- 1Division of Bacteriology, ICMR-National Institute of Cholera and Enteric Diseases, Kolkata, India
- 2Collaborative Research Centre of Okayama University for Infectious Diseases at ICMR- National Institute of Cholera and Enteric Diseases, Kolkata, India
- 3Graduate School of Medicine, Dentistry and Pharmaceutical Sciences, Okayama University, Okayama, Japan
Antimicrobial resistance (AMR) in bacteria is an important global health problem affecting humans, animals, and the environment. AMR is considered as one of the major components in the “global one health”. Misuse/overuse of antibiotics in any one of the segments can impact the integrity of the others. In the presence of antibiotic selective pressure, bacteria tend to develop several defense mechanisms, which include structural changes of the bacterial outer membrane, enzymatic processes, gene upregulation, mutations, adaptive resistance, and biofilm formation. Several components of mobile genetic elements (MGEs) play an important role in the dissemination of AMR. Each one of these components has a specific function that lasts long, irrespective of any antibiotic pressure. Integrative and conjugative elements (ICEs), insertion sequence elements (ISs), and transposons carry the antimicrobial resistance genes (ARGs) on different genetic backbones. Successful transfer of ARGs depends on the class of plasmids, regulons, ISs proximity, and type of recombination systems. Additionally, phage-bacterial networks play a major role in the transmission of ARGs, especially in bacteria from the environment and foods of animal origin. Several other functional attributes of bacteria also get successfully modified to acquire ARGs. These include efflux pumps, toxin-antitoxin systems, regulatory small RNAs, guanosine pentaphosphate signaling, quorum sensing, two-component system, and clustered regularly interspaced short palindromic repeats (CRISPR) systems. The metabolic and virulence state of bacteria is also associated with a range of genetic and phenotypic resistance mechanisms. In spite of the availability of a considerable information on AMR, the network associations between selection pressures and several of the components mentioned above are poorly understood. Understanding how a pathogen resists and regulates the ARGs in response to antimicrobials can help in controlling the development of resistance. Here, we provide an overview of the importance of genetic network and regulation of AMR in bacterial pathogens.
Introduction
Antimicrobial resistance (AMR) is a major public health concern, which has been continued to increase primarily due to inappropriate use of antibiotics in human health and in the production of food animals. An estimated 4·95 million deaths were associated with AMR in 2019, which included 1·27 million deaths directly attributable to pathogenic bacteria (ARC, 2022). Predictions of the rising magnitude of AMR-associated death might be around 10 million people by 2050, if no interventions are applied (Balouiri et al., 2016).
Since their discovery, antimicrobials have been successfully used in the treatment of different infectious diseases. However, the efficacy of many antimicrobials is progressively being compromised with the increase of resistance in disease-associated bacteria. The rapid emergence and spread of AMR continue to be a challenging problem, especially in clinical settings, animal farming, and food manufacturing (Queenan et al., 2016; Matthiessen et al., 2022). The emergence of multidrug-resistant (MDR), extensively drug-resistant and pan-drug-resistant bacterial strains severely limits existing therapeutic options (Magiorakos et al., 2012; Poulakou et al., 2014). Besides the development of new drugs, strategies to prevent the development and spread of resistance are being extensively explored (Hashempour-Baltork et al., 2019). These approaches require a clear understanding the mechanisms of AMR and the role of environmental factors that contribute to the development of resistance.
The founder effect, fitness costs within the host, and their ecological association influence the success of AMR transmission. In the presence of antibiotic selective pressure, bacteria develop several levels of defense, which include structural changes of the bacterial outer membrane, enzymatic mechanisms for antibiotic-inactivation, gene upregulation, mutations, adaptive resistance, and formation of resistant phenotypes and biofilm (Abushaheen et al., 2020). Several such factors, and their interactions between them are shown in Figure 1. Resistance might occur either through a single mechanism against multiple antibiotics or multiple mechanisms against a single antibiotic.
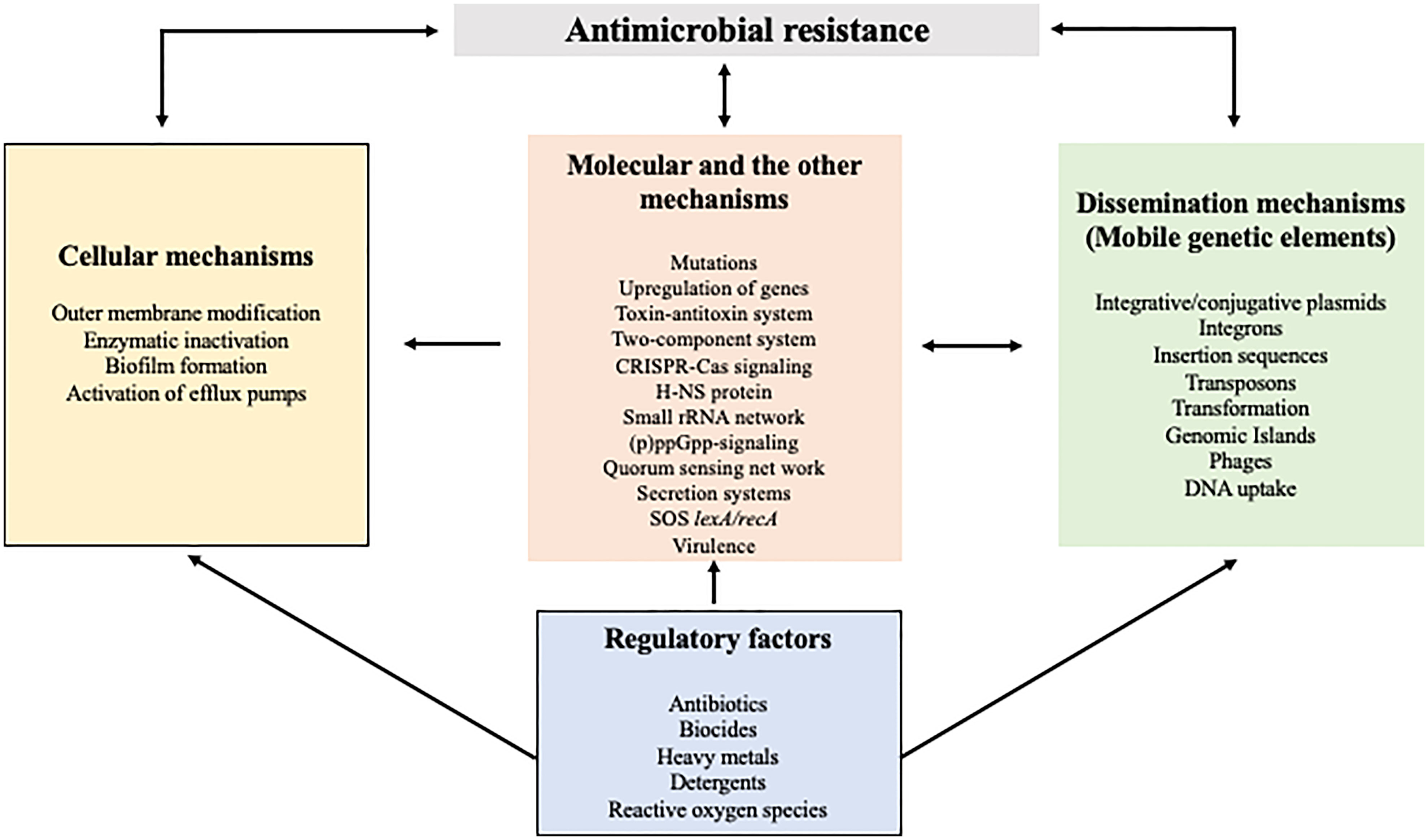
Figure 1 Common mechanisms and their interaction in the dissemination of antimicrobial resistance. As shown in different boxes, cellular and molecular mechanisms as well as mobile genetic elements are responsible for the acquisition and dissemination of AMR. The regulatory factors that prevail in the environment directly or indirectly influence many of the AMR mechanism.
Knowledge on how a pathogen becomes resistant to antimicrobials by regulating the genes is an important step towards improving the strategies to tackle the AMR problem. Antimicrobial resistance genes (ARGs) may form part of mobile genetic elements (MGEs), which can make the intracellular transfer onto plasmids or gene cassettes (Baquero et al., 2019). Gene regulatory networks permit the bacteria to generate a coordinated response to environmental challenges and shape the epidemiology of AMR. However, the relationship between selection pressure and the evolutionary change of these networks is poorly understood.
Since bacterial virulence genes can act in tandem with AMR and MGEs, there is a need to investigate these gene reservoirs and their mode of transmission. In many findings, it was reported that the co-selection of AMR takes place frequently in the presence of heavy metals, environmental toxicants, and other inorganic agents (Wales and Davies, 2015; Biswas et al., 2021). Non-antibiotic agents such as detergents and heavy metals can induce cross-protection against antimicrobials through the efflux and other systems (Hegstad et al., 2010; Sistrunk et al., 2016; Vats et al., 2022). This review focuses on several important aspects of the genetic networks and regulation of AMR in bacterial pathogens with some known examples.
Mobile genetic elements
MGEs/transposable elements are the genes that can move within a genome or be transferred from one bacterial species to another. The gene gain or loss due to MGEs could contribute to the adaptation to different environments that eventually help to form a divergent bacterial population (Jørgensen et al., 2015). The expansion of AMR in bacteria is due to intrinsic or acquired mechanisms during unstructured de novo mutations and horizontal gene transfer (HGT). Conjugation, transformation, and transduction are the three canonical mechanisms of HGT (García-Aljaro et al., 2017). MGEs can carry ARGs for different categories of antibiotics and play a significant role in their spread within and between bacterial species (Forster et al., 2022). MGEs successfully transfer ARGs en bloc on the bacterial chromosome and/or on the broad-host-range plasmids. The linkage of a well-organized gene capture and expression systems, together with the ability for vertical and horizontal transmission of ARGs signifies a powerful defense machinery used by the bacteria to resist several antimicrobials (Bencko and Šíma, 2018; Li et al., 2021). Acquisition of MGEs may have association with fitness cost and maintained due to antimicrobial selective pressure. The presence of MGE is generally alleviated by compensatory mutations in the host chromosome during MGE-host synchronization and coevolution. In the absence of antibiotic pressure, the bacteria can be outcompeted by others that do not carry any MGEs (Depardieu et al., 2007).
Gene clusters gained by HGT in bacterial genomes are referred to as genomic islands (GIs). They generally carry essential genes for genome evolution and environmental adaptation that includes bacterial fitness, metabolism, pathogenesis, AMR, etc (Juhas et al., 2009). Based on their structure and functions, GIs are also considered a superfamily of MGE. GIs have specific features that differentiate them from the core genome, which include the presence of mobility-related genes flanked by direct repeats and specific integration sites (e.g., tDNA [tRNA/tmRNA] gene) (Juhas et al., 2009). For e.g., the Salmonella Genomic Island-1 (SGI-1), an MGE found in many enterobacterial isolates, carries several MDR genes encoding resistance to ampicillin, chloramphenicol, streptomycin, sulfonamides, and tetracycline (Amar et al., 2008). However, the insertion position of SGI-1 might differ among many bacterial groups (Siebor and Neuwirth, 2022). The macrolide resistance gene ermB was found to be associated with several multidrug resistance genomic islands (MDR-GIs) in Campylobacters (Liu et al., 2019a). In many bacterial species, tetracycline encoding gene tet(O) acts as a key integration site for the horizontal transfer of ARGs. During transformation, circular intermediates are formed owing to the presence of two tet(O) direct repeats in the terminal parts of MDR-GIs (Friis et al., 2007). Integrons, insertion sequences, transposons, plasmids, bacteriophages are some of the important components that carry several MGEs whose functions including AMR are described below.
Integrons
Integrons are proficient gene capture and expression system with several gene cassettes. They play an important role in the dissemination of AMR, mainly among Gram-negative bacteria (Cambray et al., 2010). Integrative and conjugative elements (ICEs, also known as conjugative transposons) are self-transmissible MGEs, which have the combined features of prophages as well as transposons for integration and excision from the chromosomes of unrelated bacterial taxa (Akrami et al., 2019). An ICE generally contains a group of cargo genes that encodes metabolic adaptation, virulence, and resistance to antibiotics and/or heavy metals (Johnson and Grossman, 2015). Unlike plasmids, ICEs are not affected by segregational loss and are stably maintained in the host genome. Integration can occur at a single attachment site; often a tRNA gene, or in many locations that are shared by the same class of ICEs to avoid competition for the limited integration sites between different co-infecting ICEs (Cambray et al., 2010). The processing of ICE-DNA for conjugative transfer is similar to that of conjugative plasmids and rolling-circle replication (Thomas et al., 2013). This component includes a type IV secretion system, which makes an intimate contact between the donor and recipient for propagation.
Many anthropogenic factors induce bacterial gene arrangements and mutations, thereby contributing to the dissemination of genes encoding resistance for detergents, heavy metals and antimicrobials (Ghaly et al., 2017). It is well known that class 1 integrons (Intl1) are responsible for the global spread of AMR. The qacE/qacEΔ1 (encoding quaternary ammonium compound-resistance) gene cassette that confers resistance to biocides, and the mercury resistance operon (mer) has been transmitted by Tn21 provide a selective benefit in several pathogens (Cambray et al., 2010; Akrami et al., 2019). This resistance mechanism also increases bacterial membrane permeability and stimulates the production of reactive oxygen species (ROS), which possibly helps the transfer of plasmids between bacterial species (Han et al., 2019).
An integron-borne garosamine-specific aminoglycoside resistance encoding gar gene has been identified in Pseudomonas aeruginosa, Luteimonas sp., and Salmonella enterica (Böhm et al., 2020). Integron’s specificity to garosamine-containing aminoglycosides may decrease the efficacy of the semi-synthetic aminoglycoside plazomicin and evade the aminoglycoside resistance mechanisms. The gene gar is located within integron and adjacent to aph(3′)-XV, blaOXA-2, and blaVIM-1 gene cassettes provide resistance to many critically important antibiotics (Böhm et al., 2020). There are many excellent reviews on ICEs covering the structure and functions with reference to the transmission of AMR genes (Johnson and Grossman, 2015; Burrus, 2017; Delavat et al., 2017; Botelho and Schulenburg, 2021).
Insertion sequence elements
An insertion sequence encodes a transposase enzyme that catalyzes the transposition. Generally, the level of transposase expression influences the frequency of transposition. Insertion sequence elements (ISs) characteristically have concise sequences containing terminal inverted repeats at the boundaries and an open reading frame (ORF) that encodes the transposase, which is important for its mobility (Britten, 1996). To accomplish transposition, ISs generally infect the target site to generate short direct repeats. Some ISs undergo transposition using a non-replicative or cut-and-paste mechanism, while others use a replicative copy-and-paste mechanism, where the first copy remains intact, while the second copy is used at the target site (Duval-Valentin et al., 2004).
The combination of different incompatibility (InC) groups of plasmids and MGEs help in the spread of ARGs. There is a strong interaction between conjugative plasmids and ISs. In silico analysis exhibited transfer network of about 250 groups, comprising nearly 60 ARG subtypes and 50 ISs connecting conjugative plasmids in genetically distinct pathogens (Che et al., 2021). In this analysis, IS26, ISEcp1, and IS6100 are the most predominant elements mediating the transfer of ARGs. ISAba125-blaNDM-1, ISEcp1/IS26-blaCTX-M, ISApl1-mcr-1 are the ISs specifically involved in the transfer of New Delhi metallo-β-lactamase (NDM), extended-spectrum β-lactamase (ESBL) and mobilized colistin resistance (MCR)-encoding genes with different genetic backbones. Interspecies transfer mediated by IS26 and IS6100, both belonging to the IS6 family, was widely identified across many bacteria, involving about 20 genera belonging to 7 families (Cuzon et al., 2011).
Some of the ISs, specifically carry ESBL encoding genes. For e.g., homologous recombination mediated by IS26 was found to be responsible for the spread of several variants of blaNDM along with the other MDR encoding genes (Zhao et al., 2021). ISEcp1 belonging to the IS1380 family is an effective mobilizer of blaCTX-M by a unique transposition process using neighboring sequences by transposition. In several studies, ISEcp1element was shown to be associated with blaCTX-M-15 and other blaCTX alleles in both clinical and foodborne pathogens (Ranjbar et al., 2010; Shawa et al., 2021).
The genetic environment of the mcr-1 structure indicated that this colistin resistance gene could be mobilized as an ISApl1, flanked by the composite transposon (Tn6330). However, many mcr-1 structure sequences have been identified without ISApl or with a single-ended ISApl, signifying its origin from the ancestral Tn6330 by a copy-and-paste mechanism (Snesrud et al., 2018). In most of the bacteria, ISApl1 was identified either upstream or downstream of mcr with or without other ARGs (Anyanwu et al., 2020). A novel mobile resistance gene, fexA encoding resistance for florfenicol (a class of phenicol) has been detected both on the plasmid and chromosomes of Campylobacter jejuni (Tang et al., 2020a). The presence of IS1216 around fexA appears to be important in the integration of the fexA-carrying gene segment along with tet(L)-fexA-catA-tet(O) gene array.
Transposons
Transposons are a large and complex version of ISs with repetitive DNA sequences that can be transposed from one genome locus to the other (Siguier et al., 2006). This mobility can result in mutations, alter gene expression and induce chromosomal rearrangements. Transposons are directly involved in carrying the cargo genes such as ARGs, MGEs, ISs, and toxin-antitoxin modules (Babakhani and Oloomi, 2018). Conjugative transposons integrate into the DNA using different means of excision and integration compared to the classical transposons Tn5 and Tn10. After excision, the conjugative transposons form a covalently closed circular intermediate that can either reintegrate in the genome of the same bacterial cell or transferred to other bacteria by conjugation (Salyers et al., 1995).
Transposon helps acquisition and spread of ARGs in several bacterial pathogens. ISChh1-like transposon helps in acquiring MDR genes in Campylobacter, including the optrA gene that encodes an ATP-binding cassette F protein. The presence of optrA has confirmed its role in elevated minimum inhibitory concentration (MIC) to oxazolidinones and phenicols (Tang et al., 2020b). Interestingly, ISChh1-like transposon also integrates with AMR genes such as tet(O), aphA3, and aadE-sat4-aphA3 gene cluster (Tang et al., 2020b). The function of ISApl1 transposon in the mobilization of plasmid-borne mcr-1 was first established in the mid-2000s. However, majority of the sequences reported subsequently had no ISApl1 (Wang et al., 2018a). This finding suggests that the ISApl1 transposon had been stabilized over time in the host’s genome background and is currently spreading mcr-1 through plasmid transfer (Wang et al., 2018a). The Tn6330 transposon is responsible for the spread of mcr-1 between various plasmids and chromosomes (Li et al., 2018). The association between plasmids and the transposon are further discussed under the section plasmids.
Klebsiella pneumoniae strains carry different type of transposons with several ARGs. In outbreak-associated K. pneumoniae strains, the β-lactam resistance encoding genes blaTEM-1 and blaKPC-3 had Tn4401 element located upstream of the blaKPC-3 gene (Leavitt et al., 2010). A novel Tn1696-like composite transposon (designated as Tn6404) has been identified in a carbapenem-resistant isolate that carried blaIMP-4 and blaSFO-1 genes (Zhou et al., 2017). aac(6’)-Ib, ant(3’)-Ia, blaTEM and blaOXA-9 genes were found be carried by the Tn1331 (Tolmasky, 2000).
Several Tn7-like transposons have been identified to carry both an anti-MGE defense system and ARGs, indicating its multiple impacts on bacteria (Benler et al., 2021). In many pathogens, tetracycline resistance encoding tet(M) gene that spreads through Tn916-like elements carry erm(B) with or without the macrolide efflux genetic operon [mef(E)-msr(D)] (Marosevic et al., 2017). The conjugative transposon of the Tn916/Tn1545 family carry several MDR determinants in Gram-positive pathogens. Most of these transposons uniformly harbor the tetM gene (Roberts and Mullany, 2011). An uncommon IS that has 84% homology with ISEc63 of Tn3 family together with the blaKPC gene and Tn4401 fragments was found inserted in the tra operon of outbreak-associated enterobacterial isolates (Wozniak et al., 2021). These results indicate the important role of transposons, with stable integration into the target cell genome and the expression of AMR.
Plasmids
Transfer of AMR conferring plasmids by conjugation is an another major factor involved in the dissemination of ARGs. Plasmid mobilization makes fitness costs in bacteria, which has been minimized through compensatory mutations. Association between plasmids and bacteria successfully shapes the progression of AMR. Normally, conjugative plasmids transfer AMR determinants using MGEs, including integrons, transposons, and ISs. Several factors that influence the rate of plasmid transfer, as well as its functional response have been identified. In addition, the toxin-antitoxin system (TAS) help in the maintenance of MDR plasmids that has been discussed in the section TAS.
Plasmids are classified based on their incompatibility (Inc), as they have unique replication and partition systems. Plasmid and GI-encoded factors are important for the effective AMR-island excision, mobilization, integration, and regulation in the host bacteria. In some cases, the mobility of IncC or IncA/C conjugative plasmid depends on the transcriptional activation of multiple operons of the plasmid by the master activator AcaCD regulon (Figure 2). The regulatory network of AcaCD extends to the chromosomally integrated GIs, and express xis and mobIM for excision and mobilization, respectively (Figure 2) (Rivard et al., 2020). This regulon activates the expression of genes located in the SGI-1 and the MGIVchHai6, which is a GI, integrated in the trmE on chromosome I of V. cholerae non-O1/non-O139. Transfer of MGIVchHai6 confers resistance to β-lactams, sulfamethoxazole, tetracycline, chloramphenicol, trimethoprim, and streptomycin/spectinomycin (Carraro et al., 2016).
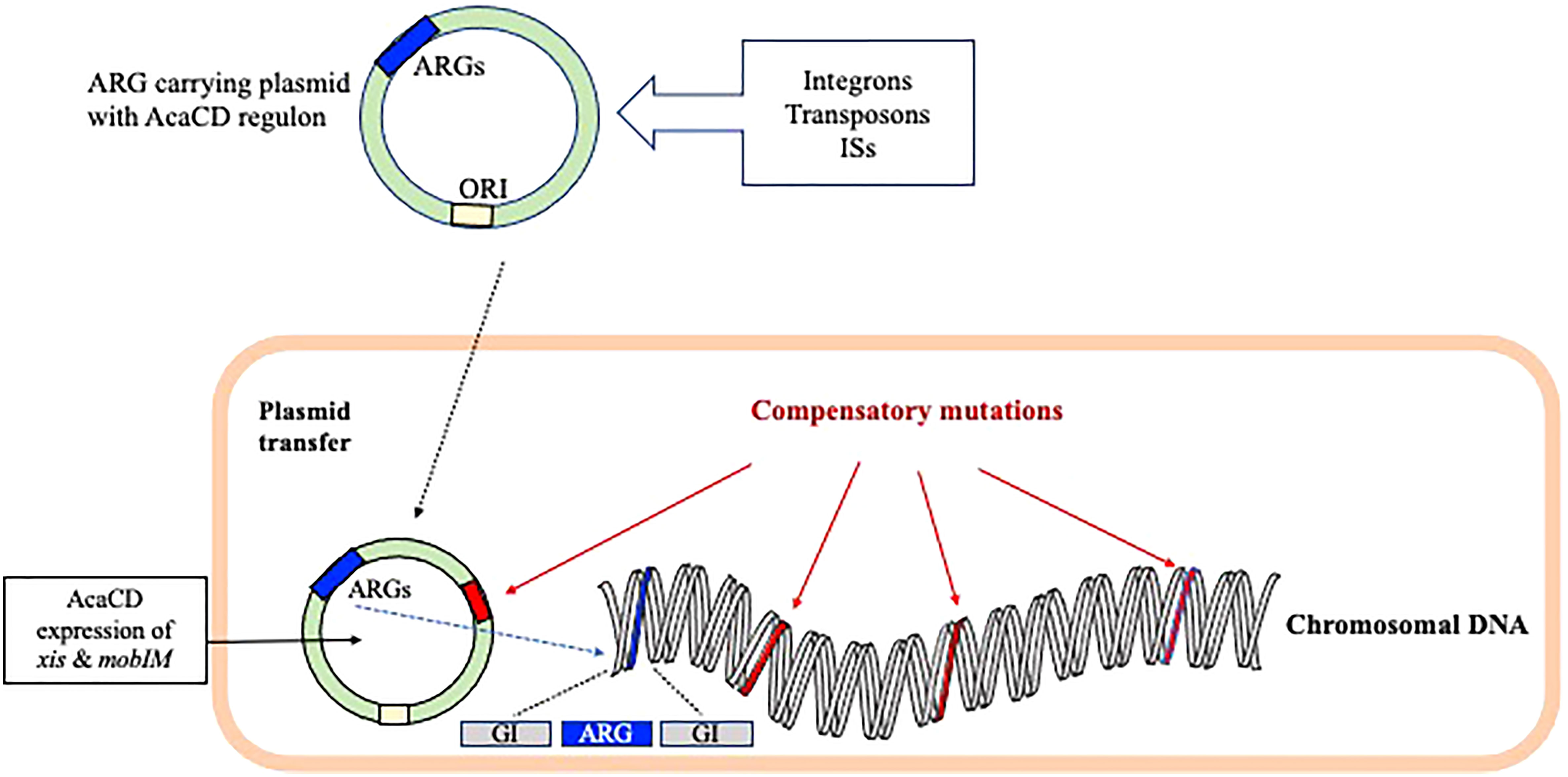
Figure 2 The role of plasmid and genomic island-encoded factors in the spread of AMR encoding genes. The mobility of selective Inc conjugative plasmid carrying the ARGs depends mostly on the master activator AcaCD regulon. The AcaCD supported expression of xis and mobIM helps in the excision and mobilization of AMR encoding genes, respectively. The fitness costs in bacteria has been minimized through plasmid/chromosomal compensatory mutations.
IncA/C plasmid in various bacterial pathogens shares a conserved backbone with several MGEs with ARGs. The IncA/C plasmid with blaCMY-2 represents a unique lineage that has been reported in a diverse group of bacteria. Mostly, the basic structure of this plasmid includes a sul2 module containing the floR-tetAR-strAB cluster and a Tn21-like element (Fernández-Alarcón et al., 2011). As shown in Figure 3, this broad host range lineage is comprised of three integration hotspots facilitating the acquisition of additional genes through integrons, IS26, ISEcp1 and ISCR2 elements (Suzuki et al., 2010). ISCR2 plays an important role in mobilizing the floR-tetA-strAB-sul2 cluster. In IncA/C plasmid lineage, the sul2 module is steadily maintained, whereas the blaCMY-2 and Tn21-like regions vary among different species. ISEcp1 existed in all the copies of IncA/C-encoded blaCMY-2 and was found to be involved in ‘one-ended transposition’ for self-mobilization along with the other ARGs (Toleman and Walsh, 2011). Transfer and replication of β-lactamase genes mediated by ISEcp1-like elements have been identified in the tra1 region on IncA/C plasmids of many bacterial species (D'Andrea et al., 2011). Plasmids with tra gene contain several ORFs that encode important proteins needed for effective conjugation.

Figure 3 An IncA/C plasmid with defined AMR gene cluster. The basic structure of the IncA/C plasmid carrying the floR-tetAR-strAB cluster is with three integration hotspots with two IS26, ISCR2 and an unknown ORF (yellow box) in between that facilitate the acquisition of additional genes.
Transmission of the blaNDM-1 gene in E. coli of animal origin is enhanced due to the presence of IncFII plasmids. IncFII plasmid with blaNDM-1 is stably maintained in the animal gut microbiome even in the absence of carbapenem selection pressure (Lin et al., 2016). The spread of frequently reported ESBL-encoding blaCTX-M-15 appears to be supported by the presence of the IncF plasmid in E. coli that contains a Tn2-blaTEM-1 transposon (Branger et al., 2018). IncX3 plasmid was shown to be important in the emergence and spread of blaNDM-5, which is associated with resistance to most of the β-lactams (Ma et al., 2020). The transconjugants showed enhanced growth and biofilm formation. This additional fitness might be one of the reasons for the global dissemination of IncX3 plasmid with blaNDM-5.
Frequently identified genes like mcr are located on the broad-host-range plasmids and their proximity to different ISs helps in the effective transmission between different bacterial species. In a bioinformatic analysis, interspecies transfer of AMR was found mediated mainly by the conjugative plasmids having ISs belonging to the IS6 family, i.e., IS26 and IS6100 (Che et al., 2021). However, such transfers are governed by multiple factors, including the genetic background of the ARGs, the fitness cost of the host, etc. Plasmid-mediated colistin resistance is prompted by phosphoethanolamine transferases encoding mcr variants that are localized on the IncX4 type plasmid. MCR-1 imposes a fitness cost to its host bacterium and hence its spread requires a high plasmid conjugation frequency. With these features, IncI2, IncX4, and IncH12 plasmids are epidemiologically successful genetic vectors that spread mcr-1 globally (Vázquez et al., 2022). In E. coli, the transcriptional regulator pixR increases plasmid transmissibility, invasion, and persistence of mcr-1-bearing plasmids (Yi et al., 2022). Transmission of some of the mcr alleles from bacterial chromosomes to MGEs seems to occur as an independent event. For e.g., a novel variant mcr-5 has been identified in colistin-resistant S. enterica Paratyphi B isolates that harbored ColE-type plasmids containing transposon of the Tn3 family (Borowiak et al., 2017). An uncommon TnAs3-like transposon was detected on a self-transmissible IncP plasmid carried unrelated AGRs, blaNDM-5 and mcr-3 in MDR clinical isolates of E. coli (Liu et al., 2017).
The hybrid plasmid of both type-1 and type-2 IncC has been detected in Vibrio alginolyticus, which displayed resistance to almost all the β-lactam antibiotics and also had a novel carbapenemase ‘Vibrio metallo-β-lactamase-1’ (Zheng et al., 2020). The chloramphenicol-florfenicol resistance gene (cfr) and its alleles that encode an rRNA methyltransferase (ermE), has been detected in many Gram-positive and Gram-negative pathogens (Tang et al., 2017). The cfr alleles generally confer resistance to phenicols, lincosamides, oxazolidinones, pleuromutilins, and streptogramin-A. The cfrC allele was detected on transferable plasmids flanked by two copies of IS26. Through homologous recombination, cfrC can loop out the intervening sequences (Deng et al., 2014). Transmission of high-level aminoglycoside resistance (16S-rRNA methylase, rmtB) and a quinolone efflux pump (qepA) in E. coli are associated with Tn3, IS26, and ISCR3 in an IncFII plasmid (Deng et al., 2011). Plasmid-mediated qepA2 and multiple chromosomally-mediated fluoroquinolone resistance determinants also increases fluoroquinolone resistance to several folds (Machuca et al., 2015). The presence of qepA2 induces survival cost in E. coli, but it was counterbalanced by the deletion of multiple antibiotic resistance (marR) gene (Machuca et al., 2015).
Integrative plasmids play an important role in the stability and spread of virulence and ARGs. S. Enteritidis-specific virulence plasmid, pSEN was found to be integrated into an IncHI2 MDR plasmid having the cephalosporin and fosfomycin resistance determinants blaCTX-M-14 and fosA3, respectively (Wong et al., 2017). The replicative transposition process has been mediated by IS26, which is usually identified in many MDR plasmids. In S. Typhimurium, IncHI2-type plasmid harboring the olaquindox/quinolone AB-encoding gene oqxAB is responsible for the ciprofloxacin resistance (Lian et al., 2019). After acquiring this plasmid, the chromosomal efflux pump genes acrAB, tolC, and yceE remain upregulated and maintained the survival of ciprofloxacin exposed S. Typhimurium.
Epigenetic compatibility of quinolone resistance (Qnr) determinants in the host genome depends on the bacterial species and other factors. Plasmids carrying the QnrA determinant are stable in E. coli, whereas the SmQnr, which was present in a Gram-negative, multidrug resistant, opportunistic pathogen Stenotrophomonas maltophilia, was found to be unstable despite both the proteins exhibiting homologous tertiary structures (Sánchez and Martínez, 2012). This mechanism signifies that the fitness costs associated with the acquisition of SmQnr may not be derived from the metabolic burden, but the acquired gene seems to be important in initiating specific changes in the host bacterial metabolic and regulatory network. The plasmid-mediated quinolone resistance determinant OqxAB-mediates resistance to many antimicrobials such as chloramphenicol, quinolones, quinoxalines, trimethoprim, etc., mostly among the members of the family Enterobacteriaceae. oqxAB flanked by IS26 elements forms a composite transposon Tn6010, which has been detected mostly in the IncHI2 plasmid (Ruiz et al., 2012; Wang et al., 2017; Li et al., 2019). This oqxAB has been clustered with several other resistance genes on the same plasmid, including aac(6’)-Ib-cr, qnrS, blaCTX-M-55, rmtB, fosA3, and floR (Wang et al., 2017).
Phages
Recent findings suggest that phage-bacterial association play a substantial role in the transmission of AMR, especially in bacteria from the environment and foods of animal origin. Metagenomic analysis of food animals, natural water bodies, effluents, and soil supplemented with animal manure supported the view on the spread of ARGs through bacteriophage DNA and prophage elements (Ross and Topp, 2015; Shousha et al., 2015; Mohan Raj and Karunasagar, 2019; Balcázar, 2020). ARGs containing extracellular DNA is protected from the action of DNase, as they are packed in the phage’s capsid proteins. These DNA fragments can be safely transferred to bacterial cells, following their integration into the specific regions of the chromosome through RecA (essential for the repair and maintenance of DNA)-dependent homologous recombination. The prophage-containing bacteria can further transfer the ARGs by HGT. Gaining prophage influences bacterial fitness through the transfer of several genes, including ARGs. Under in vitro conditions, it was demonstrated that bacteria confer fitness benefits by carrying prophage-encoded ARGs (Haaber et al., 2016; Wachino et al., 2019; Wendling et al., 2021). Findings of in silico analysis showed that the role of polyvalent-bacteriophages, that are capable of infecting more than one host, is important in the intergeneric transmission of ARGs that encode an ESBL (blaCTX-M), ABC-type efflux permease (mel), and ribosomal protection protein (tetM) loci (Gabashvili et al., 2020).
Analysis of more than thirty viromes from human and non-human sources (animals and environment) indicated that the latter group was a reservoir of ARGs (Lekunberri et al., 2017). In chicken feces and water samples, bacteriophage genomes carried several important ARGs (Yang et al., 2020; Zare et al., 2021). Moreover, several copies of ARGs, including β-lactam, aminoglycoside, and fluoroquinolone resistance have been detected in the DNA of E. coli phage YZ1 (Wang et al., 2018b). Enterotoxigenic E. coli prophage carried several ARGs, which expressed resistance to sulfamethoxazole-trimethoprim, chloramphenicol, tetracycline, aminoglycoside and narrow-spectrum β-lactamase (blaTEM-1b). This MDR transmitting prophage had Tn2 transposon with serine recombinase gene flanking the blaTEM-1b and the IntI1 together with the TnAs2 transposon (Wang et al., 2020a). In E. coli isolates of animal origin, phage-like plasmid with-mcr1 excised and formed a circular intermediate before integration into plasmids containing the ISApl1 element (Li et al., 2017a). This event may be more complex upon translocation into phage-like vectors, which can be transmitted via transduction events.
Efflux pump system
Efflux pumps are a mechanism of an advanced defense system in bacteria. Efflux pump systems (EPS) can force out antibiotics from the bacterial cell to maintain the antibiotic concentration below the lethal threshold inside the cell. Efflux pumps can confer resistance to a single or a structurally diverse class of antibiotics. The distribution of efflux pump genes and the upregulation of their expression determines the extent of resistance to many antimicrobial agents. Generally, chromosomes encode several MDR efflux pumps and their expression is controlled by point mutations in the regulatory genes (Nowak et al., 2015). Efflux pumps can be classified into several categories based on their structure, the number of transmembrane spanning regions, energy sources, and substrates. Accordingly, the major subfamilies of efflux pumps include, i) Resistance Nodulation Division (RND) family, ii) Major Facilitator Superfamily (MFS), iii) ATP (adenosine triphosphate)-binding cassette (ABC) superfamily, iv) Small Multidrug Resistance family and v) Multidrug and Toxic compound extrusion family. Several coordinated networks of efflux transporters have been identified in bacteria. Complex signaling pathways are involved in the EPS-mediated resistance mechanisms to protect bacteria (Figure 4).
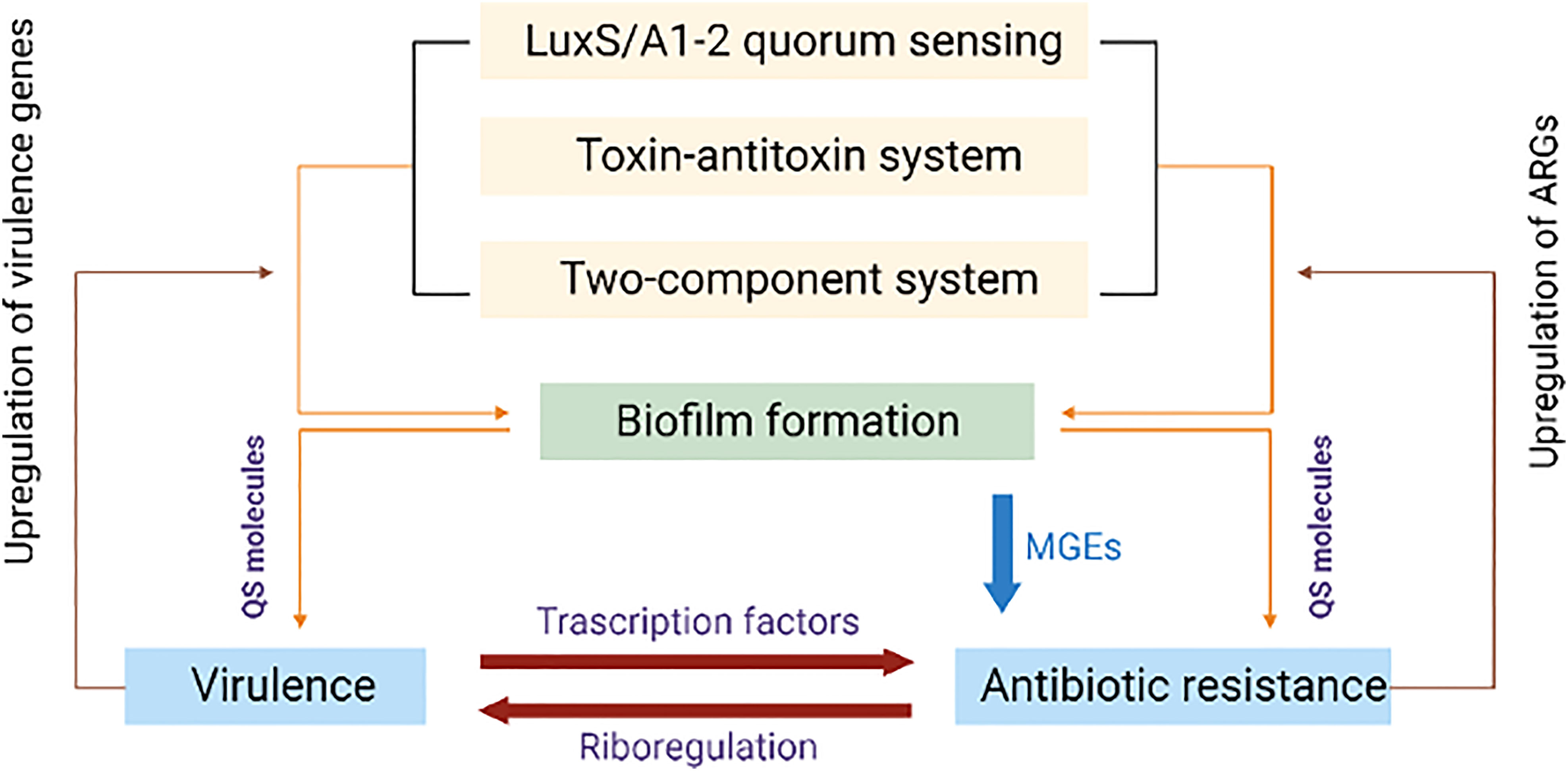
Figure 4 Diagrammatic representation of shared regulatory network mechanisms involving antibiotic resistance and virulence. Quorum sensing (QS), toxin-antitoxin (TAS) and two component systems (TCS) upregulate the biofilm formation encoding genes that successively upregulate quorum sensing molecules. These molecules influence antibiotic resistance genes and virulence. Up-regulated virulence and antibiotic resistance genes consequently upregulate QS, TAS and TCS.
RND is the major mechanism of MDR in Gram-negative bacteria. This larger efflux transporter is an inner membrane protein that networks with a periplasmic fusion protein and an outer membrane channel protein to form a tripartite complex to directly export antibiotics from the bacterial cells. Members of Enterobacteriaceae use AcrAB-TolC and OqxAB-TolC efflux pumps to resist the action of fluoroquinolones, β-lactams, tetracyclines, macrolides, oxazolidinone, quinolones, chloramphenicol (Swick et al., 2011). A three-gene operon cmeABC is the major EPS in Campylobacter and plays a key role in mediating resistance to structurally dissimilar antimicrobials. The multidrug efflux CmeDEF interacts with CmeABC and increases the antimicrobial resistance and the cell viability (Akiba et al., 2006). In C. jejuni, CmeC functions as a multidrug efflux transporter, and overexpression of the cmeGH operon significantly increases its resistance mostly to fluoroquinolones as well as exogenous hydrogen peroxide (Jeon et al., 2011). The tripartite protein components of RND are post-translationally changed to N-linked glycans in C. jejuni. The multifunctional role of N-linked glycans stabilizes the protein complexes, by improving their thermostability and increasing their propensity for protein-protein interaction, which in turn facilitates AMR through multidrug efflux pump activity (Abouelhadid et al., 2020).
Involvement of the other RND-type efflux systems such as AdeABC, AdeFGH, and AdeIJK are important for intrinsic MDR in several pathogens. Overexpression of AdeABC and AdeFGH systems in Acinetobacter baumannii induces resistance to aminoglycosides, β- lactams, chloramphenicol, fluoroquinolones, macrolides and tetracyclines (Yoon et al., 2015). AdeABC also alters membrane-associated cellular functions such as the formation of biofilm and plasmid transfer. Multi-efflux pumps simultaneously protect the pathogens against several antimicrobials. In the three multi-drug efflux pump systems (MacAB-TolC [ABC efflux], MFS drug transport system, and AcrAB-TolC) of S. Typhi, tolC, macB, acrA, acrB, and mdfA are involved in multiple resistance pathways that protect the pathogen from macrolides, chloramphenicol, tetracycline, novobiocin, quinolones, fluoroquinolones and β-lactams (Debroy et al., 2020). The genes related to tripartite efflux pumps mdtEF-tolC and the ABC family efflux pump macAB-tolC are important in Shiga toxin-producing E. coli (STEC) O157:H7 (Miryala and Ramaiah, 2019). About 20 genes are involved in multi-efflux pumps in this pathogen, which are directly or indirectly associated with the MDR.
Toxin-antitoxin system
Toxin-antitoxin system (TAS) present in the mobile genetic scaffolds consists of a stable toxin component that targets an essential cellular process and an antitoxin that counteracts the activity of the toxin. Recent findings suggest that the TAS are mostly used to lower bacterial metabolism during stress, prevent the invasion of phages, stabilize genetic elements, and support biofilm formation (Song and Wood, 2020). Depending on the molecular nature and their mode of interaction with the toxins, TAS is classified into several types. Importantly, in type I TAS, toxin and antitoxin are protein and RNA, respectively, but in the type II TAS, both toxin and antitoxin are proteins that may differ greatly among several bacterial species. TAS can either be on a plasmid or can be chromosomally encoded, which is generally associated with bacterial stress adaptation.
In vancomycin- resistant enterococci (VRE), specific TA pair, the mazEF system located on plasmids is responsible for the resistance to most major classes of antibiotics (Moritz and Hergenrother, 2006). In addition, the plasmid replicon types of pRE25, pRUM, pIP501, and pHT-β in VRE are known to be linked to glycopeptide resistance and stabilizing TA systems. Most of the VRE strains belonged to these plasmid replicon types assigned to the clonal complex 17 (Rosvoll et al., 2010). The SplTA is widely present in plasmids harboring the carbapenem resistance gene that helps in the maintenance of plasmids and provide stability to the transferred genetic elements. The SplTA and HigBA are the most prevalent plasmid-associated TAS found in carbapenem resistant A. baumannii (Jurenaite et al., 2013). Cross-resistance to chlorhexidine-colistin has also been reported in carbapenemase-producing K. pneumoniae that has the type II TAS (Bleriot et al., 2020). In V. cholerae, the mosAT encoded TAS present within the ICE-SXT (conferring resistance to sulfamethoxazole and trimethoprim) element helps to maintain sulfamethoxazole-trimethoprim resistance (Wozniak and Waldor, 2009). In Staphylococcus aureus TAS, mazEF encodes the RNase MazF and the antitoxin MazE. This gene cluster enhances tolerance to several antimicrobials (Ma et al., 2019a).
In E. coli and Salmonella, ParDE type II TAS that exists in Inc (I and IncF-type) plasmids not only helps in the plasmid stability, stress response, and biofilm formation, but also supports genes encoding resistance to aminoglycoside, quinolone, and β-lactams (Kamruzzaman and Iredell, 2019). IncX plasmids are important in the transmission of carbapenem and colistin resistance. Sequence analysis of IncX plasmids indicates the existence of RelE/ParE toxin superfamily within the IncX1 and IncX4 subgroups (Bustamante and Iredell, 2017). In MDR IncC plasmids, the TAS functions as an effective addiction module and maintains plasmid stability even in an antibiotic-free environment (Qi et al., 2021). In K. pneumoniae, an IncHI2 plasmid has been linked with HipBA and RelBE TAS, which helps the plasmid to maintain multiple ARGs, including catA2, aac(6’)-Ib, strB, strA, dfrA19, blaTEM-1, blaSHV-12, sul1, qacEΔ1, ereA, arr2, and aac3 along with several other genes encoding resistance to heavy metals (Zhai et al., 2016).
Influence of small RNAs network
Regulatory small RNAs (sRNA) are important in stabilizing the configuration of the bacterial envelope and uptake of antimicrobials by controlling porins and transporters at the cell surface. The sRNA network that controls several functions related to AMR is shown in Figure 5. The post-transcriptional network of sRNA communication is employed to identify the network centers and regulatory functions (Mediati et al., 2021). Though the sRNAs amply exists within the MGEs, their direct involvement in the regulation of ARGs has not been studied in detail. The contribution of sRNAs to intrinsic resistance has been identified in horizontally acquired ARGs. However, their AMR-related functions are complex in different bacteria. The sRNA MicF-mediated repression of outer-membrane porin OmpF in E. coli reduces membrane permeability to inhibit cephalosporin, norfloxacin, and minocycline uptake (Cohen et al., 1988). S. aureus sRNA, SprX specifically downregulates stage-V sporulation protein-G, and SpoVG, which increases resistance to glycopeptides (Eyraud et al., 2014). Over-expression of some of the sRNAs (e.g., Sr0161, Sr006) in P. aeruginosa increased resistance to meropenem and polymyxin-B (Zhang et al., 2017). A. baumannii fosfomycin efflux (AbaF) is one of the primary targets of AbsR25, which negatively regulates the major facilitator superfamily efflux pump gene abaF (Sharma et al., 2017). Interruption of this abaF indicates that it contributes to fosfomycin susceptibility, reduction in biofilm formation, and virulence. SdsR is one of the highly conserved enterobacterial sRNAs. In S. sonnei, SdsR promotes resistance to fluoroquinolone by regulating the expression of an efflux pump TolC (Gan and Tan, 2019).
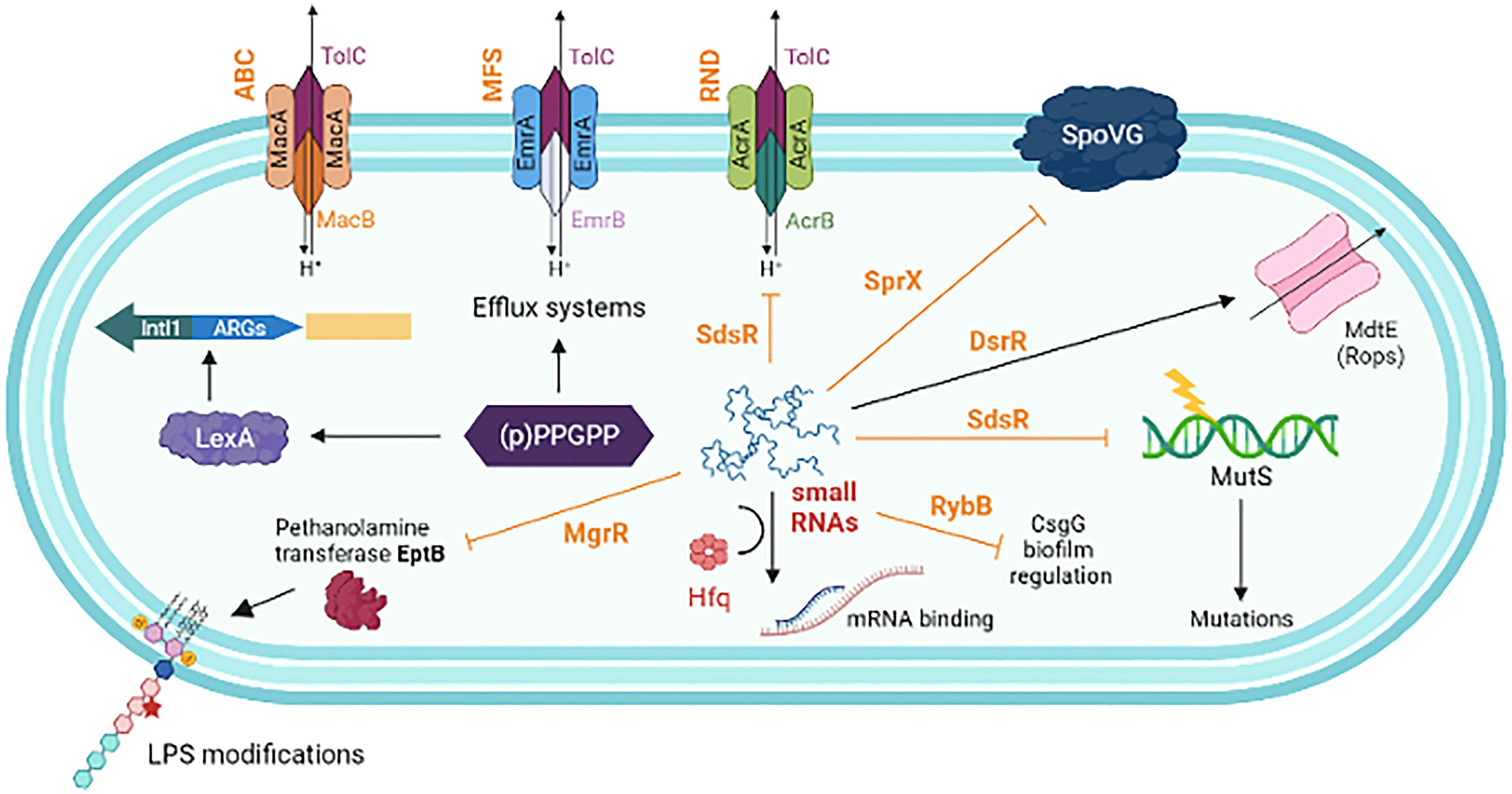
Figure 5 A schematic representation showing the interplay of different factors influence in antimicrobial resistance. (p)ppGpp increases the efficiency of HGT, AMR cassette acquisition through IntlI and upregulates the expression of various components of the efflux pumps. Non-coding small RNAs (sRNAs) play a major role in post-transcriptional regulation of gene expression. This includes, negatively regulated targets of MgrR involved in LPS modification (sensitivity to Polymyxin B); SdsR, repress the expression of tolC, the gene encoding the OMP of many multidrug resistance efflux pumps; SdsR also base-pair with mutS mRNA to repair the DNA after exposure to β-lactams; SprX (a.k.a. RsaOR) influence resistance to glycopeptides by downregulating the SpoVG; DsrA activates the expression of MdtE, which increases efflux system to antibiotic such as oxacillin, cloxacillin, erythromycin, novobiocin etc. RybB negatively influences the expression of csgD transcription, which is the master regulator of biofilm formation. sRNAs strongly interact with the co-factor Hfq, which enhances sRNA stability and facilitates base-pairing of sRNAs with multiple target mRNAs. Small RNAs are typed in dark brown. Solid arrows indicate activating interactions and T-arrows indicate inhibiting interactions.
Guanosine pentaphosphate/tetraphosphate signaling
An alarmone, guanosine pentaphosphate or tetraphosphate ([p]ppGpp) is the effector molecule, which is involved in the up-regulation of several genes involved in the bacterial response to extracellular stress, including AMR. (p)ppGpp-mediated stringent response can support microbes to survive even in the absence of specific resistance genes due to complex modulation network activities involving several targets/proteins and other small signaling molecules (Das and Bhadra, 2020). The relA gene catalyses the synthesis of (p)ppGpp during antibiotic or other stresses. In P. aeruginosa, mutations in the spoT and dksA (involved with the stringent response) induce higher levels of intracellular ppGpp concentration, which supports tolerance to quinolones (Viducic et al., 2006). In addition, (p)ppGpp helps in HGT, including the integron-mediated acquisition of AMR gene cassettes (Strugeon et al., 2016). In several pathogens, (p)ppGpp mediates resistance to vancomycin (Enterococcus faecalis), aminoglycoside (Salmonella), oxacillin (S. aureus), tetracycline and erythromycin (V. cholerae) (Abranches et al., 2009; Koskiniemi et al., 2011; Mwangi et al., 2013; Kim et al., 2018).
Quorum sensing network
The intercellular cell to cell communication mechanism through quorum-sensing (QS) controls the expression of several genes through signaling molecules called autoinducers, which plays a significant role in the adaptation and survival of bacteria. Regulation of the LuxS/AI-2 QS system has been identified in many bacterial genomes (Waters and Bassler, 2005). One of its functions is AMR that include quinolones (inhibition of DNA replication by complexing with DNA and DNA gyrase and/or topoisomerase IV), sulfonamides (prevention of tetrahydrofolate synthesis by inhibiting dihydrofolate reductase and dihydropteroate synthetase), tetracyclines (preventing aminoacyl-tRNA binding to the ribosomal-A site), β-lactams (preventing transpeptidation) and glycopeptides (preventing transpeptidation) (Wang et al., 2019). In addition, LuxS/AI-2 affects drug resistance through biofilm formation, MGEs, efflux pumps, VraSR-TCS, folate synthesis pathway, etc (Wang et al., 2019).
Co-factors and co-selection
In bacteria, many co-factors modulate the activity of AMR. Several findings now describe about the presence of chemicals, especially the heavy metal contaminations that enhance the AMR through co-selection. This section highlights the role of co-factors and co-selection in the development and dissemination of AMR.
The β-lactam antibiotics interfere with cell-wall biosynthesis by inactivating penicillin-binding proteins (PBPs), which are important for bacterial survival. The interaction between cell-wall integrity and the resistance mechanisms help in deactivation of cell-wall-targeting antibiotics through cell-wall recycling pathway. Several Gram-negative bacteria use DNA-binding proteins for ampicillin resistance such as the LysR-type transcriptional regulator (AmpR) system to mobilize resistance mechanisms in response to β-lactams by changing the composition of the cell wall-associated peptidoglycan (Dik et al., 2018). The AmpR system also releases GlcNAc-anhydro-MurNAc-oligopeptides into the periplasm and the muropeptides are transported into the cytosol through an ampG-encoded AmpG transporter (Chahboune et al., 2005). Further, the activation of the amp genes marks the expression of AmpC β-lactamase because of its cognate regulator AmpR, which regulates MDR (Dhar et al., 2018). In addition to carbapenemases, overexpression of AmpC, efflux pumps, or porin loss might contribute to the carbapenem resistance in pathogens like K. aerogenes (D'Souza et al., 2021). The network of AmpC β-lactamase overexpression has been recognized due to AmpG in conferring resistance to β-lactam antibiotics, including cephalosporins and carbapenems. Transposon insertion mutations in the AmpG β-lactamase expression pathway make the Klebsiella strains susceptible to extended- and broad-spectrum cephalosporins (D'Souza et al., 2021).
CpxAR is commonly present in Gram-negative bacteria, which sense and regulate osmotic pressure and also confer AMR. In the absence of the AcrB efflux pump, the CpxR is overexpressed, which lead to resistance to β-lactam antibiotics. Thus, modifications in the expression of outer membrane proteins (OMPs) can play an important role in acquiring resistance to aminoglycosides and β-lactams (Hu et al., 2011). Phosphoethanolamine (PEtN) transferases (eptA and eptB) confer one of the mechanisms for colistin resistance. eptA catalyzes the transfer of PEtN from phosphatidylethanolamine onto the lipid A of LPS and eptB catalyzes the addition of a PEtN moiety to the outer 3-deoxy-d-manno-octulosonic acid (Kdo) residue of a Kdo(2)-lipid A. Presence of mcr-1 supplements the activity of eptB gene in higher transcriptional expression when the E. coli exposed to a sub-inhibitory concentration of colistin (Elizabeth et al., 2022).
Several other cofactors have been identified for promoting MDR. Collateral-resistance/allogenous selection occurs when an antibiotic induces mutation(s) that express resistance to the second antibiotic. This form of selection has been described in aminoglycosides, β-lactams, macrolides, tetracyclines, and quinolones/fluoroquinolones (Baquero et al., 2021). Many other interactions between antibiotics and biocides/heavy metals, may have co-selective or counter-selective consequences on AMR (Gilbert and McBain, 2003; Ciric et al., 2011; Rensch et al., 2013). The formation of complexes between metal cations and certain antibiotics and metal-dependent efflux induces resistance to β-lactam and tetracycline (Wales and Davies, 2015). Some of the important non-antimicrobial agents that induce cross-antimicrobial resistance among pathogenic bacteria is shown in Table 1.
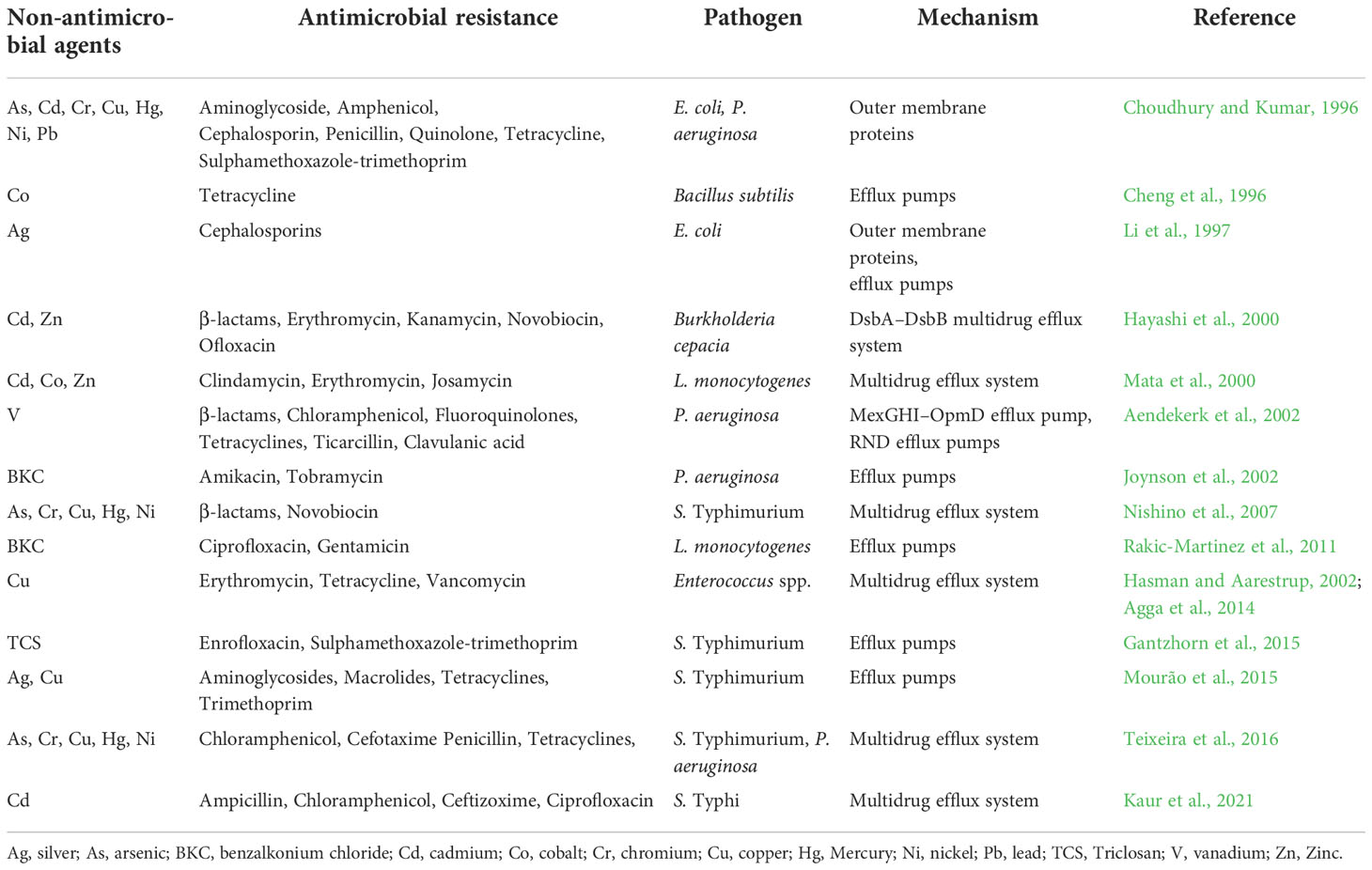
Table 1 Components of non-antimicrobial agents that induce cross-antimicrobial resistance among pathogenic bacteria.
The global repressor, histone-like nucleoid-structuring protein (H-NS) is a key transcriptional repressor of horizontally transferred genes in many bacteria. Upregulation and increased expression of genes associated with aminoglycosides, β-lactams, chloramphenicol, colistin, sulfonamides, trimethoprim and quinolones resistance are modulated by the H-NS (Rodgers et al., 2021). In A. baumannii, H-NS regulates the expression of genes involved in pathogenesis and resistance to colistin. The Δhns mutant confirms the role of H-NS as a transcriptional regulator, ameliorating the A. baumannii transcriptome associated with AMR.
The SOS response is a conserved regulatory network in bacteria that has been induced in response to DNA damage. SOS response is associated with AMR pathogens. In methicillin-resistant S. aureus (MRSA), oxacillin is one of the stimulating factors of a β-lactam-mediated SOS response through lexA/recA regulators that leads to the increased mutation and development of resistance. PBP1 also plays an important role in SOS-mediated recA activation and resistance selection in MRSA (Plata et al., 2013). Functional analysis showed that PBP1 depletion abolishes both β-lactam-induced recA expression/activation and increased mutation rates during resistance selection. Mutations enhancing the AMR involve fitness costs. However, the specific molecular mechanism of resistance in the bacterial fitness cost has not been investigated in detail. In P. aeruginosa, it was shown that the impact of mutations in the RNA polymerase gene rpoB influences the fitness cost of rifampin resistance (Qi et al., 2014). This modification occurs due to alteration in the relative transcript levels of essential genes that defend against the reduced RNA polymerase activity.
Bacteria recurrently encounter metals in their living environment, which can either be beneficial or harmful. The bacteria can use some of the metals as essential micronutrients. Co-selection of heavy metals and AMR exits as an overlapping mechanism of physiological intersection (cross-resistance) or genetic interrelation (co-regulation/co-resistance). An increase in AMR is associated in part with the co-selection of resistance to heavy metals and biocides/disinfectants used in livestock farming. These anthropogenic or non-antimicrobial agents can induce bacterial adaptations that cause decreased susceptibility or resistance to one or more antimicrobials even in the absence of any selection pressure (Table 1). Several cellular mechanisms are established to support the selection of genetic determinants associated with AMR. When E. coli strains were exposed to chlorhexidine digluconate, an increase in resistance to ampicillin, amoxicillin/clavulanic acid, cefoxitin, cefpodoxime, and cephalothin has been reported due to an increase in the RND efflux pump activity and upregulation of genes involved in cell metabolism (Wesgate et al., 2020).
Presence of plasmid-borne copper resistance genes tcrB and pcoD are linked with erythromycin, vancomycin, and tetracycline resistance with the respective encoding genes ermB, vanA, and tetB in human and animal pathogens (Hasman and Aarestrup, 2002; Agga et al., 2014). Copper efflux-associated tcrB and erythromycin resistance erm(B) genes were transferred by conjugation from the environmental Enterococcus hirae to E. faecalis (Pasquaroli et al., 2014). Intake of copper has some influence on AMR. In E. faecalis isolated from copper-fed pigs, chromosomally located tcrYAZB operon, tetM, and vanA are identified for copper, tetracycline, and vancomycin resistance (Zhang et al., 2015a). In livestock associated MRSA, genes encoding resistance to macrolides (ermT), tetracyclines (tetL) aminoglycosides (aadT), and trimethoprim (dfrK) are co-located together with copA, cadDX, and mco, which encodes resistance to copper and cadmium transporters and a copper oxidase, respectively (Gómez-Sanz et al., 2013). A similar mechanism was also reported in MDR Salmonella with an efflux mechanism for copper and silver through a chromosomally located sil operon (Mourão et al., 2015).
Reduced susceptibility to benzalkonium chloride in Listeria monocytogenes has been attributed to the presence of an EPS and linked to the reduced susceptibility to ciprofloxacin and gentamicin (Rakic-Martinez et al., 2011). Disinfectants like triclosan enhance AMR in many foodborne pathogens. Genetic changes in fabI, rpoS, and rpoD have been associated with high-level resistance to triclosan in S. Typhimurium that also increased the AMR and efflux pump activity, especially to enrofloxacin and sulphamethoxazole-trimethoprim (Gantzhorn et al., 2015). Genes encoding ESBLs and/or plasmid-mediated quinolone resistance genes and quaternary ammonium compound not only co-localize resistances, but can also co-transferred in E. coli (Li et al., 2017b).
Natural DNA transformation is an another mechanism that facilitates the transfer of genetic elements carrying AMR determinants (Winter et al., 2021). This mechanism involves the uptake of free DNA from the environment by the bacterial cells. Homologous recombination enables the internalization and integration of exogenous DNA into the host chromosome. The uptake of DNA is mediated by several proteins that help in DNA-binding, fragmentation, and transportation. For example, in C. jejuni Cj1211 strain, a Helicobacter pylori ComH3 homolog was found to be responsible for the acquisition of resistance to kanamycin or tetracycline (Jeon et al., 2008). The DNA uptake mechanism explains the acquisition of large A. baumannii resistance islands (AbaR4 and AbaR1) for carbapenem resistance in A. baumannii (Godeux et al., 2022). Intra-species genetic transformation from Campylobacter coli to C. jejuni was identified through the transfer of ermB gene that encodes resistance to macrolides in the chromosomal MDR GI (Qin et al., 2014).
Some of the susceptible pathogens may acquire indirect resistance assisted by the other juxtaposed antibiotic-resistant bacterial population. In in vitro conditions, it was shown that the presence of AMR bacteria expressing antibiotic-modifying/degrading enzymes such as β-lactamase, erythromycin esterase, tetracycline destructases, chloramphenicol acetyltransferase or the other cytoplasmic antibiotic-degrading enzymes that catalyze energy-consuming reactions, benefitted the susceptible population (Nicoloff and Andersson, 2016).
Coordinated regulation of AMR with other functional genes
The dynamics of AMR evolution depend on the interactions between various cellular components. Multifunctional proteins are essential for bacterial fitness, AMR, and virulence. The catalytic domain EptC functions as a phosphoethanolamine transferase in C. jejuni. Mutational analysis of EptC indicated its importance not only for flagellar assembly and motility, but also for conferring polymyxin-B resistance (Fage et al., 2014). However, the function of EptC cannot be generalized, as it may differ among bacterial species.
Progression of AMR includes alterations in many cellular functions, such as membrane transport, transcription, translation, metabolic activity, etc. These important changes are achieved through a well-coordinated manner to accomplish greater fitness in bacteria. For e.g., β-lactam and aminoglycoside susceptibility in E. coli is enhanced by the changes in the proton- motive force through the cell membrane and respiratory activity (Lázár et al., 2013; Suzuki et al., 2014). Metabolic homeostasis plays an important role in AMR. Alteration of metabolic activity causes S. aureus to develop resistance to daptomycin and also presents innate immunity. This change was typified by a modification in the anionic membrane phospholipid structure, prompted by point mutations in the cls2 that encodes a cardiolipin synthase (Jiang et al., 2019). The mutation in the cls2 helps S. aureus to escape neutrophil chemotaxis, which is facilitated by the decrease in bacterial membrane phosphatidylglycerol.
Triosephosphate isomerase (TpiA) acts an important role in the carbon metabolic network, pathogenesis, and aminoglycoside resistance in P. aeruginosa (Xia et al., 2020). TpiA converts glyceraldehyde 3-phosphate to dihydroxyacetone phosphate, which is an important step in the bacterial glucose metabolism. Mutation in tpiA increases the carbon metabolism, but decreases the expression of the type III secretion system (T3SS), and reduces the bacterial resistance to aminoglycosides. A mutation in another carbon metabolism encoding gene in P. aeruginosa, crcZ with a tpiA mutant background restores the expression of T3SS and conferred aminoglycoside resistance (Xia et al., 2020). In E.coli, PAP2 protein (phosphatidic acid phosphatase) is involved in the phosphatidic acid pathway, which is important in the function of MCR-1 (Choi et al., 2020).
In E. faecalis, genes encoding the phospho-N-acetylmuramoyl-pentapeptide-transferase (mraY), penicillin-binding protein (pbpC), biosynthesis of peptidoglycan layers UDP-N- acetylmuramoylalanyl-D-glutamate 2,6-diaminopimelate ligase (murEGD) shared more direct interactions in the network analysis (Naha et al., 2020). The function of these genes is interrelated to the resistance mechanisms for β-lactams, ABC transporter MDR efflux pumps, active modifications to cell wall organization, and peptidoglycan pathways. Sulfhydryl reagent variable (SHV) β-lactamases contain a large number of allelic variants that include both ESBL and non-ESBL. SHV-11 and SHV-12 are commonly found in many members of the Enterobacteriaceae, especially in K. pneumoniae expressing extensive drug-resistance. Gene network analysis using blaSHV-11 showed that the MDR is associated with gyrA, parC, glsA, osmE, yjhA, yhdT, rimL, pepB, and KPN_00437, and KPN_01875 ORFs that are responsible for several DNA metabolic processes, DNA repair, and stress response (Miryala et al., 2020). Of these, gyrA, parC, gyrB, parE, recA, dnaA, polB, dnaK, mutS, and dnaN formed center nodes in the network that comprise more than 40% of the total interactions. Among the AMR associated genes such as gyrA, nfxB, rnfC, parC, and parE, only the gyrA mutation was predominantly important (Miryala et al., 2020). Additional mutations in any one or more of these genes further increased the ciprofloxacin MIC in a gyrA mutant background (Rehman et al., 2021a). Thus, an epistatic network of gene interactions can play a significant role in fluoroquinolone resistance.
AmpR regulates the expression of several genes that are involved in different pathways, including β-lactam and non-β-lactam resistance, virulence, quorum sensing, protein phosphorylation, and other physiological processes. Loss of ampR function in P. aeruginosa enhances its virulence, thereby confirming the existence of a co-regulatory network of β- lactam resistance, overexpression of the exopolysaccharide (alginate production), and quorum sensing (Balasubramanian et al., 2011).
Antimicrobial stress can induce genetic modifications in functional proteins related to bacterial metabolism. Gentamicin-induced resistance in V. alginolyticus has caused mutations in the metabolism-associated genes: ratA, envZ, cydA1, malF (encoding cyclase, osmolarity sensor protein, cytochrome d-terminal oxidase subunit-1, maltose transporter, respectively) and the aceF, encoding a subunit of pyruvate dehydrogenase, which is a two protein complex (Zhang et al., 2019). In addition to the reduced gentamicin uptake, these mutations decreased the pyruvate cycle, redox state, and membrane potential through the Na+ translocating the NADH-quinone oxidoreductase system (Zhang et al., 2019).
In E. coli, arcA (ArcA/B two-component system and a global regulator of gene expression under microaerobic/anaerobic conditions) and gutM (DNA-binding transcription factor) deletions showed inhibition of resistance to cefixime, ciprofloxacin, and chloramphenicol. Deletion in arcA activates aerobic respiration and the production of ROS that support antibiotic-mediated cell killing (Horinouchi et al., 2020). gutM regulates genes related to glucitol utilization are involved in the biofilm formation.
The base excision repair system plays an important role in preventing mutations that influence oxidative DNA damage. A base excision repair homolog encoded by the gene cj0595c in C. jejuni has shown an endonuclease III activity for maintaining genomic stability (Dai et al., 2019). Inactivation of cj0595c resulted in higher rates of spontaneous fluoroquinolone-resistant and oxidative stress-resistant mutants in the gyrA and perR genes, respectively (Dai et al., 2019). The four-gene operon msaABCR regulates vancomycin resistance, biofilm formation, and virulence in S. aureus. MsaB, a protein product of this operon, transcriptionally regulates the expression of the crtOPQMN operon and the ohr gene (encodes organic hydroperoxide resistance), which prevents oxidative stresses (Pandey et al., 2019). Deletion of msaABCR decreases vancomycin MIC in S. aureus and downregulates several genes involved in resistance against oxidative stress generated by organic hydroperoxides (Samanta and Elasri, 2014). Suprainhibitory concentration of ciprofloxacin in Campylobacter induced significant changes in the gene expression of mfd, which encodes a transcription-repair coupling factor involved in strand-specific DNA repair (Han et al., 2008). A mutation in the mfd caused a several-fold reduction in the rate of spontaneous mutation to ciprofloxacin resistance, while overexpression of this gene increased the mutation frequency. V. cholerae exposed to subinhibitory concentrations of ciprofloxacin, tetracycline, and azithromycin induces the SOS response. This response increases the expression of recA and lexA genes as well as increased the frequency of transfer of SXT elements to other bacterial population (Mohanraj and Mandal, 2022).
The two-component system
The two-component system (TCS) is a signal transduction regulatory circuit in bacteria, which acts as an autoregulatory mechanism with a membrane-bound histidine kinase for sensing environmental signals and a cytoplasmic response regulator. The activated response regulator functions as a transcription factor that facilitates the cellular response/cell physiology through differential expression of target genes. This regulatory action activates antimicrobial defense and changes the cell physiology to increase the resistance. TCS modifies cell surface and permeability, enhances biofilm formation, and upregulates antimicrobial-degrading enzymes. Some important TCS includes VanSR-vancomycinR in S. aureus, and E. faecalis; PmrAB and PhoPQ-polymyxin-BR/colistinR in most of the Gram-negative pathogens; AmgRS- aminoglycosidesR, CopRS-β-lactam/carbapenemR in P. aeruginosa; BaeSR-ceftriaxoneR in E. coli and Salmonella; CesRK-β-lactamR in L. monocytogenes, CreBC- β-lactamR, BlrAB- β- lactamR in Aeromonas spp., etc. (Bhagirath et al., 2019; Tierney and Rather, 2019; Huang et al., 2020).
The TCS PhoPQ and PmrAB in K. pneumoniae and E. coli are controlled by additional proteins, MgrB, a negative regulator of PhoQ, and PmrD-a connector protein that activates PmrAB in response to PhoPQ stimulation (Chen et al., 2021). In regulating the polymyxin resistance, PmrD is dependent on the network organization as well as PmrB stimulation. The TCS regulator CpxAR has been reported to contribute to the MDR in several bacteria. In S. Typhimurium, CpxR regulate the colistin susceptibility via PmrAB and PhoPQ regulatory systems (Zhai et al., 2018). Generally, the TCSs PhoP-PhoQ and PmrA-PmrB control modification of lipid A of lipopolysaccharide (LPS) for adaptive peptide resistance under low Mg2+ conditions. In P. aeruginosa, CzcRS increases the resistance to heavy metals as well as carbapenems (Marguerettaz et al., 2014). ParRS directs increased resistance to aminoglycosides and the other polycationic antibiotics. This TCS also activates the LPS modification operon arnBCADTEF in the presence of subinhibitory concentrations of polymyxin and colistin (Fernández et al., 2010).
The cephalosporin sensitivity response regulator (CesR) and the cephalosporin sensitivity histidine protein kinase (CesK), encoded in the gene downstream of cesR influences the fitness of L. monocytogenes against cell wall-affecting β-lactam antimicrobials (Kallipolitis et al., 2003). In-frame deletions of cesR or its downstream positioned cesK, increases the sensitivity to β-lactams. The two-component transduction system of L. monocytogenes was found to be associated with tolerance to the cephalosporin family of antimicrobials and also to the lantibiotic nisin, which is a natural preservative, used in many food products (Cotter et al., 2002). TCS also enhances the growth of some of the pathogens in the presence of antimicrobials. Ferritin-like protein facilitates β-lactam tolerance and intrinsic resistance to cephalosporins in L. monocytogenes. However, the phosphate-response regulator (phoP) and an AraC/XylS family transcriptional regulator (axyR) that help the growth of L. monocytogenes in the presence of β-lactams have no role in the susceptibility or the tolerance to these antimicrobials (Krawczyk-Balska et al., 2012). CroSR is required for cephalosporin resistance in E. faecalis. To support this TCS, histidine-phosphorylatable phosphor carrier protein was identified to control the signaling system that modulates cephalosporin resistance (Snyder et al., 2014). CroSR-HPr association integrates antibiotic resistance and the bacterial nutritional status via various environmental stimuli.
Clustered regularly interspaced short palindromic repeats system
Clustered regularly interspaced short palindromic repeats (CRISPR) coupled with CRISPR- associated proteins (Cas) protect bacteria against invading DNA (transposons, bacteriophages, and plasmids) in a programmed, sequence-specific manner. In the absence of the CRISPR/Cas system, many bacterial pathogens carry several ARGs. Recent findings suggest that this system can be useful in controlling the progression of AMR in bacteria and can be repurposed to control the antimicrobial resistance by the expression of self-targeted CRISPR that redirects endogenic CRISPR-Cas3 activity. However, several factors could contribute to resistance against the antimicrobial action of CRISPR-Cas. This includes spontaneous mutations in the Cas genes or the other target sequences, spacer deletions due to the homologous recombination between the repeats, expression of the anti-CRISPR (acr) gene in the host genomes, and repressed expression/activity of cas-encoded proteins (Gholizadeh et al., 2020). In addition, cell to cell communications within a bacterial population through disruption of two major quorum-sensing genes, las and rhl has been shown to reduce CRISPR-Cas activity (Høyland-Kroghsbo et al., 2017).
The role of CRISPR-Cas system in AMR is not fully investigated (Shabbir et al., 2018). Recent reports indicate the importance of this system in AMR in many pathogens. Transcriptome analysis of Δcas9 mutant C. jejuni was found to be more sensitive to antibiotics than its wild strain (Shabbir et al., 2018). Sequence-based analysis indicates the association between CRISPR-Cas and ARGs in A. baumannii, E. faecium, P. aeruginosa, and S. aureus (Mortensen et al., 2021). Homologous CRISPR spacers found in S. Enteritidis strains might be associated with MDR (Haider et al., 2022). Moreover, CRISPR spacers control several genes that participate in the maintenance of the membrane integrity to overcome different stresses including AMR.
Virulence and AMR
Bacterial virulence factors are closely associated with various molecules produced by the pathogens, mainly to escape their host defense, survival and proliferation before colonization. Existence of a strong correlation between ARGs and the expression of many virulence factors have been reported in S. aureus (Obaidat et al., 2015), E. coli, and K. pneumoniae (Yang et al., 2015). Pan-resistant and MDR strains of many pathogens generally show enhanced virulence in vivo and in vitro (Qin et al., 2020). Apart from gene-encoded virulence, different phenotypic traits and cellular assemblies are also considered as virulence factors. Bacterial exo/endotoxins, specific enzymes, exopolysaccharides, flagella, fimbriae/pilli, capsules, LPS, glyco/lipoproteins are important in the pathogenesis. Intracellular changes in metabolic networks directed by protein regulators/receptors and non-coding regulatory RNAs are considered as virulence derived factors. It is important to address gene consortium, as many ARGs can act in tandem with virulence genes and other MGEs. From several investigations, it is evident that AMR and pathogenesis often exist concurrently. As shown in Figure 4, several regulatory network mechanisms exist involving AMR and virulence. However, their direct genomic association has not been well established due to complex network among the diverse species of bacterial pathogens.
In the in vivo colonization model, fluoroquinolone-resistant strain of C. jejuni with the C257T mutation in the gyrA outcompeted its clonally related fluoroquinolone-susceptible counterpart in the absence of any antimicrobial pressure (Luo et al., 2005). This enhanced fitness of the C. jejuni mutant strain also modulated its in vivo fitness. Co-existence of certain species of pathogens might support each other for enhanced growth, AMR, and virulence. The swine pathogens, Streptococcus suis and Actinobacillus pleuropneumoniae formed dual-species biofilm when co-cultured in vitro. Under this state, genes associated with exotoxins and adhesins were induced along with an increase in AMR (Wang et al., 2020b). These results suggest that the interspecies interactions may complement each other under specific conditions and play an important role in the AMR and disease progression. In the co-infection model, P. aeruginosa affects the S. aureus transcriptome and virulence with upregulation of several transporter encoding genes, especially, the Nor family of antibiotic pumps, leading to an increase in S. aureus AMR and an enhanced internalization within pulmonary epithelial cells (Briaud et al., 2019). During infection status, intrinsic and acquired ARGs provide a favorable in vivo fitness to several pathogens like P. aeruginosa, A. baumannii, and V. cholerae (Roux et al., 2015).
The outer membrane (OM) in Gram-negative bacteria acts as a barrier to different classes of antimicrobials. Colicin receptor CirA is an OM catecholate siderophore receptor involved in the iron uptake and colicin1A/B-mediated competitive killing in S. Enteritidis. The inactivation of the cirA gene caused impaired antibiotic resistance and also abruptly decreased the biofilm formation, adhesion, invasion of human epithelial cells, and proliferation in mouse macrophages (Zhang et al., 2020). Decreased expression of oprD that encodes the OM porin protein OprD increases carbapenem resistance and virulence in P. aeruginosa (Abdelraouf et al., 2011). In hypervirulent clinical K. pneumoniae, reduced expression of major OM porins (OmpK35/36) was found to be associated with carbapenem-resistant (Zhang et al., 2015b).
An assemblage of negative regulator MgrB, which is a transmembrane protein initiate the PhoPQ signaling system. Alteration of mgrB not only confers colistin resistance in K. pneumoniae, but also induces PhoPQ-governed lipid-A remodeling that enhances its virulence by reducing antimicrobial peptide susceptibility as well as host defense response (Kidd et al., 2017). The chaperone Sur (survival) is involved in the biogenesis of many OMPs and is an important member of the periplasmic network due to its genetic association with the β-barrel assembly machinery complex. The deletion mutant of sur in MDR P. aeruginosa increased susceptibility to ampicillin/sulbactam, ceftazidime, fosfomycin, and vancomycin and decreased serum resistance that led to a reduction of virulence in vivo due to several changes in the OMPs (Klein et al., 2019). LPS and capsular polysaccharide (CPS) plays a crucial role in the pathogenicity of bacteria. gnaA encodes UDP-N-acetylglucosamine C-6 dehydrogenase is confined in the CPS in A. baumannii. Higher expression of this gene plays an important role in virulence and resistance to carbapenems and cephalosporins (Xu et al., 2019). In C. jejuni, the truncation of LPS greatly reduced the intrinsic resistance to erythromycin due to enhanced permeability (Jeon et al., 2009).
Chemotaxis and swimming motility are associated with virulence in many pathogens that allow them to reach particular sites within the host (Josenhans and Suerbaum, 2002). Deletion of kanamycin resistance aphA1-encoding aminoglycoside-phosphotransferase limits the swimming ability of S. Typhimurium. Complementation with the respective allele of aphA restored the swarming phenotype, indicating the additional role of aphA in this pathogen (Brunelle et al., 2017). In many Gram-negative opportunistic pathogens, there is a strong interplay between β-lactamase regulated peptidoglycan and fitness/virulence. Peptidoglycan-remodeling enzymes are essential for the precise assembly of the T3SS machinery, flagella, invasiveness, and activation of the innate immune response (Juan et al., 2017).
Subinhibitory concentrations of tetracycline in S. Typhimurium increased its colonization ability and expression of epithelial cell invasion by up-regulating the hilD and hilA genes. These two genes regulate SPI-1 and also the fliC, fliD, fur, motA and motB genes that are associated with motility, regulation of iron acquisition systems, acid tolerance (Weir et al., 2008). Exposure of Enterococcus faecium to subinhibitory concentrations of ciprofloxacin upregulates the qnr gene that encodes quinolone resistance and collagen adhesin gene (acm). This mechanism favored not only the fluoroquinolone resistance, but also the adhesion ability of E. faecium (Sinel et al., 2017). In Shiga toxin-producing E. coli treated with subinhibitory concentrations of ciprofloxacin and tigecycline, a 6-8-fold increase in the expression levels of stx1 and stx2 has been reported (Rehman et al., 2021b).
Hemolysin is one of the major virulence factors in S. aureus. A significant relationship exists between the presence of α-hemolysin encoding gene (hla) and the antibiotic resistance of S. aureus isolates, especially to erythromycin and penicillin (Motamedi et al., 2018). The AMR activity was not noticed in deletion mutants of hla. The oqxAB gene that encodes the multidrug efflux pump OqxAB, is located on the chromosome and/or in plasmids flanked by IS26-like elements in several members of Enterobacteriaceae. This gene can co-spread both antimicrobial resistance genes (blaCTX-M, rmtB and aac(6’)-Ib) and virulence genes (Li et al., 2019). S. suis cause septicemia and meningitis in swine and humans. In MDR S. suis, a potential efflux pump SstFEG function from the upstream of the bacitracin-resistance genes bceAB and bceRS. The network of BceAB, BceRS, and SstFEG not only regulates the bacitracin efflux optimally, but also involved in enhanced colonization and higher virulence of S. suis (Ma et al., 2019b).
ESBL-plasmid-carrying curli fimbriae support adhesion and are associated with the internalization of epithelial cells. The plasmids of ESBL-producing E. coli from pandemic lineages have the potential to influence chromosomal gene expression; particularly the curli fimbriae-specific gene csgD contributes to their virulence and pandemic success (Schaufler et al., 2016). In clinical strains of K. pneumoniae, the existence of mrkD (type 3 fimbrial adhesion) was found to be associated with the blaDHA-1 (Soltani et al., 2020).
AmpC ß-lactamase regulator AmpR regulates several virulence determinants in P. aeruginosa, including pyocyanin-a redox-active compound and elastases that cause severe tissue damage during infection (Balasubramanian et al., 2012). Cysteine phosphorylation of staphylococcal accessory regulator A (SarA/MarR family) mediates vancomycin resistance and virulence in the mouse model (Sun et al., 2012). Hypervirulent MDR K. pneumoniae from diseased pig carried an ICEKpSL1, which had an intact Yersinia high-pathogenicity island for the expression of a high-virulence phenotype involving biosynthesis, regulation and the transport of the siderophore, yersiniabactin (Liu et al., 2019). This ICE could be excised from the host chromosome in the form of a circular intermediate before it spread to other bacteria. In K. pneumoniae, blaCMY-2 was significantly correlated with the presence of iutA (aerobactin siderophore ferric receptor) and rmpA (positive regulator of extracapsular polysaccharide synthesis that confers a mucoid phenotype) virulence genes (Soltani et al., 2020).
Biofilm formation is one of the virulence mechanisms that has a link with AMR. This association has been reported for gentamicin and ceftazidime resistance in E. coli, piperacillin/tazobactam, and colistin resistance in K. pneumoniae, and ciprofloxacin resistance in P. aeruginosa (Cepas et al., 2019). The BfmRS two-component system is involved in the regulation of biofilm production and is a global regulatory system that mediates the virulence-antibiotic resistance network. BfmRS-protects A. baumannii from serum complement killing through global transcriptional modulation of envelope biogenesis and defense pathways. This mechanism causes severe systemic disease in mice, and increased resistance to several antimicrobials, including the β-lactams (Geisinger et al., 2018). ESBL resistant K. pneumoniae strains expressed increased biofilm formation and resistance to serum killing through the iss gene (increased serum survival) as observed from mice mortality (Zhang et al., 2015b; Gharrah et al., 2017).
Conclusions and perspectives
Selection pressure conferred by the use of antibiotics in human medicine, animal farming, the food industry, and agriculture practice leads to a significant increase in AMR and a constant accumulation of ARGs in bacteria. Effective survival in the environment and colonization in many hosts are important components that help the pathogens to survive and spread the AMR. Controlling the spread of AMR is of prime concern that must be addressed to ensure that the treatment methods against infectious diseases remain effective. Numerous ARGs that confer resistance have been identified and characterized. The ARGs are highly intertwined and robustly regulated in a specific or through multiple bacterial genome/proteome networks. A deeper understanding of such complex networks can help in uncovering the resistance pathways and identification of specific aspects of intervention through resensitization of MDR pathogens. The advancements made in whole genome sequencing and the application of bioinformatics tools have identified several ARGs and thrown light on their role in bacterial environmental fitness, ecology of resistance, and pathogenesis. Some of this information available in public domains can be effectively used in future functional studies. Through the genomic/proteomic network, several components that play a role in resistance, as well as its controlling factors can be identified in the context of preventing the AMR and HGT.
Author contributions
TR, AG and AKM conceived and structured the review. TR and AG wrote the manuscript. GC, AKM, SD, and S-IM critically analyzed the presentation. All authors discussed the results, and reviewed and commented on the manuscript. All authors contributed to the article and approved the submitted version.
Funding
This work was supported in part by the Indian National Science Academy (INSA), Japan Initiative for Global Research Network on Infectious Diseases (J-GRID), the Ministry of Education, Culture, Sports, Science and Technology in Japan, the Japan Agency for Medical Research and Development (AMED; Grant No. JP22wm0125044), Indian Council of Medical Research, and the National Academy of Sciences (NASI), India. TR is INSA-Senior Scientist, AG is J. C. Bose Chair Professor of the NASI, India.
Acknowledgments
We thank R. Dharanidharan, Medical Biotechnology and Immunotherapy Unit, Faculty of Health Sciences, University of Cape Town, South Africa for the preparation of figures using BioRender.com.
Conflict of interest
The authors declare that the research was conducted in the absence of any commercial or financial relationships that could be construed as a potential conflict of interest.
Publisher’s note
All claims expressed in this article are solely those of the authors and do not necessarily represent those of their affiliated organizations, or those of the publisher, the editors and the reviewers. Any product that may be evaluated in this article, or claim that may be made by its manufacturer, is not guaranteed or endorsed by the publisher.
References
Abdelraouf, K., Kabbara, S., Ledesma, K. R., Poole, K., Tam, V. H. (2011). Effect of multidrug resistance-conferring mutations on the fitness and virulence of Pseudomonas aeruginosa. J. Antimicrobial. Chemotherapy 66, 1311–1317. doi: 10.1093/jac/dkr105
Abouelhadid, S., Raynes, J., Bui, T., Cuccui, J., Wren, B. W. (2020). Characterization of posttranslationally modified multidrug efflux pumps reveals an unexpected link between glycosylation and antimicrobial resistance. mBio. 11 (6), e02604–e02620. doi: 10.1128/mBio.02604-20
Abranches, J., Martinez, A. R., Kajfasz, J. K., Chávez, V., Garsin, D. A., Lemos, J. A. (2009). The molecular alarmone (p)ppGpp mediates stress responses, vancomycin tolerance, and virulence in Enterococcus faecalis. J. Bacteriology. 191, 2248–2256. doi: 10.1128/JB.01726-08
Abushaheen, M. A., Muzaheed, Fatani, A. J., Alosaimi, M., Mansy, W., George, M., et al. (2020). Antimicrobial resistance, mechanisms and its clinical significance. Disease-a-Month. 66 (6), 100971. doi: 10.1016/j.disamonth.2020.100971
Aendekerk, S., Ghysels, B., Cornelis, P., Baysse, C. (2002). Characterization of a new efflux pump, MexGHI-OpmD, from Pseudomonas aeruginosa that confers resistance to vanadium. Microbiol. (Reading) 148, 2371–2381. doi: 10.1099/00221287-148-8-2371
Agga, G. E., Scott, H. M., Amachawadi, R. G., Nagaraja, T. G., Vinasco, J., Bai, J., et al. (2014). Effects of chlortetracycline and copper supplementation on antimicrobial resistance of fecal Escherichia coli from weaned pigs. Prev. Veterinary Med. 114, 231–246. doi: 10.1016/j.prevetmed.2014.02.010
Akiba, M., Lin, J., Barton, Y. W., Zhang, Q. (2006). Interaction of CmeABC and CmeDEF in conferring antimicrobial resistance and maintaining cell viability in Campylobacter jejuni. J. Antimicrobial. Chemotherapy. 57 (1), 52–60. doi: 10.1093/jac/dki419
Akrami, F., Rajabnia, M., Pournajaf, A. (2019). Resistance integrons; a mini review. Caspian J. Internal Med. 10 (4), 370–376. doi: 10.22088/cjim.10.4.370
Amar, C. F., Arnold, C., Bankier, A., Dear, P. H., Guerra, B., Hopkins, K. L., et al. (2008). Real-time PCRs and fingerprinting assays for the detection and characterization of Salmonella genomic island-1 encoding multidrug resistance: application to 445 European isolates of Salmonella, Escherichia coli, Shigella, and Proteus. Microbial Drug Resistance 14 (2), 79–92. doi: 10.1089/mdr.2008.0812
Anyanwu, M. U., Jaja, I. F., Nwobi, O. C. (2020). Occurrence and characteristics of mobile colistin resistance (mcr) gene-containing isolates from the environment: A review. Int. J. Environ. Res. Public Health 17 (3), 1028. doi: 10.3390/ijerph17031028
ARC (2022). Global burden of bacterial antimicrobial resistance in 2019: a systematic analysis. Lancet. 399 (10325), 629–655. doi: 10.1016/S0140-6736(21)02724-0
Babakhani, S., Oloomi, M. (2018). Transposons: the agents of antibiotic resistance in bacteria. J. Basic Microbiol. 58 (11), 905–917. doi: 10.1002/jobm.201800204
Balasubramanian, D., Kong, K. F., Jayawardena, S. R., Leal, S. M., Sautter, R. T., Mathee, K. (2011). Co-Regulation of {beta}-lactam resistance, alginate production and quorum sensing in Pseudomonas aeruginosa. J. Med. Microbiol. 60 (Pt 2), 147–156. doi: 10.1099/jmm.0.021600-0
Balasubramanian, D., Schneper, L., Merighi, M., Smith, R., Narasimhan, G., Lory, S., et al. (2012). The regulatory repertoire of Pseudomonas aeruginosa AmpC ß-lactamase regulator AmpR includes virulence genes. PLos One 7 (3), e34067. doi: 10.1371/journal.pone.0034067
Balcázar, J. L. (2020). Implications of bacteriophages on the acquisition and spread of antibiotic resistance in the environment. Int. Microbiol. 23 (4), 475–479. doi: 10.1007/s10123-020-00121-5
Balouiri, M., Sadiki, M., Ibnsouda, S. K. (2016). Methods for in vitro evaluating antimicrobial activity: A review. J. Pharm. Analysis. 6 (2), 71–79. doi: 10.1016/j.jpha.2015.11.005
Baquero, F., Coque, T. M., Martínez, J. L., Aracil-Gisbert, S., Lanza, V. F. (2019). Gene transmission in the one health microbiosphere and the channels of antimicrobial resistance. Front. Microbiol. 10. doi: 10.3389/fmicb.2019.02892
Baquero, F., Martínez, J. L., Novais, Â., Rodríguez-Beltrán, J., Martínez-García, L., Coque, T. M., et al. (2021). Allogenous selection of mutational collateral resistance: old drugs select for new resistance within antibiotic families. Front. Microbiol. 12. doi: 10.3389/fmicb.2021.757833
Bencko, V., Šíma, P. (2018). A horizontal transmission of genetic information and its importance for development of antibiotics resistance. Casopis Lékaru Ceských. 157 (3), 141–145.
Benler, S., Faure, G., Altae-Tran, H., Shmakov, S., Zheng, F., Koonin, E. (2021). Cargo genes of Tn7-like transposons comprise an enormous diversity of defense systems, mobile genetic elements, and antibiotic resistance genes. mBio. 12 (6), e0293821. doi: 10.1128/mBio.02938-21
Bhagirath, A. Y., Li, Y., Patidar, R., Yerex, K., Ma, X., Kumar, A., et al. (2019). Two component regulatory systems and antibiotic resistance in gram-negative pathogens. Int. J. Mol. Sci. 20 (7), 1781. doi: 10.3390/ijms20071781
Biswas, R., Halder, U., Kabiraj, A., Mondal, A., Bandopadhyay, R. (2021). Overview on the role of heavy metals tolerance on developing antibiotic resistance in both gram-negative and gram-positive bacteria. Arch. Microbiol. 203 (6), 2761–2770. doi: 10.1007/s00203-021-02275-w
Bleriot, I., Blasco, L., Delgado-Valverde, M., Gual de Torella, A., Ambroa, A., Fernandez-Garcia, L., et al. (2020). Mechanisms of tolerance and resistance to chlorhexidine in clinical strains of Klebsiella pneumoniae producers of carbapenemase: role of new type II toxin-antitoxin system, PemIK. Toxins. 12 (9), 566. doi: 10.3390/toxins12090566
Böhm, M. E., Razavi, M., Marathe, N. P., Flach, C. F., Larsson, D. (2020). Discovery of a novel integron-borne aminoglycoside resistance gene present in clinical pathogens by screening environmental bacterial communities. Microbiome. 8 (1), 41. doi: 10.1186/s40168-020-00814-z
Borowiak, M., Fischer, J., Hammerl, J. A., Hendriksen, R. S., Szabo, I., Malorny, B. (2017). Identification of a novel transposon-associated phosphoethanolamine transferase gene, mcr-5, conferring colistin resistance in d-tartrate fermenting Salmonella enterica subsp. Enterica serovar Paratyphi B. J. Antimicrobial. Chemotherapy. 72 (12), 3317–3324. doi: 10.1093/jac/dkx327
Botelho, J., Schulenburg, H. (2021). The role of integrative and conjugative elements in antibiotic resistance evolution. Trends Microbiol. 29 (1), 8–18. doi: 10.1016/j.tim.2020.05.011
Branger, C., Ledda, A., Billard-Pomares, T., Doublet, B., Fouteau, S., Barbe, V., et al. (2018). Extended-spectrum β-lactamase-encoding genes are spreading on a wide range of Escherichia coli plasmids existing prior to the use of third-generation cephalosporins. Microbial Genomics 4 (9), e000203. doi: 10.1099/mgen.0.000203
Briaud, P., Camus, L., Bastien, S., Doléans-Jordheim, A., Vandenesch, F., Moreau, K. (2019). Coexistence with Pseudomonas aeruginosa alters Staphylococcus aureus transcriptome, antibiotic resistance and internalization into epithelial cells. Sci. Rep. 9 (1), 16564. doi: 10.1038/s41598-019-52975-z
Britten, R. J. (1996). DNA Sequence insertion and evolutionary variation in gene regulation. Proc. Natl. Acad. Sci. U.S.A. 93 (18), 9374–9377. doi: 10.1073/pnas.93.18.9374
Brunelle, B. W., Bearson, B. L., Bearson, S., Casey, T. A. (2017). Multidrug-resistant Salmonella enterica serovar Typhimurium isolates are resistant to antibiotics that influence their swimming and swarming motility. mSphere. 2 (6), e00306–e00317. doi: 10.1128/mSphere.00306-17
Burrus, V. (2017). Mechanisms of stabilization of integrative and conjugative elements. Curr. Opin. Microbiol. 38, 44–50. doi: 10.1016/j.mib.2017.03.014
Bustamante, P., Iredell, J. R. (2017). Carriage of type II toxin-antitoxin systems by the growing group of IncX plasmids. Plasmid. 91, 19–27. doi: 10.1016/j.plasmid.2017.02.006
Cambray, G., Guerout, A. M., Mazel, D. (2010). Integrons. Annu. Rev. Genet. 44, 141–166. doi: 10.1146/annurev-genet-102209-163504
Carraro, N., Rivard, N., Ceccarelli, D., Colwell, R. R., Burrus, V. (2016). IncA/C conjugative plasmids mobilize a new family of multidrug resistance islands in clinical Vibrio cholerae non-O1/non-O139 isolates from Haiti. mBio. 7 (4), e00509–e00516. doi: 10.1128/mBio.00509-16
Cepas, V., López, Y., Muñoz, E., Rolo, D., Ardanuy, C., Martí, S., et al. (2019). Relationship between biofilm formation and antimicrobial resistance in gram-negative bacteria. Microbial Drug Resistance. 25 (1), 72–79. doi: 10.1089/mdr.2018.0027
Chahboune, A., Decaffmeyer, M., Brasseur, R., Joris, B. (2005). Membrane topology of the Escherichia coli AmpG permease required for recycling of cell wall anhydromuropeptides and AmpC beta-lactamase induction. Antimicrobial. Agents Chemotherapy. 49 (3), 1145–1149. doi: 10.1128/AAC.49.3.1145-1149.2005
Chen, A. I., Albicoro, F. J., Zhu, J., Goulian, M. (2021). Effects of regulatory network organization and environment on PmrD connector activity and polymyxin resistance in Klebsiella pneumoniae and Escherichia coli. Antimicrobial. Agents Chemotherapy. 65 (3), e00889–e00820. doi: 10.1128/AAC.00889-20
Cheng, J., Hicks, D. B., Krulwich, T. A. (1996). The purified Bacillus subtilis tetracycline efflux protein TetA(L) reconstitutes both tetracycline-cobalt/H+ and Na+(K+)/H+ exchange. Proc. Natl. Acad. Sci. U.S.A. 25), 14446–14451. doi: 10.1073/pnas.93.25.14446
Che, Y., Yang, Y., Xu, X., Břinda, K., Polz, M. F., Hanage, W. P., et al. (2021). Conjugative plasmids interact with insertion sequences to shape the horizontal transfer of antimicrobial resistance genes. Proc. Natl. Acad. Sci. U.S.A. 118 (6), e2008731118. doi: 10.1073/pnas.2008731118
Choi, Y., Lee, J. Y., Lim, S. K., Ko, K. S. (2020). Intact pap2 downstream of mcr-1 appears to be required for colistin resistance. Diagn. Microbiol. Infect. Disease. 97 (1), 114997. doi: 10.1016/j.diagmicrobio.2020.114997
Choudhury, P., Kumar, R. (1996). Association of metal tolerance with multiple antibiotic resistance of enteropathogenic organisms isolated from coastal region of deltaic sunderbans. Indian J. Med. Res. 104, 148–151.
Ciric, L., Mullany, P., Roberts, A. P. (2011). Antibiotic and antiseptic resistance genes are linked on a novel mobile genetic element: Tn6087. J. Antimicrobial. Chemotherapy. 66 (10), 2235–2239. doi: 10.1093/jac/dkr311
Cohen, S. P., McMurry, L. M., Levy, S. B. (1988). marA locus causes decreased expression of OmpF porin in multiple-antibiotic-resistant (Mar) mutants of Escherichia coli. J. Bacteriology. 170 (12), 5416–5422. doi: 10.1128/jb.170.12.5416-5422.1988
Cotter, P. D., Guinane, C. M., Hill, C. (2002). The LisRK signal transduction system determines the sensitivity of Listeria monocytogenes to nisin and cephalosporins. Antimicrobial. Agents Chemotherapy. 46 (9), 2784–2790. doi: 10.1128/AAC.46.9.2784-2790.2002
Cuzon, G., Naas, T., Nordmann, P. (2011). Functional characterization of Tn4401, a Tn3-based transposon involved in blaKPC gene mobilization. Antimicrobial. Agents Chemotherapy. 55 (11), 5370–5373. doi: 10.1128/AAC.05202-11
D'Andrea, M. M., Literacka, E., Zioga, A., Giani, T., Baraniak, A., Fiett, J., et al. (2011). Evolution and spread of a multidrug-resistant Proteus mirabilis clone with chromosomal AmpC-type cephalosporinases in Europe. Antimicrobial. Agents Chemotherapy. 55 (6), 2735–2742. doi: 10.1128/AAC.01736-10
D'Souza, R., Nguyen, L. P., Pinto, N. A., Lee, H., Vu, T. N., Kim, H., et al. (2021). Role of AmpG in the resistance to β-lactam agents, including cephalosporins and carbapenems: candidate for a novel antimicrobial target. Ann. Clin. Microbiol. Antimicrobials. 20 (1), 45. doi: 10.1186/s12941-021-00446-7
Dai, L., Xia, J., Sahin, O., Zhang, Q. (2019). Identification of a nth-like gene encoding an endonuclease III in Campylobacter jejuni. Front. Microbiol. 10. doi: 10.3389/fmicb.2019.00698
Das, B., Bhadra, R. K. (2020). (p)ppGpp metabolism and antimicrobial resistance in bacterial pathogens. Front. Microbiol. 11. doi: 10.3389/fmicb.2020.563944
Debroy, R., Miryala, S. K., Naha, A., Anbarasu, A., Ramaiah, S. (2020). Gene interaction network studies to decipher the multi-drug resistance mechanism in Salmonella enterica serovar Typhi CT18 reveal potential drug targets. Microbial Pathogenesis. 142, 104096. doi: 10.1016/j.micpath.2020.104096
Delavat, F., Miyazaki, R., Carraro, N., Pradervand, N., van der Meer, J. R. (2017). The hidden life of integrative and conjugative elements. FEMS Microbiol. Rev. 41 (4), 512–537. doi: 10.1093/femsre/fux008
Deng, H., Sun, J., Ma, J., Li, L., Fang, L. X., Zhang, Q., et al. (2014). Identification of the multi-resistance gene cfr in Escherichia coli isolates of animal origin. PLos One 9 (7), e102378. doi: 10.1371/journal.pone.0102378
Deng, Y., Zeng, Z., Chen, S., He, L., Liu, Y., Wu, C., et al. (2011). Dissemination of IncFII plasmids carrying rmtB and qepA in Escherichia coli from pigs, farm workers and the environment. Clin. Microbiol. Infection. 17 (11), 1740–1745. doi: 10.1111/j.1469-0691.2011.03472.x
Depardieu, F., Podglajen, I., Leclercq, R., Collatz, E., Courvalin, P. (2007). Modes and modulations of antibiotic resistance gene expression. Clin. Microbiol. Rev. 20 (1), 79–114. doi: 10.1128/CMR.00015-06
Dhar, S., Kumari, H., Balasubramanian, D., Mathee, K. (2018). Cell-wall recycling and synthesis in Escherichia coli and Pseudomonas aeruginosa - their role in the development of resistance. J. Med. Microbiol. 67 (1), 1–21. doi: 10.1099/jmm.0.000636
Dik, D. A., Fisher, J. F., Mobashery, S. (2018). Cell-wall recycling of the gram-negative bacteria and the nexus to antibiotic resistance. Chem. Rev. 118 (12), 5952–5984. doi: 10.1021/acs.chemrev.8b00277
Duval-Valentin, G., Marty-Cointin, B., Chandler, M. (2004). Requirement of IS911 replication before integration defines a new bacterial transposition pathway. EMBO J. 23 (19), 3897–3906. doi: 10.1038/sj.emboj.7600395
Elizabeth, R., Baishya, S., Kalita, B., Wangkheimayum, J., Choudhury, M. D., Chanda, D. D., et al. (2022). Colistin exposure enhances expression of eptB in colistin-resistant Escherichia coli co-harboring mcr-1. Sci. Rep. 12 (1), 1348. doi: 10.1038/s41598-022-05435-0
Eyraud, A., Tattevin, P., Chabelskaya, S., Felden, B. (2014). A small RNA controls a protein regulator involved in antibiotic resistance in Staphylococcus aureus. Nucleic Acids Res. 42 (8), 4892–4905. doi: 10.1093/nar/gku149
Fage, C. D., Brown, D. B., Boll, J. M., Keatinge-Clay, A. T., Trent, M. S. (2014). Crystallographic study of the phosphoethanolamine transferase EptC required for polymyxin resistance and motility in Campylobacter jejuni. Acta Crystallographica Section D: Struct. Biol. 70 (Pt 10), 2730–2739. doi: 10.1107/S1399004714017623
Fernández-Alarcón, C., Singer, R. S., Johnson, T. J. (2011). Comparative genomics of multidrug resistance-encoding IncA/C plasmids from commensal and pathogenic Escherichia coli from multiple animal sources. PLos One 6 (8), e23415. doi: 10.1371/journal.pone.0023415
Fernández, L., Gooderham, W. J., Bains, M., McPhee, J. B., Wiegand, I., Hancock, R. E. (2010). Adaptive resistance to the "last hope" antibiotics polymyxin b and colistin in Pseudomonas aeruginosa is mediated by the novel two-component regulatory system ParR-ParS. Antimicrobial. Agents Chemotherapy. 54 (8), 3372–3382. doi: 10.1128/AAC.00242-10
Forster, S. C., Liu, J., Kumar, N., Gulliver, E. L., Gould, J. A., Escobar-Zepeda, A., et al. (2022). Strain-level characterization of broad host range mobile genetic elements transferring antibiotic resistance from the human microbiome. Nat. Commun. 13 (1), 1445. doi: 10.1038/s41467-022-29096-9
Friis, L. M., Pin, C., Taylor, D. E., Pearson, B. M., Wells, J. M. (2007). A role for the tet(O) plasmid in maintaining Campylobacter plasticity. Plasmid 57 (1), 18–28. doi: 10.1016/j.plasmid.2006.06.005
Gabashvili, E., Osepashvili, M., Koulouris, S., Ujmajuridze, L., Tskhitishvili, Z., Kotetishvili, M. (2020). Phage transduction is involved in the intergeneric spread of antibiotic resistance-associated blaCTX-m, mel, and tetM loci in natural populations of some human and animal bacterial pathogens. Curr. Microbiol. 77 (2), 185–193. doi: 10.1007/s00284-019-01817-2
Gan, I. N., Tan, H. S. (2019). A small RNA decreases the sensitivity of Shigella sonnei to norfloxacin. BMC Res. Notes. 12 (1), 97. doi: 10.1186/s13104-019-4124-4
Gantzhorn, M. R., Olsen, J. E., Thomsen, L. E. (2015). Importance of sigma factor mutations in increased triclosan resistance in Salmonella Typhimurium. BMC Microbiol. 15, 105. doi: 10.1186/s12866-015-0444-2
García-Aljaro, C., Ballesté, E., Muniesa, M. (2017). Beyond the canonical strategies of horizontal gene transfer in prokaryotes. Curr. Opin. Microbiol. 38, 95–105. doi: 10.1016/j.mib.2017.04.011
Geisinger, E., Mortman, N. J., Vargas-Cuebas, G., Tai, A. K., Isberg, R. R. (2018). A global regulatory system links virulence and antibiotic resistance to envelope homeostasis in Acinetobacter baumannii. PLos Pathogens. 14 (5), e1007030. doi: 10.1371/journal.ppat.1007030
Ghaly, T. M., Chow, L., Asher, A. J., Waldron, L. S., Gillings, M. R. (2017). Evolution of class 1 integrons: mobilization and dispersal via food-borne bacteria. PLos One 12 (6), e0179169. doi: 10.1371/journal.pone.0179169
Gharrah, M. M., Mostafa El-Mahdy, A., Barwa, R. F. (2017). Association between virulence factors and extended spectrum beta-lactamase producing Klebsiella pneumoniae compared to nonproducing isolates. Interdiscip. Perspect. Infect. Diseases 2017, 7279830. doi: 10.1155/2017/7279830
Gholizadeh, P., Köse, Ş., Dao, S., Ganbarov, K., Tanomand, A., Dal, T., et al. (2020). How CRISPR-cas system could be used to combat antimicrobial resistance. Infection Drug Resistance 13, 1111–1121. doi: 10.2147/IDR.S247271
Gilbert, P., McBain, A. J. (2003). Potential impact of increased use of biocides in consumer products on prevalence of antibiotic resistance. Clin. Microbiol. Rev. 16 (2), 189–208. doi: 10.1128/CMR.16.2.189-208.2003
Godeux, A. S., Svedholm, E., Barreto, S., Potron, A., Venner, S., Charpentier, X., et al. (2022). Interbacterial transfer of carbapenem resistance and large antibiotic resistance islands by natural transformation in pathogenic Acinetobacter. mBio. 13 (1), e0263121. doi: 10.1128/mbio.02631-21
Gómez-Sanz, E., Kadlec, K., Feßler, A. T., Zarazaga, M., Torres, C., Schwarz, S. (2013). Novel erm(T)-carrying multiresistance plasmids from porcine and human isolates of methicillin-resistant Staphylococcus aureus ST398 that also harbor cadmium and copper resistance determinants. Antimicrobial. Agents Chemotherapy. 57 (7), 3275–3282. doi: 10.1128/AAC.00171-13
Høyland-Kroghsbo, N. M., Paczkowski, J., Mukherjee, S., Broniewski, J., Westra, E., Bondy-Denomy, J., et al. (2017). Quorum sensing controls the Pseudomonas aeruginosa CRISPR-cas adaptive immune system. Proc. Natl. Acad. Sci. U.S.A. 1), 131–135. doi: 10.1073/pnas.1617415113
Haaber, J., Leisner, J. J., Cohn, M. T., Catalan-Moreno, A., Nielsen, J. B., Westh, H., et al. (2016). Bacterial viruses enable their host to acquire antibiotic resistance genes from neighbouring cells. Nat. Commun. 7, 13333. doi: 10.1038/ncomms13333
Haider, M. Z., Shabbir, M., Yaqub, T., Sattar, A., Maan, M. K., Mahmood, S. (2022). CRISPR-cas system: An adaptive immune system's association with antibiotic resistance in Salmonella enterica serovar Enteritidis. BioMed. Res. Int. 2022, 9080396. doi: 10.1155/2022/9080396
Han, J., Sahin, O., Barton, Y. W., Zhang, Q. (2008). Key role of mfd in the development of fluoroquinolone resistance in Campylobacter jejuni. PLos Pathog. 4 (6), e1000083. doi: 10.1371/journal.ppat.1000083
Han, Y., Zhou, Z. C., Zhu, L., Wei, Y. Y., Feng, W. Q., Xu, L., et al. (2019). The impact and mechanism of quaternary ammonium compounds on the transmission of antibiotic resistance genes. Environ. Sci. pollut. Res. Int. 26 (27), 28352–28360. doi: 10.1007/s11356-019-05673-2
Hashempour-Baltork, F., Hosseini, H., Shojaee-Aliabadi, S., Torbati, M., Alizadeh, A. M., Alizadeh, M. (2019). Drug resistance and the prevention strategies in food borne bacteria: an update review. Advanced Pharm. Bulletin. 9 (3), 335–347. doi: 10.15171/apb.2019.041
Hasman, H., Aarestrup, F. M. (2002). tcrB, a gene conferring transferable copper resistance in Enterococcus faecium: occurrence, transferability, and linkage to macrolide and glycopeptide resistance. Antimicrobial. Agents Chemotherapy. 46 (5), 1410–1416. doi: 10.1128/AAC.46.5.1410-1416.2002
Hayashi, S., Abe, M., Kimoto, M., Furukawa, S., Nakazawa, T. (2000). The dsbA-dsbB disulfide bond formation system of Burkholderia cepacia is involved in the production of protease and alkaline phosphatase, motility, metal resistance, and multi-drug resistance. Microbiol. Immunol. 44 (1), 41–50. doi: 10.1111/j.1348-0421.2000.tb01244.x
Hegstad, K., Langsrud, S., Lunestad, B. T., Scheie, A. A., Sunde, M., Yazdankhah, S. P. (2010). Does the wide use of quaternary ammonium compounds enhance the selection and spread of antimicrobial resistance and thus threaten our health? Microbial Drug Resistance 16 (2), 91–104. doi: 10.1089/mdr.2009.0120
Horinouchi, T., Maeda, T., Kotani, H., Furusawa, C. (2020). Suppression of antibiotic resistance evolution by single-gene deletion. Sci. Rep. 10 (1), 4178. doi: 10.1038/s41598-020-60663-6
Huang, J., Li, C., Song, J., Velkov, T., Wang, L., Zhu, Y., et al. (2020). Regulating polymyxin resistance in gram-negative bacteria: roles of two-component systems PhoPQ and PmrAB. Future Microbiol. 15 (6), 445–459. doi: 10.2217/fmb-2019-0322
Hu, W. S., Chen, H. W., Zhang, R. Y., Huang, C. Y., Shen, C. F. (2011). The expression levels of outer membrane proteins STM1530 and OmpD, which are influenced by the CpxAR and BaeSR two-component systems, play important roles in the ceftriaxone resistance of Salmonella enterica serovar Typhimurium. Antimicrobial. Agents Chemotherapy. 55 (8), 3829–3837. doi: 10.1128/AAC.00216-11
Jørgensen, T. S., Kiil, A. S., Hansen, M. A., Sørensen, S. J., Hansen, L. H. (2015). Current strategies for mobilome research. Front. Microbiol. 5. doi: 10.3389/fmicb.2014.00750
Jeon, B., Muraoka, W., Sahin, O., Zhang, Q. (2008). Role of Cj1211 in natural transformation and transfer of antibiotic resistance determinants in Campylobacter jejuni. Antimicrobial. Agents Chemotherapy 52 (8), 2699–2708. doi: 10.1128/AAC.01607-07
Jeon, B., Muraoka, W., Scupham, A., Zhang, Q. (2009). Roles of lipooligosaccharide and capsular polysaccharide in antimicrobial resistance and natural transformation of Campylobacter jejuni. J. Antimicrobial. Chemother. 63 (3), 462–468. doi: 10.1093/jac/dkn529
Jeon, B., Wang, Y., Hao, H., Barton, Y. W., Zhang, Q. (2011). Contribution of CmeG to antibiotic and oxidative stress resistance in Campylobacter jejuni. J. Antimicrobial. Chemotherapy. 66 (1), 79–85. doi: 10.1093/jac/dkq418
Jiang, J. H., Bhuiyan, M. S., Shen, H. H., Cameron, D. R., Rupasinghe, T., Wu, C. M., et al. (2019). Antibiotic resistance and host immune evasion in Staphylococcus aureus mediated by a metabolic adaptation. Proc. Natl. Acad. Sci. U.S.A. 9), 3722–3727. doi: 10.1073/pnas.1812066116
Johnson, C. M., Grossman, A. D. (2015). Integrative and conjugative elements (ICEs): what they do and how they work. Annu. Rev. Genet. 49, 577–601. doi: 10.1146/annurev-genet-112414-055018
Josenhans, C., Suerbaum, S. (2002). The role of motility as a virulence factor in bacteria. Int. J. Med. Microbiol. 291 (8), 605–614. doi: 10.1078/1438-4221-00173
Joynson, J. A., Forbes, B., Lambert, R. J. (2002). Adaptive resistance to benzalkonium chloride, amikacin and tobramycin: the effect on susceptibility to other antimicrobials. J. Appl. Microbiol. 93 (1), 96–107. doi: 10.1046/j.1365-2672.2002.01667.x
Juan, C., Torrens, G., González-Nicolau, M., Oliver, A. (2017). Diversity and regulation of intrinsic β-lactamases from non-fermenting and other gram-negative opportunistic pathogens. FEMS Microbiol. Rev. 41 (6), 781–815. doi: 10.1093/femsre/fux043
Juhas, M., van der Meer, J. R., Gaillard, M., Harding, R. M., Hood, D. W., Crook, D. W. (2009). Genomic islands: tools of bacterial horizontal gene transfer and evolution. FEMS Microbiol. Rev. 33 (2), 376–393. doi: 10.1111/j.1574-6976.2008.00136.x
Jurenaite, M., Markuckas, A., Suziedeliene, E. (2013). Identification and characterization of type II toxin-antitoxin systems in the opportunistic pathogen Acinetobacter baumannii. J. Bacteriology 195 (14), 3165–3172. doi: 10.1128/JB.00237-13
Kallipolitis, B. H., Ingmer, H., Gahan, C. G., Hill, C., Søgaard-Andersen, L. (2003). CesRK, a two-component signal transduction system in Listeria monocytogenes, responds to the presence of cell wall-acting antibiotics and affects beta-lactam resistance. Antimicrobial. Agents Chemotherapy. 47 (11), 3421–3429. doi: 10.1128/AAC.47.11.3421-3429.2003
Kamruzzaman, M., Iredell, J. (2019). A ParDE-family toxin antitoxin system in major resistance plasmids of Enterobacteriaceae confers antibiotic and heat tolerance. Sci. Rep. 9 (1), 9872. doi: 10.1038/s41598-019-46318-1
Kaur, U. J., Chopra, A., Preet, S., Raj, K., Kondepudi, K. K., Gupta, V., et al. (2021). Potential of 1-(1-napthylmethyl)-piperazine, an efflux pump inhibitor against cadmium-induced multidrug resistance in Salmonella enterica serovar Typhi as an adjunct to antibiotics. Braz. J. Microbiol. 52 (3), 1303–1313. doi: 10.1007/s42770-021-00492-5
Kidd, T. J., Mills, G., Sá-Pessoa, J., Dumigan, A., Frank, C. G., Insua, J. L., et al. (2017). A Klebsiella pneumoniae antibiotic resistance mechanism that subdues host defences and promotes virulence. EMBO Mol. Med. 9 (4), 430–447. doi: 10.15252/emmm.201607336
Kim, H. Y., Go, J., Lee, K. M., Oh, Y. T., Yoon, S. S. (2018). Guanosine tetra- and pentaphosphate increase antibiotic tolerance by reducing reactive oxygen species production in Vibrio cholerae. J. Biol. Chem. 293 (15), 5679–5694. doi: 10.1074/jbc.RA117.000383
Klein, K., Sonnabend, M. S., Frank, L., Leibiger, K., Franz-Wachtel, M., Macek, B., et al. (2019). Deprivation of the periplasmic chaperone surA reduces virulence and restores antibiotic susceptibility of multidrug-resistant Pseudomonas aeruginosa. Front. Microbiol. 10. doi: 10.3389/fmicb.2019.00100
Koskiniemi, S., Pränting, M., Gullberg, E., Näsvall, J., Andersson, D. I. (2011). Activation of cryptic aminoglycoside resistance in Salmonella enterica. Mol. Microbiol. 80 (6), 1464–1478. doi: 10.1111/j.1365-2958.2011.07657.x
Krawczyk-Balska, A., Marchlewicz, J., Dudek, D., Wasiak, K., Samluk, A. (2012). Identification of a ferritin-like protein of Listeria monocytogenes as a mediator of β-lactam tolerance and innate resistance to cephalosporins. BMC Microbiol. 12, 278. doi: 10.1186/1471-2180-12-278
Lázár, V., Pal Singh, G., Spohn, R., Nagy, I., Horváth, B., Hrtyan, M., et al. (2013). Bacterial evolution of antibiotic hypersensitivity. Mol. Syst. Biol. 9, 700. doi: 10.1038/msb.2013.57
Leavitt, A., Chmelnitsky, I., Ofek, I., Carmeli, Y., Navon-Venezia, S. (2010). Plasmid pKpQIL encoding KPC-3 and TEM-1 confers carbapenem resistance in an extremely drug-resistant epidemic Klebsiella pneumoniae strain. J. Antimicrobial. Chemotherapy. 65 (2), 243–248. doi: 10.1093/jac/dkp417
Lekunberri, I., Subirats, J., Borrego, C. M., Balcázar, J. L. (2017). Exploring the contribution of bacteriophages to antibiotic resistance. Environ. Pollution. 220 (Pt B), 981–984. doi: 10.1016/j.envpol.2016.11.059
Lian, X., Wang, X., Liu, X., Xia, J., Fang, L., Sun, J., et al. (2019). oqxAB-positive IncHI2 plasmid pHXY0908 increase Salmonella enterica serotype Typhimurium strains tolerance to ciprofloxacin. Front. Cell. Infection Microbiol. 9. doi: 10.3389/fcimb.2019.00242
Li, X. Z., Nikaido, H., Williams, K. E. (1997). Silver-resistant mutants of Escherichia coli display active efflux of ag+ and are deficient in porins. J. Bacteriology. 179 (19), 6127–6132. doi: 10.1128/jb.179.19.6127-6132.1997
Lin, D., Xie, M., Li, R., Chen, K., Chan, E. W., Chen, S. (2016). IncFII conjugative plasmid-mediated transmission of blaNDM-1 elements among animal-borne Escherichia coli strains. Antimicrobial. Agents Chemotherapy. 61 (1), e02285–e02216. doi: 10.1128/AAC.02285-16
Li, W., Tapiainen, T., Brinkac, L., Lorenzi, H. A., Moncera, K., Tejesvi, M. V., et al. (2021). Vertical transmission of gut microbiome and antimicrobial resistance genes in infants exposed to antibiotics at birth. J. Infect. Diseases. 224 (7), 1236–1246. doi: 10.1093/infdis/jiaa155
Liu, L., Feng, Y., Zhang, X., McNally, A., Zong, Z. (2017). New variant of mcr-3 in an extensively drug-resistant Escherichia coli clinical isolate carrying mcr-1 and blaNDM-5. Antimicrobial. Agents Chemotherapy 61 (12), e01757–e01717. doi: 10.1128/AAC.01757-17
Liu, D., Liu, W., Lv, Z., Xia, J., Li, X., Hao, Y., et al. (2019a). Emerging erm(B)-mediated macrolide resistance associated with novel multidrug resistance genomic islands in Campylobacter. Antimicrobial. Agents Chemotherapy 63 (7), e00153–e00119. doi: 10.1128/AAC.00153-19
Liu, D., Yang, Y., Gu, J., Tuo, H., Li, P., Xie, X., et al. (2019). The Yersinia high-pathogenicity island (HPI) carried by a new integrative and conjugative element (ICE) in a multidrug-resistant and hypervirulent Klebsiella pneumoniae strain SCsl1. Veterinary Microbiol. 239, 108481. doi: 10.1016/j.vetmic.2019.108481
Li, R., Xie, M., Lv, J., Wai-Chi Chan, E., Chen, S. (2017a). Complete genetic analysis of plasmids carrying mcr-1 and other resistance genes in an Escherichia coli isolate of animal origin. J. Antimicrobial. Chemotherapy 72 (3), 696–699. doi: 10.1093/jac/dkw509
Li, L., Ye, L., Kromann, S., Meng, H. (2017b). Occurrence of extended-spectrum β-lactamases, plasmid-mediated quinolone resistance, and disinfectant resistance genes in Escherichia coli isolated from ready-to-eat meat products. Foodborne Pathog. Disease. 14 (2), 109–115. doi: 10.1089/fpd.2016.2191
Li, R., Yu, H., Xie., M., Chen., K., Dong, N., Lin, D., et al. (2018). Genetic basis of chromosomally-encoded mcr-1 gene. Int. J. Antimicrobial. Agents. 51 (4), 578–585. doi: 10.1016/j.ijantimicag.2017.11.015
Li, J., Zhang, H., Ning, J., Sajid, A., Cheng, G., Yuan, Z., et al. (2019). The nature and epidemiology of OqxAB, a multidrug efflux pump. Antimicrobial. Resistance Infection Control. 8, 44. doi: 10.1186/s13756-019-0489-3
Luo, N., Pereira, S., Sahin, O., Lin, J., Huang, S., Michel, L., et al. (2005). Enhanced in vivo fitness of fluoroquinolone-resistant Campylobacter jejuni in the absence of antibiotic selection pressure. Proc. Natl. Acad. Sci. U.S.A. 102 (3), 541–546. doi: 10.1073/pnas.0408966102
Machuca, J., Briales, A., Díaz-de-Alba, P., Martínez-Martínez, L., Pascual, Á., Rodríguez-Martínez, J. M. (2015). Effect of the efflux pump QepA2 combined with chromosomally mediated mechanisms on quinolone resistance and bacterial fitness in Escherichia coli. J. Antimicrobial. Chemotherapy. 70 (9), 2524–2527. doi: 10.1093/jac/dkv144
Ma, T., Fu, J., Xie, N., Ma, S., Lei, L., Zhai, W., et al. (2020). Fitness cost of blaNDM-5-carrying p3R-IncX3 plasmids in wild-type NDM-free Enterobacteriaceae. Microorganisms. 8 (3), 377. doi: 10.3390/microorganisms8030377
Magiorakos, A. P., Srinivasan, A., Carey, R. B., Carmeli, Y., Falagas, M. E., Giske, C. G., et al. (2012). Multidrug-resistant, extensively drug-resistant and pandrug-resistant bacteria: an international expert proposal for interim standard definitions for acquired resistance. Clin. Microbiol. Infection. 18 (3), 268–281. doi: 10.1111/j.1469-0691.2011.03570.x
Ma, J., Liu, J., Zhang, Y., Wang, D., Liu, R., Liu, G., et al. (2019b). Bacitracin resistance and enhanced virulence of Streptococcus suis via a novel efflux pump. BMC Veterinary Res. 15 (1), 377. doi: 10.1186/s12917-019-2115-2
Ma, D., Mandell, J. B., Donegan, N. P., Cheung, A. L., Ma, W., Rothenberger, S., et al. (2019a). The toxin-antitoxin MazEF drives Staphylococcus aureus biofilm formation, antibiotic tolerance, and chronic infection. mBio. 10 (6), e01658–e01619. doi: 10.1128/mBio.01658-19
Marguerettaz, M., Dieppois, G., Que, Y. A., Ducret, V., Zuchuat, S., Perron, K. (2014). Sputum containing zinc enhances carbapenem resistance, biofilm formation and virulence of Pseudomonas aeruginosa. Microbial Pathogenesis. 77, 36–41. doi: 10.1016/j.micpath.2014.10.011
Marosevic, D., Kaevska, M., Jaglic, Z. (2017). Resistance to the tetracyclines and macrolide-lincosamide-streptogramin group of antibiotics and its genetic linkage - a review. Ann. Agric. Environ. Med. 24 (2), 338–344. doi: 10.26444/aaem/74718
Mata, M. T., Baquero, F., Pérez-Díaz, J. C. (2000). A multidrug efflux transporter in Listeria monocytogenes. FEMS Microbiol. Letters. 187 (2), 185–188. doi: 10.1111/j.1574-6968.2000.tb09158.x
Matthiessen, L. E., Hald, T., Vigre, H. (2022). System mapping of antimicrobial resistance to combat a rising global health crisis. Front. Public Health 10. doi: 10.3389/fpubh.2022.816943
Mediati, D. G., Wu, S., Wu, W., Tree, J. J. (2021). Networks of resistance: Small RNA control of antibiotic resistance. Trends Genet. 37 (1), 35–45. doi: 10.1016/j.tig.2020.08.016
Miryala, S. K., Anbarasu, A., Ramaiah, S. (2020). Role of SHV-11, a class a β-lactamase, gene in multidrug resistance among Klebsiella pneumoniae strains and understanding its mechanism by gene network analysis. Microbial Drug Resistance 26 (8), 900–908. doi: 10.1089/mdr.2019.0430
Miryala, S. K., Ramaiah, S. (2019). Exploring the multi-drug resistance in Escherichia coli O157:H7 by gene interaction network: A systems biology approach. Genomics 111 (4), 958–965. doi: 10.1016/j.ygeno.2018.06.002
Mohan Raj, J. R., Karunasagar, I. (2019). Phages amid antimicrobial resistance. Crit. Rev. Microbiol. 45 (5-6), 701–711. doi: 10.1080/1040841X.2019.1691973
Mohanraj, R. S., Mandal, J. (2022). Azithromycin can induce SOS response and horizontal gene transfer of SXT element in Vibrio cholerae. Mol. Biol. Rep. 49 (6), 4737–4748. doi: 10.1007/s11033-022-07323-2
Moritz, E. M., Hergenrother, P. J. (2006). Toxin-antitoxin systems are ubiquitous and plasmid-encoded in vancomycin-resistant enterococci. Proc. Natl. Acad. Sci. U S A. 104 (1), 311–316. doi: 10.1073/pnas.0601168104
Mortensen, K., Lam, T. J., Ye, Y. (2021). Comparison of CRISPR-cas immune systems in healthcare-related pathogens. Front. Microbiol. 12. doi: 10.3389/fmicb.2021.758782
Motamedi, H., Asghari, B., Tahmasebi, H., Arabestani, M. R. (2018). Identification of hemolysine genes and their association with antimicrobial resistance pattern among clinical isolates of Staphylococcus aureus in west of Iran. Advanced Biomed. Res. 7, 153. doi: 10.4103/abr.abr_143_18
Mourão, J., Novais, C., Machado, J., Peixe, L., Antunes, P. (2015). Metal tolerance in emerging clinically relevant multidrug-resistant Salmonella enterica serotype 4,[5],12:i:- clones circulating in Europe. Int. J. Antimicrobial. Agents. 45 (6), 610–616. doi: 10.1016/j.ijantimicag.2015.01.013
Mwangi, M. M., Kim, C., Chung, M., Tsai, J., Vijayadamodar, G., Benitez, M., et al. (2013). Whole-genome sequencing reveals a link between β-lactam resistance and synthetases of the alarmone (p)ppGpp in Staphylococcus aureus. Microbial Drug Resistance 3, 153–159. doi: 10.1089/mdr.2013.0053
Naha, A., Kumar Miryala, S., Debroy, R., Ramaiah, S., Anbarasu, A. (2020). Elucidating the multi-drug resistance mechanism of Enterococcus faecalis V583: A gene interaction network analysis. Gene 748, 144704. doi: 10.1016/j.gene.2020.144704
Nicoloff, H., Andersson, D. I. (2016). Indirect resistance to several classes of antibiotics in cocultures with resistant bacteria expressing antibiotic-modifying or -degrading enzymes. J. Antimicrobial. Chemotherapy 71 (1), 100–110. doi: 10.1093/jac/dkv312
Nishino, K., Nikaido, E., Yamaguchi, A. (2007). Regulation of multidrug efflux systems involved in multidrug and metal resistance of Salmonella enterica serovar Typhimurium. J. Bacteriology 189 (24), 9066–9075. doi: 10.1128/JB.01045-07
Nowak, J., Schneiders, T., Seifert, H., Higgins, P. G. (2015). The Asp20-to-Asn substitution in the response regulator AdeR leads to enhanced efflux activity of AdeB in Acinetobacter baumannii. Antimicrobial. Agents Chemotherapy. 60 (2), 1085–1090. doi: 10.1128/AAC.02413-15
Obaidat, M. M., Salman, A. E., Lafi, S. Q. (2015). Prevalence of Staphylococcus aureus in imported fish and correlations between antibiotic resistance and enterotoxigenicity. J. Food Protection 78 (11), 1999–2005. doi: 10.4315/0362-028X.JFP-15-104
Pandey, S., Sahukhal, G. S., Elasri, M. O. (2019). The msaABCR operon regulates the response to oxidative stress in Staphylococcus aureus. J. Bacteriology. 201 (21), e00417–e00419. doi: 10.1128/JB.00417-19
Pasquaroli, S., Di Cesare, A., Vignaroli, C., Conti, G., Citterio, B., Biavasco, F. (2014). Erythromycin- and copper-resistant enterococcus hirae from marine sediment and co-transfer of erm(B) and tcrB to human Enterococcus faecalis. Diagn. Microbiol. Infect. Disease. 80 (1), 26–28. doi: 10.1016/j.diagmicrobio.2014.06.002
Plata, K. B., Riosa, S., Singh, C. R., Rosato, R. R., Rosato, A. E. (2013). Targeting of PBP1 by β-lactams determines recA/SOS response activation in heterogeneous MRSA clinical strains. PLos One 8 (4), e61083. doi: 10.1371/journal.pone.0061083
Poulakou, G., Bassetti, M., Righi, E., Dimopoulos, G. (2014). Current and future treatment options for infections caused by multidrug-resistant gram-negative pathogens. Future Microbiol. 9 (9), 1053–1069. doi: 10.2217/fmb.14.58
Qi, Q., Kamruzzaman, M., Iredell, J. R. (2021). The higBA-type toxin-antitoxin system in IncC plasmids is a mobilizable ciprofloxacin-inducible system. mSphere. 6 (3), e0042421. doi: 10.1128/mSphere.00424-21
Qin, S., Wang, Y., Zhang, Q., Zhang, M., Deng, F., Shen, Z., et al. (2014). Report of ribosomal RNA methylase gene erm(B) in multidrug-resistant Campylobacter coli. J. Antimicrobial. Chemotherapy. 69 (4), 964–968. doi: 10.1093/jac/dkt492
Qin, S., Zhang, C., Schwarz, S., Li, L., Dong, H., Yao, H., et al. (2020). Identification of a novel conjugative mcr-8.2-bearing plasmid in an almost pan-resistant hypermucoviscous Klebsiella pneumoniae ST11 isolate with enhanced virulence. J. Antimicrobial. Chemotherapy. 75 (9), 2696–2699. doi: 10.1093/jac/dkaa226
Qi, Q., Preston, G. M., MacLean, R. C. (2014). Linking system-wide impacts of RNA polymerase mutations to the fitness cost of rifampin resistance in Pseudomonas aeruginosa. mBio. 5 (6), e01562. doi: 10.1128/mBio.01562-14
Queenan, K., Häsler, B., Rushton, J. (2016). A one health approach to antimicrobial resistance surveillance: is there a business case for it? Int. J. Antimicrobial. Agents. 48 (4), 422–427. doi: 10.1016/j.ijantimicag.2016.06.014
Rakic-Martinez, M., Drevets, D. A., Dutta, V., Katic, V., Kathariou, S. (2011). Listeria monocytogenes strains selected on ciprofloxacin or the disinfectant benzalkonium chloride exhibit reduced susceptibility to ciprofloxacin, gentamicin, benzalkonium chloride, and other toxic compounds. Appl. Environ. Microbiol. 77 (24), 8714–8721. doi: 10.1128/AEM.05941-11
Ranjbar, R., Giammanco, G. M., Aleo, A., Plano, M. R., Naghoni, A., Owlia, P., et al. (2010). Characterization of the first extended-spectrum beta-lactamase-producing nontyphoidal Salmonella strains isolated in Tehran, Iran. Foodborne Pathog. Disease. 7 (1), 91–95. doi: 10.1089/fpd.2009.0382
Rehman, A., Andleeb, S., Ullah, S. R., Mustafa, Z., Gul, D., Mehmood, K. (2021b). Antibiotic-mediated expression analysis of shiga toxin 1 and 2 in multi-drug-resistant shiga toxigenic Escherichia coli. Folia Microbiologica. 66 (5), 809–817. doi: 10.1007/s12223-021-00882-0
Rehman, A., Jeukens, J., Levesque, R. C., Lamont, I. L. (2021a). Gene-gene interactions dictate ciprofloxacin resistance in Pseudomonas aeruginosa and facilitate prediction of resistance phenotype from genome sequence data. Antimicrobial. Agents Chemotherapy. 65 (7), e0269620. doi: 10.1128/AAC.02696-20
Rensch, U., Klein, G., Kehrenberg, C. (2013). Analysis of triclosan-selected Salmonella enterica mutants of eight serovars revealed increased aminoglycoside susceptibility and reduced growth rates. PLos One 8 (10), e78310. doi: 10.1371/journal.pone.0078310
Rivard, N., Colwell, R. R., Burrus, V. (2020). Antibiotic resistance in Vibrio cholerae: mechanistic insights from IncC plasmid-mediated dissemination of a novel family of genomic islands inserted at trmE. mSphere. 5 (4), e00748–e00720. doi: 10.1128/mSphere.00748-20
Roberts, A. P., Mullany, P. (2011). Tn916-like genetic elements: a diverse group of modular mobile elements conferring antibiotic resistance. FEMS Microbiol. Rev. 35 (5), 856–871. doi: 10.1111/j.1574-6976.2011.00283.x
Rodgers, D., Le, C., Pimentel, C., Tuttobene, M. R., Subils, T., Escalante, J., et al. (2021). Histone-like nucleoid-structuring protein (H-NS) regulatory role in antibiotic resistance in Acinetobacter baumannii. Sci. Rep. 11 (1), 18414. doi: 10.1038/s41598-021-98101-w
Ross, J., Topp, E. (2015). Abundance of antibiotic resistance genes in bacteriophage following soil fertilization with dairy manure or municipal biosolids, and evidence for potential transduction. Appl. Environ. Microbiol. 81 (22), 7905–7913. doi: 10.1128/AEM.02363-15
Rosvoll, T. C., Pedersen, T., Sletvold, H., Johnsen, P. J., Sollid, J. E., Simonsen, G. S., et al. (2010). PCR-based plasmid typing in Enterococcus faecium strains reveals widely distributed pRE25-, pRUM-, pIP501-and pHTbeta-related replicons associated with glycopeptide resistance and stabilizing toxin-antitoxin systems. FEMS Immunol. Med. Microbiol. 58 (2), 254–268. doi: 10.1111/j.1574-695X.2009.00633.x
Roux, D., Danilchanka, O., Guillard, T., Cattoir, V., Aschard, H., Fu, Y., et al. (2015). Fitness cost of antibiotic susceptibility during bacterial infection. Sci. Trans. Med. 7 (297), 297ra114. doi: 10.1126/scitranslmed.aab1621
Ruiz, J., Pons, M. J., Gomes, C. (2012). Transferable mechanisms of quinolone resistance. Int. J. Antimicrobial. Agents. 40 (3), 196–203. doi: 10.1016/j.ijantimicag.2012.02.011
Salyers, A. A., Shoemaker, N. B., Stevens, A. M., Li, L. Y. (1995). Conjugative transposons: an unusual and diverse set of integrated gene transfer elements. Microbiol. Rev. 59 (4), 579–590. doi: 10.1128/mr.59.4.579-590.1995
Samanta, D., Elasri, M. O. (2014). The msaABCR operon regulates resistance in vancomycin-intermediate Staphylococcus aureus strains. Antimicrobial. Agents Chemotherapy 58 (11), 6685–6695. doi: 10.1128/AAC.03280-14
Sánchez, M. B., Martínez, J. L. (2012). Differential epigenetic compatibility of qnr antibiotic resistance determinants with the chromosome of Escherichia coli. PLos One 7 (5), e35149. doi: 10.1371/journal.pone.0035149
Schaufler, K., Semmler, T., Pickard, D. J., de Toro, M., de la Cruz, F., Wieler, L. H., et al. (2016). Carriage of extended-spectrum beta-lactamase-plasmids does not reduce fitness but enhances virulence in some strains of pandemic E. coli lineages. Front. Microbiol. 7. doi: 10.3389/fmicb.2016.00336
Shabbir, M. A., Wu, Q., Shabbir, M. Z., Sajid, A., Ahmed, S., Sattar, A., et al. (2018). The CRISPR-cas system promotes antimicrobial resistance in Campylobacter jejuni. Future Microbiol. 13, 1757–1774. doi: 10.2217/fmb-2018-0234
Sharma, A., Sharma, R., Bhattacharyya, T., Bhando, T., Pathania, R. (2017). Fosfomycin resistance in Acinetobacter baumannii is mediated by efflux through a major facilitator superfamily (MFS) transporter-AbaF. J. Antimicrobial. Chemotherapy 72 (1), 68–74. doi: 10.1093/jac/dkw382
Shawa, M., Furuta, Y., Mulenga, G., Mubanga, M., Mulenga, E., Zorigt, T., et al. (2021). Novel chromosomal insertions of ISEcp1-blaCTX-M-15 and diverse antimicrobial resistance genes in Zambian clinical isolates of enterobacter cloacae and Escherichia coli. Antimicrobial. Resistance Infection Control. 10 (1), 79. doi: 10.1186/s13756-021-00941-8
Shousha, A., Awaiwanont, N., Sofka, D., Smulders, F. J., Paulsen, P., Szostak, M. P., et al. (2015). Bacteriophages isolated from chicken meat and the horizontal transfer of antimicrobial resistance genes. Appl. Environ. Microbiol. 81 (14), 4600–4606. doi: 10.1128/AEM.00872-15
Siebor, E., Neuwirth, C. (2022). Overview of Salmonella genomic island 1-related elements among gamma-proteobacteria reveals their wide distribution among environmental species. Front. Microbiol. 1013. doi: 10.3389/fmicb.2022.857492
Siguier, P., Filée, J., Chandler, M. (2006). Insertion sequences in prokaryotic genomes. Curr. Opin. Microbiol. 5, 526–531. doi: 10.1016/j.mib.2006.08.005
Sinel, C., Cacaci, M., Meignen, P., Guérin, F., Davies, B. W., Sanguinetti, M., et al. (2017). Subinhibitory concentrations of ciprofloxacin enhance antimicrobial resistance and pathogenicity of Enterococcus faecium. Antimicrobial. Agents Chemotherapy. 61 (5), e02763–e02716. doi: 10.1128/AAC.02763-16
Sistrunk, J. R., Nickerson, K. P., Chanin, R. B., Rasko, D. A., Faherty, C. S. (2016). Survival of the fittest: how bacterial pathogens utilize bile to enhance infection. Clin. Microbiol. Rev. 29 (4), 819–836. doi: 10.1128/CMR.00031-16
Snesrud, E., McGann, P., Chandler, M. (2018). The birth and demise of the ISApl1-mcr-1-ISApl1 composite transposon: the vehicle for transferable colistin resistance. mBio. 9 (1), e02381–e02317. doi: 10.1128/mBio.02381-17
Snyder, H., Kellogg, S. L., Skarda, L. M., Little, J. L., Kristich, C. J. (2014). Nutritional control of antibiotic resistance via an interface between the phosphotransferase system and a two-component signaling system. Antimicrobial. Agents Chemotherapy 58 (2), 957–965. doi: 10.1128/AAC.01919-13
Soltani, E., Hasani, A., Rezaee, M. A., Pirzadeh, T., Oskouee, M. A., Hasani, A., et al. (2020). Virulence characterization of Klebsiella pneumoniae and its relation with ESBL and AmpC beta-lactamase associated resistance. Iranian J. Microbiol. 12 (2), 98–106.
Song, S., Wood, T. K. (2020). Toxin/antitoxin system paradigms: toxins bound to antitoxins are not likely activated by preferential antitoxin degradation. Advanced Biosystems. 4 (3), e1900290. doi: 10.1002/adbi.201900290
Strugeon, E., Tilloy, V., Ploy, M. C., Da Re, S. (2016). The stringent response promotes antibiotic resistance dissemination by regulating integron integrase expression in biofilms. mBio. 7 (4), e00868–e00816. doi: 10.1128/mBio.00868-16
Sun, F., Ding, Y., Ji, Q., Liang, Z., Deng, X., Wong, C. C., et al. (2012). Protein cysteine phosphorylation of SarA/MgrA family transcriptional regulators mediates bacterial virulence and antibiotic resistance. Proc. Natl. Acad. Sci. U.S.A. 38), 15461–15466. doi: 10.1073/pnas.1205952109
Suzuki, H., Yano, H., Brown, C. J., Top, E. M. (2010). Predicting plasmid promiscuity based on genomic signature. J. Bacteriol. 192 (22), 6045–6055. doi: 10.1128/JB.00277-10
Suzuki, S., Horinouchi, T., Furusawa, C. (2014). Prediction of antibiotic resistance by gene expression profiles. Nat. Commun. 5, 5792. doi: 10.1038/ncomms6792
Swick, M. C., Morgan-Linnell, S. K., Carlson, K. M., Zechiedrich, L. (2011). Expression of multidrug efflux pump genes acrAB-tolC, mdfA, and norE in Escherichia coli clinical isolates as a function of fluoroquinolone and multidrug resistance. Antimicrobial. Agents Chemotherapy. 55 (2), 921–924. doi: 10.1128/AAC.00996-10
Tang, Y., Dai, L., Sahin, O., Wu, Z., Liu, M., Zhang, Q. (2017). Emergence of a plasmid-borne multidrug resistance gene cfr(C) in foodborne pathogen Campylobacter. J. Antimicrobial. Chemotherapy. 72 (6), 1581–1588. doi: 10.1093/jac/dkx023
Tang, Y., Lai, Y., Wang, X., Lei, C., Li, C., Kong, L., et al. (2020b). Novel insertion sequence ISChh1-like mediating acquisition of optrA gene in foodborne pathogen Campylobacter coli of swine origin. Veterinary Microbiol. 252, 108934. doi: 10.1016/j.vetmic.2020.108934
Tang, B., Tang, Y., Zhang, L., Liu, X., Chang, J., Xia, X., et al. (2020a). Emergence of fexA in mediating resistance to florfenicols in Campylobacter. Antimicrobial. Agents Chemotherapy. 64 (7), e00260–e00220. doi: 10.1128/AAC.00260-20
Teixeira, P., Tacão, M., Alves, A., Henriques, I. (2016). Antibiotic and metal resistance in a ST395 Pseudomonas aeruginosa environmental isolate: A genomics approach. Mar. pollut. Bulletin. 110 (1), 75–81. doi: 10.1016/j.marpolbul.2016.06.086
Thomas, J., Lee, C. A., Grossman, A. D. (2013). A conserved helicase processivity factor is needed for conjugation and replication of an integrative and conjugative element. PLos Genet. 9 (1), e1003198. doi: 10.1371/journal.pgen.1003198
Tierney, A. R., Rather, P. N. (2019). Roles of two-component regulatory systems in antibiotic resistance. Future Microbiol. 14 (6), 533–552. doi: 10.2217/fmb-2019-0002
Toleman, M. A., Walsh, T. R. (2011). Combinatorial events of insertion sequences and ICE in gram-negative bacteria. FEMS Microbiol. Rev. 35 (5), 912–935. doi: 10.1111/j.1574-6976.2011.00294.x
Tolmasky, M. E. (2000). Bacterial resistance to aminoglycosides and beta-lactams: the Tn1331 transposon paradigm. Front. Bioscience. 5, D20–D29. doi: 10.2741/tolmasky
Vats, P., Kaur, U. J., Rishi, P. (2022). Heavy metal-induced selection and proliferation of antibiotic resistance: A review. J. Appl. Microbiol. 132 (6), 4058–4076. doi: 10.1111/jam.15492
Vázquez, X., Fernández, J., Bances., M., de Toro., M., Ladero, V., Rodicio, R., et al. (2022). Colistin resistance in monophasic isolates of Salmonella enterica ST34 collected from meat-derived products in Spain, with or without CMY-2 co-production. Front. Microbiol. 12. doi: 10.3389/fmicb.2021.735364
Viducic, D., Ono, T., Murakami, K., Susilowati, H., Kayama, S., Hirota, K., et al. (2006). Functional analysis of spoT, relA and dksA genes on quinolone tolerance in Pseudomonas aeruginosa under nongrowing condition. Microbiol. Immunol. 50 (4), 349–357. doi: 10.1111/j.1348-0421.2006.tb03793.x
Wachino, J. I., Jin, W., Kimura, K., Arakawa, Y. (2019). Intercellular transfer of chromosomal antimicrobial resistance genes between Acinetobacter baumannii strains mediated by prophages. Antimicrobial. Agents Chemotherapy. 63 (8), e00334–e00319. doi: 10.1128/AAC.00334-19
Wales, A. D., Davies, R. H. (2015). Co-Selection of resistance to antibiotics, biocides and heavy metals, and its relevance to foodborne pathogens. Antibiotics. 4 (4), 567–604. doi: 10.3390/antibiotics4040567
Wang, Y., Gong, S., Dong, X., Li, J., Grenier, D., Yi, L. (2020b). In vitro mixed biofilm of Streptococcus suis and Actinobacillus pleuropneumoniae impacts antibiotic susceptibility and modulates virulence factor gene expression. Front. Microbiol. 11. doi: 10.3389/fmicb.2020.00507
Wang, Y., Liu, B., Grenier, D., Yi, L. (2019). Regulatory mechanisms of the LuxS/AI-2 system and bacterial resistance. Antimicrobial. Agents Chemotherapy. 63 (10), e01186–e01119. doi: 10.1128/AAC.01186-19
Wang, R., van Dorp, L., Shaw, L. P., Bradley, P., Wang, Q., Wang, X., et al. (2018a). The global distribution and spread of the mobilized colistin resistance gene mcr-1. Nat. Commun. 9 (1), 1179. doi: 10.1038/s41467-018-03205-z
Wang, M., Zeng, Z., Jiang, F., Zheng, Y., Shen, H., Macedo, N., et al. (2020a). Role of enterotoxigenic Escherichia coli prophage in spreading antibiotic resistance in a porcine-derived environment. Environ. Microbiol. 22 (12), 4974–4984. doi: 10.1111/1462-2920.15084
Wang, Q., Zeng, X., Yang, Q., Yang, C. (2018b). Identification of a bacteriophage from an environmental multidrug-resistant E. coli isolate and its function in horizontal transfer of ARGs. Sci. Total Environment. 639, 617–623. doi: 10.1016/j.scitotenv.2018.05.213
Wang, J., Zhi, C. P., Chen, X. J., Guo, Z. W., Liu, W. L., Luo, J., et al. (2017). Characterization of oqxAB in Escherichia coli isolates from animals, retail meat, and human patients in guangzhou, China. Front. Microbiol. 8. doi: 10.3389/fmicb.2017.01982
Waters, C. M., Bassler, B. L. (2005). Quorum sensing: cell-to-cell communication in bacteria. Annu. Rev. Cell Dev. Biol. 21, 319–346. doi: 10.1146/annurev.cellbio.21.012704.131001
Weir, E. K., Martin, L. C., Poppe, C., Coombes, B. K., Boerlin, P. (2008). Subinhibitory concentrations of tetracycline affect virulence gene expression in a multi-resistant Salmonella enterica subsp. Enterica serovar Typhimurium DT104. Microbes Infection. 10 (8), 901–907. doi: 10.1016/j.micinf.2008.05.005
Wendling, C. C., Refardt, D., Hall, A. R. (2021). Fitness benefits to bacteria of carrying prophages and prophage-encoded antibiotic-resistance genes peak in different environments. Evolution. 75 (2), 515–528. doi: 10.1111/evo.14153
Wesgate, R., Fanning, S., Hu, Y., Maillard, J. Y. (2020). Effect of exposure to chlorhexidine residues at "During use" concentrations on antimicrobial susceptibility profile, efflux, conjugative plasmid transfer, and metabolism of Escherichia coli. Antimicrobial. Agents Chemotherapy. 64 (12), e01131–e01120. doi: 10.1128/AAC.01131-20
Winter, M., Buckling, A., Harms, K., Johnsen, P. J., Vos, M. (2021). Antimicrobial resistance acquisition via natural transformation: context is everything. Curr. Opin. Microbiol. 64, 133–138. doi: 10.1016/j.mib.2021.09.009
Wong, M. H., Chan, E. W., Chen, S. (2017). IS26-mediated formation of a virulence and resistance plasmid in Salmonella enteritidis. J. Antimicrobial. Chemotherapy. 72 (10), 2750–2754. doi: 10.1093/jac/dkx238
Wozniak, A., Figueroa, C., Moya-Flores, F., Guggiana, P., Castillo, C., Rivas, L., et al. (2021). A multispecies outbreak of carbapenem-resistant bacteria harboring the blaKPC gene in a non-classical transposon element. BMC Microbiol. 21 (1), 107. doi: 10.1186/s12866-021-02169-3
Wozniak, R. A., Waldor, M. K. (2009). A toxin-antitoxin system promotes the maintenance of an integrative conjugative element. PLos Genet. 5 (3), e1000439. doi: 10.1371/journal.pgen.1000439
Xia, Y., Wang, D., Pan, X., Xia, B., Weng, Y., Long, Y., et al. (2020). TpiA is a key metabolic enzyme that affects virulence and resistance to aminoglycoside antibiotics through CrcZ in Pseudomonas aeruginosa. mBio. 11 (1), e02079–e02019. doi: 10.1128/mBio.02079-19
Xu, Q., Chen, T., Yan, B., Zhang, L., Pi, B., Yang, Y., et al. (2019). Dual role of gnaA in antibiotic resistance and virulence in Acinetobacter baumannii. Antimicrobial. Agents Chemotherapy. 63 (10), e00694–e00619. doi: 10.1128/AAC.00694-19
Yang, Y., Xie, X., Tang, M., Liu, J., Tuo, H., Gu, J., et al. (2020). Exploring the profile of antimicrobial resistance genes harboring by bacteriophage in chicken feces. Sci. Total Environ. 700, 134446. doi: 10.1016/j.scitotenv.2019.134446
Yang, Y., Zhang, A., Lei, C., Wang, H., Guan, Z., Xu, C., et al. (2015). Characteristics of plasmids coharboring 16S rRNA methylases, CTX-m, and virulence factors in Escherichia coli and Klebsiella pneumoniae isolates from chickens in China. Foodborne Pathog. Disease. 12 (11), 873–880. doi: 10.1089/fpd.2015.2025
Yi, L., Durand, R., Grenier, F., Yang, J., Yu, K., Burrus, V., et al. (2022). PixR, a novel activator of conjugative transfer of IncX4 resistance plasmids, mitigates the fitness cost of mcr-1 carriage in Escherichia coli. mBio. 13 (1), e0320921. doi: 10.1128/mbio.03209-21
Yoon, E. J., Chabane, Y. N., Goussard, S., Snesrud, E., Courvalin, P., Dé, E., et al. (2015). Contribution of resistance-nodulation-cell division efflux systems to antibiotic resistance and biofilm formation in Acinetobacter baumannii. mBio. 6 (2), e00309–e00315. doi: 10.1128/mBio.00309-15
Zare, S., Derakhshandeh, A., Mohammadi, A., Noshadi, M. (2021). Abundance of antibiotic resistance genes in bacteria and bacteriophages isolated from wastewater in Shiraz. Mol. Biol. Res. Commun. 10 (2), 73–83. doi: 10.22099/mbrc.2021.39468.1584
Zhai, Y., He, Z., Kang, Y., Yu, H., Wang, J., Du, P., et al. (2016). Complete nucleotide sequence of pH11, an IncHI2 plasmid conferring multi-antibiotic resistance and multi-heavy metal resistance genes in a clinical Klebsiella pneumoniae isolate. Plasmid. 86, 26–31. doi: 10.1016/j.plasmid.2016.04.001
Zhai, Y. J., Huang, H., Liu, J., Sun, H. R., He, D., Pan, Y. S., et al. (2018). CpxR overexpression increases the susceptibility of acrB and cpxR double-deleted Salmonella enterica serovar Typhimurium to colistin. J. Antimicrobial. Chemotherapy. 73 (11), 3016–3024. doi: 10.1093/jac/dky320
Zhang, Z., Du, W., Wang, M., Li, Y., Su, S., Wu, T., et al. (2020). Contribution of the colicin receptor CirA to biofilm formation, antibiotic resistance, and pathogenicity of Salmonella enteritidis. J. Basic Microbiol. 60 (1), 72–81. doi: 10.1002/jobm.201900418
Zhang, Y. F., Han, K., Chandler, C. E., Tjaden, B., Ernst, R. K., Lory, S. (2017). Probing the sRNA regulatory landscape of P. aeruginosa: post-transcriptional control of determinants of pathogenicity and antibiotic susceptibility. Mol. Microbiol. 106 (6), 919–937. doi: 10.1111/mmi.13857
Zhang, S., Wang, J., Jiang, M., Xu, D., Peng, B., Peng, X. X., et al. (2019). Reduced redox-dependent mechanism and glucose-mediated reversal in gentamicin-resistant Vibrio alginolyticus. Environ. Microbiol. 21 (12), 4724–4739. doi: 10.1111/1462-2920.14811
Zhang, S., Wang, D., Wang, Y., Hasman, H., Aarestrup, F. M., Alwathnani, H. A., et al. (2015a). Genome sequences of copper resistant and sensitive Enterococcus faecalis strains isolated from copper-fed pigs in Denmark. Standards Genomic Sci. 10, 35. doi: 10.1186/s40793-015-0021-1
Zhang, Y., Zeng, J., Liu, W., Zhao, F., Hu, Z., Zhao, C., et al. (2015b). Emergence of a hypervirulent carbapenem-resistant Klebsiella pneumoniae isolate from clinical infections in China. J. Infect. 71 (5), 553–560. doi: 10.1016/j.jinf.2015.07.010
Zhao, Q. Y., Zhu, J. H., Cai, R. M., Zheng, X. R., Zhang, L. J., Chang, M. X., et al. (2021). IS26 is responsible for the evolution and transmission of blaNDM-harboring plasmids in Escherichia coli of poultry origin in China. mSystems 6 (4), e0064621. doi: 10.1128/mSystems.00646-21
Zheng, Z., Cheng, Q., Chan, E. W., Chen, S. (2020). Genetic and biochemical characterization of VMB-1, a novel metallo-β-lactamase encoded by a conjugative, broad-host range IncC plasmid from vibrio spp. Advanced Biosystems. 4 (3), e1900221. doi: 10.1002/adbi.201900221
Keywords: CRiSPR/Cas, efflux pump, mobile genetic elements, phages, quorum sensing, toxin-antitoxin, Two- component system, antimicrobial resistance
Citation: Ramamurthy T, Ghosh A, Chowdhury G, Mukhopadhyay AK, Dutta S and Miyoshi S-i (2022) Deciphering the genetic network and programmed regulation of antimicrobial resistance in bacterial pathogens. Front. Cell. Infect. Microbiol. 12:952491. doi: 10.3389/fcimb.2022.952491
Received: 25 May 2022; Accepted: 25 October 2022;
Published: 23 November 2022.
Edited by:
Mona Mohamed Sobhy, Animal Production Research Institute (APRI), EgyptReviewed by:
Barbara Kędzierska, University of Gdansk, PolandLaura A. Mike, University of Toledo, United States
Sameh AbdelGhani, Beni-Suef University, Egypt
Copyright © 2022 Ramamurthy, Ghosh, Chowdhury, Mukhopadhyay, Dutta and Miyoshi. This is an open-access article distributed under the terms of the Creative Commons Attribution License (CC BY). The use, distribution or reproduction in other forums is permitted, provided the original author(s) and the copyright owner(s) are credited and that the original publication in this journal is cited, in accordance with accepted academic practice. No use, distribution or reproduction is permitted which does not comply with these terms.
*Correspondence: Thandavarayan Ramamurthy, cmFtYW11cnRoeS50QGljbXIuZ292Lmlu