Shedding Light on Autophagy During Human Tuberculosis. A Long Way to Go
- 1Centre d’Immunologie de Marseille-Luminy, Aix Marseille Université, INSERM, CNRS, Marseille, France
- 2Departamento de Química Biológica, Facultad de Ciencias Exactas y Naturales, Universidad de Buenos Aires, Buenos Aires, Argentina
- 3Instituto de Química Biológica de la Facultad de Ciencias Exactas y Naturales (IQUIBICEN), Facultad de Ciencias Exactas y Naturales, Universidad de Buenos Aires, Consejo Nacional de Investigaciones Científicas y Técnicas, Buenos Aires, Argentina
Immunity against Mycobacterium tuberculosis (Mtb) is highly complex, and the outcome of the infection depends on the role of several immune mediators with particular temporal dynamics on the host microenvironment. Autophagy is a central homeostatic mechanism that plays a role on immunity against intracellular pathogens, including Mtb. Enhanced autophagy in macrophages mediates elimination of intracellular Mtb through lytic and antimicrobial properties only found in autolysosomes. Additionally, it has been demonstrated that standard anti-tuberculosis chemotherapy depends on host autophagy to coordinate successful antimicrobial responses to mycobacteria. Notably, autophagy constitutes an anti-inflammatory mechanism that protects against endomembrane damage triggered by several endogenous components or infectious agents and precludes excessive inflammation. It has also been reported that autophagy can be modulated by cytokines and other immunological signals. Most of the studies on autophagy as a defense mechanism against Mycobacterium have been performed using murine models or human cell lines. However, very limited information exists about the autophagic response in cells from tuberculosis patients. Herein, we review studies that face the autophagy process in tuberculosis patients as a component of the immune response of the human host against an intracellular microorganism such as Mtb. Interestingly, these findings might contribute to recognize new targets for the development of novel therapeutic tools to combat Mtb. Actually, either as a potential successful vaccine or a complementary immunotherapy, efforts are needed to further elucidate the role of autophagy during the immune response of the human host, which will allow to achieve protective and therapeutic benefits in human tuberculosis.
Introduction
Mycobacterium tuberculosis (Mtb) has killed nearly 1000 million people since the XIX century. And although an affordable and effective treatment is available to fight this pathogen, tuberculosis (TB), together with COVID19 in 2020-2021, is the leading cause of death from a single infectious agent. Therefore, improvement of treatment is included among the central aims of developing new strategies against this disease. Accordingly, it has been proposed that supplementing anti-TB therapy with host response modulators will augment standard TB treatment (Madhur et al., 2016). However, the immune response against Mtb is highly complex. The outcome of TB infection depends, at least in part, on several immune mediators that display critical temporal roles on the host microenvironment (Sodhi et al., 1997; Ottenhoff et al., 1998; Chen et al., 2008; Pasquinelli et al., 2009; Cooper, 2010; Jurado et al., 2012; Mayer-Barber et al., 2014; Pellegrini et al., 2021). It has been suggested that host-directed therapies (HDT) could be untapped strategies as complementary therapies against TB, augmenting the host defences and/or limiting tissue damage associated with infection (Martínez-Colón and Moore, 2018; Wan et al., 2018; Xiong et al., 2018). In this context, autophagy arises as an attractive therapeutic target, but currently available data on autophagy in TB patients and the potential clinical use of this cellular process remain insufficient. Here, we review the current knowledge of autophagy as a potential complement of anti-TB chemotherapy.
Autophagy
Autophagy is an evolutionarily-conserved cellular process that mediates the lysosomal degradation of cytoplasmic components and damaged organelles, allowing eukaryotic cells to generate nutrients under starvation conditions and maintain cellular homeostasis. Three types of autophagy have been described: chaperone-mediated autophagy, microautophagy, and macroautophagy, herein referred to as autophagy (Jacomin et al., 2018). Importantly, autophagy participates in innate and adaptive immunity against intracellular pathogens, including Mtb (Gutierrez et al., 2004). Actually, increased autophagy in macrophages eliminates intracellular Mtb via lytic and antimicrobial mechanisms of the autolysosomes (Ponpuak et al., 2010). Notably, autophagy constitutes an anti-inflammatory mechanism that protects against endomembrane damage triggered by several endogenous components or infectious agents and precludes excessive inflammation (Castillo et al., 2012; Deretic and Levine, 2018). The autophagy process can be modulated by different immunological mediators (Djavaheri-Mergny et al., 2006). In particular, critical cytokines regulate both positively and negatively the autophagic response affecting survival of mycobacteria (Harris et al., 2007). Besides, the importance of the host autophagy process to manage an effective antimicrobial effect on mycobacteria during chemotherapy has been reported (Kim et al., 2012). A better understanding of the connections between autophagy and the immune response may have wide applications given that the pathology accompanying several diseases involves some form of inflammation (Deretic and Levine, 2018).
Autophagy in TB Patients
Most of the studies that investigated autophagy as a defense mechanism against Mtb have been accomplished in murine cell lines, mouse models, primary culture cells, or human cell lines infected with the pathogen. However, very limited information regarding the study of the autophagic response in TB patients is available. During the past decade, we have studied Mtb-induced autophagy in two populations of patients with active disease, classified according to their T cell responses to the bacterium. Briefly, high responder (HR) TB patients displayed significant T cell proliferation and IFN-γ production against Mtb-antigen (Ag), while low responder (LR) TB patients displayed weak or no T cell responses to the antigen (Pasquinelli et al., 2004). Interestingly, we detected the highest autophagy levels in healthy donor (HD)´s monocytes whereas the lowest quantities were observed in monocytes from LR patients (Rovetta et al., 2014). Accordingly, it has been reported that Beclin-1, a signaling hub of autophagy, is increased in alveolar macrophages from TB patients and that those individuals with higher Beclin-1 levels achieve faster bacillary sterilization (Yu et al., 2016). Recently, we observed that autophagy levels decreased significantly in neutrophils from TB patients as compared to HD (Pellegrini et al., 2020). Moreover, a direct correlation between neutrophil numbers and TB severity was detected (Pellegrini et al., 2020). Given that during Mtb infection autophagy protects against massive inflammation (Deretic and Levine, 2018), the reduced levels of autophagy observed in neutrophils from TB patients could be related to the frequent harmful inflammatory responses that take place during active disease.
Effect of the Diversity of Mtb Strains on the Autophagy Process
The immune response to Mtb is influenced by factors both from the host and the bacteria (Sousa et al., 2020). Accordingly, some studies have demonstrated a differential ability of different Mtb strains to modulate autophagy. In particular, Li et al. described that clinical isolates from Mtb differ in their ability to induce autophagosome formation (Li et al., 2016). The authors investigated the effect of more than 180 Mtb clinical isolates on the autophagy process in THP-1 macrophages. Interestingly, they observed that the capacity of inducing autophagy varied significantly among different isolates. Notably, patients infected with Mtb strains that displayed reduced autophagy-inducing ability showed more severe disease and displayed adverse treatment outcomes, suggesting that an autophagy deficiency induced by Mtb isolates augmented the risk of poor clinical outcomes in TB patients (Li et al., 2016).
The majority of the studies on the host immune response to Mtb infection have been performed using the laboratory strain H37Rv (see Tables 1–3). Moreover, most of the research performed with samples from TB patients does not consider the original Mtb strain that infected the host. Thus, investigation of the effect of Mtb genetic variability on the modulation of the autophagy process is of great interest.
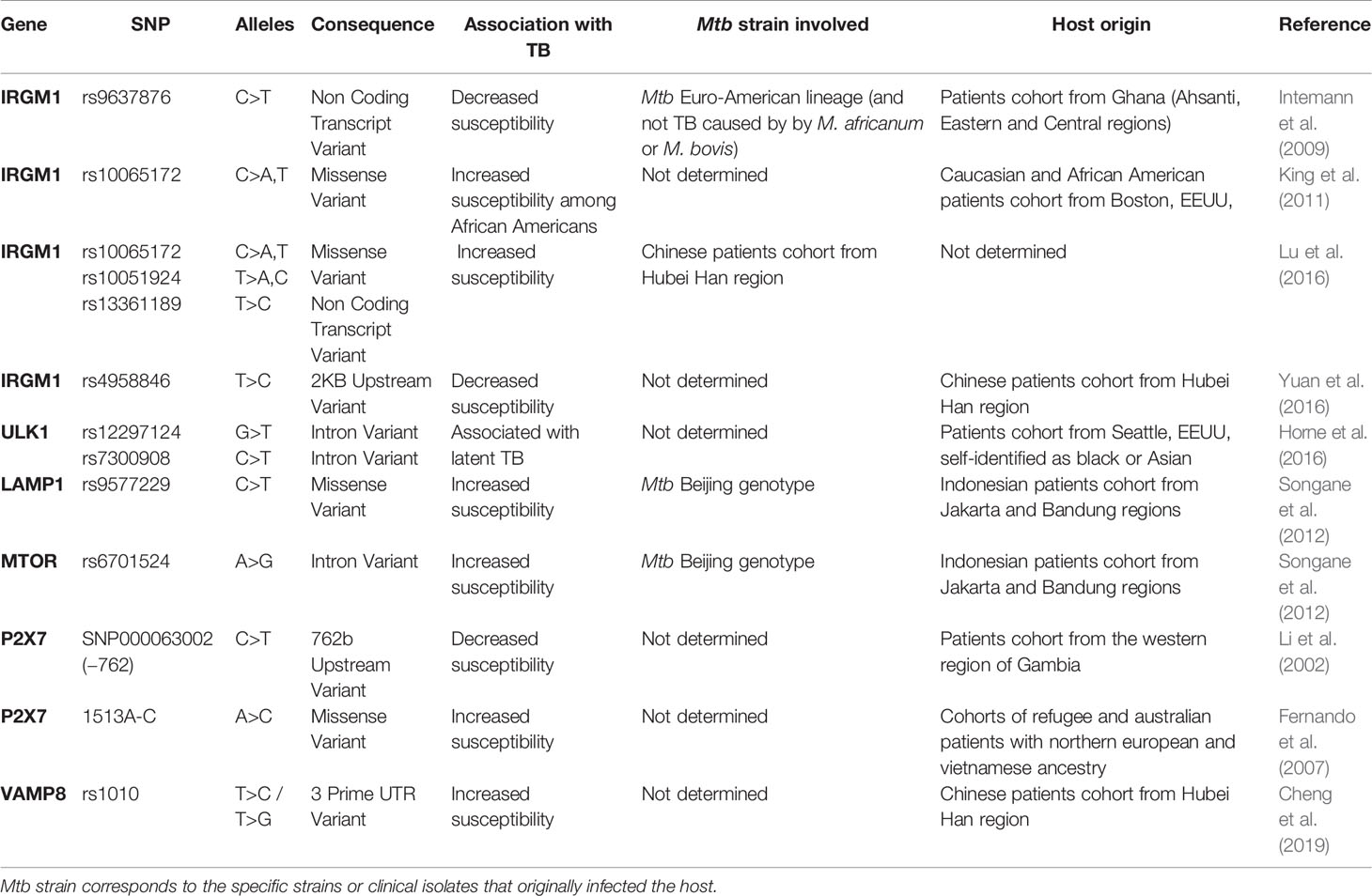
Table 3 SNPs in autophagy-related genes associated with TB. List of SNPs in genes codifying for proteins involved in autophagy that have been found to be associated with increasing or decreasing susceptibility to active TB.
Genome sequence analysis has identified seven phylogeographic Mtb lineages: four referred to as evolutionarily “ancient” and three as “modern”. Interestingly, the “modern” strains were shown to display high virulence (Romagnoli et al., 2018). Therefore, Romagnoli et al. investigated the impact of the genetic diversity of Mtb strains on the host innate immune response by evaluating the autophagy response. Remarkably, the authors demonstrated that modern Mtb strains are able to avoid the autophagy machinery affecting the regulation of specific T-cell responses.
Together, the studies described above might suggest a possible limitation of using autophagy as a novel therapy against Mtb. However, on the other side, it was proposed that analyses of autophagosome formation by diverse clinical isolates of Mtb might contribute to the evaluation of TB outcomes (Li et al., 2016). Furthermore, the study of the genetic variability of Mtb on autophagy modulation was proposed to have translational implications for the design of HDT, which should consider both the autophagic and immunogenic properties of the lineage of the Mtb candidate. Accordingly, by studying 681 TB patients Sousa et al. showed that Mtb isolates from cases with mild disease stimulate strong cytokine responses in contrast to bacteria from patients with severe TB, indicating that Mtb strains manipulate host-pathogen interactions to drive variable TB severities. Then, they suggest to include Mtb genetic diversity in the development of HDT (Sousa et al., 2020). Finally, external autophagy modulators might act as adjuvants in Mtb treatment helping to overcome autophagy regulation/inhibition by pathogenic strains.
Immunological Mediators
Autophagy is a process recognized to be regulated by cytokines and other immunological signals (Djavaheri-Mergny et al., 2006; Harris et al., 2007; Goletti et al., 2013; Chen H. et al., 2015; Pelissier-Rota et al., 2015; Martínez-Colón and Moore, 2018; Wan et al., 2018). TNF was originally shown to induce autophagy in Ewing sarcoma cells (Djavaheri-Mergny et al., 2006). Recently, TNF was demonstrated to promote the autophagy of Mtb-infected osteoclasts and constrain the apoptosis of mature osteoclasts (Liu W. et al., 2020). Furthermore, Liu et al. suggest that their data describe a novel osteoarticular TB-activated cytokine network where autophagy could have an important role in the pathogenesis of osteoarticular TB, pointing out the use of drugs such as TNF for treating this type of TB (Liu W. et al., 2020). Moreover, IFN-γ augments the autophagy process in macrophages and other cells (Gutierrez et al., 2004; Goletti et al., 2013), whereas IL-4, IL-10 and IL-13 inhibited autophagy in murine macrophages and human cell lines (Harris et al., 2007; Park et al., 2011). Accordingly, it has been demonstrated that autophagy participates in the immune response of TB patients against Mtb, in direct association with the specific IFN-γ levels secreted against the pathogen (Rovetta et al., 2014). By blocking Mtb-Ag-induced IFN-γ, a marked reduction of autophagy was measured in monocytes from HR patients. In contrast, the incorporation of small quantities of IFN-γ significantly augmented autophagy in LR patients (Rovetta et al., 2014). We also demonstrated that IL-17A increased autophagy in infected monocytes from HR patients (Tateosian et al., 2017). However, in severe LR TB patients’ monocytes, a defect in the ERK1/2 signaling pathway prevented an augment in autophagy caused by IL-17A. Both IFN-γ and IL-17A increased the levels of autophagy in HR patients, promoting mycobacterial killing (Tateosian et al., 2017). Besides, Dang et al. demonstrated that addition of IL-26 to human M. Leprae infected monocytes induced autophagy (Dang et al., 2019). Furthermore, LC3-positive autophagosomes were mainly detected in lesions from T-lep (tuberculoid) as compared with L-lep (lepromatous) patients, indicating that M. Leprae dampened autophagy in human cells as an immune escape mechanism (Silva et al., 2017). It has been reported that type I glycoproteins such as SLAMF1 recruit molecules like Beclin-1 to the phagosome, participating in the connection to the cellular machinery that controls bacterial killing (Berger et al., 2010; Ma et al., 2012). Accordingly, we recently demonstrated that human neutrophils express SLAMF1 upon Mtb-stimulation, a protein that colocalized with LC3B+ vesicles (Pellegrini et al., 2020). Furthermore, SLAMF1 activation augmented neutrophil autophagy induced by Mtb, and neutrophils from TB patients showed reduced levels of SLAMF1 and lower amounts of autophagy against Mtb as compared to HD (Pellegrini et al., 2020). The eicosanoids, a family of potent biologically active lipid mediators, modulate immune responses in Mtb infection and have been suggested as potential HDT targets. Actually, manipulation of PGE2 and/or 5-LO was suggested to potentially counteract the type I IFN response in patients with severe TB as a HDT against Mtb (Mayer-Barber et al., 2014). Recently, we reported that PGE2 promotes autophagy in monocytes and neutrophils cultured with Mtb. We demonstrated that PGE2 augmented the percentage of LC3+ neutrophils and monocytes upon Mtb-Ag stimulation. Furthermore, the exogenous addition of this eicosanoid triggered a functional autophagy flux both in monocytes and lymphocytes from TB patients (Pellegrini et al., 2021). Thus, according to our results, PGE2 might be a new target for the development of novel therapeutic tools to fight Mtb. Table 1 summarizes the data mentioned in this section.
Non-Coding RNAs in Autophagy Modulation During Human Tuberculosis
In recent years, there was a growing body of evidence suggesting a critical role of non-coding RNAs (ncRNAs) in regulating host-pathogen interactions and immunity. A variety of pathogens, including Mtb, have been described to modulate the expression of these modulators by evading host responses and influencing the outcome of the infection (Staedel and Darfeuille, 2013; Zhang et al., 2019). Actually, some authors have proposed that miRNAs/lncRNAs regulation is an important strategy employed by Mtb to survive inside host cells (Kundu and Basu, 2021). Mycobacteria can alter the host miRNA expression profile for their benefit, affecting antimicrobial responses, cytokine production, metabolism and inflammation, among other processes (Yang and Ge, 2018). Moreover, the differential miRNA and lncRNA profiles detected in clinical samples from TB patients have led to an increasing interest in their use as TB biomarkers (Sabir et al., 2018). Importantly, some of these TB-associated ncRNAs play a role in the regulation of autophagy during Mtb infection, although most of these studies have been performed in murine models or cell lines.
Few reports have explored the role of these intermediaries in autophagy by using primary human cells from TB patients (Table 2). Accordingly, by analyzing GSE 29190 and GSE34608 miRNA microarray datasets Kim et al. detected that only 10 miRNA were differentially expressed in peripheral blood mononuclear cells (PBMCs) and biopsies from lungs and lymph nodes from TB patients, for example, miR-144* (Kim et al., 2017). Importantly, the authors demonstrated that miR-144* targets DRAM2 (an interactor of Beclin 1 and UVRAG) in human monocytes/macrophages, thus affecting autophagosome formation (Kim et al., 2017). Consequently, DRAM2 levels were decreased in monocytes from TB patients as compared to HD (Liu G. et al., 2020).
Furthermore, some studies have used primary cells obtained directly from the site of infection. Accordingly, Chen et al. demonstrated that miR-30A suppresses the elimination of intracellular Mtb by inhibiting autophagy. In fact, a higher concentration of miR-30A in alveolar macrophages from bronchoalveolar lavage (BAL) of smear-positive patients were detected in comparison with smear-negative patients and HD. Moreover, the expression of this miRNA decreased upon anti-TB treatment (Chen Z. et al., 2015). Besides, circAGFG1 was found to upregulate autophagy in Mtb–infected alveolar macrophages by targeting miRNA-1257, which in turn suppresses Notch signaling pathway (Shi et al., 2020).
Notably, Li et al. observed that PCED1B-AS1, a 410-bp lncRNA, is down-regulated in TB patients, which is accompanied by increased autophagy (Li et al., 2019). This function is carried out through binding with miR-155 to control its expression. This observation is concordant with a previous work demonstrating that Mtb can manipulate cellular miR-155 expression to regulate Atg3 levels, decreasing autophagosome formation in human dendritic cells (Etna et al., 2018). Finally, one study has explored the role of miR-889 and autophagy to maintain latent TB status (Chen et al., 2020). Chen et al. observed an increased miR-899 expression in latent TB individuals as compared to HD, which was significantly restored after anti-TB therapy. The authors identified the cytokine TWEAK as the target of miR-899, which inhibits autophagy and maintains mycobacterial survival in a human TB granuloma model (Chen et al., 2020). In summary, the increasing evidence found in murine models and cell lines demonstrates that some miRNAs/lncRNAs directly participate in the host response to Mtb by modulating autophagy. These studies were partially confirmed in human studies as we summarized here. Then, ncRNA-based therapeutics appear as an attractive target to directly modulate autophagy as novel HDT. However, an efficient drug design including ncRNA should be protected from degradation and successful delivery to the site of infection has to be ensured (Singh et al., 2021).
Genetic Association Studies in Autophagy-Related Genes
Host and environmental factors have been shown to play a role in the pathogenesis and development of TB. For thousands of years, Mtb has co-evolved with humans, suggesting a powerful evolutionary pressure between the host and pathogen genomes, and therefore a strong impact of genetic factors on the development of different TB stages (Campbell and Tishkoff, 2008; Comas et al., 2013; Galagan, 2014). One particularly powerful approach to assess the role of some processes in humans is to investigate whether genetic variation influences susceptibility to infection. Single Nucleotide Polymorphisms (SNPs) are believed to be the true source of variability among humans (Pacheco and Moraes, 2009; Singh et al., 2017) and it is expected that the variants in genes involved in the pathogen-host interaction are influencing resistance/susceptibility to the disease.
Some reports have investigated SNPs in genes involved in the autophagy process, leading to important evidence about its role during human TB. For instance, IRGM1, a GTPase effector protein that plays an essential role in autophagy induction, has been studied in several populations. Rs9637876 IRGM1 SNP was associated with decreased susceptibility to TB caused only by Mtb Euro-American lineage in Ghana (Intemann et al., 2009). Moreover, the rs10065172 SNP within its coding region was associated with susceptibility to TB among African-Americans and in a Chinese population (King et al., 2011; Lu et al., 2016). Interestingly, this polymorphism was previously associated with mortality of patients with severe sepsis (Kimura et al., 2014). Furthermore, Yuan et al. identified three polymorphisms in the IRGM1 promoter region and found that CT genotype of rs4958846 decreased the risk of pulmonary TB in comparison with TT genotype (Yuan et al., 2016). In addition, Horne et al. selected ULK1 and GABARAP as candidate genes since they play fundamental roles in autophagy initiation and autophagosome maturation, respectively. Thus, they identified 2 SNPs in ULK1 (rs12297124 and rs7300908) in Asian participants that were significantly associated with latent TB. Moreover, ULK1-deficient cells had increased Mtb replication, decreased TNF response to stimulation, and impaired autophagy. Intriguingly, a previous work had investigated 22 polymorphisms of 14 autophagy genes in an Indonesian population. The authors found associations between SNPs in LAMP1 and MTOR genes and infection with Mtb Beijing genotype, but all those associations lost statistical significance after correction for multiple testing (Songane et al., 2012). Similarly, no associations were found in ATG5 (rs2245214, c.574-12777G>C) and NOD2 (rs2066844, c.2104C>T) genes in Romania (Cucu et al., 2017). Besides, 2 SNPs in the P2X7 gene coding for a plasma receptor that mediates ATP-induced autophagy, both in the promoter (Li et al., 2002) and the coding region (Fernando et al., 2007) were found to be associated with protection against TB. Finally, Cheng et al. found that rs1010 SNP in the VAMP8 gene is significantly associated with pulmonary TB in a Chinese Han population (Cheng et al., 2019). More comprehensive studies are required to evaluate the contribution of autophagy in different contexts because these studies are influenced by ethnicity, infection strains, polygenicity, among others. Table 3 resumes the results cited in this section.
Autophagy Activating Compounds for Human Host Directed Therapy in Tuberculosis
Autophagy modulation may signify a promising HDT strategy to fight human TB (Yuk et al., 2009). However, the clinical knowledge about HDT implementation is still widely deficient. HDT compounds combined with current TB drugs could shorten and improve treatments against Mtb infection. Therefore, autophagy activation by newborn drugs, soluble mediators or agents administered alone or in combination with anti-TB antibiotics still requires long-term clinical trials. Nevertheless, preclinical studies revealed that repurposing licensed drugs with a demonstrated potential to induce autophagy showed an effective therapeutic manipulation of host immunity against Mtb infection. These drugs displaying safe and pharmacokinetic profiles are promising for the evaluation of their effectiveness in randomized and controlled clinical trials. Accordingly, several clinical trials (clinicaltrilas.gov) have been conducted implementing dietary supplementation of the immunomodulator Vitamin D3. Innate immunity mediated by Vitamin D3 conferred protection against infection with Mtb (Yuk et al., 2009). Interestingly, Vitamin D3 and autophagy are physiologically linked via human cathelicidin (hCAP-18/LL-37), which activates transcription of autophagy-related genes such as Beclin-1 and Atg5 (Yuk et al., 2009). In the last ten years, numerous trials were performed supplementing Vitamin D3 as an adjunctive therapy. Nevertheless, differences in these trial outcomes have hampered the interpretation about Vitamin D3 efficacy as HDT for TB. Moreover, the impact of Vitamin D3 as an adjunctive therapy displayed no effect on culture conversion and sputum smear negativization (Ralph et al., 2013; Daley et al., 2015; Tukvadze et al., 2015; Ganmaa et al., 2017; Wu et al., 2018). However, genetic variation in the Vitamin D receptor gene was suggested to modify the effects of adjunctive Vitamin D3 in TB patients (Jolliffe et al., 2016). Additionally, multiple randomized trials suggested that adjunctive Vitamin D treatment has limited effect in improving clinical and immunologic outcomes during active Mtb infection despite evidence that specific VDR polymorphisms are predictive of sputum conversion time (Xia et al., 2014; Grobler et al., 2016; Zittermann et al., 2016). A phase 2 clinical study in TB patients (NCT02968927) assess the anti-inflammatory effects of Vitamin D3 in combination with 3 other adjunctive HDT compounds: CC-11050, Everolimus and Auranofin (Wallis et al., 2021). The CC-11050 is a type 4 phosphodiesterase inhibitor that displays anti-inflammatory properties (Subbian et al., 2016a; Subbian et al., 2016b); Everolimus, a serine/threonine-protein kinase mTOR inhibitor, is an autophagy inducer (Cerni et al., 2019) and Auranofin is an anti-inflammatory gold salt with antimicrobial activity against Mtb (Harbut et al., 2015). The preliminary results confirmed that CC-11050 and Everolimus are safe and well tolerated indicating a potential benefit to current TB treatment (Wallis et al., 2021). In other studies, Metformin, the AMPK-activating antidiabetic drug, was shown to inhibit the intracellular replication of Mtb, restrict disease immunopathology and enhance conventional anti-TB drug efficacy (Singhal et al., 2014). Moreover, in a pre-clinical study metformin administration in combination with either isoniazid (INH) or ethionamide (ETH) was reported to decrease Mtb load in lungs of infected mice (Singhal et al., 2014). Besides, the combined therapy including metformin with standard TB antibiotics was associated with beneficial consequences on clinical outcomes in TB (Singhal et al., 2014). Furthermore, an ongoing randomized clinical trial (NCT-CTRI/2018/01/011176) is evaluating the safety and efficacy of metformin as an adjunct used with rifampicin (RIF), INH, ETO and pyrazinamide (PZA) in patients with pulmonary TB (Padmapriyadarsini et al., 2019). Moreover, a new clinical trial in TB/HIV co-infected patients (Phase II A randomized, open-label trial, NCT04930744) is analyzing the effect of metformin with standard anti-TB drugs plus anti-retroviral therapy (Sullivan and Ben Amor, 2012; Marupuru et al., 2017).
Besides, statins (cholesterol-lowering drugs that inhibit β-hydroxy β-methylglutaryl-CoA (HMG-CoA) reductase) reduce the risk of coronary disorders and hypercholesterolemia. However, statins can also influence immunologic responses (Parihar et al., 2014). In pre-clinical models, statins such as Simvastatin, Rosuvastatin and Atorvastatin decreased Mtb load by enhancing autophagy, phagosomal maturation, and reducing pulmonary pathology, which suggests a potential role for statins as HDT in TB (Lobato et al., 2014; Parihar et al., 2014). Consequently, statins are among the most promising HDT agents for TB. The purpose of the numerous clinical trials that are currently undergoing is to assess the security, tolerance and pharmacokinetics of Pravastatin (NCT03882177) or Atorvastatin (NCT04147286) as adjunctive therapy when combined with standard TB drugs in adults infected with Mtb. There is still a long way to go by investigating many other repurposing licensed drugs with the ability to induce autophagy. For example, the mucokinetic Ambroxol (Choi et al., 2018), the antidiarrheal drug Loperamide (Lee, 2015; Juárez et al., 2016), the anti-protozoal drug Nitazoxanide (Lam et al., 2012), the anti-seizure drug Carbamazepine and Valproic acid (Schiebler et al., 2015), psychotropic or antidepressant drugs such as Nortriptyline, Fluoxetine and Prochlorperazine edisylate and Fluoxetine (Sundaramurthy et al., 2013; Stanley et al., 2014) are some of the drugs with potential use as HDT for TB treatment.
Manipulating Autophagy to Improve Vaccination Against TB
The role of autophagy as a defense mechanism allows to hypothesize that vaccines that increase the autophagic response might be more effective in preventing the reactivation of latency or the acquisition of active TB. In fact, autophagy could be key in the development of effective TB vaccines since this process has the potential to improve the host immune response against Mtb. The attenuated Mycobacterium bovis Bacillus Calmette-Guérin (BCG) is effective in protecting against pulmonary and extrapulmonary TB in children up to 10 years old (Sterne et al., 1998; Abubakar et al., 2013), but protection against the pulmonary form of TB in adults remains highly controversial (Hatherill et al., 2020). BCG is able to affect the activation of T cells by evading phagosome maturation, autophagy, and by reducing MHC-II expression of antigen-presenting cells (APCs) (Russell, 2001). To avoid these deficiencies, an autophagy-inducing, TLR-2 activating C5 peptide from Mtb-derived CFP-10 protein was overexpressed in BCG in combination with Ag85B. This recombinant BCG was shown to induce stronger and longer-lasting immunity, increasing protection in a TB murine model (Khan et al., 2019). Furthermore, overexpression of Ag85B in BCG induced autophagy in APCs and increased immunogenicity in mice, indicating that vaccine efficacy can be augmented by enhancing autophagy-mediated antigen presentation (Jagannath et al., 2009). Therefore, exacerbation of autophagy could contribute to increase the immune response conferred by BCG. Interestingly, BCG was also genetically modified to improve its immunogenicity by replacing the urease C encoding gene with the listeriolysin encoding gene from Listeria monocytogenes. As a result, BCGΔureC::hly (VPM1002) was demonstrated to promote apoptosis and autophagy and facilitate the release of mycobacterial antigens into the cytosol (Nieuwenhuizen et al., 2017). The use of VPM1002 vaccine in preclinical trials has been shown to be more effective and safer than BCG (Nieuwenhuizen et al., 2017).
Perspectives
For most countries, the end of TB as an epidemic disease and a public health problem still remains an aspiration rather than a reality. Current treatments still depend on antibiotic therapy and, considering the increasing antibiotic resistance, additional therapeutic targets are becoming progressively essential. To this end, the modulation of the autophagy process arises as an attractive goal. However, a deeper study of the cellular mechanisms that operate in humans are required, especially in TB patients where the infective status of each subject might have a special impact on autophagy modulation. As described here, at present the information regarding the human autophagic response during Mtb infection is very limited and precludes a better understanding of the process. In fact, the patients’ genetic background, among other factors, could be determinant in the development of their specific response to Mtb infection, influencing the effectiveness of a particular treatment. Furthermore, most of the existing studies are focused on autophagy in macrophages as the main target for Mtb, but this process is implicated in a wide variety of cell types. For example, autophagy has been shown to be critical during T cell activation and differentiation, central processes in TB immunity. Thus, an expanded analysis over the autophagy process in other cells such as lymphocytes, dendritic cells, neutrophils, basophils, among others would be necessary. Moreover, a broad examination of the immune responses of TB patients following an autophagy-modulating treatment would be extremely informative.
Based on the mentioned findings reported by several authors and our studies, we proposed a schematic summary of the potential role of autophagy in TB patients according to their immunological response to Mtb (Figure 1). Briefly, immunological mediators such as cytokines, lipid mediators or ncRNAs, influence autophagy in TB patients with different immunological response to the bacteria. Implementing novel HDT strategies such as the modulation of autophagy as adjuvant therapy or novel vaccines might improve the treatment of TB patients. Current and future studies on autophagy-based therapeutic candidates may contribute to possible therapeutic/prevention improvements against TB, directly impacting the lives of millions of individuals infected with Mtb.
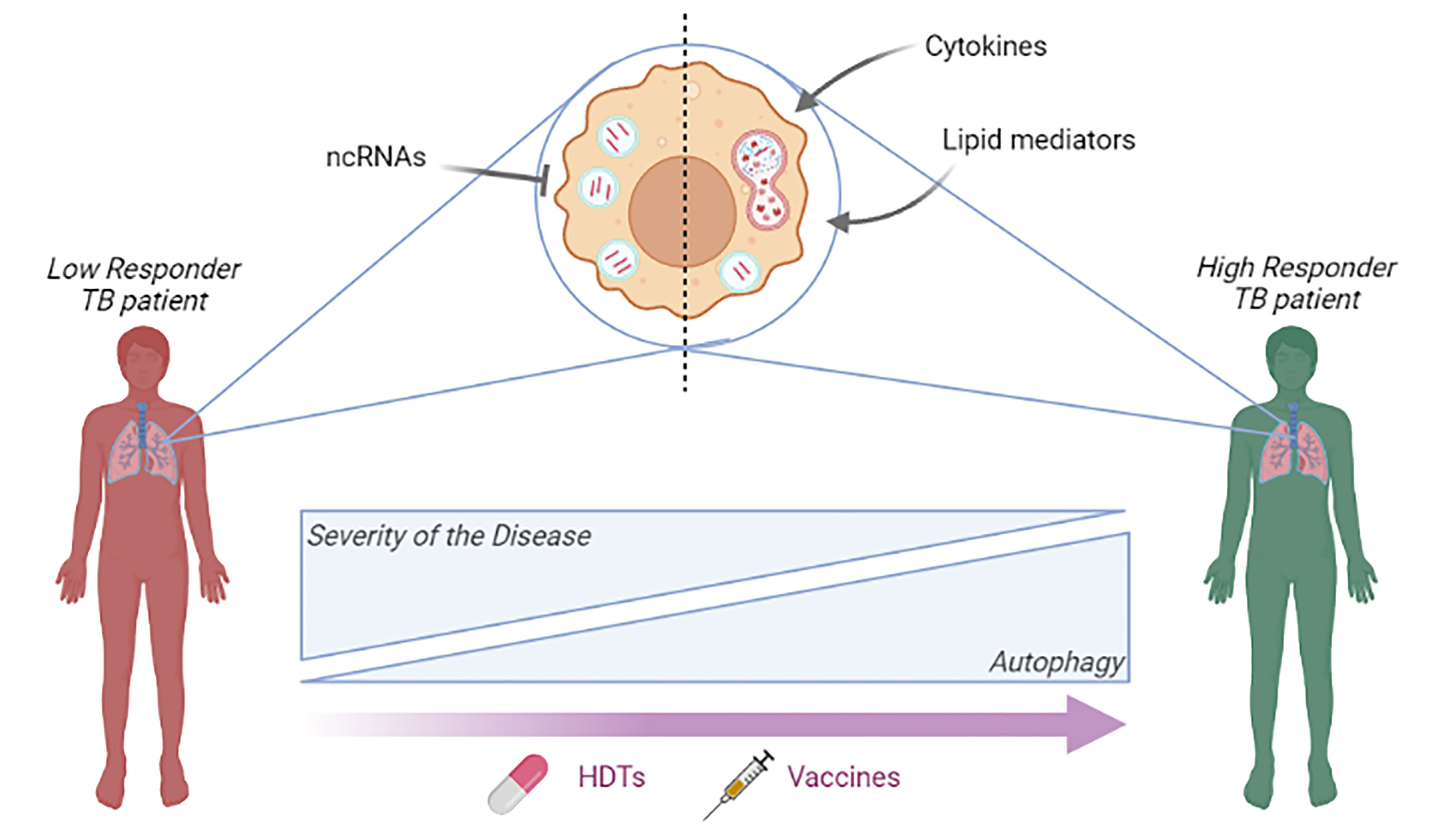
Figure 1 A simplified model of the role of autophagy in TB patients according to their immunological response to Mtb. The potential influence of immunological mediators and ncRNAs on autophagy is shown. High responder TB patients correspond to patients that display strong T cell immunity to Mtb, which correlates with milder manifestations of the disease (Pasquinelli et al., 2004; Jurado et al., 2012) and robust autophagic responses, as previously demonstrated (Rovetta et al., 2014; Tateosian et al., 2017). In contrast, patients with weak or no T cell responses to Mtb are associated with more severe disease (Pasquinelli et al., 2004; Jurado et al., 2012) and diminished autophagy (Rovetta et al., 2014; Tateosian et al., 2017). The purple arrow indicates the hypothetical impact of new HDT strategies (e.g.: autophagy as adjuvant therapy) in the treatment of TB patients and novel vaccines inducing an autophagic response. Figure created with BioRender.com..
Author Contributions
The writing-original draft preparation as well as the writing-review, and editing of the manuscript was in charge of JP, NT, MM, and VG. JP and VG were responsible for the conceptualization and organization of the present work. VG was in charge of supervision and project administration. All authors have read and agreed to the final version of the manuscript.
Funding
This work was supported by grants from Agencia Nacional de Promoción Científica y Tecnológica (ANPCyT) [PICT-2017-1451 and PICT-2019-01617 to VG] and Universidad de Buenos Aires (UBACyT) [20020170100127BA to VG].
Conflict of Interest
The authors declare that the research was conducted in the absence of any commercial or financial relationships that could be construed as a potential conflict of interest.
Publisher’s Note
All claims expressed in this article are solely those of the authors and do not necessarily represent those of their affiliated organizations, or those of the publisher, the editors and the reviewers. Any product that may be evaluated in this article, or claim that may be made by its manufacturer, is not guaranteed or endorsed by the publisher.
Acknowledgments
We are thankful to Dr. Ana Rovetta, Dr. Analía Trevani and Dr. María Isabel Colombo for their contributions.
References
Abubakar, I., Pimpin, L., Ariti, C., Beynon, R., Mangtani, P., Sterne, J., et al. (2013). Systematic Review and Meta-Analysis of the Current Evidence on the Duration of Protection by Bacillus Calmette-Guérin Vaccination Against Tuberculosis. Health Technol. Assess. 17, (37). doi: 10.3310/hta17370
Berger, S. B., Romero, X., Ma, C., Wang, G., Faubion, W. A., Liao, G., et al. (2010). SLAM Is a Microbial Sensor That Regulates Bacterial Phagosome Functions in Macrophages. Nat. Immunol. 11, 920–927. doi: 10.1038/ni.1931
Campbell, M. C., Tishkoff, S. A. (2008). African Genetic Diversity: Implications for Human Demographic History, Modern Human Origins, and Complex Disease Mapping. Annu. Rev. Genomics Hum. Genet. 9, 403–433. doi: 10.1146/annurev.genom.9.081307.164258
Castillo, E. F., Dekonenko, A., Arko-Mensah, J., Mandell, M. A., Dupont, N., Jiang, S., et al. (2012). Autophagy Protects Against Active Tuberculosis by Suppressing Bacterial Burden and Inflammation. Proc. Natl. Acad. Sci. U. S. A. 109, E3168–E3176. doi: 10.1073/pnas.1210500109
Cerni, S., Shafer, D., To, K., Venketaraman, V. (2019). Investigating the Role of Everolimus in mTOR Inhibition and Autophagy Promotion as a Potential Host-Directed Therapeutic Target in Mycobacterium Tuberculosis Infection. J. Clin. Med. 8, E232. doi: 10.3390/jcm8020232
Chen, H., Chen, L., Cheng, B., Jiang, C. (2015). Cyclic Mechanical Stretching Induces Autophagic Cell Death in Tenofibroblasts Through Activation of Prostaglandin E2 Production. Cell. Physiol. Biochem. 36, 24–33. doi: 10.1159/000374050
Chen, D.-Y., Chen, Y.-M., Lin, C.-F., Lo, C.-M., Liu, H.-J., Liao, T.-L. (2020). MicroRNA-889 Inhibits Autophagy To Maintain Mycobacterial Survival in Patients With Latent Tuberculosis Infection by Targeting TWEAK. mBio 11, e03045–e03019. doi: 10.1128/mBio.03045-19
Chen, M., Divangahi, M., Gan, H., Shin, D. S. J., Hong, S., Lee, D. M., et al. (2008). Lipid Mediators in Innate Immunity Against Tuberculosis: Opposing Roles of PGE2 and LXA4 in the Induction of Macrophage Death. J. Exp. Med. 205, 2791–2801. doi: 10.1084/jem.20080767
Cheng, S., Sun, C., Lao, W., Kang, H. (2019). Association of VAMP8 Rs1010 Polymorphism With Host Susceptibility to Pulmonary Tuberculosis in a Chinese Han Population. Genet. Test Mol. Biomarkers 23, 299–303. doi: 10.1089/gtmb.2018.0265
Chen, Z., Wang, T., Liu, Z., Zhang, G., Wang, J., Feng, S., et al. (2015). Inhibition of Autophagy by MiR-30a Induced by Mycobacteria Tuberculosis as a Possible Mechanism of Immune Escape in Human Macrophages. Jpn. J. Infect. Dis. 68, 420–424. doi: 10.7883/yoken.JJID.2014.466
Choi, S. W., Gu, Y., Peters, R. S., Salgame, P., Ellner, J. J., Timmins, G. S., et al. (2018). Ambroxol Induces Autophagy and Potentiates Rifampin Antimycobacterial Activity. Antimicrob. Agents Chemother. 62, e01019–e01018. doi: 10.1128/AAC.01019-18
Comas, I., Coscolla, M., Luo, T., Borrell, S., Holt, K. E., Kato-Maeda, M., et al. (2013). Out-Of-Africa Migration and Neolithic Coexpansion of Mycobacterium Tuberculosis With Modern Humans. Nat. Genet. 45, 1176–1182. doi: 10.1038/ng.2744
Cooper, A. M. (2010). Editorial: Be Careful What You Ask for: Is the Presence of IL-17 Indicative of Immunity? J. Leukocyte Biol. 88, 221–223. doi: 10.1189/jlb.0310146
Cucu, M. G., Streața, I., Riza, A. L., Cimpoeru, A. L., Șerban-Șoșoi, S., Ciocoiu, A., et al. (2017). Polymorphisms in Autophagy Genes and Active Pulmonary Tuberculosis Susceptibility in Romania. Rev. Romana Med. Laborator. 25, 47–53. doi: 10.1515/rrlm-2017-0002
Daley, P., Jagannathan, V., John, K. R., Sarojini, J., Latha, A., Vieth, R., et al. (2015). Adjunctive Vitamin D for Treatment of Active Tuberculosis in India: A Randomised, Double-Blind, Placebo-Controlled Trial. Lancet Infect. Dis. 15, 528–534. doi: 10.1016/S1473-3099(15)70053-8
Dang, A. T., Teles, R. M., Weiss, D. I., Parvatiyar, K., Sarno, E. N., Ochoa, M. T., et al. (2019). IL-26 Contributes to Host Defense Against Intracellular Bacteria. J. Clin. Invest. 129, 1926–1939. doi: 10.1172/JCI99550
Deretic, V., Levine, B. (2018). Autophagy Balances Inflammation in Innate Immunity. Autophagy 14, 243–251. doi: 10.1080/15548627.2017.1402992
Djavaheri-Mergny, M., Amelotti, M., Mathieu, J., Besançon, F., Bauvy, C., Souquère, S., et al. (2006). NF-KappaB Activation Represses Tumor Necrosis Factor-Alpha-Induced Autophagy. J. Biol. Chem. 281, 30373–30382. doi: 10.1074/jbc.M602097200
Etna, M. P., Sinigaglia, A., Grassi, A., Giacomini, E., Romagnoli, A., Pardini, M., et al. (2018). Mycobacterium Tuberculosis-Induced miR-155 Subverts Autophagy by Targeting ATG3 in Human Dendritic Cells. PloS Pathog. 14, e1006790. doi: 10.1371/journal.ppat.1006790
Fernando, S. L., Saunders, B. M., Sluyter, R., Skarratt, K. K., Goldberg, H., Marks, G. B., et al. (2007). A Polymorphism in the P2X7 Gene Increases Susceptibility to Extrapulmonary Tuberculosis. Am. J. Respir. Crit. Care Med. 175, 360–366. doi: 10.1164/rccm.200607-970OC
Galagan, J. E. (2014). Genomic Insights Into Tuberculosis. Nat. Rev. Genet. 15, 307–320. doi: 10.1038/nrg3664
Ganmaa, D., Munkhzul, B., Fawzi, W., Spiegelman, D., Willett, W. C., Bayasgalan, P., et al. (2017). High-Dose Vitamin D3 During Tuberculosis Treatment in Mongolia. A Randomized Controlled Trial. Am. J. Respir. Crit. Care Med. 196, 628–637. doi: 10.1164/rccm.201705-0936OC
Goletti, D., Petruccioli, E., Romagnoli, A., Piacentini, M., Fimia, G. M. (2013). Autophagy in Mycobacterium Tuberculosis Infection: A Passepartout to Flush the Intruder Out? Cytokine Growth Factor Rev. 24, 335–343. doi: 10.1016/j.cytogfr.2013.01.002
Grobler, L., Nagpal, S., Sudarsanam, T. D., Sinclair, D. (2016). Nutritional Supplements for People Being Treated for Active Tuberculosis. Cochrane Database Syst. Rev. 6, CD006086. doi: 10.1002/14651858.CD006086.pub4
Gutierrez, M. G., Master, S. S., Singh, S. B., Taylor, G. A., Colombo, M. I., Deretic, V. (2004). Autophagy Is a Defense Mechanism Inhibiting BCG and Mycobacterium Tuberculosis Survival in Infected Macrophages. Cell 119, 753–766. doi: 10.1016/j.cell.2004.11.038
Harbut, M. B., Vilchèze, C., Luo, X., Hensler, M. E., Guo, H., Yang, B., et al. (2015). Auranofin Exerts Broad-Spectrum Bactericidal Activities by Targeting Thiol-Redox Homeostasis. Proc. Natl. Acad. Sci. U. S. A. 112, 4453–4458. doi: 10.1073/pnas.1504022112
Harris, J., De Haro, S. A., Master, S. S., Keane, J., Roberts, E. A., Delgado, M., et al. (2007). T Helper 2 Cytokines Inhibit Autophagic Control of Intracellular Mycobacterium Tuberculosis. Immunity 27, 505–517. doi: 10.1016/j.immuni.2007.07.022
Hatherill, M., White, R. G., Hawn, T. R. (2020). Clinical Development of New TB Vaccines: Recent Advances and Next Steps. Front. Microbiol. 10, 3154. doi: 10.3389/fmicb.2019.03154
Horne, D. J., Graustein, A. D., Shah, J. A., Peterson, G., Savlov, M., Steele, S., et al. (2016). Human ULK1 Variation and Susceptibility to Mycobacterium tuberculosis Infection. J. Infect. Dis. 214, 1260–1267. doi: 10.1093/infdis/jiw347
Intemann, C. D., Thye, T., Niemann, S., Browne, E. N. L., Amanua Chinbuah, M., Enimil, A., et al. (2009). Autophagy Gene Variant IRGM –261t Contributes to Protection From Tuberculosis Caused by Mycobacterium Tuberculosis But Not by M. Africanum Strains. PloS Pathog. 5, e1000577. doi: 10.1371/journal.ppat.1000577
Jacomin, A.-C., Gul, L., Sudhakar, P., Korcsmaros, T., Nezis, I. P. (2018). What We Learned From Big Data for Autophagy Research. Front. Cell Dev. Biol. 6, 92. doi: 10.3389/fcell.2018.00092
Jagannath, C., Lindsey, D. R., Dhandayuthapani, S., Xu, Y., Hunter, R. L., Eissa, N. T. (2009). Autophagy Enhances the Efficacy of BCG Vaccine by Increasing Peptide Presentation in Mouse Dendritic Cells. Nat. Med. 15, 267–276. doi: 10.1038/nm.1928
Jolliffe, D. A., Walton, R. T., Griffiths, C. J., Martineau, A. R. (2016). Single Nucleotide Polymorphisms in the Vitamin D Pathway Associating With Circulating Concentrations of Vitamin D Metabolites and Non-Skeletal Health Outcomes: Review of Genetic Association Studies. J. Steroid Biochem. Mol. Biol. 164, 18–29. doi: 10.1016/j.jsbmb.2015.12.007
Juárez, E., Carranza, C., Sánchez, G., González, M., Chávez, J., Sarabia, C., et al. (2016). Loperamide Restricts Intracellular Growth of Mycobacterium Tuberculosis in Lung Macrophages. Am. J. Respir. Cell Mol. Biol. 55, 837–847. doi: 10.1165/rcmb.2015-0383OC
Jurado, J. O., Pasquinelli, V., Alvarez, I. B., Peña, D., Rovetta, A. I., Tateosian, N. L., et al. (2012). IL-17 and IFN-γ Expression in Lymphocytes From Patients With Active Tuberculosis Correlates With the Severity of the Disease. J. Leukoc. Biol. 91, 991–1002. doi: 10.1189/jlb.1211619
Khan, A., Bakhru, P., Saikolappan, S., Das, K., Soudani, E., Singh, C. R., et al. (2019). An Autophagy-Inducing and TLR-2 Activating BCG Vaccine Induces a Robust Protection Against Tuberculosis in Mice. NPJ Vaccines 4, 1–19. doi: 10.1038/s41541-019-0122-8
Ke, Z., Lu, J., Zhu, J., Yang, Z., Jin, Z., Yuan, L. (2020). Down-Regulation of lincRNA-EPS Regulates Apoptosis and Autophagy In BCG-Infected RAW264.7 Macrophages Via JNK/MAPK Signaling Pathway. Infect. Genet. Evol. 77, 104077. doi: 10.1016/j.meegid.2019.104077
Kim, J. K., Lee, H.-M., Park, K.-S., Shin, D.-M., Kim, T. S., Kim, Y. S., et al. (2017). MIR144* Inhibits Antimicrobial Responses Against Mycobacterium Tuberculosis in Human Monocytes and Macrophages by Targeting the Autophagy Protein DRAM2. Autophagy 13, 423–441. doi: 10.1080/15548627.2016.1241922
Kim, J.-J., Lee, H.-M., Shin, D.-M., Kim, W., Yuk, J.-M., Jin, H. S., et al. (2012). Host Cell Autophagy Activated by Antibiotics Is Required for Their Effective Antimycobacterial Drug Action. Cell Host Microbe 11, 457–468. doi: 10.1016/j.chom.2012.03.008
Kimura, T., Watanabe, E., Sakamoto, T., Takasu, O., Ikeda, T., Ikeda, K., et al. (2014). Autophagy-Related IRGM Polymorphism Is Associated With Mortality of Patients With Severe Sepsis. PloS One 9, e91522. doi: 10.1371/journal.pone.0091522
King, K. Y., Lew, J. D., Ha, N. P., Lin, J. S., Ma, X., Graviss, E. A., et al. (2011). Polymorphic Allele of Human IRGM1 Is Associated With Susceptibility to Tuberculosis in African Americans. PloS One 6, e16317. doi: 10.1371/journal.pone.0016317
Kundu, M., Basu, J. (2021). The Role of microRNAs and Long Non-Coding RNAs in the Regulation of the Immune Response to Mycobacterium Tuberculosis Infection. Front. Immunol. 12, 687962. doi: 10.3389/fimmu.2021.687962
Lam, K. K. Y., Zheng, X., Forestieri, R., Balgi, A. D., Nodwell, M., Vollett, S., et al. (2012). Nitazoxanide Stimulates Autophagy and Inhibits Mtorc1 Signaling and Intracellular Proliferation of Mycobacterium Tuberculosis. PloS Pathog. 8, e1002691. doi: 10.1371/journal.ppat.1002691
Lee, K. J. (2015). Pharmacologic Agents for Chronic Diarrhea. Intest. Res. 13, 306–312. doi: 10.5217/ir.2015.13.4.306
Li, C. M., Campbell, S. J., Kumararatne, D. S., Bellamy, R., Ruwende, C., McAdam, K. P. W. J., et al. (2002). Association of a Polymorphism in the P2X7 Gene With Tuberculosis in a Gambian Population. J. Infect. Dis. 186, 1458–1462. doi: 10.1086/344351
Li, M., Cui, J., Niu, W., Huang, J., Feng, T., Sun, B., et al. (2019). Long Non-Coding PCED1B-AS1 Regulates Macrophage Apoptosis and Autophagy by Sponging miR-155 in Active Tuberculosis. Biochem. Biophys. Res. Commun. 509, 803–809. doi: 10.1016/j.bbrc.2019.01.005
Li, F., Gao, B., Xu, W., Chen, L., Xiong, S. (2016). The Defect in Autophagy Induction by Clinical Isolates of Mycobacterium Tuberculosis Is Correlated With Poor Tuberculosis Outcomes. PloS One 11, e0147810. doi: 10.1371/journal.pone.0147810
Liu, F., Chen, J., Wang, P., Li, H., Zhou, Y., Liu, H. (2018). MicroRNA-27a Controls The Intracellular Survival Of Mycobacterium Tuberculosis by Regulating Calcium-Associated Autophagy. Nat. Commun. 9, 4295. doi: 10.1038/s41467-018-06836-4
Liu, G., Wan, Q., Li, J., Hu, X., Gu, X., Xu, S. (2020). Silencing miR-125b-5p Attenuates Inflammatory Response and Apoptosis Inhibition in Mycobacterium Tuberculosis-Infected Human Macrophages by Targeting DNA Damage-Regulated Autophagy Modulator 2 (DRAM2). Cell Cycle 19, 3182–3194. doi: 10.1080/15384101.2020.1838792
Liu, W., Zhou, J., Niu, F., Pu, F., Wang, Z., Huang, M., et al. (2020). Mycobacterium Tuberculosis Infection Increases the Number of Osteoclasts and Inhibits Osteoclast Apoptosis by Regulating TNF-α-Mediated Osteoclast Autophagy. Exp. Ther. Med. 20, 1889–1898. doi: 10.3892/etm.2020.8903
Lobato, L. S., Rosa, P. S., Ferreira, J., da, S., Neumann, A. da S., da Silva, M. G., et al. (2014). Statins Increase Rifampin Mycobactericidal Effect. Antimicrob. Agents Chemother. 58, 5766–5774. doi: 10.1128/AAC.01826-13
Lu, Y., Li, Q., Peng, J., Zhu, Y., Wang, F., Wang, C., et al. (2016). Association of Autophagy-Related IRGM Polymorphisms With Latent Versus Active Tuberculosis Infection in a Chinese Population. Tuberculosis 97, 47–51. doi: 10.1016/j.tube.2016.01.001
Madhur, S., Ashish, S., Rajeev, R., Anuradha, G., Sanketkumar, P., Amit, M. (2016). Opportunities and Challenges for Host-Directed Therapies in Tuberculosis. Curr. Pharm. Design 22, 2599–2604. doi: 10.2174/1381612822666160128150636
Martínez-Colón, G. J., Moore, B. B. (2018). Prostaglandin E2 as a Regulator of Immunity to Pathogens. Pharmacol. Ther. 185, 135–146. doi: 10.1016/j.pharmthera.2017.12.008
Marupuru, S., Senapati, P., Pathadka, S., Miraj, S. S., Unnikrishnan, M. K., Manu, M. K. (2017). Protective Effect of Metformin Against Tuberculosis Infections in Diabetic Patients: An Observational Study of South Indian Tertiary Healthcare Facility. Braz. J. Infect. Dis. 21, 312–316. doi: 10.1016/j.bjid.2017.01.001
Ma, C., Wang, N., Detre, C., Wang, G., O’Keeffe, M., Terhorst, C. (2012). Receptor Signaling Lymphocyte-Activation Molecule Family 1 (Slamf1) Regulates Membrane Fusion and NADPH Oxidase 2 (NOX2) Activity by Recruiting a Beclin-1/Vps34/ultraviolet Radiation Resistance-Associated Gene (UVRAG) Complex. J. Biol. Chem. 287, 18359–18365. doi: 10.1074/jbc.M112.367060
Mayer-Barber, K. D., Andrade, B. B., Oland, S. D., Amaral, E. P., Barber, D. L., Gonzales, J., et al. (2014). Host-Directed Therapy of Tuberculosis Based on Interleukin-1 and Type I Interferon Crosstalk. Nature 511, 99–103. doi: 10.1038/nature13489
Nieuwenhuizen, N. E., Kulkarni, P. S., Shaligram, U., Cotton, M. F., Rentsch, C. A., Eisele, B., et al. (2017). The Recombinant Bacille Calmette–Guérin Vaccine VPM1002: Ready for Clinical Efficacy Testing. Front. Immunol. 8, 1147. doi: 10.3389/fimmu.2017.01147
Ottenhoff, T. H. M., Kumararatne, D., Casanova, J.-L. (1998). Novel Human Immunodeficiencies Reveal the Essential Role of Type-1 Cytokines in Immunity to Intracellular Bacteria. Immunol. Today 19, 491–494. doi: 10.1016/S0167-5699(98)01321-8
Pacheco, A. G., Moraes, M. O. (2009). Genetic Polymorphisms of Infectious Diseases in Case-Control Studies. Dis. Markers 27, 173–186. doi: 10.3233/DMA-2009-0654
Padmapriyadarsini, C., Bhavani, P. K., Natrajan, M., Ponnuraja, C., Kumar, H., Gomathy, S. N., et al. (2019). Evaluation of Metformin in Combination With Rifampicin Containing Antituberculosis Therapy in Patients With New, Smear-Positive Pulmonary Tuberculosis (METRIF): Study Protocol for a Randomised Clinical Trial. BMJ Open 9, e024363. doi: 10.1136/bmjopen-2018-024363
Parihar, S. P., Guler, R., Khutlang, R., Lang, D. M., Hurdayal, R., Mhlanga, M. M., et al. (2014). Statin Therapy Reduces the Mycobacterium Tuberculosis Burden in Human Macrophages and in Mice by Enhancing Autophagy and Phagosome Maturation. J. Infect. Dis. 209, 754–763. doi: 10.1093/infdis/jit550
Park, H.-J., Lee, S. J., Kim, S.-H., Han, J., Bae, J., Kim, S. J., et al. (2011). IL-10 Inhibits the Starvation Induced Autophagy in Macrophages via Class I Phosphatidylinositol 3-Kinase (PI3K) Pathway. Mol. Immunol. 48, 720–727. doi: 10.1016/j.molimm.2010.10.020
Pasquinelli, V., Quiroga, M. F., Martínez, G. J., Zorrilla, L. C., Musella, R. M., Bracco, M. M., et al. (2004). Expression of Signaling Lymphocytic Activation Molecule-Associated Protein Interrupts IFN-Gamma Production in Human Tuberculosis. J. Immunol. 172, 1177–1185. doi: 10.4049/jimmunol.172.2.1177
Pasquinelli, V., Townsend, J. C., Jurado, J. O., Alvarez, I. B., Quiroga, M. F., Barnes, P. F., et al. (2009). IFN-Gamma Production During Active Tuberculosis Is Regulated by Mechanisms That Involve IL-17, SLAM, and CREB. J. Infect. Dis. 199, 661–665. doi: 10.1086/596742
Pelissier-Rota, M. A., Pelosi, L., Meresse, P., Jacquier-Sarlin, M. R. (2015). Nicotine-Induced Cellular Stresses and Autophagy in Human Cancer Colon Cells: A Supportive Effect on Cell Homeostasis via Up-Regulation of Cox-2 and PGE2 Production. Int. J. Biochem. Cell Biol. 65, 239–256. doi: 10.1016/j.biocel.2015.06.013
Pellegrini, J. M., Martin, C., Morelli, M. P., Schander, J. A., Tateosian, N. L., Amiano, N. O., et al. (2021). PGE2 Displays Immunosuppressive Effects During Human Active Tuberculosis. Sci. Rep. 11, 13559. doi: 10.1038/s41598-021-92667-1
Pellegrini, J. M., Sabbione, F., Morelli, M. P., Tateosian, N. L., Castello, F. A., Amiano, N. O., et al. (2020). Neutrophil Autophagy During Human Active Tuberculosis Is Modulated by SLAMF1. Autophagy 17 (9), 2629–2638. doi: 10.1080/15548627.2020.1825273
Ponpuak, M., Davis, A. S., Roberts, E. A., Delgado, M. A., Dinkins, C., Zhao, Z., et al. (2010). Delivery of Cytosolic Components by Autophagic Adaptor Protein P62 Endows Autophagosomes With Unique Antimicrobial Properties. Immunity 32, 329–341. doi: 10.1016/j.immuni.2010.02.009
Ralph, A. P., Waramori, G., Pontororing, G. J., Kenangalem, E., Wiguna, A., Tjitra, E., et al. (2013). L-Arginine and Vitamin D Adjunctive Therapies in Pulmonary Tuberculosis: A Randomised, Double-Blind, Placebo-Controlled Trial. PloS One 8, e70032. doi: 10.1371/journal.pone.0070032
Romagnoli, A., Petruccioli, E., Palucci, I., Camassa, S., Carata, E., Petrone, L., et al. (2018). Clinical Isolates of the Modern Mycobacterium Tuberculosis Lineage 4 Evade Host Defense in Human Macrophages Through Eluding IL-1β-Induced Autophagy. Cell Death Dis. 9, 624. doi: 10.1038/s41419-018-0640-8
Rovetta, A. I., Peña, D., Hernández Del Pino, R. E., Recalde, G. M., Pellegrini, J., Bigi, F., et al. (2014). IFNG-Mediated Immune Responses Enhance Autophagy Against Mycobacterium Tuberculosis Antigens in Patients With Active Tuberculosis. Autophagy 10, 2109–2121. doi: 10.4161/15548627.2014.981791
Russell, D. G. (2001). Mycobacterium Tuberculosis: Here Today, and Here Tomorrow. Nat. Rev. Mol. Cell Biol. 2, 569–578. doi: 10.1038/35085034
Sabir, N., Hussain, T., Shah, S. Z. A., Peramo, A., Zhao, D., Zhou, X. (2018). miRNAs in Tuberculosis: New Avenues for Diagnosis and Host-Directed Therapy. Front. Microbiol. 9, 602. doi: 10.3389/fmicb.2018.00602
Schiebler, M., Brown, K., Hegyi, K., Newton, S. M., Renna, M., Hepburn, L., et al. (2015). Functional Drug Screening Reveals Anticonvulsants as Enhancers of mTOR-Independent Autophagic Killing of Mycobacterium Tuberculosis Through Inositol Depletion. EMBO Mol. Med. 7, 127–139. doi: 10.15252/emmm.201404137
Shi, Q., Wang, J., Yang, Z., Liu, Y. (2020). CircAGFG1modulates Autophagy and Apoptosis of Macrophages Infected by Mycobacterium Tuberculosis via the Notch Signaling Pathway. Ann. Transl. Med. 8, 645. doi: 10.21037/atm.2020-20-3048
Silva, B. J., de, A., Barbosa, M.G. de M., Andrade, P. R., Ferreira, H., Nery, J.A. da C., et al. (2017). Autophagy Is an Innate Mechanism Associated With Leprosy Polarization. PloS Pathog. 13, e1006103. doi: 10.1371/journal.ppat.1006103
Singhal, A., Jie, L., Kumar, P., Hong, G. S., Leow, M. K.-S., Paleja, B., et al. (2014). Metformin as Adjunct Antituberculosis Therapy. Sci. Transl. Med. 6, 263ra159. doi: 10.1126/scitranslmed.3009885
Singh, D. P., Bagam, P., Sahoo, M. K., Batra, S. (2017). Immune-Related Gene Polymorphisms in Pulmonary Diseases. Toxicology 383, 24–39. doi: 10.1016/j.tox.2017.03.020
Singh, A. K., Ghosh, M., Kumar, V., Aggarwal, S., Patil, S. A. (2021). Interplay Between miRNAs and Mycobacterium Tuberculosis: Diagnostic and Therapeutic Implications. Drug Discov. Today 26, 1245–1255. doi: 10.1016/j.drudis.2021.01.021
Sodhi, A., Gong, J., Silva, C., Qian, D., Barnes, P. F. (1997). Clinical Correlates of Interferon γ Production in Patients With Tuberculosis. Clin. Infect. Dis. 25, 617–620. doi: 10.1086/513769
Songane, M., Kleinnijenhuis, J., Alisjahbana, B., Sahiratmadja, E., Parwati, I., Oosting, M., et al. (2012). Polymorphisms in Autophagy Genes and Susceptibility to Tuberculosis. PloS One 7, e41618. doi: 10.1371/journal.pone.0041618
Sousa, J., Cá, B., Maceiras, A. R., Simões-Costa, L., Fonseca, K. L., Fernandes, A. I., et al. (2020). Mycobacterium Tuberculosis Associated With Severe Tuberculosis Evades Cytosolic Surveillance Systems and Modulates IL-1β Production. Nat. Commun. 11, 1949. doi: 10.1038/s41467-020-15832-6
Staedel, C., Darfeuille, F. (2013). MicroRNAs and Bacterial Infection. Cell. Microbiol. 15, 1496–1507. doi: 10.1111/cmi.12159
Stanley, S. A., Barczak, A. K., Silvis, M. R., Luo, S. S., Sogi, K., Vokes, M., et al. (2014). Identification of Host-Targeted Small Molecules That Restrict Intracellular Mycobacterium Tuberculosis Growth. PloS Pathog. 10, e1003946. doi: 10.1371/journal.ppat.1003946
Sterne, J. A. C., Rodrigues, L. C., Guedes, I. N. (1998). Does the Efficacy of BCG Decline With Time Since Vaccination? Int. J. Tuberc. Lung Dis. 2, 200–207.
Subbian, S., Koo, M.-S., Tsenova, L., Khetani, V., Zeldis, J. B., Fallows, D., et al. (2016a). Pharmacologic Inhibition of Host Phosphodiesterase-4 Improves Isoniazid-Mediated Clearance of Mycobacterium Tuberculosis. Front. Immunol. 7, 238. doi: 10.3389/fimmu.2016.00238
Subbian, S., Tsenova, L., Holloway, J., Peixoto, B., O’Brien, P., Dartois, V., et al. (2016b). Adjunctive Phosphodiesterase-4 Inhibitor Therapy Improves Antibiotic Response to Pulmonary Tuberculosis in a Rabbit Model. EBioMedicine 4, 104–114. doi: 10.1016/j.ebiom.2016.01.015
Sullivan, T., Ben Amor, Y. (2012). The Co-Management of Tuberculosis and Diabetes: Challenges and Opportunities in the Developing World. PloS Med. 9, e1001269. doi: 10.1371/journal.pmed.1001269
Sundaramurthy, V., Barsacchi, R., Samusik, N., Marsico, G., Gilleron, J., Kalaidzidis, I., et al. (2013). Integration of Chemical and RNAi Multiparametric Profiles Identifies Triggers of Intracellular Mycobacterial Killing. Cell Host Microbe 13, 129–142. doi: 10.1016/j.chom.2013.01.008
Tateosian, N. L., Pellegrini, J. M., Amiano, N. O., Rolandelli, A., Casco, N., Palmero, D. J., et al. (2017). IL17A Augments Autophagy in Mycobacterium Tuberculosis-Infected Monocytes From Patients With Active Tuberculosis in Association With the Severity of the Disease. Autophagy 13, 1191–1204. doi: 10.1080/15548627.2017.1320636
Tukvadze, N., Sanikidze, E., Kipiani, M., Hebbar, G., Easley, K. A., Shenvi, N., et al. (2015). High-Dose Vitamin D3 in Adults With Pulmonary Tuberculosis: A Double-Blind Randomized Controlled Trial. Am. J. Clin. Nutr. 102, 1059–1069. doi: 10.3945/ajcn.115.113886
Wallis, R. S., Ginindza, S., Beattie, T., Arjun, N., Likoti, M., Edward, V. A., et al. (2021). Adjunctive Host-Directed Therapies for Pulmonary Tuberculosis: A Prospective, Open-Label, Phase 2, Randomised Controlled Trial. Lancet Respir. Med. 9, 897–908. doi: 10.1016/S2213-2600(20)30448-3
Wan, M., Tang, X., Rekha, R. S., Muvva, S. S. V. J. R., Brighenti, S., Agerberth, B., et al. (2018). Prostaglandin E2 Suppresses Hcap18/LL-37 Expression in Human Macrophages via EP2/EP4: Implications for Treatment of Mycobacterium Tuberculosis Infection. FASEB J. 32, 2827–2840. doi: 10.1096/fj.201701308
Wu, H.-X., Xiong, X.-F., Zhu, M., Wei, J., Zhuo, K.-Q., Cheng, D.-Y. (2018). Effects of Vitamin D Supplementation on the Outcomes of Patients With Pulmonary Tuberculosis: A Systematic Review and Meta-Analysis. BMC Pulm. Med. 18, 108. doi: 10.1186/s12890-018-0677-6
Xia, J., Shi, L., Zhao, L., Xu, F. (2014). Impact of Vitamin D Supplementation on the Outcome of Tuberculosis Treatment: A Systematic Review and Meta-Analysis of Randomized Controlled Trials. Chin. Med. J. (Engl.) 127, 3127–3134. doi: 10.3760/cma.j.issn.0366-6999.20140702
Xiong, W., Wen, Q., Du, X., Wang, J., He, W., Wang, R., et al. (2018). Novel Function of Cyclooxygenase-2: Suppressing Mycobacteria by Promoting Autophagy via the Protein Kinase B/Mammalian Target of Rapamycin Pathway. J. Infect. Dis. 217, 1267–1279. doi: 10.1093/infdis/jiy033
Yang, T., Ge, B. (2018). miRNAs in Immune Responses to Mycobacterium Tuberculosis Infection. Cancer Lett. 431, 22–30. doi: 10.1016/j.canlet.2018.05.028
Yuan, L., Ke, Z., Ma, J., Guo, Y., Li, Y. (2016). IRGM Gene Polymorphisms and Haplotypes Associate With Susceptibility of Pulmonary Tuberculosis in Chinese Hubei Han Population. Tuberc. (Edinb.) 96, 58–64. doi: 10.1016/j.tube.2015.10.014
Yuk, J.-M., Shin, D.-M., Lee, H.-M., Yang, C.-S., Jin, H. S., Kim, K.-K., et al. (2009). Vitamin D3 Induces Autophagy in Human Monocytes/Macrophages via Cathelicidin. Cell Host Microbe 6, 231–243. doi: 10.1016/j.chom.2009.08.004
Yu, Q., Zhu, X., Di, W., Jiang, Y. (2016). High Beclin-1 Expression in Human Alveolar Macrophage Significantly Correlate With the Bacteriologic Sterilization in Pulmonary Tuberculosis Patients. Clin. Lab. 62, 1003–1007. doi: 10.7754/clin.lab.2015.150833
Zhang, C., Fu, Q., Ding, M., Chen, T., Lu, X., Zhong, Y., et al. (2019). Comprehensive Analysis of Differentially Expressed Serum microRNAs in Humans Responding to Brucella Infection. Ann. Transl. Med. 7, 301. doi: 10.21037/atm.2019.05.74
Keywords: autophagy, tuberculosis, human, host-directed therapy, immunology & infectious diseases
Citation: Pellegrini JM, Tateosian NL, Morelli MP and García VE (2022) Shedding Light on Autophagy During Human Tuberculosis. A Long Way to Go. Front. Cell. Infect. Microbiol. 11:820095. doi: 10.3389/fcimb.2021.820095
Received: 22 November 2021; Accepted: 13 December 2021;
Published: 05 January 2022.
Edited by:
Hua Niu, Affiliated Hospital of Guilin Medical University, ChinaReviewed by:
Marisa Ponpuak, Mahidol University, ThailandCopyright © 2022 Pellegrini, Tateosian, Morelli and García. This is an open-access article distributed under the terms of the Creative Commons Attribution License (CC BY). The use, distribution or reproduction in other forums is permitted, provided the original author(s) and the copyright owner(s) are credited and that the original publication in this journal is cited, in accordance with accepted academic practice. No use, distribution or reproduction is permitted which does not comply with these terms.
*Correspondence: Verónica Edith García, vgarcia@qb.fcen.uba.ar