- 1Max Planck Institute for Meteorology, Hamburg, Germany
- 2NSF National Center for Atmospheric Research, Boulder, CO, United States
- 3Center für Erdsystemforschung und Nachhaltigkeit, University of Hamburg, Hamburg, Germany
- 4Faculty of Environment, Science and Economy, University of Exeter, Exeter, United Kingdom
- 5Laboratoire de Météorologie Dynamique, Institut Pierre-Simon Laplace, CNRS, Ecole Normale Supérieure, Université PSL, Sorbonne Université, Ecole Polytechnique, Paris, France
- 6School of GeoSciences, University of Edinburgh, Scotland, United Kingdom
- 7Department of the Geophysical Sciences, The University of Chicago, Chicago, IL, United States
- 8Department of Physics, University of Auckland, Auckland, New Zealand
- 9Centro de Investigaciones del Mar y la Atmósfera (CIMA)/Universidad de Buenos Aires-CONICET, Buenos Aires, Argentina
- 10Georges Lemaître Center for Earth and Climate Research, Université Catholique de Louvain, Louvain-la-Neuve, Belgium
- 11Institute for Climate, Energy and Disaster Solutions, The Australian National University, Canberra, ACT, Australia
- 12Department of Computer Science and School of the Environment, University of Toronto, Toronto, ON, Canada
- 13Program in Science, Technology, and Society, Center for International Security and Cooperation, Stanford University, Stanford, CA, United States
- 14GERICS Climate Service Center Germany, Helmholtz-Zentrum Hereon, Hamburg, Germany
- 15Department of Earth and Environmental Science, University of Pennsylvania, Philadelphia, PA, United States
- 16Laboratoire des Sciences du Climat et de l’Environnement (UMR CEA-CNRS-UVSQ 8212), Université Paris Saclay et Institut Pierre Simon Laplace, Paris, France
- 17NASA Goddard Institute for Space Studies, New York, NY, United States
- 18School of Animal, Plant and Environmental Sciences, University of Witwatersrand, Johannesburg, South Africa
- 19Climate and Environmental Physics, Physics Institute, Oeschger Centre for Climate Change Research, University of Bern, Bern, Switzerland
- 20GEOMAR Helmholtz Centre for Ocean Research Kiel and Kiel University, Kiel, Germany
- 21Laboratory of Atmospheric Science and Geophysical Fluid Dynamics (LASG), Institute of Atmospheric Physics (IAP), Chinese Academy of Sciences, Beijing, China
Knowledge of the functioning of the climate system, including the physical, dynamical and biogeochemical feedback processes expected to occur in response to anthropogenic climate forcing, has increased substantially over recent decades. Today, climate science is at a crossroads, with new and urgent demands arising from the needs of society to deal with future climate change, and the need for the climate science community to refine its strategic goals to meet these demands rapidly. All possible—but currently unknown—worlds in 2050, with a larger global population, unprecedented climate conditions with higher temperatures, more frequent extreme weather events, sea level rise, disrupted ecosystems, changes in habitability and increased climate-induced displacement and migration, and the emergence of new geopolitical tensions, will require limiting society’s vulnerability both through mitigation measures to minimize further warming and through the implementation of innovative adaptation initiatives. The development of a skillful climate information system, based on the most advanced Earth system science, will be required to inform decision-makers and the public around the world about the local and remote impacts of climate change, and guide them in optimizing their adaptation and mitigation agendas. This information will also help manage renewable resources in a warmer world and strengthen resilience to the expected interconnected impacts of climate change. In this paper, we summarize the major advances needed to understand the multiscale dynamics of the Earth system. We highlight the need to develop an integrated information system accessible to decision-makers and citizens in all parts of the world, and present some of the key scientific questions that need to be addressed to inform decisions on mitigation and adaptation. Finally, we speculate about the values and ethics of climate science and the nature of climate research in a world that will be increasingly affected by global warming in a geopolitical context very different from that of recent decades.
1 Introduction
Climate science has evolved steadily over the past century, driven initially by attempts to observe and understand the atmosphere, land surface, ocean, and cryosphere, and to explain observed natural variability on a range of time scales, from interannual to glacial cycles. Through these efforts, Arrhenius (1896) discovered in the late 19th century, that the release of carbon dioxide in the atmosphere would lead to an increase in the Earth’s surface temperature. By using principles of physical chemistry to explain part of the greenhouse effect in the Earth’s atmosphere, Arrhenius’ work played an important role in establishing modern climate science. Since then, meticulous measurements of atmospheric CO2 concentrations, beginning in 1957, have quantified a long-term increase caused by the burning of fossil fuels. The key insight that these rising CO2 levels would eventually lead to a warming of the troposphere and an associated cooling of the stratosphere was confirmed by the development of the first climate models, a breakthrough recently recognized by the 2021 Nobel Prize in Physics. Prior to this recent recognition, advances in climate sciences led to the well-known “Charney Report” (Charney et al., 1979), the first scientific consensus report that used the then state-of-the-art scientific models and perspectives to establish a rough quantitative scientific consensus on the role of observed carbon dioxide increases in expected anthropogenic climate change. The report summarized the state of the art, but also highlighted gaps in knowledge. It was the latter that subsequently triggered a series of consequential international climate conferences and policy interactions at the UN level. In particular, the first World Climate Conference, organized by the World Meteorological Organization (WMO), led to the establishment of the World Climate Research Program (WCRP)1 in 1980. The Intergovernmental Panel on Climate Change (IPCC) and the Global Climate Observing System (GCOS) program were established at the second World Climate Conference a decade later. The United Nations Framework Convention on Climate Change (UNFCCC) began its work in 1992. The intensification of climate-related impacts over recent decades has highlighted the need for the co-construction of knowledge and actionable and trustworthy climate information, leading in 2009 to the creation of the Global Framework for Climate Services (GFCS)2 during the WMO Third World Climate Conference.
The WCRP originated as a joint research initiative of the World Meteorological Organization and the International Council of Scientific Unions (ICSU), now the International Science Council (ISC); in the 1990s UNESCO’s Intergovernmental Oceanographic Commission (IOC) joined as a third co-sponsor. At its inception, the WCRP was established to coordinate international research to advance scientific knowledge of the climate system and to use this knowledge to quantify climate variability and predictability. It was also intended to identify and mitigate possible human-induced climate changes that could affect the well-being of humankind. Since then, the organization has played a central role in organizing and coordinating major international climate research projects and experiments that are too large for any single nation to support.
Tremendous progress has been made over the last decades by the climate research community, coordinated and stimulated by WCRP, in understanding the fundamental processes associated with the multiscale dynamics of the Earth system, particularly the physical and biogeochemical interactions between the atmosphere, ocean, hydrology, continental biosphere, and cryosphere. Early examples include the Tropical Ocean/Global Atmosphere Project (TOGA, 1985–1994) and the World Ocean Circulation Experiment (WOCE, 1990–2002), which focused on natural variability (interannual time scales) and the role of the ocean. The list of subsequent projects and achievements is long and covers the entire climate system, from the atmosphere to the ocean, the cryosphere, the land surface and their interactions. The development of improved observations, simulations and projections using advanced Earth system models, and the implementation of detection and attribution methods have made it possible to document the emergence of climate change signals in the context of natural climate variability.
Fundamental to the success of the WCRP has been the development of the necessary infrastructure, including a global climate observing system and coupled climate and Earth system models. In particular, the WCRP’s Climate Model Intercomparison Project (CMIP) assessments of climate model performance and projections of anthropogenic climate change are a major methodological innovation in computational science. Climate and Earth system models vary along many dimensions, from their dynamical cores to model grids to data exchange between sub-models; by definition, none are perfect representations of the real Earth; all have biases; and because of their complexity, it is difficult to know if and when a model result is right for the wrong reasons. Therefore, in addition to a comprehensive climate modeling system, a suite of climate observations is needed to test models through model-observational comparison studies, while improving our understanding of climate processes, identifying robust climate dynamics and feedbacks at play, and providing robust forecasts and projections of the state of the climate system under prescribed emission scenarios.
In 1992, the United Nations Framework Convention on Climate Change (UNFCCC) called for a stabilization of greenhouse gas concentrations in the atmosphere at a level that would prevent dangerous anthropogenic interference with the climate system. The climate knowledge produced by the international scientific community, and subsequently assessed by the IPCC, led to the recognition by 196 nations in 2013 that the “human influence on the climate system is clear” (IPCC, Climate Change, 2013). The vulnerability of natural and human systems to climate change and the urgent need to ensure a sustainable development of the planet convinced the international community that global warming should be limited to 2°C or less (2015 Paris Agreement). The agreement requires a fundamental change in the direction of climate research, calling for a shift to address society’s pressing knowledge and information needs. The WCRP’s renewed vision, as summarized in the latest WCRP Strategic Plan (WCRP, 2019), and the recently published WCRP Science and Implementation Plan (WCRP, 2020), reflects this evolving mission. It is based on a broad and holistic perspective and calls for the development of active partnerships among groups involved in Earth system research with the aim of providing relevant information to society. Earth system science views the planet as a complex and interactive system with physical, biological and chemical interactions between the atmosphere, the hydrosphere (ocean, lakes, rivers), the cryosphere (ice, frozen soil and snow), the lithosphere (land and geological processes) and the biosphere (life, ecosystems, biodiversity).
The development of Earth system models (Flato, 2011) that represent the coupled climate system and global carbon cycle has revealed the strong linear relationship between cumulative human emissions of CO₂ and global surface temperature increase since pre-industrial times (Allen et al., 2009; Matthews and Caldeira, 2008) as well as the lack of significant warming or cooling after CO₂ emissions cease (Matthews and Caldeira, 2008; Solomon et al., 2009; MacDougall et al., 2020). The relationship between CO₂ emissions and global warming is characterized by a metric, TCRE—the Transient Climate Response to cumulative CO2 emissions—and has been estimated in the IPCC AR6 (IPCC, 2021) to be 0.45°C per 1,000 Gt CO2, with a likely range of 0.27–0.63°C (IPCC, 2021). These new findings also led to the concept of the “remaining carbon budget,” first introduced in the IPCC AR5 (IPCC, Climate Change, 2013). The most recent estimate of the remaining carbon budget is about 200 billion tons of CO₂ for a 50% chance of limiting warming to below 1.5°C (Forster et al., 2024), equivalent to about 5 years of current CO₂ emissions (Friedlingstein et al., 2023). This underscores, more than any other climate science finding, the urgency and ambition of climate action if the temperature limits set by the Paris Agreement are to be met.
Advancing our fundamental understanding of the Earth system remains critical, but climate research must also prioritize the generation of societally relevant and actionable information that directly supports mitigation and adaptation strategies, and avoids inadequate responses such as maladaptation. Beyond simply generating knowledge, climate science must now provide society with the tools and frameworks needed to promote a sustainable future for humanity and all life on Earth. This means applying climate knowledge and implementing climate services now and, in the future, to support resilience, ecosystem restoration, and human well-being in the face of climate challenges.
The purpose of this paper is to provide an overview of current climate research priorities, highlighting major advances and emerging frontiers. More fundamentally, the paper aims to identify the directions in which climate research needs to advance in order to enable global societies to effectively manage the impacts of global warming, to reduce the escalation of potential impacts of climate change and to ultimately halt global warming. This synthesis will also consider the urgency of action needed to limit temperature rise to well below 2°C and as close as possible to 1.5°C, which requires global decarbonization and acceleration of mitigation within the next two decades. The paper includes a discussion of current scientific questions and current approaches to addressing these questions (Section 2). The directions of climate research for the next 1–2 decades are described in Section 3. The need to develop climate information services is emphasized in Section 4. Concluding remarks and speculation about the future of climate science in a world that may face us in 50 to a hundred years are provided in Section 5.
2 Current climate research
The increasingly rapid human-induced changes in the Earth’s climate have led to profound and widespread changes in the atmosphere, ocean, cryosphere, and biosphere. Recent work produced by several scientific institutions around the world and often coordinated by the WCRP has greatly improved our understanding of the dynamics of variability, feedback mechanisms, and teleconnections in and between the atmosphere, ocean, and cryosphere, and more generally of rapid or irreversible changes and extremes in the climate system (see, e.g., Ghil and Lucarini, 2020; Wunderling et al., 2024 and the different IPCC reports through which knowledge propagated). Significant progress has been made in quantifying changes in the Earth’s energy budget, in understanding the drivers of changes in precipitation, in the global carbon cycle, and in biogeochemical feedback loops under a changing climate. Finally, several key indicators of the state of the climate system are now better observed and projected.
The increase in the frequency and intensity of extremes, reaching unprecedented magnitudes for several recent weather and climate events (heatwaves, droughts, storms, extreme rainfall and flood, intense tropical cyclones, floods, wildfires) and the remarkable spike in 2023/2024 (e.g., Samset et al., 2024; Cheng et al., 2024; Cattiaux et al., 2024; Raghuraman et al., 2024) in global surface temperature illustrates the complexity (nonlinearity) and interconnectedness of the climate system. Raghuraman et al. (2024) call for a comprehensive systemic understanding, and underscore the urgent need to develop sustainable solutions based on an understanding of the Earth’s climate as an integrated system. Clearly, science is needed to inform the responses to the complex risks arising from the inevitable impacts of climate change that are already being experienced today, and the unavoidable future impacts that are already being committed.
The uptake of anthropogenic heat into the Earth system, overwhelmingly into the global ocean, results in the thermal expansion of the ocean. Furthermore, ocean mass increases due to the melting of glaciers and polar ice sheets. Both effects have led to an acceleration of sea level rise from about 2 mm per year in the 1990s to more than 4 mm per year in the last decade (Hamlington et al., 2024). Sea level rise of up to 80–100 cm is projected by the end of the century, with large uncertainties arising mainly from emission scenarios and the related global warming levels. In addition, there are larger uncertainties at the upper end due to highly uncertain processes in the polar ice sheets, which could lead to an accelerated rate of rise during this century and beyond, if instability is triggered under increased global warming, and to several more meters in subsequent centuries (Van der Wal et al., 2022).
The current approach to climate science rests on three pillars: (1) observations (including retrospective observations from historical sources and paleoclimate archives), (2) theory (understanding) and modeling, and (3) the generation of relevant information, e.g., through climate services. Together, these three pillars have developed complementary approaches to detect, simulate and understand changes in the climate system. They form the basis for the required information system.
2.1 Observations
Currently, a wealth of climate observations is being obtained from a variety of platforms, including ground-based, ship-based, and satellite-based remote sensing, coordinated by the Global Climate Observing System (GCOS) or the Global Ocean Observing System (GOOS), as well as an autonomous measuring network (Argo). These observations, analyzed in terms of climate processes, climate variability, and climate trends, have been the basis for quantifying the signals of climate change, including, but not limited to, global mean warming, Arctic amplification, Arctic Sea ice loss, and sea level rise. They provide one of the strongest lines of evidence for anthropogenic climate change.
A wide range of observations are also combined with numerical circulation models in a synthesis step to produce quantitative estimates of the changing climate. Examples are atmospheric reanalyzes, which combine observations with numerical atmospheric prediction models to provide a 4-dimensional evolution of the atmosphere and surface fields over the modern era (roughly since about 1950). Atmospheric reanalyzes have also been extended into the past, but with fewer observational constraints, mainly surface pressure fields (e.g., Compo et al., 2005). Similar work is ongoing in the ocean and is now being extended to the coupled Earth system. Community-based assessments of atmospheric and oceanic reanalyzes have revealed differences, particularly in their trends and variability during the satellite era (1980 to present) and have suggested the use of a reanalysis ensemble rather than deterministic reanalyzes (see Dee et al., 2011; Gelaro et al., 2017; Kobayashi et al., 2015; Hersbach et al., 2020).
2.2 Theory and modeling
The 2021 Nobel Prize in Physics was awarded in part to Syukuro Manabe and Klaus Hasselmann “for the physical modeling of the Earth’s climate, the quantification of its variability, and the reliable prediction of global warming.” Manabe’s successful early projections of the response of global mean temperature and the vertical temperature distribution in the atmosphere to anthropogenic forcing, including Arctic amplification, quantification of climate variability, and Hasselmann’s fingerprinting of the effects of anthropogenic forcing in observations of that variability are a testament to our theoretical understanding of the climate system. Their legacy of using models across a hierarchy of physical complexity continues today as a fruitful way to understand the impacts of climate change. It underscores the importance of not only simulating the response to climate change using state-of-the-art climate models but also understanding the impacts of climate change (Held, 2005).
Climate model simulations allow us to examine the response of the climate system to different forcings, especially those associated with higher greenhouse gas concentrations (IPCC, 2021; IPCC, Climate Change, 2013; IPCC, Climate Change, 2007; IPCC, Climate Change, 2001). Together with impact models driven by climate model output, they assess the risk of climate change to natural and human systems and can be evaluated through simulations of past observed and reconstructed climate. Such projections are typically made under the WCRP’s CMIP protocol (Durack et al., 2017; IPCC, 2018). Carbon cycle modeling has also been instrumental in establishing the concept of the “remaining global carbon budget” as a key policy tool (IPCC, Climate Change, 2013). Advances are now being made in high-resolution modeling to simulate convection and scale interactions, with implications for the representation of global-regional scale interactions and the occurrence and intensity of local extremes. Progress is also being made in representing land use, water use, and urbanization on the land surface and in the hydrological cycle through the development of advanced Earth System Models (ESMs).
There has been an explosion of new techniques for combining paleoclimate evidence with climate models or other lines of evidence, leading to major advances in quantitative knowledge of long-term climate change and in the determination of climate sensitivity over the past two decades (Knutti et al., 2017). Progress has been made in understanding the characteristics of and changes in extreme events, with increasing confidence in changes in extreme heat and drought in some regions. Progress has also been made in understanding changes in extreme precipitation, although gaps remain. In particular, the combination of climate observations and climate models to improve climate predictions is lacking. Ongoing efforts focus on the initialization of seasonal to decadal predictions. However, better use of existing observations should lead to improvements in climate models through parameter estimation.
2.3 Generation of relevant information
To develop effective climate change strategies and policies, decision-makers need clear, actionable information to plan and prioritize resources for climate action on a regular basis. This information should include climate projections (e.g., projected changes in temperature and precipitation, expected changes in the frequency and intensity of extreme meteorological and hydrological events), insights into regional vulnerabilities (e.g., flood and drought risks), expected economic impacts (e.g., costs associated with investments in mitigation and adaptation strategies), sectoral impacts (e.g., effect of climate change on forests, agriculture, health), feasible options for reducing greenhouse gas emissions in different sectors of the economy, and guidance on building community resilience. Decision-makers also need legal and financial knowledge of the tools available to drive action, such as carbon pricing and renewable energy incentives.
Finally, paleoclimatic studies, specifically the reconstruction of past climate variations based on proxy records, have helped understand the complex behavior of the climate system, and are putting in perspective the scientific investigations on the potential impacts of human activities on tomorrow’s climate (Kaufman and Masson-Delmotte, 2024). Paleoclimatic studies have led to advances in the quantitative understanding of past climate variations (e.g., response of the earth system to natural forcing, analysis of a large spectrum of internal variability, characteristics of slow components of the climate system; empirical evidence for abrupt climate changes, e.g., Berger and Loutre, 2002; Yin et al., 2021). The confidence in climate models has improved through systematic comparisons with paleoclimate evidence. Paleoenvironmental information has allowed to better understand ecosystem adaptations and the limits to adaptation. The combination of paleoclimate evidence with process-based understanding has provided constrains for future climate projections, and specifically for the quantification of the climate sensitivity.
3 Climate research over the next decades
The success of the WCRP’s work to date in demonstrating that humans are influencing the climate, and the resulting political will to mitigate global warming, has raised new scientific questions about climate science that urgently need to be addressed, both with respect to mitigation (including decisions on mitigation pathways, e.g., short-lived forcers versus CO2) and to adaptation. More broadly, we need to deal with, and understand, a complex nonlinear system and its adaptation to a new climate state. This requires the adoption of a fully systemic approach to climate research.
The development of robust, relevant, actionable, and accurate climate information in support, for example, of the Sustainable Development Goals adopted by the United Nations in 2015, requires addressing several scientific questions of a fundamental nature. Without advances in the most fundamental aspects of our understanding of the climate system, limited progress will be made in providing the expected contributions to science-based decision making needed to maintain a sustainable future. Fundamental research together with the use of new technologies (e.g., observing systems, modeling, artificial intelligence, digital twins) and the development of products (e.g., data, projections, risk assessments, early warning systems) are the tools (Figure 1) that the scientific community will provide to address societal requirements. The following paragraphs provide examples of research questions for the future.
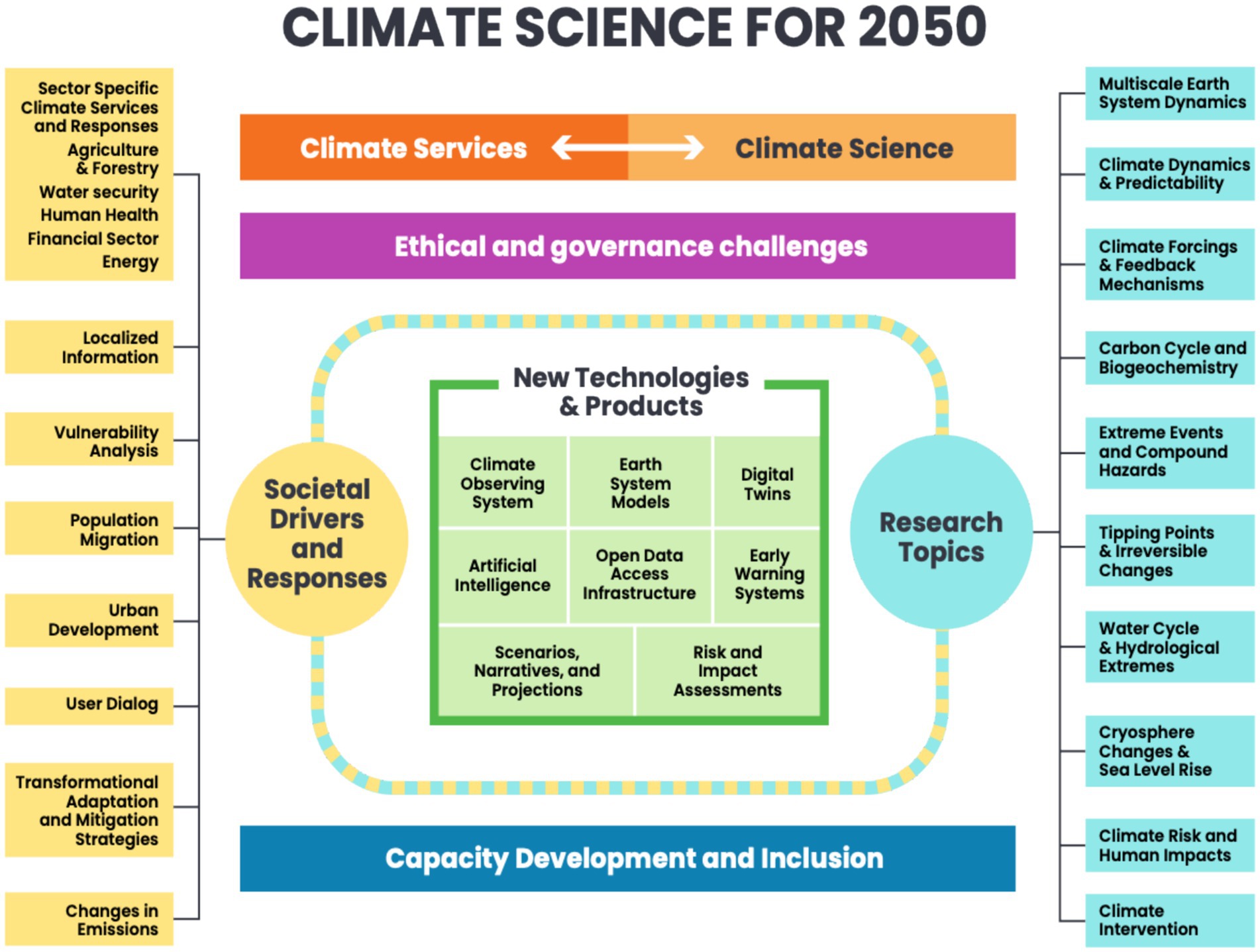
Figure 1. Schematic diagram showing the relations between fundamental climate research, the use of new technologies to develop climate products in support of societal requirements to respond to the challenge posed by present and future climate change.
3.1 Climate dynamics
Understand the complex and often non-linear and multi-scale interactions between the ocean, the atmosphere, the cryosphere and the land surface, including heat, water and carbon transports by each component and between them.
An understanding of future changes in atmospheric and oceanic circulation, and the role of internal variability and forcing—all critical aspects of regional climate change—is needed. In addition, a better understanding of the complex role of biogeochemical interactions in the Earth system leading to climate feedbacks at elevated temperatures is required. Climate and climate risk projections remain uncertain. A probabilistic treatment of climate risks in a future climate is therefore often not possible or misleading, requiring more detailed simulations of extremes with high-resolution models.
There is still a distinct lack of process understanding of the role of the ocean and land and their interactions with the atmosphere. However, focused field campaigns and monitoring efforts have been very useful in advancing the understanding of specific monsoon processes. Further coordinated work is urgently needed to improve the understanding of internal variability, tropical-extratropical interactions, ocean-land-atmosphere interactions, coastal ocean processes, eddies, jets, more accurate localization of extremes, storms, and monsoon systems on intra-seasonal time scales. As the need for further research on predictability at timescales from weeks to years will increase in the future, there is a need for a better understanding of the underlying natural variability, which is likely to change as the large-scale climate changes.
Cryospheric changes strongly interact with both the ocean and the atmosphere through freshwater input, polar heat release, polar heat and moisture advection, and deep-water formation. Permafrost thawing also strongly interacts with the carbon and methane cycles. These interactions need to be better understood for more comprehensive long-term climate projections, including better assessment of possible tipping points and irreversible changes. This includes a better understanding of dynamic ice-sheet processes, thresholds for nonlinear system responses in a warming world, and, in particular, an advanced understanding of ice-sheet instability processes associated with temperature thresholds and long-term committed changes. Achieving these advances poses the challenge of obtaining the necessary local observations at the ocean–atmosphere-cryosphere interfaces to quantify their interactions.
In short, a better understanding of the physical and biogeochemical interlinkages and interactions between the different components of the Earth system is required to fully characterize and predict the evolution of the multi-scale climate dynamics.
3.2 Climate forcing and feedback mechanisms
Understand and quantify the importance of feedback mechanisms.
Recent global temperature changes have highlighted the lack of a full system understanding of climate processes, and in particular the need to account for nonlinearities, unintended consequences, and feedbacks. Effective radiative forcing trends, driven by greenhouse gas (GHG) concentrations and actions that have reduced aerosol levels, are driving some of the increases in Earth’s energy changes.
The scenarios used to drive Earth system projections are one of the dominant sources of uncertainty in future climate characteristics beyond 2040. These scenarios are typically developed on the timescale of IPCC assessment cycles. However, anthropogenic forcing evolves over time. In particular, the Covid-19 pandemic led to a 5.4% decrease in CO2 emissions (Laughner et al., 2021), and recent estimates suggest that we are currently following the RCP 4.5 scenario (Hausfather, 2023). Climate forcing by methane and nitrous oxide also lie along high emission scenarios. In addition, anthropogenic aerosols have changed globally, especially in the northern hemisphere as a result of improved air quality measures, and have significant impacts on regional climate. Large uncertainties also remain about land and ocean carbon sinks, despite important recent advances in knowledge.
Updating scenarios to account for the evolving nature of the forcing will be necessary to ensure a diversity of projections. The operationalization of anthropogenic forcing is a promising strategy to address this scientific gap. The activities and model intercomparisons planned for CMIP7 begin to address this challenge (CMIP7 Forces Task Team and Regional Aerosol Model Intercomparison Project).
We need to better understand the sources, fate, and impacts of short-lived climate forcers, including methane, fluorinated gases, secondary organic aerosols, and tropospheric ozone. The issue is also important from an air quality perspective, as emissions of anthropogenic gases such as nitrogen oxides, sulfur dioxide, carbon monoxide, and volatile organic compounds are a source of secondary pollutants, including ozone and aerosol particles, with adverse health effects. Cloud feedbacks remain uncertain; some of this uncertainty is due to changes in the water content of high clouds, as well as phase changes. There are strong reasons to expect some climate dependence of climate sensitivity; for example, the ice-albedo feedback changes as ice melts.
The direct effects of clouds and so-called indirect effects from aerosols remain a major issue for climate models. Recent studies suggesting that ship emissions abatement may have reduced cloudiness over the North Atlantic, causing the warming observed in 2023, but there is a lack of sufficient data to determine how large this effect was (e.g., Hansen et al., 2023; Hodnebrog et al., 2024; Schmidt, 2024) or if the observed warming reflected internal sea surface variability (Samset et al., 2024). In the North Pacific, China’s aerosol reduction from 2010 to 2020 plays a role (Wang et al., 2024). Cloud feedbacks, considered positive in AR6, vary spatially, uncertainties remain large, and there are still large differences between models. Changes in planetary albedo and low cloud feedbacks may have accelerated radiative forcing recently.
3.3 Carbon cycle
Develop comprehensive Earth System models to investigate carbon cycling processes through the atmosphere, the ocean and the biosphere and its responses to changing climate conditions.
Future Earth System Model (ESM) research will need to further improve understanding of the major global biogeochemical cycles and how they are affected by ongoing climate change. On land, improved representation of the nitrogen cycle is urgently needed (Arora et al., 2020). The ability of terrestrial ecosystems to continue to act as a strong carbon sink will depend on the evolution of soil nitrogen mineralization and availability for plant productivity. Climate change also increases the risk of severe droughts and the associated risk of increased wildfires, which are already leading to additional CO2 emissions, reduced forest cover, and potentially long-term changes in vegetation dynamics (Bowman et al., 2009; Flannigan et al., 2009). In this regard, there is a need to better understand the decadal effects of wildfires taking into account regrowth. Clearly, uncertainties in the modeled water cycle influence, and interact with, uncertainties in the carbon cycle. Better representation of permafrost and abrupt thaw will also be needed in future ESMs, as ecosystems contain large below-ground organic carbon stocks that could be destabilized under warming conditions (Koven et al., 2011; Schuur et al., 2022). A new frontier is to map the role of microbes in soils, including permafrost, and in the ocean.
On the ocean side, the representation of marine biology and its response to climate change, including the limits of the adaptive capacity of phytoplankton to warming temperatures, will need to be improved in ESMs (Sauterey et al., 2023). We need to be aware that climate change affects the entire ocean circulation, with complex consequences for the ocean’s transport properties, but also for the ocean’s heat and carbon cycle affecting the ocean’s role as a heat and carbon sink relative to the atmosphere. In addition, the combination of extreme marine heat waves with ocean acidification and other extreme events in the ocean can have severe impacts on marine life (e.g., Romanou et al., 2024). The role of coastal oceans in this complex interplay is not yet fully understood. Anticipating the interplay between changes in ocean dynamics from small-scale eddies and storms to the large-scale gyre and meridional overturning circulation and vertical stratification, as well as their role in nutrient and light limitation of phytoplankton growth and their impact on the ocean’s carbon sink, must be adequately represented in ESMs and assessed against available observations from international programs such as the GOOS.
3.4 Land use and land degradation
Develop advanced models to describe change in land use and land cover in relation to climate change.
Understanding land use and land degradation is important because of its impact on climate through water and energy fluxes, and the significant socio-economic and ecological consequences, and ultimately food and water security. Despite research efforts to assess land use change and its impacts on carbon, nitrogen, energy and water fluxes, fundamental challenges remain, particularly in understanding the trade-offs and co-benefits of sustainable land management practices, including those related to carbon sequestration and sustainable agriculture. There is a growing need for more advanced models of land use and land cover change. Among the problems to be addressed are the responses of soil carbon and microbial processes to climate warming, changes in soil moisture, changes in plant organic matter inputs, acclimation processes of photosynthesis and growth to concurrent changes in temperature and atmospheric CO2 concentrations, and the likelihood of wildfire episodes. An improved quantitative understanding of soil ecosystem processes will be critical for future projections of land-climate interactions and feedbacks.
Progress on land-use change issues and land-based climate solutions at local to global scales will inform effective climate mitigation and adaptation strategies. The link between weather-related renewable water resources and the way these resources are managed is driven by economic considerations and processes. How water is valued by societies (monetary or regulatory value) is critical.
Ensuring food security for a growing global population requires healthy land resources and flourishing ecosystems. Yet our current agricultural practices are causing soils worldwide to be eroded up to 100 times faster than natural processes replenish them. At least 70%of all ice-free land has been altered, impacting over 3.2 billion people. At current rates, 90% of land will bear our imprint by 2050. The impacts of land degradation will be felt by most of the world’s population. Land degradation also changes and disrupts rainfall patterns, exacerbates extreme weather like droughts or floods, and drives further climate change. It results in social and political instability, which drives poverty, conflict, and migration.
Land degradation neutrality can halt, and then reverse, this alarming picture of the future. To achieve this objective, it is important (1) to avoid new land degradation, (2) to reduce existing degradation by adopting sustainable land management practices that should increase biodiversity, soil health, and food production; and (3) to ramp up efforts to restore and return degraded lands to a natural or more productive state. These actions represent a major opportunity to contribute to sustainable development by scaling up good practices and pilot activities through large-scale transformative projects and programs. These initiatives can generate multiple benefits, including positive changes in human well-being, poverty alleviation, and the restoration of terrestrial ecosystems and their services.
3.5 Extreme events
Project frequency and intensity of extreme weather events (hurricanes, storms, heatwaves, droughts, flooding, extreme sea level events, marine heatwaves, compound—cascading events) in response to climate change.
Extreme weather and climate events, such as heatwaves, floods, and droughts, have catastrophic environmental, economic, and social impacts, resulting in fatalities and monetary losses, and affecting the socio-economic stability of regions, sometimes for decades (e.g., Young and Hsiang, 2024). In addition to improved monitoring and information on these events, a better understanding of extremes and their role in global climate change is needed.
Observed and projected changes in extremes are highly localized and vary widely from region to region. As the intensity of precipitation and heat-related extremes will continue to increase under anthropogenic climate change, their estimation is important. However, large uncertainties arise from the inadequate quality and sampling of observational data. Uncertainties also result from insufficient resolution of model simulations. State-of-the-art models, due partly to resolution limitations, fail to fully capture Rossby wave behavior (e.g., quasi-resonant amplification) implicated in many of the persistent summer weather extremes in the Northern Hemisphere in recent years. The incidence of this phenomenon is likely increasing in occurrence due to human-caused warming (Guimaraes et al., 2024). Limited model resolution also makes it difficult to apply advanced statistical methodologies to estimate climate extremes. Furthermore, advanced statistical methodologies, including artificial intelligence, for estimating climate extremes have not yet been exploited to the full extent and require validation and refinement. Effective comparisons and cross-validation of extremes between estimates derived from observational point data and model gridded outputs is therefore very difficult to perform. Substantial efforts are needed to improve our ability to characterize climate risks, particularly those related to the carbon cycle’s response to climate change and the potential impacts of tipping points as land-vegetation-carbon cycle interactions are not presently captured in their full complexity in climate models, with many processes and interactions missing (Canadell and Jackson, 2021).
Understanding how precipitation patterns and extremes evolve in a warming climate is crucial. Increased heating leads to greater evapotranspiration and thus surface drying, thereby increasing intensity and duration of drought. However, the water holding capacity of air increases by about 7% per 1°C warming, which leads to increased water vapor in the atmosphere. Thus, storms, whether isolated thunderstorms, extratropical rain, hail or snowstorms, or tropical cyclones and hurricanes, fueled by increased moisture, are producing the more intense precipitation events that are widely observed to be occurring, even in places where annual precipitation is decreasing (Trenberth, 2011; IPCC, 2022). In turn, the increase in heavy precipitation is expected to increase the risk of flooding when natural and man-made drainage capacity is overwhelmed and when land use changes (urbanization, agricultural practices) increase surface runoff. Hydrological responses to changes in precipitation and evaporation are complex and vary between regions and catchment types. Under some circumstances, the hydrological system can amplify changes to the driving climate variables (primarily precipitation). For example, in many mountainous regions, because more precipitation occurs as rain instead of snow with warming, and snow melts earlier, there is increased runoff and risk of flooding in early spring, but increased risk of low river flows and/or drought in deep summer, especially over interior continental areas. However, with more precipitation per unit of upward motion in the atmosphere, the atmospheric circulation weakens, causing monsoons to falter. In the tropics and subtropics, strong patterns of precipitation are dominated by shifts as sea surface temperatures change; El Niño is a good example. A better understanding of changes in sea surface temperatures (SSTs) patterns and their relation to changes in the atmospheric circulation is critical to advance improved predictions of extreme precipitation patterns.
Flood risk management and the design of flood protection systems are almost exclusively based on the observed historical record and inferred rates of return of extreme precipitation and flood events even though well-understood climate physics highlights an increase in heavy rainfall affecting both the likelihood of occurrence, return time, and intensity of current and future events. Guidance is urgently needed in this area; floods are one of the world’s most damaging and dangerous natural hazards, with major populations and assets at risk. Similarly, the water systems developed in the 20th century to provide reliable and clean water for the world’s major cities were based on estimates of the inter-seasonal and inter-annual variability derived from observations of the water sources (primarily streamflow) that supplied these water systems. Constraints in water resources are also affected by evapotranspiration.
Processes occur at many time scales ranging from multi-decadal variability down to the timescales associated with individual cumulus cloud formation. El Niño variations, for example, occur over broad areas of the Pacific Ocean on a 2-7-year time cycle, while the regulation of local tropical SSTs appears to be related to feedbacks between evaporation, clouds, and solar radiation on much smaller time scales of days to weeks.
3.6 Tipping points
Identify possible abrupt and irreversible changes (e.g., Amazon, Antarctica), evaluate metrics to monitor critical climate system components prone to tipping, so-called tipping elements, and develop physics-based early warning systems.
The Earth System may respond nonlinearly to anthropogenic climate change by transitioning to different long-term states with important consequences for human society. Tipping elements are a paleoclimatic fact (Lenton et al., 2008). Tipping elements are the components of the Earth System that may respond nonlinearly to climate forcing, and tipping points refer to critical transition thresholds. Possible tipping elements include the slowing or collapse of the Atlantic Meridional Overturning Circulation (AMOC), the destabilization of marine methane hydrate deposits, the multi-meter sea level rise from the loss of the large Greenland and Antarctic ice sheets, the release of carbon from thawing permafrost, large-scale shifts in boreal forest ecosystems, the disruption of tropical seasonal monsoons, the possible collapse of the Amazon forest affected by both drought and deforestation (Wang et al., 2023). Tipping elements could dynamically interact with each other, producing compounding effects and even triggering a cascade with the potential to alter Earth’s long-term climate trajectory on geologic timescales (Lenton et al., 2019; Steffen et al., 2018). However, there are gaps in model knowledge regarding the conditions under which these critical transitions could occur. An emerging issue is related to regional tipping points, such as shifts in monsoon systems, changes in ocean upwelling systems, or ecosystem disturbances/biodiversity loss due to disappearance of Arctic and Antarctic Sea ice, coral loss, loss of extra-polar mountain glaciers, changes in cloud cover, etc. Understanding the mechanisms and assessing the risks of regional tipping points will require the next generation of climate models, with much higher resolution, more extensive coupling, and better resolved processes. Tipping points may not only be associated with climate phenomena, but also with changes in human behavior.
3.7 Water and water management in the earth system
The vital role of water in all phases is clear, yet for many aspects, observations are not available to adequately determine the long-term mean, let alone the variability and changes over time. This is especially true for clouds, precipitation and evaporation.
There is a need to develop integrated hydrological studies based on observations and models to investigate the water cycle through the atmosphere, ocean, cryosphere and biosphere, but also through the soil/ground. Such studies can provide new insights needed to understand the profound consequences of climate change on water, and water-related model capabilities and deficiencies are particularly important for water managers. Understanding the role of groundwater storage and flow in this overall consideration of the general water cycle is essential but has been largely neglected in the past.
Various products representing evaporation, including reanalyzes and in-situ analyses using bulk formulas, do not agree (Trenberth and Fasullo, 2013; Cheng et al., 2020). Precipitation data sets remain inadequate. A goal of having at least hourly observations of precipitation has been nominally achieved, but uncertainties over the oceans and some land areas, especially in mountainous terrain, remain too large for reliable use. On land, the complexity of topography and land cover limits the ability to properly assess percolation and runoff, affecting soil moisture estimates.
Relatively high concentration combined with strong absorption in the infrared portion of the spectrum makes water vapor the most important greenhouse gas in the atmosphere. The complex energy balance is restored by evaporative cooling at the surface and subsequent heating in the atmosphere by condensation of water vapor but is further complicated by cloud formation.
Most reservoir system design and operation studies are based on experience and assumptions of statistical stationarity, with little or no consideration of causality, variability, and trends in the climate system. There is a great need to develop the ability to predict variability in the climate system at seasonal to decadal timescales or water resources applications. This is partly because models are often unable to reconstruct past trends convincingly, and because model spatial resolution is often insufficient to meet the needs of hydrologic applications.
Climate model precipitation is often tuned to get the monthly amount roughly right to meet energy constraints, but frequency, duration, magnitude, intensity, time of day, and type remain major challenges (Trenberth et al., 2017). Climate model errors in the location of key features such as the ITCZ and monsoon rains, as well as regions of ocean boundary currents, upwelling and down welling systems that influence surface temperature patterns, can be large because the features are in the wrong location and do not have the correct annual cycle. In most models, precipitation occurs too early, too often, and with insufficient intensity, resulting in recycling that is too large and a lifetime of moisture in the atmosphere that is too short. These biases in turn affect runoff and soil moisture. High-resolution cloud-resolving models improve some of the precipitation characteristics and may point the way forward, but they cannot yet be run globally for the long times required for climate purposes. Improved parameterizations are also needed.
Many models do not conserve water mass, and the ocean is often treated as an infinite source of water. The temperature and energy in precipitation are often not properly accounted for, and it remains a challenge to properly represent runoff, stream and river flows, and the entry of fresh but possibly polluted water into the ocean. This affects ocean salinity and density, and perturbations in circulation systems and associated sea surface temperature patterns feed back into the atmosphere.
Continued focus on high-quality, high-resolution observational data in space and time, along with appropriate climate model output, will highlight model shortcomings and point to where developments are needed, both in terms of higher model resolutions and improved parameterizations (e.g., Gettelman et al., 2024).
3.8 Research on geoengineering/climate intervention
Investigate the potential impacts, long-term risks, unintended consequences and the ethical aspects of geoengineering/climate intervention.
Geoengineering research, sometimes referred to as “climate intervention,” is framed as relevant to explore alternative approaches to complement adaptation and mitigation, and to offset some of the consequences of greenhouse gas-induced climate change (Hurrell et al., 2024). However, the proposed manipulation of the Earth’s climate on a global scale to counteract the effects of global warming raises many ethical, scientific, technical and governance issues. In a context where private interests are investing in related research, there is a need to inform public deliberations with scientific research that examines the proposed ideas, their scientific robustness, their potential efficacy and limitations in use, and the direct or unintended risks of negative impacts—with respect to deliberate modification of the Earth’s energy balance/solar radiation, other large-scale deliberate modifications of components of the climate system (e.g., ice sheets, ocean biogeochemistry, etc.), as well as large-scale removal of carbon dioxide.
Among the issues to be addressed are the effectiveness and scalability of the proposed methods, the potential environmental impacts, including the possibility of regional climate disruption, damage to ecosystems, their functions and services, loss of biodiversity, the ecological risks of using techniques such as ocean fertilization or carbon sequestration, the risk of fundamentally interfering with the dynamics of chemical processes in the upper atmosphere, the termination problem, the time scale of expected deployment and discernible effects, as well as the committed duration of deployment and related requirements (energy, resources, water, land.) as well as the integrated costs of these approaches. The latter must necessarily consider the costs of loss and damage caused by inadequate or vested interests, or their appeal to vested interests, and the consequences of reckless delays, lack of reduction of greenhouse gas emissions.
Studies of climate intervention must address ethical challenges (Preston, 2013), the international and political dimensions of the problem, aspects related to power relations and embedded values, new risks of conflict, and issues of equity and justice, and finally the intergenerational commitment and consequences of postponing addressing and resolving the root problem of anthropogenic climate change, i.e., the continued use of fossil fuels and deforestation.
4 Development of climate technologies, products and information services
In recent decades, technology has made tremendous progress, greatly improving our ability to observe the Earth system and to model/project its evolution through improved climate models. Climate models, together with observations, are fundamental tools for the development of a science-based climate information system. They are designed to assimilate measurements, facilitate data analysis and interpretation, and can be used to make climate projections.
Technology is also opening new horizons for the design and development of a comprehensive information system, suitable to support decision making. Access to information should be easily available to different actors in society and ultimately to every citizen of the world.
4.1 Climate observing system
Near real-time monitoring of the state of the climate system and its natural and anthropogenic forcing, the development of operational capabilities to attribute and predict emerging changes, and the establishment of a global climate information system (Trenberth et al., 2016) are opportunities that are within reach. A sustained climate observing system, covering all essential climate variables as defined by GCOS and GOOS, will continue to be an essential backbone for such an approach. This includes in situ and remote sensing elements for all climate components, be it atmosphere, ocean, cryosphere or land surface. Soil moisture and subsurface water flow are also essential variables to be covered in this context. New opportunities to fill observation gaps and build sustainable observation systems must be considered. For example, new satellite missions will provide open access to global data for all. Improved oceanic and terrestrial carbon estimates, improved process representations in land surface models, and satellite observational records are greatly enhancing our understanding of the Earth system. Bringing all observations together to improve understanding, monitor change, and improve climate models are all challenges that need to be addressed to advance climate services through the provision of improved information. Data rescue and the exploration of proxy information will also have to play a key role, as will the use of paleo-reconstructions of the pre-instrumental climate record to better understand long-term climate variability through Earth system reanalyzes.
4.2 Climate and earth system models
The current generation of Earth system models operates at a coarse resolution and does not simulate small-scale processes with high fidelity. This resolution limitation has several implications. First, it affects the ability to accurately project extreme events and identify potential thresholds in the climate system that involve small-scale processes. In addition, it does not provide accurate and actionable information at the scale of interest to decision makers. Increasing the resolution of climate models to 1 km or better is therefore essential to better resolve processes such as cloud dynamics, the hydrological and carbon cycles, or land surface processes such as glaciers or vegetation. Important ocean processes, biogeochemistry, and ecosystems, as well as ocean-cryosphere interactions, also operate at these small scales on land and in the ocean and need to be resolved in a full Earth system approach. Kilometer-scale models will greatly improve the realism of simulations and become available for broader applications. They will also be indispensable for early warning systems.
Society and practitioners seek trustworthy future climate information at the scale of cities and communities, typically between 1 and 10 km, but large ensembles of such simulations into the future at the kilometer scale are prohibitively expensive with currently available computing platforms. First, large ensembles of such simulations at ~25 km resolution are becoming available, already demonstrating the benefits of increased resolution in capturing extremes more accurately. All future high-resolution simulations must be open and accessible to all (Blanken et al., 2022; Stevens et al., 2024; Wilkinson et al., 2016). In addition, this information is needed not only on climate timescales, but actionable and usable information is needed on timescales of 5 to 20 years, requiring the development of multi-year to decadal predictions and an understanding of what is and is not predictable on these timescales. Another major challenge is the development of coupled regional models. The most relevant processes and interactions will differ by region, such as coastal versus inland communities, and urban versus rural environments, requiring different representations of coupled system processes.
Improving model simulations and the associated development of a climate information system requires a concerted effort to refine current models and incorporate new methodologies, while challenging them with observations and ensuring that climate models remain robust, relevant, and capable of meeting the pressing needs of society. Several issues need to be addressed simultaneously: spatial and temporal model resolution, model complexity, assimilation of observations, reanalysis of past climate and improved understanding of paleoclimate variability, and the need for large ensembles to sample climate variability, extremes, and remaining model uncertainties. These needs call for a strengthening of service initiatives with a greater focus on impacts and attribution of weather, climate and water events, with further integration across disciplines, including social and economic sciences. Global climate ensembles on the scale of a few kilometers will be required to meet these future demands for reliability and relevance. The digital revolution, increasing amounts of data, the growing role of the private sector, and the global transition to a zero-carbon economy and environmental services will be important factors in the coming decades.
The opportunities offered by quantum computing should be kept under observation. Machine learning and artificial intelligence are beginning to transform the value chain for integrated modeling, with global access to cloud computing potentially removing some of the barriers to exploiting large amounts of data (Jain et al., 2022). It is important, however, to avoid “black box” approaches and maintain parallel work on theoretical, physical-based understanding of climate processes, which is critical for the required confidence in climate projections.
4.3 Digital twins of the earth system
We consider digital twins of the Earth system to be advanced virtual models that accurately simulate the behavior of the Earth’s climate and ecosystems in real time. These digital replicas integrate vast amounts of data from multiple sources (e.g., satellites, sensors, historical records) and use sophisticated algorithms, artificial intelligence, and machine learning to provide detailed simulations and predictions of climate patterns, environmental changes, and human impacts on the planet.
In the context of climate adaptation, regional or local digital twins are particularly useful for scenario planning of adaptation strategies (Stevens et al., 2024; Richter, 2025). For example, a city planning to build flood protection can use a digital twin to simulate the effectiveness of different infrastructure projects under different climate change scenarios. This allows decision makers to choose the best course of action for climate adaptation based on solid data and projections. Cities are increasingly using digital twins to plan climate-resilient infrastructure. For example, they can simulate the impact of heat waves or rising temperatures on urban areas, helping planners design cooling strategies such as green roofs or new urban layouts that reduce heat exposure. These models also allow cities to optimize their energy and water systems for future climate conditions. Digital twins can also simulate how climate change will affect ecosystems, helping conservationists monitor endangered species and habitats. By predicting how specific regions will be affected, digital twins provide insight into which areas may need conservation efforts or more resilient agricultural practices. Issues related to the greening of cities and the reduction in urban air pollution (i.e., biogenic organic compounds, allergens) could also be addressed in a framework that accounts for the interfaces between physical, biological and chemical effects. A major challenge will be to represent the key interactions between the physical/biogeochemical systems with the social/human systems.
4.4 Artificial intelligence and machine learning
The quiet revolution in numerical weather prediction over the last few decades (Bauer et al., 2015) is giving way to the era of global kilometer-scale machine learning tools. Machine learning, an important new tool whose use in developing new modeling approaches, combining dynamical and statistical downscaling, and systematically addressing risk components, is becoming more widespread. Important developments are underway that use machine learning to improve and innovate parameterizations in the atmosphere and ocean. Together with other developments, they are leading to advances in research-based climate prediction, forecasting, and the development of early warning systems for climate extremes and hazards. Existing machine learning models are already beating conventional physically based weather forecast models in deterministic scores, for some variables but not others, and are much faster by several orders of magnitudes than physics-based models (Lam et al., 2023; Bi et al., 2023). However, we see limitations of the application of machine learning tools in using and building on existing knowledge and dynamical insights. Moreover, their application out of sample (e.g., for future climate or record-shattering events) remains a challenge. So, the community will always need to use machine learning tools as complementary approaches to numerical modeling, and theory. In addition, the performance of machine learning is always conditional on the availability of sufficient observations, reanalysis and their quality.
4.5 Information in support of effective strategies for adaptation and mitigation
Substantial climate science questions remain to be addressed for both mitigation decisions (including decisions on mitigation pathways, e.g., how best to articulate mitigation focused on CO2 and short-lived forcers) and adaptation decisions. This includes the detection and attribution of avoided emissions and the response to mitigation, which is complicated by the inertia of the climate system and strong climate variability, especially on interannual timescales (e.g., Tebaldi and Friedlingstein, 2013). Questions about losses and damages associated with climate change are increasingly being raised (see, e.g., Newman and Noy, 2023; Otto, 2023; Otto and Fabian, 2024). However, risks are also influenced by adaptation and vulnerability, and there is scientific debate about the extent to which attribution results are ready for litigation (King et al., 2023, 2024; see also Coumou et al., 2024). There are also important emerging questions about climate risk, which is difficult to quantify because many processes, such as vegetation die-offs and transitions, biosphere fire response and thresholds, and interactions of climate with society, are not well represented in our current modeling capabilities (Sherwood et al., 2024). Temperature thresholds occur in crops and ecosystems (including corals) associated with breaches of temperature tolerance or increased incidence of agricultural and ecological drought associated with increased evaporation (see IPCC, 2021). Even processes within climate physics, such as the retreat and melting of polar ice sheets or the thawing of permafrost, are difficult to quantify because of the challenges of modeling interactions across multiple scales and across climate system components. Climate risks associated with interactions within the Earth and climate systems and with society need to be better characterized, as they can lead to rapid changes, nonlinearities, and tipping points. Not all high impact events are low probability events (Sherwood et al., 2024), and early warning of rapid transitions or risks of high impact events is critical. To better characterize climate risk, new scientific tools and approaches need to be developed, as discussed in the following subsections.
4.6 Climate information for all
The United Nations Secretary-General announced in 2022 that he would lead a new effort to ensure that every person on Earth is protected by early warning systems within 5 years, now called “Early Warnings for All,” and invited the World Meteorological Organization (WMO) to lead this activity. While this announcement primarily addressed weather-related risks, it is also needed for climate-related impacts and should be expanded to include food, water and health. This in turn requires not only seamless forecasting of weather and climate change, but also the interaction of this information with managed and natural systems and society.
Any early warning system for climate impacts will be based on several pillars, all related to extremes, disasters, and risks: (1) information about what is happening, why it is happening, and what it means for the future; (2) disaster risk knowledge; (3) hazard detection, observation, monitoring, analysis, and prediction; (4) warning dissemination and communication; (5) preparedness and response capabilities; and (6) evaluation to reflect on the measures taken and to improve the system based on lessons learned. Again, climate research is needed in each of these pillars to make them a reality. There is real value in addressing climate variability and change in pursuit of ambitious development goals, and environmental justice must be addressed as a fundamental right.
4.7 Climate services
Cross-cutting opportunities include co-designing climate services for all and understanding socio-economic vulnerability to climate change (see also Figures 1, 2 which highlight the relation between climate science and climate services). Partnerships are very important to facilitate access to data. Also, co-design of climate information on time scales of weeks to years is and will be essential for socio-economic sectors to reduce the negative impacts of natural climate variability modified by anthropogenic climate change (Vera, 2024). In terms of impact attribution, there is value in piloting operational attribution, even if it starts at a basic level and despite the challenge of considering multiple climatic and non-climatic contributors to a disaster. Stakeholder-driven work and co-production ensure that the information generated is actionable and can be used directly in adaptation planning.
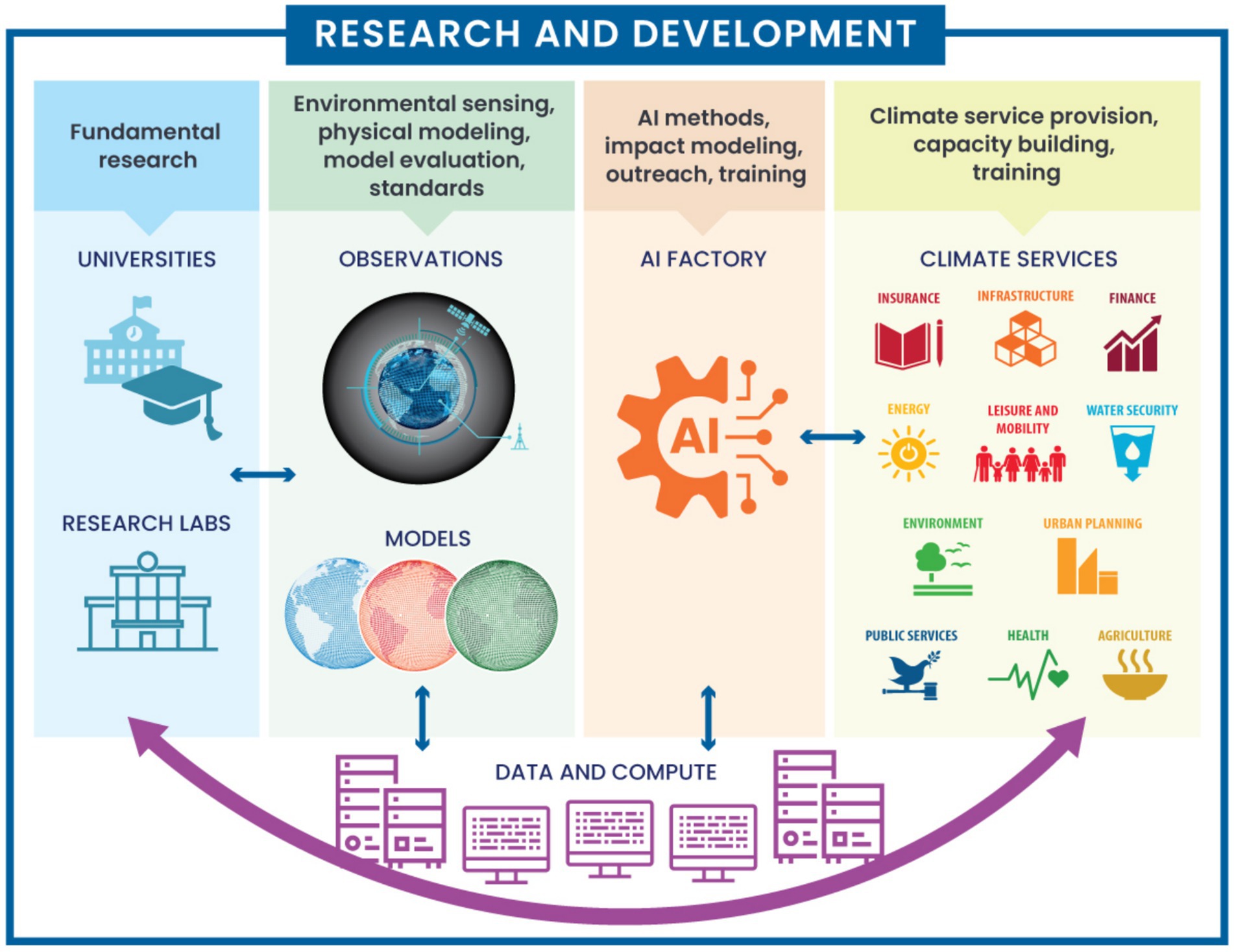
Figure 2. Representation of the flow linking fundamental research to the provision of information for climate services including observation and model prediction tools and “artificial intelligence factories” to local and societal relevant information. Adapted from Bjorn Stevens, Max Planck Institute for Meteorology.
Agencies, governments and the private sector must significantly increase their multilateral, accessible and equitable investments in developing actionable climate information and implementing climate adaptation options and loss and damage assessments based on climate science. The Global Framework for Climate Services, established by the World Meteorological Organization, is an important player to foster the development of climate services worldwide (Hewitt et al., 2020). Several countries have started to implement nation-wide climate service centers (see concrete examples in Europe by Golding et al., 2025), and the National Framework for Climate Services (NFCS) is an important driver to this development.
Improved climate change projections (and associated uncertainties) should be accompanied by contextual information, including for cities and human settlements. This needs to be complemented by the tools and data infrastructure required to make these data available and usable by all, and by building the contextual knowledge and capacity to use these data in an informed way. The use of new methods such as artificial intelligence to downscale information to relevant local scales and improved visualization techniques to facilitate data access and interpretation should be implemented. Long-term, sustained, high-quality and accessible observations and paleoclimate reconstructions should be conducted, making well-coordinated use of both remotely sensed and in-situ observations to increase spatial and temporal coverage and to sample past extreme events and their consequences. These are needed to monitor the impact of human behavior on climate, to improve climate assessments and projections, and to support climate-related decision making by exploring a range of adaptation options, mitigation pathways and model uncertainties. Improved climate information and early warning services should be established at local and regional levels to provide actionable information for adaptation and disaster risk reduction strategies. In addition, improved risk management, particularly in developing regions, requires more skillful sub-seasonal and seasonal forecasts at local scales, integrated with information on non-climatic factors describing vulnerability and exposure conditions (Vera, 2024).
4.7.1 Water security
Water security will become an increasingly real and pressing issue in the future: by 2030, 85% of the world’s population will live in arid regions with serious challenges for water: too much, too little, too polluted, while managing water and saving excess water in times of too much for the inevitable times of too little. The impact of climate change on water security and availability depends on resilience, capacity and policy (Trenberth, 2011; Trenberth and Asrar, 2012; Hegerl et al., 2015; Trenberth et al., 2016). A new water culture is needed, with education at all levels and informed by the latest science on water changes in a warming world. The risks of coastal and other erosion make the development of adequate drainage systems a priority.
4.7.2 Agriculture
One of the key sectors is agriculture; it is on the frontline of climate change impacts, but it is also a contributor to climate change, and emission reductions and enhanced carbon sequestration are needed in all sectors, including agri-food systems. This can lead to severe food insecurity, particularly in developing countries, and mitigation should not adversely affect the lives and livelihoods of all other sectors/populations vulnerable to the impacts of climate change. A just transition should apply to the agri-food sector on an equal footing with all other sectors. Furthermore, among the climate services that should underpin adaptation and mitigation actions in the agricultural sector are those provided by the forestry sector. Forests cover large areas of the planet and provide a wide range of valuable ecosystem services, including soil conservation and water and carbon cycling. However, their management continues to involve highly complex decisions. Challenges remain, such as how to maximize forest-based climate change mitigation activities while protecting biodiversity and ecosystem health and being socio-economically feasible.
4.7.3 Human health
Climate services will need to provide information on health-related climate indicators such as (1) expected changes in the frequency and intensity of heat waves and associated risks of heat-related illnesses, (2) conditions conducive to the spread of vector-borne diseases (malaria, dengue, Lyme disease, etc.), (3) data on rainfall and flooding conducive to the spread of diseases such as cholera, and (4) trends and warnings on air quality (particulate matter, ozone, allergens) responsible for respiratory diseases and heart failure. Climate services must contribute to the identification of vulnerable groups and hotspots of climate-related risks. They must support the development of climate-related healthcare systems and recommend preventive measure plans.
Assessing vulnerability to weather and climate events is an essential first step, followed by planning and building resilience of critical and interconnected infrastructures (energy, communication, water and health). Engaging users and sector experts to identify the climate conditions and thresholds that drive impacts in human and natural systems, helping to better identify risks, assess the impacts of irreversible changes, develop and deliver actionable climate information, provide an appropriate regulatory framework, and prioritize the best adaptation options are all essential actions. Climate services need to take a systemic approach with emphasis on the water-energy-agriculture-biodiversity-health nexus.
4.8 Capacity development and inclusion
Capacity building for interdisciplinary research and action goes beyond skills transfer, especially in the Global South. We need to rethink our roles as scientists and publishers, funders and individuals, and unequal partnerships in favor of peer and mutual partnerships. Climate literacy, the limitations of local expertise and data, and the importance of providing more training opportunities/programs (e.g., through the WCRP Academy) are all issues that need attention. Engagement necessarily involves building mutual learning relationships with interested parties at all levels and in all sectors, including extension services. For this dialog, information relevant to the stakeholder context is critical for developing and adapting evidence-based and actionable climate information that integrates local and indigenous knowledge and is delivered through climate services tailored to the stakeholder’s cultural realities.3,4 It is essential to provide climate information that is relevant to stakeholders’ climate and non-climate stressors in ways that match their competence and capacity to assimilate useful and actionable information, while building the scientific community’s understanding of the heterogeneity of stakeholder lived experiences.
Finally, the climate research community needs to strengthen the leadership of early and mid-career researchers, especially those from underrepresented groups and from the Global South, at both national and international levels. This includes recognizing the growing role of younger generations in addressing climate change and its impacts, as they will inherit a warmer world with more climate-related extreme events and will have to address the most challenging aspects of mitigation (Thiery et al., 2021). It is also necessary to expand the international climate research agenda to include emerging topics such as citizen science, urban studies, extreme weather events, artificial intelligence/machine learning techniques, climate intervention and integrate context in actionable climate information to inform societal responses.
5 The future
Research over the past decades has been successful in providing quantitative information on many of the processes that govern multiscale climate variability and change, and in producing projections of key climate variables in response to different scenarios of anthropogenic forcing. The challenge now is to translate these results into reliable scientific information for decision making (e.g., by policy makers, governments, economic sectors including industry, agriculture, insurance and reinsurance groups). This is also necessary to scientifically and credibly inform the design of strategies needed to reduce future societal vulnerability and maximize resilience under new climatic and societal conditions. These issues and their implications for society need to be addressed in the broader context of other challenges, changes and pressures that will affect society, such as economic trends, geopolitical tensions or simply conflicts over resources. Figure 3 (from Steffen et al., 2020) presents a simplified representation of the interactions between geosphere and the biosphere (including forcing and feedback mechanisms) and of the coupling the human system. Future research calls for a holistic approach that integrates the different elements that will ultimately determine the evolution of the Earth system.
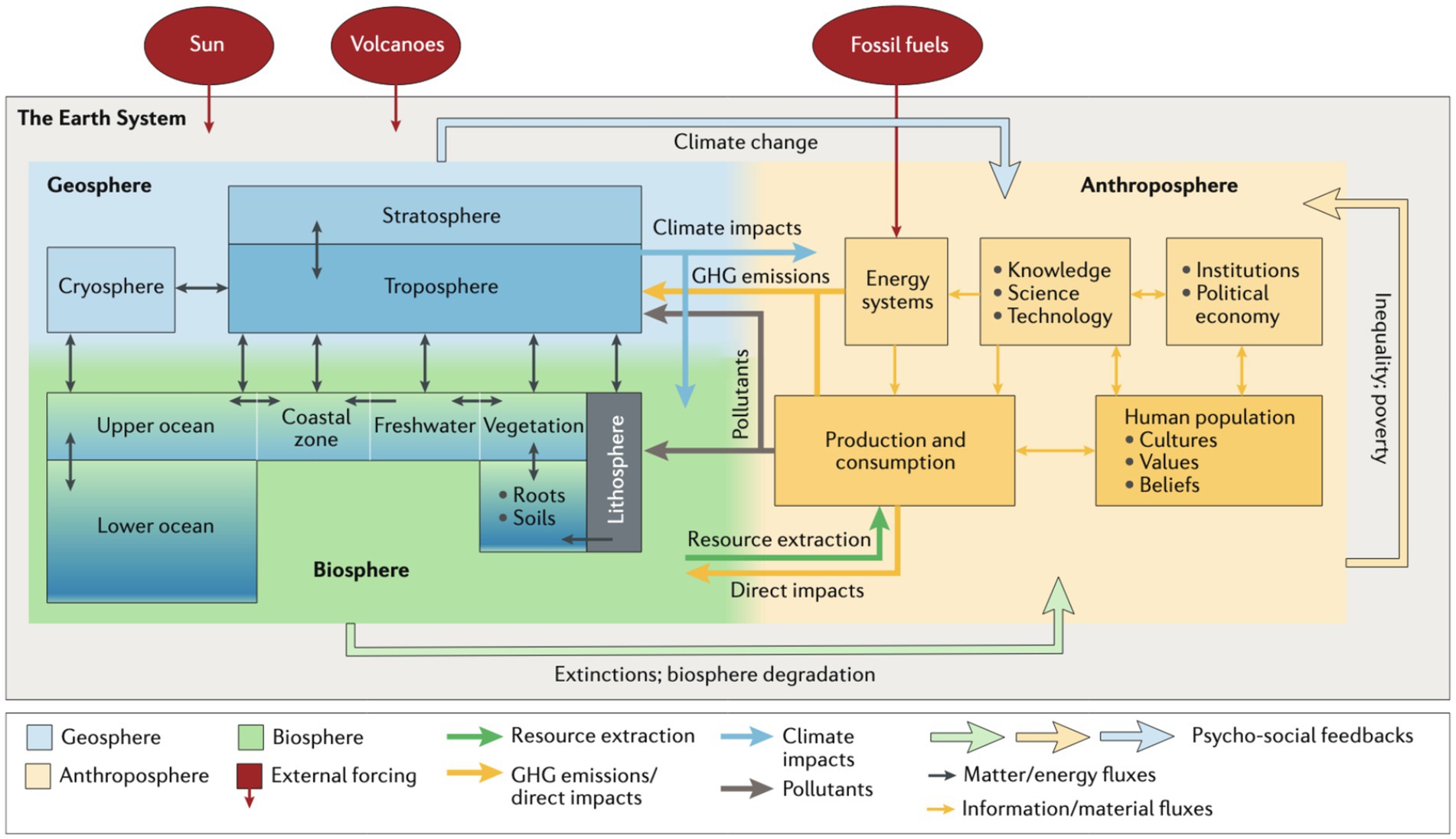
Figure 3. Representation of the Earth system, including the physical and biogeochemical processes affecting planet Earth, as well as the internal dynamics of the human system (anthroposphere) described by a system of production and consumption. This system is “forced” by energy systems and is modulated by human societies, which are in turn influenced by their cultures, values, institutions and level of education. Interactions within the Earth system are two-way, with anthropogenic emissions of greenhouse gases and other pollutants, and resource extraction leading to impacts that reverberate through the geosphere-biosphere system. Feedbacks to the anthroposphere are also important, including the direct impacts of climate change and biosphere degradation, as well as psychosocial feedbacks from the rest of the Earth system and within the anthroposphere. Source: Steffen et al. (2020); https://www.nature.com/articles/s43017-019-0005-6. Reproduced with permission.
As the Club of Rome noted more than a decade ago (Randers, 2012), the world of 2050 will be very different from the world of today. Population will continue to grow but is expected to peak around 2070. Gross domestic product (GDP) growth is expected to slow, and large disparities between different regions of the world will persist, leading to the continuation or possible intensification of political and social tensions. Fossil CO2 emissions have increased dramatically in the past, quadrupling since 1960 (Friedlingstein et al., 2023). If sufficient measures were taken to transform the current energy system into a decarbonized system, CO2 emissions could peak in the coming years. However, current policy and economic/societal developments do not suggest that this path will be followed and that substantial and sustained global reductions in greenhouse gas emissions will actually occur soon. Instead, current policies imply that global mean temperature will continue to rise, exceeding 1.5°C above 1850–1900 already by the late 2020s, with prospects of possibly reaching 2°C by 2050 and approaching 3°C by 2,100. Such magnitudes of changes are known to lead to an escalation of adverse impacts on ecosystems and their services, human populations and economic activities. They will pose increasing risks to water and food security, public health, the habitability of some regions, and biodiversity. The costs of supporting adaptation and protecting citizens and property from disasters will explode. It is therefore crucial to accelerate the reduction in greenhouse gas emissions, but this will depend on societal, economic and political choices.
The impacts of climate change are already falling disproportionately on regions that have contributed least to past emissions. 3.5 billion people now live in vulnerable regions, and 1 billion are exposed to the consequences of future sea-level rise. Continued population growth in several regions of the world and the associated demands for energy, food and water will further exacerbate tensions. The ongoing impacts of sea level rise and climate change will challenge the future habitability of regions, including climate change hotspots (Byers et al., 2018; Thiery et al., 2021; Werning et al., 2024). As a result, climate change will increase forced displacement, adding to other drivers of migration and increasing the number of environmental refugees in many countries. Without peace, societies will find it increasingly difficult to implement mitigation and adaptation measures. Climate research for 2050 will therefore need to be developed in a geopolitical context in which issues of security and peace will play a dominant role. Recent conflicts highlight the challenges of climate-resilience peacebuilding, especially in regions where a warmer world implies increased constraints on water resources.
In this context, what are the key scientific questions that climate research will have to address in the decades ahead?
First and foremost, the scientific community must continue to assess the vulnerability of populations and ecosystems to global and regional climate change and provide information on expected local impacts. This science-based information will help governments, economic actors and all citizens to sustain life on Earth and to implement ambitious mitigation policies and effective environmental protection measures. These policies must seek to reduce economic and social inequalities and recognize the importance of environmental justice. Researchers must help to evaluate, on a scientific basis, the effectiveness of policies to reduce emissions and protect the environment.
Adaptation measures must also be designed and implemented to limit immediate risks (e.g., the consequences of extreme weather events) and to cope with the long-term effects of anthropogenic climate change. Climate change will have undesirable effects on the daily lives of people in all regions, some of whom will have to even abandon geographical areas and seek refuge in more hospitable regions. Economic sectors such as agriculture, transport, humanitarian aid and tourism will have to adapt to new climatic conditions. The scientific community must therefore provide the information needed to develop policies that are adapted to the changes that will occur in each of the world’s regions. Data systems that are easily accessible to decision-makers must provide a range of objective information that is applicable at the local level and that takes into account impacts, including the availability of resources such as water and food.
Ultimately, if greenhouse gas emissions are not reduced sufficiently to mitigate climate change, i.e., if the transition to a net-zero-emissions global society is too slow, society may need to resort to interventions including the removal of greenhouse gases from the atmosphere through the process of capture and sequestration. Such initiatives, which are now being considered by some governments and industrial companies, raise serious ethical questions and international political problems. Research by the scientific community on the efficacy and possible consequences of such measures, their plausibility and limitations, and the risks of serious trade-offs (e.g., land rights, food security) must accompany the discussions of political leaders on these issues (National Academies of Sciences, Engineering, and Medicine, 2021). If such measures are effectively implemented, the climate system will need to be carefully monitored to assess the conditions under which a new climate equilibrium might be reached. Climate science advances are needed to ensure that the proposed responses do not generate new types of risks. A report released in 2024 by the “Scientific Advise Mechanism (SAM) to the European Commission,” states that none of the technologies that have been proposed to deal with solar radiation modification including stratospheric aerosol injection and cloud brightening, is mature. Deploying such technologies could have negative impacts on ecosystems, change rainfall patterns, and hamper food production. Moreover, they would not address the direct impacts of greenhouse gases, such as ocean acidification or changes in vegetation patterns.
Integrated scientific approaches should lead to a better understanding of the coupled physical, chemical and biological interactions between the atmosphere, ocean, cryosphere, biosphere and society, and to a better assessment of the risks of irreversible climate and environmental change. The possible existence of thresholds (i.e., tipping points) beyond which large-scale changes could occur must be investigated, as they could affect sensitive areas such as the Amazon rainforest, polar permafrost, ocean or atmospheric circulations (e.g., the Atlantic Meridional Overturning Circulation) or continental ice sheets. Table 1 provides a list of major and urgent research directions for climate science.
Finally, more effective communication mechanisms need to be established between researchers, governments, the private sector and citizens. The role of climate services needs to be expanded, strengthened and perhaps redefined. These climate services will need to develop effective ways to translate observational and model data into actionable climate information that enables informed decision-making and resilience building, facilitates community input, and fills critical data gaps in cities and informal settlements, oceans, and data-poor regions. Digital twin approaches offer ways to connect science and engineering to the adaptation challenge. Climate services will need to advocate for the principles and practices of open science and open education, and work with global science funders to support their effective adoption around the world, including in the Global South, and to raise the visibility and value of regional knowledge. Climate science must be collectively led around the world in setting priorities and allocating resources to foster greater collaboration, shared and equitable leadership, and alignment with local understandings of scientific challenges and opportunities, including climate literacy. Solutions proposed by climate services must be fair and equitable in a spirit of environmental justice.
The task of ensuring the large-scale and unprecedented societal transformation required to make society resilient to climate change requires the provision of reliable natural and social science information. It also requires political commitment to define and implement policies that reduce the risks of major climate-related disruptions and support adaptation. This will require leadership, engagement, communication, education, and citizen participation under new and strong ethical considerations. The responsibility of climate science in 2025 and beyond is to ensure that all these challenges are addressed and supported.
Author contributions
GB: Conceptualization, Supervision, Writing – original draft, Writing – review & editing. DS: Conceptualization, Writing – original draft, Writing – review & editing. PF: Writing – original draft, Writing – review & editing. GH: Writing – review & editing, Writing – original draft. TS: Writing – original draft, Writing – review & editing. KT: Writing – original draft, Writing – review & editing. JR: Writing – review & editing, Writing – original draft. CV: Writing – review & editing, Writing – original draft. AB: Writing – review & editing, Writing – original draft. HC: Writing – review & editing, Writing – original draft. SE: Writing – review & editing, Writing – original draft. PE: Writing – review & editing, Writing – original draft. DJ: Writing – review & editing. MM: Writing – review & editing, Writing – original draft. VM-D: Writing – original draft, Writing – review & editing. GS: Writing – review & editing, Writing – original draft. MS: Writing – review & editing, Writing – original draft. TFS: Writing – original draft, Writing – review & editing. MV: Writing – review & editing, Writing – original draft. GW: Writing – review & editing, Writing – original draft.
Funding
The author(s) declare that financial support was received for the research and/or publication of this article. GB and JR are partially supported by NCAR; the NSF-National Center for Atmospheric Research is sponsored by the US National Science Foundation. DS received partial support through the DFG supported Excellence Cluster CLICCS of the University of Hamburg. TS acknowledges support from NSF (AGS-2300037) and National Oceanic and Atmospheric Administration (NA23OAR4310597).
Acknowledgments
This paper is partly based on the outcome of the 2nd Open Science Conference of the World Climate Research Program (WCRP) held in Kigali, Rwanda and hosted by the Rwanda Environment Management Authority (REMA) on behalf of the Government of Rwanda. The authors acknowledge the Kigali Declaration, one of the key outcomes of the WCRP Open Science Conference 2023, available under https://www.wcrp-climate.org/conferences/WCRP-OSC-2023/KD/WCRP-Kigali-Declaration-2024-c.pdf. The conference was sponsored by several agencies listed under https://wcrp-osc2023.org/sponsors.
Conflict of interest
The authors declare that the research was conducted in the absence of any commercial or financial relationships that could be construed as a potential conflict of interest.
The reviewer GK declared a past co-authorship with the author GH to the handling editor.
Generative AI statement
The author(s) declare that no Gen AI was used in the creation of this manuscript.
Publisher’s note
All claims expressed in this article are solely those of the authors and do not necessarily represent those of their affiliated organizations, or those of the publisher, the editors and the reviewers. Any product that may be evaluated in this article, or claim that may be made by its manufacturer, is not guaranteed or endorsed by the publisher.
Footnotes
1. ^https://www.wcrp-climate.org
3. ^https://www.frontiersin.org/journals/climate/articles/10.3389/fclim.2022.885494/full
4. ^https://www.frontiersin.org/journals/climate/articles/10.3389/fclim.2022.871987/full
References
Allen, M. R., Frame, D. J., Huntingford, C., Jones, C. D., Lowe, J. A., Meinshausen, M., et al. (2009). Warming caused by cumulative carbon emissions towards the trillionth tonne. Nature 458, 1163–1166. doi: 10.1038/nature08019
Arrhenius, S. (1896). On the influence of carbonic acid in the air upon the temperature of the ground. Philos. Mag. 41, 237–276. doi: 10.1080/14786449608620846
Arora, V. K., Katavouta, A., Williams, R. G., Jones, C. D., Brovkin, V., Friedlingstein, P., et al. (2020). Carbon–concentration and carbon–climate feedbacks in CMIP6 models and their comparison to CMIP5 models, Biogeosciences, 17, 4173–4222. doi: 10.5194/bg-17-4173-2020
Bauer, P., Thorpe, A., and Brunet, G. (2015). The quiet revolution of numerical weather prediction. Nature 525, 47–55. doi: 10.1038/nature14956
Berger, A., and Loutre, M. F. (2002). An exceptionally long interglacial ahead? Science 297, 1287–1288. doi: 10.1126/science.1076120
Bi, K., Xie, L., Zhang, H., Chen, X., Gu, X., and Tian, Q. (2023). Accurate medium-range global weather forecasting with 3D neural networks. Nature 619, 533–538. doi: 10.1038/s41586-023-06185-3
Blanken, P. D., Brunet, D., Dominguez, C., Goursaud Oger, S., Hussain, S., Jain, M., et al. (2022). Atmospheric perspectives on integrtated coordinated, open, neetworked (ICON) science, earth and space. Science 9:e2021EA002204. doi: 10.1029/2021EA002204
Bowman, D. M. J. S., Balch, J. K., Artaxo, P., Bond, W. J., Carlson, J. M., Cohrane, M. A., et al. (2009). Fire in the earth system. Science 30, 255–260. doi: 10.1016/j.tree.2015.03.005
Byers, E. A., Gidden, M., Leclere, D., Balkovic, J., Burek, P., Ebi, K. L., et al. (2018). Global exposure and vulnerability to multi-sector development and climate change hotspots. Environ. Res. Lett. 13:5. doi: 10.1088/1748-9326/aabf45
Canadell, J. G., and Jackson, R. B. (2021). Ecosystem Collapse and Climate Change: An Introduction. In: Canadell, J.G., Jackson, R.B. (eds) Ecosystem Collapse and Climate Change. Ecological Studies (Springer, Cham). 241.
Cattiaux, J., Ribes, A., and Thompson, V. (2024). Searching for the Most extreme temperature events in recent history. Bull. Amer. Meteor. Soc. 105, E239–E256. doi: 10.1175/BAMS-D-23-0095.1
Charney, J. G., Arakawa, A., Baker, D. J., Bolin, B., Dickinson, R. E., Goody, R. M., et al. (1979). Carbon dioxide and climate: A scientific assessment. Washington, DC: National Academy of Sciences Press.
Cheng, L., Abraham, J., Trenberth, K. E., Boyer, T., Mann, M. E., Zhu, J., et al. (2024). New record ocean temperatures and related climate indicators in 2023. Adv. Atmos. Sci. 41, 1068–1082. doi: 10.1007/s00376-024-3378-5
Cheng, L., Trenberth, K. E., Gruber, N., Abraham, J. P., Fasullo, J., Li, G., et al. (2020). Improved estimates of changes in upper ocean salinity and the hydrological cycle. J. Clim. 33, 10357–10381. doi: 10.1175/JCLI-D-20-0366.1
Compo, G. P., Whitaker, J. S., and Sardeshmukh, P. D. (2005). Feasibility od a 100=year reanalysis using only surface pressure data. Bull. Amer. Soc, 87:175–190.
Coumou, D., Arias, P. A., Bastos, A., Gotangco Gonzales, C. K., Hegerl, G. C., et al. (2024). How can event attribution science underpin financial decisions on loss and damage? PNAS Nexus 3:8. doi: 10.1093/pnasnexus/pgae277
Dee, D. P., Uppala, S., and Simmons, A. J. (2011). The ERA-interim reanalysis: configuration and performance of the data assimilation system. Quart. J. Roy. Meteor. Soc. 137, 553–597. doi: 10.1002/qj.828
Durack, P. J., Taylor, K. E., Eyring, V., Ames, S. K., Doutriaux, C., et al. (2017). Technical report: input4MIPs: Making [CMIP] model forcing more transparent. UD Department of Energy, Office of Scientific and Technical Information.
Flannigan, M. D., Stocks, B. J., Turetsky, M. R., and Wotton, B. M. (2009). Impact of climate change on fire activity and fire management in the circumboreal forest. Glob. Chang. Biol. 15, 549–560. doi: 10.1111/J.1365-2486.2008.01660.X
Forster, P. M., Smith, C., Walsh, T., Lamb, W. F., and Lamboll, R. (2024). Indicators of global climate change 2023: annual update of key indicators of the state of the climate system and human influence. Earth Syst. Sci. Data 16, 2625–2658. doi: 10.5194/essd-16-2625-2024
Friedlingstein, P., O'Sullivan, M., Jones, M. W., Andrew, R. M., Bakker, D. C. E., Hauck, J., et al. (2023). Global carbon budget 2023. Earth Syst. Sci. Data 15, 5301–5369. doi: 10.5194/essd-15-5301-2023
Gelaro, R., McCarty, W., Suárez, M. J., and Todling, R. (2017). The modern-era retrospective analysis for research and applications, version 2 (MERRA-2). J. Clim. 30, 5419–5454. doi: 10.1175/JCLI-D-16-0758.1
Gettelman, A., Eidhammer, T., Duffy, M. L., McCoy, D. T., Song, C., and Watson-Parris, D. (2024). The interaction between climate forcing and feedbacks. J. Geophys. Res. 129:e2024JD040857. doi: 10.1029/2024JD040857
Ghil, M., and Lucarini, V. (2020). The physics of climate variability and climate change. Rev. Mod. Phys. 92:035002. doi: 10.1103/RevModPhys.92.035002
Golding, N., Lambkin, K., Wilson, L., De Troch, R., Fischer, A. M., Hygen, H. O., et al. (2025). Developing national frameworks for climate services: experiences, challenges and learnings from across Europe. Climate Services 37:852.
Guimaraes, S. O., Mann, M. E., Rahmstorf, S., Petri, S., Steinman, B. A., Brouillette, D. J., et al. (2024). Increased projected changes in quasi-resonant amplification and persistent summer weather extremes in the latest multimodel climate projections. Sci. Rep. 14:21991. doi: 10.1038/s41598-024-72787-0
Hamlington, B. D., Bellas-Manley, A., Willis, J. K., Fournier, S., Vinogradova, N., Nerem, R. S., et al. (2024). The rate of global sea level rise doubled during the past three decades. Commun. Earth Environ. 5:501. doi: 10.1038/s43247-024-01761-5
Hansen, J., Sato, M., Simons, L., et al. (2023). Global warming in the pipeline. Oxford Open Clim. Change 3. doi: 10.1093/oxfclm/kgad008
Hausfather. (2023). Emissions are no longer following the worst case scenario. Available online at: https://www.theclimatebrink.com/p/emissions-are-no-longer-following (Accessed April 24, 2025).
Hegerl, G., Black, E., Allan, R. P., Ingram, W. J., Polson, D., Trenberth, K., et al. (2015). Challenges in quantifying changes in the global water cycle. Bull. Amer. Meteor. Soc. 96, 1097–1115. doi: 10.1175/BAMS-D-13-00212.1
Held, I. M. (2005). The gap between simulation and understanding in climate modeling. Bull. Amer. Meteor. Soc. 86, 1609–1614. doi: 10.1175/BAMS-86-11-1609
Hersbach, H., Bell, B., and Berrisford, P. (2020). The ERA5 global reanalysis. Quart. J. Roy. Meteor. Soc. 146, 1999–2049. doi: 10.1002/qj.3803
Hewitt, C. D., Allis, E., Mason, S. J., Muth, M., and Pulwarty, R., (2020). Making Society Climate Resilient: International Progress under the Global Framework for Climate Services. Bull. Amer. Meteor. Soc. 101. doi: 10.1175/BAMS-D-18-0211.1
Hodnebrog, Ø., Myhre, G., Jouan, C., Andrews, T., Forster, P. M., Jia, H., et al. (2024). Recent reductions in aerosol emissions have increased Earth’s energy imbalance. Commun Earth Environ 5:166. doi: 10.1038/s43247-024-01324-8
Hurrell, J. W., Haywood, J. M., Lawrence, P. J., Lennard, C. J., and Oschlies, A. (2024). Climate intervention research in the world climate research Programme: a perspective. Front. Clim. 6:1505860. doi: 10.3389/fclim.2024.1505860
IPCC (2018). “Summary for policymakers” in Global warming of 1.5°C. An IPCC special report on the impacts of global warming of 1.5°C above pre-industrial levels and related global greenhouse gas emission pathways, in the context of strengthening the global response to the threat of climate change, sustainable development, and efforts to eradicate poverty. eds. V. Masson-Delmotte, P. Zhai, H.-O. Pörtner, and D. Roberts (Cambridge, UK and New York, NY, USA: Cambridge University Press), 3–24.
IPCC (2021). “Climate change 2021: the physical science basis.” Contribution of working group I to the sixth assessment report of the intergovernmental panel on climate change [V. P Masson-Delmotte, A. Pirani Zhai, S.L. Connors, C. Péan, and S. Berger (eds.)]. Cambridge University Press, Cambridge, United Kingdom and New York, NY, USA, 2391 pp.
IPCC (2022): “Climate change 2022: Impacts, adaptation, and vulnerability.” Contribution of working group II to the sixth assessment report of the intergovernmental panel on climate change [H.-O. Pörtner, D.C. Roberts, M. Tignor, and E.S. Poloczanska, (eds.)]. Cambridge University Press. Cambridge University Press, Cambridge, UK and New York, NY, USA, 3056.
IPCC, Climate Change (2001) in The scientific basis. Contribution of working group I to the second assessment report of the intergovernmental panel on climate change. ed. J. T. Houghton (Cambridge: Cambridge University Press), 881.
IPCC, Climate Change (2007) in The physical science basis. Contribution of working group I to the fourth assessment report of the intergovernmental panel on climate change. ed. S. Solomon (Cambridge: Cambridge University Press), 996.
IPCC, Climate Change (2013) in The physical science basis. Contribution of working group I to the fifth assessment report of the intergovernmental panel on climate change. ed. T. F. Stocker (Cambridge: Cambridge University Press), 1535.
Jain, S., Mindlin, J., Koren, G., Gulizia, C., Steadman, C., Langendijk, G. S., et al. (2022). Are we at risk of losing the current generation of climate researchers to data science? AGU Advances 3:e2022AV000676. doi: 10.1029/2022AV000676
Kaufman, D., and Masson-Delmotte, V. (2024). Opinion: distribute paleoscience information across the next intergovernmental panel on climate change reports. Clim. Past 20, 2587–2594. doi: 10.5194/cp-20-2587-2024
King, A. D., Grose, M. R., Kimutai, J., Pinto, I., and Harrington, L. J. (2023). Event attribution is not ready for a major role in loss and damage. Nat. Clim. Chang. 13, 415–417. doi: 10.1038/s41558-023-01651-2
King, A. D., Ziehn, T., Chamberlain, M., Borowiak, A. R., Brown, J. R., Cassidy, L., et al. (2024). Exploring climate stabilisation at different global warming levels in ACCESS-ESM-1.5. Earth Syst. Dynam. 15, 1353–1383. doi: 10.5194/esd-15-1353-2024
Knutti, R., Rugenstein, M., and Hegerl, G. (2017). Beyond equilibrium climate sensitivity. Nat. Geosci. 10, 727–736. doi: 10.1038/ngeo3017
Kobayashi, S., Ota, Y., and Harada, Y. (2015). The JRA-55 reanalysis: general specifications and basic characteristics. J. Meteor. Soc. Japan 93, 5–48. doi: 10.2151/jmsj.2015-001
Koven, C. D., Ringeval, B., Friedlingstein, P., Ciais, P., Cadule, P., Khvorostyanov, D., et al. (2011). Permafrost carbon-climate feedbacks accelerate global warming. Proc. Natl. Acad. Sci. USA 108, 14769–14774. doi: 10.1073/pnas.1103910108
Lam (2023). Learning skillful medium-range global weather forecasting, Science, 382, 1416–1421. doi: 10.1126/science.adi2336
Laughner, J. L., Neu, J. L., Schimel, D., Wennberg, P. O., Barsanti, K., Bowman, K. W., et al. (2021). Societal shifts due to COVID-19 reveal large-scale complexities and feedbacks between atmospheric chemistry and climate change. Proc. Natl. Acad. Sci. USA 118:46. doi: 10.1073/pnas.2109481118
Lenton, T. M., Held, H., Kriegler, E., Hall, J. W., Lucht, W., Rahmstorf, S., et al. (2008). Tipping elements in the Earth's climate system. Proc. Natl. Acad. Sci. 105, 1786–1793. doi: 10.1073/pnas.0705414105
Lenton, T. M., Rockström, J., Gaffney, O., Rahmstorf, S., Richardson, K., Steffen, W., et al. (2019). Climate tipping points—too risky to bet against. Nature 575, 592–595. doi: 10.1038/d41586-019-03595-0
MacDougall, A. H., Frölicher, T. L., Jones, C. D., and Rogelj, J. (2020). Is there warming in the pipeline? A multi-model analysis of the zero emissions commitment from CO2. Biogeosciences 17, 2987–3016. doi: 10.5194/bg-17-2987-2020
Matthews, H. D., and Caldeira, K. (2008). Stabilizing climate requires near-zero emissions. Geophys. Res. Lett. 35:L04705. doi: 10.1029/2007GL032388
National Academies of Sciences, Engineering, and Medicine (2021). Reflecting sunlight: Recommendations for solar geoengineering research and research governance. Washington, D.C: The National Academies Press.
Newman, R., and Noy, I. (2023). The global costs of extreme weather that are attributable to climate change. Nat. Commun. 14:6103. doi: 10.1038/s41467-023-41888-1
Otto, F. E. L. (2023). Attribution of extreme events to climate change. Annu. Rev. Environ. Resour. 48, 813–828. doi: 10.1146/annurev-environ-112621-083538
Otto, F. E. L., and Fabian, F. (2024). Equalising the evidence base for adaptation and loss and damages. Global Pol. 15, 64–74. doi: 10.1111/1758-5899.13269
Preston, C. J. (2013). Ethics and geoengineering: reviewing the moral issues raised by solar radiation management and carbon dioxide removal. WIREs Clim Change 4, 23–37. doi: 10.1002/wcc.198
Raghuraman, S. P., Soden, B., Clement, A., Vecchi, G., Menemenlis, S., and Yang, W. (2024). The 2023 global warming spike was driven by the El Niño–southern oscillation. Atmos. Chem. Phys. 24, 11275–11283. doi: 10.5194/acp-24-11275-2024
Randers, J. (2012). A global forecast for the next forty years. White River Junction, VT: Chelsea Green Publishing, 397.
Richter, J. H. (2025). Earth System Predictability Across Time Scales for a Resilient Society: A Research Community Perspective (2025 ).
Romanou, A., Hegerl, G. C., Seneviratne, S. I., Abis, B., Bastos, A., Conversi, A., et al. (2024). Extreme events contributing to tipping elements and tipping points. Surv. Geophys. doi: 10.1007/s10712-024-09863-7
Samset, B. H., Lund, M. T., Fuglestvedt, J. S., and Wilcox, L. J. (2024). 2023 temperatures reflect steady global warming and internal sea surface temperature variability. Nat. Commun. 5:460. doi: 10.1038/s43247-024-01637-8
Sauterey, B., Gland, G. L., Cermeño, P., Aumont, Q., Lévy, M., and Vallina, S. M. (2023). Phytoplankton adaptive resilience to climate change collapses in case of extreme events – A modeling study, Ecological Modelling, 483, 110437. doi: 10.1016/j.ecolmodel.2023.110437
Schuur, E. A. G., Abbott, B. W., Commane, P., Ernakovich, J., Euskirchen, E., Hugelius, G., et al. (2022). Permafrost and climate change: Carbon cycle feedbacks from the warming Arctic, Annual Review of Environment and Resources. 47:343–371. doi: 10.1146/annurev-environ-012220-011847
Schmidt, G. (2024). Climate models can’t explain 2023’s huge heat anomaly — we could be in uncharted territory. Nature 627:467. doi: 10.1038/d41586-024-00816-z
Sherwood, S. C., Hegerl, G., Braconnot, P., Friedlingstein, P., Goelzer, H., Harris, N. R. P., et al. (2024). Uncertain pathways to a future safe climate. Earth's Future 12:e2023EF004297. doi: 10.1029/2023EF004297
Solomon, S., Plattner, G., Knutti, R., and Friedlingstein, P. (2009). Irreversible climate change due to carbon dioxide emissions. Proc. Natl. Acad. Sci. USA 106, 1704–1709. doi: 10.1073/pnas.0812721106
Steffen, W., Richardson, K., Rockström, J., Schellnhuber, H. J., Dube, O. P., Dutreuil, S., et al. (2020). The emergence and evolution of earth system science. Nat Rev Earth Environ 1, 54–63. doi: 10.1038/s43017-019-0005-6
Steffen, W., Rockström, J., Richardson, K., Lenton, T. M., Folke, C., Liverman, D., et al. (2018). Trajectories of the earth system in the Anthropocene. Proc. Natl. Acad. Sci. USA 115, 8252–8259. doi: 10.1073/pnas.1810141115
Stevens, B., Adami, S., Ali, T., Anzt, H., Aslan, Z., Attinger, S., et al. (2024). Earth virtualization engines (EVE). Earth Syst. Sci. Data 16, 2113–2122. doi: 10.5194/essd-16-2113-2024
Tebaldi, C. P., and Friedlingstein, P. (2013). Delayed detection of climate mitigation benefits due to climate inertia and variability. Proc. Natl. Acad. Sci. USA 110, 17229–17234. doi: 10.1073/pnas.1300005110
Thiery, W., Lange, S., Rogelj, J., Hanasaki, N., Hickler, T., Huber, V., et al. (2021). Intergenerational inequities in exposure to climate extremes. Science 374, 158–160. doi: 10.1126/science.abi7339
Trenberth, K. E. (2011). Changes in precipitation with climate change. Clim. Res. 47, 123–138. doi: 10.3354/cr00953
Trenberth, K. E., and Asrar, G. R. (2012). Challenges and opportunities in water cycle research: WCRP contributions. Surv. Geophys. 35, 515–532. doi: 10.1007/s10712-012-9214-y
Trenberth, K. E., and Fasullo, J. T. (2013). Regional energy and water cycles: transports from ocean to land. J. Clim. 26, 7837–7851. doi: 10.1175/JCLI-D-13-00008.1
Trenberth, K. E., Marquis, M., and Zebiak, S. (2016). The vital need for a climate information system. Nat. Clim. Chang. 6, 1057–1059. doi: 10.1038/NCLIM-16101680
Trenberth, K. E., Zhang, Y., and Gehne, M. (2017). Intermittency in precipitation: duration, frequency, intensity, and amounts using hourly data. J. Hydrometeorol. 18, 1393–1412. doi: 10.1175/JHM-D-16-0263.1
Van der Wal, R. S. W., Nicholls, R. J., Behar, D., McInnes, K., Stammer, D., and Lowe, J. A. (2022). A high-end estimate of sea level rise for practitioners. Earth’s Future 10:e2022EF002751. doi: 10.1029/2022EF002751
Vera, C. (2024). Climate Services in South America. Oxford Research Encyclopedia of Climate Science. doi: 10.1093/acrefore/9780190228620.013.963
Wang, S., Foster, A., Lenz, E. A., Kessler, J. D., Stroeve, J. C., Anderson, L. O., et al. (2023). Mechanisms and impacts of earth system and impacts of earth system tipping elements. Rev. Geophys. 61. doi: 10.1029/2021RG000757
Wang, H., Zheng, X. T., Cai, W., and Zhou, L. (2024). Atmosphere teleconnections from abatement of China aerosol emissions exacerbate Northeast Pacific warm blob events. Proc. Natl. Acad. Sci. USA 121:e2313797121. doi: 10.1073/pnas.2313797121
WCRP (2019). World climate research Programme strategic plan 2019–2028 : WCRP Publication. Geneva, Switzerland: World Meteorological Organization.
WCRP, World climate research Programme strategic plan, climate science for society (2020). World Meteorological Organization.
Werning, M., Hooke, D., Krey, V., Riahi, K., and van Ruijven, B. (2024). Global warming level indicators of climate change and hotspots of exposure. Environ. Res. Climate 3:e045015. doi: 10.1088/2752-5295/ad8300
Wilkinson, M., Dumontier, M., and Aalbersberg, I. (2016). The FAIR guiding principles for scientific data management and stewardship. Sci Data 3:160018. doi: 10.1038/sdata.2016.18
Wunderling, N., von der Heydt, A. S., Aksenov, Y., Barker, S., Bastiaansen, R., Brovkin, V., et al. (2024). Climate tipping point interactions and cascades: a review. Earth Syst. Dynam. 15, 41–74. doi: 10.5194/esd-15-41-2024
Yin, Q. Z., Wu, Z. P., Berger, A., Goosse, H., and Hodell, D. (2021). Insolation triggered abrupt weakening of Atlantic circulation at the end of interglacials. Science 373, 1035–1040. doi: 10.1126/science.abg1737
Keywords: climate, climate change, climate impacts, resilience, forcing and feedbacks, information
Citation: Brasseur G, Stammer D, Friedlingstein P, Hegerl G, Shaw T, Trenberth K, Richter J, Vera C, Berger A, Cleugh H, Easterbrook S, Edwards P, Jacob D, Mann M, Masson-Delmotte V, Schmidt G, Scholes M, Stocker T, Visbeck M and Wu G (2025) Climate science for 2050. Front. Clim. 7:1554685. doi: 10.3389/fclim.2025.1554685
Edited by:
Phil Renforth, Heriot-Watt University, United KingdomReviewed by:
Andreas Marc Fischer, Federal Office of Meteorology and Climatology, SwitzerlandGerbrand Koren, Utrecht University, Netherlands
Copyright © 2025 Brasseur, Stammer, Friedlingstein, Hegerl, Shaw, Trenberth, Richter, Vera, Berger, Cleugh, Easterbrook, Edwards, Jacob, Mann, Masson-Delmotte, Schmidt, Scholes, Stocker, Visbeck and Wu. This is an open-access article distributed under the terms of the Creative Commons Attribution License (CC BY). The use, distribution or reproduction in other forums is permitted, provided the original author(s) and the copyright owner(s) are credited and that the original publication in this journal is cited, in accordance with accepted academic practice. No use, distribution or reproduction is permitted which does not comply with these terms.
*Correspondence: G. P. Brasseur, Z3V5LmJyYXNzZXVyQG1waW1ldC5tcGcuZGU=