- 1Basic Medical Science College, Qiqihar Medical University, Qiqihar, China
- 2Medical School of Nantong University, Nantong University, Nantong, China
- 3Department of Infection Control, The Second Affiliated Hospital of Qiqihar Medical University, Qiqihar, China
- 4Department of Nuclear Medicine, The Third Affiliated Hospital of Qiqihar Medical University, Qiqihar, China
- 5Department of Breast Surgery, Harbin Medical University Cancer Hospital, Harbin, China
- 6The Academic Affairs Office, Qiqihar Medical University, Qiqihar, China
- 7Department of Operating Room, Qiqihar First Hospital, Qiqihar, China
Adult neurogenesis is the process of differentiation of neural stem cells (NSCs) into neurons and glial cells in certain areas of the adult brain. Defects in neurogenesis can lead to neurodegenerative diseases, mental disorders, and other maladies. This process is directionally regulated by transcription factors, the Wnt and Notch pathway, the extracellular matrix, and various growth factors. External factors like stress, physical exercise, diet, medications, etc., affect neurogenesis and the gut microbiota. The gut microbiota may affect NSCs through vagal, immune and chemical pathways, and other pathways. Traditional Chinese medicine (TCM) has been proven to affect NSCs proliferation and differentiation and can regulate the abundance and metabolites produced by intestinal microorganisms. However, the underlying mechanisms by which these factors regulate neurogenesis through the gut microbiota are not fully understood. In this review, we describe the recent evidence on the role of the gut microbiota in neurogenesis. Moreover, we hypothesize on the characteristics of the microbiota-gut-brain axis based on bacterial phyla, including microbiota’s metabolites, and neuronal and immune pathways while providing an outlook on TCM’s potential effects on adult neurogenesis by regulating gut microbiota.
Introduction
The brain development and normal brain function are closely related to neuron and glial cells cytogenesis and activation. This process is also closely related to neural stem cells (NSCs) differentiation. NSCs are a kind of blast cells with multi-directional differentiation potential and self-renewal ability, which can proliferate and differentiate into neurons, astrocytes, and oligodendrocytes, either during embryonic development or in the adult brain (Altman and Das, 1965; Bottes et al., 2021), although NSCs remain dormant for a long time after the embryonic stage. In the adult brain, NSCs are found in the ventricular-subventricular zone (V-SVZ) lining the lateral ventricles, where olfactory bulb neurons, astrocytes, and oligodendrocytes are generated, and the subgranular zone (SGZ) of the dentate gyrus (DG) in the hippocampus, where neurons are mainly generated (Ming and Song, 2011; Song et al., 2016; Mizrak et al., 2019). In addition, the hypothalamus may be another neurogenic region in the brain: dorsal α1 region, dorsal α2 region, and adjacent median eminence (Andreotti et al., 2019). NSCs proliferation and differentiation is a finely controlled and complex process. In V-SVZ, the embryonic radial glia can differentiate into NSCs of the V-SVZ (B1 cells). As adults, B1 cells undergo symmetric division to complete cell self-renewal or undergo asymmetric division to form intermediate progenitors (C cells), which further generate neuroblasts (A cells) (Ponti et al., 2013; Fuentealba et al., 2015; Obernier et al., 2018). In the SGZ, these NSCs are known as radial glia-like cells (or type 1 cells); when activated, they produce actively proliferating neural progenitor cells (NPCs or type 2 cells), which proliferate and produce neuroblasts (type 3 cells), which exit the cell cycle and differentiate into neurons (Urban et al., 2019; Harris et al., 2021). Elucidating the regulatory mechanism of NSCs proliferation and differentiation is crucial for understanding nervous system development, nerve repair, and cell transplantation for the treatment of nervous system diseases. Current studies have shown that the main factor for self-renewal, differentiation, and maintenance of NSCs is genetic and depends on the co-integration of multiple cellular signaling systems in the microenvironment, including the Wnt, Notch, Sonic hedgehog (SHH) pathways, etc.
Currently, the gut microbiota has been shown to play an important role in regulating neuronal and glial function, interfering with neuron and glial cytogenesis and activation through the microbiota-gut-brain (MGB) axis, which is mainly composed of the enteric nervous system, central nervous system (CNS), and immune system (Cryan et al., 2019; Gershon and Margolis, 2021). The gut microbiota establishes two-way communication with the brain through its metabolites, which cross the blood-brain barrier (BBB), pass through the vagus nerve, or induce peripheral immunity (Morais et al., 2021). Therefore, changes in the structure of the gut microbiota may affect NSCs differentiation and neurogenesis.
Traditional Chinese medicine (TCM) is a medical system with a long history and is widely used in the prevention and treatment of nervous system diseases and regulation of body homeostasis. The active ingredients of TCM play an important role in regulating NSCs differentiation, and neuron and glia generation and activation (Qin et al., 2017; Wang X. F. et al., 2021). Many reports have indicated that TCM can impact the composition and metabolism of the gut microbiota (Feng et al., 2019). Gut microbiota can release signaling substances that promote the development and maintenance of host digestive, immune, metabolic, and neurobiological functions, and these signaling substances also be regulated by drug. TCM has shown great potential in regulating the gut microbiota to influence NSCs. This review highlights several advanced mechanisms of signaling pathways in neurogenesis and systematically discusses potential TCM strategies to regulate NSCs through the gut microbiota.
Signaling pathways involved in NSCs proliferation and differentiation
The proliferation, differentiation and migration of NSCs in the neurogenic niches maintain neurogenesis by interacting with the glial cells, extracellular matrix and microenvironment of the niches. Table 1 summarizes the effects of cellular and microenvironmental components of different niches on NSCs. Changes in the cell, the extracellular matrix and microenvironmental components affect the function of NSCs, that is, by changing external factors and affecting relevant signal pathways, such as Notch, Wnt, SHH, FOXO, and other pathways, proteins or factors (Figures 1–3), and influence neurogenesis. Thus, changes in the abundance of the gut microbiota may be able to influence adult neurogenesis by altering the cells, the extracellular matrix and components of the microenvironment in certain ways.
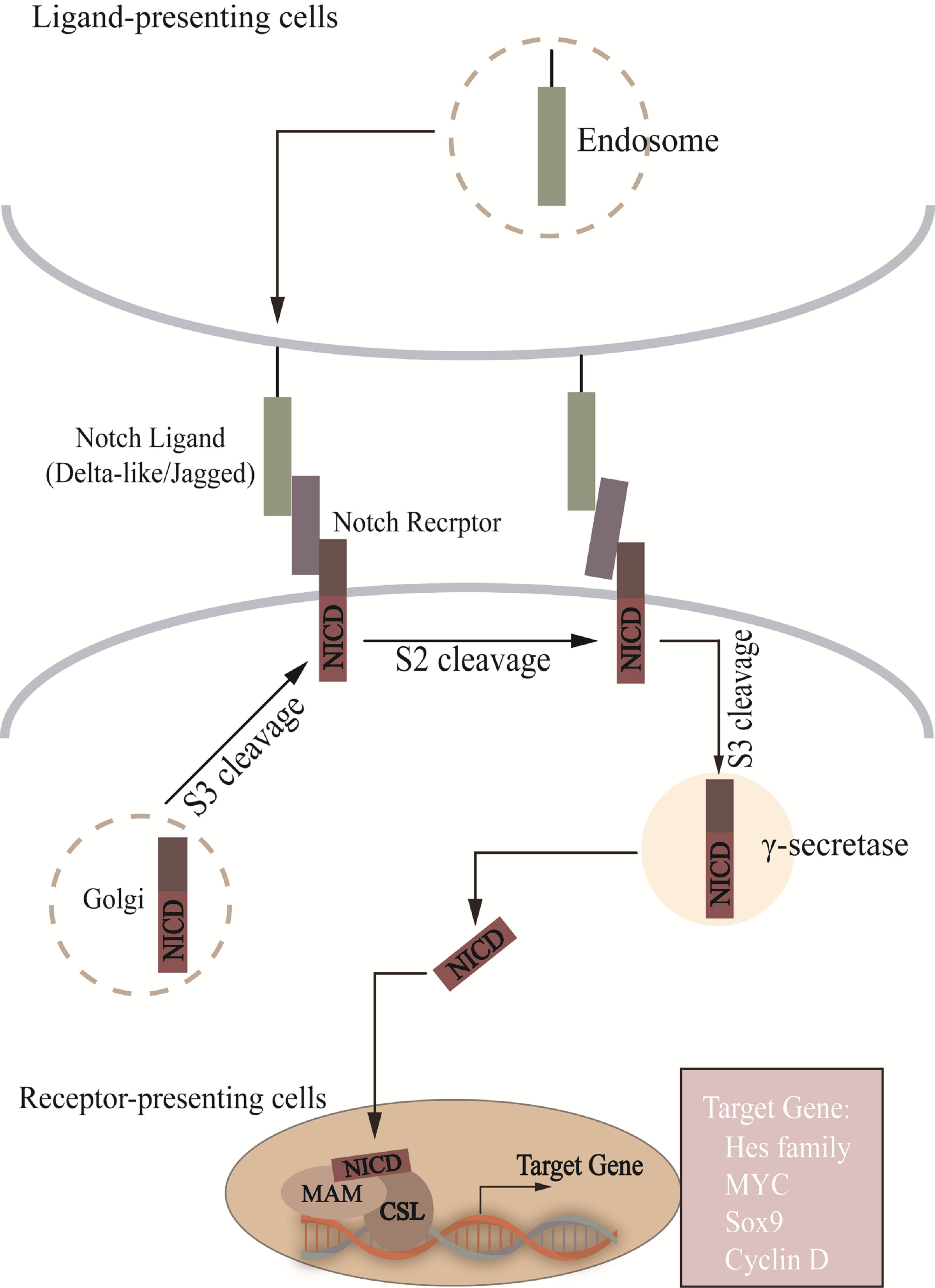
Figure 1 In Notch signal pathway, Notch is cut into heterodimer (S1 cleavage) in Golgi and transferred to cell membrane. S2 site is exposed after ligand-receptor interaction, which passes through AMAD metalloproteases and γ-secretase enzyme processing, translocation to the nucleus to combine with CSL, recruitment of MAM, and activation of target gene transcription. NICD, notch intracellular structural domain; MAM, mastermind; CSL, CBF1/RBP-J/Su(H)/Lag-1.
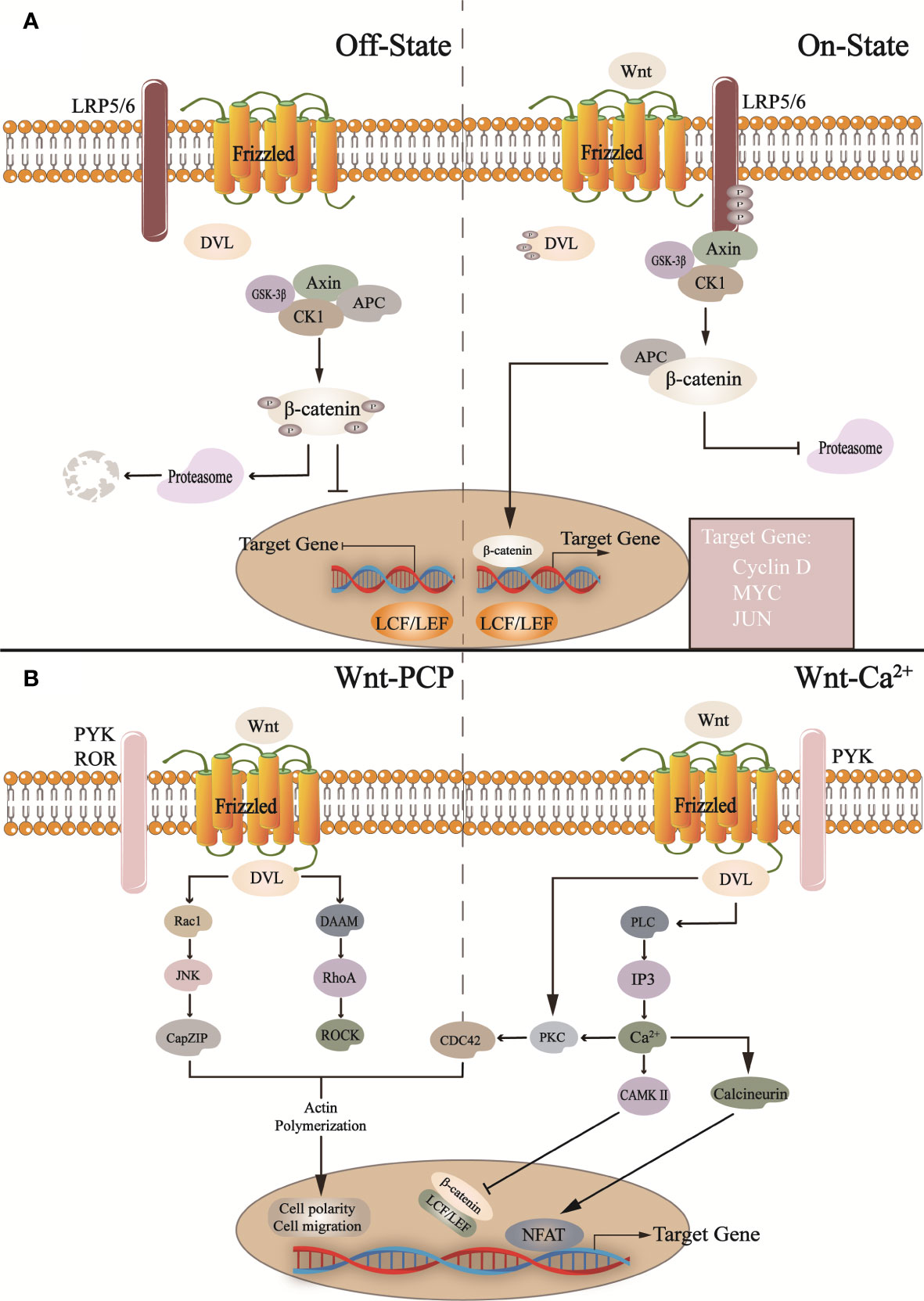
Figure 2 In the canonical Wnt pathway (A): In the absence of Wnt activation, the cytoplasmic β-catenin is phosphorylated by the destruction complex (Axin, CK1, GSK-3β, APC) and then degraded by proteasome after a series of reactions. When Wnt binds to Frizzled and LRP5/6 receptors, it activates DVL, inactive GSK-3β, and inhibits proteasome degradation β-catenin, and β-catenin translocates into the nucleus and binds with LCF/LEF to regulate target gene transcription. In the non-canonical Wnt pathway. CK1, casein kinase 1; GSK-3b, glycogen synthase kinase 3β; APC, adenomatous polyposis coli; LPR5/6, low-density lipoprotein-related receptors 5 and 6; DVL, Dishevelled. (B) In the Wnt/PCP pathway, Wnt binds to other receptors (such as ROR, RYK, etc.), activates DVL, and promotes downstream pathway transduction, such as Roc1, JNK, etc. This is related to cell migration and cell polarity. In addition, in the Wnt/Ca2+ pathway, it activates phospholipase C (PLC), triggers intracellular Ca2+ release, and regulates NFAT and β-catenin, which regulates the expression of related genes. Roc1, regulator of cullins 1; JNK, c-Jun N-terminal kinase; CapZIP, CapZ-interacting protein; DAAM, Dishevelled associated activator of morphogenesis; RhoA, Ras homolog gene family member A; ROCK, Rho-associated, coiled-coil containing kinases; PLC, phospholipase C; IP3, inositol 1,4,5-trisphosphate; PKC, protein kinase C; CAMKII, calcium/calmodulin-dependent protein kinase II; NFAT, nuclear factor of activated T-cells; PYK, RYK receptor-like tyrosine kinase; ROR, receptor tyrosine kinase-like orphan receptor.
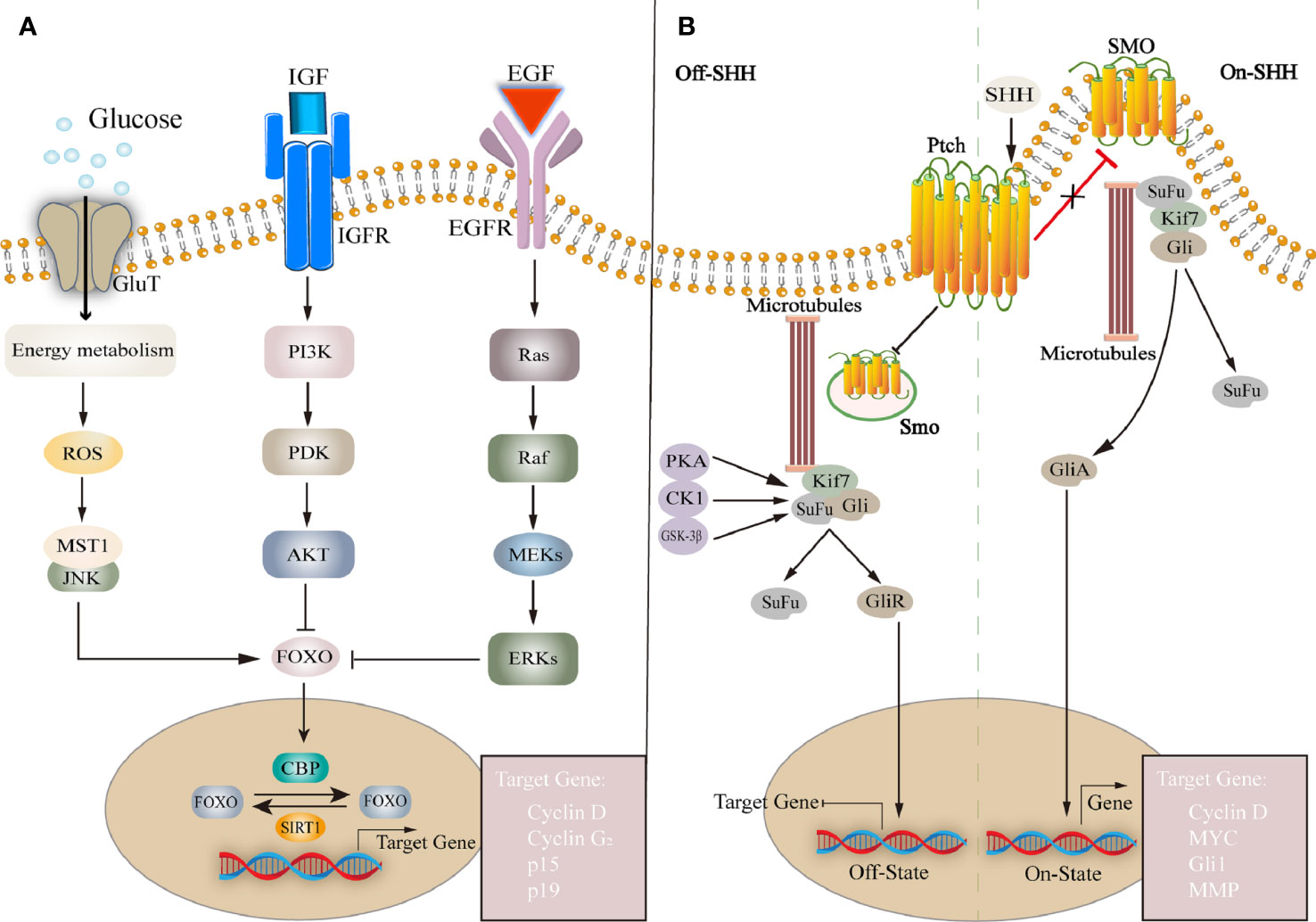
Figure 3 (A) FOXO family is a group of transcription factors that regulate the expression of downstream genes and affect cell proliferation, cell cycle, etc. FOXO is affected by many factors, such as insulin or IGF, EGF, glucose, etc. Regulated transcription by regulating histone acetyltransferases CBP and deacetylase IGF, insulin-like growth factor; EGF, epidermal growth factor; ROS, reactive oxygen species; MST1, mammalian sterile 20-like kinase 1; PI3K, phosphoinositide 3-kinase; PDK, phosphoinositide-dependent protein kinase; AKT, protein kinase B; FOXO, forkhead box O; SIRT1, silencing information regulator 2 related enzyme 1. (B) SHH pathway: Without SHH, Ptch inhibits Smo activity, Gli is phosphorylated by PKA, forming a repressor GliR, which is translocated to the nucleus to inhibit target gene transcription. Extracellular SHH binds to Ptch, and Smo is no longer inhibited. forming a repressor GliA, translocated to the nucleus and activation of target gene expression. Ptch, Patched; Smo, Smoothened; SuFu, suppressor of fused; Kif7, kinesin family member 7; Gli, Glioma-associated oncogene.
During brain development, both in embryos and adult animals, the Notch pathway is involved in NSCs regulation at various developmental stages, maintaining the number of NSCs in the brain in a dynamic balance, which is crucial for the development and maturation of the CNS (Figure 1) (Androutsellis-Theotokis et al., 2006; Mizutani et al., 2007; Imayoshi et al., 2013). The Notch pathway consists of Notch receptors (Notch1-4) and ligands such as Delta-like ligands and Jagged (Hori et al., 2013). The Notch receptor is activated by binding with ligands from adjacent cells. Receptor-ligand binding is followed by the release of the Notch intracellular structural domain (NICD) and Nβ peptide in the presence of ADAM metalloproteinase and γ-secretase. NICD enters the nucleus and is recognized by the transcriptional coactivator Mastermind-like proteins after binding to the DNA binding protein CSL (CBF1/RBP-J/Su(H)/Lag-1) and activates transcription (Kopan and Ilagan, 2009). Then the complex induces inhibitory transcription factors such as Hes, Cyclin D. We hypothesize that changes in the abundance of gut microbiota may affect the expression of Notch ligands and receptors and, to some extent, the function of NSCs and neurogenesis. It was found that after lead (Pb) exposure the intestinal permeability of mice was increased and the abundance of gut microbiota, such as Bacteroides, Lactobacillus, etc. was altered. The expression of Notch1, RBP-J, Hes1 and Hes2 was also upregulated in the DG and olfactory bulb (Sun et al., 2022). This suggests that after the intervention of TCM, it may affect the abundance of gut microbiota, thereby affecting Notch pathway, which in turn affects NSCs differentiation.
The Wnt pathway widely regulates the maintenance and differentiation of various stem cells in vivo. Wnt pathway dysregulation is an important cause of CNS diseases, such as Alzheimer’s disease (AD), Parkinson’s disease (PD), and spinal cord injury (Inestrosa and Arenas, 2010; Garcia-Velazquez and Arias, 2017). The Wnt pathway is divided into canonical Wnt/β-catenin and non-canonical Wnt/PCP and Wnt/Ca2+ pathways (Figure 2) (Lie et al., 2005; Butler and Wallingford, 2017). The canonical Wnt/β-catenin signaling pathway is mainly involved in NSCs proliferation and differentiation. Wnt is secreted outside the cell through covalent lipid modification in the endoplasmic reticulum (Clevers et al., 2014; Mehta et al., 2021). In the absence of extracellular Wnt ligands from the canonical pathway, β-catenin is degraded by a complex composed of glycogen synthase kinase 3β (GSK-3β) and casein kinase 1(CK1), Axin, etc., and the expression of Wnt target genes is suppressed (Gao et al., 2021). When Wnt ligands bind to Frizzled and Low-density Lipoprotein-related Receptors 5 and 6, they activate downstream signals, reducing β-catenin degradation. After nuclear transfer, the complex binds to the nuclear transcription factor lymphoid enhancer factor/T cell factor complex to induce target genes’ transcription (Macdonald and He, 2012; Hua et al., 2018) and influence NSCs proliferation by stimulating cell cycle-related factors, such as C-myc, Cyclin-D (Alvarez-Palomo et al., 2021; Liu B. C. et al., 2021; Wang et al., 2016a). Similarly, the lack of gut microbiota down-regulates the expression of neural genes, and gut microbiota metabolites timely save the process, especially affecting Wnt pathway (Rea et al., 2022).
SHH pathway also plays an important role in neurogenesis. The mammalian hedgehog family has three homologous genes Shh, Dhh, and Ihh, encoding hedgehog ligands, and two cell membrane surface molecular receptors Patched (Ptch) and Smoothened (Smo). Ptch negatively regulates protein kinase A (PKA) signaling whereas Smo is a necessary receptor for SHH signal transmission. When the Hedgehog ligand binds to Ptch, its inhibition of Smo stops, and Smo is activated to enter the cell and activate the downstream glioma-associated oncogene (Gli) family (Carballo et al., 2018). Without SHH ligand, Ptch is restricted to the base of the primary cilium and prevents Smo activity, PKA, GSK-3β, and the Gli repressor produced after phosphorylated CK1 enters the nucleus to inhibit the transcription of SHH target genes (Rana et al., 2021), such as CyclinD, Nmyc, Bmi1, Hey2, etc. Among them, Gli1 causes NSCs cycle arrest, reduces cell proliferation, and induces NSCs apoptosis. On the contrary, Gli1 inhibition leads to early maturation of NSCs derived oligodendrocytes in vitro (Galvin et al., 2008; Namchaiw et al., 2019). In the presence of SHH ligands, SHH and Ptch binding alleviated Smo inhibition and moved to the top of primary cilium, inducing Gli protein to separate from Kinesin family member 7 and Suppressor of Fused, and enter the nucleus to initiate transcription of target genes (Ingham et al., 2011; Briscoe and Therond, 2013). Long-term SHH activation can lead to the accumulation of quiescent neural stem cells in the V-SVZ, while the number of activated neural stem cells transiently increases and later decreases. Taurine, a metabolite of the gut microbiota, plays a unique role in participating in the SHH pathway. It intervenes to regulate the expression of SHH in NPCs and promotes cell proliferation and improves mitochondrial function (Ramos-Mandujano et al., 2014).
Regulation of neurogenesis by gut microbiota
Gut microbiota is a huge microbial community that inhabits the inner surface of the intestinal mucosa and the intestinal cavity. It is composed of bacteria, viruses, and fungi. Substances produced by the microbiota-metabolism mainly crosstalk between the brain and the gut via the MGB axis (Mayer et al., 2022). There is also evidence that the gut microbiota plays a role in influencing NSCs activities (Ogbonnaya et al., 2015). A high sugar diet decreases short chain fatty acids (SCFAs) in vivo, causes BBB damage, and decreases the number of NSCs and mature neurons. An effect effectively reversed after SCFAs intervention (Tang C. F. et al., 2022). In addition, butyrate production increased in aseptic mice transplanted with the gut microbiota of aged mice, which stimulated an increase in the number of hippocampal neurons (Kundu et al., 2019). In this section, we will introduce in detail the mechanism by which the gut microbiota may affect the differentiation of NSCs from the MGB axis (Figure 4 and Table 2).
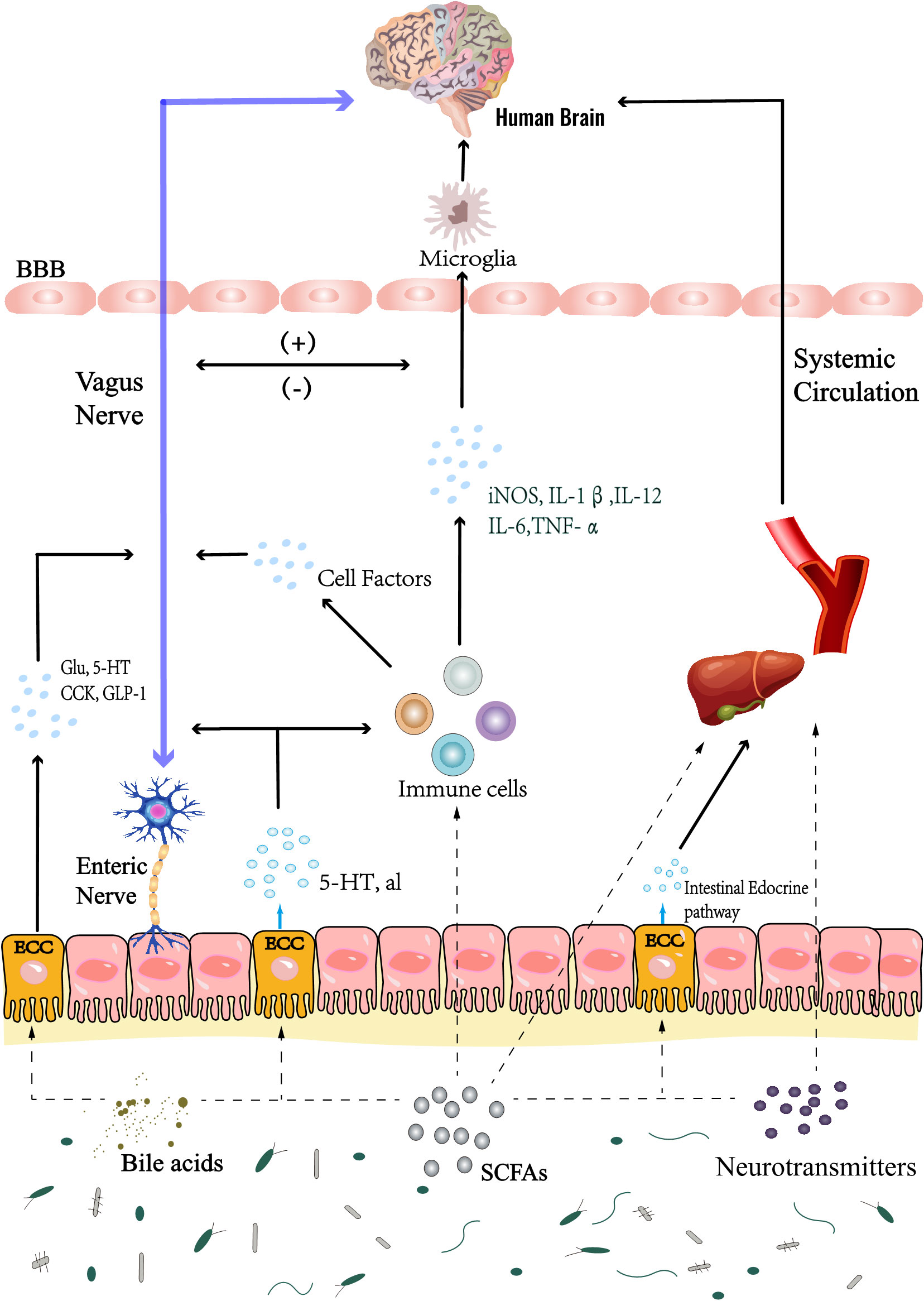
Figure 4 Gut microbiota mainly interacts with the brain through Chemical, Neuronal and Immune pathways. Metabolites of gut microbiota, such as SCFAs, neurotransmitters, bile acids, etc., directly affect the body and brain activities; In addition, the microbiota can also play this role by regulating the activities of enteroendocrine cells, such as ECCs. In the immune pathway, on the one hand, inflammatory factors can change the permeability of blood brain barrier and affect brain activity, these inflammatory factors also affect the brain resident immune cells; On the other hand, it can also affect brain inflammation through cellular immune pathway, which is through intestinal immune cells; Microbial metabolites also regulate the activities of brain resident immune cells. At the same time, inflammatory stimulation can also stimulate the vagus nerve, which also regulates brain activities, such as triggering the activation of HPA axis, pain conduction, etc. In addition, microbial metabolites and secretion of enteroendocrine cells directly affect the vagus nerve, or stimulate the intestinal nervous system to indirectly affect the vagus nerve, and establish a neural pathway to interact with the brain. SCFAs, short chain fatty acids. HPA axis, hypothalamic-pituitary-adrenal axis. ECCs, enterochromaffin cells. BBB, blood-brain barrier; Glu, glutamic acid; 5-HT, 5-hydroxytryptamine; CCK, cholecystokinin; GLP-1, glucagon-like peptide-1.
Chemical pathways of the microbiota-gut-brain axis
Gut microbiota metabolites can directly or indirectly regulate brain activity through neuroendocrine and other pathways. Bacterial metabolites such as SCFAs, bile acids, vitamins, and other substances have direct effects. SCFAs are the main products of dietary fiber fermentation by intestinal microorganisms. They are mainly composed of acetate, propionate, butyrate, etc.; after being absorbed by intestinal epithelial cells, they pass through the portal vein to the liver, and through the systemic circulation directly communicate with the CNS (Dalile et al., 2019). In this case, SCFAs produced by gut microbiota, such as F. prausnitzii, Eubacterium rectale, Bacteroides, act on the hippocampus through the BBB and regulate neurogenesis (Tan et al., 2019). As an important metabolite of gut microbiota, the activation of SCFAs and their receptors play an important role in the regulation of neurogenesis: Butyrate activates receptors GPR41 (FFAR3) and GPR43 (FFAR2), enhances pituitary growth hormone release, and promotes brain neurogenesis (Aberg, 2010; Miletta et al., 2014); Mice lacking FFAR2 (Ffar2-/-) showed microglial cell morphology, dysfunction and cellular defects. FFAR2 signaling was also found to be expressed on splenic Iba-1+ myeloid cells in the red marrow, thus SCFAs may interact with FFAR2 receptors in peripheral myeloid cells to maintain normal microglia function and may be involved in the regulation of neurogenesis (Erny et al., 2015); Intervention with butyrate (histone deacetylase inhibitor) reduced cell proliferation and significantly increased apoptotic cells. In contrast, activation of the butyrate receptor GPR41 (FFAR3) reduced the elevation of histone acetylation and prevented the anti-proliferative and pro-apoptotic effects of butyrate (Wu et al., 2012). At the same time, some studies directly show that SCFAs are involved in the regulation of neurogenesis, compared with the control group, SCFAs intervention promoted the proliferation of hNPCs, and changed the expression of genes related to neurogenesis, proliferation and apoptosis, such as neurogenesis related genes Atr, Ndn, proliferation related genes Cdk2, E2f1, Vegfa, and apoptosis related genes Bcl2, Bid, Casp8, Fas (Yang L. L. et al., 2020); of these, physiological concentrations of SCFAs (acetate: butyrate: propionate in a ratio of approximately 30:1:2) all increased the proliferation rate of hNPCs. At the same order of magnitude, butyrate exerted a better effect on neurogenesis (Yang L. L. et al., 2020). In addition, systemic injection of sodium butyrate up-regulated histone hyperacetylation in the brain and liver, upregulating brain-derived neurotrophic factor (BDNF), nerve growth factor, and glial cell, glial cell line-derived neurotrophic factor (GDNF) expression in mouse hippocampus after intracerebroventricular injection of sodium butyrate (Varela et al., 2015). Bill acids have played a unique role in influencing the activities of the central nervous system. Although bile acids may be synthesized locally in the brain, they are mostly absorbed from the systemic circulation. In the brain, cytochrome P450 46A1 (CYP46A1) is involved in cholesterol clearance (Lorbek et al., 2012), this provides raw materials for bile acid synthesis. While bile acids also are synthesized in the liver and secreted to the intestine. After being modified by the gut microbiota, they are transported to the liver through the portal vein. A small amount of bile acids enters the brain through the BBB through the systemic circulation (Monteiro-Cardoso et al., 2021). In this scenario, the gut microbiota influences neurogenesis via the bile acid metabolism pathway. Tauroursodeoxycholic acid promotes NSCs proliferation and differentiation in mouse SGZ, and regulates mitochondrial function and cell cycle signals (Xavier et al., 2014; Soares et al., 2018); this phenomenon may also be related to lipid metabolism (Fernandes et al., 2020). Tauroursodeoxycholic acid produced by modification of gut microbiota regulates NSCs differentiation after blood circulation into the brain (Song et al., 2017; Chen et al., 2020b). The gut microbiota can often provide the host with a source of vitamins, the most common are Vitamin K, Vitamin C, and Vitamin B (folic acid), which may also regulate neurogenesis (Steinert et al., 2020). Compared with the control group, Notch1 and Hes1 expressions were up-regulated and the Mash1 expression was down-regulated after folic acid administration. Folic acid activates Notch signaling, promoting the proliferation of hippocampal NSCs (Zhang et al., 2008; Zhang et al., 2012). Warfarin, an antagonist of Vitamin K, induced the proliferation of SVZ cells, and Vitamin K significantly reversed this phenomenon after the intervention, which may be related to Vitamin K-dependent proteins (Gely-Pernot et al., 2012). Meanwhile, a Vitamin K derivative, methylnaphthoquinone, could also regulate neuronal differentiation of NPCs (Sakane et al., 2017). Vitamin C also appears to play a potential role in regulating neurogenesis, increasing the number of DCX-positive neuroblasts and BrdU+-NeuN+ cells in the hippocampal DG. This is possibly related to the upregulation of BDNF expression (Nam et al., 2019). The tryptophan in dietary proteins is metabolized by metabolic enzymes in the gut microbiota to produce indole, tryptamine, etc. across the intestinal tight junction, or induce enterochromaffin cells (ECCs), enteroendocrine L-cells, etc. to release a series of substances to affect host activities (Roager and Licht, 2018). Compared with the specific pathogen-free control mice group, the content of indole derivatives in the serum of the germ-free (GF) group decreased as did neurogenesis. From this, we can infer that indole has an effect on neurogenesis after crossing the BBB (Wei et al., 2021). Moreover, indole supplementation up-regulated postsynaptic density protein-95 (PSD-95) and synaptophysin (SYP) expression in the hippocampus of WT C57BL/6J mice, while activating the Wnt/β-catenin pathway and up-regulating its downstream targets VEGF and Neurog2 (Wei et al., 2021). SCFAs, indoles, bile acids, and gut microbiota produce and secrete serotonin (5-HT) by affecting ECCs (Benninghoff et al., 2010; Morris et al., 2017; Legan et al., 2022); the 5-HT produced in the gut cannot directly cross the BBB (Legan et al., 2022). However, tryptophan can cross the BBB and serve to synthesize 5-HT in the brain, which stimulates the corresponding receptors in different neurogenic regions to induce proliferation and neurogenesis: 5-HT1A heteroreceptor are involved in DG and SVZ; 5-HT1B autoreceptors modulate the role of 5-HT in SVZ and DG, 5-HT1B heterotypic receptors regulate cell proliferation in SGZ; 5-HT2A and 5-HT2C receptors selectively regulate cell proliferation in SGZ and SVZ. (Banasr et al., 2004; Liu N. et al., 2021). Taken together, some gut microbiota metabolites which cross the BBB could potentially regulate CNS activity, including neurogenesis.
Neuronal pathways involved in the microbiota-gut-brain axis
The neuronal connections of the MGB axis underlie the fastest and most direct brain-gut interaction; the gastrointestinal tract interacts with the brain through the autonomic nervous system, of which the vagus nervous system is the main driver (Bonaz et al., 2018; Morais et al., 2021). The vagus nerve descends through the enteric nervous system to the gut, and enteric ganglia and nerve fibers make up two major plexuses: the submucosal and the myenteric nerve plexis (Wang and Powley, 2007). As a second brain, enteroendocrine cells are the first-order neurons underlying brain-gut interactions (Kaelberer et al., 2018). Gut microbiota metabolites stimulate Enteroendocrine L-cell, ECCs to generate 5-HT, glucagon like peptide-1 (GLP-1), cholecystokinin and other substances, and transmit signals to the enteric nervous system (Bellono et al., 2017; Kuwahara et al., 2020). On the one hand, ECCs secreted peptides/hormones act directly on vagal afferent fibers (Latorre et al., 2016), and on the other hand, secretions from the ECCs communicate with the vagus nerve via the enteric nervous system, intrinsic primary afferent neurons (Kuwahara et al., 2020). The vagus nerve might be involved in neurogenesis as evidenced by the fact that compared with the control group, capsaicin treatment resulted in unmyelinated vagus nerve injury, significantly reducing the number of doublecortin-positive cells in the DG and activated microglia (Ronchi et al., 2012). In another study, transplantation of fecal microbiota from old mice into young mice reduced neurogenesis, a process that may be related to decreased vagal activity (Rei et al., 2022). This suggests that the neuronal pathways of MGB axis could be involved in the regulation of glia and neurogenesis. First, the vagus nerve stimulates microglia activation in pathological conditions. For instance, in AD, the number of microglia branches and total branch length were significantly different under non-invasive vagus nerve stimulation (Kaczmarczyk et al., 2017). At the same time, vagus nerve stimulation down-regulated Toll-like receptor 4 (TLR4) expression in microglia in the acute phase of stroke and promoted microglia polarization to the M2 phenotype (Zhang et al., 2021). In addition, the vagus nerve regulates BDNF content in the brain; after vagotomy, BDNF expression in the hippocampus is down-regulated and hippocampal cell proliferation, neonatal cell survival, and neurogenesis were reduced. Therefore, mouse gut microbiota could induce changes in hippocampal BDNF and affect neurogenesis through the vagal pathway (Bercik et al., 2011; O’Leary et al., 2018). L. rhamnosus (JB-1) feeding upregulates the receptors for γ-aminobutyric acid (GABA) GABAAα2 receptor expression in the DG and CA3 regions (Bravo et al., 2011), while simultaneously down-regulating GABAAα1 receptor expression in DG, CA3, and CA1; this phenomenon was reversed after sub-diaphragmatic vagotomy (Bravo et al., 2011). In general, GABAA activation inhibits cell proliferation, affects migration, maturation and differentiation (Pallotto and Deprez, 2014). At the same time, the dorsal vagal complex of the adult brain stem is a neurogenic region (Bauer et al., 2005; Charrier et al., 2006), the vagus nerve perceives sensory stimulation from gut microbiota and transmits to this region to affect neurogenesis. For example, ghrelin regulates the secretion of growth hormone and energy balance of the dorsal motor nucleus of the vague, and promotes neuronal proliferation, but at present there is controversy about which way to mediate (Zhang et al., 2004; Perello et al., 2022). In addition, vagus nerve stimulation activates the nucleus tractus solitarii; preproglucagon neurons in the nucleus tractus solitarii are the main source of GLP-1 in the brain (Holt et al., 2019; Cooper et al., 2021). The GLP-1 analog (Val8) increases the number of BrdU+ cells in the DG of the hippocampus and DG neurogenesis compared to controls (Mcgovern et al., 2012), as did two other GLP-1 analogs, Exendin-4 and liraglutide (Hamilton et al., 2011). Therefore, the vagus nerve could modulate neurogenesis by affecting central GLP-1 levels.
Immune pathways associated with the microbiota-gut-brain axis
The crosstalk between gut microbiota and immune system is the basis for the connections between the brain and microorganisms through immune pathways. The CNS and gut microbiota can interact through the immune system, which is also affected by the CNS and gut microbiota (Zheng et al., 2020). Some studies show that gut microbiota and metabolites affect brain microglia cells, which may further affect neurogenesis. Compared with the control group, the expression of activated genes such as Mapk8 or Fcgr2β in the microglia of the GF mice group was down-regulated as was B2m gene expression (the MHC class I related β2 microglobulin) and the microglia tended to be immature M0 type (Erny et al., 2015), these reveals the defect of microglia in GF mice; but the conditions were reversed after colonization and SCFAs diet (Erny et al., 2015). Therefore, we think that gut microbe-derived SCFAs enter the systemic circulation and cross the BBB to regulate microglial activity (Huuskonen et al., 2004). The gut microbiota affects BBB permeability as shown by increased BBB permeability in GF mice relative to control mice (Braniste et al., 2014); the expression of occludin and claudin-5 in the GF frontal cortex, striatum and hippocampus was reduced and the number of intact tight junctions significantly reduced (Braniste et al., 2014). In addition, there are other potential mechanisms by which the microbiota regulates microglia and astrocytes, such as microbe-associated molecular patterns, vagus nerve pathways, etc. (Wang Y. et al., 2018). For example, intestinal microbial metabolites such as H2S stimulate the vagus nerve and regulate microglial polarization (Chen et al., 2022; Ni et al., 2022), which regulates NSCs proliferation and differentiation (Shigemoto-Mogami et al., 2014). In some cases, the peripheral immune system can affect the central immune system. Similarly, some peripheral cytokines may enter the CNS through an abnormal BBB, neural route, and immune cell infiltration (Beurel et al., 2020). Therefore, changes in the gut microbiota may modulate the central immune system and glial function and could therefore have various effects on neurogenesis, a hypothesis confirmed by some studies. Antibiotics decreased Ly6Chi monocyte populations and neurogenesis in the mouse brain and bone marrow. These effects were reversed by probiotic treatment (Mohle et al., 2016). This suggests that Ly6Chi monocytes play a mediate between gut microbiota and neurogenesis. IL-4-driven microglia in the CNS activates the BDNF-TrkB pathway to induce neurogenesis in the hippocampus (Zhang J. et al., 2021). IFN-γ injection into the ventricle increased density and area of mouse hippocampal glial cells, activated microglia-mediated neuroinflammation, increased TNF-α, iNOS, etc. (Zhang J. et al., 2020) while inhibiting neural stem/precursor cells (NSPCs) proliferation and promoted the apoptosis of immature neurons (Zhang J. et al., 2020). In addition, the microglial activation-derived miR-146a-5p downregulates kruppel-like factor 4(KLF4) and cyclin-dependentkinase-like 5(CDKL5) expression in the DG region of rats, inhibiting NSCs proliferation and differentiation (Fan et al., 2022). Normal mice transplanted with fecal bacteria from AD mice developed memory impairment and reduced neurogenesis in the hippocampus, while TNF-α and IL-1β increased BDNF expression was downregulation (Kim et al., 2021). Moreover, changes in gut microbiota of mice can lead to increased colitis (Kim et al., 2021), characterized by changes in gut microbiota, like increased abundance of Verrucomicrobia and Proteobacteria. In such a situation, IFN-γ, IL-1β, TNF-α were up-regulated in the distal colon, resulting in microglial activation in adulthood and impaired hippocampal neurogenesis (Salvo et al., 2020). This evidence suggests that alterations in the gut microbiota modulate CNS immunity and alter glial function, which may affect neurogenesis.
Traditional Chinese Medicine can affect neurogenesis by regulating gut microbiota
A potential association between dietary regulation and neurogenesis was found in some randomized controlled trials (Mohamed et al., 2013; Brandhorst et al., 2015; Ashton et al., 2019; Kim et al., 2020) suggests that external factors may regulate neurogenesis through gut microbiota. As TCM can be orally administrated, it inevitably interacts with the gut microbiota, improving TCM oral availability while TCM also impacts the gut microbiota and the MGB axis (Yang et al., 2014). Here we speculate that TCM can regulate neurogenesis under physiological or pathological conditions by remodeling the gut microbiota. Below, we summarize TCM’s effects on the most common types of bacteria in the human body: Bacteroidetes, Firmicutes, Proteobacteria, and Actinobacteria (Figure 5 and Table 3).
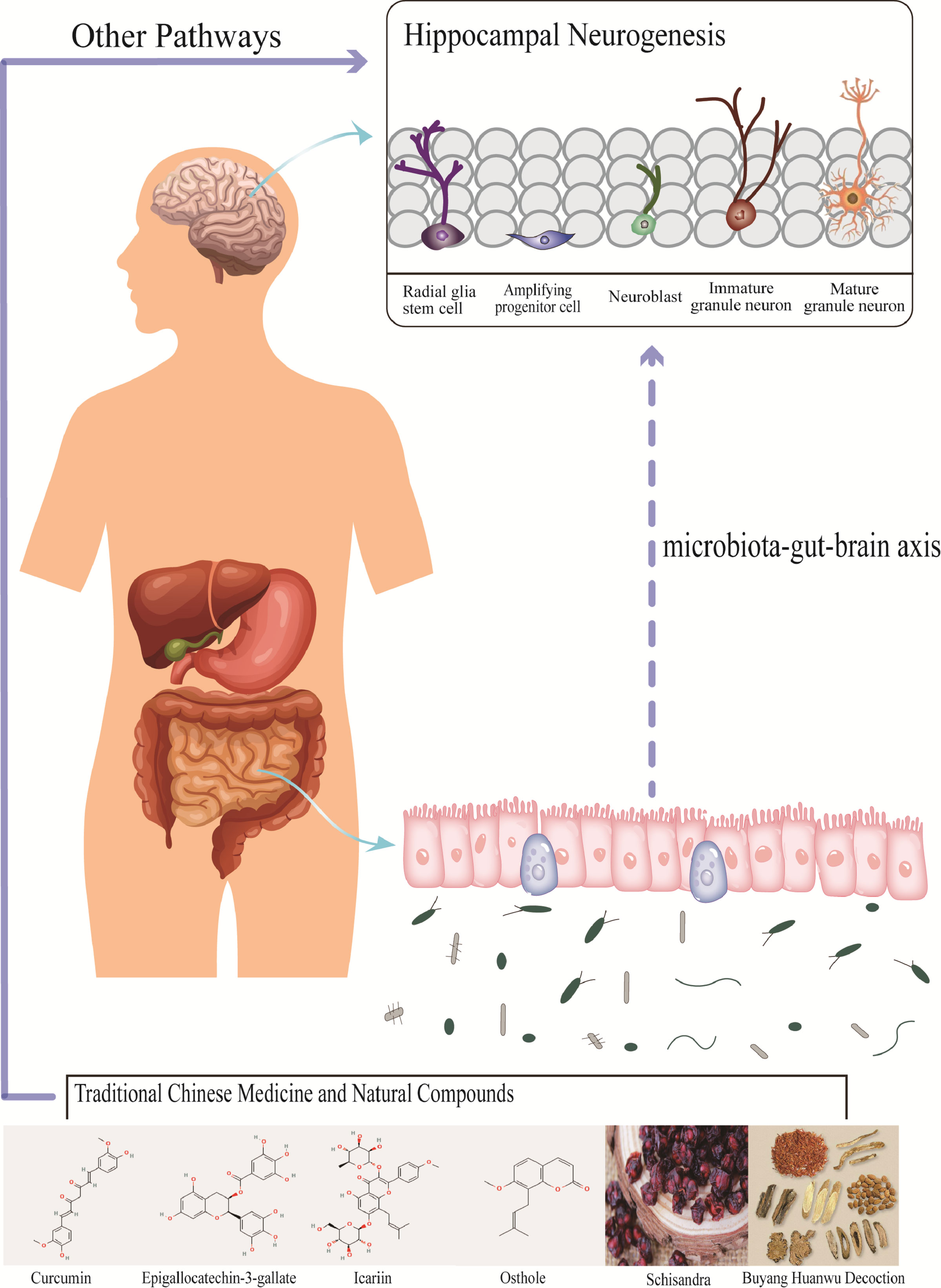
Figure 5 Traditional Chinese medicine and natural compounds affect the abundance of gut microbiota, and change the niche of neural stem cells through the three major pathways of microbiota-gut-brain axis, which may affect neurogenesis. However, there is little evidence that most Traditional Chinese medicine and natural compounds change neurogenesis through this pathway.
Bacteroidetes
Bacteroidetes maintain the balance of gut microbiota and are closely related to CNS diseases. For example, increased Bacteroidetes levels were found in patients with AD, major depressive disorder, and myasthenia gravis (Jiang et al., 2015; Vogt et al., 2017; Moris et al., 2018), and decreased levels in patients with PD (Unger et al., 2016). At the same time, changes in Bacteroidetes abundance are accompanied by changes in microglia, astrocytes, etc. (Shi et al., 2021). This suggests that Bacteroidetes may regulate NSCs and neurogenesis. Curcumin treatment corrected the relative abundance of Bacteroidetes in a mouse anxiety model, increasing SCFAs content in the feces, affecting the glycerophospholipid metabolism in the prefrontal cortex, possibly by regulating circulatory pathways (Zhang et al., 2022). In another study, cAMP-response element binding protein activation enhanced BDNF expression by increasing glycerophospholipids (Hossain et al., 2022). Meanwhile, Curcumin intervention up-regulated 5-HT(1a) receptor and BDNF, increasing neurogenesis (Xu et al., 2007). This suggests that curcumin may modulate neurogenesis by affecting chemical pathways in the MGB axis by modulating Bacteroidetes activity. In addition, Scutellaria baicalensis Georgi also exerts similar pharmacological effects in brain injuries induced by diabetes, mice treated with Astragalus membranaceus reduced Bacteroidetes abundance, upregulation of BDNF expression and promotion of hippocampal mitogenesis (Li X. et al., 2022). Baicalin, an effective component of Scutellaria baicalensis Georgi, reduces the abundance of parabacteroides, prevotella, and Bacteroides in mouse intestine, reducing the proinflammatory factors IL-1β, IL-6 and TNF-α in the cerebral cortex (Gao et al., 2018b); in the DG, it promotes cell proliferation and differentiation, and upregulates hippocampal Wnt/β-catenin signaling pathway related protein expression (Xiao et al., 2021). This suggests that the gut microbiota may influence neurogenesis by affecting brain inflammation. Similarly, Astragalus and its active components affect neurogenesis through MGB axis immune pathways, which may be related to microglia activation (Li et al., 2020), but the specific mechanism needs to be further confirmed. In vitro, Astragaloside VI promotes the differentiation of primary NSCs into neurons and astrocytes, similarly to Astragalus flavor, which up-regulated Notch1, Jagged1, Mash1, Ngn1, and Ngn2 expression in NSCs (Gao et al., 2022). Epigallo-catechin-3-gallate (EGCG), the main component of Camellia sinensis (green tea), increases the abundance of Bacteroides uniformis, Bacteroides vulgatus, Bacteroides stercoris, Bacteroides thetaiotaomicron, and Bacteroides cellulosilyticus in human gut microbiota (Liu Z. et al., 2020). In preclinical studies, EGCG enhanced hippocampal neurogenesis, improved learning and memory in mice, and upregulated SHH, Ptch and Gli1 expression in the mouse hippocampus (Wang et al., 2012). Crossing the intestinal epithelial barrier and the BBB regulates hippocampal neural differentiation after EGCG interacts with the gut microbiota (Faria et al., 2011; Granja et al., 2019). Qisheng Wan formula increased Bacteroidetes abundance in AD mice while reducing NF-κB, IL-6, and TNF-α content (Xiong et al., 2022); one of its active ingredients, Polygala tenuifolia, promotes APP-NSCs proliferation and migration and neuronal differentiation (Wang X. F. et al., 2021), while another ingredient, Acorus tatarinowii Schott, also affects neurogenesis, microglial function and can activate PKA-CREB signaling, enhancing nerve growth factor induced differentiation and neurite length of PC12 cells (Cai et al., 2016; Lam et al., 2016). Lipopolysaccharide, amyloids, and other substances produced by Bacteroidetes affect neuroinflammation, possibly by regulating the brain inflammatory microenvironment to regulate neurogenesis (Zhao and Lukiw, 2018), as confirmed by the effects of TCM presented above. In summary, most TCM compounds regulate neurogenesis by interfering with the chemical and immune pathways of the MGB axis, accompanied by changes in Bacteroidetes abundance.
Firmicutes
Firmicutes transplantation can be used in the treatment of brain diseases. Lactobacillus, as a unique Firmicutes of bacteria, is one of the important microbiota for maintaining the micro-ecological health of the human gut, some studies have found increased 5-HT and BDNF concentration in the serum of constipated patients who received Lactobacillus reuteri DSM-17938 (Riezzo et al., 2019), Lactobacillus rhamnosus HN001 could effectively prevent and treat postpartum depression (Slykerman et al., 2017). This evidence indicates that Firmicutes may regulate brain activity and treatment of central nervous system diseases and injuries. Using a Buyang Huanwu Decoction (BHD) in cerebellar ischemia model mice significantly down-regulated the relative abundance of Streptococcus, Coprococcus_2, Enterococcus, etc, and while up-regulating the relative abundance of Lactobacillus, Ruminococcaceae_UCG-002, etc. (Tang R. et al., 2022). This lowered the levels of proinflammatory cytokines in peripheral blood and inhibited microglial activation (Tang R. et al., 2022). At the same time, BHD promoted NSCs proliferation in middle cerebellar artery occlusion mice and promoted astrocyte and neuronal differentiation, which may be related to Jak/Stat3/Cyclin D1 and EGFR/PI3K/Akt/Bad/14-3-3 pathways (Chen X. et al., 2020). The altered abundance of Firmicutes after BHD intervention may affect peripheral and central immunity, thus regulating NSCs proliferation and differentiation. In addition, curcumin promoted hippocampal neurogenesis and enhanced NSCs proliferation in APP/PS1 mice, up-regulating Hes1 and NICD expression and activating the Notch pathway (Li et al., 2019). In the gut of APP/PS1 mice, curcumin down-regulated the abundance of Lactobacillaceae, producing substances such as demethylcurcumin and bisdemethoxycurcumin which alleviate the pathological changes of AD (Sun et al., 2020). Changes in the gut microbiota may modulate gene expression in neurogenesis-related pathways; however, there is currently no clear evidence for the specific mechanisms. In some mental diseases, curcumin increases the content of 5-HT, BDNF, etc. in the hippocampus, and modulates related signaling changes in the peripheral intestinal system (Yu et al., 2015); this may be related to the hypothalamic-pituitary-adrenal (HPA) axis of the MGB axis, as curcumin reduces the ratio of adrenal gland weight to body weight and adrenal cortex thickness, down-regulating serum corticosterone levels and up-regulating hippocampal glucocorticoid receptor expression (Xu et al., 2006). In the SGZ, andrographolide promotes NSCs proliferation and neurogenesis, up-regulating hippocampal β-catenin expression, inhibiting GSK-3β activity, and up-regulating the expression of Wnt target gene NeuroD1 (Varela-Nallar et al., 2015). Moreover, Andrographolide increased the abundance of Firmicutes in the gut, significantly reducing neuroinflammation in mouse brain tissue and enhancing BDNF expression (Saha et al., 2021). This suggests that Andrographolide may affect BDNF expression through the MGB axis, affecting Wnt signaling in NSCs (Yang et al., 2016; Li et al., 2017), and may be involved in neuroinflammation. Increased abundance of intestinal Firmicutes in AD mice after Ginkgolide B treatment could improve AD pathology and cognitive dysfunction (Liu J. et al., 2021). In vitro, Ginkgolide B promotes NSCs neuronal differentiation in the SVZ, activates the Wnt/β-catenin pathway, and upregulates the target gene Axin2 (Li et al., 2018). This may be related to the Firmicutes regulating the permeability of the BBB and promoting Ginkgolide B to cross the BBB to regulate hippocampal activity (Lin et al., 2022). In addition, Resveratrol packaged with selenium nanomaterials (TGN-Res@SeNPs) reversed the alterations of Firmicutes in AD, increasing Lactobacillus, Lachnospiraceae_NK4A136_group, etc (Li et al., 2021); after treatment, levels of neurotransmitters such as glutamate and GABA recovered in the hippocampus of mice, increased total antioxidant capacity (T-AOC), CAT, GSH-Px, and IL-10 levels in mouse brain tissue and decreased MDA, IL-1β, IL-6, TNF-α (Li et al., 2021). This may be because Resveratrol modulates the abundance of Firmicutes, such as Lachnospiraceae_NK4A136_group, which are closely related to inflammation and oxidation and may affect neurogenesis by altering the microenvironment (Li and Barres, 2018). A high-fat diet can damage cognitive function, which may be related to neuroinflammation (Huang and Yun, 2020). When high-fat diet mice were treated with a water extract from a processed Polygonum multiflorum modulate, a significant decrease in the relative abundance of Firmicutes was observed (Gu et al., 2020); in addition, Polygonum multiflorum Thunberg complex orally Composition-12 (PMC-12) promoted cell proliferation and neuronal differentiation in the DG and significantly up-regulated BDNF and p-CREB expression in the hippocampus. However, the underlying mechanism by which Polygonum multiflorum affects the MGB axis remains unclear (Park et al., 2016).
Proteobacteria
Changes in Proteobacteria can predict changes in the total brain and cortex of rhesus monkeys in infancy (Rendina et al., 2021). Proteobacteria levels differ between healthy individuals, and those with mild cognitive impairment and AD; these difference gradually increase with disease deterioration (Guo et al., 2020). The density of DCX-positive cells in the DG of Bilophila wadsworthia (Belongs to Proteobacteria) colonized mice decreased, showing a similar effect on mouse hippocampus to that of a ketogenic diet, affecting hippocampal neurogenesis (Olson et al., 2021). Berberine, a TCM compound, could increase the abundance of dopa/dopamine producing bacteria such as Escherichia-Shigella and Pseudomonas (Wang Y. et al., 2021). Berberine neuronal differentiation of C17.2 NSCs in vitro, upregulates Nestin, microtubule-associated protein 2 (MAP2) and tubulin β 3 Class III (TUBB3) expression, this indicates that the proliferation and neuronal differentiation of NSCs are promoted after the intervention. This may be closely related to the activation of Wnt/β-catenin pathway (Shou et al., 2019). Berberine might affect central dopamine production by regulating Proteobacteria abundance, further affecting Wnt/β-catenin through Dopamine D2 receptor to promote neurogenesis in PD (Marchetti et al., 2022). Ginsenoside Rg1 was shown to reduce tau content in the hippocampus of AD model and upregulated Proteobacteria abundance (Wang S. et al., 2020), promoting NSCs growth and proliferation, down-regulating β-catenin, C-myc expression and upregulating GSK-3β expression (Xiang et al., 2019). This may be caused by changes in bacterial tryptophan metabolism, reducing peripheral blood 5-HT levels and affecting hippocampal neurotransmitters, thereby regulating NSCs proliferation and differentiation (Chen et al., 2022); however, the specific mechanism warrants further research. Similarly, BHD decreased the abundance of Escherichia-Shigella and Klebsiella (Tang R. et al., 2022). The combination of Puerariae Lobatae Radix (PLR) and Chuanxiong Rhizoma (CXR) could partially reduce the enrichment of intestinal pathogens Escherichia_Shigella, Proteus, Klebsiella, etc. in a mouse model of ischemic stroke (Chen R. et al., 2019). Plasma fluorescein isothiocyanate-dextran concentrations and intestinal permeability decreased, and claudin-5 and ZO-1 levels in the brain and colon returned to normal levels after this treatment. This suggests that PLR and CXR can prevent brain gut-barrier disruption caused by ischemic stroke (Chen R. et al., 2019). Moreover, Rhizome Chuanxiong and the other three TCM compounds could promote NSCs proliferation in vitro, and also promote the proliferation of hippocampus in vivo, while inhibiting hyperactivity of the HPA axis (Pao et al., 2012). This suggests that the Chuanxiong may affect neurogenesis through the gut microbiota and HPA axis, but this needs further confirmation.
Actinobacteria
Actinobacteria levels are low in the human intestine, but they are extremely important to maintain intestinal homeostasis. Differences in Actinobacteria emerge early in infancy, arising particularly between cesarean and vaginal births (Gronlund et al., 1999; Kabeerdoss et al., 2013). In a clinical trial, transplantation of Bifidobacterium longum NCC3001 of Actinobacteria improved depressive manifestations and improved quality of life in patients with irritable bowel syndrome, which was associated with altered amygdala and frontal limbic activity (Pinto-Sanchez et al., 2017). This suggests that Actinobacteria could affect neurogenesis. Ginsenoside Rg3, one of the main components of red ginseng, is fermented and metabolized to ginsenoside Rd in the intestine by Bifidobacterium. After treatment with ginsenoside Rg3, serum corticosterone and adrenal cortical hormones were reduced. In the brain, 5-HT was up-regulated and norepinephrine content was reduced, leading to increased expression of central BDNF (Han et al., 2020; Sur and Lee, 2022). Simultaneously, ginsenosides Rd promoted the proliferation of hippocampal NSCs but Andrographolide but did not affect differentiation (Lin et al., 2012). Therefore, we speculate that red ginseng regulates central neurotransmitters and BDNF through the HPA axis under the action of intestinal Actinobacteria, which further affects NSCs differentiation. Paeoniflorin modulates gut microbiota in depressive rats, upregulating Roseburia abundance, and has antidepressant effects; the gut microbiota metabolizes paeoniflorin to produce benzoic acid, which can cross the BBB to regulate the CNS (Yu et al., 2019). Paeoniflorin up-regulated BDNF expression, enhanced NSCs proliferation in the hippocampus and promoted astrocyte differentiation (Chen L B. et al., 2019). Sodium benzoate can increase BDNF expression in neurons in a dose-dependent and time-dependent manner (Jana et al., 2013; Guo et al., 2020). This suggests that the benzoic acid produced by Actinobacteria metabolization of paeoniflorin may directly regulate neurogenesis. At present, there are few studies on the effects of TCM on Actinobacteria, but their role in regulating neurogenesis warrants further research.
Conclusions and future prospects
Accumulating evidence suggests that impaired neurogenesis exist in pathological states of the CNS (Koh and Park, 2017; Berger et al., 2020). Neurogenesis, including NSCs growth, development, and differentiation in the hippocampus is tightly regulated by Notch, Wnt, and the extracellular matrix. The regulation of these pathways is affected by external factors, so it can regulate neurogenesis by regulating external stimuli. Based on this theory, gut microbiota plays a potential role in neurogenesis.
Some studies have found that the hippocampus is sensitive to diet (Hueston et al., 2017; Davidson and Stevenson, 2022); since changes in dietary habits and nutrition directly affect gut microbiota activity, this may in turn affect hippocampal activity. TCM is often taken orally through the digestive system. Accordingly, TCM could modulate hippocampal activity through the gut microbiota.
Numerous clinical and preclinical studies provide robust data on the microbiome. The MGB axis allows gut-brain interactions: the gut microbiota regulates CNS development and function through neural, immune, metabolic, and other pathways through the MGB axis. The way of administration of TCM plays a huge advantage in regulating gut microbiota. Therefore, we speculate that TCM regulates gut microbiota abundance, affects MGB axis, and further exerts the pharmacological effect of CNS.
In this review, we highlighted the role of gut microbiota on neurogenesis through the MGB axis and summarized the potential of TCM for modulating the CNS through this pathway. Even if the relevant mechanism has not been fully and systematically investigated, existing data have closely linked both phenomena. The connection between neurogenesis and the gastrointestinal tract may lead to strategies to treat CNS diseases by targeting gut-brain interactions. In addition, the connection between neural activity and systemic metabolism also provides a new research direction for neurogenesis. In-depth research on this topic not only provides suggestions and guidelines for TCM therapy for some neurological diseases, but also improves the theory of neurodevelopment.
Author contributions
CZ, PX and XL provided the writing of articles. HuiZ, CT, SZ organized tables and figures, HaiZ, WL, and LS revised the article and put forward key suggestions. JW, BZ and WL provided financial support. All authors contributed to the article and approved the submitted version.
Funding
This study was supported by the Scientific Research Projectsof Provincial Colleges and Universities in Heilongjiang Province (2021-KYYWF-0379) and Clinical Research Fund of Qiqihar Academy of Medical Sciences (QMSI2021L-03).
Conflict of interest
The authors declare that the research was conducted in the absence of any commercial or financial relationships that could be construed as a potential conflict of interest.
Publisher’s note
All claims expressed in this article are solely those of the authors and do not necessarily represent those of their affiliated organizations, or those of the publisher, the editors and the reviewers. Any product that may be evaluated in this article, or claim that may be made by its manufacturer, is not guaranteed or endorsed by the publisher.
References
Aberg, D. (2010). Role of the growth hormone/insulin-like growth factor 1 axis in neurogenesis. Endocr. Dev. 17, 63–76. doi: 10.1159/000262529
Altman, J., Das, G. D. (1965). Autoradiographic and histological evidence of postnatal hippocampal neurogenesis in rats. J. Comp. Neurol. 124 (3), 319–335. doi: 10.1002/cne.901240303
Alvarez-Palomo, A. B., Requena-Osete, J., Delgado-Morales, R., Moreno-Manzano, V., Grau-Bove, C., Tejera, A. M., et al. (2021). A synthetic mRNA cell reprogramming method using CYCLIN D1 promotes DNA repair, generating improved genetically stable human induced pluripotent stem cells. Stem Cells 39 (7), 866–881. doi: 10.1002/stem.3358
Andreotti, J. P., Silva, W. N., Costa, A. C., Picoli, C. C., Bitencourt, F., Coimbra-Campos, L., et al. (2019). Neural stem cell niche heterogeneity. Semin. Cell Dev. Biol. 95, 42–53. doi: 10.1016/j.semcdb.2019.01.005
Androutsellis-Theotokis, A., Leker, R. R., Soldner, F., Hoeppner, D. J., Ravin, R., Poser, S. W., et al. (2006). Notch signalling regulates stem cell numbers in vitro and in vivo. Nature 442 (7104), 823–826. doi: 10.1038/nature04940
Angot, E., Loulier, K., Nguyen-Ba-Charvet, K. T., Gadeau, A. P., Ruat, M., Traiffort, E. (2008). Chemoattractive activity of sonic hedgehog in the adult subventricular zone modulates the number of neural precursors reaching the olfactory bulb. Stem Cells 26 (9), 2311–2320. doi: 10.1634/stemcells.2008-0297
Ashton, M. M., Berk, M., Ng, C. H., Hopwood, M., Dodd, S., Turner, A., et al. (2019). Efficacy of adjunctive garcinia mangostana Linn (mangosteen) pericarp for bipolar depression: Study protocol for a proof-of-concept trial. Braz. J. Psychiatry 41 (3), 245–253. doi: 10.1590/1516-4446-2018-0114
Banasr, M., Hery, M., Printemps, R., Daszuta, A. (2004). Serotonin-induced increases in adult cell proliferation and neurogenesis are mediated through different and common 5-HT receptor subtypes in the dentate gyrus and the subventricular zone. Neuropsychopharmacol 29 (3), 450–460. doi: 10.1038/sj.npp.1300320
Bao, H., Asrican, B., Li, W., Gu, B., Wen, Z., Lim, S. A., et al. (2017). Long-range GABAergic inputs regulate neural stem cell quiescence and control adult hippocampal neurogenesis. Cell Stem Cell. 21 (5), 604–617.e5. doi: 10.1016/j.stem.2017.10.003
Bauer, S., Hay, M., Amilhon, B., Jean, A., Moyse, E. (2005). In vivo neurogenesis in the dorsal vagal complex of the adult rat brainstem. Neuroscience 130 (1), 75–90. doi: 10.1016/j.neuroscience.2004.08.047
Bellono, N. W., Bayrer, J. R., Leitch, D. B., Castro, J., Zhang, C., O’Donnell, T. A., et al. (2017). Enterochromaffin cells are gut chemosensors that couple to sensory neural pathways. Cell 170 (1), 185–198.e16. doi: 10.1016/j.cell.2017.05.034
Benninghoff, J., Gritti, A., Rizzi, M., Lamorte, G., Schloesser, R. J., Schmitt, A., et al. (2010). Serotonin depletion hampers survival and proliferation in neurospheres derived from adult neural stem cells. Neuropsychopharmacol 35 (4), 893–903. doi: 10.1038/npp.2009.181
Bercik, P., Denou, E., Collins, J., Jackson, W., Lu, J., Jury, J., et al. (2011). The intestinal microbiota affect central levels of brain-derived neurotropic factor and behavior in mice. Gastroenterology 141 (2), 599–609, 609.e1-3. doi: 10.1053/j.gastro.2011.04.052
Berger, T., Lee, H., Young, A. H., Aarsland, D., Thuret, S. (2020). Adult hippocampal neurogenesis in major depressive disorder and alzheimer’s disease. Trends Mol. Med. 26 (9), 803–818. doi: 10.1016/j.molmed.2020.03.010
Beurel, E., Toups, M., Nemeroff, C. B. (2020). The bidirectional relationship of depression and inflammation: Double trouble. Neuron 107 (2), 234–256. doi: 10.1016/j.neuron.2020.06.002
Bonaguidi, M. A., Peng, C. Y., Mcguire, T., Falciglia, G., Gobeske, K. T., Czeisler, C., et al. (2008). Noggin expands neural stem cells in the adult hippocampus. J. Neurosci. 28 (37), 9194–9204. doi: 10.1523/JNEUROSCI.3314-07.2008
Bonaz, B., Bazin, T., Pellissier, S. (2018). The vagus nerve at the interface of the microbiota-Gut-Brain axis. Front. Neurosci. 12. doi: 10.3389/fnins.2018.00049
Bond, A. M., Bhalala, O. G., Kessler, J. A. (2012). The dynamic role of bone morphogenetic proteins in neural stem cell fate and maturation. Dev. Neurobiol. 72 (7), 1068–1084. doi: 10.1002/dneu.22022
Bottes, S., Jaeger, B. N., Pilz, G. A., Jorg, D. J., Cole, J. D., Kruse, M., et al. (2021). Long-term self-renewing stem cells in the adult mouse hippocampus identified by intravital imaging. Nat. Neurosci. 24 (2), 225–233. doi: 10.1038/s41593-020-00759-4
Brandhorst, S., Choi, I. Y., Wei, M., Cheng, C. W., Sedrakyan, S., Navarrete, G., et al. (2015). A periodic diet that mimics fasting promotes multi-system regeneration, enhanced cognitive performance, and healthspan. Cell Metab. 22 (1), 86–99. doi: 10.1016/j.cmet.2015.05.012
Braniste, V., Al-Asmakh, M., Kowal, C., Anuar, F., Abbaspour, A., Toth, M., et al. (2014). The gut microbiota influences blood-brain barrier permeability in mice. Sci. Transl. Med. 6 (263), 263ra158. doi: 10.1126/scitranslmed.3009759
Bravo, J. A., Forsythe, P., Chew, M. V., Escaravage, E., Savignac, H. M., Dinan, T. G., et al. (2011). Ingestion of lactobacillus strain regulates emotional behavior and central GABA receptor expression in a mouse via the vagus nerve. Proc. Natl. Acad. Sci. U. S. A. 108 (38), 16050–16055. doi: 10.1073/pnas.1102999108
Briscoe, J., Therond, P. P. (2013). The mechanisms of hedgehog signalling and its roles in development and disease. Nat. Rev. Mol. Cell Biol. 14 (7), 416–429. doi: 10.1038/nrm3598
Bryant, R. J., Bentley, R. (1976). Menaquinone biosynthesis: conversion of o-succinylbenzoic acid to 1,4-dihydroxy-2-naphthoic acid and menaquinones by escherichia coli extracts. Biochemistry-US 15 (22), 4792–4796. doi: 10.1021/bi00667a007
Butler, M. T., Wallingford, J. B. (2017). Planar cell polarity in development and disease. Nat. Rev. Mol. Cell Biol. 18 (6), 375–388. doi: 10.1038/nrm.2017.11
Cai, Q., Li, Y., Mao, J., Pei, G. (2016). Neurogenesis-promoting natural product alpha-asarone modulates morphological dynamics of activated microglia. Front. Cell. Neurosci. 10. doi: 10.3389/fncel.2016.00280
Cai, N. N., Wang, Z. Z., Zhu, X. C., Jiang, Y., Zhu, W. Q., Yang, R., et al. (2020). Schisandrin a and b enhance the dentate gyrus neurogenesis in mouse hippocampus. J. Chem. Neuroanat. 105, 101751. doi: 10.1016/j.jchemneu.2020.101751
Carballo, G. B., Honorato, J. R., de Lopes, G., Spohr, T. (2018). A highlight on sonic hedgehog pathway. Cell Commun. Signal. 16 (1), 11. doi: 10.1186/s12964-018-0220-7
Charrier, C., Coronas, V., Fombonne, J., Roger, M., Jean, A., Krantic, S., et al. (2006). Characterization of neural stem cells in the dorsal vagal complex of adult rat by in vivo proliferation labeling and in vitro neurosphere assay. Neuroscience 138 (1), 5–16. doi: 10.1016/j.neuroscience.2005.10.046
Chen, X., Chen, H., He, Y., Fu, S., Liu, H., Wang, Q., et al. (2020a). Proteomics-guided study on buyang huanwu decoction for its neuroprotective and neurogenic mechanisms for transient ischemic stroke: Involvements of EGFR/PI3K/Akt/Bad/14-3-3 and Jak2/Stat3/Cyclin D1 signaling cascades. Mol. Neurobiol. 57 (10), 4305–4321. doi: 10.1007/s12035-020-02016-y
Chen, H., Feng, Z., Min, L., Deng, W., Tan, M., Hong, J., et al. (2022). Vagus nerve stimulation reduces neuroinflammation through microglia polarization regulation to improve functional recovery after spinal cord injury. Front. Neurosci. 16. doi: 10.3389/fnins.2022.813472
Chen, Z., Lin, Y., Zhou, Q., Xiao, S., Li, C., Lin, R., et al. (2022). Ginsenoside Rg1 mitigates morphine dependence via regulation of gut microbiota, tryptophan metabolism, and serotonergic system function. Biomed. Pharmacother. 150, 112935. doi: 10.1016/j.biopha.2022.112935
Chen, L. B., Qiu, F. M., Zhong, X. M., Hong, C., Huang, Z. (2019). Promoting neurogenesis in hippocampal dentate gyrus of chronic unpredictable stress-induced depressive-like rats with paeoniflorin. J. Integr. Neurosci. 18 (1), 43–49. doi: 10.31083/j.jin.2019.01.116
Chen, X., Wang, J., Gao, X., Wu, Y., Gu, G., Shi, M., et al. (2020b). Tauroursodeoxycholic acid prevents ER stress-induced apoptosis and improves cerebral and vascular function in mice subjected to subarachnoid hemorrhage. Brain Res. 1727, 146566. doi: 10.1016/j.brainres.2019.146566
Chen, R., Wu, P., Cai, Z., Fang, Y., Zhou, H., Lasanajak, Y., et al. (2019). Puerariae lobatae radix with chuanxiong rhizoma for treatment of cerebral ischemic stroke by remodeling gut microbiota to regulate the brain-gut barriers. J. Nutr. Biochem. 65, 101–114. doi: 10.1016/j.jnutbio.2018.12.004
Christopherson, K. S., Ullian, E. M., Stokes, C. C., Mullowney, C. E., Hell, J. W., Agah, A., et al. (2005). Thrombospondins are astrocyte-secreted proteins that promote CNS synaptogenesis. Cell 120 (3), 421–433. doi: 10.1016/j.cell.2004.12.020
Clevers, H., Loh, K. M., Nusse, R. (2014). Stem cell signaling. an integral program for tissue renewal and regeneration: Wnt signaling and stem cell control. Science 346 (6205), 1248012. doi: 10.1126/science.1248012
Cooper, C. M., Farrand, A. Q., Andresen, M. C., Beaumont, E. (2021). Vagus nerve stimulation activates nucleus of solitary tract neurons via supramedullary pathways. J. Physiol. 599 (23), 5261–5279. doi: 10.1113/JP282064
Cryan, J. F., O’Riordan, K. J., Cowan, C., Sandhu, K. V., Bastiaanssen, T., Boehme, M., et al. (2019). The microbiota-Gut-Brain axis. Physiol. Rev. 99 (4), 1877–2013. doi: 10.1152/physrev.00018.2018
Dalile, B., Van Oudenhove, L., Vervliet, B., Verbeke, K. (2019). The role of short-chain fatty acids in microbiota-gut-brain communication. Nat. Rev. Gastroenterol. Hepatol. 16 (8), 461–478. doi: 10.1038/s41575-019-0157-3
Davidson, T. L., Stevenson, R. J. (2022). Appetitive interoception, the hippocampus and western-style diet. Rev. Endocr. Metab. Disord. 23 (4), 845–859. doi: 10.1007/s11154-021-09698-2
Dong, J., Pan, Y. B., Wu, X. R., He, L. N., Liu, X. D., Feng, D. F., et al. (2019). A neuronal molecular switch through cell-cell contact that regulates quiescent neural stem cells. Sci. Adv. 5 (2), eaav4416. doi: 10.1126/sciadv.aav4416
Engevik, M. A., Luck, B., Visuthranukul, C., Ihekweazu, F. D., Engevik, A. C., Shi, Z., et al. (2021). Human-derived bifidobacterium dentium modulates the mammalian serotonergic system and gut-brain axis. Cell Mol. Gastroenterol. Hepatol. 11 (1), 221–248. doi: 10.1016/j.jcmgh.2020.08.002
Erny, D., Hrabe, D. A. A., Jaitin, D., Wieghofer, P., Staszewski, O., David, E., et al. (2015). Host microbiota constantly control maturation and function of microglia in the CNS. Nat. Neurosci. 18 (7), 965–977. doi: 10.1038/nn.4030
Erturk-Hasdemir, D., Ochoa-Reparaz, J., Kasper, D. L., Kasper, L. H. (2021). Exploring the gut-brain axis for the control of CNS inflammatory demyelination: Immunomodulation by bacteroides fragilis’ polysaccharide a. Front. Immunol. 12. doi: 10.3389/fimmu.2021.662807
Fan, C., Li, Y., Lan, T., Wang, W., Long, Y., Yu, S. Y. (2022). Microglia secrete miR-146a-5p-containing exosomes to regulate neurogenesis in depression. Mol. Ther. 30 (3), 1300–1314. doi: 10.1016/j.ymthe.2021.11.006
Faria, A., Pestana, D., Teixeira, D., Couraud, P. O., Romero, I., Weksler, B., et al. (2011). Insights into the putative catechin and epicatechin transport across blood-brain barrier. Food Funct. 2 (1), 39–44. doi: 10.1039/c0fo00100g
Fasina, O. B., Wang, J., Mo, J., Osada, H., Ohno, H., Pan, W., et al. (2022). Gastrodin from gastrodia elata enhances cognitive function and neuroprotection of AD mice via the regulation of gut microbiota composition and inhibition of neuron inflammation. Front. Pharmacol. 13. doi: 10.3389/fphar.2022.814271
Feng, W., Ao, H., Peng, C., Yan, D. (2019). Gut microbiota, a new frontier to understand traditional Chinese medicines. Pharmacol. Res. 142, 176–191. doi: 10.1016/j.phrs.2019.02.024
Fernandes, M. B., Costa, M., Ribeiro, M. F., Siquenique, S., Sa, S. S., Martins, J., et al. (2020). Reprogramming of lipid metabolism as a new driving force behind tauroursodeoxycholic acid-induced neural stem cell proliferation. Front. Cell Dev. Biol. 8. doi: 10.3389/fcell.2020.00335
Ferron, S. R., Charalambous, M., Radford, E., Mcewen, K., Wildner, H., Hind, E., et al. (2011). Postnatal loss of Dlk1 imprinting in stem cells and niche astrocytes regulates neurogenesis. Nature 475 (7356), 381–385. doi: 10.1038/nature10229
Fredrich, M., Hampel, M., Seidel, K., Christ, E., Korf, H. W. (2017). Impact of melatonin receptor-signaling on zeitgeber time-dependent changes in cell proliferation and apoptosis in the adult murine hippocampus. Hippocampus 27 (5), 495–506. doi: 10.1002/hipo.22706
Fuentealba, L. C., Rompani, S. B., Parraguez, J. I., Obernier, K., Romero, R., Cepko, C. L., et al. (2015). Embryonic origin of postnatal neural stem cells. Cell 161 (7), 1644–1655. doi: 10.1016/j.cell.2015.05.041
Galvin, K. E., Ye, H., Erstad, D. J., Feddersen, R., Wetmore, C. (2008). Gli1 induces G2/M arrest and apoptosis in hippocampal but not tumor-derived neural stem cells. Stem Cells 26 (4), 1027–1036. doi: 10.1634/stemcells.2007-0879
Gao, L., Huang, P., Dong, Z., Gao, T., Huang, S., Zhou, C., et al. (2018a). Modified xiaoyaosan (MXYS) exerts anti-depressive effects by rectifying the brain blood oxygen level-dependent fMRI signals and improving hippocampal neurogenesis in mice. Front. Pharmacol. 9. doi: 10.3389/fphar.2018.01098
Gao, H., Huang, N., Wang, W., Zhang, L., Cai, L., Chen, M., et al. (2022). Astragalus flavone induces proliferation and differentiation of neural stem cells in a cerebral infarction model. Med. Sci. Monit. 28, e933830. doi: 10.12659/MSM.933830
Gao, J., Liao, Y., Qiu, M., Shen, W. (2021). Wnt/beta-catenin signaling in neural stem cell homeostasis and neurological diseases. Neuroscientist 27 (1), 58–72. doi: 10.1177/1073858420914509
Gao, L., Li, J., Zhou, Y., Huang, X., Qin, X., Du, G. (2018b). Effects of baicalein on cortical proinflammatory cytokines and the intestinal microbiome in senescence accelerated mouse prone 8. ACS Chem. Neurosci. 9 (7), 1714–1724. doi: 10.1021/acschemneuro.8b00074
Garcia-Velazquez, L., Arias, C. (2017). The emerging role of wnt signaling dysregulation in the understanding and modification of age-associated diseases. Ageing Res. Rev. 37, 135–145. doi: 10.1016/j.arr.2017.06.001
Gely-Pernot, A., Coronas, V., Harnois, T., Prestoz, L., Mandairon, N., Didier, A., et al. (2012). An endogenous vitamin K-dependent mechanism regulates cell proliferation in the brain subventricular stem cell niche. Stem Cells 30 (4), 719–731. doi: 10.1002/stem.1045
Gershon, M. D., Margolis, K. G. (2021). The gut, its microbiome, and the brain: connections and communications. J. Clin. Invest. 131 (18), e143768. doi: 10.1172/JCI143768
Gonzalez-Reyes, L. E., Chiang, C. C., Zhang, M., Johnson, J., Arrillaga-Tamez, M., Couturier, N. H., et al. (2019). Sonic hedgehog is expressed by hilar mossy cells and regulates cellular survival and neurogenesis in the adult hippocampus. Sci. Rep. 9 (1), 17402. doi: 10.1038/s41598-019-53192-4
Granja, A., Neves, A. R., Sousa, C. T., Pinheiro, M., Reis, S. (2019). EGCG intestinal absorption and oral bioavailability enhancement using folic acid-functionalized nanostructured lipid carriers. Heliyon 5 (7), e02020. doi: 10.1016/j.heliyon.2019.e02020
Gronlund, M. M., Lehtonen, O. P., Eerola, E., Kero, P. (1999). Fecal microflora in healthy infants born by different methods of delivery: permanent changes in intestinal flora after cesarean delivery. J. Pediatr. Gastroenterol. Nutr. 28 (1), 19–25. doi: 10.1097/00005176-199901000-00007
Guo, F., Zhang, Z., Liang, Y., Yang, R., Tan, Y. (2020). Exploring the role and mechanism of sodium benzoate in CUMS-induced depression model of rats. Neuro Endocrinol. Lett. 41 (4), 205–212.
Gu, W., Yang, M., Bi, Q., Zeng, L. X., Wang, X., Dong, J. C., et al. (2020). Water extract from processed polygonum multiflorum modulate gut microbiota and glucose metabolism on insulin resistant rats. BMC Complement. Med. Ther. 20 (1), 107. doi: 10.1186/s12906-020-02897-5
Hamilton, A., Patterson, S., Porter, D., Gault, V. A., Holscher, C. (2011). Novel GLP-1 mimetics developed to treat type 2 diabetes promote progenitor cell proliferation in the brain. J. Neurosci. Res. 89 (4), 481–489. doi: 10.1002/jnr.22565
Han, J., Calvo, C. F., Kang, T. H., Baker, K. L., Park, J. H., Parras, C., et al. (2015). Vascular endothelial growth factor receptor 3 controls neural stem cell activation in mice and humans. Cell Rep. 10 (7), 1158–1172. doi: 10.1016/j.celrep.2015.01.049
Han, S. K., Joo, M. K., Kim, J. K., Jeung, W., Kang, H., Kim, D. H. (2020). Bifidobacteria-fermented red ginseng and its constituents ginsenoside Rd and protopanaxatriol alleviate Anxiety/Depression in mice by the amelioration of gut dysbiosis. Nutrients 12 (4), 901. doi: 10.3390/nu12040901
Harris, L., Rigo, P., Stiehl, T., Gaber, Z. B., Austin, S., Masdeu, M., et al. (2021). Coordinated changes in cellular behavior ensure the lifelong maintenance of the hippocampal stem cell population. Cell Stem Cell. 28 (5), 863–876.e6. doi: 10.1016/j.stem.2021.01.003
Holt, M. K., Richards, J. E., Cook, D. R., Brierley, D. I., Williams, D. L., Reimann, F., et al. (2019). Preproglucagon neurons in the nucleus of the solitary tract are the main source of brain GLP-1, mediate stress-induced hypophagia, and limit unusually Large intakes of food. Diabetes 68 (1), 21–33. doi: 10.2337/db18-0729
Hori, K., Sen, A., Artavanis-Tsakonas, S. (2013). Notch signaling at a glance. J. Cell Sci. 126 (Pt 10), 2135–2140. doi: 10.1242/jcs.127308
Hossain, M. S., Mawatari, S., Fujino, T. (2022). Plasmalogens, the vinyl ether-linked glycerophospholipids, enhance learning and memory by regulating brain-derived neurotrophic factor. Front. Cell Dev. Biol. 10. doi: 10.3389/fcell.2022.828282
Hsieh, Y. C., Puche, A. C. (2015). GABA modulation of SVZ-derived progenitor ventral cell migration. Dev. Neurobiol. 75 (8), 791–804. doi: 10.1002/dneu.22249
Huang, Y., Yun, K. (2020). Study on effects and mechanism of lead and high-fat diet on cognitive function and central nervous system in mice. World Neurosurg. 138, 758–763. doi: 10.1016/j.wneu.2020.01.165
Hua, Y., Yang, Y., Li, Q., He, X., Zhu, W., Wang, J., et al. (2018). Oligomerization of frizzled and LRP5/6 protein initiates intracellular signaling for the canonical WNT/beta-catenin pathway. J. Biol. Chem. 293 (51), 19710–19724. doi: 10.1074/jbc.RA118.004434
Hueston, C. M., Cryan, J. F., Nolan, Y. M. (2017). Stress and adolescent hippocampal neurogenesis: diet and exercise as cognitive modulators. Transl. Psychiatry 7 (4), e1081. doi: 10.1038/tp.2017.48
Hurtado-Chong, A., Yusta-Boyo, M. J., Vergano-Vera, E., Bulfone, A., de Pablo, F., Vicario-Abejon, C. (2009). IGF-I promotes neuronal migration and positioning in the olfactory bulb and the exit of neuroblasts from the subventricular zone. Eur. J. Neurosci. 30 (5), 742–755. doi: 10.1111/j.1460-9568.2009.06870.x
Huuskonen, J., Suuronen, T., Nuutinen, T., Kyrylenko, S., Salminen, A. (2004). Regulation of microglial inflammatory response by sodium butyrate and short-chain fatty acids. Br. J. Pharmacol. 141 (5), 874–880. doi: 10.1038/sj.bjp.0705682
Imayoshi, I., Isomura, A., Harima, Y., Kawaguchi, K., Kori, H., Miyachi, H., et al. (2013). Oscillatory control of factors determining multipotency and fate in mouse neural progenitors. Science 342 (6163), 1203–1208. doi: 10.1126/science.1242366
Inestrosa, N. C., Arenas, E. (2010). Emerging roles of wnts in the adult nervous system. Nat. Rev. Neurosci. 11 (2), 77–86. doi: 10.1038/nrn2755
Ingham, P. W., Nakano, Y., Seger, C. (2011). Mechanisms and functions of hedgehog signalling across the metazoa. Nat. Rev. Genet. 12 (6), 393–406. doi: 10.1038/nrg2984
Jana, A., Modi, K. K., Roy, A., Anderson, J. A., van Breemen, R. B., Pahan, K. (2013). Up-regulation of neurotrophic factors by cinnamon and its metabolite sodium benzoate: therapeutic implications for neurodegenerative disorders. J. Neuroimmune. Pharmacol. 8 (3), 739–755. doi: 10.1007/s11481-013-9447-7
Jiang, H., Ling, Z., Zhang, Y., Mao, H., Ma, Z., Yin, Y., et al. (2015). Altered fecal microbiota composition in patients with major depressive disorder. Brain Behav. Immun. 48, 186–194. doi: 10.1016/j.bbi.2015.03.016
Jin, K., Zhu, Y., Sun, Y., Mao, X. O., Xie, L., Greenberg, D. A. (2002). Vascular endothelial growth factor (VEGF) stimulates neurogenesis in vitro and in vivo. Proc. Natl. Acad. Sci. U. S. A. 99 (18), 11946–11950. doi: 10.1073/pnas.182296499
Kabeerdoss, J., Ferdous, S., Balamurugan, R., Mechenro, J., Vidya, R., Santhanam, S., et al. (2013). Development of the gut microbiota in southern Indian infants from birth to 6 months: a molecular analysis. J. Nutr. Sci. 2, e18. doi: 10.1017/jns.2013.6
Kaczmarczyk, R., Tejera, D., Simon, B. J., Heneka, M. T. (2017). Microglia modulation through external vagus nerve stimulation in a murine model of alzheimer’s disease. J. Neurochem. 146 (1), 76–85. doi: 10.1111/jnc.14284
Kaelberer, M. M., Buchanan, K. L., Klein, M. E., Barth, B. B., Montoya, M. M., Shen, X., et al. (2018). A gut-brain neural circuit for nutrient sensory transduction. Science 361 (6408). doi: 10.1126/science.aat5236
Karimipour, M., Rahbarghazi, R., Tayefi, H., Shimia, M., Ghanadian, M., Mahmoudi, J., et al. (2019). Quercetin promotes learning and memory performance concomitantly with neural stem/progenitor cell proliferation and neurogenesis in the adult rat dentate gyrus. Int. J. Dev. Neurosci. 74, 18–26. doi: 10.1016/j.ijdevneu.2019.02.005
Kim, N., Jeon, S. H., Ju, I. G., Gee, M. S., Do, J., Oh, M. S., et al. (2021). Transplantation of gut microbiota derived from alzheimer’s disease mouse model impairs memory function and neurogenesis in C57BL/6 mice. Brain Behav. Immun. 98, 357–365. doi: 10.1016/j.bbi.2021.09.002
Kim, C., Pinto, A. M., Bordoli, C., Buckner, L. P., Kaplan, P. C., Del, A. I., et al. (2020). Energy restriction enhances adult hippocampal neurogenesis-associated memory after four weeks in an adult human population with central obesity; a randomized controlled trial. Nutrients 12 (3), 638. doi: 10.3390/nu12030638
Kim, H., Shin, J., Kim, S., Kim, S., Cho, B., Park, S. J., et al. (2022). Bifidobacterium bifidum BGN4 and bifidobacterium longum BORI promotes neuronal rejuvenation in aged mice. Biochem. Biophys. Res. Commun. 603, 41–48. doi: 10.1016/j.bbrc.2022.03.024
Kinoshita, A., Shqirat, M., Kageyama, R., Ohtsuka, T. (2022). Modification of gene expression and soluble factor secretion in the lateral ventricle choroid plexus: Analysis of the impacts on the neocortical development. Neurosci. Res. 177, 38–51. doi: 10.1016/j.neures.2021.12.005
Koh, S. H., Park, H. H. (2017). Neurogenesis in stroke recovery. Transl. Stroke. Res. 8 (1), 3–13. doi: 10.1007/s12975-016-0460-z
Kong, L., Hu, Y., Yao, Y., Jiao, Y., Li, S., Yang, J. (2015). The coumarin derivative osthole stimulates adult neural stem cells, promotes neurogenesis in the hippocampus, and ameliorates cognitive impairment in APP/PS1 transgenic mice. Biol. Pharm. Bull. 38 (9), 1290–1301. doi: 10.1248/bpb.b15-00142
Kopan, R., Ilagan, M. X. (2009). The canonical notch signaling pathway: unfolding the activation mechanism. Cell 137 (2), 216–233. doi: 10.1016/j.cell.2009.03.045
Kundu, P., Lee, H. U., Garcia-Perez, I., Tay, E., Kim, H., Faylon, L. E., et al. (2019). Neurogenesis and prolongevity signaling in young germ-free mice transplanted with the gut microbiota of old mice. Sci. Transl. Med. 11 (518), eaau4760. doi: 10.1126/scitranslmed.aau4760
Kuwahara, A., Matsuda, K., Kuwahara, Y., Asano, S., Inui, T., Marunaka, Y. (2020). Microbiota-gut-brain axis: enteroendocrine cells and the enteric nervous system form an interface between the microbiota and the central nervous system. BioMed. Res. 41 (5), 199–216. doi: 10.2220/biomedres.41.199
Lam, K. Y., Chen, J., Lam, C. T., Wu, Q., Yao, P., Dong, T. T., et al. (2016). Asarone from acori tatarinowii rhizoma potentiates the nerve growth factor-induced neuronal differentiation in cultured PC12 cells: A signaling mediated by protein kinase a. PloS One 11 (9), e0163337. doi: 10.1371/journal.pone.0163337
Latorre, R., Sternini, C., De Giorgio, R., Greenwood-Van, M. B. (2016). Enteroendocrine cells: a review of their role in brain-gut communication. Neurogastroenterol. Motil. 28 (5), 620–630. doi: 10.1111/nmo.12754
Lee, K. S., Lim, B. V., Chang, H. K., Yang, H. Y., Bahn, G. H., Paik, E. K., et al. (2005). Liuweidihuang-tang improves spatial memory function and increases neurogenesis in the dentate gyrus in rats. Fitoterapia 76 (6), 514–519. doi: 10.1016/j.fitote.2005.04.022
Legan, T. B., Lavoie, B., Mawe, G. M. (2022). Direct and indirect mechanisms by which the gut microbiota influence host serotonin systems. Neurogastroenterol. Motil. 34 (10), e14346. doi: 10.1111/nmo.14346
Lepko, T., Pusch, M., Muller, T., Schulte, D., Ehses, J., Kiebler, M., et al. (2019). Choroid plexus-derived miR-204 regulates the number of quiescent neural stem cells in the adult brain. EMBO J. 38 (17), e100481. doi: 10.15252/embj.2018100481
Li, Q., Barres, B. A. (2018). Microglia and macrophages in brain homeostasis and disease. Nat. Rev. Immunol. 18 (4), 225–242. doi: 10.1038/nri.2017.125
Li, M. Y., Chang, C. T., Han, Y. T., Liao, C. P., Yu, J. Y., Wang, T. W. (2018). Ginkgolide b promotes neuronal differentiation through the wnt/beta-catenin pathway in neural stem cells of the postnatal mammalian subventricular zone. Sci. Rep. 8 (1), 14947. doi: 10.1038/s41598-018-32960-8
Lie, D. C., Colamarino, S. A., Song, H. J., Desire, L., Mira, H., Consiglio, A., et al. (2005). Wnt signalling regulates adult hippocampal neurogenesis. Nature 437 (7063), 1370–1375. doi: 10.1038/nature04108
Li, J., Han, Y., Li, M., Nie, C. (2019). Curcumin promotes proliferation of adult neural stem cells and the birth of neurons in alzheimer’s disease mice via notch signaling pathway. Cell. Reprogram. 21 (3), 152–161. doi: 10.1089/cell.2018.0027
Li, E., Kim, Y., Kim, S., Sato, T., Kojima, M., Park, S. (2014). Ghrelin stimulates proliferation, migration and differentiation of neural progenitors from the subventricular zone in the adult mice. Exp. Neurol. 252, 75–84. doi: 10.1016/j.expneurol.2013.11.021
Li, X. T., Liang, Z., Wang, T. T., Yang, J. W., Ma, W., Deng, S. K., et al. (2017). Brain-derived neurotrophic factor promotes growth of neurons and neural stem cells possibly by triggering the phosphoinositide 3-Kinase/AKT/Glycogen synthase kinase-3beta/beta-catenin pathway. CNS Neurol. Disord. Drug Targets. 16 (7), 828–836. doi: 10.2174/1871527316666170518170422
Li, Y., Liu, T., Li, Y., Han, D., Hong, J., Yang, N., et al. (2020). Baicalin ameliorates cognitive impairment and protects microglia from LPS-induced neuroinflammation via the SIRT1/HMGB1 pathway. Oxid. Med. Cell. Longev. 2020, 4751349. doi: 10.1155/2020/4751349
Lin, T., Liu, Y., Shi, M., Liu, X., Li, L., Liu, Y., et al. (2012). Promotive effect of ginsenoside Rd on proliferation of neural stem cells in vivo and in vitro. J. Ethnopharmacol. 142 (3), 754–761. doi: 10.1016/j.jep.2012.05.057
Lin, P., Tan, R., Yu, P., Li, Y., Mo, Y., Li, W., et al. (2022). Autophagic degradation of claudin-5 mediated by its binding to a clostridium perfringens enterotoxin fragment modulates endothelial barrier permeability. FEBS Lett. 596 (7), 924–937. doi: 10.1002/1873-3468.14315
Li, M., Qian, S. (2016). Gastrodin protects neural progenitor cells against amyloid beta (1-42)-Induced neurotoxicity and improves hippocampal neurogenesis in amyloid beta (1-42)-Injected mice. J. Mol. Neurosci. 60 (1), 21–32. doi: 10.1007/s12031-016-0758-z
Liu, Z., de Bruijn, W., Bruins, M. E., Vincken, J. P. (2020). Reciprocal interactions between epigallocatechin-3-gallate (EGCG) and human gut microbiota In vitro. J. Agric. Food Chem. 68 (36), 9804–9815. doi: 10.1021/acs.jafc.0c03587
Liu, B. C., Liu, F. Y., Gao, X. Y., Chen, Y. L., Meng, Q. Q., Song, Y. L., et al. (2021). Global transcriptional analyses of the wnt-induced development of neural stem cells from human pluripotent stem cells. Int. J. Mol. Sci. 22 (14), 7473. doi: 10.3390/ijms22147473
Liu, Y., Sanderson, D., Mian, M. F., Mcvey, N. K., Forsythe, P. (2021). Loss of vagal integrity disrupts immune components of the microbiota-gut-brain axis and inhibits the effect of lactobacillus rhamnosus on behavior and the corticosterone stress response. Neuropharmacology 195, 108682. doi: 10.1016/j.neuropharm.2021.108682
Liu, N., Sun, S., Wang, P., Sun, Y., Hu, Q., Wang, X. (2021). The mechanism of secretion and metabolism of gut-derived 5-hydroxytryptamine. Int. J. Mol. Sci. 22 (15), 7931. doi: 10.3390/ijms22157931
Liu, J., Ye, T., Zhang, Y., Zhang, R., Kong, Y., Zhang, Y., et al. (2021). Protective effect of ginkgolide b against cognitive impairment in mice via regulation of gut microbiota. J. Agric. Food Chem. 69 (41), 12230–12240. doi: 10.1021/acs.jafc.1c05038
Liu, J., Zhang, T., Wang, Y., Si, C., Wang, X., Wang, R. T., et al. (2020). Baicalin ameliorates neuropathology in repeated cerebral ischemia-reperfusion injury model mice by remodeling the gut microbiota. Aging (Albany. NY). 12 (4), 3791–3806. doi: 10.18632/aging.102846
Li, R., Wang, F., Dang, S., Yao, M., Zhang, W., Wang, J. (2022). Integrated 16S rRNA gene sequencing and metabolomics analysis to investigate the important role of osthole on gut microbiota and serum metabolites in neuropathic pain mice. Front. Physiol. 13. doi: 10.3389/fphys.2022.813626
Li, C., Wang, N., Zheng, G., Yang, L. (2021). Oral administration of resveratrol-Selenium-Peptide nanocomposites alleviates alzheimer’s disease-like pathogenesis by inhibiting abeta aggregation and regulating gut microbiota. ACS Appl. Mater. Interfaces. 13 (39), 46406–46420. doi: 10.1021/acsami.1c14818
Li, X., Zhao, T., Gu, J., Wang, Z., Lin, J., Wang, R., et al. (2022). Intake of flavonoids from astragalus membranaceus ameliorated brain impairment in diabetic mice via modulating brain-gut axis. Chin. Med. 17 (1), 22. doi: 10.1186/s13020-022-00578-8
Lorbek, G., Lewinska, M., Rozman, D. (2012). Cytochrome P450s in the synthesis of cholesterol and bile acids–from mouse models to human diseases. FEBS J. 279 (9), 1516–1533. doi: 10.1111/j.1742-4658.2011.08432.x
Macdonald, B. T., He, X. (2012). Frizzled and LRP5/6 receptors for wnt/beta-catenin signaling. Cold Spring Harb. Perspect. Biol. 4 (12), a007880. doi: 10.1101/cshperspect.a007880
Marchetti, B., Giachino, C., Tirolo, C., Serapide, M. F. (2022). “Reframing” dopamine signaling at the intersection of glial networks in the aged parkinsonian brain as innate Nrf2/Wnt driver: Therapeutical implications. Aging Cell. 21 (4), e13575. doi: 10.1111/acel.13575
Mayer, E. A., Nance, K., Chen, S. (2022). The gut-brain axis. Annu. Rev. Med. 73, 439–453. doi: 10.1146/annurev-med-042320-014032
Ma, D., Zhao, L., Zhang, L., Li, Y., Zhang, L., Li, L. (2021). Icariin promotes survival, proliferation, and differentiation of neural stem cells In vitro and in a rat model of alzheimer’s disease. Stem Cells Int. 2021, 9974625. doi: 10.1155/2021/9974625
Mcgovern, S. F., Hunter, K., Holscher, C. (2012). Effects of the glucagon-like polypeptide-1 analogue (Val8) GLP-1 on learning, progenitor cell proliferation and neurogenesis in the C57B/16 mouse brain. Brain Res. 1473, 204–213. doi: 10.1016/j.brainres.2012.07.029
Mcpherson, C. A., Aoyama, M., Harry, G. J. (2011). Interleukin (IL)-1 and IL-6 regulation of neural progenitor cell proliferation with hippocampal injury: differential regulatory pathways in the subgranular zone (SGZ) of the adolescent and mature mouse brain. Brain Behav. Immun. 25 (5), 850–862. doi: 10.1016/j.bbi.2010.09.003
Mehta, S., Hingole, S., Chaudhary, V. (2021). The emerging mechanisms of wnt secretion and signaling in development. Front. Cell Dev. Biol. 9. doi: 10.3389/fcell.2021.714746
Miletta, M. C., Petkovic, V., Eble, A., Ammann, R. A., Fluck, C. E., Mullis, P. E. (2014). Butyrate increases intracellular calcium levels and enhances growth hormone release from rat anterior pituitary cells via the G-protein-coupled receptors GPR41 and 43. PloS One 9 (10), e107388. doi: 10.1371/journal.pone.0107388
Ming, G. L., Song, H. (2011). Adult neurogenesis in the mammalian brain: significant answers and significant questions. Neuron 70 (4), 687–702. doi: 10.1016/j.neuron.2011.05.001
Mizrak, D., Levitin, H. M., Delgado, A. C., Crotet, V., Yuan, J., Chaker, Z., et al. (2019). Single-cell analysis of regional differences in adult V-SVZ neural stem cell lineages. Cell Rep. 26 (2), 394–406.e5. doi: 10.1016/j.celrep.2018.12.044
Mizutani, K., Yoon, K., Dang, L., Tokunaga, A., Gaiano, N. (2007). Differential notch signalling distinguishes neural stem cells from intermediate progenitors. Nature 449 (7160), 351–355. doi: 10.1038/nature06090
Mohamed, S., Lee, M. T., Jaffri, J. M. (2013). Cognitive enhancement and neuroprotection by catechin-rich oil palm leaf extract supplement. J. Sci. Food Agric. 93 (4), 819–827. doi: 10.1002/jsfa.5802
Mohle, L., Mattei, D., Heimesaat, M. M., Bereswill, S., Fischer, A., Alutis, M., et al. (2016). Ly6C(hi) monocytes provide a link between antibiotic-induced changes in gut microbiota and adult hippocampal neurogenesis. Cell Rep. 15 (9), 1945–1956. doi: 10.1016/j.celrep.2016.04.074
Monteiro-Cardoso, V. F., Corliano, M., Singaraja, R. R. (2021). Bile acids: A communication channel in the gut-brain axis. Neuromol. Med. 23 (1), 99–117. doi: 10.1007/s12017-020-08625-z
Morais, L. H., Schreiber, H. T., Mazmanian, S. K. (2021). The gut microbiota-brain axis in behaviour and brain disorders. Nat. Rev. Microbiol. 19 (4), 241–255. doi: 10.1038/s41579-020-00460-0
Moris, G., Arboleya, S., Mancabelli, L., Milani, C., Ventura, M., de Los, R. C., et al. (2018). Fecal microbiota profile in a group of myasthenia gravis patients. Sci. Rep. 8 (1), 14384. doi: 10.1038/s41598-018-32700-y
Morris, G., Berk, M., Carvalho, A., Caso, J. R., Sanz, Y., Walder, K., et al. (2017). The role of the microbial metabolites including tryptophan catabolites and short chain fatty acids in the pathophysiology of immune-inflammatory and neuroimmune disease. Mol. Neurobiol. 54 (6), 4432–4451. doi: 10.1007/s12035-016-0004-2
Namchaiw, P., Wen, H., Mayrhofer, F., Chechneva, O., Biswas, S., Deng, W. (2019). Temporal and partial inhibition of GLI1 in neural stem cells (NSCs) results in the early maturation of NSC derived oligodendrocytes in vitro. Stem Cell Res. Ther. 10 (1), 272. doi: 10.1186/s13287-019-1374-y
Nam, S. M., Seo, M., Seo, J. S., Rhim, H., Nahm, S. S., Cho, I. H., et al. (2019). Ascorbic acid mitigates d-galactose-Induced brain aging by increasing hippocampal neurogenesis and improving memory function. Nutrients 11 (1), 176. doi: 10.3390/nu11010176
Ni, S. J., Yao, Z. Y., Wei, X., Heng, X., Qu, S. Y., Zhao, X., et al. (2022). Vagus nerve stimulated by microbiota-derived hydrogen sulfide mediates the regulation of berberine on microglia in transient middle cerebral artery occlusion rats. Phytother. Res. 36 (7), 2964–2981. doi: 10.1002/ptr.7490
Obernier, K., Cebrian-Silla, A., Thomson, M., Parraguez, J. I., Anderson, R., Guinto, C., et al. (2018). Adult neurogenesis is sustained by symmetric self-renewal and differentiation. Cell Stem Cell. 22 (2), 221–234.e8. doi: 10.1016/j.stem.2018.01.003
Ogbonnaya, E. S., Clarke, G., Shanahan, F., Dinan, T. G., Cryan, J. F., O’Leary, O. F. (2015). Adult hippocampal neurogenesis is regulated by the microbiome. Biol. Psychiatry 78 (4), e7–e9. doi: 10.1016/j.biopsych.2014.12.023
O’Keeffe, G. C., Barker, R. A., Caldwell, M. A. (2009). Dopaminergic modulation of neurogenesis in the subventricular zone of the adult brain. Cell Cycle 8 (18), 2888–2894. doi: 10.4161/cc.8.18.9512
O’Leary, O. F., Ogbonnaya, E. S., Felice, D., Levone, B. R., Conroy, C. L., Fitzgerald, P., et al. (2018). The vagus nerve modulates BDNF expression and neurogenesis in the hippocampus. Eur. Neuropsychopharmacol. 28 (2), 307–316. doi: 10.1016/j.euroneuro.2017.12.004
Olson, C. A., Iniguez, A. J., Yang, G. E., Fang, P., Pronovost, G. N., Jameson, K. G., et al. (2021). Alterations in the gut microbiota contribute to cognitive impairment induced by the ketogenic diet and hypoxia. Cell Host Microbe 29 (9), 1378–1392.e6. doi: 10.1016/j.chom.2021.07.004
Pallotto, M., Deprez, F. (2014). Regulation of adult neurogenesis by GABAergic transmission: signaling beyond GABAA-receptors. Front. Cell. Neurosci. 8. doi: 10.3389/fncel.2014.00166
Palma, V., Lim, D. A., Dahmane, N., Sanchez, P., Brionne, T. C., Herzberg, C. D., et al. (2005). Sonic hedgehog controls stem cell behavior in the postnatal and adult brain. Development 132 (2), 335–344. doi: 10.1242/dev.01567
Pao, L. H., Lu, S. W., Sun, G. G., Chiou, S. H., Ma, K. H. (2012). Three Chinese herbal medicines promote neuroproliferation in vitro, and reverse the effects of chronic mild stress on behavior, the HPA axis, and proliferation of hippocampal precursor cell in vivo. J. Ethnopharmacol. 144 (2), 261–269. doi: 10.1016/j.jep.2012.09.002
Park, H. R., Kim, J. Y., Lee, Y., Chun, H. J., Choi, Y. W., Shin, H. K., et al. (2016). PMC-12, a traditional herbal medicine, enhances learning memory and hippocampal neurogenesis in mice. Neurosci. Lett. 617, 254–263. doi: 10.1016/j.neulet.2016.02.036
Perello, M., Cornejo, M. P., De Francesco, P. N., Fernandez, G., Gautron, L., Valdivia, L. S. (2022). The controversial role of the vagus nerve in mediating ghrelin’s actions: gut feelings and beyond. IBRO. Neurosci. Rep. 12, 228–239. doi: 10.1016/j.ibneur.2022.03.003
Pinto-Sanchez, M. I., Hall, G. B., Ghajar, K., Nardelli, A., Bolino, C., Lau, J. T., et al. (2017). Probiotic bifidobacterium longum NCC3001 reduces depression scores and alters brain activity: A pilot study in patients with irritable bowel syndrome. Gastroenterology 153 (2), 448–459.e8. doi: 10.1053/j.gastro.2017.05.003
Planques, A., Oliveira, M. V., Dubreuil, C., Prochiantz, A., Di Nardo, A. A. (2019). OTX2 signals from the choroid plexus to regulate adult neurogenesis. eNeuro 6 (2), ENEURO.0262-18.2019. doi: 10.1523/ENEURO.0262-18.2019
Ponti, G., Obernier, K., Guinto, C., Jose, L., Bonfanti, L., Alvarez-Buylla, A. (2013). Cell cycle and lineage progression of neural progenitors in the ventricular-subventricular zones of adult mice. Proc. Natl. Acad. Sci. U. S. A. 110 (11), E1045–E1054. doi: 10.1073/pnas.1219563110
Pous, L., Deshpande, S. S., Nath, S., Mezey, S., Malik, S. C., Schildge, S., et al. (2020). Fibrinogen induces neural stem cell differentiation into astrocytes in the subventricular zone via BMP signaling. Nat. Commun. 11 (1), 630. doi: 10.1038/s41467-020-14466-y
Qin, W., Chen, S., Yang, S., Xu, Q., Xu, C., Cai, J. (2017). The effect of traditional Chinese medicine on neural stem cell proliferation and differentiation. Aging Dis. 8 (6), 792–811. doi: 10.14336/AD.2017.0428
Ramos-Mandujano, G., Hernandez-Benitez, R., Pasantes-Morales, H. (2014). Multiple mechanisms mediate the taurine-induced proliferation of neural stem/progenitor cells from the subventricular zone of the adult mouse. Stem Cell Res. 12 (3), 690–702. doi: 10.1016/j.scr.2014.02.009
Rana, T., Behl, T., Sehgal, A., Sachdeva, M., Mehta, V., Sharma, N., et al. (2021). Exploring sonic hedgehog cell signaling in neurogenesis: Its potential role in depressive behavior. Neurochem. Res. 46 (7), 1589–1602. doi: 10.1007/s11064-021-03307-z
Rea, V., Bell, I., Ball, T., Van Raay, T. (2022). Gut-derived metabolites influence neurodevelopmental gene expression and wnt signaling events in a germ-free zebrafish model. Microbiome 10 (1), 132. doi: 10.1186/s40168-022-01302-2
Rei, D., Saha, S., Haddad, M., Rubio, A. H., Perlaza, B. L., Berard, M., et al. (2022). Age-associated gut microbiota impair hippocampus-dependent memory in a vagus-dependent manner. JCI Insight 7 (15), e147700. doi: 10.1172/jci.insight.147700
Rendina, D. N., Lubach, G. R., Lyte, M., Phillips, G. J., Gosain, A., Pierre, J. F., et al. (2021). Proteobacteria abundance during nursing predicts physical growth and brain volume at one year of age in young rhesus monkeys. FASEB J. 35 (6), e21682. doi: 10.1096/fj.202002162R
Ribeiro, F. F., Xapelli, S. (2021). Intervention of brain-derived neurotrophic factor and other neurotrophins in adult neurogenesis. Adv. Exp. Med. Biol. 1331, 95–115. doi: 10.1007/978-3-030-74046-7_8
Riezzo, G., Chimienti, G., Orlando, A., D’Attoma, B., Clemente, C., Russo, F. (2019). Effects of long-term administration of lactobacillus reuteri DSM-17938 on circulating levels of 5-HT and BDNF in adults with functional constipation. Benef. Microbes 10 (2), 137–147. doi: 10.3920/BM2018.0050
Roager, H. M., Licht, T. R. (2018). Microbial tryptophan catabolites in health and disease. Nat. Commun. 9 (1), 3294. doi: 10.1038/s41467-018-05470-4
Ronchi, G., Ryu, V., Fornaro, M., Czaja, K. (2012). Hippocampal plasticity after a vagus nerve injury in the rat. Neural Regen. Res. 7 (14), 1055–1063. doi: 10.3969/j.issn.1673-5374.2012.14.003
Rosa, A. I., Goncalves, J., Cortes, L., Bernardino, L., Malva, J. O., Agasse, F. (2010). The angiogenic factor angiopoietin-1 is a proneurogenic peptide on subventricular zone stem/progenitor cells. J. Neurosci. 30 (13), 4573–4584. doi: 10.1523/JNEUROSCI.5597-09.2010
Rosa, A. I., Grade, S., Santos, S. D., Bernardino, L., Chen, T. C., Relvas, J., et al. (2016). Heterocellular contacts with mouse brain endothelial cells Via laminin and alpha6beta1 integrin sustain subventricular zone (SVZ) Stem/Progenitor cells properties. Front. Cell. Neurosci. 10. doi: 10.3389/fncel.2016.00284
Saha, P., Skidmore, P. T., Holland, L. A., Mondal, A., Bose, D., Seth, R. K., et al. (2021). Andrographolide attenuates gut-Brain-Axis associated pathology in gulf war illness by modulating bacteriome-virome associated inflammation and microglia-neuron proinflammatory crosstalk. Brain Sci. 11 (7), 905. doi: 10.3390/brainsci11070905
Sakane, R., Kimura, K., Hirota, Y., Ishizawa, M., Takagi, Y., Wada, A., et al. (2017). Synthesis of novel vitamin K derivatives with alkylated phenyl groups introduced at the omega-terminal side chain and evaluation of their neural differentiation activities. Bioorg. Med. Chem. Lett. 27 (21), 4881–4884. doi: 10.1016/j.bmcl.2017.09.038
Salvo, E., Stokes, P., Keogh, C. E., Brust-Mascher, I., Hennessey, C., Knotts, T. A., et al. (2020). A murine model of pediatric inflammatory bowel disease causes microbiota-gut-brain axis deficits in adulthood. Am. J. Physiol. Gastrointest. Liver. Physiol. 319 (3), G361–G374. doi: 10.1152/ajpgi.00177.2020
Sawamoto, K., Wichterle, H., Gonzalez-Perez, O., Cholfin, J. A., Yamada, M., Spassky, N., et al. (2006). New neurons follow the flow of cerebrospinal fluid in the adult brain. Science 311 (5761), 629–632. doi: 10.1126/science.1119133
Shan, X., Tomlinson, L., Yang, Q., Colognato, H. (2018). Distinct requirements for extracellular and intracellular MMP12 in the development of the adult V-SVZ neural stem cell niche. Stem Cell Rep. 10 (3), 984–999. doi: 10.1016/j.stemcr.2018.01.038
Shi, H., Ge, X., Ma, X., Zheng, M., Cui, X., Pan, W., et al. (2021). A fiber-deprived diet causes cognitive impairment and hippocampal microglia-mediated synaptic loss through the gut microbiota and metabolites. Microbiome 9 (1), 223. doi: 10.1186/s40168-021-01172-0
Shigemoto-Mogami, Y., Hoshikawa, K., Goldman, J. E., Sekino, Y., Sato, K. (2014). Microglia enhance neurogenesis and oligodendrogenesis in the early postnatal subventricular zone. J. Neurosci. 34 (6), 2231–2243. doi: 10.1523/JNEUROSCI.1619-13.2014
Shou, J. W., Cheung, C. K., Gao, J., Shi, W. W., Shaw, P. C. (2019). Berberine protects C17.2 neural stem cells from oxidative damage followed by inducing neuronal differentiation. Front. Cell. Neurosci. 13. doi: 10.3389/fncel.2019.00395
Sierra, A., Encinas, J. M., Deudero, J. J., Chancey, J. H., Enikolopov, G., Overstreet-Wadiche, L. S., et al. (2010). Microglia shape adult hippocampal neurogenesis through apoptosis-coupled phagocytosis. Cell Stem Cell. 7 (4), 483–495. doi: 10.1016/j.stem.2010.08.014
Silva-Vargas, V., Doetsch, F. (2014). A new twist for neurotrophins: endothelial-derived NT-3 mediates adult neural stem cell quiescence. Neuron 83 (3), 507–509. doi: 10.1016/j.neuron.2014.07.029
Slykerman, R. F., Hood, F., Wickens, K., Thompson, J., Barthow, C., Murphy, R., et al. (2017). Effect of lactobacillus rhamnosus HN001 in pregnancy on postpartum symptoms of depression and anxiety: A randomised double-blind placebo-controlled trial. EBioMedicine 24, 159–165. doi: 10.1016/j.ebiom.2017.09.013
Soares, R., Ribeiro, F. F., Xapelli, S., Genebra, T., Ribeiro, M. F., Sebastiao, A. M., et al. (2018). Tauroursodeoxycholic acid enhances mitochondrial biogenesis, neural stem cell pool, and early neurogenesis in adult rats. Mol. Neurobiol. 55 (5), 3725–3738. doi: 10.1007/s12035-017-0592-5
Song, J., Olsen, R. H., Sun, J., Ming, G. L., Song, H. (2016). Neuronal circuitry mechanisms regulating adult mammalian neurogenesis. Cold Spring Harb. Perspect. Biol. 8 (8), a018937. doi: 10.1101/cshperspect.a018937
Song, X., Wang, W., Ding, S., Liu, X., Wang, Y., Ma, H. (2021). Puerarin ameliorates depression-like behaviors of with chronic unpredictable mild stress mice by remodeling their gut microbiota. J. Affect. Disord. 290, 353–363. doi: 10.1016/j.jad.2021.04.037
Song, C., Wang, B., Tan, J., Zhu, L., Lou, D. (2017). Discovery of tauroursodeoxycholic acid biotransformation enzymes from the gut microbiome of black bears using metagenomics. Sci. Rep. 7, 45495. doi: 10.1038/srep45495
Steinert, R. E., Lee, Y. K., Sybesma, W. (2020). Vitamins for the gut microbiome. Trends Mol. Med. 26 (2), 137–140. doi: 10.1016/j.molmed.2019.11.005
Sun, Z. Z., Li, X. Y., Wang, S., Shen, L., Ji, H. F. (2020). Bidirectional interactions between curcumin and gut microbiota in transgenic mice with alzheimer’s disease. Appl. Microbiol. Biotechnol. 104 (8), 3507–3515. doi: 10.1007/s00253-020-10461-x
Sun, J., Wang, F., Hu, X., Yang, C., Xu, H., Yao, Y., et al. (2018). Clostridium butyricum attenuates chronic unpredictable mild stress-induced depressive-like behavior in mice via the gut-brain axis. J. Agric. Food Chem. 66 (31), 8415–8421. doi: 10.1021/acs.jafc.8b02462
Sun, L., Zou, Y., Su, P., Xue, C., Wang, D., Zhao, F., et al. (2022). Lead exposure induced neural stem cells death via notch signaling pathway and gut-brain axis. Oxid. Med. Cell. Longev. 2022, 7676872. doi: 10.1155/2022/7676872
Sur, B., Lee, B. (2022). Ginsenoside Rg3 modulates spatial memory and fear memory extinction by the HPA axis and BDNF-TrkB pathway in a rat post-traumatic stress disorder. J. Nat. Med. 76 (4), 821–831. doi: 10.1007/s11418-022-01636-z
Tang, C. F., Wang, C. Y., Wang, J. H., Wang, Q. N., Li, S. J., Wang, H. O., et al. (2022). Short-chain fatty acids ameliorate depressive-like behaviors of high fructose-fed mice by rescuing hippocampal neurogenesis decline and blood-brain barrier damage. Nutrients 14 (9), 1882. doi: 10.3390/nu14091882
Tang, R., Yi, J., Lu, S., Chen, B., Liu, B. (2022). Therapeutic effect of buyang huanwu decoction on the gut microbiota and hippocampal metabolism in a rat model of cerebral ischemia. Front. Cell Infect. Microbiol. 12. doi: 10.3389/fcimb.2022.873096
Tan, H., Zhai, Q., Chen, W. (2019). Investigations of bacteroides spp. towards next-generation probiotics. Food Res. Int. 116, 637–644. doi: 10.1016/j.foodres.2018.08.088
Tong, C. K., Fuentealba, L. C., Shah, J. K., Lindquist, R. A., Ihrie, R. A., Guinto, C. D., et al. (2015). A dorsal SHH-dependent domain in the V-SVZ produces Large numbers of oligodendroglial lineage cells in the postnatal brain. Stem Cell Rep. 5 (4), 461–470. doi: 10.1016/j.stemcr.2015.08.013
Unger, M. M., Spiegel, J., Dillmann, K. U., Grundmann, D., Philippeit, H., Burmann, J., et al. (2016). Short chain fatty acids and gut microbiota differ between patients with parkinson’s disease and age-matched controls. Parkinsonism. Relat. Disord. 32, 66–72. doi: 10.1016/j.parkreldis.2016.08.019
Urban, N., Blomfield, I. M., Guillemot, F. (2019). Quiescence of adult mammalian neural stem cells: A highly regulated rest. Neuron 104 (5), 834–848. doi: 10.1016/j.neuron.2019.09.026
Varela-Nallar, L., Arredondo, S. B., Tapia-Rojas, C., Hancke, J., Inestrosa, N. C. (2015). Andrographolide stimulates neurogenesis in the adult hippocampus. Neural Plast. 2015, 935403. doi: 10.1155/2015/935403
Varela, R. B., Valvassori, S. S., Lopes-Borges, J., Mariot, E., Dal-Pont, G. C., Amboni, R. T., et al. (2015). Sodium butyrate and mood stabilizers block ouabain-induced hyperlocomotion and increase BDNF, NGF and GDNF levels in brain of wistar rats. J. Psychiatr. Res. 61, 114–121. doi: 10.1016/j.jpsychires.2014.11.003
Vogt, N. M., Kerby, R. L., Dill-Mcfarland, K. A., Harding, S. J., Merluzzi, A. P., Johnson, S. C., et al. (2017). Gut microbiome alterations in alzheimer’s disease. Sci. Rep. 7 (1), 13537. doi: 10.1038/s41598-017-13601-y
Wang, J., Cui, Y., Yu, Z., Wang, W., Cheng, X., Ji, W., et al. (2019). Brain endothelial cells maintain lactate homeostasis and control adult hippocampal neurogenesis. Cell Stem Cell. 25 (6), 754–767.e9. doi: 10.1016/j.stem.2019.09.009
Wang, S., Ishima, T., Zhang, J., Qu, Y., Chang, L., Pu, Y., et al. (2020). Ingestion of lactobacillus intestinalis and lactobacillus reuteri causes depression- and anhedonia-like phenotypes in antibiotic-treated mice via the vagus nerve. J. Neuroinflamm. 17 (1), 241. doi: 10.1186/s12974-020-01916-z
Wang, Y., Li, M., Xu, X., Song, M., Tao, H., Bai, Y. (2012). Green tea epigallocatechin-3-gallate (EGCG) promotes neural progenitor cell proliferation and sonic hedgehog pathway activation during adult hippocampal neurogenesis. Mol. Nutr. Food Res. 56 (8), 1292–1303. doi: 10.1002/mnfr.201200035
Wang, L., Lu, J., Zeng, Y., Guo, Y., Wu, C., Zhao, H., et al. (2020). Improving alzheimer’s disease by altering gut microbiota in tree shrews with ginsenoside Rg1. FEMS Microbiol. Lett. 367 (4), fnaa011. doi: 10.1093/femsle/fnaa011
Wang, X., Mao, X., Xie, L., Greenberg, D. A., Jin, K. (2009). Involvement of Notch1 signaling in neurogenesis in the subventricular zone of normal and ischemic rat brain in vivo. J. Cereb. Blood Flow Metab. 29 (10), 1644–1654. doi: 10.1038/jcbfm.2009.83
Wang, X., Ma, S., Yang, B., Huang, T., Meng, N., Xu, L., et al. (2018). Resveratrol promotes hUC-MSCs engraftment and neural repair in a mouse model of alzheimer’s disease. Behav. Brain Res. 339, 297–304. doi: 10.1016/j.bbr.2017.10.032
Wang, F. B., Powley, T. L. (2007). Vagal innervation of intestines: afferent pathways mapped with new en bloc horseradish peroxidase adaptation. Cell Tissue Res. 329 (2), 221–230. doi: 10.1007/s00441-007-0413-7
Wang, J., Shen, Y., Zhang, Y., Zhang, R., Tang, X., Fang, L., et al. (2016a). Recent evidence of the regulatory role of PPARs in neural stem cells and their underlying mechanisms for neuroprotective effects. Curr. Stem Cell Res. Ther. 11 (3), 188–196. doi: 10.2174/1574888x10666150416113630
Wang, Y., Tong, Q., Ma, S. R., Zhao, Z. X., Pan, L. B., Cong, L., et al. (2021). Oral berberine improves brain dopa/dopamine levels to ameliorate parkinson’s disease by regulating gut microbiota. Signal Transduct. Target. Ther. 6 (1), 77. doi: 10.1038/s41392-020-00456-5
Wang, Y., Wang, Z., Wang, Y., Li, F., Jia, J., Song, X., et al. (2018). The gut-microglia connection: Implications for central nervous system diseases. Front. Immunol. 9. doi: 10.3389/fimmu.2018.02325
Wang, X. F., Xiao, H. H., Wu, Y. T., Kong, L., Chen, J. C., Yang, J. X., et al. (2021). Active constituent of polygala tenuifolia attenuates cognitive deficits by rescuing hippocampal neurogenesis in APP/PS1 transgenic mice. BMC Complement. Med. Ther. 21 (1), 267. doi: 10.1186/s12906-021-03437-5
Wang, J., Ye, F., Cheng, X., Zhang, X., Liu, F., Liu, G., et al. (2016b). The effects of LW-AFC on intestinal microbiome in senescence-accelerated mouse prone 8 strain, a mouse model of alzheimer’s disease. J. Alzheimers Dis. 53 (3), 907–919. doi: 10.3233/JAD-160138
Waterhouse, E. G., An, J. J., Orefice, L. L., Baydyuk, M., Liao, G. Y., Zheng, K., et al. (2012). BDNF promotes differentiation and maturation of adult-born neurons through GABAergic transmission. J. Neurosci. 32 (41), 14318–14330. doi: 10.1523/JNEUROSCI.0709-12.2012
Wei, G. Z., Martin, K. A., Xing, P. Y., Agrawal, R., Whiley, L., Wood, T. K., et al. (2021). Tryptophan-metabolizing gut microbes regulate adult neurogenesis via the aryl hydrocarbon receptor. Proc. Natl. Acad. Sci. U.S.A. 118 (27), e2021091118. doi: 10.1073/pnas.2021091118
Wilhelmsson, U., Faiz, M., de Pablo, Y., Sjoqvist, M., Andersson, D., Widestrand, A., et al. (2012). Astrocytes negatively regulate neurogenesis through the Jagged1-mediated notch pathway. Stem Cells 30 (10), 2320–2329. doi: 10.1002/stem.1196
Willis, C. M., Nicaise, A. M., Krzak, G., Ionescu, R. B., Pappa, V., D’Angelo, A., et al. (2022). Soluble factors influencing the neural stem cell niche in brain physiology, inflammation, and aging. Exp. Neurol. 355, 114124. doi: 10.1016/j.expneurol.2022.114124
Wittko, I. M., Schanzer, A., Kuzmichev, A., Schneider, F. T., Shibuya, M., Raab, S., et al. (2009). VEGFR-1 regulates adult olfactory bulb neurogenesis and migration of neural progenitors in the rostral migratory stream in vivo. J. Neurosci. 29 (27), 8704–8714. doi: 10.1523/JNEUROSCI.5527-08.2009
Wu, J., Tian, W. J., Liu, Y., Wang, H. J., Zheng, J., Wang, X., et al. (2020). Ependyma-expressed CCN1 restricts the size of the neural stem cell pool in the adult ventricular-subventricular zone. EMBO J. 39 (5), e101679. doi: 10.15252/embj.2019101679
Wu, J., Zhou, Z., Hu, Y., Dong, S. (2012). Butyrate-induced GPR41 activation inhibits histone acetylation and cell growth. J. Genet. Genomics 39 (8), 375–384. doi: 10.1016/j.jgg.2012.05.008
Xavier, J. M., Morgado, A. L., Rodrigues, C. M., Sola, S. (2014). Tauroursodeoxycholic acid increases neural stem cell pool and neuronal conversion by regulating mitochondria-cell cycle retrograde signaling. Cell Cycle 13 (22), 3576–3589. doi: 10.4161/15384101.2014.962951
Xiang, Y., Wang, S. H., Wang, L., Wang, Z. L., Yao, H., Chen, L. B., et al. (2019). Effects of ginsenoside Rg1 regulating wnt/beta-catenin signaling on neural stem cells to delay brain senescence. Stem Cells Int. 2019, 5010184. doi: 10.1155/2019/5010184
Xiao, Z., Cao, Z., Yang, J., Jia, Z., Du, Y., Sun, G., et al. (2021). Baicalin promotes hippocampal neurogenesis via the wnt/beta-catenin pathway in a chronic unpredictable mild stress-induced mouse model of depression. Biochem. Pharmacol. 190, 114594. doi: 10.1016/j.bcp.2021.114594
Xiao, L., Wang, M., Zou, K., Li, Z., Luo, J. (2022). Effects of ginsenoside Rg1 on proliferation and directed differentiation of human umbilical cord mesenchymal stem cells into neural stem cells. Neuroreport 33 (10), 413–421. doi: 10.1097/WNR.0000000000001795
Xie, Z., Lu, H., Yang, S., Zeng, Y., Li, W., Wang, L., et al. (2020). Salidroside attenuates cognitive dysfunction in senescence-accelerated mouse prone 8 (SAMP8) mice and modulates inflammation of the gut-brain axis. Front. Pharmacol. 11. doi: 10.3389/fphar.2020.568423
Xiong, W., Zhao, X., Xu, Q., Wei, G., Zhang, L., Fan, Y., et al. (2022). Qisheng wan formula ameliorates cognitive impairment of alzheimer’s disease rat via inflammation inhibition and intestinal microbiota regulation. J. Ethnopharmacol. 282, 114598. doi: 10.1016/j.jep.2021.114598
Xu, Y., Ku, B., Cui, L., Li, X., Barish, P. A., Foster, T. C., et al. (2007). Curcumin reverses impaired hippocampal neurogenesis and increases serotonin receptor 1A mRNA and brain-derived neurotrophic factor expression in chronically stressed rats. Brain Res. 1162, 9–18. doi: 10.1016/j.brainres.2007.05.071
Xu, Y., Ku, B., Tie, L., Yao, H., Jiang, W., Ma, X., et al. (2006). Curcumin reverses the effects of chronic stress on behavior, the HPA axis, BDNF expression and phosphorylation of CREB. Brain Res. 1122 (1), 56–64. doi: 10.1016/j.brainres.2006.09.009
Xu, Y., Xie, M., Xue, J., Xiang, L., Li, Y., Xiao, J., et al. (2020). EGCG ameliorates neuronal and behavioral defects by remodeling gut microbiota and TotM expression in drosophila models of parkinson’s disease. FASEB J. 34 (4), 5931–5950. doi: 10.1096/fj.201903125RR
Yang, J. W., Ma, W., Luo, T., Wang, D. Y., Lu, J. J., Li, X. T., et al. (2016). BDNF promotes human neural stem cell growth via GSK-3beta-mediated crosstalk with the wnt/beta-catenin signaling pathway. Growth Factors. 34 (1-2), 19–32. doi: 10.3109/08977194.2016.1157791
Yang, L. L., Millischer, V., Rodin, S., Macfabe, D. F., Villaescusa, J. C., Lavebratt, C. (2020). Enteric short-chain fatty acids promote proliferation of human neural progenitor cells. J. Neurochem. 154 (6), 635–646. doi: 10.1111/jnc.14928
Yang, Y., Zhang, Z., Li, S., Ye, X., Li, X., He, K. (2014). Synergy effects of herb extracts: pharmacokinetics and pharmacodynamic basis. Fitoterapia 92, 133–147. doi: 10.1016/j.fitote.2013.10.010
Yang, S., Zhou, H., Wang, G., Zhong, X. H., Shen, Q. L., Zhang, X. J., et al. (2020). Quercetin is protective against short-term dietary advanced glycation end products intake induced cognitive dysfunction in aged ICR mice. J. Food Biochem. 44 (4), e13164. doi: 10.1111/jfbc.13164
Yan, T., Wang, N., Liu, B., Wu, B., Xiao, F., He, B., et al. (2021). Schisandra chinensis ameliorates depressive-like behaviors by regulating microbiota-gut-brain axis via its anti-inflammation activity. Phytother. Res. 35 (1), 289–296. doi: 10.1002/ptr.6799
Yu, J. M., Kim, J. H., Song, G. S., Jung, J. S. (2006). Increase in proliferation and differentiation of neural progenitor cells isolated from postnatal and adult mice brain by wnt-3a and wnt-5a. Mol. Cell. Biochem. 288 (1-2), 17–28. doi: 10.1007/s11010-005-9113-3
Yu, Y., Wu, S., Li, J., Wang, R., Xie, X., Yu, X., et al. (2015). The effect of curcumin on the brain-gut axis in rat model of irritable bowel syndrome: involvement of 5-HT-dependent signaling. Metab. Brain Dis. 30 (1), 47–55. doi: 10.1007/s11011-014-9554-z
Yu, J. B., Zhao, Z. X., Peng, R., Pan, L. B., Fu, J., Ma, S. R., et al. (2019). Gut microbiota-based pharmacokinetics and the antidepressant mechanism of paeoniflorin. Front. Pharmacol. 10. doi: 10.3389/fphar.2019.00268
Zhang, T., Dong, K., Xiao, L., Li, G., Zhang, Z. (2020). Effects of Co-administration of icariin and panax notoginseng saponins on intestinal microbiota and hippocampal protein expression in a mouse model of alzheimer’s disease. Neuropsychiatr. Dis. Treat. 16, 2169–2179. doi: 10.2147/NDT.S253972
Zhang, J., He, H., Qiao, Y., Zhou, T., He, H., Yi, S., et al. (2020). Priming of microglia with IFN-gamma impairs adult hippocampal neurogenesis and leads to depression-like behaviors and cognitive defects. Glia 68 (12), 2674–2692. doi: 10.1002/glia.23878
Zhang, X., Huang, G., Liu, H., Chang, H., Wilson, J. X. (2012). Folic acid enhances notch signaling, hippocampal neurogenesis, and cognitive function in a rat model of cerebral ischemia. Nutr. Neurosci. 15 (2), 55–61. doi: 10.1179/1476830511Y.0000000025
Zhang, W., Lin, T. R., Hu, Y., Fan, Y., Zhao, L., Stuenkel, E. L., et al. (2004). Ghrelin stimulates neurogenesis in the dorsal motor nucleus of the vagus. J. Physiol. 559 (Pt 3), 729–737. doi: 10.1113/jphysiol.2004.064121
Zhang, X., Liu, H., Cong, G., Tian, Z., Ren, D., Wilson, J. X., et al. (2008). Effects of folate on notch signaling and cell proliferation in neural stem cells of neonatal rats in vitro. J. Nutr. Sci. Vitaminol. (Tokyo). 54 (5), 353–356. doi: 10.3177/jnsv.54.353
Zhang, L., Liu, Y., Wang, S., Long, L., Zang, Q., Ma, J., et al. (2021). Vagus nerve stimulation mediates microglia M1/2 polarization via inhibition of TLR4 pathway after ischemic stroke. Biochem. Biophys. Res. Commun. 577, 71–79. doi: 10.1016/j.bbrc.2021.09.004
Zhang, J., Rong, P., Zhang, L., He, H., Zhou, T., Fan, Y., et al. (2021). IL4-driven microglia modulate stress resilience through BDNF-dependent neurogenesis. Sci. Adv. 7 (12), eabb9888. doi: 10.1126/sciadv.abb9888
Zhang, F., Zhou, Y., Chen, H., Jiang, H., Zhou, F., Lv, B., et al. (2022). Curcumin alleviates DSS-induced anxiety-like behaviors via the microbial-Brain-Gut axis. Oxid. Med. Cell. Longev. 2022, 6244757. doi: 10.1155/2022/6244757
Zhao, J., Cheng, Y. Y., Fan, W., Yang, C. B., Ye, S. F., Cui, W., et al. (2015). Botanical drug puerarin coordinates with nerve growth factor in the regulation of neuronal survival and neuritogenesis via activating ERK1/2 and PI3K/Akt signaling pathways in the neurite extension process. CNS Neurosci. Ther. 21 (1), 61–70. doi: 10.1111/cns.12334
Zhao, Y., Lukiw, W. J. (2018). Bacteroidetes neurotoxins and inflammatory neurodegeneration. Mol. Neurobiol. 55 (12), 9100–9107. doi: 10.1007/s12035-018-1015-y
Zhao, H. B., Qi, S. N., Dong, J. Z., Ha, X. Q., Li, X. Y., Zhang, Q. W., et al. (2014). Salidroside induces neuronal differentiation of mouse mesenchymal stem cells through notch and BMP signaling pathways. Food Chem. Toxicol. 71, 60–67. doi: 10.1016/j.fct.2014.05.031
Zheng, D., Liwinski, T., Elinav, E. (2020). Interaction between microbiota and immunity in health and disease. Cell Res. 30 (6), 492–506. doi: 10.1038/s41422-020-0332-7
Zhuang, P. W., Cui, G. Z., Zhang, Y. J., Zhang, M. X., Guo, H., Zhang, J. B., et al. (2013). Baicalin regulates neuronal fate decision in neural stem/progenitor cells and stimulates hippocampal neurogenesis in adult rats. CNS Neurosci. Ther. 19 (3), 154–162. doi: 10.1111/cns.12050
Keywords: neurogenesis, gut microbiota, traditional Chinese medicine, microbiota-gut-brain axis (MGB axis), neural stem cell (NSC)
Citation: Zhang C, Xue P, Zhang H, Tan C, Zhao S, Li X, Sun L, Zheng H, Wang J, Zhang B and Lang W (2022) Gut brain interaction theory reveals gut microbiota mediated neurogenesis and traditional Chinese medicine research strategies. Front. Cell. Infect. Microbiol. 12:1072341. doi: 10.3389/fcimb.2022.1072341
Received: 17 October 2022; Accepted: 07 November 2022;
Published: 08 December 2022.
Edited by:
Lianlin Su, Nanjing University of Chinese Medicine, ChinaReviewed by:
Huantian Cui, Shandong University, ChinaYi Tao, Zhejiang University of Technology, China
Copyright © 2022 Zhang, Xue, Zhang, Tan, Zhao, Li, Sun, Zheng, Wang, Zhang and Lang. This is an open-access article distributed under the terms of the Creative Commons Attribution License (CC BY). The use, distribution or reproduction in other forums is permitted, provided the original author(s) and the copyright owner(s) are credited and that the original publication in this journal is cited, in accordance with accepted academic practice. No use, distribution or reproduction is permitted which does not comply with these terms.
*Correspondence: Weiya Lang, bGFuZ3dlaXlhQDEyNi5jb20=
†These authors have contributed equally to this work and share first authorship