- 1Molecular Parasitology Unit, Medical Technology Department, University of Antofagasta, Antofagasta, Chile
- 2Centro de Salud El Salvador, El Salvador, Chile
- 3Departamento de Fisiologia, Facultad de Ciencias Biológicas, Universidad de Concepción, Concepción, Chile
- 4Department of Biochemistry & Molecular Biology, Michael Smith Laboratories, University of British Columbia, Vancouver, BC, Canada
- 5Laboratório de Biologia Molecular e Sistêmica de Tripanossomatídeos (Labtryp), Instituto Carlos Chagas Fiocruz (ICC-Fiocruz), Curitiba, Paraná, Brazil
- 6Millennium Institute of Immunology and Immunotherapy, Facultad de Ciencias Biológicas, Pontificia Universidad Católica de Chile, Santiago, Chile
- 7Departamento de Endocrinología, Facultad de Medicina, Pontificia Universidad Católica de Chile, Santiago, Chile
- 8Research Center in Immunology and Biomedical Biotechnology of Antofagasta, Universidad de Antofagasta, Antofagasta, Chile
Introduction: Oral transmission of T. cruzi is probably the most frequent transmission mechanism in wild animals. This observation led to the hypothesis that consuming raw or undercooked meat from animals infected with T. cruzi may be responsible for transmitting the infection. Therefore, the general objective of this study was to investigate host-pathogen interactions between the parasite and gastric mucosa and the role of meat consumption from infected animals in the oral transmission of T. cruzi.
Methods: Cell infectivity assays were performed on AGS cells in the presence or absence of mucin, and the roles of pepsin and acidic pH were determined. Moreover, groups of five female Balb/c mice were fed with muscle tissue obtained from mice in the acute phase of infection by the clone H510 C8C3hvir of T. cruzi, and the infection of the fed mice was monitored by a parasitemia curve. Similarly, we assessed the infective capacity of T. cruzi trypomastigotes and amastigotes by infecting groups of five mice Balb/c females, which were infected orally using a nasogastric probe, and the infection was monitored by a parasitemia curve. Finally, different trypomastigote and amastigote inoculums were used to determine their infective capacities. Adhesion assays of T. cruzi proteins to AGS stomach cells were performed, and the adhered proteins were detected by western blotting using monoclonal or polyclonal antibodies and by LC-MS/MS and bioinformatics analysis.
Results: Trypomastigote migration in the presence of mucin was reduced by approximately 30%, whereas in the presence of mucin and pepsin at pH 3.5, only a small proportion of parasites were able to migrate (∼6%). Similarly, the ability of TCTs to infect AGS cells in the presence of mucin is reduced by approximately 20%. In all cases, 60–100% of the animals were fed meat from mice infected in the acute phase or infected with trypomastigotes or amastigotes developed high parasitemia, and 80% died around day 40 post-infection. The adhesion assay showed that cruzipain is a molecule of trypomastigotes and amastigotes that binds to AGS cells. LC-MS/MS and bioinformatics analysis, also confirmed that transialidase, cysteine proteinases, and gp63 may be involved in TCTs attachment or invasion of human stomach cells because they can potentially interact with different proteins in the human stomach mucosa. In addition, several human gastric mucins have cysteine protease cleavage sites.
Discussion: Then, under our experimental conditions, consuming meat from infected animals in the acute phase allows the T. cruzi infection. Similarly, trypomastigotes and amastigotes could infect mice when administered orally, whereas cysteinyl proteinases and trans-sialidase appear to be relevant molecules in this infective process.
Introduction
Chagas disease, caused by the protozoan Trypanosoma cruzi, affects 6–7 million people worldwide, with an annual incidence of 28 thousand cases in the Americas (PAHO/WHO, 2022). Human infections occur via fecal feces released by contaminated insect vectors belonging to the Reduviidae family, blood transfusion, vertical transmission, organ transplantation, laboratory accidents, and oral infections (Schmunis and Yadon, 2010; Sánchez and Ramírez, 2013). As a result of various initiatives aimed at controlling vector transmission and eradicating household vectors, the incidence of Chagas disease has been reduced in recent years (Coura and Vĩas, 2010; Rassi et al., 2010; Coura, 2015). Similarly, the massive implementation of serological screening techniques in blood banks in endemic regions has significantly reduced post-transfusion transmission of Chagas disease (Rassi et al., 2010; de Arias et al., 2022). However, in recent decades, oral transmission as a result of the ingestion of contaminated food and beverages as a result of liquefaction of whole triatomines or contamination with feces from infected triatomines has begun to occupy a relevant place in the mechanisms of transmission of Chagas disease (Coura, 2006; Coura, 2015; de Noya and González, 2015). Currently, oral transmission of T. cruzi is the most significant transmission route in Brazil (70–80% of cases) (Coura, 2006). In addition, some outbreaks have been described in several South American countries, such as Venezuela, Colombia, Bolivia, Argentina, French Guyana and Ecuador (Coura et al., 1997; Benchimol Barbosa, 2006; Dias et al., 2008; De Noya et al., 2010; Shikanai-Yasuda and Carvalho, 2012; Ramírez et al., 2013; Sánchez and Ramírez, 2013). These outbreaks were associated with consuming contaminated food and beverages such as wild animal meat, vegetables, sugar cane extract, açaí pulp, guava juice, bacaba, babaçu, and palma wine (Ferreira et al., 2001; Pereira et al., 2009; Barbosa-Ferreira et al., 2010). Furthermore, from 1968 to 2000, 50% of acute cases in the Amazon region were attributed to oral transmission (Shikanai-Yasuda and Carvalho, 2012; Bruneto et al., 2021), and these numbers later reached 70% between 2000 and 2010 (Shikanai-Yasuda and Carvalho, 2012). Venezuela has also reported the most significant outbreak described to date, with two distinct occurrences affecting 103 and 88 people, respectively. These outbreaks affect adults and children from urban and rural schools (De Noya et al., 2010; Sánchez and Ramírez, 2013). The mortality rate of orally infected patients is higher (0–44%) than that of classical vectorial transmission through triatomine fecal contamination after biting (<5–10%) (López-García and Gilabert, 2023). Oral transmission is currently the most frequent form of transmission (Coura, 2015).
Many observations have suggested that carnivorism may play a central role in T. cruzi transmission, especially in wildlife, when predators devorate their prey (Coura et al., 2002; Coura, 2006). However, this hypothesis has not been experimentally demonstrated.
In this study, we demonstrate that consuming raw meat transmits the oral infection by T. cruzi, and both trypomastigote and amastigote stages are infective for mice when administered by a nasogastric tube. Additionally, cysteinyl proteinases (among them, cruzipain) and trans-sialidase (TS) appear to be involved in parasite adhesion or invasion of gastric cells since both molecules are associated with AGS stomach cells.
Material and methods
Parasite cell lines and cell culture
The virulent T. cruzi cell line, C8C3hvir, and low-virulence C8C3lvir were obtained from clone H510 C8C3, as previously described (San Francisco et al., 2017). Vero cells were infected with tissue culture-derived trypomastigotes (TCTs) as described previously (Araya et al., 2008). Five days later, the supernatants containing more than 95% TCT were collected by centrifugation.
Trypomastigote migration assay using gastric mucin layer
These tests were performed as previously described (Cortez et al., 2012). Briefly, polycabonate transwell filters (3 μm pores, diameter) were coated with 50 μl of a solution of gastric mucin dissolved in Dulbecco’s Modified Eagle’s Medium (DMEM) (10 mg/mL) and the migration of TCT (1x107/well) was assayed over a period of 1 h.
Trypomastigote treatment with pepsin
Trypomastigotes were treatment or not with pepsin as previously described (Cortez et al., 2012). Briefly, TCTs, were incubated for 30 minutes in a solution of pepsin (2 mg/mL) dissolved in DMEM medium adjusted to pH 3.5. At the end of the incubation period, the number of trypomastigotes was determined by using a Neubauer chamber. In another set of experiments, trypomastigotes treated or not with pepsin for 30 min were lysed with PBS plus 0.1% NP-40 containing cOmplete™ Mini EDTA-free Protease Inhibitor Cocktail (Thermo Fisher Scientific, Rockford, IL, USA) and subjected to sodium dodecyl sulfate-polyacrylamide gel electrophoresis. ImageJ v2.0 software was used to perform densitometric analysis of the gels,. To obtain consistent results, each gel was repeated at least three times.
In vitro invasion assay in the presence or absence of mucin
AGS cells (ATCC CRL-1739), a cell line isolated from human gastric adenocarcinoma from stomach tissue, were seeded at 5 × 104 cells/well in 4-well Lab-Tek Chamber Slides (NUNC, Thermo Fisher Scientific, Roskilde, Denmark). The TCT invasion assay was performed according to a previously reported method using a C8C3hvir and C8C3lvir at a parasite:cell ratio equal to 10:1 (Araya et al., 2008). Parasites were then placed in contact with the cells in the absence or presence of 500 μg mL-1 of gastric mucin from the porcine stomach (type III; Sigma), as previously reported (Neira et al., 2003). The cells were incubated for 3 h at 37°C. The cells were washed three times with phosphate-buffered saline (PBS), fixed with methanol, and stained with 4′,6-diamidino-2-fenilindol (DAPI). The number of parasites in 500 cells was determined using a BX51 fluorescence microscope (Olympus Corporation, Tokyo, Japan). Images of T. cruzi-infected cells were obtained by confocal immunofluorescence microscopy (Leica TCS SP8 microscope, Leica Microsystems GmbH, Wetzlar, Alemania).
In vivo infection and parasitemia curve
Four- to six-week-old female BALB/c mice were infected with TCTs from C8C3hvir cell line through intraperitoneal injection (1×105 parasites per mouse). Parasitemia was monitored every two days starting on day 2 post-inoculation, as previously described (Brener, 1962), and experiments were stopped on day 20, near peak parasitemia. The animals were euthanized, and the muscles were dissected and used to feed female mice, as previously described (Rani et al., 2019). In these experiments, each animal was fed infected mouse meat and placed in an individual cage to control the experiment and avoid competition for the food source, thus guaranteeing total intake of the infected meat. The mice that ingested the meat of the infected animals had the same characteristics, and in the last 24 h, they received water ad libitum with solid food deprivation. Parasitemia was evaluated from the second day onwards, as described above.
For oral infection, four to six-week-old female Balb/c mice were infected with T. cruzi TCTs or amastigotes via the oral route (1 × 105 parasites per mouse) using a nasopharyngeal probe adapted to a 1 ml syringe. Starting on day 5 post-inoculation, parasitemia was monitored every two days, twice a week, by examining 5 µL blood samples collected from the tail and observed under a phase contrast microscope (Staquicini et al., 2010). In all cases, gastric mucosal infection was monitored by qPCR, where total genomic DNA was extracted from stomach of the infected mice using the Wizard Genomic DNA Purification Kit (Promega) according to the manufacturer’s instructions. DNA concentration was quantified using an Infinite 200 PRO UV spectrophotometer (Tecan, Männedorf, Switzerland) and adjusted to 25 ng/μL as previously described (San Francisco et al., 2022).
To assess the potential role of cysteinyl proteinases in parasite-host interactions during the oral route, TCTs were treated with (2S,3S)-trans-Epoxysuccinyl-L-leucylamido-3-methylbutane ethyl ester (E64d) as previously described (San Francisco et al., 2017). Briefly, TCTs were incubated for 45 min at room temperature with 50 µM of the cell-permeable cysteinyl proteinases inhibitor, E64d. At the end of this incubation period, parasite viability was assessed by motility and membrane integrity was evaluated by propidium iodide staining. Subsequently, the TCTs were washed and used for oral infections using a nasopharyngeal probe.
All experiments were conducted according to the Institutional Ethical Committee for Animal Experimentation regulations, and all protocols were approved by them (CEIC REV/2013).
SDS-PAGE and immunoblots
TCTs or amastigotes from the C8C3hvir T. cruzi cell line were lysed by sonication (PBS 1X containing cOmplete™, Mini, EDTA-free Protease Inhibitor Cocktail) (Thermo Fisher, Rockford, IL, USA), and protein concentration was determined (Bradford, 1976). Then, soluble extracts of TCT or amastigotes were incubated with AGS cell cultures at 4°C for 4 h. Finally, the cell culture medium was removed, and the cultures were washed three times with PBS. The cultures were lysed (NP-40 1%, PBS 1X containing cOmplete™, Mini, EDTA-free Protease Inhibitor Cocktail) (Thermo Fisher, Rockford IL, USA) and the soluble extract was analyzed by SDS-PAGE on a 12% acrylamide gel and electrotransferred to nitrocellulose membranes in a Trans-Blot Turbo (Bio-Rad Hercules CA, USA). Immunoreactivity was determined by incubation with a polyclonal antibody against cruzipain (Czp) (either prepared by us (JG) or provided by Dr. Juan José Cazzulo of the Universidad Nacional de San Martin, Buenos Aires, Argentina); mouse anti-recombinant complement regulatory protein (CRP) antibody (provided by Dr. Karen Norris of Department of Infectious Diseases in the College of Veterinary Medicine, University of Georgia, Athens, USA), and monoclonal antibody 39 against trans-sialidase (TS) (provided by Dr. Sergio Schenkman of the Federal University of Sao Paulo, Brazil), monoclonal antibody 2C2 against Ssp4 amastigote antigen (amastin) (Andrews et al., 1987). Anti-rabbit or anti-mouse IgG peroxidase-labeled antibodies were used as secondary antibodies. Immunoblots were developed using enhanced chemiluminescence assay (ECL), (Thermo Fisher, Rockford, IL, USA). Using the Gel-Capture software, ECL Images were captured in a ChemiDoc MP Imaging System (BioRad, Hercules, CA, USA).
Membrane shaving and in-gel protein digestion
AGS cells were incubated with soluble extracts of T. cruzi TCT from the C8C3hvir cell line for 4h at 4°C as described above. The cultures were washed three times with PBS, and the cells were trypsinized. The cells and trypsinized material were collected and centrifuged at 1800 rpm, and the supernatant containing the peptides obtained by shaving was concentrated using a Speed Vac (Rees et al., 2015). In-gel protein digestion was performed as described by San Francisco et al. (2022).
Reverse phase-liquid chromatography-tandem mass spectrometry (RP- LC-MS/MS) analysis
Peptide concentrations were measured on NanoDrop One (Thermo Fisher - A205, scopes) to load approximately 50 ng of peptides on TimsTOF Pro2 (Bruker Daltonics) with CaptiveSpray source coupled to nanoElute UHPLC (Bruker Daltonics) using Bruker’s PepSep Ten column (10 cm x 75 µm inner diameter, 1.9 µm particle size with 20 µm emitter) heated to 40°C. Buffer A consisted of 0.1% formic acid and 0.5% acetonitrile in water, and buffer B consisted of 0.1% formic acid and 99.4% acetonitrile in water. A standard 30 min gradient was used, increasing buffer B percentages from 2% to 12% over 15 min and then to 33% over 15 min. The column was washed with 95% B over 8 min. The NanoElute thermostat temperature was set at 7°C. The analysis was performed at a 0.4 μL/min flow rate.
The TimsTOF Pro2 was set to DIA-PASEF mode with positive polarity for the MS scan window from 100 to 1700 m/z. The capillary voltage was set to 1800V, drying gas to 3L/min, and drying temperature to 180°C. The MS1 scan was followed by PASEF ramps containing 22 non-overlapping 35 m/z isolation windows, covering the mass range 319.5 – 1089.5 Da. Ion mobility range (1/K0) was set to 0.70 – 1.35 V·s/cm2, with 100 ms ramp time, 100 ms accumulation time, 100% duty cycle, and 9.42 Hz ramp rate. The collision energy was ramped linearly as a mobility function from 27eV at 1/K0 = 0.70 V·s/cm2 to 55eV at 1/K0 = 1.35 V·s/cm2.
The acquired data were searched with DIA-NN (PMID31768060) version 1.8.1 with open access library search method, using UniProt’s human-reviewed protein sequences, three Trypanosoma cruzi reference proteomes (UP000002296, UP000246078, UP000246121), and manually curated common contaminants (226 entries). Other search parameters include Trypsin/P digestion mode with 1 missed cleavage, 1 maximum number of variable modification, N-terminal M excision, carbamidomethylation of C, and oxidation of M options enabled, peptide length ranged 7-30, precursor charge ranged 1-4, precursor m/z ranged 300-1800, and fragment ion m/z ranged 200-1800. Precursor False Discovery Rate (FDR) was set to 1%, with 0 Mass accuracy and MS1 accuracy (for the “auto” option of mass tolerance), enabling heuristic protein inference, using isotopologues, match between run (MBR), and no shared spectra. Protein inference is set as “Protein name from FASTA”, Double-pass mode for neural network classifier, Robust LC (high precision) for Quantification Strategy, RT-dependent mode for Cross-run normalization, and Smart profiling mode for Library generation.
The mass spectrometry proteomics data have been deposited to the ProteomeXchange Consortium via the PRIDE (PubMed ID: 34723319) partner repository with the dataset identifier PXD044267.
Protein-protein interactions analysis
Protein-protein interactions (PPIs) were mapped and visualized using the Search Tool for the Retrieval of Interacting Genes/Proteins (STRING) (Doncheva et al., 2023) in Cytoscape v.3.10 (Shannon et al., 2003) using StringApp 2.0 (Doncheva et al., 2023) to retrieve the cross-species network. First, a full-string network was generated with the PPIs of Homo sapiens and T. cruzi with a confidence score cutoff of ≥ 0.4. The PPIs involving membrane-related T. cruzi proteins and human proteins identified by LC-MS/MS in this study and their neighbors up to one level of interaction were mapped into the networks. The maps were drawn using the Cytoscape software. Next, we analyzed gene–tissue associations of human proteins mapped in the interaction networks to identify their scores in the stomach tissue. For this purpose, TISSUE 2.0.database (Palasca et al., 2018) integrated into Cytoscape StringApp was used, and the proteins were ranked according to the expression score in that tissue.
Prediction of cysteine protease cleavage sites
The cysteine protease cleavage sites were predicted using GPS-CCD 1.0 tool (Liu et al., 2011) with a high cut-off value (≥ 0.654). The top 10 scored cleavage sites are shown for each mucin mapped in the interaction analysis using DOG 2.0 (Ren et al., 2009). These domains were identified using the conserved domain database (CDD-NCBI) (Wang et al., 2023) and the specific hits added to the DOG 2.0. Next, the sequence window (a total of 10 amino acids) of the predicted cleavage sites were analyzed to map the amino acid motifs upstream to the cleavage site. The kpLogo tool was used, and the single amino acid motifs calculated as the most significant were visualized as k-mer logos.
Statistical analyses
Statistical analysis was performed using Prism 5 software (GraphPad Software) using Student’s t-test and analysis of variance (ANOVA). The significance of the T. cruzi experiments, in which differences in infectivity or cell invasion between the C8C3hvir and C8C3lvir cell lines were compared. Statistical significance was set at p<0.01, which was considered significant.
Results
The ability of TCTs to traverse a mucin layer was evaluated in microtiter plates with transwell filters coated with gastric mucin, by counting the number of TCTs traslocated through the mucin layer after 30 minutes incubation. As shown in Figure 1A, when migration experiments in the presence or absence of mucin were performed, the migration of TCTs was reduced by approximately 30% in the presence of mucin (Figure 1A). An even more significant reduction in migration was observed in migration experiments in the presence of mucin and pepsin at pH 3.5. In these cases, only a small number of parasites (∼ 6%) had migrated (Figure 1A). To examine the susceptibility of TCTs to pepsin, they were incubated for 30 min in DMEM medium pH 3.5 containing pepsin (2 mg/mL-1). TCTs were highly sensitive to pepsin lysis, and only 25.5% of C8C3hvir parasites survived (Figure 1B). To gain preliminary insights into the effect of pepsin on the surface proteins of TCTs, an SDS-PAGE gel was developed. As shown in Figure 1C, expression of at least four bands between 40 and 90 kDa was reduced after pepsin treatment, suggesting that they could probably be digested by pepsin. These differences were also visualized in the densitometric analysis, where statistically significant differences were observed in the intensity of the bands in the pepsin-treated parasite run with respect to the controls that did not receive this treatment (Figure 1D).
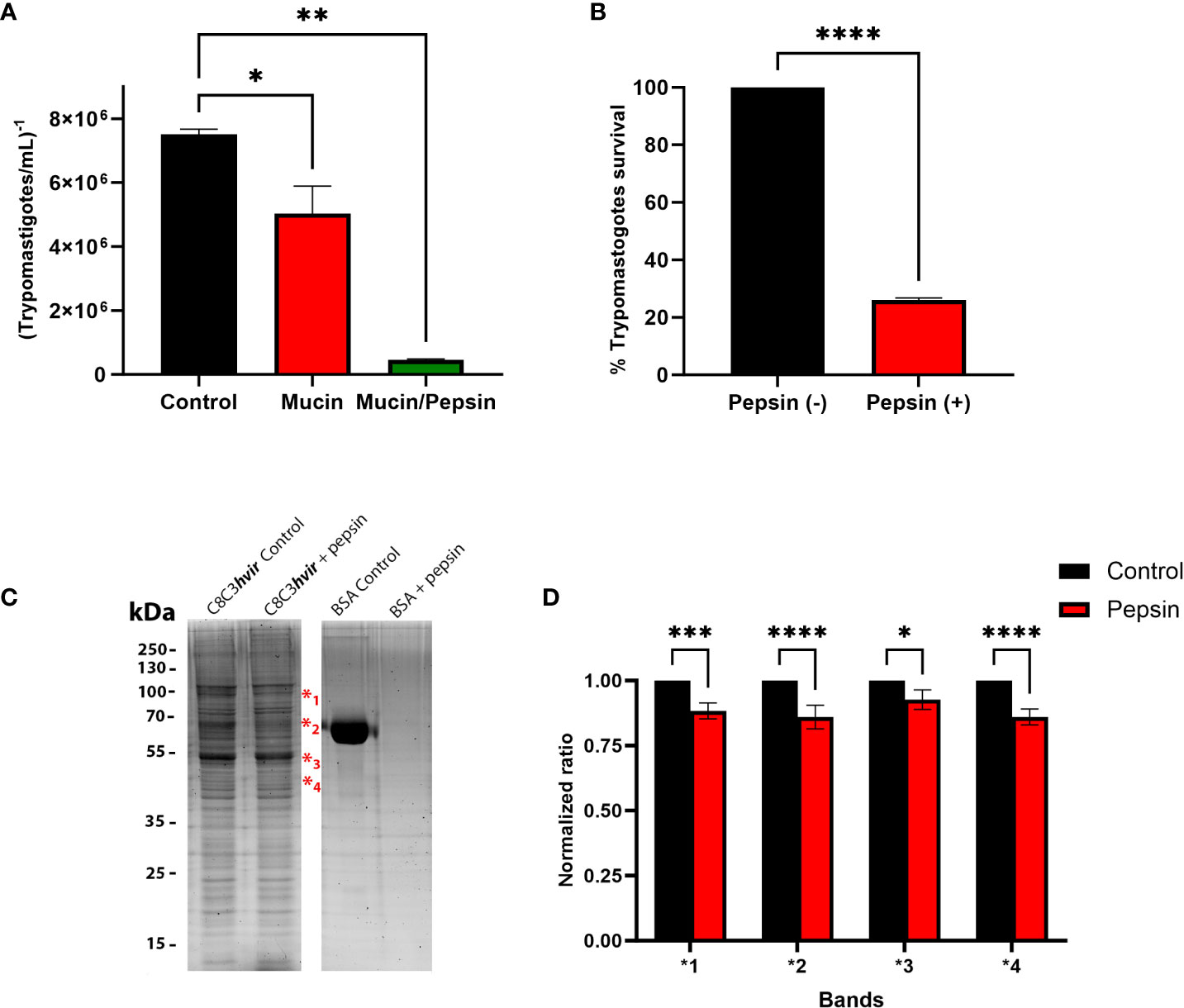
Figure 1 Evaluation of Trypanosoma cruzi trypomastigote migration through gastric mucin. (A) Gastric mucin was deposited on transwell chamber filters. After 30 min, a suspension of tryponastigotes (1x107) was added and incubated for 1 h in the presence or absence of pepsin at pH 3.5. The number of trypomastigotes that migrated after 1 h of incubation was determined using the Neubauer chamber count and compared with controls, in which gastric mucin was absent. (B) Trypomastigotes were treated or untreated with pepsin for 30 min and at the end of the incubation period were counted in a Neubauer chamber and the percentage of survival was determined. (C) Trypomastigotes treated or untreated with pepsin for 30 min were lysed and subjected to 12% sodium dodecyl sulfate-polyacrylamide gel electrophoresis. The gels were stained with Sypro Ruby. (D) Densitometric analysis of gel were performed using imageJ 2.0v software, where each band that showed a decrease in intensity after treatment of the parasites with pepsin was compared with the homologous band of the untreated control. Bars are represented as the mean ± SEM of the least three independent experiments. ***P < 0.0002 (band 1); *P < 0.0127; ****P < 0.0001 (band 2 and 4) vs corresponding control; Two way anova. ** p=0.0016.
Next, we evaluated the capacity of TCTs to invade AGS human stomach cells in the presence or absence of gastric mucin, in an attempt to mimic the in vivo situation in which trypomastigotes interact with and traverse the mucous layer before coming into contact with stomach epithelial cells. An invasion assay using two T. cruzi cell lines (high virulence and low virulence) showed that both T. cruzi, C8C3hvir and C8C3lvir could invade AGS cells. However, the C8C3hvir cell line was at least three times more invasive than C8C3lvir. Therefore, it was selected for experimental studies in mice. In the experiments in which mucin was present, a decrease of approximately 20% in the invasiveness of both cell lines was observed, as reflected by the lower number of intracellular parasites after 3 h of incubation compared to that observed in the absence of mucin (Figure 2A). As shown in Figure 2B, AGS cells were highly infective for TCTs of the virulent cell line (C8C3hvir), while the low virulence cell line (C8C3lvir) was poorly infective against these cells (Figures 2B, C).
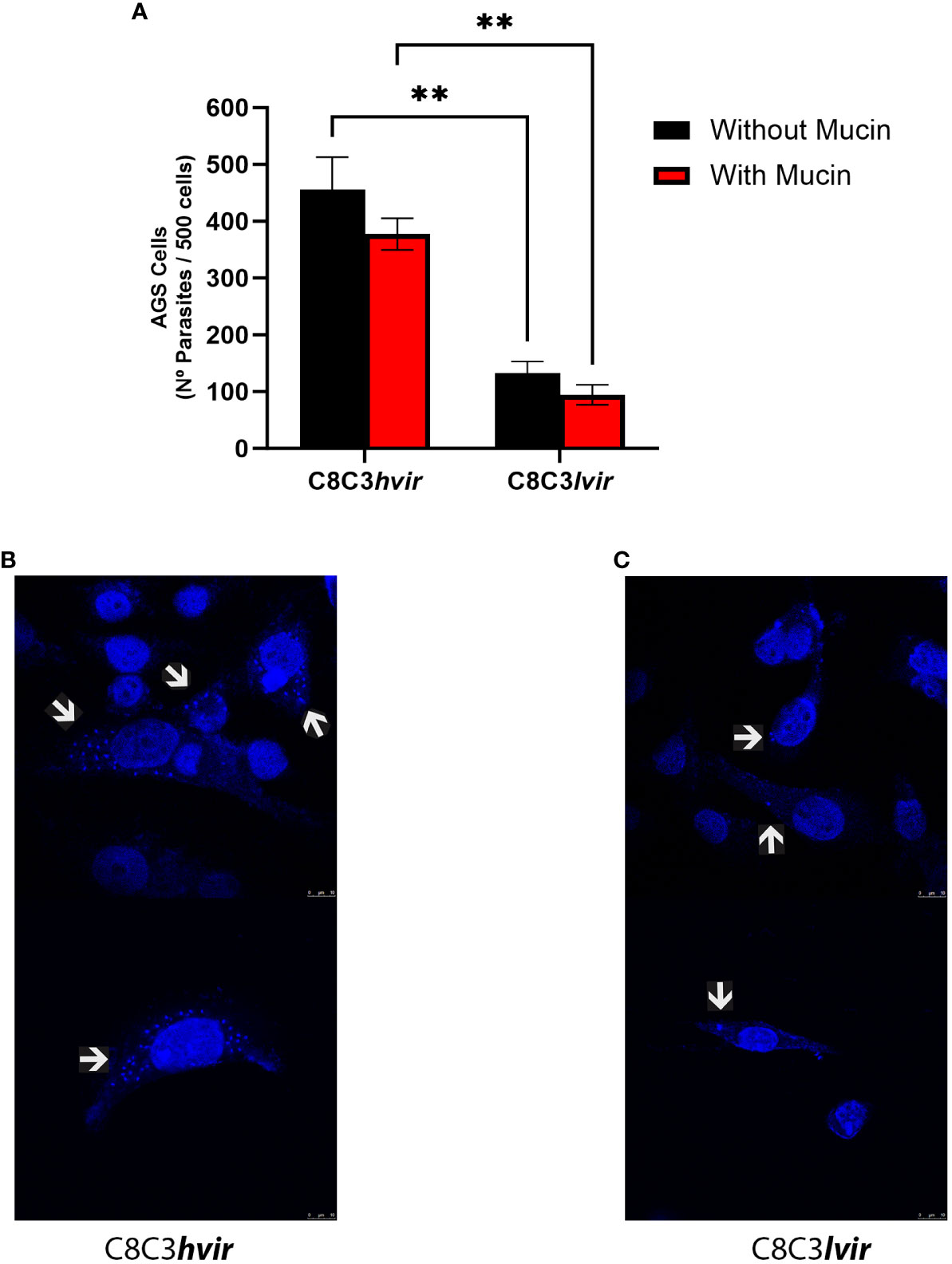
Figure 2 In vitro infectivity of Trypanosoma cruzi C8C3hvir and C8C3lvir cell lines. (A) AGS cells were infected with trypomastigotes from either C8C3hvir or C8C3lvir cell lines, in presence or absence of mucin, using a parasite/cell ratio 10:1. After 3h, cultures were washed three times with phosphate-buffered saline and stained with 4′,6-diamidino-2-fenilindol. Results are expressed as the mean ± SD of parasites/500 cells from three experiments performed in triplicate. Representative images of the infectivity of the C8C3hvir (B) and C8C3lvir (C) T.cruzi cell lines for AGS cells were acquired using a Leica TCS SP8 confocal fluorescence microscope with a 700 Hz gain and a DMOD direct intensity modulated solid-state violet laser. Without mucin ** p=0.0066; With mucin ** p=0.0087.
To evaluate the possibility of T. cruzi transmission by carnivorism, we initially infected mice intraperitoneally, which were sacrificed in the acute phase at the peak of parasitemia (Figure 3A). The sacrificed animals were dissected, and raw meat was given as food to uninfected mice that had been starved for two days. Oral infection with T. cruzi in animals was monitored using parasitemia curve analysis. As shown in Figure 3B, three of the five mice (60%) were infected with T. cruzi and died due to the infection.
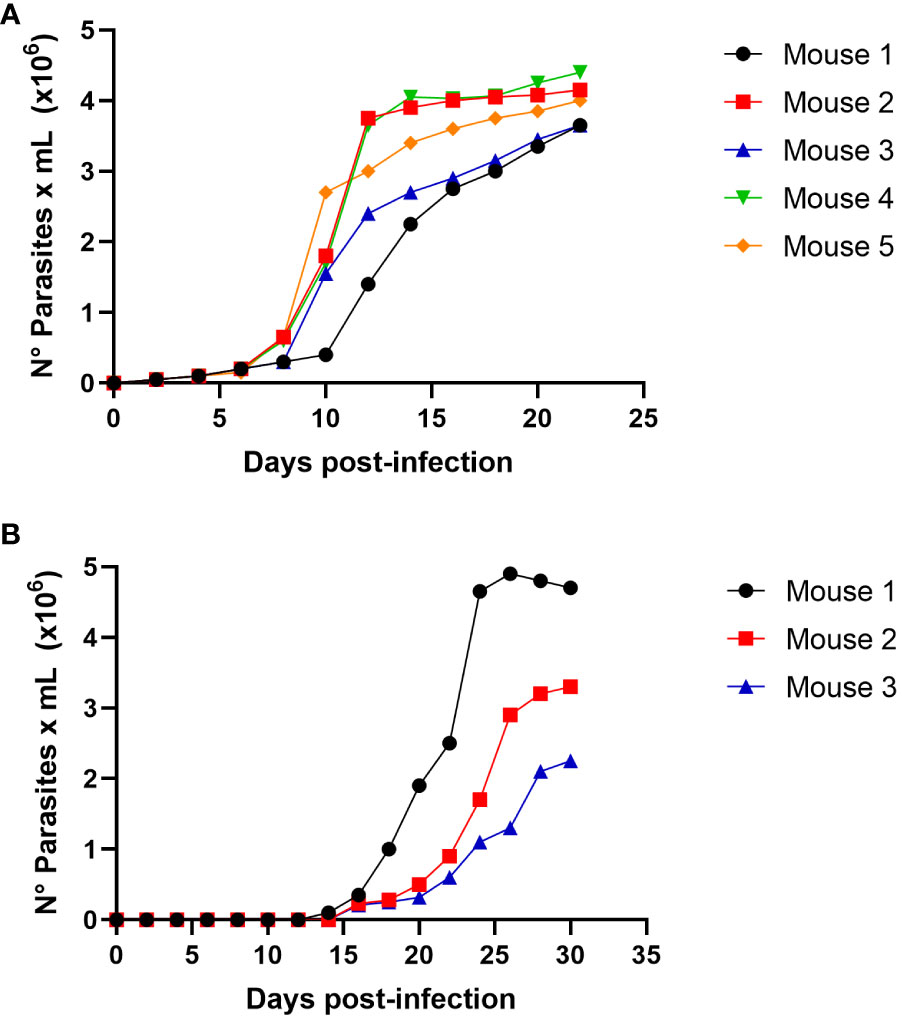
Figure 3 Trypanosoma cruzi oral infection by mice carnivorism (A) Groups of 5 female Balb/c mice were infected intraperitoneally with 1x105 trypomastigotes derived from cell culture of T.cruzi C8C3hvir cell line. Parasitemia curve was determined by counting (every 2 days) the number of parasites in a blood sample obtained through a small cut in the tail of the mouse. (B) Groups of 5 female Balb/c mice were fed with mice muscles obtained at the mice acute infection phase, as shown in Figure (A). The appearance and evolution of parasitemia was monitored as described in (A). Results are expressed as mean ± 1 SD and represent at least 3 experiments performed with the same protocols.
We determined whether the infection produced by raw meat from the infected mice resulted from trypomastigotes or intracellular amastigotes. Then, experimental infections were performed using a nasogastric probe and different parasite stages and inoculums. When a group of mice was experimentally infected with different amastigotes inocula using a nasogastric probe, all animals were infected (Figure 4). One hundred % of mice were infected with an inoculum of 3 × 105 cells (Figure 4B), whereas 80% were infected with an inoculum of 2 × 105 cells (Figure 4C). Moreover, 60% of the mice were infected with the inoculum of 1x105 (Figure 4D). Also, as shown in Figure 5A, trypomastigotes were infectives when inoculums of 4× 105 cells were used, resulting in four of five infected mice (80%). When inoculums of 1×105 were used, 4 of 5 mice inoculated (80%) were also infected (Figure 5B). It is well known t It is well known that the route of parasite administration is critical for the course of infection, with different courses of parasitemia and mortality. Thus, inoculation in the mouth (oral gavage) results in higher levels of parasitemia and mortality than intragastric or nasogastric infections (Barreto-de-Albuquerque et al., 2015). In oral infection experiments with TCTs or amastigotes, we preferred to use a nasogastric tube instead of oral infection. We chose this procedure for two reasons. First, since the objective was to evaluate the infectivity of TCTs and amastigotes, we decided to determine the infective capacity of the gastric mucosa, avoiding contact of the parasites with the oral mucosa or upper digestive tract. Second, we tried to avoid premature mortality in infected mice.
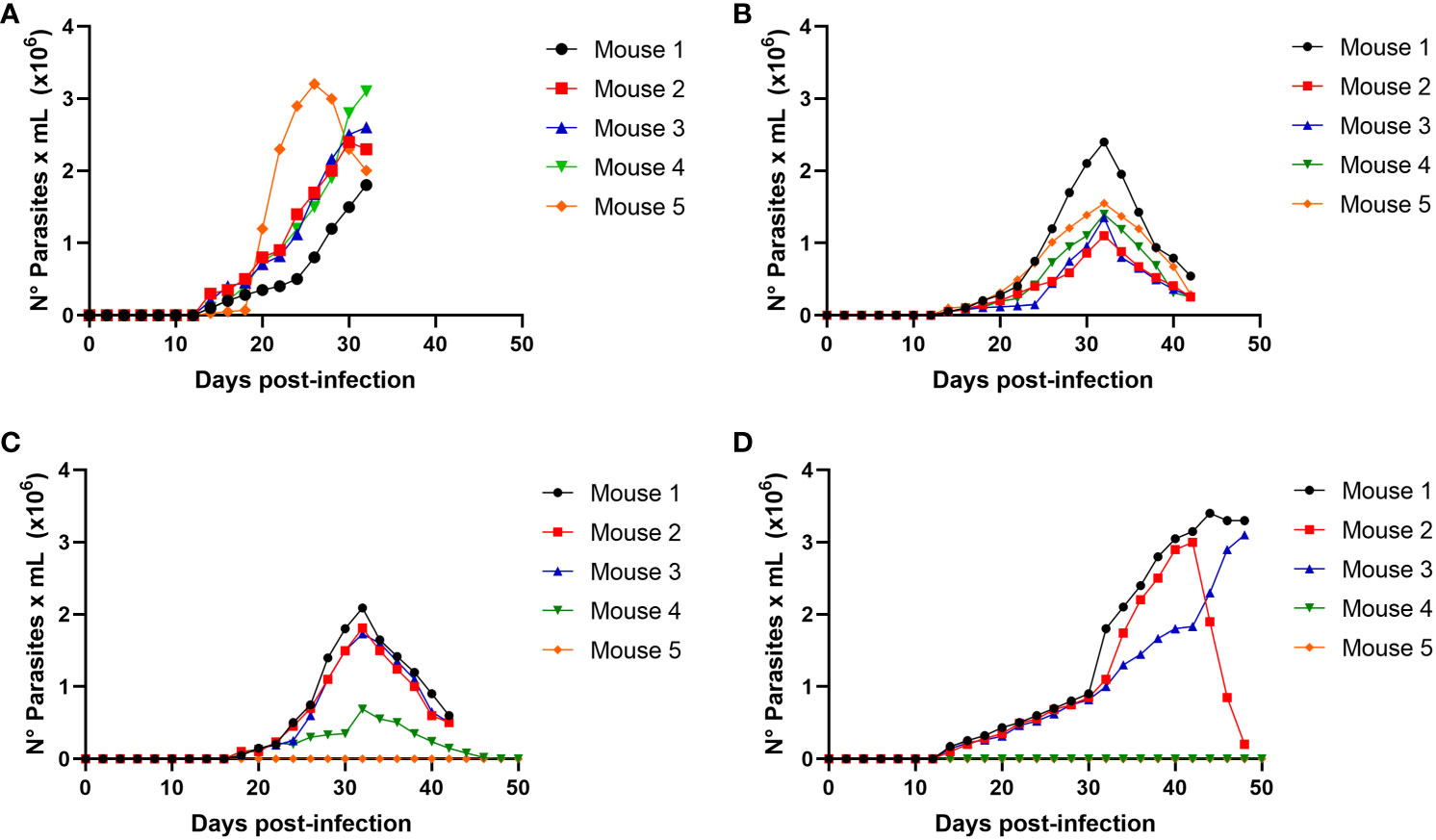
Figure 4 Oral infectivity of Trypanosoma cruzi amastigotes. Groups of five female Balb/c mice were orally infected using a nasogastric probe with 4 × 105 (A), 3 × 105 (B), 2 × 105 (C), and 1 × 105 (D) T. cruzi amastigotes from the C8C3hvir cell line. The parasitemia curve was determined by counting (every 2 days) the number of parasites in a blood sample obtained from a small cut in the tail of the mouse. Results are expressed as mean ± 1 SD and represent at least 3 experiments performed with the same protocols.
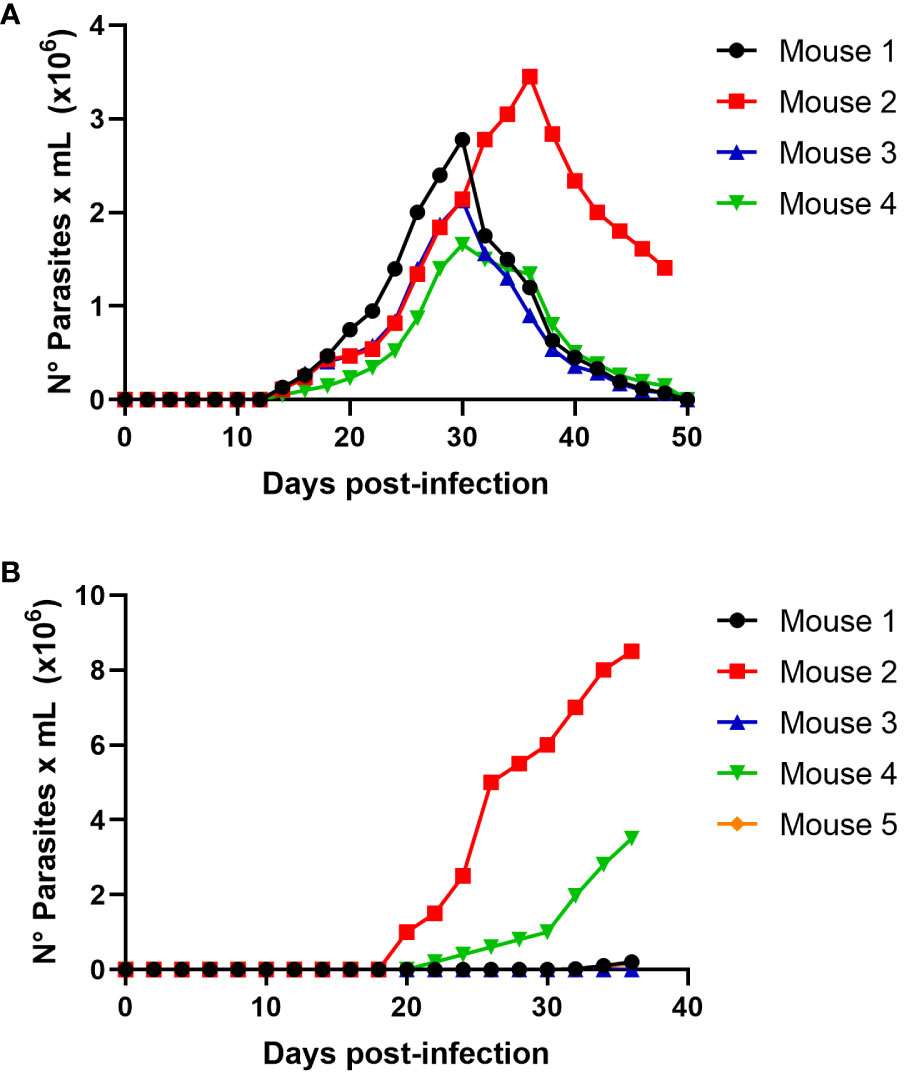
Figure 5 Oral infectivity of Trypanosoma cruzi trypomastigotes. Groups of five female Balb/c mice were orally infected using a nasogastric probe with × 4x105 (A) and 1 × 105 (B) T. cruzi trypomastigotes from the C8C3hvir cell line. Parasitemia curve was determined by counting (every 2 days) the number of parasites in a blood sample obtained through a small cut in the tail of the mouse. Results are expressed as the mean ± 1 SD and represent at least three experiments performed using the same protocols.
Next, we evaluated the virulence molecules that could be involved in parasite binding to mammalian stomach cells. To do this, we used trypomastigote or amastigote cell lysates of a recognized highly virulent T. cruzi cell line, C8C3hvir, which expresses the main virulence factors described in T. cruzi. Thus, blots of human stomach AGS cells incubated with amastigote cell lysates were probed with different antibodies, and only antibodies raised against cruzipain and the ssp4 epitope, recognized by the monoclonal antibody 2C2, were detected (Figure 6A). In trials with trypomastigote lysates, we observed that only antibodies against cruzipain and transialidase were able to detect the proteins associated with AGS cells (Figure 6B). In contrast, CRP or gp85 were not detected by immunoblot assays (Data not shown).
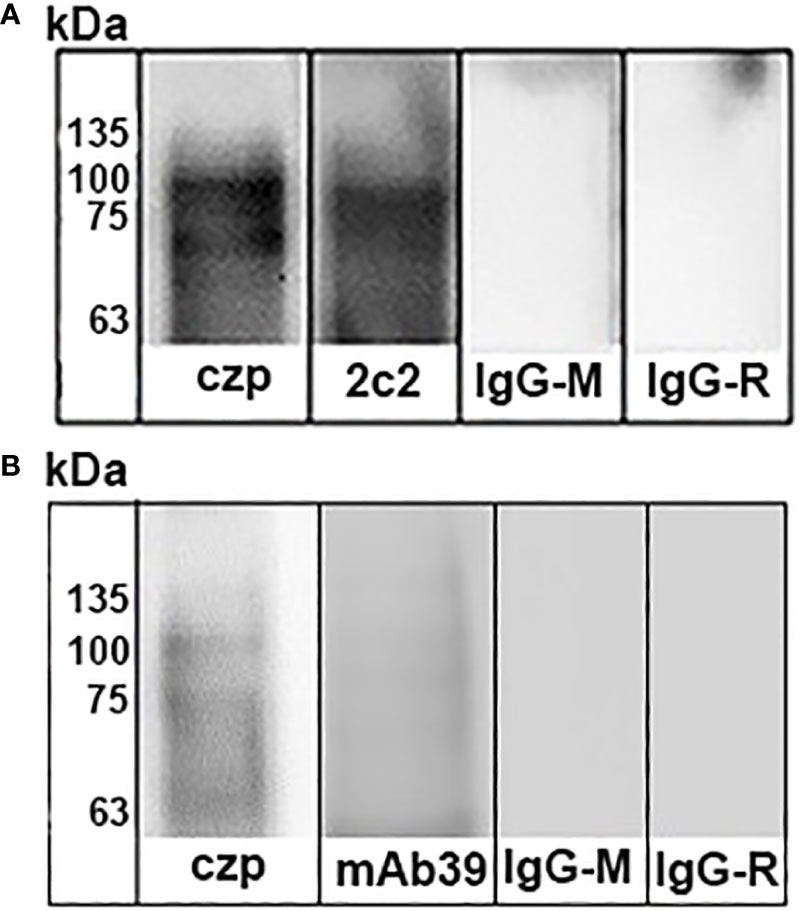
Figure 6 Identification of Trypanosoma cruzi binding to stomach cells. T. cruzi trypomastigotes (A) or amastigotes (B) were lysed by sonication and then centrifuged at 12.000 rpm for 10 min. Supernatants were incubated in flasks containing AGS cells for 4 h at 4°C. The cultures were washed four times and lysed with detergent Nonidet P-40. Lysates were centrifuged at 12.000 rpm for 10 min, and the supernatants were subjected to SDS-PAGE and transferred to nitrocellulose membranes. Immunoblots were then incubated with antibodies raised against cruzipain (Lane 1) and Ssp-4 epitope (Lane 2). b) Trypomatigote Immunoblots were incubated with antibodies raised against cruzipain (Lane 1) and trans-sialidase (Lane 2). Finally, the blots were incubated with secondary antibodies IgG-horseradish labeled and developed using enhanced chemiluminescence.
To deepen our knowledge of the interaction of TCTs with human stomach cells, the experimental shaving approach and subsequent protein identification by LC/MS-MS showed 33 proteins that would eventually interact during the T. cruzi adhesion/invasion process. Considering that the AGS cell cultures were incubated with the TCTs lysate, it is possible that the proteins located in the cytoplasm and organelles are not likely to participate in the invasive processes. Therefore, considering only parasite membrane proteins, it was possible to identify trans-sialidases, cysteinyl proteinases, and Gp63 (Table 1).
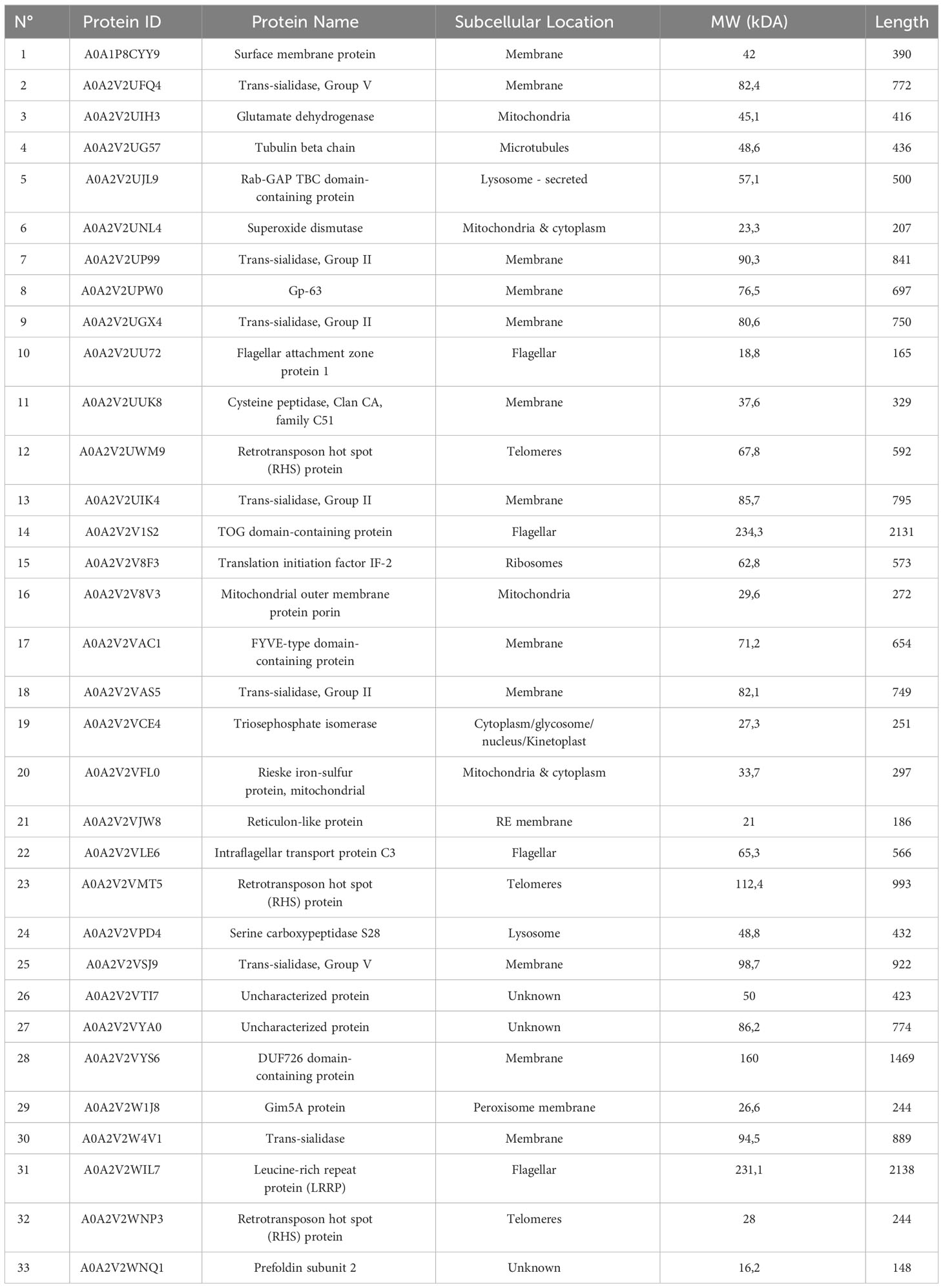
Table 1 Trypanosoma cruzi trypomastigotes proteins identified after incubation of parasite with human gastric adenocarcinoma from stomach cells.
Based on protein LC-MS/MS identification and in silico protein-protein interaction (PPI) analysis, we mapped the interaction network of T. cruzi membrane proteins and human proteins. Nine proteins were mapped, interacting with 34 human proteins (Figure 7). Among the T. cruzi proteins are cruzipain, cysteine peptidase, GP63, trans-sialidases, and mucin, among others. Human proteins involved in PPIs such Apolipoprotein L1, Cathepsin D heavy chain, Cathepsin B heavy chain, Catenin beta-1, Serum albumin, T-cell surface glycoprotein CD4 shown a high level of stomach expression level (Table 2).
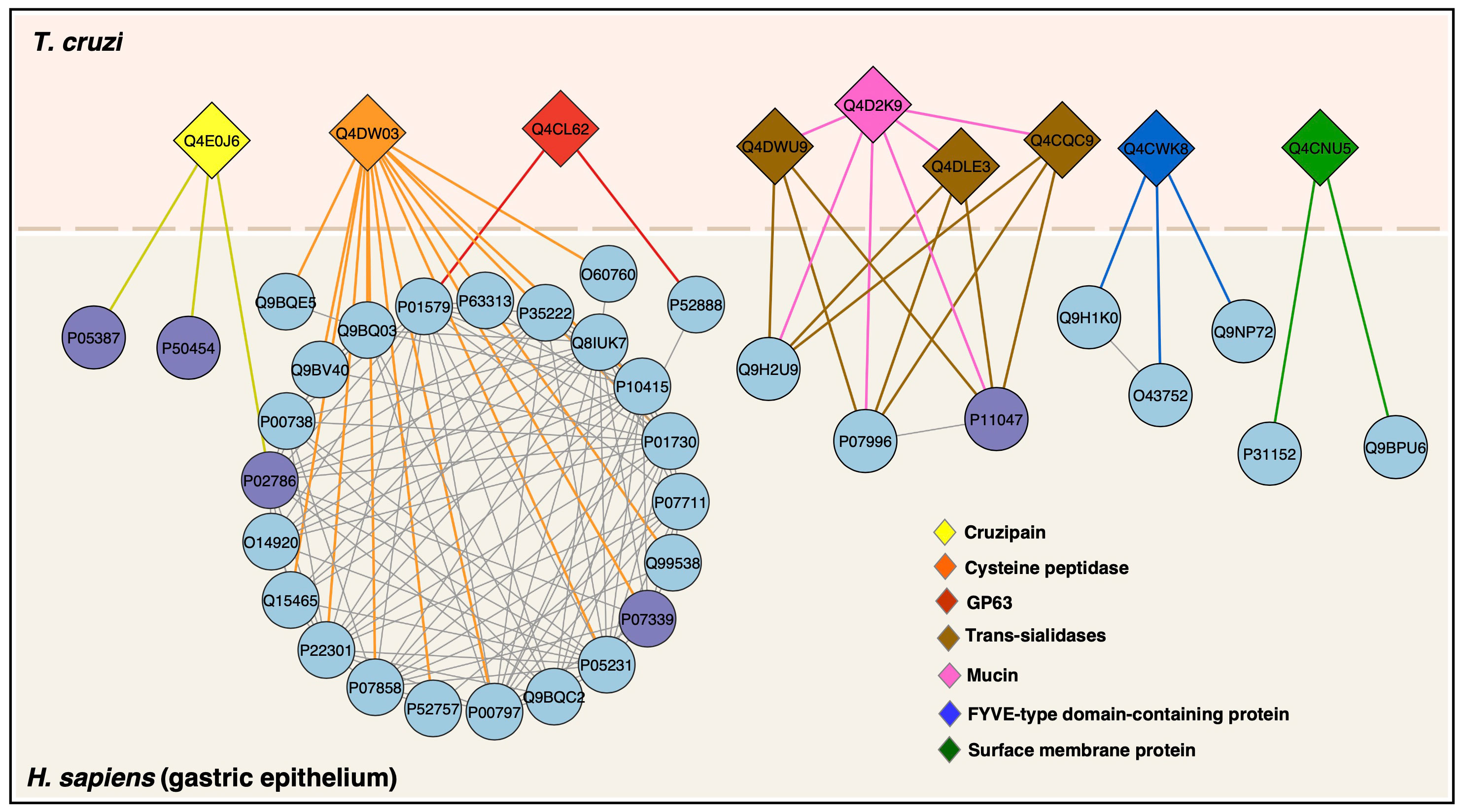
Figure 7 Protein–protein interactions between T. cruzi trypomastigotes and gastric epithelium. T. cruzi membrane proteins are colored diamonds and human proteins (circles) with predicted interactions are in lightblue and proteins identified by LC-MS/MS in this study are in purple. StringApp 2.0 was used to retrieve and visualize the cross-species network.
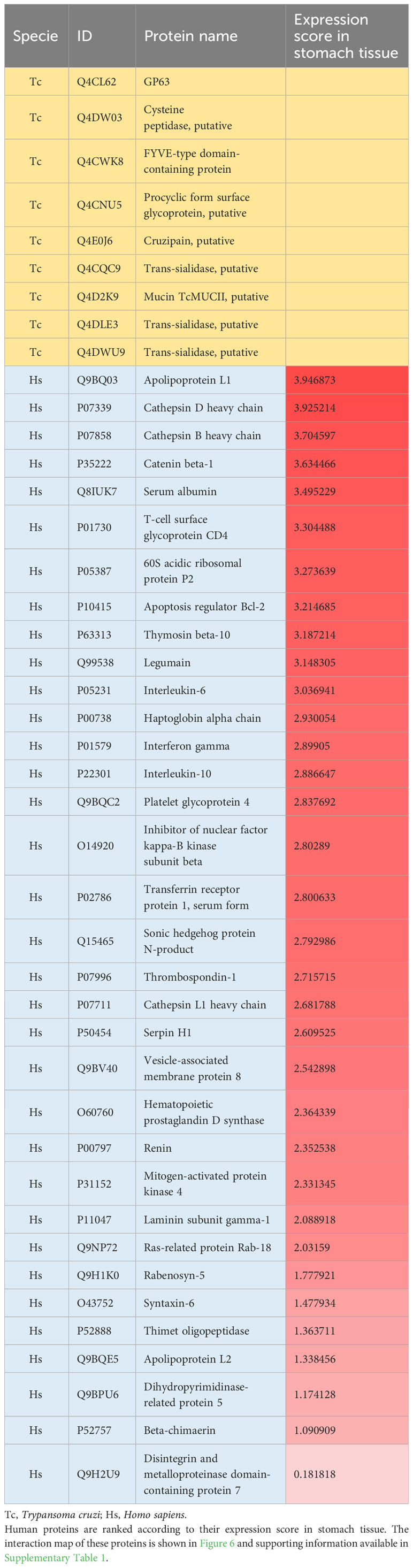
Table 2 Trypanosoma cruzi (Tc) membrane and human (Hs) proteins mapped with protein-protein interactions and the gene-tissue association for human proteins.
We then analyzed PPIs between human mucins and T. cruzi proteins (Figure 8). We found six mucin proteins that interacted with 33 T. cruzi proteins. All mucins shown a high level of expression in stomach tissue, except for Mucin-15 (Table 3).
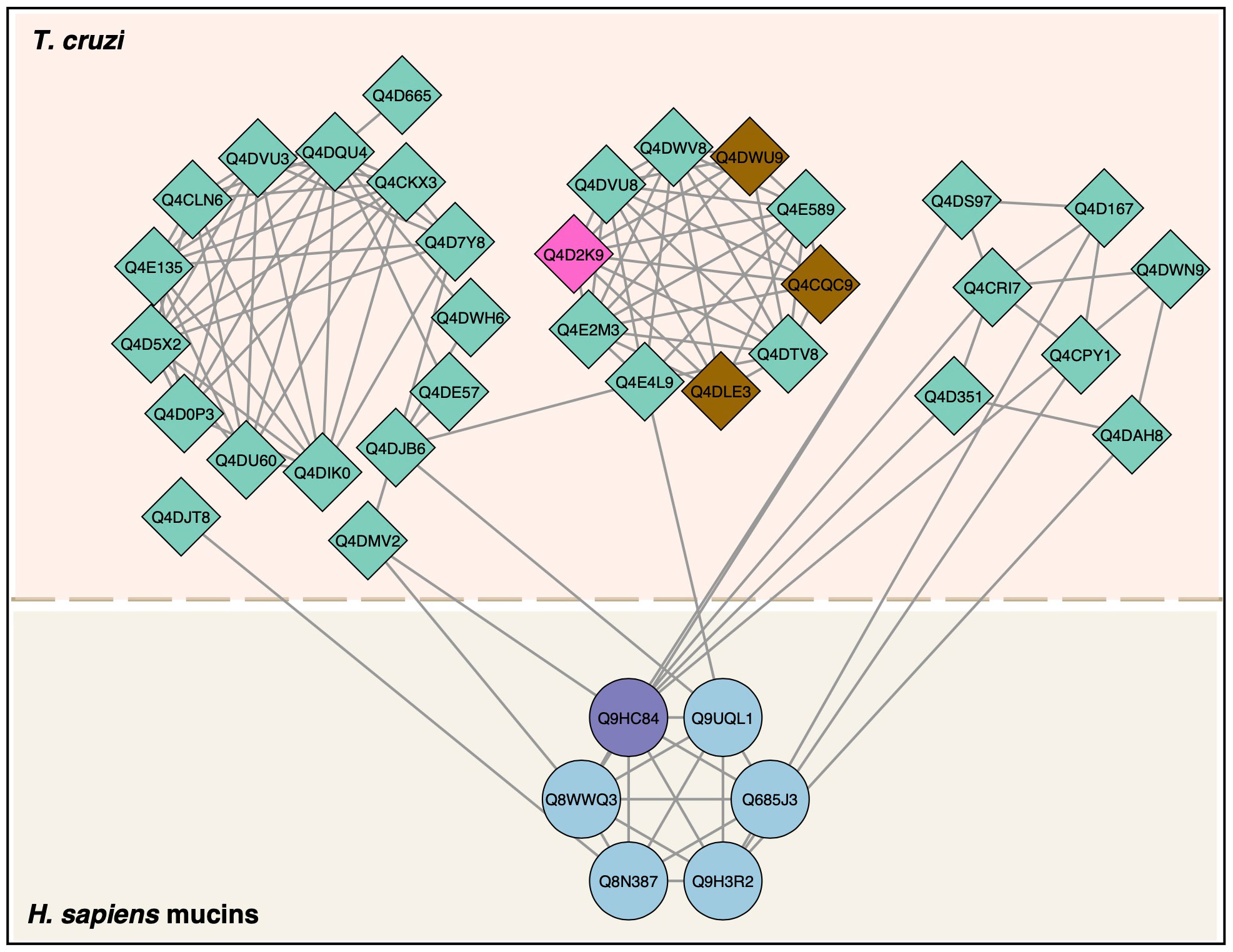
Figure 8 Protein–protein interactions between T. cruzi trypomastigote proteins and human mucins. T. cruzi proteins (diamonds) and human proteins (circles) interactions were mapped according to StringApp 2.0 database. T. cruzi proteins detected by LC-MS/MS in this study were mucin (pink) and trans-sialidase (brown). The human mucin identified by LC-MS/MS in this study was purple.
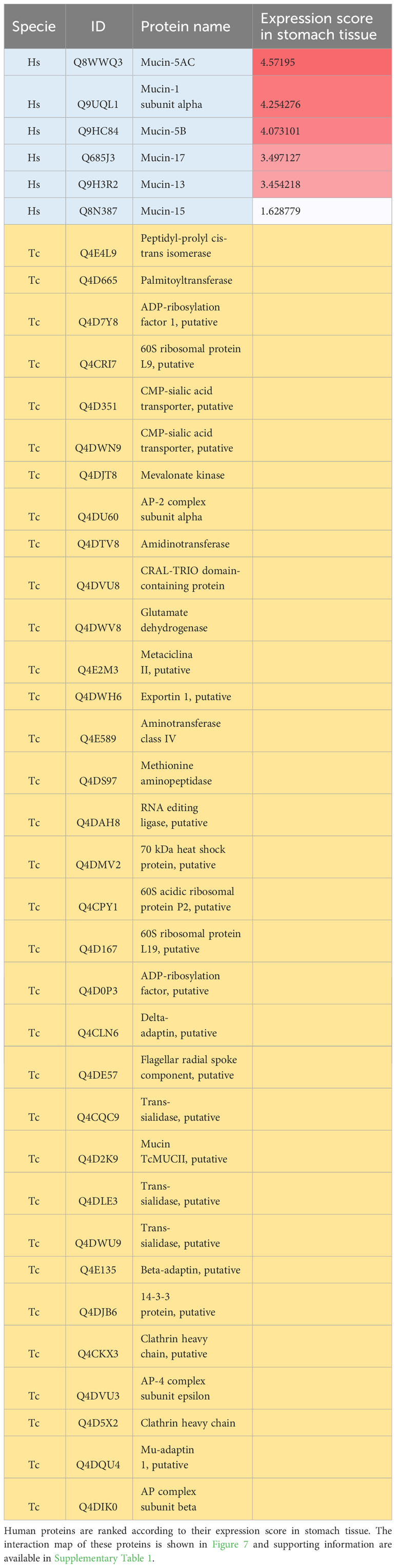
Table 3 Human (Hs) mucin proteins and T. cruzi (Tc) proteins mapped with protein-protein interactions with T. cruzi proteins.
Our search for cysteine protease cleavage sites in human mucins using in silico prediction returned tens to thousands of cleavage sites in mucins (Supplementary Table 2). We mapped the ten best-ranked sites based on their prediction scores and found that all mucins had this score (Figure 9A). Our analysis allowed us to extrapolate the cleavage sites for calpain and cruzipain proteases based on the amino acid motifs present in the P1 and P2 sites of the substrates, in this case in mucins (Figure 9B).
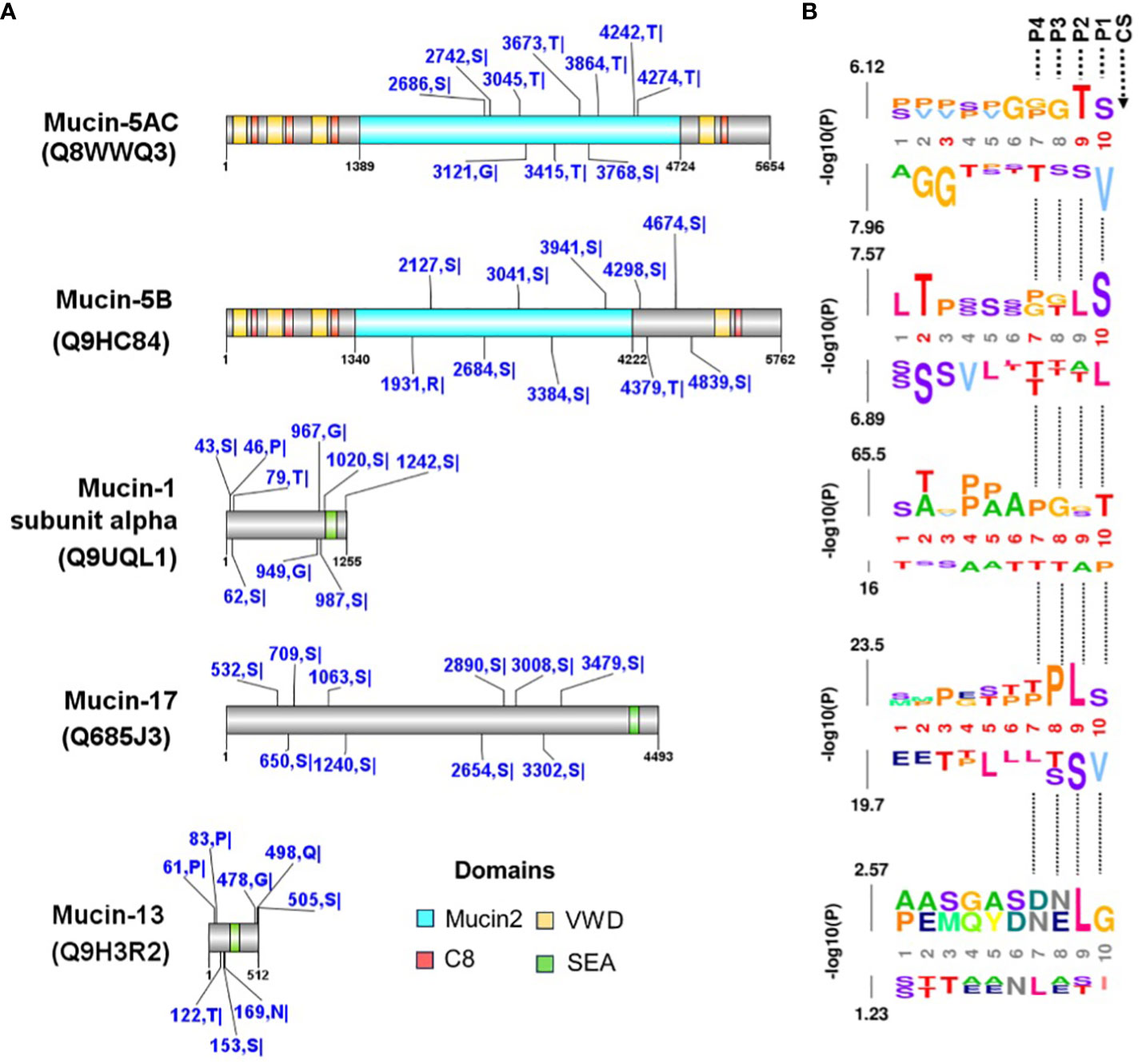
Figure 9 Prediction of cysteine protease cleavage sites in mucin proteins. (A) The cleavage sites predicted with a high cut-off for mucins mapped with PPIs between T. cruzi (see Table 3 and Figure 8). The top ten scored cleavage sites are shown for each mucin. The domains were identified in the CDD-NCBI conserved domain database, and specific hits are shown. The predicted sites are shown in blue with the amino acid positions and abbreviations. The domains are the Mucin-2 protein WxxW repeating region (this family is a repeating region found on mucins 2 and 5), C8 is a domain that contains eight conserved cysteine residues, VWD is a von Willebrand factor (vWF) type D domain and contains several type D domains, the and SEA domain is proposed to regulate or bind carbohydrate side chains. Recently, proteolytic activity has been demonstrated for the SEA domain. (B) The predicted sequence window upstream of the cleavage site was analyzed using the k-mer probability logo (kpLogo) tool for detection and visualization of position-specific amino acid motifs. P1-P4 are the positions of substrate amino acids related to cleavage site (CS) predicted.
Finally, to corroborate in vivo the possible role of cysteine proteinases like cruzipain, the main T. cruzi cysteine proteinase seems to play an important role in parasite binding to the stomach cells. We performed oral infection in mice using a nasopharyngeal probe, in which trypomastigotes or amastigotes were treated with or without E 64d (Figure 10). Thus, as shown in Figure 10A, the infectivity of trypomastigotes previously treated with E 64d was dramatically reduced, while the three mice infected with untreated trypomastigotes developed high parasitemia (Figure 10A). In the same way, when amastigotes were pretreated with E64d, parasitemia was significantly decreased (Figure 10B).
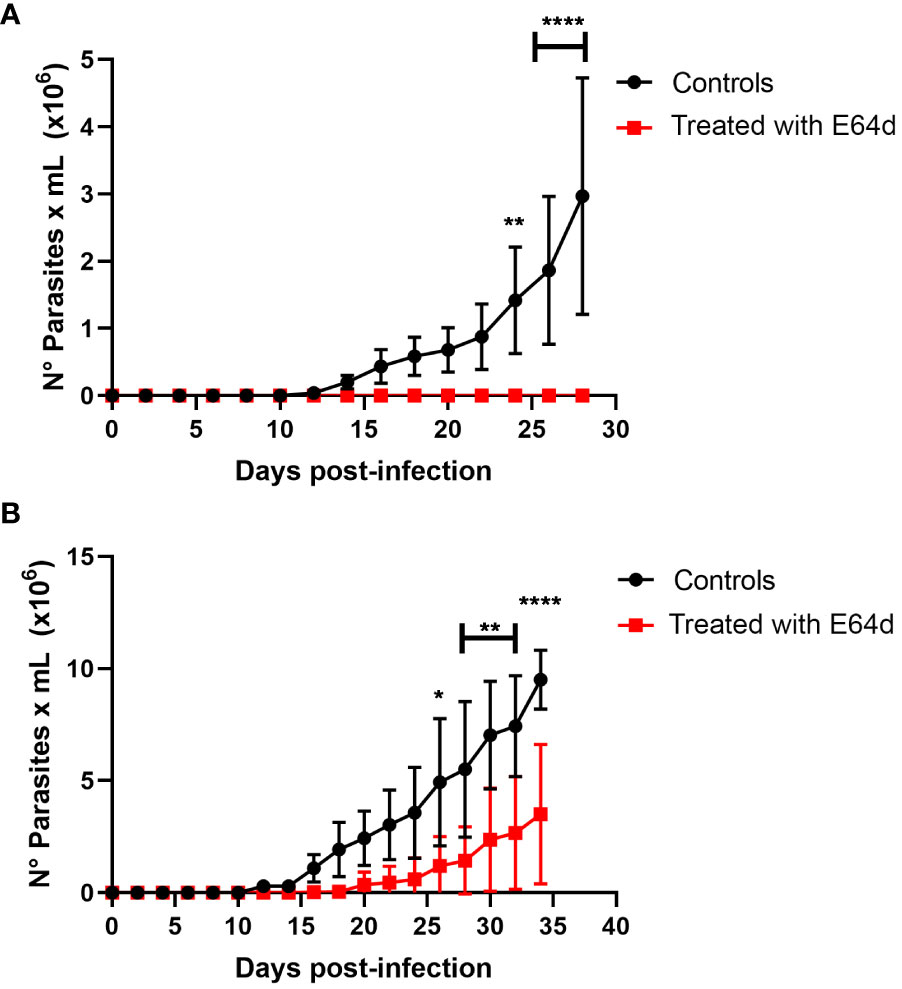
Figure 10 Role of cruzipain in oral Trypanosoma cruzi infection. Groups of 5 female Balb/c mice were orally infected using a nasogastric probe with 4x105 trypomastigotes (A) or 1x105 amastigotes (B) from T.cruzi C8C3hvir cell line, with or without E 64d cysteine proteinase inhibitor. Parasitemia curve was determined by counting (every 2 days) the number of parasites in a blood sample obtained through a small cut in the tail of the mouse. The results are expressed as the mean ± 1 SD and represent at least three experiments performed in triplicate. **P < 0,01, ***P < 0,001; Two-way ANOVA.
Discussion
T. cruzi infection is still highly prevalent in Latin America, where active transmission still occurs, especially in Venezuela, Colombia, and Bolivia, where vectors have reappeared (Lidani et al., 2019). However, 70% of the 1,000 cases of acute Chagas disease described in the first 10 years of this century have resulted from oral infections (Shikanai-Yasuda and Carvalho, 2012; Bruneto et al., 2021).
Initially, Chagas disease was endemoenzootic, where the T.cruzi cycle in wild environments was maintained in great part by oral infections, when different animals ingested infected vectors or through carnivorism in the prey-predator relationship (Coura et al., 2002; Coura, 2006). However, the latter hypothesis states that it naturally occurs and still occurs in the wild environments of the continent has not been confirmed, and there is no experimental evidence that meat consumption from animals infected with T. cruzi can transmit infection. Therefore, this study aimed to demonstrate the role of meat intake from infected animals in the oral transmission of T. cruzi.
To approach this question, we fed mice meat from mice in the acute phase of T. cruzi infection at the parasitemia peak (Figure 3A). Three of the five mice (60%) were infected with T. cruzi and died from infection (Figure 3B). This observation demonstrated that the intake of infected animal meat could play a role in the oral transmission of T. cruzi infection. To our knowledge, this is the first experimental evidence demonstrating the role of carnivorism in Chagas disease transmission. However, Aufderheide et al. (2004) studied 283 mummified bodies from sites in southern Peru and northern Chile and demonstrated a Chagas’ disease prevalence rate of approximately 41% over the past 9,000 years. They suggested that oral transmission might have occurred by ingesting infected, uncooked meat, such as guinea pigs (Cavia porcellus). If the raw meat from T.cruzi infected mice was used to infect the mice that were consumed, trypomastigotes or intracellular amastigotes could be responsible for the infection. Experimental infections were performed using different inoculums at these two parasite stages to investigate this.
When mice were infected with a nasogastric probe with different amastigotes inoculums, all animals were infected (Figure 4). One hundred percent of the mice were infected with an inoculum of 3×105 (Figure 4B), whereas 80% were infected with an inoculum of 2×105 (Figure 4C). Moreover, 60% of the mice were infected with the inoculum of 1x105 (Figure 4D). Similarly, trypomastigotes were infective when inoculums of 4 × 105 were used, resulting in four of the five infected mice (80%) (Figure 5A). When inoculums of 1 × 105 were used, 4 of 5 mice inoculated (80%) were also infected (Figure 5B). This evidence shows that both, TCTs and amastigotes can invade the gastric mucosa and cause oral infections. To our knowledge, the role of amastigotes in oral transmission of T. cruzi has not been evaluated. However, unlike metacyclics trypomastigotes (MT), it has been proposed that blood trypomastigotes are inefficient in infecting mice via the oral route (Hoft, 1996). The reason for these differences in infectivity between these two stages is not well known but could be due to the migration capacity of the two infectious forms through the gastric mucin layer (Cortez et al., 2012). When tested for the possible use of TCTs, it was found that the parasite traversed the mucin layer at a two-fold lower number than metacyclic trypomastigote (MT). The possibility of blood trypomastigotes being more susceptible to pepsin digestion at an acidic pH has also been proposed (Cortez et al., 2006). In our study, we observed that TCTs from the C8C3hvir cell line were highly susceptible to pepsin lysis. This susceptibility and reduced ability to pass through mucin may explain why not all mice were infected. Similarly, differences in parasitemia peaks with respect to MT infections were observed. How can this TCTs delay the peak of parasitemia be explained? One of the most likely explanations could be based on the fact that MTs are highly resistant to degradation by pepsin (Cortez et al., 2012)). Therefore, the total inoculum administered can be used to infect stomach host cells. However, our experiments on migration through mucin in the presence or absence of pepsin at acidic pH and the in vitro infection of AGS cells in the presence of mucin showed that the situation with TCTs is completely different from that with MTs. Only a small number of TCTs cross mucin and can resist pepsin and acidic pH. Therefore, if we extrapolate this condition to what could occur in vivo, it is possible to hypothesize that only a very small portion of the TCTs inoculum would survive and invade the stomach cells. Since there is a small amount of infecting inoculum, the development of the infectious process would be delayed; therefore, the parasitemia peak would also be delayed.
However, we demonstrated that TCTs from the virulent cell line C8C3hvir and amastigotes were highly infectious in mice. Nevertheless, the origin of the observed differences in the infectivity of blood trypomastigotes and TCTs may also be due to their genetic background. Little information is available regarding the genotypes associated with oral T. cruzi infections. However, DTU 1 seems to be the most frequently encountered, where haplotipes TcId has been identified in T cruzi isolates from rats, triatomines, and patients (Marcili et al., 2009; Andrade et al., 2011; Ramírez et al., 2013; Díaz-Bello et al., 2014). As the C8C3hvir cell line corresponds to DTU 1, this may partly explain its ability to cause oral infections. Nevertheless, studies of metacyclic forms of different strains have suggested that they are poorly infective (Maeda et al., 2016). However, our evidence using TCTs suggests otherwise. Thus, it is possible to theorize that DTU 1 could be poorly infective if transmitted by contamination with triatomine feces containing metacyclic forms of the parasite but may be highly infective when consumed in meat from infected animals having blood trypomastigotes or amastigotes.
If trypomastigotes and amastigotes are infectives by the oral route, we raise the question of which molecules could be involved in parasite binding to mammalian stomach cells. When the immunoblots of AGS cells incubated with amastigote cell lysates were incubated with different antibodies, only antibodies raised against cruzipain and the Ssp-4 epitope, recognized by the monoclonal antibody 2C2, were detected (Figure 6A). In trials with trypomastigote lysates, we observed that only antibodies against cruzipain and transialidase were able to detect the proteins associated with AGS cells (Figure 6B), whereas immunoblots in which antibodies against CRP or gp85 did not detect reactivity.
A shaving experiment was performed to confirm these observations, and proteins associated with the surface of human stomach AGS cells were identified by LC-MS/MS (Table 1). Among the T. cruzi TCT membrane proteins identified, several trans-sialidases were observed in addition to cysteinyl proteinases and Gp63. The presence of trans-sialidase and cysteine proteinases was consistent with our observations derived from the western blot assays. Gp63 was not studied by western blotting. However, the role of Gp63 in T. cruzi infectivity has been suggested by Cuevas et al. (2003). On the other hand, trans-sialidase is a unique T. cruzi enzyme involved in transferring sialic acid from host glycoconjugates to mucins (Eugenia Giorgi and De Lederkremer, 2011). TS is involved in several virulence mechanisms, including invasion and host cell recognition (Magdesian et al., 2007; Dc-Rubin and Schenkman, 2012; Freire-de-Lima et al., 2012). The presence of sialylated epitopes on the surface of infective forms of T. cruzi increases infectivity (Piras et al., 1987; Schenkman et al., 1991). Similarly, sialylation and desialylation of the host cell surface can modulate parasite adherence and penetration (Freire-de-Lima et al., 2012). Therefore, it is possible to hypothesize that stomach mucus could be a source of sialic acid that positively regulates the invasion of the stomach epithelium through parasite TS activity. Considering that among the molecules detected both in the blots and in the shaving experiment associated with LC-MS/MS, we identified cruzipain and cysteine proteases, we decided to deepen our knowledge regarding the potential role of this protease in oral T. cruzi infection.
We then performed oral infection in mice, in which trypomastigotes or amastigotes treated with or without E64d were used to infect the mice (Figure 10). As shown in Figure 10A, the infectivity of trypomastigotes previously treated with E64d was dramatically reduced, whereas three mice infected with untreated trypomastigotes developed high parasitemia (Figure 10A). In contrast, parasitemia significantly decreased when amastigotes were pretreated with E64d (Figure 10B). These results suggest that cruzipain, the principal T. cruzi cysteinyl proteinases (and other parasite cysteine proteinases), plays an important role in parasite association with stomach cells. According to electron microscopy studies, Czp is located in the flagellar pocket of the TCTs being the main secreted lysosomal peptidase in T. cruzi (Souto-Padrón et al., 1990). Several studies using synthetic irreversible cysteine peptidase inhibitors have demonstrated that T. cruzi infection, host immune evasion, and intracellular growth depend on Czp activity (Meirelles et al., 1992; Tomas et al., 1997; Scharfstein et al., 2000; McKerrow et al., 2008; Doyle et al., 2011). Pretreatment of TCTs of the C8C3hvir cell line with E64d has been shown to significantly inhibit their infectivity both in vivo and in vitro (San Francisco et al., 2017). In the stomach, the gastric mucosa is the first natural barrier responsible for protecting the host against internal insults, such as the acidic pH of gastric juice, and external insults, such as microorganisms (Deng et al., 2021). Thus, cruzipain and other cysteinyl proteinases may play a critical role in the invasion of TCTs into the gastric mucosa. Although the molecular mechanisms involved in this process are unknown, it is possible to speculate that these proteases could participate in mucus degradation, an event before parasite contact with stomach epithelial cells. The participation of cysteinyl proteinases in the degradation of gastric mucus before parasite contact with the intestinal epithelium has been described in Entamoeba histolytica (Lidell et al., 2006).
In MT, it has been described that the surface glycoprotein gp82 plays a central role in establishing T. cruzi infection in mice via the oral route (Yoshida, 2008). gp82 is critical for oral T. cruzi infection because it binds gastric mucin in the mucus layer, which protects the stomach mucosa (Staquicini et al., 2010). It has been proposed that upon binding to gastric mucin, MT migrates through the mucus layer and reaches the underlying epithelial cells, invading in a gp82-mediated manner (Neira et al., 2003; Cortez et al., 2006; Covarrubias et al., 2007). However, no trypomastigote molecules have been reported to play a role in bloodstream trypmomastigotes or their counterparts in TCTs.
Here, we observed that cruzipain and transialidase were involved in parasite binding to stomach cells (Figure 6). In contrast, cruzipain digests fibronectin (FN). The interaction between fibronectin and T. cruzi has also been previously described. Experiments with TCT revealed the involvement of fibronectin in target cell adhesion/invasion (Ouaissi et al., 1985). Using T. cruzi CL strain metacyclic forms, it was reported that fibronectin present in the cell culture medium bound to metacyclic forms is digested by cruzipain. Treating HeLa cells with purified recombinant cruzipain increased parasite internalization, whereas treatment with cysteinyl proteinase inhibitors had the opposite effect. This evidence confirms that the cysteine proteinase of T. cruzi metacyclic forms, through its fibronectin-degrading activity, is involved in host cell invasion (Maeda et al., 2014). We speculated that cruzipain acts on stomach cells to degrade fibronectin and other glycoproteins in the extracellular matrix and promotes parasite invasion.
Several pieces of evidence strongly suggest that C8C3hvir virulence is related to the differential expression of well-known virulence factors, including Czp and TS (San Francisco et al., 2017). In addition, parasite treatment with E64d strongly reduced parasite oral infection capacity and parasitemia levels in BALB/c mice. Thus, the concerted action of cysteinyl proteinases and TS could contribute to the success of TCTs in the invasion of the gastric mucosa. Although the molecule that binds gastric mucins is unknown, trans-sialidase could act on the O-linked mucins present in the mucous layer of the stomach, removing sialic acid and sialydating the parasite membrane, making it more invasive. On the other hand, cysteinyl proteinases could degrade the gastric mucins, favoring the progression of TCTs through the mucous layer to finally access the stomach epithelium, where the classical mechanisms of invasion could operate.
Our in silico analysis of PPIs allowed us to identify important T. cruzi proteins involved in interactions with host proteins, including trans-sialidases and cruzipain, as well as proteins identified by LC-MS/MS in our proteomic dataset. Furthermore, these proteins interact with human proteins with high expression in stomach tissue, as seen in the gene-tissue association analysis.
Our interaction analysis involving host mucins, despite having demonstrated interaction with gp85 or gp82, nevertheless demonstrated that human mucins interact with T. cruzi protein complexes that are related to the transfer of sialic acid, such as the CMP-sialic acid transporter, putative, and trans-sialidase itself.
Since the activity of cysteine proteases is important in host cell interaction and invasion, we predicted substrate cleavage sites for cysteine proteases in mucin proteins, specifically for calpains, an important family of Ca+2-dependent cysteine proteases that contain a nucleophilic cysteine in the catalytically active site. The expression and cellular localization of T. cruzi calpains revealed that are distributed in different cellular components in all life cycle forms of the parasite, including plasma membrane (Ennes-Vidal et al., 2020) and thus, if calpains cleave mucins, they can regulate the interaction of the parasite in stomach cells. Thus, we predicted the calpain cleavage sites in mucins that were mapped in the interaction network with T. cruzi proteins. Site prediction showed that mucins have several cleavage sites for the cysteine protease calpains (Figure 9A). Another important family of cysteine proteases are cathepsins, which include cruzipain. The studies on substrate profiling of cysteine proteases showed that cruzipain has a preference for hydrophobic residues (such as Leucine and Glycine) in the P2 position of the substrates (Choe et al., 2006; Dos Reis et al., 2006; Ren et al., 2009; Wang et al., 2023). Our k-mer probability logo analysis of sequence window upstream predicted sites (Figure 9B) corroborates these previous results for cruzipain and reinforces the potential relationship of T. cruzi cysteine proteases, both calpain and cruzipains, with the invasion of stomach cells.
In summary, it was concluded that, under our experimental conditions, consuming meat from infected animals in the acute phase allows the transmission of T. cruzi infection. However, the possibility that oral transmission may be feasible when animals are in the indeterminate or chronic phase cannot be ruled out. At these stages of T. cruzi infection, the success of oral transmission may depend on the organ and tissue parasite load and the genetic haplotype of T. cruzi, which could determine its ability to resist acidic pH, cross mucins, and infect gastric cells. Thus, it is possible to postulate that the DTUs involved may be critical to the success of oral infection. Indeed, Hoft et al. (1996) reported poor infectivity of blood trypomastigotes in Tulahuen strain (TC VI). On the other hand, Cortez et al. 2012, also suggested that trypomastigotes were poorly infective but used Y strain (Tc II). In addition, the intrinsic virulence of each strain or isolate, indicated by a higher expression of virulence factors such as Cz, TS, CRP, and gp63, among others, should be considered (Osorio et al., 2012). Furthermore, upregulation of bioenergetic and biosynthetic pathways has been proposed as a new aspect to be considered in T. cruzi virulence (San Francisco et al. 2022). In addition, the genetic background of the host should also be considered, and a strain or isolate of a given DTU that is infective for one species might not be infective for another.
Transmission via the oral route is an emerging scenario in Chagas disease. This transmission mechanism displays a habitual character in the primitive and endemic cycle of the parasite, which occurs through consuming contaminated food and/or beverages by dejection of triatomine feces (PAHO/WHO, 2022). Here, we experimentally demonstrate that carnivorism is a mechanism of T. cruzi transmission. Although this is perhaps the major mechanism by which parasite infection is transmitted in the wild, the possibility of human transmission cannot be ruled out. In endemic areas where peridomestic and wild vectors are present concomitantly with rabbits, guinea pigs, lambs, and others, the meat of infected animals could be a source of human infection. Therefore, sanitary education of the population is necessary to prevent the ingestion of raw meat and to encourage the use of previously frozen or cooked meat. Therefore, if Chagas disease is a food-borne infection, implementing prophylactic measures, such as sanitization, freezing, or using heat, accompanied by qPCR detection methods, should be considered (de Oliveira et al., 2019). Furthermore, implementing health education programs for populations living in endemic areas of potential risk from the perspective of oral transmission of Chagas disease and the implementation of traveler advisories for tourists visiting these areas is an absolute necessity. These traveler advisories should emphasize avoiding the consumption of tropical fruit juices, pulps, and exotic meats (Franco-Paredes et al., 2020; López-García and Gilabert, 2023). Thus, considering that oral transmission of Chagas disease involves animals, the environment, and humans, only a concerted strategy under the One Health concept would succeed in controlling this form of transmission of this parasitic infection (López-García and Gilabert, 2023).
Finally, experimental studies will be necessary to define the differences observed in the infectivity of blood trypomastigotes or their equivalents, the TCTs. These should be oriented to define whether their infectivity depends on the genetic background of the parasite, on the infected species and its genetic background, or both.
Data availability statement
The datasets presented in this study can be found in online repositories. The names of the repository/repositories and accession number(s) can be found in the article/Supplementary Material.
Ethics statement
The animal study was approved by Comité de Ética de la Investigación Científica de la Universidad de Antofagasta. The study was conducted in accordance with the local legislation and institutional requirements.
Author contributions
VT: Conceptualization, Investigation, Writing – original draft, Writing – review & editing, Data curation, Formal analysis, Methodology, Validation. VC: Investigation, Data curation, Formal analysis. BG: Data curation, Investigation, Methodology, Writing – review & editing, Software, Supervision. JSF: Investigation, Methodology, Data curation, Software, Supervision, Writing – review & editing. AC: Data curation, Investigation, Software, Formal analysis, Resources, Writing – review & editing. JLV: Data curation, Investigation, Methodology, Formal analysis, Funding acquisition, Resources, Writing – review & editing. KM: Data curation, Investigation, Resources, Software, Methodology, Writing – original draft. LJF: Data curation, Formal analysis, Funding acquisition, Methodology, Resources, Writing – review & editing, Conceptualization, Software. RdA: Bioinformatic analysis, Writing-review and edition. AK: Funding acquisition, Writing – review & editing. JG: Conceptualization, Funding acquisition, Resources, Writing – review & editing, Investigation, Supervision, Visualization, Writing – original draft.
Funding
The author(s) declare financial support was received for the research, authorship, and/or publication of this article. JG acknowledges the financial support extended by Seedling Grants SEM-17-02 and the Bridge Fund for Research of Excellence at the University of Antofagasta. We are grateful to Agencia Nacional de Investigación y Desarrollo (ANID)-Millennium Science Initiative Program-ICN09_016: Millennium Institute on Immunology and Immunotherapy (ICN09_016; former P09/016-F). JSF was supported by Antofagasta University Fellowship. This work was partially supported by the Ministerio de Educación and Universidad de Antofagasta (MINEDUC-UA) project, code ANT 1999, and Vicerrectoria de Investigacion Innovación y Postgrado de la Universidad de Antofagasta (to JLV).
Acknowledgments
Special thanks to Mr. Juan Carlos Andrade of the Molecular Parasitology Unit, Universidad de Antofagasta, for their technical assistance; Ms. Marlene Zuñiga, MSc, from the Microscopy Unit, Instituto Antofagasta, for her invaluable help in obtaining the confocal microscopy images and to Ms. Valentina Morales for the preparation and editing of the reference list. We are also grateful to the Scientific Research Ethics Committee of Universidad de Antofagasta.
Conflict of interest
The authors declare that the research was conducted in the absence of any commercial or financial relationships that could be construed as a potential conflict of interest.
The author(s) declared that they were an editorial board member of Frontiers, at the time of submission. This had no impact on the peer review process and the final decision.
Publisher’s note
All claims expressed in this article are solely those of the authors and do not necessarily represent those of their affiliated organizations, or those of the publisher, the editors and the reviewers. Any product that may be evaluated in this article, or claim that may be made by its manufacturer, is not guaranteed or endorsed by the publisher.
Supplementary material
The Supplementary Material for this article can be found online at: https://www.frontiersin.org/articles/10.3389/fcimb.2024.1297099/full#supplementary-material
References
Andrade, S. G., Campos, R. F., Steindel, M., Guerreiro, M. L., Magalhães, J. B., de Almeida, M. C., et al. (2011). Biological, biochemical and molecular features of Trypanosoma cruzi strains isolated from patients infected through oral transmission during a 2005 outbreak in the state of Santa Catarina, Brazil: its correspondence with the new T. cruzi Taxonomy Consensus (2009). Mem Inst Oswaldo Cruz 106 (8), 948–956. doi: 10.1590/S0074-02762011000800009
Andrews, N. W., Hong, K., Robbins, E. S., Nussenzweig, V. (1987). Stage-specific surface antigens expressed during the morphogenesis of vertebrate forms of Trypanosoma cruzi. Exp. Parasitol. 64 (3), 474–484. doi: 10.1016/0014-4894(87)90062-2
Araya, J., Cornejo, A., Orrego, P., Cordero, E., Cortéz, M., Olivares, H., et al. (2008). Calcineurin B of the human protozoan parasite Trypanosoma cruzi is involved in cell invasion. Microbes Infect. 10 (8), 892–900. doi: 10.1016/j.micinf.2008.05.003
Aufderheide, A. C., Salo, W., Madden, M., Streitz, J., Buikstra, J., Guhl, F., et al. (2004). A 9,000-year record of Chagas’ disease. Proc. Natl. Acad. Sci. U.S.A. 101 (7), 2034–2039. doi: 10.1073/pnas.0307312101
Barbosa-Ferreira, J. M., Guerra, J. A. D. O., De Santana Filho, F. S., Magalhães, B. M. L., Coelho, LIARC, Barbosa, M. D. G. V. (2010). Cardiac involvement in Acute Chagas’ Disease cases in the Amazon region. Arq Bras. Cardiol. 94 (6), 147–149.
Barreto-de-Albuquerque, J., Silva-dos-Santos, D., Pérez, A. R., Berbert, L. R., de Santana-van-Vliet, E., Farias-de-Oliveira, D. A., et al. (2015). Trypanosoma cruzi Infection through the Oral Route Promotes a Severe Infection in Mice: New Disease Form from an Old Infection? PloS Negl. Trop. Dis. 9 (6), e0003849. doi: 10.1371/journal.pntd.0003849
Benchimol Barbosa, P. R. (2006). The oral transmission of Chagas’ disease: an acute form of infection responsible for regional outbreaks. Int. J. Cardiol. 112 (1), 132–133. doi: 10.1016/j.ijcard.2005.11.087
Bradford, M. M. (1976). A rapid and sensitive method for the quantitation of microgram quantities of protein utilizing the principle of protein-dye binding. Anal. Biochem. 72 (1–2), 248–254. doi: 10.1016/0003-2697(76)90527-3
Brener, Z. (1962). Therapeutic activity and criterion of cure on mice experimentally infected with Trypanosoma cruzi - PubMed. Inst Med. Trop. Sao Paulo 4, 389–396.
Bruneto, E. G., Fernandes-Silva, M. M., Toledo-Cornell, C., Martins, S., Ferreira, J. M. B., Corrêa, V. R., et al. (2021). Case-fatality from orally-transmitted acute chagas disease: A systematic review and meta-analysis. Clin. Infect. Dis. 72 (6), 1084–1092. doi: 10.1093/cid/ciaa1148
Choe, Y., Leonetti, F., Greenbaum, D. C., Lecaille, F., Bogyo, M., Brömme, D., et al. (2006). Substrate profiling of cysteine proteases using a combinatorial peptide library identifies functionally unique specificities. J. Biol. Chem. 281 (18), 12824–12832. doi: 10.1074/jbc.M513331200
Cortez, M., Silva, M. R., Neira, I., Ferreira, D., Sasso, G. R. S., Luquetti, A. O., et al. (2006). Trypanosoma cruzi surface molecule gp90 downregulates invasion of gastric mucosal epithelium in orally infected mice. Microbes Infect. 8 (1), 36–44. doi: 10.1016/j.micinf.2005.05.016
Cortez, C., Yoshida, N., Bahia, D., Sobreira, T. J. P. (2012). Structural basis of the interaction of a Trypanosoma cruzi surface molecule implicated in oral infection with host cells and gastric mucin. PloS One 7 (7), e42153. doi: 10.1371/journal.pone.0042153
Coura, J. R. (2006). [Transmission of chagasic infection by oral route in the natural history of Chagas disease]. Rev. Soc. Bras. Med. Trop. 39 Suppl 3, 113–117.
Coura, J. R. (2015). The main sceneries of Chagas disease transmission. The vectors, blood and oral transmissions–a comprehensive review. Mem Inst Oswaldo Cruz 110 (3), 277–282.
Coura, J. R., De Abreu, L. L., Willcox, H. P. F., Petana, W. (1997). [Comparative controlled study on the use of benznidazole, nifurtimox and placebo, in the chronic form of Chagas’ disease, in a field area with interrupted transmission. I. Preliminary evaluation]. Rev. Soc. Bras. Med. Trop. 30 (2), 139–144. doi: 10.1590/S0037-86821997000200009
Coura, J. R., Junqueira, A. C. V., Fernandes, O., Valente, S. A. S., Miles, M. A. (2002). Emerging Chagas disease in amazonian Brazil. Trends Parasitol. 18 (4), 171–176. doi: 10.1016/S1471-4922(01)02200-0
Coura, J., Vĩas, P. (2010). Chagas disease: a new worldwide challenge. Nature 465 (7301), S6–S7. doi: 10.1038/nature09221
Covarrubias, C., Cortez, M., Ferreira, D., Yoshida, N. (2007). Interaction with host factors exacerbates Trypanosoma cruzi cell invasion capacity upon oral infection. Int. J. Parasitol. 37 (14), 1609–1616. doi: 10.1016/j.ijpara.2007.05.013
Cuevas, I. C., Cazzulo, J. J., Sánchez, D. O. (2003). gp63 homologues in Trypanosoma cruzi: surface antigens with metalloprotease activity and a possible role in host cell infection. Infect. Immun. 71 (10), 5739–5749. doi: 10.1128/IAI.71.10.5739-5749.2003
Dc-Rubin, S. S. C., Schenkman, S. (2012). Trypanosoma cruzi trans-sialidase as a multifunctional enzyme in Chagas’ disease. Cell Microbiol. 14 (10), 1522–1530. doi: 10.1111/j.1462-5822.2012.01831.x
de Arias, A. R., Monroy, C., Guhl, F., Sosa-Estani, S., Santos, W. S., Abad-Franch, F. (2022). Chagas disease control-surveillance in the Americas: the multinational initiatives and the practical impossibility of interrupting vector-borne Trypanosoma cruzi transmission. Mem Inst Oswaldo Cruz 117 (1), e210130.
Deng, Z., Zhao, Y., Ma, Z., Zhang, M., Wang, H., Yi, Z., et al. (2021). Pathophysiological role of ion channels and transporters in gastrointestinal mucosal diseases. Cell Mol. Life Sci. 78 (24), 8109. doi: 10.1007/s00018-021-04011-5
De Noya, B. A., Díaz-Bello, Z., Colmenares, C., Ruiz-Guevara, R., Mauriello, L., Zavala-Jaspe, R., et al. (2010). Large urban outbreak of orally acquired acute Chagas disease at a school in Caracas, Venezuela. J. Infect. Dis. 201 (9), 1308–1315. doi: 10.1086/651608
de Noya, B. A., González, O. N. (2015). An ecological overview on the factors that drives to Trypanosoma cruzi oral transmission. Acta Trop. 151 (1), 94–102. doi: 10.1016/j.actatropica.2015.06.004
de Oliveira, A. C., Soccol, V. T., Rogez, H. (2019). Prevention methods of foodborne Chagas disease: Disinfection, heat treatment and quality control by RT-PCR. Int. J. Food Microbiol. 301, 34–40. doi: 10.1016/j.ijfoodmicro.2019.04.009
Dias, J. P., Bastos, C., Araújo, E., Mascarenhas, A. V., Netto, E. M., Grassi, F., et al. (2008). Acute Chagas disease outbreak associated with oral transmission. Rev. Soc. Bras. Med. Trop. 41 (3), 296–300. doi: 10.1590/S0037-86822008000300014
Díaz-Bello, Z., Thomas, M. C., López, M. C., Zavala-Jaspe, R., Noya, O., De Noya, B. A., et al. (2014). Trypanosoma cruzi genotyping supports a common source of infection in a school-related oral outbreak of acute Chagas disease in Venezuela. Epidemiol. Infect. 142 (1), 156–162. doi: 10.1017/S0950268813000757
Doncheva, N. T., Morris, J. H., Holze, H., Kirsch, R., Nastou, K. C., Cuesta-Astroz, Y., et al. (2023). Cytoscape stringApp 2.0: analysis and visualization of heterogeneous biological networks. J. Proteome Res. 22 (2), 637–646.
Dos Reis, F. C. G., Júdice, W. A. S., Juliano, M. A., Juliano, L., Scharfstein, J., Ana Paula, A. P. C. (2006). The substrate specificity of cruzipain 2, a cysteine protease isoform from Trypanosoma cruzi. FEMS Microbiol. Lett. 259 (2), 215–220. doi: 10.1111/j.1574-6968.2006.00267.x
Doyle, P. S., Zhou, Y. M., Hsieh, I., Greenbaum, D. C., McKerrow, J. H., Engel, J. C. (2011). The Trypanosoma cruzi protease cruzain mediates immune evasion. PloS Pathog. 7 (9), e1002139. doi: 10.1371/journal.ppat.1002139
Ennes-Vidal, V., Pitaluga, A. N., Britto CFDP de, C., Branquinha, M. H., Dos Santos, A. L. S., Menna-Barreto, R. F. S., et al. (2020). Expression and cellular localization of Trypanosoma cruzi calpains. Mem Inst Oswaldo Cruz 115 (9), 1–11.
Eugenia Giorgi, M., De Lederkremer, R. M. (2011). Trans-sialidase and mucins of Trypanosoma cruzi: an important interplay for the parasite. Carbohydr Res. 346 (12), 1389–1393. doi: 10.1016/j.carres.2011.04.006
Ferreira, C. S., Martinho, P. C., Neto, V. A., Cruz, R. R. B. (2001). Pasteurization of human milk to prevent transmission of Chagas disease. Rev. Inst Med. Trop. Sao Paulo 43 (3), 161–162. doi: 10.1590/S0036-46652001000300008
Franco-Paredes, C., Villamil-Gómez, W. E., Schultz, J., Henao-Martínez, A. F., Parra-Henao, G., Rassi, A., et al. (2020). A deadly feast: Elucidating the burden of orally acquired acute Chagas disease in Latin America - Public health and travel medicine importance. Travel Med. Infect. Dis. 36, 101565. doi: 10.1016/j.tmaid.2020.101565
Freire-de-Lima, L., Oliveira, I. A., Neves, J. L., Penha, L. L., Alisson-Silva, F., Dias, W. B., et al. (2012). Sialic acid: a sweet swing between mammalian host and Trypanosoma cruzi. Front. Immunol. 3. doi: 10.3389/fimmu.2012.00356
Hoft, D. F. (1996). Differential mucosal infectivity of different life stages of Trypanosoma cruzi. Am. J. Trop. Med. Hyg 55 (4), 360–364. doi: 10.4269/ajtmh.1996.55.360
Hoft, D. F., Farrar, P. L., Kratz-Owens, K., Shaffer, D. (1996). Gastric invasion by Trypanosoma cruzi and induction of protective mucosal immune responses. Infect. Immun. 64 (9), 3800–3810. doi: 10.1128/iai.64.9.3800-3810.1996
Lidani, K. C. F., Andrade, F. A., Bavia, L., Damasceno, F. S., Beltrame, M. H., Messias-Reason, I. J., et al. (2019). Chagas disease: from discovery to a worldwide health problem. Front. Public Heal 7 (6). doi: 10.3389/fpubh.2019.00166
Lidell, M. E., Moncada, D. M., Chadee, K., Hansson, G. C. (2006). Entamoeba histolytica cysteine proteases cleave the MUC2 mucin in its C-terminal domain and dissolve the protective colonic mucus gel. Proc. Natl. Acad. Sci. U.S.A. 103 (24), 9298–9303. doi: 10.1073/pnas.0600623103
Liu, Z., Cao, J., Gao, X., Ma, Q., Ren, J., Xue, Y. (2011). GPS-CCD: A novel computational program for the prediction of calpain cleavage sites. PloS One 6 (4), e19001. doi: 10.1371/journal.pone.0019001
López-García, A., Gilabert, J. A. (2023). Oral transmission of Chagas disease from a One Health approach: A systematic review. Trop. Med. Int. Health 28, 689–698.
Maeda, F. Y., Clemente, T. M., Macedo, S., Cortez, C., Yoshida, N. (2016). Host cell invasion and oral infection by Trypanosoma cruzi strains of genetic groups TcI and TcIV from chagasic patients. Parasit Vectors 9 (1), 189. doi: 10.1186/s13071-016-1455-z
Maeda, F. Y., Cortez, C., Izidoro, M. A., Juliano, L., Yoshida, N. (2014). Fibronectin-degrading activity of Trypanosoma cruzi cysteine proteinase plays a role in host cell invasion. Infect. Immun. 82 (12), 5166–5174. doi: 10.1128/IAI.02022-14
Magdesian, M. H., Tonelli, R. R., Fessel, M. R., Silveira, M. S., Schumacher, R. I., Linden, R., et al. (2007). A conserved domain of the gp85/trans-sialidase family activates host cell extracellular signal-regulated kinase and facilitates Trypanosoma cruzi infection. Exp. Cell Res. 313 (1), 210–218. doi: 10.1016/j.yexcr.2006.10.008
Marcili, A., Valente, V. C., Valente, S. A., Junqueira, A. C. V., da Silva, F. M., Pinto, A. Y. d. N., et al. (2009). Trypanosoma cruzi in Brazilian Amazonia: Lineages TCI and TCIIa in wild primates, Rhodnius spp. and in humans with Chagas disease associated with oral transmission. Int. J. Parasitol. 39 (5), 615–623.
McKerrow, J. H., Rosenthal, P. J., Swenerton, R., Doyle, P. (2008). Development of protease inhibitors for protozoan infections. Curr. Opin. Infect. Dis. 21 (6), 668. doi: 10.1097/QCO.0b013e328315cca9
Meirelles, M. N. L., Juliano, L., Carmona, E., Silva, S. G., Costa, E. M., Murta, A. C. M., et al. (1992). Inhibitors of the major cysteinyl proteinase (GP57/51) impair host cell invasion and arrest the intracellular development of Trypanosoma cruzi in vitro. Mol. Biochem. Parasitol. 52 (2), 175–184. doi: 10.1016/0166-6851(92)90050-T
Neira, I., Silva, F. A., Cortez, M., Yoshida, N. (2003). Involvement of Trypanosoma cruzi metacyclic trypomastigote surface molecule gp82 in adhesion to gastric mucin and invasion of epithelial cells. Infect. Immun. 71 (1), 557–561. doi: 10.1128/IAI.71.1.557-561.2003
Osorio, L., Ríos, I., Gutiérrez, B., González, J. (2012). Virulence factors of Trypanosoma cruzi: who is who? Microbes Infect. 14 (15), 1390–1402.
Ouaissi, M. A., Cornette, J., Capron, A. (1985). Trypanosoma cruzi: modulation of parasite-cell interaction by plasma fibronectin. Eur. J. Immunol. 15 (11), 1096–1101. doi: 10.1002/eji.1830151106
PAHO/WHO. (2022). Chagas disease (United States: Pan American Health Organization). Available at: https://www.paho.org/en/topics/chagas-disease.
Palasca, O., Santos, A., Stolte, C., Gorodkin, J., Jensen, L. J. (2018). TISSUES 2.0: An integrative web resource on mammalian tissue expression. Database 2018, bay003.
Pereira, K. S., Schmidt, F. L., Guaraldo, A. M. A., Franco, R. M. B., Dias, V. L., Passos, L. A. C. (2009). Chagas’ disease as a foodborne illness. J. Food Prot 72 (2), 441–446. doi: 10.4315/0362-028X-72.2.441
Piras, M. M., Henríquez, D., Piras, R. (1987). The effect of fetuin and other sialoglycoproteins on the in vitro penetration of Trypanosoma cruzi trypomastigotes into fibroblastic cells. Mol. Biochem. Parasitol. 22 (2–3), 135–143. doi: 10.1016/0166-6851(87)90043-0
Ramírez, J. D., Montilla, M., Cucunubá, Z. M., Floréz, A. C., Zambrano, P., Guhl, F. (2013). Molecular epidemiology of human oral Chagas disease outbreaks in Colombia. PloS Negl. Trop. Dis. 7 (2), e2041. doi: 10.1371/journal.pntd.0002041
Rani, S., Cerqueira-Cézar, C. K., Murata, F. H. A., Sadler, M., Kwok, O. C. H., Pradhan, A. K., et al. (2019). Toxoplasma gondii tissue cyst formation and density of tissue cysts in shoulders of pigs 7 and 14 days after feeding infected mice tissues. Vet. Parasitol. 269, 13–15. doi: 10.1016/j.vetpar.2019.04.004
Rassi, A., Rassi, A., Marin-Neto, J. A. (2010). Chagas disease. Lancet (London England) 375 (9723), 1388–1402. doi: 10.1016/S0140-6736(10)60061-X
Rees, M. A., Kleifeld, O., Crellin, P. K., Ho, B., Stinear, T. P., Smith, A. I., et al. (2015). Proteomic characterization of a natural host-pathogen interaction: repertoire of in vivo expressed bacterial and host surface-associated proteins. J. Proteome Res. 14 (1), 120–132. doi: 10.1021/pr5010086
Ren, J., Wen, L., Gao, X., Jin, C., Xue, Y., Yao, X. (2009). DOG 1.0: illustrator of protein domain structures. Cell Res. 19 (2), 271–273.
Sánchez, L., Ramírez, J. (2013). Congenital and oral transmission of American trypanosomiasis: an overview of physiopathogenic aspects. Parasitology 140 (2), 147–159. doi: 10.1017/S0031182012001394
San Francisco, J., Astudillo, C., Vega, J. L., Catalán, A., Gutiérrez, B., Araya, J. E., et al. (2022). Trypanosoma cruzi pathogenicity involves virulence factor expression and upregulation of bioenergetic and biosynthetic pathways. Virulence 13 (1), 1827–1848. doi: 10.1080/21505594.2022.2132776
San Francisco, J., Barría, I., Gutiérrez, B., Neira, I., Muñoz, C., Sagua, H., et al. (2017). Decreased cruzipain and gp85/trans-sialidase family protein expression contributes to loss of Trypanosoma cruzi trypomastigote virulence. Microbes Infect. 19 (1), 55–61. doi: 10.1016/j.micinf.2016.08.003
Scharfstein, J., Schmitz, V., Morandi, V., Capella, M. M. A., Lima, A. P. C. A., Morrot, A., et al. (2000). Host cell invasion by Trypanosoma cruzi is potentiated by activation of bradykinin B(2) receptors. J. Exp. Med. 192 (9), 1289–1299. doi: 10.1084/jem.192.9.1289
Schenkman, S., Jiang, M. S., Hart, G. W., Nussenzweig, V. (1991). A novel cell surface trans-sialidase of Trypanosoma cruzi generates a stage-specific epitope required for invasion of mammalian cells. Cell 65 (7), 1117–1125. doi: 10.1016/0092-8674(91)90008-M
Schmunis, G., Yadon, Z. (2010). Chagas disease: A Latin American health problem becoming a world health problem. Acta Trop. 115 (1–2), 14–21. doi: 10.1016/j.actatropica.2009.11.003
Shannon, P., Markiel, A., Ozier, O., Baliga, N. S., Wang, J. T., Ramage, D., et al. (2003). Cytoscape: a software environment for integrated models of biomolecular interaction networks. Genome Res. 13 (11), 2498–2504. doi: 10.1101/gr.1239303
Shikanai-Yasuda, M. A., Carvalho, N. B. (2012). Oral transmission of Chagas disease. Clin. Infect. Dis. 54 (6), 845–852. doi: 10.1093/cid/cir956
Souto-Padrón, T., Campetella, O. E., Cazzulo, J. J., De Souza, W. (1990). Cysteine proteinase in Trypanosoma cruzi: immunocytochemical localization and involvement in parasite-host cell interaction. J. Cell Sci. 96 (Pt 3), 485–490. doi: 10.1242/jcs.96.3.485
Staquicini, D. I., Martins, R. M., Macedo, S., Sasso, G. R. S., Atayde, V. D., Juliano, M. A., et al. (2010). Role of GP82 in the selective binding to gastric mucin during oral infection with Trypanosoma cruzi. PloS Negl. Trop. Dis. 4 (3), e613. doi: 10.1371/annotation/a81cf9ae-ac77-4f3b-a917-336d6616461d
Tomas, A. M., Miles, M. A., Kelly, J. M. (1997). Overexpression of cruzipain, the major cysteine proteinase of Trypanosoma cruzi, is associated with enhanced metacyclogenesis. Eur. J. Biochem. 244 (2), 596–603. doi: 10.1111/j.1432-1033.1997.t01-1-00596.x
Wang, J., Chitsaz, F., Derbyshire, M. K., Gonzales, N. R., Gwadz, M., Lu, S., et al. (2023). The conserved domain database in 2023. Nucleic Acids Res. 51 (D1), D384–D388. doi: 10.1093/nar/gkac1096
Keywords: Trypanosoma cruzi, oral infection, cruzipain, trans-sialidase, host-pathogen interaction
Citation: Torres V, Contreras V, Gutiérrez B, Francisco JS, Catalán A, Vega JL, Moon K-M, Foster LJ, de Almeida RF, Kalergis AM and González J (2024) Oral infectivity through carnivorism in murine model of Trypanosoma cruzi infection. Front. Cell. Infect. Microbiol. 14:1297099. doi: 10.3389/fcimb.2024.1297099
Received: 19 September 2023; Accepted: 11 January 2024;
Published: 22 February 2024.
Edited by:
Martin Craig Taylor, University of London, United KingdomReviewed by:
Belkisyole Alarcon De Noya, Central University of Venezuela, VenezuelaCristian Cortez, Pontificia Universidad Católica de Valparaíso, Chile
Copyright © 2024 Torres, Contreras, Gutiérrez, San Francisco, Catalán, Vega, Moon, Foster, de Almeida, Kalergis and González. This is an open-access article distributed under the terms of the Creative Commons Attribution License (CC BY). The use, distribution or reproduction in other forums is permitted, provided the original author(s) and the copyright owner(s) are credited and that the original publication in this journal is cited, in accordance with accepted academic practice. No use, distribution or reproduction is permitted which does not comply with these terms.
*Correspondence: Jorge González, am9yZ2UuZ29uemFsZXpAdWFudG9mLmNs
†These authors have contributed equally to this work
‡ORCID: Juan San Francisco, orcid.org/0000-0002-1313-5014
José Luis Vega, orcid.org/0000-0001-9333-8729
Leonard J. Foster, orcid.org/0000-0001-8551-4817
Rafael F. de Almeida, orcid.org/0000-0002-6064-1876
Alexis M. Kalergis, orcid.org/0000-0001-7622-5263
Jorge González, orcid.org/0000-0003-3719-944X