Assessing in vivo and in vitro biofilm development by Streptococcus dysgalactiae subsp. dysgalactiae using a murine model of catheter-associated biofilm and human keratinocyte cell
- 1UCIBIO - Applied Molecular Biosciences Unit, Dept. Ciências da Vida, NOVA School of Science and Technology, Caparica, Portugal
- 2i4HB, Associate Laboratory - Institute for Health and Bioeconomy, Faculdade de Ciências e Tecnologia, Universidade NOVA de Lisboa, Caparica, Portugal
- 3Instituto de Microbiologia Paulo de Góes, Universidade Federal do Rio de Janeiro, Rio de Janeiro, Brazil
- 4Centro de Investigação Interdisciplinar Egas Moniz (CiiEM), Egas Moniz - Cooperativa de Ensino Superior CRL, Quinta da Granja, Portugal
Streptococcus dysgalactiae subsp. dysgalactiae (SDSD) is an important agent of bovine mastitis. This infection causes an inflammatory reaction in udder tissue, being the most important disease-causing significant impact on the dairy industry. Therefore, it leads to an increase in dairy farming to meet commercial demands. As a result, there is a major impact on both the dairy industry and the environment including global warming. Recurrent mastitis is often attributed to the development of bacterial biofilms, which promote survival of sessile cells in hostile environments, and resistance to the immune system defense and antimicrobial therapy. Recently, we described the in vitro biofilm development on abiotic surfaces by bovine SDSD. In that work we integrated microbiology, imaging, and computational methods to evaluate the biofilm production capability of SDSD isolates on abiotic surfaces. Additionally, we reported that bovine SDSD can adhere and internalize human cells, including human epidermal keratinocyte (HEK) cells. We showed that the adherence and internalization rates of bovine SDSD isolates in HEK cells are higher than those of a SDSD DB49998-05 isolated from humans. In vivo, bovine SDSD can cause invasive infections leading to zebrafish morbidity and mortality. In the present work, we investigated for the first time the capability of bovine SDSD to develop biofilm in vivo using a murine animal model and ex-vivo on human HEK cells. Bovine SDSD isolates were selected based on their ability to form weak, moderate, or strong biofilms on glass surfaces. Our results showed that SDSD isolates displayed an increased ability to form biofilms on the surface of catheters implanted in mice when compared to in vitro biofilm formation on abiotic surface. A greater ability to form biofilm in vitro after animal passage was observed for the VSD45 isolate, but not for the other isolates tested. Besides that, in vitro scanning electron microscopy demonstrated that SDSD biofilm development was visible after 4 hours of SDSD adhesion to HEK cells. Cell viability tests showed an important reduction in the number of HEK cells after the formation of SDSD biofilms. In this study, the expression of genes encoding BrpA-like (biofilm regulatory protein), FbpA (fibronectin-binding protein A), HtrA (serine protease), and SagA (streptolysin S precursor) was higher for biofilm grown in vivo than in vitro, suggesting a potential role for these virulence determinants in the biofilm-development, host colonization, and SDSD infections. Taken together, these results demonstrate that SDSD can develop biofilms in vivo and on the surface of HEK cells causing important cellular damages. As SDSD infections are considered zoonotic diseases, our data contribute to a better understanding of the role of biofilm accumulation during SDSD colonization and pathogenesis not only in bovine mastitis, but they also shed some lights on the mechanisms of prosthesis-associated infection and cellulitis caused by SDSD in humans, as well.
Introduction
Streptococcus dysgalactiae subsp. dysgalactiae (SDSD) has been considered an important bovine mastitis pathogen (Abdelsalam et al., 2010; Zadoks et al., 2011; Cervinkova et al., 2013; Rato et al., 2013) that causes severe economic repercussions over milk production. In addition, the association of SDSD with human infections has been reported (Koh et al., 2009; Park et al., 2012; Jordal et al., 2015; Chennapragada et al., 2018). Nevertheless, the role and importance of SDSD in human pathogenesis remain mostly unclear. Despite that, it is remarkable that SDSD is amongst the bacterial agents able to cause prosthetic joint infections (Park et al., 2012).
It is well known that biofilm is an important mechanism on the pathogenesis of medical device-associated infections, such as orthopedic prostheses (Ronin et al., 2022). Biofilms play an essential role in bacterial pathogenesis, promoting persistent infections and contributing to therapy failure. Biofilm formation involves various phases, including adhesion of the bacterial cells to the biotic and abiotic surfaces, in which diverse bacterial factors are involved (Kumar et al., 2017; Jamal et al., 2018). The great majority of the studies on bacterial biofilms have been based on in vitro growth on abiotic surfaces, which might be relevant for pathogens that grow on pacemakers, catheters, protheses and other implantable medical devices, increasing the risk of infections in hospital environments. Despite that, most bacterial host infections require biofilm formation on biotic surfaces as the initial stage of colonization or infection (Chao et al., 2017).
Additionally, host microenvironments, especially plasma proteins, are important for bacterial adherence to biotic or abiotic surfaces and biofilm formation during the process of a natural infection (Speziale et al., 2014). Therefore, in vivo models are important to gain a better understanding of the mechanisms involved in the development of biofilms and associated diseases. In the in vivo models of device-related infections (also called murine foreign body model), the foreign body can be inserted into the organ or into the subcutaneous space. The latter involves inserting a 1 cm segment of a catheter containing bacterium inoculum under animals’ skin (Prabhakara et al., 2011; Trøstrup et al., 2013; Kissoyan et al., 2016; See et al., 2016). Previous studies have shown that the murine model of prosthetic implant infection mediated by Staphylococcus aureus stimulates host responses like those observed in human infections (Prabhakara et al., 2011). Additionally, animal models have proved to be very useful providing excellent results in studies aimed at the development of new antibacterial agents and alternative therapies (Proetzel and Wiles, 2010; Cusumano et al., 2014). Other in vivo models of biofilm development have been described, such as those involving central venous catheter implantation in rats (Chauhan et al., 2016). This last model is more suitable for studies on the pathogenesis of bloodstream infections related to biofilm formation on catheter surfaces, while the murine model involving insertion of catheters into subcutaneous space would be more useful for studies on the role played by biofilm in foreign body infections (Prabhakara et al., 2011; Chauhan et al., 2016).
The ability of SDSD to form biofilms on abiotic surfaces was recently reported by us using confocal laser scanning microscopy, transmission electron microscopy and scanning electron microscopy (Alves-Barroco et al., 2019). We have also demonstrated for the first time that SDSD isolated from bovines can adhere to and internalize into human cells, including human epidermal keratinocyte (HEK) cells. Notably, the adherence and internalization rates of these SDSD isolates in HEK were higher than those of S. pyogenes and SDSD DB49998-05 (GCS-Si) isolates from humans (Roma-Rodrigues et al., 2016). Besides that, there are histological evidence that SDSD can cause invasive infections in a zebrafish model leading to morbidity and mortality (Alves-Barroco et al., 2018).
The first report of SDSD biofilm involvement in prosthetic joint infections in humans was provided in 2012. The patient was treated by re-implantation with an application of antibiotic-impregnated cement spacer (Park et al., 2012).
In the present work, we used BALB/c mice for a biofilm model via catheter implant to investigate the ability of SDSD isolates to form biofilms in vivo. Additionally, we compared in vivo and in vitro biofilm developments by SDSD isolates collected from bovines. We also investigated the capability of these isolates to develop biofilm on human keratinocyte (HEK) cells, since this bacterium can zoonotically infect humans causing, for example, cellulitis (Chennapragada et al., 2018). Finally, the expression profile of genes associated with virulence, including biofilm development and modulation, in other streptococci was analyzed both in vivo and in vitro to gain some insights on biofilm formation by SDSD isolates.
Materials and methods
Ethics
The animal experimentation was approved by the ethics committee on the use of animals from Centro de Ciências da Saúde, Universidade Federal do Rio de Janeiro, Brazil (#01200.001568/2013-87- CEAU).
Biofilm formation assay on abiotic surfaces
The ability to form biofilms by 37 SDSD isolates from bovine clinical mastitis obtained between 2011 and 2013 [collection II, (Alves-Barroco et al., 2021)] was evaluated on polystyrene and glass surface (borosilicate test tubes) according to previously described protocols (Genteluci et al., 2015; Alves-Barroco et al., 2019; Xu et al., 2022). For a comparative analysis, 18 SDSD isolates from bovine clinical mastitis (collection I) (Rato et al., 2013; Alves-Barroco et al., 2019), obtained between 2002 and 2003, were included in the study. Sample collection design followed the international (Directive 2010/63/EU of the European parliament, on the protection of animals used for scientific purposes) and national (Decreto-Lei n° 113/2013) welfare regulations and guidelines (ARRIVE) was previously approved by the Portuguese “Direção Geral de Alimentação e Veterinária (DGAV)” (authorization document 0421/000/000/2013). In addition, the two authors have a level C FELASA certification (Federation of European Laboratory Animal Science Associations).
To evaluate the biofilm production on glass surfaces, the bovine SDSD isolates were streaked on blood agar plates and incubated at 37 °C for 18 h in a 5% (v/v) CO2 incubator. About 5 colonies were transferred to Tryptic Soy Broth (TSB; Becton, Dickinson and Company, Le Pont de Claix, France) supplemented with 0.5% (w/v) glucose and incubated at 37 °C until the middle of the exponential growth phase. The pure culture (OD570 = 0.6) was diluted 1:40 in a glass test tube (16 x 1,05 x 100 mm, NORMAX, Portugal) containing TSB supplemented with glucose (final volume 4 mL) and incubated at 37 °C for 20 h. Then, the supernatant was removed, and the glass tube washed with sterile saline solution [0.85% (w/v) NaCl]. The tubes were incubated at 65 °C for 1 h for drying. Biofilms were resuspended in 4 mL of saline and the OD at 600 nm measured. The isolates were defined as non-biofilm producers: OD600 ≤ 0.099, weak: OD600 between 0.1–0.299, moderate: between 0.3–0.599, or strong biofilm producers: OD600>0.600.
On polystyrene surfaces, after growing to the middle of the exponential phase, the bacterial culture (OD570 = 0.6) was diluted 1:2 in TSB supplemented with glucose in a 96 well plate (final volume 200 µL/well). The 96 well plate was sealed and incubated at 37 °C for 20 h. The supernatant was removed, and the wells washed with saline to remove non-adherent bacteria. Then, the plates were incubated at 65 °C for 1 h for drying and fixing biofilm. The biofilm was stained with crystal violet 1% (w/v) for 1 min. The wells were washed with sterile distilled water until the dye from the negative control-wells was completely removed. The OD570 of the stained biofilm was directly measured in a plate reader (Infinite M200, Tecan, Männedorf, Switzerland). Interpretation of biofilm formation was performed according to the criteria previously described (Alves-Barroco et al., 2019) and the isolates were therefore categorized as follows: non producer: OD ≤ ODctrl, (ODctrl = 0.060); weak producer: ODctrl < OD ≤ 2 × ODctrl; moderate producer: 2 × ODctrl < OD ≤ 4 × ODctrl. strong producer: OD > 4 × ODctrl
SDSD biofilm formation on human keratinocyte cells
This assay was based on previously described protocols (Roma-Rodrigues et al., 2015) with few modifications. Bacteria were grown at 37°C in Todd Hewitt Broth (THB; Oxoid; Basingstoke, UK) supplemented with 0.5% (w/v) yeast extract until the middle exponential growth phase. The infection was started by adding bacterial suspension (containing 106 bacterial cells) in Dulbecco’s Modified Eagle’s Medium (DMEM; ThermoFisher Scientific; Waltham, MA, USA) supplemented with 10% (v/v) fetal bovine serum (ThermoFisher Scientific) to 104 human epidermal keratinocyte (HEK) cells (ATCC-PCS-200-010, ATCC, Manassas, VA, USA). The infected culture was incubated at 37 °C, 5% (v/v) CO2, and 99% relative humidity. After 2 h and 4 h of incubation, HEK cells were washed with phosphate buffer saline (PBS, 137 mM NaCl, 2.7 mM KCl, 10 mM Na2HPO4, and 1.8 mM KH2PO4, pH 7.4) (Sigma-Aldrich, St. Louis, MO, USA), to remove non-adhered bacteria, and then fixed with 2% (v/v) glutaraldehyde (Sigma-Aldrich) in PBS for 2 h at room temperature. The HEK cells were washed with PBS (three times), post-fixed with 1.0% (w/v) osmium tetroxide (Sigma-Aldrich) at 4°C for 1 h, and then processed as previously described (García-Pérez et al., 2003). The infected HEK cells were visualized using a scanning electron microscope (JEOL JSM-5400).
Viability of HEK cells was determined using MTS [3-(4,5-dimethylthiazol-2-yl)-5-(3-carboxymethoxyphenyl)-2-(4-sulfophenyl)-2H-tetrazolium, inner salt] assay as previously described (Fernandes et al., 2017). HEK cells were seeded in 96-well plates (ThermoFisher Scientific) and grown at 37 °C, 5% (v/v) CO2, and 99% relative humidity for 24 h, before incubations in the same conditions for 2 h, 4 h, and 6 h with SDSD cells or bacterial growth supernatant. After the incubation period, the culture medium was removed and, after washing HEK cells with PBS, fresh medium containing 10% MTS reagent (ThermoFisher Scientific) was added to each well. The 96-well plate was incubated at 37°C, in the same atmosphere, for 60 min. The absorbance (Abs) was measured in a microplate reader at 490 nm (Infinite M200, Tecan, Männedorf, Switzerland). The following equation was applied: cell viability (%) = 100 x [mean Abs of SDSD cells (or mean Abs bacterial growth supernatant)/mean Abs of control group without SDSD cells or bacterial growth supernatant].
In vitro biofilm formation on the surface of an intravenous catheter segment
Exponentially growing SDSD cells in TSB supplemented with 0.5% (w/v) glucose were harvested by centrifugation and diluted in the fresh broth. A volume of 10 μL [containing 104 colony forming units (CFU)] was injected into the lumen of a 1 cm segment of the polyurethane catheter (C-953-J-UDLM; Cook Inc., Bloomington, IN, USA). Then, the catheter was placed into the well of a 24-well plate and incubated for 72 h. To count the SDSD cells adhered, the catheter was washed with PBS twice, to remove non-adherent bacteria, and placed in fresh broth. After sonication (15 min; 38.5–40.5 kHz, in ice), the CFU/mL was determined using Todd Hewitt Agar (THA). Biofilm was assessed by counting the SDSD cells adhered to the catheter.
In vivo biofilm formation
The in vivo assays using a mouse foreign-body model were performed as described in Genteluci et al., 2015. Briefly, young adult BALB/c mice (age between 8 to 10 weeks), obtained from NCAL-UFRJ (https://ccs.ufrj.br/paginas/sobre-o-ccs/coordenacoes/cambe), were anesthetized, and a subcutaneous incision was created to introduce a 1 cm segment of a polyurethane intravenous catheter containing 10 μL of the bacterial suspension (106 CFU). The catheter was implanted subcutaneously (at least 1.5 cm from the incision). after 72 hours of infection, the animals were euthanized, and the catheter segments removed. After that, the catheter segments were washed with 0.15 M NaCl to remove any planktonic bacteria and placed in a tube containing 1 mL of saline. After sonication (15 min, 38.5–40.5 kHz, in ice), CFU/mL was determined using THA. Biofilm was assessed by counting the SDSD cells adhered to the catheter.
To investigate whether animal passage can increase the ability of SDSD to accumulate biofilms in vitro, cells collected from the catheter implanted in the mice were inoculated in TSB containing 0.5% (w/v) glucose at 37 °C for 18 h. Then, aliquots (with and without animal passage) were obtained to assess biofilm formation on the glass surface following the protocol described above.
Reverse transcription quantitative PCR
Expression levels of genes associated with biofilm formation in other streptococci were evaluated using sessile cells recovered from in vivo and in vitro biofilms. RNA was extracted using NucleoSpin RNAII kit (Macherey-Nagel, Dueren, Germany) according to the manufacturer’s instructions to comparatively quantify transcripts of genes encoding BrpA-like (biofilm regulatory protein), FbpA (fibronectin-binding protein A), HtrA (serine protease), and SagA (streptolysin S precursor). The cDNA was synthesized using the SuperScript first-strand synthesis system (Invitrogen) according to the manufacturer’s instructions. The RT-qPCR reaction mixture (20 μL) contained NZY qPCR Green Master Mix (NZYTech, Lisbon, Portugal), 1 μL cDNA, and 0.5 μM of the forward and reverse primers described in Table 1. PCR conditions included an initial denaturation for 10 min at 95°C, followed by 30 cycles of amplification consisting of denaturation for 15 s at 95°C, and annealing for 30 s at 58°C and extension for 45 s at 60 °C. The critical Ct was defined as the cycle in which fluorescence becomes detectable above the background fluorescence. The expression levels were normalized using the 16S rRNA gene as an internal standard. Each assay was performed with at least three independent RNA samples.
Statistical analysis
GraphPad Prism version 7.0 was used for statistical analysis. All data were expressed as mean ± SEM from at least three independent (biological) experiments. The statistical significance was determined for each data set using the student’s t-test, and statistical significance was considered when p < 0.05.
For comparison purposes, biofilm developments between SDSD isolates recovered from catheter implanted in mice (in vivo) and in vitro assay were both measured by CFU. In the case of biofilm developments by SDSD on glass surfaces before and after animal passage, biofilm growth was measured by OD determination.
Results and discussion
Biofilm formation assay on abiotic surfaces
As a first approach, the ability of 37 SDSD isolates (collection II) to form biofilms on glass and polystyrene surfaces was evaluated. For a comparative analysis, the results obtained for 18 SDSD isolates also obtained from bovines (collection I), shown in Alves-Barroco et al. (2019), were included in the study. Overall, despite some differences, the results obtained point to a high in vitro biofilm-forming ability by most SDSD isolates with isolates from the collection II showing a greater ability to accumulate biofilms than those from the collection I (Figure 1).
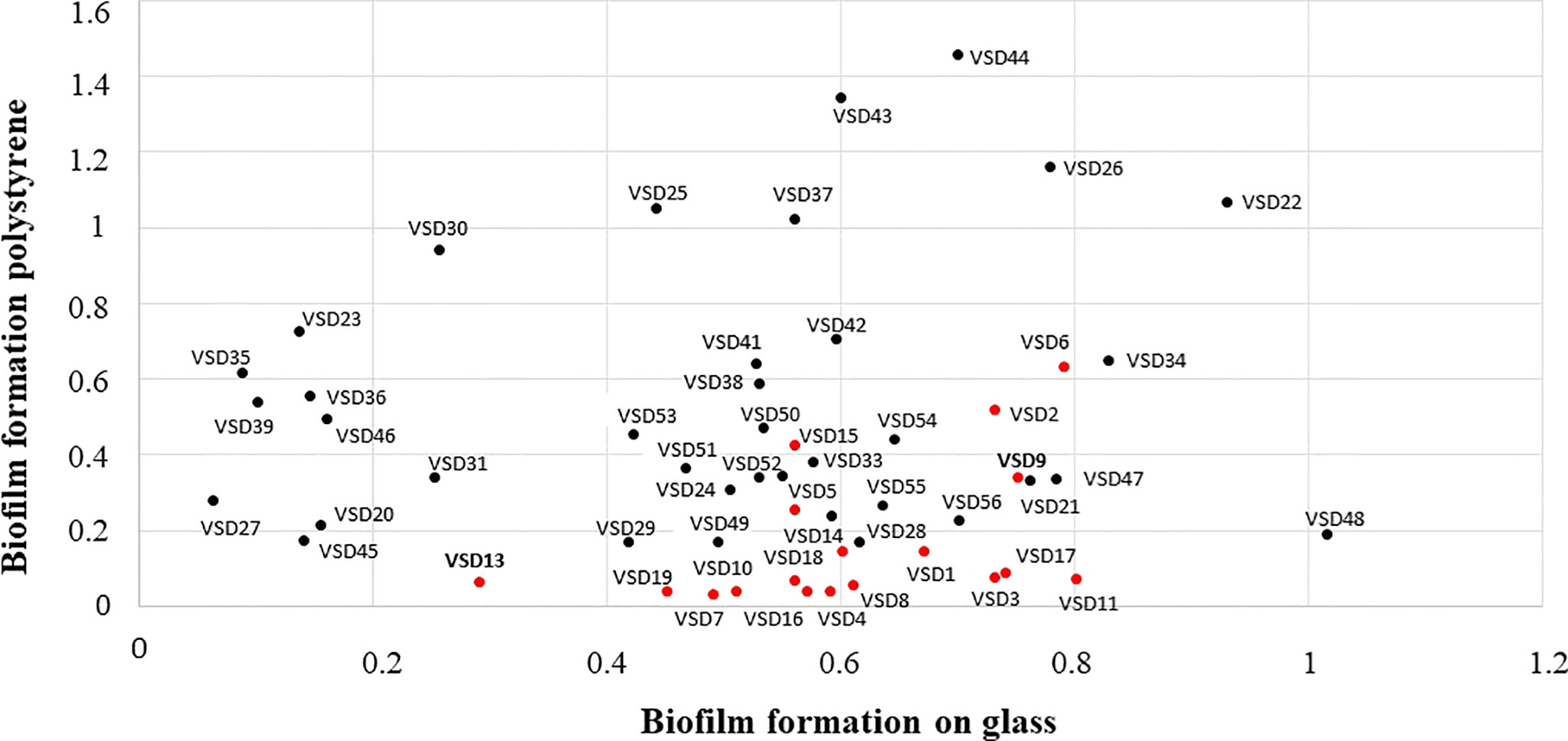
Figure 1 Comparison of the in vitro ability to form biofilms on abiotic surfaces by bovine SDSD isolates of clinical and subclinical mastitis in Portugal, during 2002-2003 (collection I, red circles) and 2011-2013 (collection II, black circles). Interpretation criteria for biofilm formation on polystyrene surface: i) non-producer: OD ≤ ODctrl; ii) weak producer: OD ≤ ODctrl x 2; iii) moderate producer: ODctrl x 2 < OD ≤ ODctrl x 4; and iv) strong producer: OD > ODctrl x 4 Interpretation criteria for in vitro biofilm formation on glass surface: i) non-producer: OD600 ≤ 0.099; ii) weak producer; OD600 ≥ 0.1 ≤ 0.299; iii) moderate producer OD600: ≥ 0.3 ≤ 0.599; and iv) strong producer OD600 > 0.600. ODctrl = DO determined for the control.
SDSD biofilm formation on human keratinocyte cells
HEK cells have an important role in host defense, providing a physical and immunological barrier against pathogenic bacteria. The adherence and/or invasion of Group G streptococci in HEK cells was associated with the severity of skin infections, e.g., necrotizing fasciitis (Siemens et al., 2015). We previously reported for the first time that bovine SDSD isolates are capable to adhere and internalize several human cells, including HEK cells (Roma-Rodrigues et al., 2016); Alves-Barroco et al., 2018. Here, the ability of SDSD isolates to form biofilms on HEK cells was analyzed by scanning electron microscope (Figure 2). Our results demonstrated the formation of an extracellular polymeric matrix by the growth of SDSD biofilms after 2 h of infection of HEK cells (Figure 2A). After 4 h of infection, it was possible to visualize a typical biofilm architecture (Figure 2B).
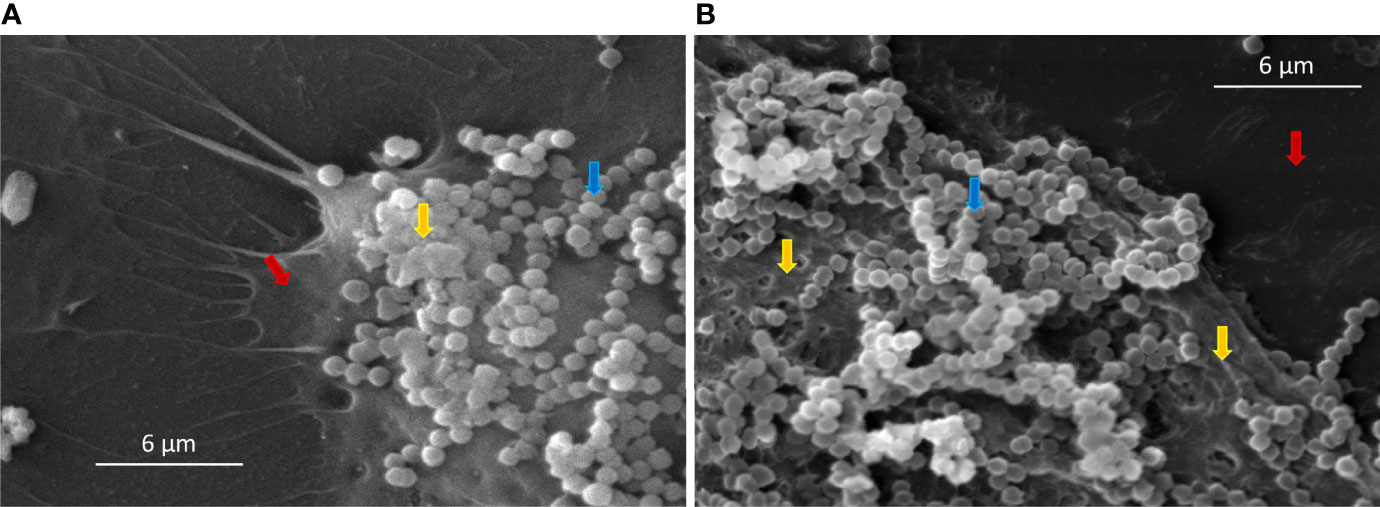
Figure 2 Scanning electron microscopy (SEM) of SDSD biofilms formed by VSD13 after (A) 2 h and (B) 4 h on human keratinocyte cells. Blue arrow: SDSD VSD13 cells; yellow arrow: formation of the extracellular polymeric matrix; red arrow: human keratinocyte cells.
Similar results were observed by Matsue and co-workers in investigations with other streptococci. They showed that after 2 h incubation of HEK cells with Streptococcus dysgalactiae subsp. equisimilis (SDSE), the percentage of adhered bacterial was on average 70%. Furthermore, the adherence of SDSE on HEK cells was about 10 times higher than that on polystyrene surfaces (Matsue et al., 2020). Previous studies have also shown the formation of biofilms by Streptococcus pyogenes in HEK cells. Visually, S. pyogenes biofilms formed on HEK cells were similar to biofilms on abiotic surfaces; however, S. pyogenes biofilms on HEK cells were more resistant to antimicrobial therapy (Marks et al., 2014). Marks and co-workers demonstrated that during coculture, the S. pyogenes biofilm extended about 20–30 µm above the HEK cells; however, S. pyogenes biofilms did not induce HEK cell death, as the keratinocytes layer remained intact during the experiment (Marks et al., 2014). Contrary to what was observed for S. pyogenes (Marks et al., 2014), SDSD biofilm induced a decline in viability of the HEK cells over time (Figure 3). After 6 h incubation with VSD13 isolate grown in biofilm condition or with its filtered culture supernatant, the viability of HEK cells was 32% and 86%, respectively. Our results also showed that the development of biofilms on the HEK cell monolayers exhibited greater cytotoxicity than extracellular products from bacterial growth (Figure 3). Thus, these data could indicate that SDSD biofilm may develop during the process of skin/soft tissue infections, suggesting that it might be important in the pathogenesis of human SDSD cellulitis.
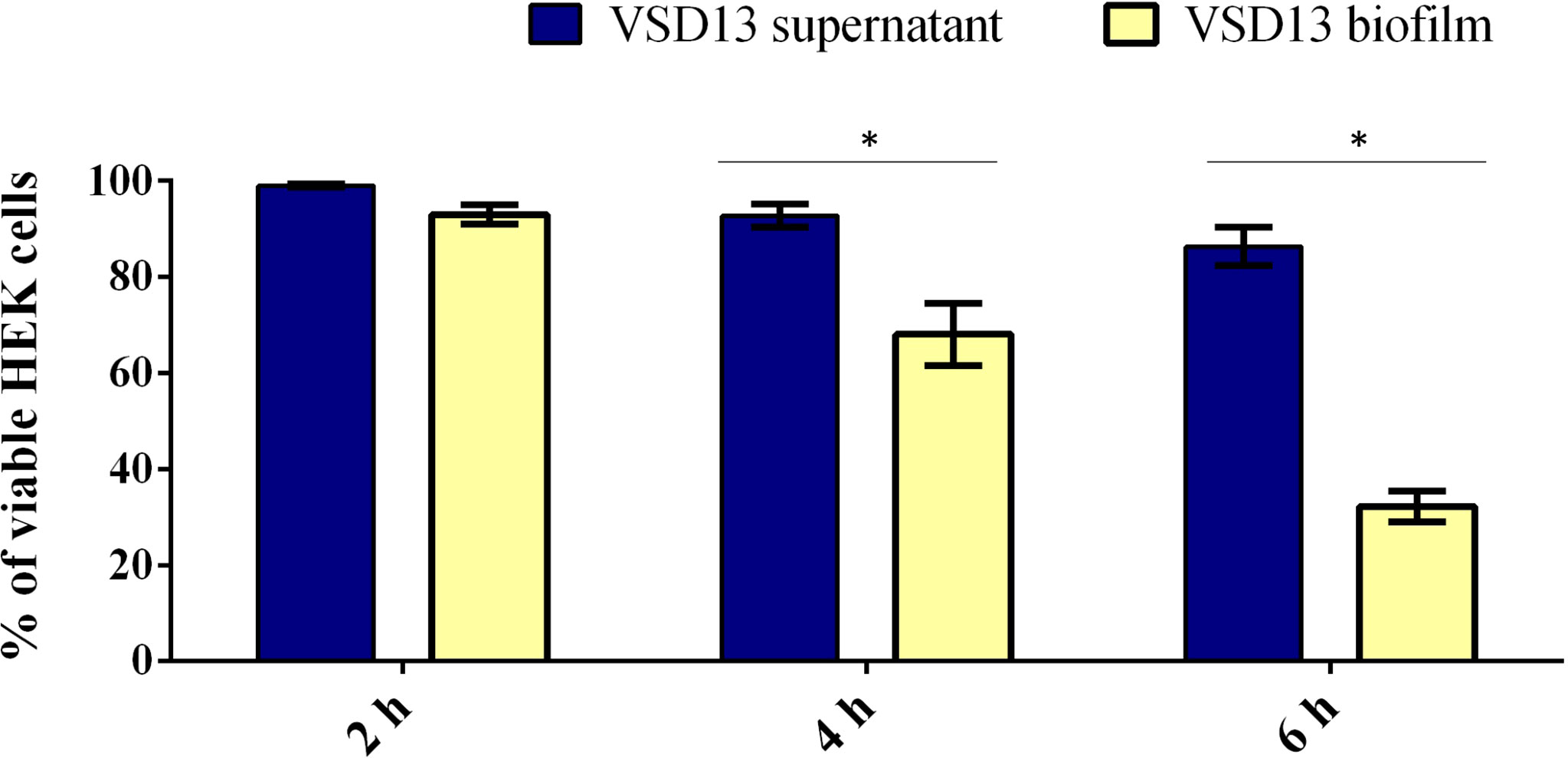
Figure 3 Viability of HEK cells exposure to SDSD VSD13 sessile cells or bacterial supernatants for 2 h, 4 h and 6 h. The following equation was applied: cell viability (%) = 100 x [mean Abs SDSD cells (or mean Abs bacterial growth supernatant)/mean Abs control group]. Data are the average of at least three independent (biological) assays with three technical replicates each. Error bars correspondent to standard deviation. Statistically significant differences were observed in the viability of HEK cells exposure to SDSD VSD13 sessile cells and bacterial supernatants at 4 h and 6 h, * p < 0.05.
Together, they suggest an important role for SDSD biofilm formation on HEK cells, which may contribute to the development of deeper tissue infections and bacterial dissemination. In fact, in recent years, the association of SDSD with human infections such as cellulitis has been reported (Chennapragada et al., 2018; Nathan et al., 2021), and one case of cellulitis rapidly progressed to septic shock (Nathan et al., 2021). Indeed, we have recently reported for the first time the resistance to conventional antibiotics associated with biofilm formation by SDSD (Alves-Barroco et al., 2022), which may complicate the treatment of some infections associated with this subspecies.
In vivo Biofilm Formation
To reduce the number of sacrificed animals and to compare in vivo and in vitro biofilm formation and accumulation, SDSD isolates were selected based on their in vitro ability to form strong (n=2; isolates VSD9 and VSD22; collection I and II, respectively), moderate (n=1; isolate VSD16), or weak (n=2; isolates VSD13 and VSD45; collection I and II, respectively) biofilms on the glass surface. All SDSD isolates tested, including weak biofilm producers in vitro, were able to develop biofilm in vivo (Figure 4).
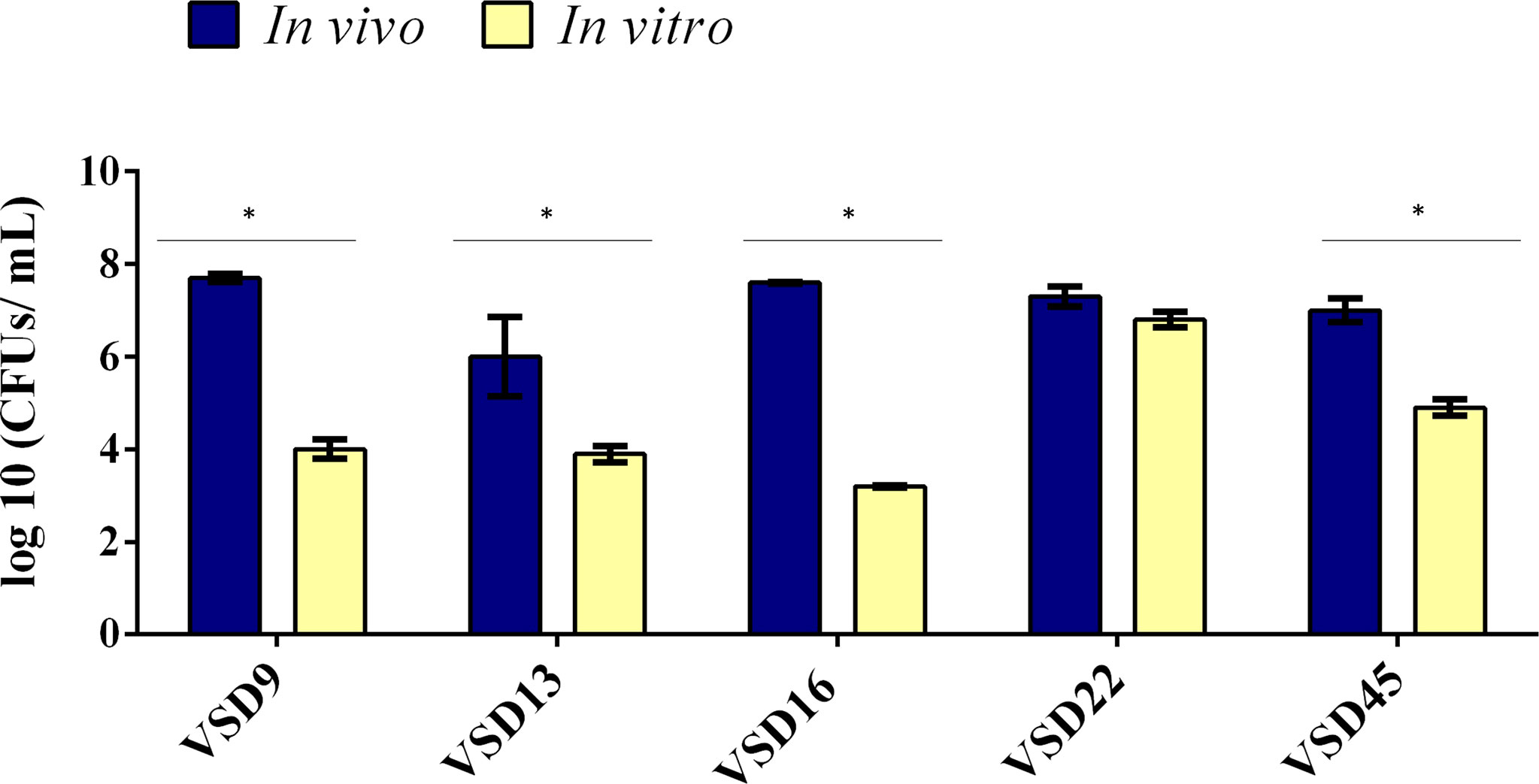
Figure 4 Comparison of biofilm development by bovine SDSD isolates recovered from catheter implanted in mice and by the in vitro assay. Statistically significant differences were observed in the formation of biofilms in vivo and in vitro, * p < 0.05.
The results showed an important increased ability to develop biofilm on catheter implanted in mice compared with the respective biofilm formed in vitro, except for the SDSD isolate VSD22, which already produced a very strong biofilm in vitro. Overall, the results suggest that the capability of SDSD isolated from bovines to develop strong biofilm in vivo is independent of the ability to form biofilms in vitro on abiotic surfaces. A possible limitation of this study is the fact that we used a collection of SDSD isolates from 2002 to 2013. However, it is important to emphasize that our results were not influenced by SDSD collection period, being similar for the isolates obtained in 2002-2003 or 2011-2013. Indeed, our data contribute for a better understanding of the pathogenic mechanisms of diseases not only in animals but also in humans, such as cellulitis and prosthetic joint infections that happened during these periods (Koh et al., 2009; Park et al., 2012).
The quantification of in vitro versus in vivo biofilms (CFU/mL) varied, respectively, from 9.8 x103 to 4.0 x107 for VSD9 isolate, from 8.0 x103 to 7.8 x106 for VSD13 isolate, from 1.8 x103 to 3.4 x106 for VSD16 isolate, and from 7.4 x104 to 6.4 x106 for VSD45 isolate. An increased ability (approx. 2.2 times) to form biofilm in vitro (glass surface) after animal passage was observed for the SDSD isolate VSD45 (weak biofilm producer in vitro, Figure 5), but not for the other isolates tested.
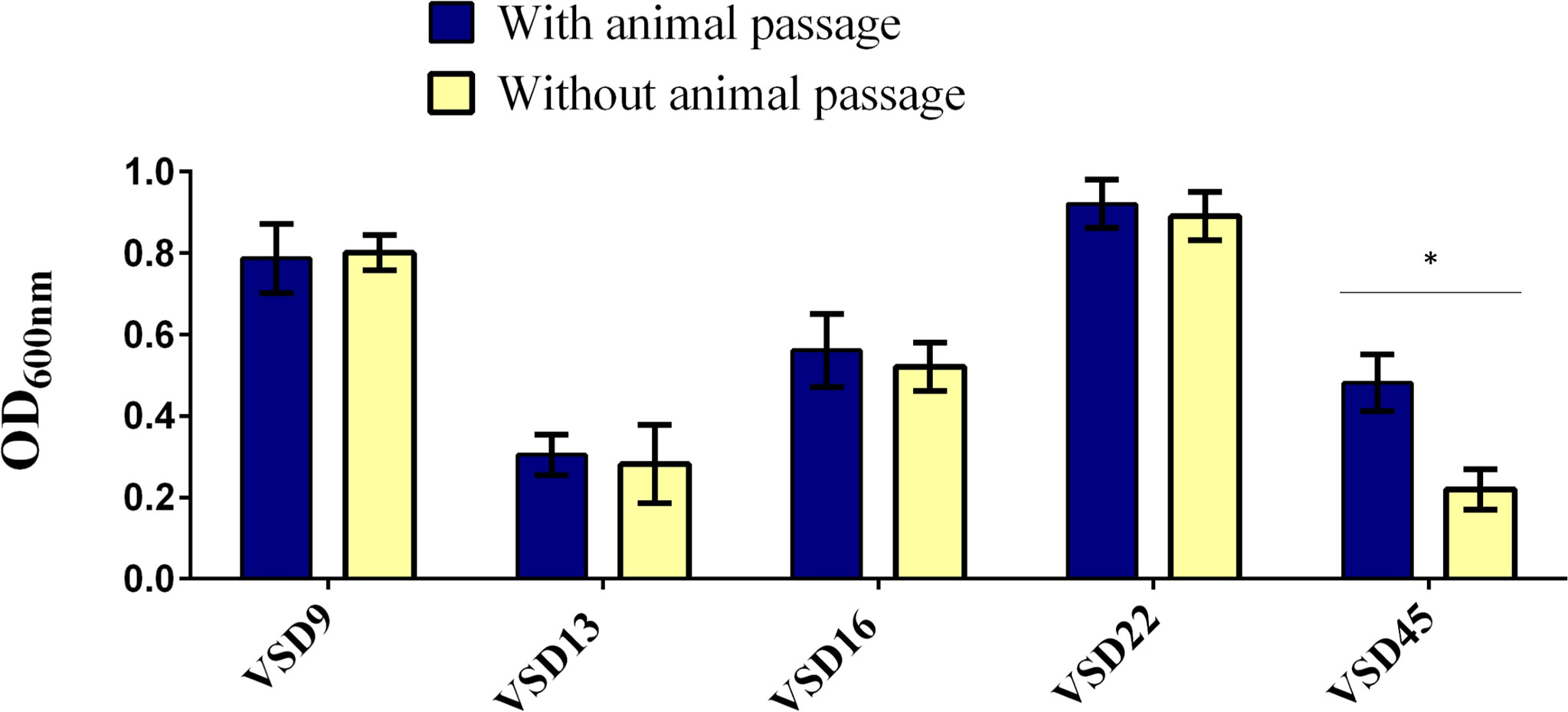
Figure 5 Biofilm development on glass surfaces by the representative biofilm producers with and without animal passage. Statistically significant differences were observed in the formation of biofilms after animal passage for VSD45, * p < 0.05. No significant differences were observed in the biofilm development on glass surfaces after animal passage for VSD9, VSD13, VSD16 and VSD22.
Once the role of biofilms in infections was recognized, different in vitro and in vivo models of infections were developed. The in vitro models, although more simplistic, contributed to the current knowledge of the biofilm. These models are also used to investigate the role of genes involved in biofilm formation and to screen antimicrobial agents capable of disintegrating bacterial biofilms. However, in vitro models ignore important parameters and host factors. The in vivo models have contributed to a better understanding of bacterial adhesion, invasion and cytotoxicity factors, as well as the mechanisms involved in host inflammatory responses. There is no gold-standard model since each model can provide a specific answer. Information about models of biofilm-related infections and their applications is reviewed in Lebeaux et al., 2013.
The foreign-body mouse model used in the present work has also been successfully applied in previous studies with different bacterial species to analyze the ability of bacteria to form biofilms (Ferreira et al., 2012; Marks et al., 2014; Genteluci et al., 2015). Genteluci and co-workers showed that SDSE isolates can form biofilms in vivo, regardless of their ability to form biofilms in vitro. Marks and co-workers showed that S. pyogenes non-biofilm producers on abiotic surfaces can form biofilms on epidermal cells with characteristics similar to an in vivo colonization or infection (Marks et al., 2014). Although far from a complete understanding of the multiple factors that control the interactions between the pathogen and the host, animal models provide a better understanding of the biofilm within the context of the host. Taking all our data together, it can be concluded that in vivo growth increases the SDSD ability to form biofilms, possibly reflecting an important impact during some SDSD infections in bovine and in human hosts.
Expression Profiles of Genes Associated With Biofilm Formation
In vivo colonization by the group of pyogenic streptococci requires a series of interactions between the pathogen and the host, involving differential gene expression in both (Alves-Barroco et al., 2020). Our data revealed that in vivo, a similar number of sessile cells were recovered from SDSD isolates previously classified as weak and strong biofilm producers in vitro. This difference might be explained by changes in gene expression profiles associated with the regulation of biofilm formation (Marks et al., 2014). To test this hypothesis, we compared the expression of some biofilm-associated genes in sessile cells of SDSD isolates grown in vivo and in vitro; except for VSD22 isolate, as no difference was observed between in vivo and in vitro biofilm formation. Indeed, a remarkably increased expression was observed for the genes encoding BrpA-like (biofilm regulatory protein) and FbpA (fibronectin-binding protein A) for all bacterial biofilms collected from catheters recovered from the mice model (Figures 6A, B). The brpA-like gene was upregulated ~182, 112, 335, and 144-fold for VSD9, VSD13, VAS16, and VSD45, respectively, while fbpA was upregulated ~369, 822, 1419, and 708-fold for VSD9, VSD13, VAS16, and VSD45, respectively. The mRNA expression of htrA (encoding serine protease) was more dramatically increased for VSD9 (796-fold) and VSD13 (1441-fold) isolates, and mRNA expression of sagA (encoding streptolysin S percussor) for VSD13 isolate (~9.8-fold) (Figures 6C, D).
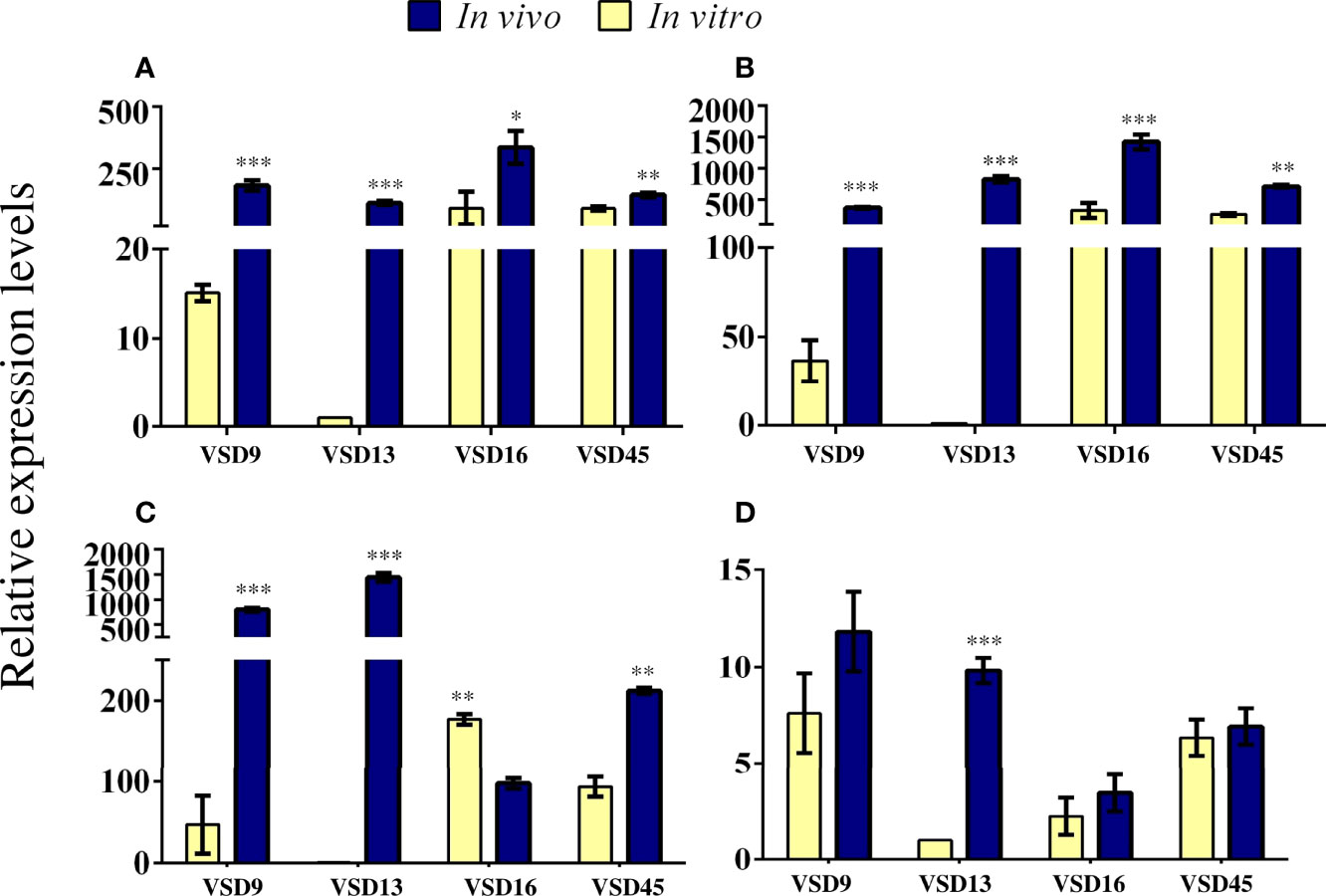
Figure 6 Relative expression levels of (A) brpA-like (B) fbpA (C) htrA and (D) sagA genes in sessile cells generated in vivo compared with those formed in vitro. RT-qPCR was expressed as the mean of three biologically independent experiments. The bar represents the standard deviation. The calibration sample was the cDNA for VSD13 biofilm grown in vitro. Statistically significant differences were observed for gene expression in SDSD biofilm grown in vivo or in vitro: * p < 0.05; ** p < 0.01; *** p < 0.001.
The role of the biofilm regulatory protein A (BrpA) in autolysis and cell division of the Streptococcus mutans has been shown. In vitro, the BrpA-deficient mutant of S. mutans maintained its adherence property, but the ability to form biofilms was considerably affected. Additionally, the deficiency of BrpA impaired cell envelope stress responses and acid and oxidative stress tolerance. (Bitoun et al., 2012; Bitoun et al., 2014). The biofilm-producing SDSD isolates carry a brpA-like gene, which expression level was associated with the ability to form biofilms jn vitro (Alves-Barroco et al., 2019). Therefore, our data showing a parallel increase of biofilm accumulation and brpA-like gene expression in the in vivo model corroborate a role played by this gene in the development of biofilm by SDSD.
Studies estimated that initial adherence to host cells is mainly mediated by adhesins, such as fibronectin-binding proteins, that allow adhesion to provide biofilm development on host tissues and/or bacterial internalization into host cells (Cue et al., 2000; Šmitran et al., 2018). Several fibronectin-binding proteins are produced by S. dysgalactiae, with different binding affinities and properties (Alves-Barroco et al., 2020). These proteins provide adherence to human cells such as fibroblasts and keratinocytes cells, contributing to biofilm development in vivo and consequently to persistent infections (Collin and Olsén, 2003; Brandt and Spellerberg, 2009). In this work, we observed an increased expression of fibronectin-binding protein A (fbpA) gene for sessile cells of SDSD grown in vivo compared with in vitro (Figure 6B). These results corroborate studies showing the important role of fibronectin-binding proteins in biofilm formation (Brandt and Spellerberg, 2009; O'Neill et al., 2009).
The high-temperature requirement protein A (HtrA, also known as DegP), is a serine protease widely distributed among streptococci (Alves-Barroco et al., 2020). HtrA homologs are responsible for the degradation of abnormal proteins in response to environmental stress. These proteins have also been identified in Gram-positive isolates (Kim and Kim, 2005). In Streptococcus mutans, the deletion of the htrA gene causes decreased ability to respond to environmental stress (Diaz-Torres and Russell, 2001). In S. pyogenes, the deletion of htrA gene affected the expression of several virulence genes (Lyon and Caparon, 2004). In addition to the proteolytic properties, this enzyme can adhere to the extracellular matrix of host tissues (Lyon and Caparon, 2004; Kim and Kim, 2005). Herein, differential expression of the htrA gene was observed between in vitro and in vivo biofilms. Isolates VSD9, VSD13 and VSD45 exhibited an increased htrA gene expression in vivo (Figure 6C). Interestingly, the VSD16 isolate exhibited a decreased expression of this gene (Figure 6C). Unlike other VSD isolates, such as VSD9 and VSD10, which produce extracellular matrices mostly composed of protein, VSD16 biofilm shows mucus-like material in the biofilm extracellular matrix (Alves-Barroco et al., 2019), suggesting that the matrix might be formed mostly by extracellular DNA (eDNA) or by complexes of eDNA and proteins (Alves-Barroco et al., 2019). Taken together, these results indicate that the VSD16 isolate may have a different biofilm formation pathway.
The sagA gene encodes the mature streptolysin S (SLS) toxin responsible mainly for the β-hemolytic activity among the pyogenic group of streptococci (Datta et al., 2005; Molloy et al., 2011). The SLS operon encodes the sagA gene (the structural propeptide), followed by genes that provide the conversion of SagA propeptide into SLS (sagB to D), leader cleavage (sagE), and transport across the membrane (sagF to I). The S. pyogenes SLS causes host soft-tissue damages, impacts phagocytes, and contributes to translocation across the epithelial barrier (Molloy et al., 2011). SLS also promotes programmed cell death and enhances inflammation in HEK cells (Flaherty et al., 2015). Studies revealed that SLS promotes host-associated biofilm formation (Vajjala et al., 2019), besides inducing mitochondrial damage and consequently macrophage death (Tsao et al., 2019). Datta and co-works reported that all SLS operon is required for the functional expression of streptolysin S (Datta et al., 2005). The loss of SagB-I observed in SDSD isolates from bovine origin is associated with loss of β-hemolytic activity (Alves-Barroco et al., 2021); however, the sagA gene has been maintained in the bovine SDSD genome, which possibly indicates an additional function to the product of this gene (Alves-Barroco et al., 2021). Some studies suggested that SagA plays an important role in the regulation of several virulence determinants, including M proteins (Datta et al., 2005; Molloy et al., 2011). The mechanisms by which sagA mRNA regulates virulence in Streptococcus have been the subject of investigations. In the present study, a high and significant increase in sagA expression was observed in vivo for the sessile cells of VSD13 isolate (Figure 6D), suggesting that the regulation of sagA expression may differ in a strain-specific manner.
The multiple factors that control the interactions between the pathogen and the host is still far from being fully understood. Nonetheless, the animal models provide better knowledge of biofilm within the host context. Importantly, the in vivo biofilm growth of SDSD isolates trigger distinct stress pathways that lead to the upregulation of the brpA-like, fbpA, htrA, and sagA genes that may play an important role in the host colonization and infection. Although previous studies suggest that SDSD may have different host preferences (Porcellato et al., 2021), together, the results presented in the present work suggest that SDSD isolates from bovine origin are able to infect other hosts, and may have a potential zoonotic capability.
Conclusions
To the best of our knowledge, the present study demonstrates for the first time in literature the ability of SDSD collected from bovines to form biofilm in vivo and suggests that the mechanism underlying biofilm development appears to be multifactorial. Despite that, the increase of fbpA transcripts in all sessile cells grown in vivo suggest a possible role for fibronectin-binding protein A in biofilm formation/accumulation. Indeed, the number of brpA-like gene transcripts was also higher in sessile cells corroborating a role of biofilm regulatory protein A in biofilm modulation of SDSD. Thus, future studies with knockout mutants are important to define exactly the role of each of these genes in biofilm development and SDSD-associated infections. In this work, we demonstrated that the capability of bovine SDSD to develop strong biofilm in vivo is independent on the ability to form biofilms in vitro on the abiotic surface. Moreover, we also provide data that show that bovine SDSD can form biofilms ex vivo on the surface of HEK cells causing important cellular damages. Due to SDSD ability to cause severe zoonotic infections, our data contribute to a better understanding of the role of biofilm accumulation during SDSD colonization and pathogenesis in human skin infections and possibly in bovine mastitis as well. Additional studies are required toward a better understanding of the mechanisms associated with the regulation of biofilm formation by SDSD isolates and the precise role of biofilm development in SDSD infections.
Data availability statement
The original contributions presented in the study are included in the article/supplementary material. Further inquiries can be directed to the corresponding authors.
Ethics statement
The animal study was reviewed and approved by Centro de Ciências da Saúde, Universidade Federal do Rio de Janeiro, Brazil (#01200.001568/2013-87- CEAU).
Author contributions
AMF and ARF were involved in the study conception and design, coordination, and revision of the final version of the manuscript. CA-B contributed to biofilm formation assay in vivo and in vitro and analysis of the expression of genes associated with the formation of biofilms, and statistical analysis. CA-B and APM contributed to analysis of SDSD biofilm formation in human keratinocyte cell. CA-B, MA, BF-C and SF contributed to subcutaneous catheter implantation in the animal model. CA-B and MG contributed to biofilm formation on glass and polystyrene assay. CA-B wrote the draft version of the manuscript. All authors read and corrected the manuscript.
Funding
This work was supported in part by grants # 307672/2019-0 from Conselho Nacional de Desenvolvimento Científico e Tecnológico (CNPq); # E-26/200.952/2021, # E-26/010.002435/2019 and # E-26/010.001280 from Fundação de Amparo à Pesquisa do Rio de Janeiro (FAPERJ); and # 001 from Coordenação de Aperfeiçoamento de Pessoal de Nível Superior (CAPES). This work is also financed by national funds from FCT - Fundação para a Ciência e a Tecnologia, I.P., in the scope of the project UIDP/04378/2020 and UIDB/04378/2020 of the Research Unit on Applied Molecular Biosciences - UCIBIO and the project LA/P/0140/2020 of the Associate Laboratory Institute for Health and Bioeconomy - i4HBand also by projects PTDC/CVT-EPI/4651/2012 and PTDC/CVT-EPI/6685/2014. FCT-MEC is also acknowledged for grant SFRH/BD/118350/2016 to CA-B.
Conflict of interest
The authors declare that the research was conducted in the absence of any commercial or financial relationships that could be construed as a potential conflict of interest.
Publisher’s note
All claims expressed in this article are solely those of the authors and do not necessarily represent those of their affiliated organizations, or those of the publisher, the editors and the reviewers. Any product that may be evaluated in this article, or claim that may be made by its manufacturer, is not guaranteed or endorsed by the publisher.
References
Abdelsalam, M., Chen, S. C., Yoshida, T. (2010). Dissemination of streptococcal pyrogenic exotoxin G (spegg) with an IS-like element in fish isolates of Streptococcus dysgalactiae. FEMS Microbiol. Lett. 309, 105–113. doi: 10.1111/j.1574-6968.2010.02024.x
Alves-Barroco, C., Caço, J., Roma-Rodrigues, C., Fernandes, A. R., Bexiga, R., Oliveira, M., et al. (2021). New insights on Streptococcus dysgalactiae subsp. dysgalactiae isolates. Front. Microbiol. 12. doi: 10.3389/fmicb.2021.686413
Alves-Barroco, C., Paquete-Ferreira, J., Santos-Silva, T., Fernandes, A. R. (2020). Singularities of pyogenic streptococcal biofilms - from formation to health implication. Front. Microbiol. 11. doi: 10.3389/fmicb.2020.584947
Alves-Barroco, C., Rivas-García, L., Fernandes, A. R., Baptista, P. V. (2022). Light triggered enhancement of antibiotic efficacy in biofilm elimination mediated by gold-silver alloy nanoparticles. Front. Microbiol. 13. doi: 10.3389/fmicb.2022.841124
Alves-Barroco, C., Roma-Rodrigues, C., Balasubramanian, N., Guimarães, M. A., Ferreira-Carvalho, B. T., Muthukumaran, J., et al. (2019). Biofilm development and computational screening for new putative inhibitors of a homolog of the regulatory protein BrpA in streptococcus dysgalactiae subsp. dysgalactiae. IJMM 309 (3-4), 169–181. doi: 10.1016/j.ijmm.2019.02.001
Alves-Barroco, C., Roma-Rodrigues, C., Raposo, L. R., Brás, C., Diniz, M., Caço, J., et al. (2018). Streptococcus dysgalactiae subsp. dysgalactiae isolated from milk of the bovine udder as emerging pathogens: In vitro and in vivo infection of human cells and zebrafish as biological models. MicrobiologyOpen 8 (1), e00623. doi: 10.1002/mbo3.623
Bitoun, J. P., Liao, S., Xie, G. G., Beatty, W. L., Wen, Z. T. (2014). Deficiency of BrpB causes major defects in cell division, stress responses and biofilm formation by Streptococcus mutans. Microbiol. (Reading England) 160 (Pt 1), 67–78. doi: 10.1099/mic.0.072884-0
Bitoun, J. P., Liao, S., Yao, X., Ahn, S. J., Isoda, R., Nguyen, A. H., et al. (2012). BrpA is involved in regulation of cell envelope stress responses in Streptococcus mutans. Appl. Environ. Microbiol. 78 (8), 2914–2922. doi: 10.1128/AEM.07823-11
Brandt, C. M., Spellerberg, B. (2009). Human infections due to Streptococcus dysgalactiae subspecies equisimilis. Clin. Infect. Dis. 49 (5), 766–772. doi: 10.1086/605085
Cervinkova, D., Vlkova, H., Borodacova, I., Makovcova, J., Babak, V., Lorencova, A, et al (2013). Prevalence of mastitis pathogens in milk from clinically healthy cows. Vet. Med 58 (11), 567–575. doi: 10.17221/7138-VETMED
Chao, Y., Bergenfelz, C., Håkansson, A. P. (2017). In vitro and in vivo biofilm formation by pathogenic streptococci. Methods Mol. Biol. (Clifton N.J.) 1535, 285–299. doi: 10.1007/978-1-4939-6673-8_19
Chauhan, A., Ghigo, J. M., Beloin, C. (2016). Study of in vivo catheter biofilm infections using pediatric central venous catheter implanted in rat. Nat. Protoc. 11, 525–541. doi: 10.1038/nprot.2016.033
Chennapragada, S. S., Ramphul, K., Barnett, B. J., Mejias, S. G., Lohana, P. (2018). A rare case of Streptococcus dysgalactiae subsp. dysgalactiae human zoonotic infection. Cureus 10 (7), e2901. doi: 10.7759/cureus.2901
Collin, M., Olsén, A. (2003). Extracellular enzymes with immunomodulating activities: variations on a theme in Streptococcus pyogenes. Infect. Immun. 71 (6), 2983–2992. doi: 10.1128/IAI.71.6.2983-2992.2003
Cue, D., Southern, S. O., Southern, P. J., Prabhakar, J., Lorelli, W., Smallheer, J. M., et al. (2000). A nonpeptide integrin antagonist can inhibit epithelial cell ingestion of Streptococcus pyogenes by blocking formation of integrin alpha 5beta 1-fibronectin-M1 protein complexes. PNAS U.S.A. 97 (6), 2858–2863. doi: 10.1073/pnas.050587897
Cusumano, Z. T., Watson, M. E., Caparon, M. G. (2014). Streptococcus pyogenes arginine and citrulline catabolism promotes infection and modulates innate immunity. Infect. Immun. 82, 233–242. doi: 10.1128/IAI.00916-13
Datta, V., Myskowski, S. M., Kwinn, L. A., Chiem, D. N., Varki, N., Kansal, R. G., et al. (2005). Mutational analysis of the group a streptococcal operon encoding streptolysin s and its virulence role in invasive infection. Mol. Microbiol. 56 (3), 681–695. doi: 10.1111/j.1365-2958.2005.04583.x
Diaz-Torres, M. L., Russell, R. R. B. (2001). HtrA protease and processing of extracellular proteins of Streptococcus mutans. FEMS Microbiol. Lett. 204, 23–28. doi: 10.1016/S0378-1097(01)00374-3
Fernandes, A. R., Jesus, J., Martins, P., Figueiredo, S., Rosa, D., Martins, L. M., et al. (2017). Multifunctional gold-nanoparticles: A nanovectorization tool for the targeted delivery of novel chemotherapeutic agents. J. Control. Release 245, 52–61. doi: 10.1016/j.jconrel.2016.11.021
Ferreira, F. A., Souza, R. R., Bonelli, R. R., Américo, M. A., Fracalanzza, S. E. L., Figueiredo, A. M. S. (2012). Comparison of in vitro and in vivo systems to study ica-independent Staphylococcus aureus biofilms. J. Microbiol. Methods 88, 393–398. doi: 10.1016/j.mimet.2012.01.007
Flaherty, R. A., Puricelli, J. M., Higashi, D. L., Park, C. J., Lee, S. W. (2015). Streptolysin s promotes programmed cell death and enhances inflammatory signaling in epithelial keratinocytes during group a Streptococcus infection. Infect. Immun. 83 (10), 4118–4133. doi: 10.1128/IAI.00611-15
Genteluci, G. L., Silva, L. G., Souza, M. C., Glatthardt, T., de Mattos, M. C., Ejzemberg, R., et al (2015). Assessment and characterization of biofilm formation among human isolates of Streptococcus dysgalactiae subsp. equisimilis. IJMM 305 (8), 937–947. doi: 10.1016/j.ijmm.2015.10.004
García-Pérez, B. E., Mondragón-Flores, R., Luna-Herrera, J. (2003). Internalization of Mycobacterium tuberculosis by macropinocytosis in non-phagocytic cells. Microb. Pathog. 35, 49–55. doi: 10.1016/S0882-4010(03)00089-5
Genteluci, G. L., Silva, L. G., Souza, M. C., Glatthardt, T., de Mattos, M. C., Ejzemberg, R., et al. (2015). Assessment and characterization of biofilm formation among human isolates of Streptococcus dysgalactiae subsp. equisimilis. IJMM 305 (8), 937–947. doi: 10.1016/j.ijmm.2015.10.004
Jamal, M., Ahmad, W., Andleeb, S., Jalil, F., Imran, M., Nawaz, M. A., et al. (2018). Bacterial biofilm and associated infections. JCMA 81 (1), 7–11. doi: 10.1016/j.jcma.2017.07.012
Jordal, S., Glambek, M., Oppegaard, O., Kittang, B. R. (2015). New tricks from an old cow: Infective endocarditis caused by Streptococcus dysgalactiae subsp. dysgalactiae. J. Clin. Microbiol. 53, 731–734. doi: 10.1128/JCM.02437-14
Kim, D. Y., Kim, K. K. (2005). Structure and function of HtrA family proteins, the key players in protein quality control. journal of biochemistry and molecular biology. J. Biochem. Mol. Biol. 38 (3), 266–274. doi: 10.5483/bmbrep.2005.38.3.266
Kissoyan, K. A., Bazzi, W., Hadi, U., Matar, G. M. (2016). The inhibition of Pseudomonas aeruginosa biofilm formation by micafungin and the enhancement of antimicrobial agent effectiveness in BALB/c mice. Biofouling 32 (7), 779–786. doi: 10.1080/08927014.2016.1199021
Koh, T. H., Sng, L. H., Yuen, S. M., Thomas, C. K., Tan, P. L., Tan, S. H., et al (2009). Streptococcal cellulitis following preparation of fresh raw seafood. Zoonoses Public Health 56 (4), 206–208. doi: 10.1111/j.1863-2378.2008.01213.x
Kumar, A., Alam, A., Rani, M., Ehtesham, N. Z., Hasnain, S. E. (2017). Biofilms: Survival and defense strategy for pathogens. Int. J. Med. Microbiol. 307, 481–489. doi: 10.1016/j.ijmm.2017.09.016
Lebeaux, D., Chauhan, A., Rendueles, O., Beloin, C. (2013). From in vitro to in vivo models of bacterial biofilm-related infections. Pathogens 2 (2), 288–356. doi: 10.3390/pathogens2020288
Lyon, W. R., Caparon, M. G. (2004). Role for serine protease HtrA (DegP) of Streptococcus pyogenes in the biogenesis of virulence factors SpeB and the hemolysin streptolysin s. Infect. Immun. 72 (3), 1618–1625. doi: 10.1128/IAI.72.3.1618-1625.2004
Marks, L. R., Mashburn-Warren, L., Federle, M. J., Hakansson, A. P. (2014). Streptococcus pyogenes biofilm growth in vitro and in vivo and its role in colonization, virulence, and genetic exchange. J. Infect. Dis. 210 (1), 25–34. doi: 10.1093/infdis/jiu058
Matsue, M., Ogura, K., Sugiyama, H., Miyoshi-Akiyama, T., Takemori-Sakai, Y., Iwata, Y., et al. (2020). Pathogenicity characterization of prevalent-type Streptococcus dysgalactiae subsp. equisimilis strains. Front. Microbiol. 11. doi: 10.3389/fmicb.2020.00097
Molloy, E. M., Cotter, P. D., Hill, C., Mitchell, D. A., Ross, R. P. (2011). Streptolysin s-like virulence factors: The continuing sagA. Nat. Rev. Microbiol. 9, 670–681. doi: 10.1038/nrmicro2624
Nathan, B., Pillai, V., Ayyan, S. M., Ss, A., Prakash Raju, K. (2021). Streptococcus dysgalactiae subspecies dysgalactiae infection presenting with septic shock. Cureus 13 (1), e12465. doi: 10.7759/cureus.12465
O'Neill, E., Humphreys, H., O'Gara, J. P. (2009). Carriage of both the fnbA and fnbB genes and growth at 37 degrees c promote FnBP-mediated biofilm development in meticillin-resistant Staphylococcus aureus clinical isolates. J. Med. Microbiol. 58 (Pt 4), 399–402. doi: 10.1099/jmm.0.005504-0
Park, M. J., Eun, I. S., Jung, C. Y., Ko, Y. C., Kim, Y. J., Kim, C. K., et al. (2012). Streptococcus dysgalactiae subspecies dysgalactiae infection after total knee arthroplasty: a case report. Knee Surg. Relat. Res. 24, 120–123. doi: 10.5792/ksrr.2012.24.2.120
Porcellato, D., Smistad, M., Skeie, S. B., Jørgensen, H. J., Austbø, L., Oppegaard, O. (2021). Whole genome sequencing reveals possible host species adaptation of Streptococcus dysgalactiae. Sci. Rep 11, 1–13. doi: 10.1038/s41598-021-96710-z
Prabhakara, R., Harro, J. M., Leid, J. G., Keegan, A. D., Prior, M. L., Shirtliff, M. E. (2011). Suppression of the inflammatory immune response prevents the development of chronic biofilm infection due to methicillin-resistant Staphylococcus aureus. Infect. Immun. 79 (12), 5010–5018. doi: 10.1128/IAI.05571-11
Proetzel, G., Wiles, M. V. (2010). Mouse models for drug discovery. Methods Protoc. 602, 395–403. doi: 10.1007/978-1-60761-058-8
Rato, M. G., Bexiga, R., Florindo, C., Cavaco, L. M., Vilela, C. L., Santos-Sanches, I. (2013). Antimicrobial resistance and molecular epidemiology of streptococci from bovine mastitis. Vet. Microbiol. 161, 286–294. doi: 10.1016/j.vetmic.2012.07.043
Roma-Rodrigues, C., Alves-Barroco, C., Raposo, L. R., Costa, M. N., Fortunato, E., Baptista, P. V., et al. (2015). Infection of human keratinocytes by Streptococcus dysgalactiae subspecies dysgalactiae isolated from milk of the bovine udder. Microbes Infect 18 (4), 290–293. doi: 10.1016/j.micinf.2015.11.005
Ronin, D., Boyer, J., Alban, N., Natoli, R. M., Johnson, A., Kjellerup, B. V. (2022). Current and novel diagnostics for orthopedic implant biofilm infections: a review. APMIS 130 (2), 59–81. doi: 10.1111/apm.13197
See, J. X., Samudi, C., Saeidi, A., Menon, N., Choh, L. C., Vadivelu, J., et al. (2016). Experimental persistent infection of BALB/c mice with small-colony variants of Burkholderia pseudomallei leads to concurrent upregulation of PD-1 on T cells and skewed Th1 and Th17 responses. PloS Negl. Trop. Dis. 10 (3), e0004503. doi: 10.1371/journal.pntd.0004503
Siemens, N., Kittang, B. R., Chakrakodi, B., Oppegaard, O., Johansson, L., Bruun, T., et al. (2015). Increased cytotoxicity and streptolysin O activity in group G streptococcal strains causing invasive tissue infections. Sci. Rep. 5, 1–12. doi: 10.1038/srep16945
Šmitran, A., Vuković, D., Opavski, N., Gajić, I., Marinković, J., Božić, L., et al. (2018). Influence of subinhibitory antibiotic concentration on Streptococcus pyogenes adherence and biofilm production. Acta Microbiol. Immunol. Hung 65 (2), 229–240. doi: 10.1556/030.65.2018.026
Speziale, P., Pietrocola, G., Foster, T. J., Geoghegan, J. A. (2014). Protein-based biofilm matrices in staphylococci. Front. Cell Infect. Microbiol. 4. doi: 10.3389/fcimb.2014.00171
Trøstrup, H., Thomsen, K., Christophersen, L. J., Hougen, H. P., Bjarnsholt, T., Jensen, P.Ø., et al. (2013). Pseudomonas aeruginosa biofilm aggravates skin inflammatory response in BALB/c mice in a novel chronic wound model. Tissue Repair Soc. 21 (2), 292–299. doi: 10.1111/wrr.12016
Tsao, N., Kuo, C. F., Cheng, M. H., Lin, W. C., Lin, C. F., Lin, Y. S. (2019). Streptolysin s induces mitochondrial damage and macrophage death through inhibiting degradation of glycogen synthase kinase-3β in Streptococcus pyogenes infection. Sci. Rep. 9 (1), 5371. doi: 10.1038/s41598-019-41853-3
Vajjala, A., Biswas, D., Tay, W. H., Hanski, E., Kline, K. A. (2019). Streptolysin-induced endoplasmic reticulum stress promotes group a streptococcal host-associated biofilm formation and necrotising fasciitis. Cell. Microbiol. 21 (1), e12956. doi: 10.1111/cmi.12956
Xu, K. Z., Tan, X. J., Chang, Z. Y., Li, J. J. (2022). 2-tert-Butyl-1,4-benzoquinone, a food additive oxidant, reduces virulence factors of Chromobacterium violaceum. LWT-Food Sci. Technol. 163, 113569. doi: 10.1016/j.lwt.2022.113569
Keywords: host-pathogen interaction, bovine mastitis, bacterial cytotoxicity, biofilm development, SDSD pathogenesis
Citation: Alves-Barroco C, Botelho AMN, Américo MA, Fracalanzza SEL, de Matos APA, Guimaraes MA, Ferreira-Carvalho BT, Figueiredo AMS and Fernandes AR (2022) Assessing in vivo and in vitro biofilm development by Streptococcus dysgalactiae subsp. dysgalactiae using a murine model of catheter-associated biofilm and human keratinocyte cell. Front. Cell. Infect. Microbiol. 12:874694. doi: 10.3389/fcimb.2022.874694
Received: 12 February 2022; Accepted: 29 June 2022;
Published: 19 July 2022.
Edited by:
Samuel Lee, Dartmouth College, United StatesCopyright © 2022 Alves-Barroco, Botelho, Américo, Fracalanzza, de Matos, Guimaraes, Ferreira-Carvalho, Figueiredo and Fernandes. This is an open-access article distributed under the terms of the Creative Commons Attribution License (CC BY). The use, distribution or reproduction in other forums is permitted, provided the original author(s) and the copyright owner(s) are credited and that the original publication in this journal is cited, in accordance with accepted academic practice. No use, distribution or reproduction is permitted which does not comply with these terms.
*Correspondence: Alexandra R. Fernandes, ma.fernandes@fct.unl.pt; Agnes Marie Sá Figueiredo, agnes@micro.ufrj.br
†These authors share last authorship