- Department of Microbiology and Cell Biology, Montana State University, Bozeman, MT, United States
Toxoplasma gondii infects approximately one-third of the world’s population resulting in a chronic infection with the parasite located in cysts in neurons in the brain. In most immunocompetent hosts the chronic infection is asymptomatic, but several studies have found correlations between Toxoplasma seropositivity and neuropsychiatric disorders, including Schizophrenia, and some other neurological disorders. Host-parasite interactions of bradyzoites in cysts in neurons is not well understood due in part to the lack of suitable in vitro human neuronal models. The advent of stem cell technologies in which human neurons can be derived in vitro from human induced pluripotent stem cells (hiPSCs) or direct conversion of somatic cells generating induced neurons (iNs), affords the opportunity to develop in vitro human neuronal culture systems to advance the understanding of T. gondii in human neurons. Human neurons derived from hiPSCs or iNs, generate pure human neuron monolayers that express differentiated neuronal characteristics. hiPSCs also generate 3D neuronal models that better recapitulate the cytoarchitecture of the human brain. In this review, an overview of iPSC-derived neurons and iN protocols leading to 2D human neuron cultures and hiPSC-derived 3D cerebral organoids will be given. The potential applications of these 2D and 3D human neuronal models to address questions about host-parasite interactions of T. gondii in neurons and the parasite in the CNS, will be discussed. These human neuronal in vitro models hold the promise to advance the understanding of T. gondii in human neurons and to improve the understanding of neuropathogenesis of chronic toxoplasmosis.
1 Introduction
Toxoplasma gondii is an intracellular protozoan parasite with approximately one-third of the worlds’ population chronically infected. In chronically infected individuals, the parasite resides in the central nervous system (CNS) within neurons in cysts, that persist for the lifetime of the individual (Ferguson and Hutchison, 1987). In immunocompromised individuals, such as AIDS patients or individuals undergoing chemotherapy, the parasite can reactivate from the cyst stage and differentiate into the rapidly replicating tachyzoite stage causing a severe to potentially fatal encephalitis (Luft and Remington, 1992). The chronic infection in immunocompetent individuals is typically asymptomatic, though several studies have found correlations between Toxoplasma seropositivity and neuropsychiatric disorders such as Schizophrenia, prenatal depression, and suicidal thoughts and the chronic infection has also been associated with cryptogenic epilepsy and mild cognitive effects in elderly individuals, further indicating the chronic infection exerts effects on neuronal activity in the central nervous system (Yazar et al., 2003; Groer et al., 2011; Okusaga et al., 2011; Pedersen et al., 2012; Torrey et al., 2012; Beste et al., 2014; Sutterland et al., 2015; Xiao et al., 2018; Burgdorf et al., 2019; Oncu-Oner and Can, 2022). The mechanisms by which the parasite affects neuronal activity and CNS functions are not well understood.
Neurons are the predominant host cell for the bradyzoite stage and cysts in the chronic infection (Melzer et al., 2010; Cabral et al., 2016). Despite the central importance of bradyzoites and cysts in neurons in the chronic infection, these stages of the parasites’ life cycle, remain poorly understood. Studies on the effects of T. gondii infection in neurons is primarily derived from in vivo studies in mice. Early ultrastructural studies done in chronically infected mice found the synapse of infected neurons containing cysts remains intact, indicating parasite infection does not affect neuronal transmission (Sims et al., 1989). More recent in vivo studies in chronically infected mice however found neurons infected with the cyst stage are functionally silenced, have altered neurotransmitter levels and neural connectivity, and there is evidence of disruption of glutamate regulation by astrocytes and a loss of perisomatic inhibitory synapses, all indicating the parasite significantly alters neuronal functions (Prandovszky et al., 2011; Haroon et al., 2012; Brooks et al., 2015; David et al., 2016; Carrillo et al., 2020). Studies of Toxoplasma-infected neurons in vitro are limited to the study of the parasite in either primary neurons from rodents or human neuronal cell lines which do not display mature neuron characteristics (Sahm et al., 1997; Creuzet et al., 1998; Fagard et al., 1999; Schluter et al., 2001). Neither of these in vitro culture systems are adequate for study of effects of bradyzoites and cysts on human neuronal functions. A study in human neurons demonstrated neurons could support cyst development but was inadequate for long term culture needed for cyst maturation or the study of impact on neuronal functions (Halonen et al., 1996). Finally, the recent finding that bradyzoites and cysts are dynamic and heterogeneous entities in the chronic infection, as opposed to static structures as long thought, highlights the lack of understanding of these crucial stages and underscores the need for better in vitro neuronal models in which these stages of the parasites life cycle in human neurons can be studied (Watts et al., 2015).
A better understanding of the host-parasite interactions of T. gondii in human neurons and of the parasite effects on neuronal function is limited due to lack of suitable in vitro human neuronal models. The advent of cellular reprogramming technologies in which somatic cells can be used to derive functional human neurons in vitro, either via induced pluripotent stem cells (iPSCs) or via direct conversion of somatic cells to induced neurons (iNs), affords the opportunity to develop in vitro human neuronal culture systems to better understand host/parasite interactions of T. gondii in human neurons. Both hiPSC-derived neurons and iNs can generate two-dimensional (2D) neuronal monolayer cultures enabling cellular and molecular mechanistic studies to be done while hiPSC can also be used to derive three-dimensional (3D) cerebral organoids, affording disease modeling studies of neurological effects of T. gondii in the brain to be conducted. The use of stem cell technologies in Parkinson’s Disease (PD), Alzheimer’s Disease (AD), and Schizophrenia, has revolutionized the study and understanding of these neurological disorders (Brennand et al., 2011; Logan et al., 2019; Ferrari et al., 2020; Powell et al., 2020; D'Souza et al., 2021; Legault et al., 2022). These cellular reprogramming approaches have not been widely used in the study of T. gondii in the central nervous system (CNS) although they offer many of the same benefits such as providing an in vitro source of differentiated, mature, functional human neurons, enabling mechanistic studies, drug discovery and disease modeling studies, to be done.
Here, a review of iPSC-derived neuron and iN protocols leading to 2D neuronal monolayer cultures and hiPSC-derivation of 3D cerebral organoids models will be given with the goal of this review to summarize differentiation strategies of hiPSC-derived neurons, iNs, and 3D brain organoids, and to encourage the utilization of these in vitro-derived human neuronal models to address outstanding questions about host-parasite interactions of T. gondii in the human neuron host cell. A few Toxoplasma studies have used these approaches and a summary of the findings and potential applications of these 2D and 3D human neuronal models to address outstanding questions about the biology of T. gondii in neurons and neuropathogenesis of T. gondii chronic infection will be discussed. These cellular reprogramming approaches to generate human neuronal in vitro models hold the promise to advance the understanding of T. gondii interactions in human neurons and of neuropathogenesis of chronic toxoplasmosis.
2 Neurodifferentiation strategies
Human neurons are generated in vitro either from human induced pluripotent stem cells (hiPSCs) or via direct conversion producing iNs (Figure 1). Both approaches generate 2D monolayers of relatively pure populations of differentiated, functional neurons. In addition, 3D organoid cultures can be produced from hiPSCs. Here an overview of methods used in the generation of hiPSC-derived neurons vs. direct conversion of iNs will be given, with a comparison of advantages and disadvantages of each approach.
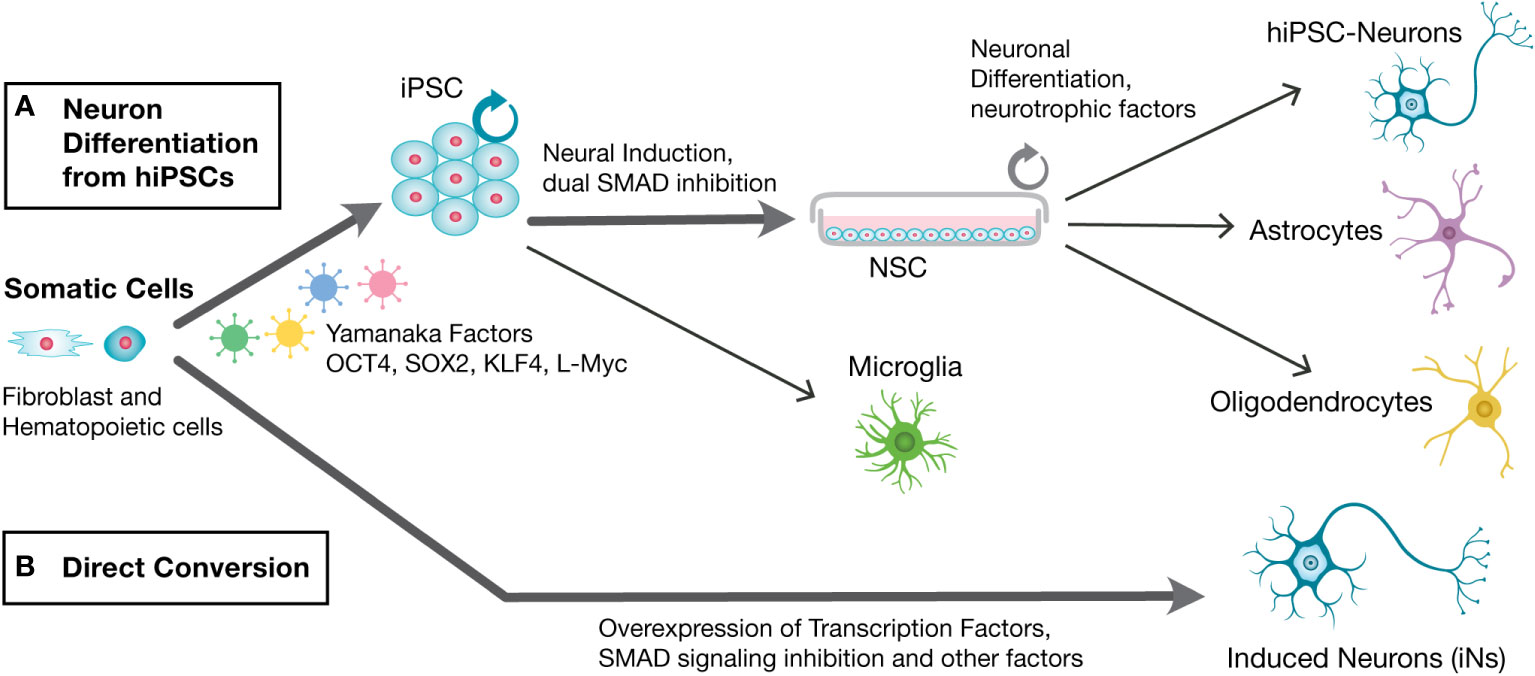
Figure 1 Schematic of steps involved in the generation of in vitro generation of human neurons from hiPSCs and direct conversion of induced neurons (iNs). Human primary fibroblasts or other somatic primary cells are used to generate human neurons in vitro via (A). Neuronal differentiation from hiPSCs or (B). Direct conversion into iNs. Neuronal differentiation from hiPSCs involves first reprogramming into hiPSCs via Yamanaka Factors, followed by neural induction into neural stem cells (NSCs) via dual SMAD inhibition, and differentiation with the aid of neurotrophic factors to generate hiPSC-neurons. hiPSCs-generated NSCs can also generate astrocytes and oligodendrocytes, while microglia can be generated from hiPSCs. Direct conversion into iNs involves overexpression of neuronal transcription factors, SMAD inhibition and other factors, to generate Induced Neurons (iNs).
2.1 hiPSC-derived neuronal cells
Human somatic cells exposed to Yamanaka factors, OCT4, KLKF4, SOX2 and c-MYC (OKSM), are reprogrammed into iPSCs which can be differentiated into different cell lineages, as first demonstrated in 2007 (Takahashi et al., 2007). In the years since the advent of iPSC technology refinements and improvements have occurred (Mertens et al., 2016; Traxler et al., 2019). Most differentiation protocols involve application of extrinsic factors to guide the differentiation process towards a cell fate, mimicking the regionalization processes that occur during the developmental process, followed by specific growth factors. In brief, hiPSC can be neutralized via dual SMAD signaling inhibition producing neural stem cell (NSCs), followed by addition of specific growth factors such as BDNF and GDNF and cAMP to induce differentiation into postmitotic neurons (Figure 1A) (Chambers et al., 2009; Shi et al., 2017). Differentiation into postmitotic neurons from NSCs typically takes 3-4 weeks. NSCs are multipotent and can be differentiated into astrocytes or oligodendrocytes with differentiation to these glial cell types typically requiring at least 60 days in in vitro culture (Hirbec et al., 2020). hiPSCs can be induced toward the mesodermal lineage and microglia generated, via specific growth factors, in about 15 days (Abud et al., 2017; Douvaras et al., 2017; Pandya et al., 2017).
2.2 Induced neurons
Human neurons can be generated in vitro through direct conversion of somatic cells into iNs (Figure 1B). Human fibroblasts can be converted into iNs via a combination of 3 transcription factors, BRN2, ACSL1, and MYTL1, the BAM reprogramming factors, in addition to the neurodifferentiation factor, NEUROD1 (Pang et al., 2011). It is now understood that during direct conversion, so called pioneering transcription factors bind closed chromatin structures and coordinate the binding of secondary transcription factors, to initiate a new cell fate (Mertens et al., 2016). Now, numerous conversion strategies have been developed involving the use of differing combinations of pioneering transcription factors, small molecules, and miRNAs (Schluter et al., 2001; Vierbuchen et al., 2010; Ladewig et al., 2012; Wapinski et al., 2013; Zhao et al., 2015). Most direct conversion protocols generate iNs from fibroblasts as the somatic cell, but diverse cells have been used for generating iNs, including blood cells, glial cells, pericytes and post-mortem brain tissues (Zhao et al., 2012; Rose et al., 2018; Traxler et al., 2019).
2.3 Generation of neuron subtypes from iNs and hiPSC-iNs
Subtype specific neurons, including glutaminergic, GABAergic, dopaminergic, and serotonergic neurons, can be generated either via from hiPSCs or iNs. Initial methods to derive neuronal subtypes from hiPSCs first created NSCs and then derived glutaminergic, GABAergic, and serotonergic neurons via mimicking developmental cues (Tao and Zhang, 2016). However, hiPSCs-NSC derived neuronal subtypes required long differentiation times leading to generation of heterogenous cell populations. More recently direct conversion of hiPSC to iNs (iPSC-iNs), called forward programming, has been found to generate highly pure populations of specific subtypes of neurons in an easy, reproducible manner (Flitsch et al., 2020; Canals et al., 2021). Forward programming is done via applying overexpression of lineage-specific transcription factors (TFs). This TF-based approach can generate dopaminergic neurons, glutaminergic neurons, GABAergic neurons and motor neurons from hiPSCs (Canals et al., 2021). Direct conversion of iNs similarly has been accomplished via the use of pioneering transcription factors in combination with factors shown to be important in neural subtype determination, with protocols to generate dopaminergic, GABAergic, and serotonergic neurons, now available (Vierbuchen et al., 2010; Caiazzo et al., 2011; Pfisterer et al., 2011; Victor et al., 2014; Mertens et al., 2015; Vadodaria et al., 2016; Xu et al., 2016; Mertens et al., 2018).
2.4 Cerebral organoids: 3D neuronal models
3D cerebral organoids contain multiple brain-specific cell types, obtain specialized function, and achieve an organ-like organization and overcome of the limitations of 2D neuronal monolayers (Liu et al., 2018; Logan et al., 2019; Pacitti et al., 2019). Cerebral organoids are derived from hiPSCs via both guided and unguided methods (Koo et al., 2019; Qian et al., 2019). Guided methods are dependent upon iPSCs intrinsic ability to assemble and differentiate towards the neuronal fate and yield cerebral organoids with the major features of the developing cortex, such as the outer subventricular zone and neural subtypes constituting all 6 cortical layers, formed (Koo et al., 2019; Sidhaye and Knoblich, 2021). In unguided methods, region-specific cerebral organoids are directed by region-specific growth factors, differentiation factors and specific cellular inhibitors. Protocols to generate region-specific cerebral organoids, including forebrain, midbrain, cerebellar, hippocampal, and hypothalamic organoids, are now established (Muguruma et al., 2015; Sakaguchi et al., 2015; Qian et al., 2016; Qian et al., 2018).
Cerebral organoids have a wider application for in vitro neurological disease modeling than 2D monolayers and have been successfully used in Alzheimer’s Disease, Parkinson’s Disease, and Schizophrenia (Choi et al., 2014; Tieng et al., 2014; Lee et al., 2016; Raja et al., 2016; Stachowiak et al., 2017; Amin and Pasca, 2018). Disadvantages of organoids include the need for more complex methods for characterization and analysis and the cost and the time to generate cerebral organoids.
2.5 hiPSC-neurons vs. iNs 2D neuronal models: Advantages and disadvantages
hiPSC-neurons and iNs are attractive in vitro 2D neuronal models for studying neuronal diseases because of their human origin, affording the ability to conduct mechanistic studies and screen for therapeutic targets, in human neurons. hiPSC-neurons and iNs have distinct advantages and disadvantages in terms of time to generate cells, capacity for expansion, number of cells that can be generated, epigenetic status and capability as models for neurological diseases (Mertens et al., 2016; Mertens et al., 2018; Traxler et al., 2019). The choice of the in vitro neuron culture method used, should be balanced with the research questions addressed and the practical experimental needs, to determine if these methods are worth the time and effort. Foremost amongst these considerations when thinking of using hiPSC or iN approach, are those of time and cost efficiency, expandability, epigenetic effects, and age-related phenotypes, each of which are briefly discussed below and summarized in Table 1.
2.5.1 Time and cost efficiency of hiPSCs vs. iNs
The generation of iPSC clones typically takes 2-3 months, but they can then be expanded indefinitely and provide an infinite supply of cells. Differentiation of iPSCs to neurons takes 6-15 weeks. NSCs can be established in about 2-3 weeks which can then be used as a stable intermediate cell line that can generate neurons in 3-4 weeks. Several iPSC and NSC cell lines are commercially available from ATCC (www.atcc.org), WiCell, (www.wicell.org), a variety of Schizophrenia-derived PSC cell lines are publicly available (Dobrindt et al., 2021) and several iPSC cell lines are available from NIH Center for Regenerative Medicine Program (https://commonfund.nih.gov/stemcells/lines). Conversely, iNs can be generated more quickly with direct conversion of fibroblasts to iNs generated in 1-3 weeks or with another 5-6 weeks required to generate fully mature neurons (Mertens et al., 2018; Traxler et al., 2019).
2.5.2 Expandability and cell numbers
As iPSCs can be expanded infinitely and NSCs are self-renewing, both iPSC and NSCs can generate large numbers of neurons and thus suitable for metabolomic, proteomic, transcriptomic, and genomic analyses. Conversely, direct iN conversion does not involve an expandable, intermediate stage and given the neurons generated are post mitotic, the direct conversion of iNs approach is limited in the numbers of neurons that can be produced.
2.5.3 Epigenetic and age-related effects
iPSC reprogramming involves chromatin remodeling that causes the epigenetic state of the cell to ‘reset’ into an embryonic-like state, with multiple rounds of cell division thought to select for and repair macromolecular damage, leaving iPSCs into what has been called a ‘rejuvenated cell’ (Mertens et al., 2018; Traxler et al., 2019). Conversely, iNs conserve the age-related epigenetic landscape and other cellular properties from the cell of origin and does not erase the cellular aging markers, with iNs derived from fibroblasts showing the transcriptomic and functional signatures of the age of the parent fibroblast (Mertens et al., 2015). The age-related epigenetic landscape of iNs may have advantages for disease modeling type studies where aged, patient-specific neurons in culture are desired.
3 hiPSC and iNs: new in vitro human neuronal models to study host/parasite relationship of T. gondii in neuron host cell and neuropathogenesis
These 2D and 3D in vitro human neuronal models open new possibilities for investigating T. gondii in human neurons and neuropathogenesis of T. gondii chronic infection. A few studies with T. gondii have been conducted using these in vitro human neuronal models which demonstrate their potential use in the study of T. gondii in neuronal cells. A brief description of each of these studies are given below, followed by a discussion of how these approaches could be applied to address outstanding questions of T. gondii in the brain, which is summarized in Table 2.
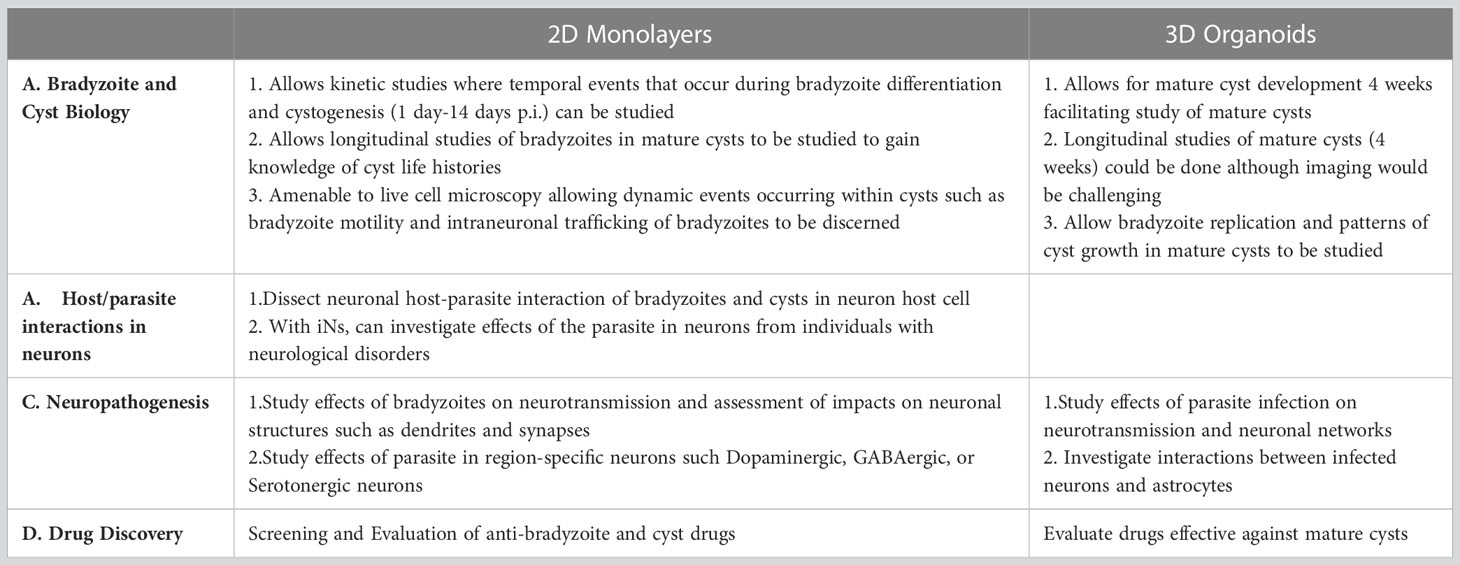
Table 2 Outstanding questions of T. gondii in neurons and in the CNS that could be addressed using 2D and 3D Human Neuronal Models.
3.1 Neuronal monolayers: 2D human neuronal models
3.1.1 Use of hiPSC-derived neurons
Human neurons were derived from NCRM-1 cells, a neural stem cell (NSC) line obtained from NIH, using a similar protocol as outlined in Figure 1A (Tanaka et al., 2016; Halonen, 2017). Human neurons were infected with T. gondii tachyzoites (type II strain) and at low parasite to host cell ratios (1:100), the majority of infected neurons supported spontaneous cystogenesis, with tachyzoite to bradyzoite stage conversion beginning within the first 12 hrs. post infection (p.i.) and mature cysts generated by 96h p.i. Mature cysts developed near the neuronal soma and in the dendritic processes and could be maintained in neurons for up to 14 days p.i. (Figure 2A).
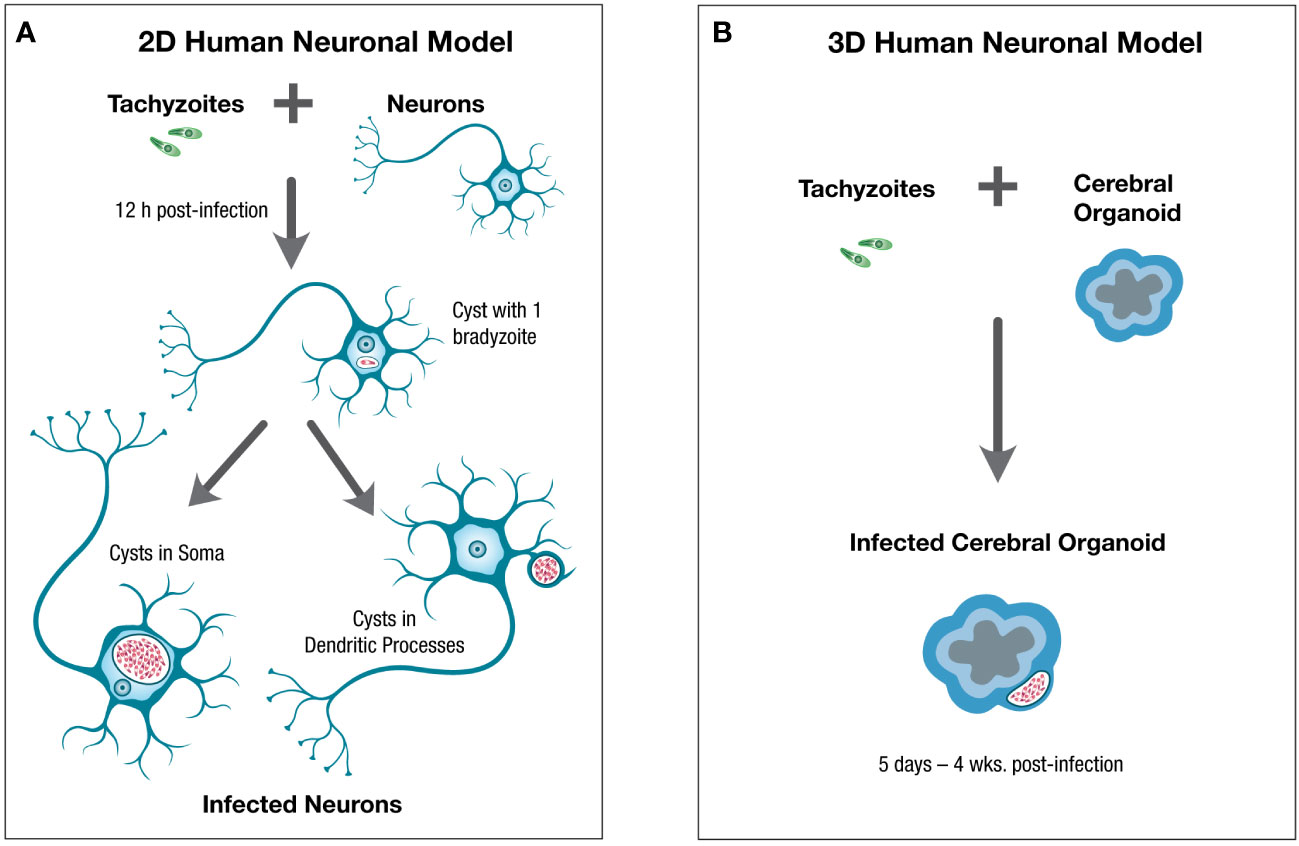
Figure 2 Schematic of 2D and 3D human neuronal in vitro models for the study of T. gondii in neurons and the central nervous system. Human neuronal in vitro models can be generated for the study of T. gondii in human neurons and the central nervous system using (A). 2D human neuronal monolayers or (B). 3D human neuronal models. For 2D neuronal models hiPSC-derived neurons can be infected with tachyzoites which convert to bradyzoites within 12hr post-infection (p.i.), leading to spontaneous cystogenesis with mature cysts located in the neuronal soma and dendritic processes by 4 days p.i. and which can be maintained for up to 14 days p.i. For 3D human neuronal models, cerebral organoids can be infected with tachyzoites which convert to bradyzoites and generate mature cysts by 5 days p.i. with cysts persisting in the outer layer of cerebral organoids, where mature neurons and some glial cells are found, for 4 weeks post-infection or longer. Tachyzoites are green, bradyzoites are red.
This study demonstrated that hiPSC-derived human neurons is an effective in vitro model to study spontaneous cystogenesis and host/parasite interactions during cystogenesis and of mature cysts, in the human neuron host cell. As this method generates differentiated neurons generated from NSCs, a self-renewing population, this method has the advantage of expandability, capable of generating large numbers of human neurons and thus conducive to transcriptomic, proteomic, and other such analyses.
3.1.2 Use of schizophrenia-derived iNs to study T. gondii in neurons
Peripheral fibroblasts were used to generate iNs from several patients with genetic variations linked to schizophrenia (SZ) and iNs from normal controls, and growth of T. gondii, RH strain, in disease vs. normal controls iNs, characterized (Passeri et al., 2016). Tachyzoites infected and generated cysts in both SZ-iNs and normal iNs, with some differences in growth found in the SZ-iNs vs. normal-iNs.
This iN study demonstrated mature human neurons can be generated from peripheral fibroblasts from patients with various brain disorders. The use of patient-derived iNs allows the heterogeneity of disease-associated phenotypes to be incorporated into investigation of molecular mechanisms underlying individual predisposition to Toxoplasma infection and various brain disorders.
3.2 Brain organoids: 3D human neuronal models
Two studies have investigated using 3D human neuronal models to study T. gondii infection in the brain (Seo et al., 2020; Correa Leite et al., 2021). In both 3D cerebral organoid models, infection with Toxoplasma tachyzoites, of both Me49 and RH strains, lead to bradyzoite differentiation and formation of mature cysts, with cysts persisting up to 4 weeks post-infection, within the cerebral organoids (Figure 2B).
Both studies demonstrated 3D organoid neuronal models can recapitulate the in vivo biology of T. gondii in the brain. Persistence of cysts for up to 4 weeks in these 3D models is a major advantage as these 3D models would allow studies on mature cysts to be conducted. Additionally, brain organoids contain multiple neural cell types, including neurons, neural progenitors, and glia, allowing dynamic interactions between neural cells to be studied and generation of neural cells with greater cell maturity and improved cell functionality (Liu et al., 2018; Pacitti et al., 2019). Infection of 3D organoid models also induced neural cell death, alteration in neural gene expression and triggering of the release of inflammatory markers in response to Toxoplasma infection, indicating these 3D neuronal organoids are also good models to address more complex interactions of the parasite in the brain.
3.3 Potential of 2D and 3D human neuronal models for T. gondii studies
3.3.1 Use of 2D neuron monolayers to study T. gondii host/parasite interactions
2D neuronal models derived from hiPSCs offer many advantages to study T. gondii in neurons, such as the ability to create monolayers of relatively pure neurons that exhibit differentiated neuronal morphology that are conducive to single-cell or population-based assays and afford opportunities to address questions about the biology of bradyzoites and cysts in neurons. A major advantage of this in vitro-derived human neuron culture is to allow neuron host-cell specific responses to Toxoplasma infection to be addressed. Several recent studies indicate neuron-specific responses are important. For example, the protein effector export of the bradyzoites stage was found to be slightly different in neurons vs. fibroblasts with indication the nuclear effector stability or effector export was less efficient in neurons (Mayoral et al., 2020). Additionally, a novel mechanism of IFN- induced bradyzoite formation and cyst conversion, via depletion of intracellular glutamine, was recently identified in human glutamatergic neurons (Bando et al., 2021). As it is known the molecular mechanism of IFN-response is different in mice and humans these in vitro-derived human neurons would also facilitate other studies investigating mechanisms of IFN-effects on T. gondii in human neurons (Ohshima et al., 2014; Saeij and Frickel, 2017; Bando et al., 2018). Other advantages of in vitro-derived human neurons are they form monolayers that are amenable to microscopical analysis and would allow host/parasite interactions of bradyzoites in cysts in neurons during encystment and of mature cysts, to be addressed. Additionally, this in vitro human neuron culture model facilitates cyst development up through 2 weeks and would facilitate longitudinal studies of individual cysts in neurons in which dynamic aspects of bradyzoites and cysts could be studied, and could yield new information and insights into life histories of cysts in neurons. Other advantages of 2D human neuronal monolayers are summarized in Table 2.
3.3.2 Use of 3D neuronal models to study T. gondii interactions in the CNS
Cerebral organoids would allow study of fully mature cysts as one of the limitations of the 2D human neuron monolayer for the study of T. gondii infection in neurons is the inability to maintain cysts for longer than 14 days post-infection, while 3D cerebral organoids allowed for cysts to persist for at least 4 weeks post-infection (Figure 2B). Thus, cerebral organoids may allow studies addressing behavior of bradyzoites in mature cysts, such as bradyzoite replication and patterns of cyst growth, to be studied. Cerebral organoids would also allow more complex questions of neuropathogenesis of T. gondii in the brain to be addressed such as the effects of infection on neuronal networks and the interactions between infected neurons and astrocytes. As neuron-astrocyte interactions have been shown to be involved in the impact of the parasite on neurotransmission, the ability to address the role of astrocytes on neuropathogenesis of T. gondii is an attractive aspect of 3D cerebral organoid models (David et al., 2016). Advantages of 3D cerebral organoids to address questions of T. gondii interactions in human CNS is summarized in Table 2.
3.3.3 Limitations of 2D and 3D human neuronal models
While 2D and 3D neuronal models have the potential to create in vitro human neuronal models to address outstanding questions of host-parasite interactions of T. gondii in neurons and neuronal tissues, there are distinct limitations of each model, especially as applies to T. gondii studies, which need to be considered, as stipulated below.
3.3.3.1 Conversion efficiency of 2D neuronal models
Low conversion efficiencies often occur with differentiation protocols for hiPSC-neurons and iNs. For hiPSC-human neurons, standardization of protocols and reagents have greatly improved protocol efficiencies and batch-to-batch consistency. Human iPSCs and NSCs for example are available from commercial sources such as ATCC and WiCell, with expansion and differentiation medias available and/or easy to make with the addition of defined supplements, making these protocols feasible for most Toxoplasma labs with established tissue culture. In evidence of this is the recent paper in which human iPSC-derived glutamatergic neurons were used to investigate molecular mechanisms of IFN- stimulated cyst formation in human neurons (Bando et al., 2021). iN protocols are not as well developed as hiPSCs, with iN protocols not standardized or reagents commercially available, and low conversion efficiencies and high variability in efficiency between reprogramming experiments, still a problem of most neural reprogramming protocols (D'Souza et al., 2021; Legault et al., 2022). Improved iN protocols are continually being developed and improved as for example, the recent induced dopaminergic neuron (iDANs) protocol which reports 90% conversion efficiency (Powell et al., 2021). These iDANs may provide a good in vitro model for midbrain dopaminergic neurons and addressing questions of association T. gondii and Schizophrenia. Thus, while iN protocols have improved in recent years, low conversion efficiencies are still common and need to be considered in context of the experimental question, if this method of generating in vitro human neurons is used.
3.3.3.2 Issues of complexity of 3D organoids
As 3D cerebral organoids are generated from iPSCs they have many of the same limitations of hiPSC-derived neuronal 2D monolayers including differentiation efficiency and batch-to-batch variability. However, as with hiPSCs, in recent years 3D organoid protocols have progressed such that there are now simple brain organoid protocols and multiple specific brain-region protocols, that can be done in a reproducible and predictable manner (Sidhaye and Knoblich, 2021). Limitations however still exist. For one, 3D organoids have mixed populations of cells which complicate studies using bulk RNASeq, as cell identities of transcripts cannot be identified and thus these types of studies with 3D organoids would require more complex methods for analysis. However single-cell transcriptomics and a variety of other assays including live-cell imaging, electrophysiology, calcium dynamics and immunohistochemistry studies can be done, albeit they require more effort (Sloan et al., 2018).
3.3.3.3 Lack of immune system components
Both 2D and 3D neuronal models lack an immune system component and thus do not fully recapitulate the in vivo environment of the CNS. Microglia, for example which play a crucial role in neuronal maturation and functioning and are implicated in neurodegeneration and psychiatric disorders such as Schizophrenia, are absent. Microglia can be generated from hiPSCs as diagrammed in Figure 1A, and some co-cultures of 3D hiPSC-organoids with hiPSC-derived microglia that infiltrate the organoid when added, have been used successfully in a few Alzheimer’s Disease studies (Abud et al., 2017; Song et al., 2019). As microglia have important roles in the biology of T. gondii in the brain, such as contributing to the loss of perisomatic inhibitory synapses following T. gondii infection (Carrillo et al., 2020), incorporation of microglia into 2D and 3D neurological models could be important for some studies. However, this would entail a complicated tissue culture process and the time and cost, needs to be carefully considered and balanced with the experimental question, in consideration of incorporation of microglia into these 2D and 3D human neuronal models.
4 Conclusions
The advent of stem cell technologies to generate human neuronal in vitro models has revolutionized the study of neurological disorders such as Parkinson’s Disease, Alzheimer’s Disease and Schizophrenia. The use of these human neuronal in vitro methods has a similar potential to advance the understanding of T. gondii in human neurons and of neuropathogenesis of the parasite in the CNS.
Author contributions
SH wrote all sections of the manuscript, assembled information for the tables and designed the Figures with the aid of Kristen Drumheller, a Graphic Designer from University Communications Dept. at Montana State University.
Acknowledgments
I gratefully acknowledge Kristen Drumheller for her expertise as a graphic designer and the University Communications Department at Montana State University for their technical help, in the preparation of the Figures for this project.
Conflict of interest
The author declares that the research was conducted in the absence of any commercial or financial relationships that could be construed as a potential conflict of interest.
Publisher’s note
All claims expressed in this article are solely those of the authors and do not necessarily represent those of their affiliated organizations, or those of the publisher, the editors and the reviewers. Any product that may be evaluated in this article, or claim that may be made by its manufacturer, is not guaranteed or endorsed by the publisher.
References
Abud, E. M., Ramirez, R. N., Martinez, E. S., Healy, L. M., Nguyen, C. H. H., Newman, S. A., et al. (2017). iPSC-derived human microglia-like cells to study neurological diseases. Neuron 94 (2), 278–93.e9. doi: 10.1016/j.neuron.2017.03.042
Amin, N. D., Pasca, S. P. (2018). Building models of brain disorders with three-dimensional organoids. Neuron 100 (2), 389–405. doi: 10.1016/j.neuron.2018.10.007
Bando, H., Fukuda, Y., Watanabe, N., Olawale, J. T., Kato, K. (2021). Depletion of intracellular glutamine pools triggers toxoplasma gondii stage conversion in human glutamatergic neurons. Front. Cell Infect. Microbiol. 11, 788303. doi: 10.3389/fcimb.2021.788303
Bando, H., Sakaguchi, N., Lee, Y., Pradipta, A., Ma, J. S., Tanaka, S., et al. (2018). Toxoplasma effector TgIST targets host IDO1 to antagonize the IFN-gamma-Induced anti-parasitic response in human cells. Front. Immunol. 9, 2073. doi: 10.3389/fimmu.2018.02073
Beste, C., Getzmann, S., Gajewski, P. D., Golka, K., Falkenstein, M. (2014). Latent toxoplasma gondii infection leads to deficits in goal-directed behavior in healthy elderly. Neurobiol. Aging. 35 (5), 1037–1044. doi: 10.1016/j.neurobiolaging.2013.11.012
Brennand, K. J., Simone, A., Jou, J., Gelboin-Burkhart, C., Tran, N., Sangar, S., et al. (2011). Modelling schizophrenia using human induced pluripotent stem cells. Nature 473 (7346), 221–225. doi: 10.1038/nature09915
Brooks, J. M., Carrillo, G. L., Su, J., Lindsay, D. S., Fox, M. A., Blader, I. J. (2015). Toxoplasma gondii infections alter GABAergic synapses and signaling in the central nervous system. mBio 6 (6), e01428–e01415. doi: 10.1128/mBio.01428-15
Burgdorf, K. S., Trabjerg, B. B., Pedersen, M. G., Nissen, J., Banasik, K., Pedersen, O. B., et al. (2019). Large-Scale study of toxoplasma and cytomegalovirus shows an association between infection and serious psychiatric disorders. Brain Behav. Immun. 79, 152–158. doi: 10.1016/j.bbi.2019.01.026
Cabral, C. M., Tuladhar, S., Dietrich, H. K., Nguyen, E., MacDonald, W. R., Trivedi, T., et al. (2016). Neurons are the primary target cell for the brain-tropic intracellular parasite toxoplasma gondii. PLoS Pathog. 12 (2), e1005447. doi: 10.1371/journal.ppat.1005447
Caiazzo, M., Dell'Anno, M. T., Dvoretskova, E., Lazarevic, D., Taverna, S., Leo, D., et al. (2011). Direct generation of functional dopaminergic neurons from mouse and human fibroblasts. Nature 476 (7359), 224–227. doi: 10.1038/nature10284
Canals, I., Quist, E., Ahlenius, H. (2021). Transcription factor-based strategies to generate neural cell types from human pluripotent stem cells. Cell Reprogram. 23 (4), 206–220. doi: 10.1089/cell.2021.0045
Carrillo, G. L., Ballard, V. A., Glausen, T., Boone, Z., Teamer, J., Hinkson, C. L., et al. (2020). Toxoplasma infection induces microglia-neuron contact and the loss of perisomatic inhibitory synapses. Glia 68 (10), 1968–1986. doi: 10.1002/glia.23816
Chambers, S. M., Fasano, C. A., Papapetrou, E. P., Tomishima, M., Sadelain, M., Studer, L. (2009). Highly efficient neural conversion of human ES and iPS cells by dual inhibition of SMAD signaling. Nat. Biotechnol. 27 (3), 275–280. doi: 10.1038/nbt.1529
Choi, S. H., Kim, Y. H., Hebisch, M., Sliwinski, C., Lee, S., D'Avanzo, C., et al. (2014). A three-dimensional human neural cell culture model of alzheimer's disease. Nature 515 (7526), 274–278. doi: 10.1038/nature13800
Correa Leite, P. E., de Araujo Portes, J., Pereira, M. R., Russo, F. B., Martins-Duarte, E. S., Almeida Dos Santos, N., et al. (2021). Morphological and biochemical repercussions of toxoplasma gondii infection in a 3D human brain neurospheres model. Brain Behav. Immun. Health 11, 100190. doi: 10.1016/j.bbih.2020.100190
Creuzet, C., Robert, F., Roisin, M. P., Van Tan, H., Benes, C., Dupouy-Camet, J., et al. (1998). Neurons in primary culture are less efficiently infected by toxoplasma gondii than glial cells. Parasitol. Res. 84 (1), 25–30. doi: 10.1007/s004360050351
D'Souza, G. X., Rose, S. E., Knupp, A., Nicholson, D. A., Keene, C. D., Young, J. E. (2021). The application of in vitro-derived human neurons in neurodegenerative disease modeling. J. Neurosci. Res. 99 (1), 124–140. doi: 10.1002/jnr.24615
David, C. N., Frias, E. S., Szu, J. I., Vieira, P. A., Hubbard, J. A., Lovelace, J., et al. (2016). GLT-1-Dependent disruption of CNS glutamate homeostasis and neuronal function by the protozoan parasite toxoplasma gondii. PLoS Pathog. 12 (6), e1005643. doi: 10.1371/journal.ppat.1005643
Dobrindt, K., Zhang, H., Das, D., Abdollahi, S., Prorok, T., Ghosh, S., et al. (2021). Publicly available hiPSC lines with extreme polygenic risk scores for modeling schizophrenia. Complex Psychiatry 6 (3-4), 68–82. doi: 10.1159/000512716
Douvaras, P., Sun, B., Wang, M., Kruglikov, I., Lallos, G., Zimmer, M., et al. (2017). Directed differentiation of human pluripotent stem cells to microglia. Stem Cell Rep. 8 (6), 1516–1524. doi: 10.1016/j.stemcr.2017.04.023
Fagard, R., Van Tan, H., Creuzet, C., Pelloux, H. (1999). Differential development of toxoplasma gondii in neural cells. Parasitol. Today 15 (12), 504–507. doi: 10.1016/S0169-4758(99)01568-9
Ferguson, D. J., Hutchison, W. M. (1987). An ultrastructural study of the early development and tissue cyst formation of toxoplasma gondii in the brains of mice. Parasitol. Res. 73 (6), 483–491. doi: 10.1007/BF00535321
Ferrari, E., Cardinale, A., Picconi, B., Gardoni, F. (2020). From cell lines to pluripotent stem cells for modelling parkinson's disease. J. Neurosci. Methods 340, 108741. doi: 10.1016/j.jneumeth.2020.108741
Flitsch, L. J., Laupman, K. E., Brustle, O. (2020). Transcription factor-based fate specification and forward programming for neural regeneration. Front. Cell Neurosci. 14, 121. doi: 10.3389/fncel.2020.00121
Groer, M. W., Yolken, R. H., Xiao, J. C., Beckstead, J. W., Fuchs, D., Mohapatra, S. S., et al. (2011). Prenatal depression and anxiety in toxoplasma gondii-positive women. Am. J. Obstet Gynecol. 204 (5), 433 e1–433 e7. doi: 10.1016/j.ajog.2011.01.004
Halonen, S. K. (2017). Use of human neurons derived via cellular reprogramming methods to study host-parasite interactions of toxoplasma gondii in neurons. Cells 6 (4), 1–14. doi: 10.3390/cells6040032
Halonen, S. K., Lyman, W. D., Chiu, F. C. (1996). Growth and development of toxoplasma gondii in human neurons and astrocytes. J. Neuropathol. Exp. Neurol. 55 (11), 1150–1156. doi: 10.1097/00005072-199611000-00006
Haroon, F., Handel, U., Angenstein, F., Goldschmidt, J., Kreutzmann, P., Lison, H., et al. (2012). Toxoplasma gondii actively inhibits neuronal function in chronically infected mice. PLoS One 7 (4), e35516. doi: 10.1371/journal.pone.0035516
Hirbec, H., Deglon, N., Foo, L. C., Goshen, I., Grutzendler, J., Hangen, E., et al. (2020). Emerging technologies to study glial cells. Glia 68 (9), 1692–1728. doi: 10.1002/glia.23780
Koo, B., Choi, B., Park, H., Yoon, K. J. (2019). Past, present, and future of brain organoid technology. Mol. Cells 42 (9), 617–627. doi: 10.14348/molcells.2019.0162
Ladewig, J., Mertens, J., Kesavan, J., Doerr, J., Poppe, D., Glaue, F., et al. (2012). Small molecules enable highly efficient neuronal conversion of human fibroblasts. Nat. Methods 9 (6), 575–578. doi: 10.1038/nmeth.1972
Lee, H. K., Velazquez Sanchez, C., Chen, M., Morin, P. J., Wells, J. M., Hanlon, E. B., et al. (2016). Three dimensional human neuro-spheroid model of alzheimer's disease based on differentiated induced pluripotent stem cells. PLoS One 11 (9), e0163072. doi: 10.1371/journal.pone.0163072
Legault, E. M., Bouquety, J., Drouin-Ouellet, J. (2022). Disease modeling of neurodegenerative disorders using direct neural reprogramming. Cell Reprogram. 24 (5), 228–251. doi: 10.1089/cell.2021.0172
Liu, C., Oikonomopoulos, A., Sayed, N., Wu, J. C. (2018). Modeling human diseases with induced pluripotent stem cells: from 2D to 3D and beyond. Development 145 (5), 1–6. doi: 10.1242/dev.156166
Logan, S., Arzua, T., Canfield, S. G., Seminary, E. R., Sison, S. L., Ebert, A. D., et al. (2019). Studying human neurological disorders using induced pluripotent stem cells: From 2D monolayer to 3D organoid and blood brain barrier models. Compr. Physiol. 9 (2), 565–611. doi: 10.1002/cphy.c180025
Luft, B. J., Remington, J. S. (1992). Toxoplasmic encephalitis in AIDS. Clin. Infect. Dis. an Off. Publ. Infect. Dis. Soc. America. 15 (2), 211–222. doi: 10.1093/clinids/15.2.211
Mayoral, J., Shamamian, P., Jr., Weiss, L. M. (2020). In vitro characterization of protein effector export in the bradyzoite stage of toxoplasma gondii. mBio 11 (2), 1–17. doi: 10.1128/mBio.00046-20
Melzer, T. C., Cranston, H. J., Weiss, L. M., Halonen, S. K. (2010). Host cell preference of toxoplasma gondii cysts in murine brain: A confocal study. J. Neuroparasitol. 1, 1–12. doi: 10.4303/jnp/N100505
Mertens, J., Marchetto, M. C., Bardy, C., Gage, F. H. (2016). Evaluating cell reprogramming, differentiation and conversion technologies in neuroscience. Nat. Rev. Neurosci. 17 (7), 424–437. doi: 10.1038/nrn.2016.46
Mertens, J., Paquola, A. C. M., Ku, M., Hatch, E., Bohnke, L., Ladjevardi, S., et al. (2015). Directly reprogrammed human neurons retain aging-associated transcriptomic signatures and reveal age-related nucleocytoplasmic defects. Cell Stem Cell. 17 (6), 705–718. doi: 10.1016/j.stem.2015.09.001
Mertens, J., Reid, D., Lau, S., Kim, Y., Gage, F. H. (2018). Aging in a dish: iPSC-derived and directly induced neurons for studying brain aging and age-related neurodegenerative diseases. Annu. Rev. Genet. 52, 271–293. doi: 10.1146/annurev-genet-120417-031534
Muguruma, K., Nishiyama, A., Kawakami, H., Hashimoto, K., Sasai, Y. (2015). Self-organization of polarized cerebellar tissue in 3D culture of human pluripotent stem cells. Cell Rep. 10 (4), 537–550. doi: 10.1016/j.celrep.2014.12.051
Ohshima, J., Lee, Y., Sasai, M., Saitoh, T., Su Ma, J., Kamiyama, N., et al. (2014). Role of mouse and human autophagy proteins in IFN-gamma-induced cell-autonomous responses against toxoplasma gondii. J. Immunol. 192 (7), 3328–3335. doi: 10.4049/jimmunol.1302822
Okusaga, O., Langenberg, P., Sleemi, A., Vaswani, D., Giegling, I., Hartmann, A. M., et al. (2011). Toxoplasma gondii antibody titers and history of suicide attempts in patients with schizophrenia. Schizophr. Res. 133 (1-3), 150–155. doi: 10.1016/j.schres.2011.08.006
Oncu-Oner, T., Can, S. (2022). Meta-analysis of the relationship between toxoplasma gondii and schizophrenia. Ann. Parasitol. 68 (1), 103–110. doi: 10.17420/ap6801.414
Pacitti, D., Privolizzi, R., Bax, B. E. (2019). Organs to cells and cells to organoids: The evolution of in vitro central nervous system modelling. Front. Cell Neurosci. 13, 129. doi: 10.3389/fncel.2019.00129
Pandya, H., Shen, M. J., Ichikawa, D. M., Sedlock, A. B., Choi, Y., Johnson, K. R., et al. (2017). Differentiation of human and murine induced pluripotent stem cells to microglia-like cells. Nat. Neurosci. 20 (5), 753–759. doi: 10.1038/nn.4534
Pang, Z. P., Yang, N., Vierbuchen, T., Ostermeier, A., Fuentes, D. R., Yang, T. Q., et al. (2011). Induction of human neuronal cells by defined transcription factors. Nature 476 (7359), 220–223. doi: 10.1038/nature10202
Passeri, E., Jones-Brando, L., Bordon, C., Sengupta, S., Wilson, A. M., Primerano, A., et al. (2016). Infection and characterization of toxoplasma gondii in human induced neurons from patients with brain disorders and healthy controls. Microbes Infect. 18 (2), 153–158. doi: 10.1016/j.micinf.2015.09.023
Pedersen, M. G., Mortensen, P. B., Norgaard-Pedersen, B., Postolache, T. T. (2012). Toxoplasma gondii infection and self-directed violence in mothers. Arch. Gen. Psychiatry 69 (11), 1123–1130. doi: 10.1001/archgenpsychiatry.2012.668
Pfisterer, U., Kirkeby, A., Torper, O., Wood, J., Nelander, J., Dufour, A., et al. (2011). Direct conversion of human fibroblasts to dopaminergic neurons. Proc. Natl. Acad. Sci. U.S.A. 108 (25), 10343–10348. doi: 10.1073/pnas.1105135108
Powell, S. K., O'Shea, C. P., Shannon, S. R., Akbarian, S., Brennand, K. J. (2020). Investigation of schizophrenia with human induced pluripotent stem cells. Adv. Neurobiol. 25, 155–206. doi: 10.1007/978-3-030-45493-7_6
Powell, S. K., O'Shea, C., Townsley, K., Prytkova, I., Dobrindt, K., Elahi, R., et al. (2021). Induction of dopaminergic neurons for neuronal subtype-specific modeling of psychiatric disease risk. Mol. Psychiatry, 1–12 doi: 10.1038/s41380-021-01273-0
Prandovszky, E., Gaskell, E., Martin, H., Dubey, J. P., Webster, J. P., McConkey, G. A. (2011). The neurotropic parasite toxoplasma gondii increases dopamine metabolism. PloS One 6 (9), e23866. doi: 10.1371/journal.pone.0023866
Qian, X., Jacob, F., Song, M. M., Nguyen, H. N., Song, H., Ming, G. L. (2018). Generation of human brain region-specific organoids using a miniaturized spinning bioreactor. Nat. Protoc. 13 (3), 565–580. doi: 10.1038/nprot.2017.152
Qian, X., Nguyen, H. N., Song, M. M., Hadiono, C., Ogden, S. C., Hammack, C., et al. (2016). Brain-Region-Specific organoids using mini-bioreactors for modeling ZIKV exposure. Cell 165 (5), 1238–1254. doi: 10.1016/j.cell.2016.04.032
Qian, X., Song, H., Ming, G. L. (2019). Brain organoids: advances, applications and challenges. Development 146 (8), 1–12. doi: 10.1242/dev.166074
Raja, W. K., Mungenast, A. E., Lin, Y. T., Ko, T., Abdurrob, F., Seo, J., et al. (2016). Self-organizing 3D human neural tissue derived from induced pluripotent stem cells recapitulate alzheimer's disease phenotypes. PLoS One 11 (9), e0161969. doi: 10.1371/journal.pone.0161969
Rose, S. E., Frankowski, H., Knupp, A., Berry, B. J., Martinez, R., Dinh, S. Q., et al. (2018). Leptomeninges-derived induced pluripotent stem cells and directly converted neurons from autopsy cases with varying neuropathologic backgrounds. J. Neuropathol. Exp. Neurol. 77 (5), 353–360. doi: 10.1093/jnen/nly013
Saeij, J. P., Frickel, E. M. (2017). Exposing toxoplasma gondii hiding inside the vacuole: a role for GBPs, autophagy and host cell death. Curr. Opin. Microbiol. 40, 72–80. doi: 10.1016/j.mib.2017.10.021
Sahm, M., Fischer, H. G., Gross, U., Reiter-Owona, I., Seitz, H. M. (1997). Cyst formation by toxoplasma gondii in vivo and in brain-cell culture: a comparative morphology and immunocytochemistry study. Parasitol. Res. 83 (7), 659–665. doi: 10.1007/s004360050315
Sakaguchi, H., Kadoshima, T., Soen, M., Narii, N., Ishida, Y., Ohgushi, M., et al. (2015). Generation of functional hippocampal neurons from self-organizing human embryonic stem cell-derived dorsomedial telencephalic tissue. Nat. Commun. 6, 8896. doi: 10.1038/ncomms9896
Schluter, D., Deckert, M., Hof, H., Frei, K. (2001). Toxoplasma gondii infection of neurons induces neuronal cytokine and chemokine production, but gamma interferon- and tumor necrosis factor-stimulated neurons fail to inhibit the invasion and growth of T. gondii. Infect. Immun. 69 (12), 7889–7893. doi: 10.1128/IAI.69.12.7889-7893.2001
Seo, H. H., Han, H. W., Lee, S. E., Hong, S. H., Cho, S. H., Kim, S. C., et al. (2020). Modelling toxoplasma gondii infection in human cerebral organoids. Emerg. Microbes Infect. 9 (1), 1943–1954. doi: 10.1080/22221751.2020.1812435
Shi, Y., Inoue, H., Wu, J. C., Yamanaka, S. (2017). Induced pluripotent stem cell technology: a decade of progress. Nat. Rev. Drug Discovery 16 (2), 115–130. doi: 10.1038/nrd.2016.245
Sidhaye, J., Knoblich, J. A. (2021). Brain organoids: an ensemble of bioassays to investigate human neurodevelopment and disease. Cell Death Differ. 28 (1), 52–67. doi: 10.1038/s41418-020-0566-4
Sims, T. A., Hay, J., Talbot, I. C. (1989). An electron microscope and immunohistochemical study of the intracellular location of toxoplasma tissue cysts within the brains of mice with congenital toxoplasmosis. Br. J. Exp. Pathol. 70 (3), 317–325.
Sloan, S. A., Andersen, J., Pasca, A. M., Birey, F., Pasca, S. P. (2018). Generation and assembly of human brain region-specific three-dimensional cultures. Nat. Protoc. 13 (9), 2062–2085. doi: 10.1038/s41596-018-0032-7
Song, L., Yuan, X., Jones, Z., Vied, C., Miao, Y., Marzano, M., et al. (2019). Functionalization of brain region-specific spheroids with isogenic microglia-like cells. Sci. Rep. 9 (1), 11055. doi: 10.1038/s41598-019-47444-6
Stachowiak, E. K., Benson, C. A., Narla, S. T., Dimitri, A., Chuye, L. E. B., Dhiman, S., et al. (2017). Cerebral organoids reveal early cortical maldevelopment in schizophrenia-computational anatomy and genomics, role of FGFR1. Transl. Psychiatry 7 (11), 6. doi: 10.1038/s41398-017-0054-x
Sutterland, A. L., Fond, G., Kuin, A., Koeter, M. W., Lutter, R., van Gool, T., et al. (2015). Beyond the association. toxoplasma gondii in schizophrenia, bipolar disorder, and addiction: systematic review and meta-analysis. Acta Psychiatr. Scand. 132 (3), 161–179. doi: 10.1111/acps.12423
Takahashi, K., Tanabe, K., Ohnuki, M., Narita, M., Ichisaka, T., Tomoda, K., et al. (2007). Induction of pluripotent stem cells from adult human fibroblasts by defined factors. Cell 131 (5), 861–872. doi: 10.1016/j.cell.2007.11.019
Tanaka, N., Ashour, D., Dratz, E., Halonen, S. (2016). Use of human induced pluripotent stem cell-derived neurons as a model for cerebral toxoplasmosis. Microbes Infect. 18 (7-8), 496–504. doi: 10.1016/j.micinf.2016.03.012
Tao, Y., Zhang, S. C. (2016). Neural subtype specification from human pluripotent stem cells. Cell Stem Cell. 19 (5), 573–586. doi: 10.1016/j.stem.2016.10.015
Tieng, V., Stoppini, L., Villy, S., Fathi, M., Dubois-Dauphin, M., Krause, K. H. (2014). Engineering of midbrain organoids containing long-lived dopaminergic neurons. Stem Cells Dev. 23 (13), 1535–1547. doi: 10.1089/scd.2013.0442
Torrey, E. F., Bartko, J. J., Yolken, R. H. (2012). Toxoplasma gondii and other risk factors for schizophrenia: an update. Schizophr. Bull. 38 (3), 642–647. doi: 10.1093/schbul/sbs043
Traxler, L., Edenhofer, F., Mertens, J. (2019). Next-generation disease modeling with direct conversion: a new path to old neurons. FEBS Lett. 593 (23), 3316–3337. doi: 10.1002/1873-3468.13678
Vadodaria, K. C., Marchetto, M. C., Mertens, J., Gage, F. H. (2016). Generating human serotonergic neurons in vitro: Methodological advances. Bioessays 38 (11), 1123–1129. doi: 10.1002/bies.201600127
Victor, M. B., Richner, M., Hermanstyne, T. O., Ransdell, J. L., Sobieski, C., Deng, P. Y., et al. (2014). Generation of human striatal neurons by microRNA-dependent direct conversion of fibroblasts. Neuron 84 (2), 311–323. doi: 10.1016/j.neuron.2014.10.016
Vierbuchen, T., Ostermeier, A., Pang, Z. P., Kokubu, Y., Sudhof, T. C., Wernig, M. (2010). Direct conversion of fibroblasts to functional neurons by defined factors. Nature 463 (7284), 1035–1041. doi: 10.1038/nature08797
Wapinski, O. L., Vierbuchen, T., Qu, K., Lee, Q. Y., Chanda, S., Fuentes, D. R., et al. (2013). Hierarchical mechanisms for direct reprogramming of fibroblasts to neurons. Cell 155 (3), 621–635. doi: 10.1016/j.cell.2013.09.028
Watts, E., Zhao, Y., Dhara, A., Eller, B., Patwardhan, A., Sinai, A. P. (2015). Novel approaches reveal that toxoplasma gondii bradyzoites within tissue cysts are dynamic and replicating entities in vivo. mBio 6 (5), e01155–e01115. doi: 10.1128/mBio.01155-15
Xiao, J., Prandovszky, E., Kannan, G., Pletnikov, M. V., Dickerson, F., Severance, E. G., et al. (2018). Toxoplasma gondii: Biological parameters of the connection to schizophrenia. Schizophr. Bull. 44 (5), 983–992. doi: 10.1093/schbul/sby082
Xu, Z., Jiang, H., Zhong, P., Yan, Z., Chen, S., Feng, J. (2016). Direct conversion of human fibroblasts to induced serotonergic neurons. Mol. Psychiatry 21 (1), 62–70. doi: 10.1038/mp.2015.101
Yazar, S., Arman, F., Yalcin, S., Demirtas, F., Yaman, O., Sahin, I. (2003). Investigation of probable relationship between toxoplasma gondii and cryptogenic epilepsy. Seizure 12 (2), 107–109. doi: 10.1016/S1059-1311(02)00256-X
Zhao, J., He, H., Zhou, K., Ren, Y., Shi, Z., Wu, Z., et al. (2012). Neuronal transcription factors induce conversion of human glioma cells to neurons and inhibit tumorigenesis. PLoS One 7 (7), e41506. doi: 10.1371/journal.pone.0041506
Keywords: human neurons, human iPSCs, spontaneous cystogenesis, bradyzoites, cerebral organoids
Citation: Halonen SK (2023) Use of in vitro derived human neuronal models to study host-parasite interactions of Toxoplasma gondii in neurons and neuropathogenesis of chronic toxoplasmosis. Front. Cell. Infect. Microbiol. 13:1129451. doi: 10.3389/fcimb.2023.1129451
Received: 22 December 2022; Accepted: 14 February 2023;
Published: 08 March 2023.
Edited by:
Emma Harriet Wilson, University of California, Riverside, United StatesReviewed by:
Anita Koshy, University of Arizona, United StatesCopyright © 2023 Halonen. This is an open-access article distributed under the terms of the Creative Commons Attribution License (CC BY). The use, distribution or reproduction in other forums is permitted, provided the original author(s) and the copyright owner(s) are credited and that the original publication in this journal is cited, in accordance with accepted academic practice. No use, distribution or reproduction is permitted which does not comply with these terms.
*Correspondence: Sandra K. Halonen, c2hhbG9uZW5AbW9udGFuYS5lZHU=