). Safety and Immunogenicity of an Inactivated Whole Cell Tuberculosis Vaccine Booster in Adults Primed With BCG: A Randomized, Controlled Trial of DAR-901. PloS One 12 (5), e0175215. doi: 10.1371/journal.pone.0175215
Wallis, R. S., Zumla, A. (2016). Vitamin D as Adjunctive Host-Directed Therapy in Tuberculosis: A Systematic Review. Open Forum Infect. Dis. 3 (3), 151. doi: 10.1093/ofid/ofw151
Wang, X., Wang, Y., Gou, W., Lu, Q., Peng, J., Lu, S. (2013). Role of Mesenchymal Stem Cells in Bone Regeneration and Fracture Repair: A Review. Int. Orthop. 37 (12), 2491–2498. doi: 10.1007/s00264-013-2059-2
Williams, A., Reljic, R., Naylor, I., Clark, S. O., Falero-Diaz, G., Singh, M., et al. (2004). Passive Protection With Immunoglobulin A Antibodies Against Tuberculous Early Infection of the Lungs. Immunology 111 (3), 328–333. doi: 10.1111/j.1365-2567.2004.01809.x
Wu, H., Luo, D., Li, C., Zhang, H., Shunxian, A., Zhang, Y., et al. (2018). Chicoric Acid Improves Heart and Blood Responses to Hypobaric Hypoxia in Tibetan Yaks. Am. J. Chin. Med. 46 (2), 339–355. doi: 10.1142/s0192415x18500179
Xu, P., Xu, J.-C., Chen, X.-N., Ye, Z.-J., Wu, M.-Y. (2015). Autologous Cytokine-Induced Killer (CIK) Immunotherapy in a Case of Disseminated Tuberculosis. Sarcoidosis Vasc. Diffuse Lung Dis. 32 (1), 83–86.
Yamasaki, K., Gallo, R. L. (2008). Antimicrobial Peptides in Human Skin Disease. Eur. J. Dermatol. 18 (1), 11–21. doi: 10.1684/ejd.2008.0304
Yan, S., Liu, R., Mao, M., Liu, Z., Zhang, W., Zhang, Y., et al. (2019). Therapeutic Effect of Bacillus Calmette-Guerin Polysaccharide Nucleic Acid on Mast Cell at the Transcriptional Level. PeerJ 7, e7404. doi: 10.7717/peerj.7404
Yan, L., Xiao-Ling, S., Zheng-Yan, C., Guo-Ping, L., Sen, Z., Zhuang, C. (2013). HSP70/CD80 DNA Vaccine Inhibits Airway Remodeling by Regulating the Transcription Factors T-Bet and GATA-3 in a Murine Model of Chronic Asthma. Arch. Med. Sci. 9 (5), 906–915. doi: 10.5114/aoms.2013.33180
Yuan, X.-L., Wen, Q., Ni, M.-D., Wang, L.-K. (2016). Immune Formulation-Assisted Conventional Therapy on Anti-Infective Effectiveness of Multidrug-Resistant Mycobacterium Tuberculosis Infection Mice. Asian Pac. J. Trop. Med. 9 (3), 293–297. doi: 10.1016/j.apjtm.2016.01.031
Yuk, J.-M., Shin, D.-M., Lee, H.-M., Yang, C.-S., Jin, H. S., Kim, K.-K., et al. (2009). Vitamin D3 Induces Autophagy in Human Monocytes/Macrophages via Cathelicidin. Cell Host Microbe 6 (3), 231–243. doi: 10.1016/j.chom.2009.08.004
Zhang, Y., Liu, J., Wang, Y., Xian, Q., Shao, L., Yang, Z., et al. (2012). Immunotherapy Using IL-2 and GM-CSF is a Potential Treatment for Multidrug-Resistant Mycobacterium Tuberculosis. Sci. China Life Sci. 55 (9), 800–806. doi: 10.1007/s11427-012-4368-x
Keywords: immunotherapy, tuberculosis, immunoactive substances, tuberculosis therapeutic vaccines, chemical agents, cellular therapy
Citation: Mi J, Liang Y, Liang J, Gong W, Wang S, Zhang J, Li Z and Wu X (2021) The Research Progress in Immunotherapy of Tuberculosis. Front. Cell. Infect. Microbiol. 11:763591. doi: 10.3389/fcimb.2021.763591
Received: 24 August 2021; Accepted: 27 October 2021;
Published: 15 November 2021.
Edited by:
Thomas Rudel, Julius Maximilian University of Würzburg, GermanyReviewed by:
Jianping Xie, Southwest University, ChinaMarta Alonso-Hearn, NEIKER-Instituto Vasco de Investigación y Desarrollo Agrario, Spain
Copyright © 2021 Mi, Liang, Liang, Gong, Wang, Zhang, Li and Wu. This is an open-access article distributed under the terms of the Creative Commons Attribution License (CC BY). The use, distribution or reproduction in other forums is permitted, provided the original author(s) and the copyright owner(s) are credited and that the original publication in this journal is cited, in accordance with accepted academic practice. No use, distribution or reproduction is permitted which does not comply with these terms.
*Correspondence: Xueqiong Wu, eHVlcWlvbmd3dUAxMzkuY29t
†These authors have contributed equally to this work