- 1Physiology Unit, Faculty of Veterinary Medicine, Norwegian University of Life Sciences, Oslo, Norway
- 2Department of Biological Sciences, University of Mary Washington, Fredericksburg, VA, United States
The pituitary gland controls many important physiological processes in vertebrates, including growth, homeostasis, and reproduction. As in mammals, the teleost pituitary exhibits a high degree of plasticity. This plasticity permits changes in hormone production and secretion necessary to meet the fluctuating demands over the life of an animal. Pituitary plasticity is achieved at both cellular and population levels. At the cellular level, hormone synthesis and release can be regulated via changes in cell composition to modulate both sensitivity and response to different signals. At the cell population level, the number of cells producing a given hormone can change due to proliferation, differentiation of progenitor cells, or transdifferentiation of specific cell types. Gonadotropes, which play an important role in the control of reproduction, have been intensively investigated during the last decades and found to display plasticity. To ensure appropriate endocrine function, gonadotropes rely on external and internal signals integrated at the brain level or by the gonadotropes themselves. One important group of internal signals is the sex steroids, produced mainly by the gonadal steroidogenic cells. Sex steroids have been shown to exert complex effects on the teleost pituitary, with differential effects depending on the species investigated, physiological status or sex of the animal, and dose or method of administration. This review summarizes current knowledge of the effects of sex steroids (androgens and estrogens) on gonadotrope cell plasticity in teleost anterior pituitary, discriminating direct from indirect effects.
Introduction
Teleost fish comprise the largest vertebrate group with close to 30,000 species (1), including well established model species such as zebrafish (Danio rerio) and Japanese medaka (Oryzias latipes), which provide valuable tools for basic research on vertebrate physiology (2, 3). In addition, numerous teleosts, such as salmonids, seabreams, basses, tilapia, and other species with regional importance, have commercial and ecological or societal value and are subjects of applied research related to aquaculture or conservation.
Compared to tetrapods, teleosts experienced an additional whole genome duplication, known as the 3R (4). Some teleosts, such as the salmonids, have also had a fourth duplication event (4R) (5). Therefore, teleost fish can potentially possess 2 to 4 times more genes than other vertebrates, and although many of the duplicated genes have been lost through teleost evolution, some have been conserved and developed new or expanded functions. Fish reproductive physiology has been extensively investigated over the last decades, due to the high economic interest of controlling fish reproduction in aquaculture species and for evolutionary aspects as the main regulatory mechanisms are conserved among vertebrates.
In all vertebrates, the reproductive function is controlled through the physiological connections of the brain - pituitary - gonadal (BPG) axis, where the pituitary gonadotropes play a central role (6, 7). Located in the anterior pituitary (adenohypophysis), gonadotropes produce and release into the blood circulation the two gonadotropins (follicle-stimulating and luteinizing hormones, Fsh and Lh, respectively) which stimulate gonadal gametogenesis and steroidogenesis. Gonadotropins are heterodimeric proteins consisting of an α-subunit, common to both Lh and Fsh, and a unique β-subunit that confers the biological specificity (8). Interestingly, contrary to mammals and birds, in teleosts, the two gonadotropins are generally produced by discrete gonadotrope cell types; Lh cells and Fsh cells (9, 10).
Located below the hypothalamus, the pituitary is composed of two main parts with different developmental origins. The neurohypophysis (posterior pituitary) originates from a down-growth of the diencephalon and contains projections from neuroendocrine cells mainly located in the preoptic-hypothalamic region of the brain. The anterior pituitary originates from the placodal ectoderm at the anterior neural ridge which invaginates and subsequently separates from the stomodeum, a thickening of the ectoderm that forms the epithelium of the oral cavity (11). The anterior pituitary contains several hormone producing cells, including the gonadotropes which are localized to the proximal pars distalis (PPD).
Unlike in mammals where the different endocrine cell types are mosaically distributed in the adult anterior pituitary, in teleosts they are spatially discrete through the entire lifespan (6, 11). However, in both mammals and teleosts, the anterior pituitary shows high plasticity at both cellular and population levels, allowing the anterior pituitary to meet the demands for hormonal production as they change over the life cycle of an animal (12). At the cellular level, cellular activity (hormone production and release) can be modified by varying regulatory ligand sensitivity through the presence and number of receptors, or by altering rates of hormone synthesis and secretion, the latter corresponding to the hormone release as defined by Jena (13) (Figure 1A). At the population level, the number of cells of each endocrine cell type can change (Figure 1B). This can be due to proliferation of the endocrine cells (Figure 2A), differentiation of progenitor cells (Figure 2B), phenotypic conversion (transdifferentiation) of an endocrine cell into another cell type (Figure 2C), or cell death (apoptosis) (Figure 2D).
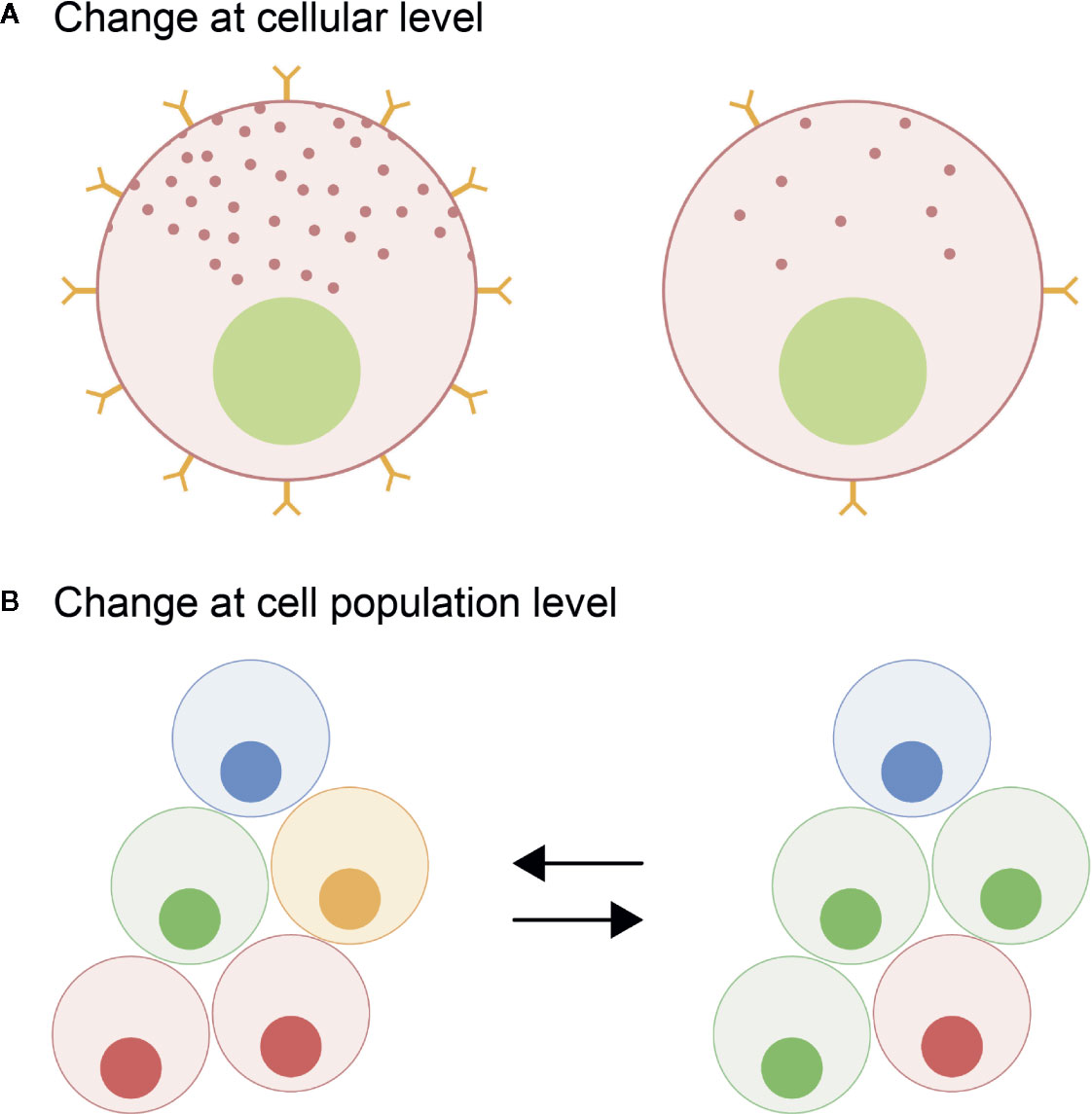
Figure 1 Schematic representation of the plasticity of the pituitary cells leading to a change in hormone production quantity. At the cellular level (A), the activity of the endocrine cell (hormone synthesis and release) can be modulated through the regulation of the number of different receptors thus changing sensitivity of the pituitary cells to inputs and/or by changing the hormone production rates. At the population level (B), the number of specific cell types can be modified changing the proportion of the different endocrine cell types in the pituitary.
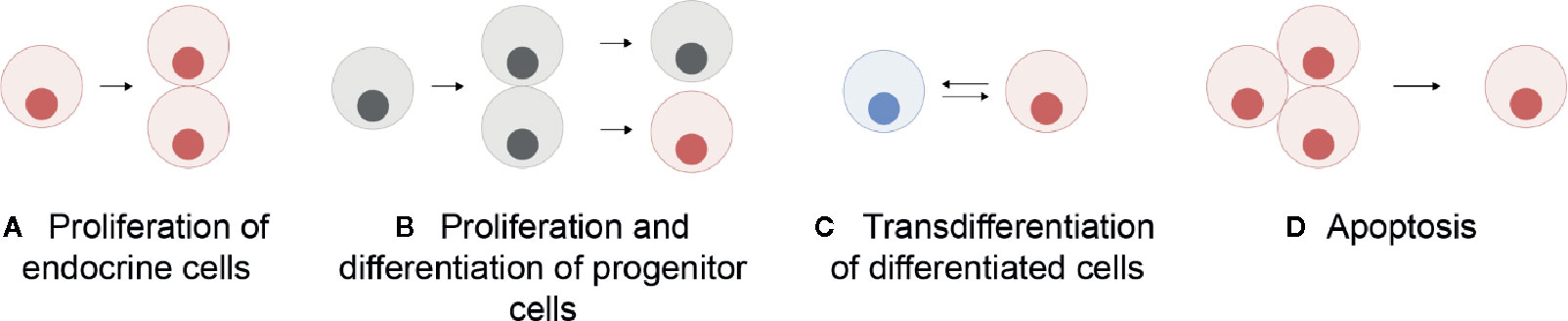
Figure 2 Schematic representation of the mechanisms allowing a change in the number of a specific endocrine cell type in the pituitary: proliferation (mitosis) of endocrine cells themselves (A), proliferation of progenitor cells followed by their differentiation (B), transdifferentiation (phenotypic conversion) of other differentiated cells (C), and cell apoptosis (D). Grey cells represent undifferentiated progenitor cells and colored cells represent differentiated cells.
The pituitary endocrine cell population with the highest capacity for plasticity is likely the gonadotropes. Gonadotrope plasticity (cell activity and cell number) is regulated by a myriad of brain factors primarily released from the preoptic-hypothalamic region. The main brain factors in this regard are gonadotropin-releasing hormone (Gnrh), dopamine (DA), and Kiss in teleosts (Figure 3; for review see (7, 14, 15)). Gnrh serves as the main stimulator of gonadotropes (7, 16). The neuro-hypothalamic Kiss system also plays an important stimulatory role (15). In contrast, DA appears to be the main gonadotrope inhibitor (7, 17). Other neuroendocrine factors regulating gonadotropes include Gnih, neuropeptide Y, GABA (7), but these will not be discussed in this review.
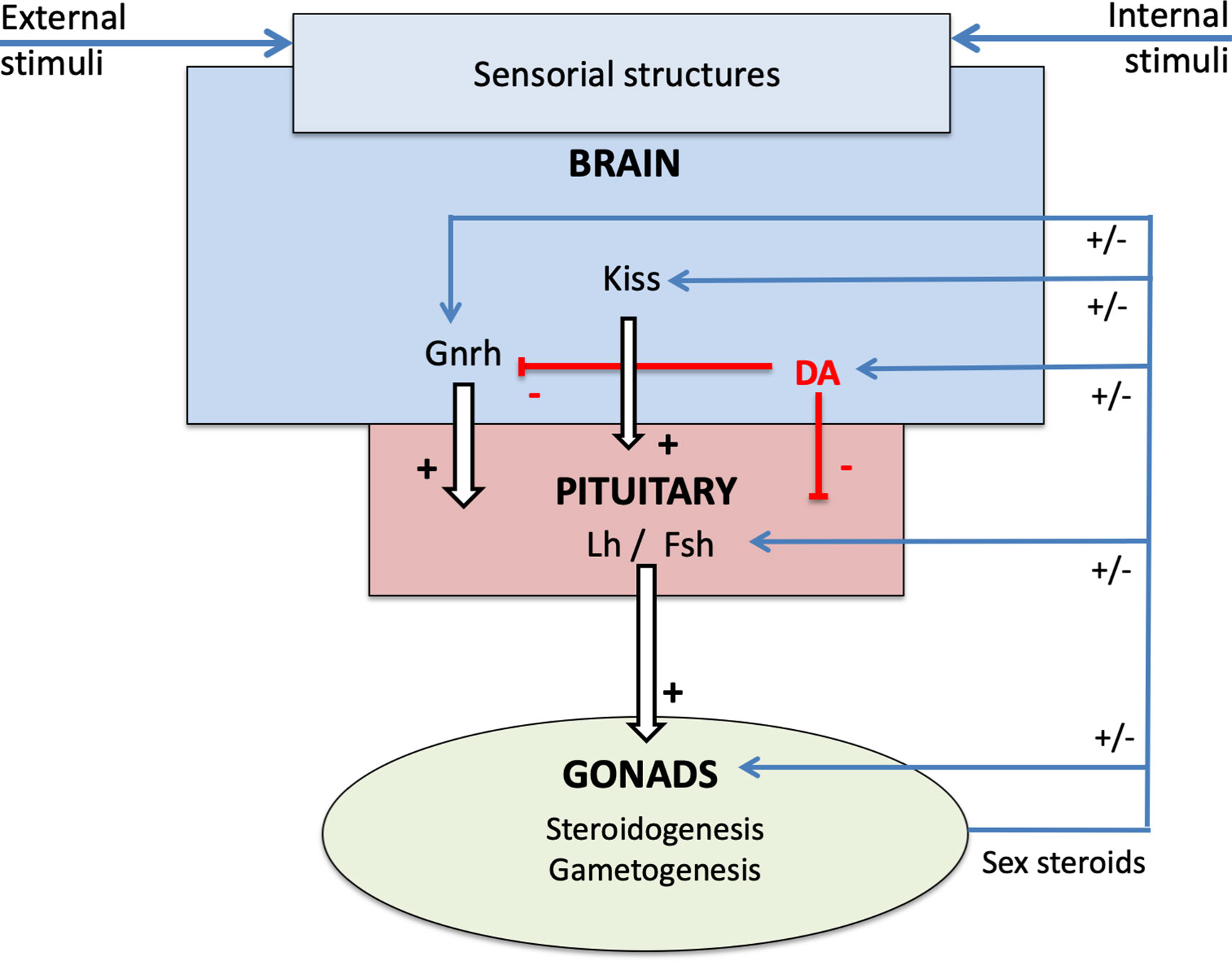
Figure 3 Schema of the neuroendocrine control of reproduction in teleosts, presenting the brain-pituitary-gonad (BPG) axis and the role of sex steroids in the retro-controls at the different levels.
In addition to signals from the brain, the plasticity of gonadotropes is also modulated by negative and positive feedback via endocrine signals from peripheral organs. Among these signals are the sex steroids. Sex steroids are synthesized from cholesterol, predominantly by the steroidogenic cells of the gonads, and circulate at different levels in males and females (18). Two major classes of sex steroids, androgens and estrogens, were classically delineated as male- and female-specific hormones as they were mainly synthesized by the testes and ovaries, respectively, and found to promote male and female secondary sex characteristics. We now know that both androgens and estrogens are essential regulators in both males and females.
Steroidogenesis primarily occurs in the gonads, in testicular Leydig cells in males, and ovarian granulosa and theca cells in females (19). However, granulosa cells are not strictly steroidogenic but process steroid precursors from theca cells. For instance, testosterone (T) from theca cells is aromatized in granulosa cells to 17β-estradiol (E2), the most prevalent and potent form of circulating estrogen in fish, a process regulated through maturation-dependent levels of aromatase. Aromatase is a member of the P450 cytochrome enzyme superfamily and encoded by the cyp19a1 gene, which exists in two forms in teleosts: cyp19a1a and cyp19a1b, the former expressed in the ovary and the latter in the brain and pituitary of both sexes (20, 21). While T and the even more potent, non-aromatizable, hormone 5α-dihydrotestosterone (DHT) are the active androgens in mammals, the non-aromatizable 11-ketotestosterone (11-KT) is the main active androgen in most teleosts (22, 23). However, DHT is also found in the circulation in both male and female fathead minnow (Pimephales promelas) (24, 25), and is the predominant steroid produced by urohaze-goby (Glossogobius olivaceus) testis tissue in vitro (26). Moreover, activity of 5-α reductase, which converts T to DHT, has been detected in many tissues, including the brain and pituitary, in several teleost species (25, 27), indicating that there may be a still undescribed biological role of DHT in teleosts. Finally, there is a third class of vertebrate sex steroids, the progestogens, which can be converted through several steps to T, 11-KT and E2, cortisol, and other steroids (18), but will not be further covered in this review.
Interestingly, in vertebrates, sex steroids can also be produced in other tissues, including the central nervous system, either via de novo synthesis from cholesterol or from other steroid intermediates produced in the periphery, thus allowing the tissue to autonomously utilize and modulate local steroid signaling (28, 29). In teleosts, expression of numerous enzymes, including aromatase, involved in sex steroid biosynthesis has been described in several brain areas (30, 31).
In target cells, sex steroids bind both nuclear and transmembrane receptors driving complex signaling responses (for reviews see (32–34)). In most vertebrates, genome duplications have led to two cytoplasmic estrogen receptors (ESR; ESR1 and ESR2), and one androgen receptor (AR). Teleost fish, however, often possess multiple paralogs of each receptor due to the additional whole genome duplications that occurred before and within the teleost group (3R/4R), adding complexity to sex steroid receptor signaling. Indeed, in most teleost species, while a single Esr1 has been maintained, two Esr2 (Esr2a and Esr2b), and two Ar (Arα and Arβ) have been conserved (35, 36).
Considering the progress in the field made in recent years, this review aims to summarize current knowledge regarding the effects of androgens and estrogens on gonadotrope activity and number in teleosts. Because these steroids can act on all levels of the BPG axis, we also aim to delineate their indirect effects via the brain from their direct effects on the gonadotropes.
Sex Steroids Are Mediators of Gonadotrope Plasticity
Effects on Gonadotrope Activity
Effects on Sensitivity to Regulatory Signals
Sex steroids can alter gonadotrope activity by changing gonadotrope cell sensitivity to regulatory signals, through modulation of the levels of Gnrh or DA receptors. Although molecular mechanisms by which steroids regulate Gnrh and DA receptors are well documented in mammals, much less is known in fish.
In mammals, GnRH signaling occurs through two paralogous receptors (37), whereas additional paralogs have been identified in teleosts, with up to six receptors in Atlantic salmon (38). In spite of this genomic difference, effects of sex steroids on GnRH receptor expression has been found across taxa. For example, E2 up-regulated pituitary GnRH-R expression in several mammalian studies, including in cows (39, 40), rats (41, 42), and ewes (43, 44), while T also up-regulated pituitary GnRH-R in rats (45). In teleosts, the effects of sex steroids on gnrhr expression have been investigated in only a limited number of species which have yielded disparate results. In the European sea bass (Dicentrarchus labrax), Gnrh receptor transcript levels in gonadotropes strongly increased at the time of spawning, when E2 is elevated (46), suggesting a stimulatory effect of E2 as seen in mammals. Conversely, in African catfish (Clarias gariepinus), castration increased pituitary Gnrh receptor content (47). This effect was reversed by treatment with androstenedione (AS), but not with non-aromatizable 11β-hydroxyandrostenedione (11β-OHA4). Finally, in goldfish (Carassius auratus), T-enhanced Lh-responsiveness to Gnrh was shown to be independent of changes in pituitary Gnrh receptor affinity or number (48).
Interestingly, among teleost species studied, only one of the multiple paralogs appears to be directly up-regulated by sex steroids in each species. Furthermore, if we apply the nomenclature and molecular phylogeny from (38) for gnrhr genes to the published studies, we find that it is the same isoform across species. For example, E2 treatment in female Nile tilapia (Oreochromis niloticus), increased mRNA levels of both gnrhr2ba1 (gnrhr1, according to the authors) and gnrhr2ba2 (gnrhr3) in vivo, but only gnrhr2ba1 (gnrhr1) in vitro, suggesting E2 directly regulates gnrhr2ba1 but indirectly regulates gnrhr2ba2 (49). Similarly, in the male black porgy (Acanthopagrus schlegeli), E2 and T increased the expression of gnrhr2ba1 (gnrhrI) (50, 51), but not gnrhr1cb (gnrhrII) (50). Finally, in pituitary cultures from Atlantic cod (Gadus morhua), E2 and T stimulated the expression of gnrhr2ba1 (gnrhr2a) but not gnrhr1cb (gnrh1b) in mature and post-spawning fish, and DHT increased expression of only gnrhr2ba1 in post-spawning fish (52). While three species cannot represent the entire diversity of teleosts, they suggest that this receptor acquired a sensitivity to sex steroids in a common ancestor and this has been conserved through evolution.
In mammals, it is well established that E2 modulates pituitary expression of type 2 dopamine receptors (D2), as demonstrated in rats (53–55). Regarding DA receptors, two DA receptor families have been described in vertebrates: type 1 dopamine receptors (D1) and D2. Similar to Gnrh-r, multiple paralogs within each family are found in teleosts (56). In vitro experiments have demonstrated that pituitary D2, but not D1, plays a role in the dopaminergic inhibition of gonadotropin synthesis and secretion in several teleost species (17). D2 has been localized to the PPD in many teleosts, and to Lh cells in rainbow trout (Oncorhynchus mykiss) (57) and zebrafish (58). Sex steroid regulation of pituitary D2 has been studied in few teleost species, with divergent effects. In rainbow trout, D2 antagonist decreased the stimulatory effect of Gnrh3 on Lh cells (59), whereas in Nile tilapia, d2 mRNA levels increased in females following E2 treatment, both in vivo and in vitro (49). In European eel, neither E2 nor T affected pituitary d2 levels (60).
Effects on Hormone Synthesis and Secretion
Gonadectomy (GDX) experiments and in vivo or ex vivo steroid treatments in a wide variety of species have demonstrated the significant role of the gonadal feedback loop in regulating gonadotropin synthesis and secretion in teleosts, with sex steroids exerting both negative and positive effects (Table 1).
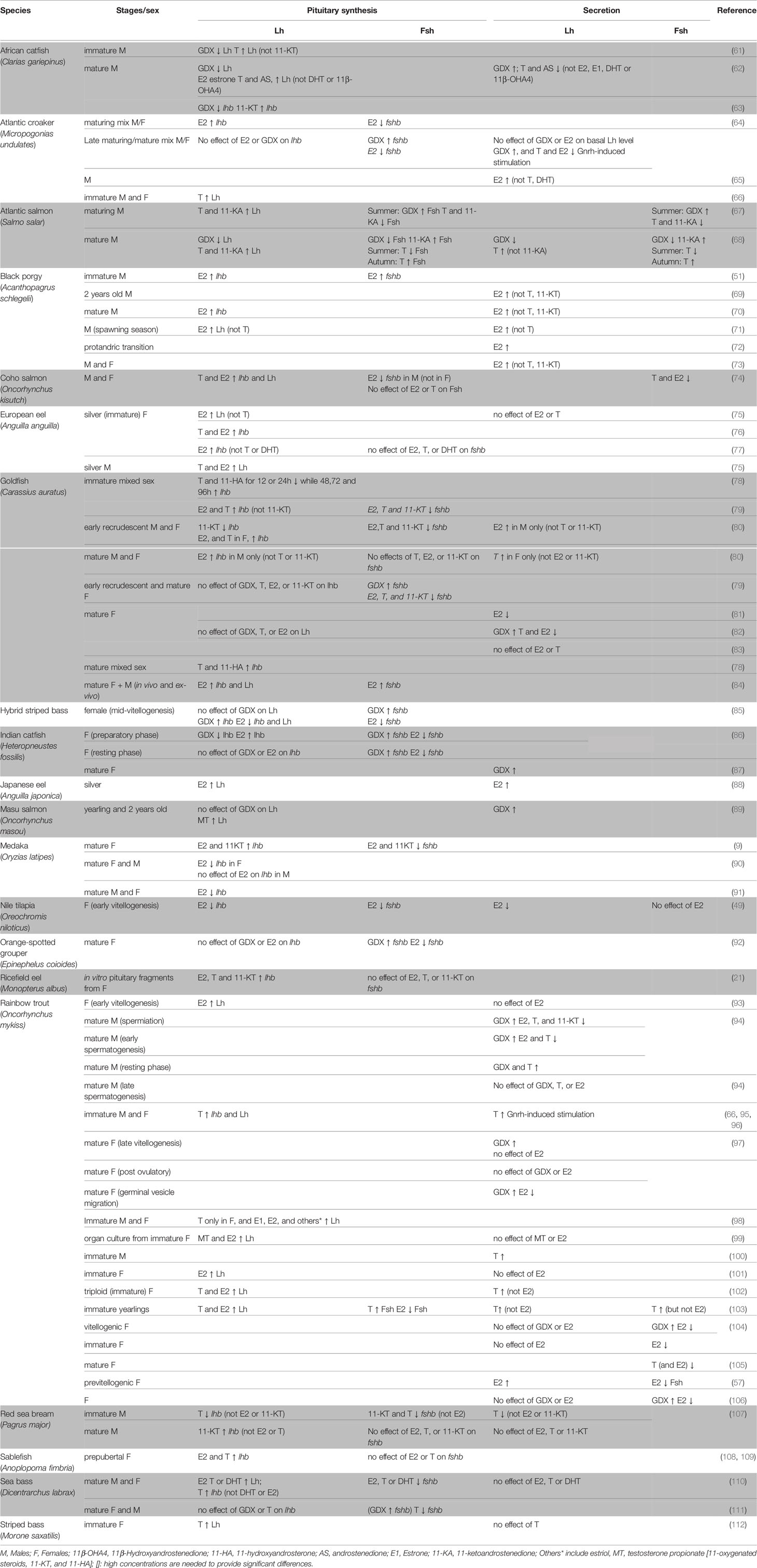
Table 1 Effects of gonadectomy or sex steroid treatments in vivo or ex vivo in different teleost species.
Clearly, the effects of sex steroids on Lh and Fsh synthesis depend on developmental stage, reproductive status, sex, and even the duration and dose of the experimental treatment. For instance, in goldfish, lhb mRNA levels increased in juveniles, but not adults, following T and E2 treatments (79, 82). Sex-specific effects have been seen in European eel (Anguilla anguilla), where intraperitoneal E2 injections strongly increased pituitary Lh levels in immature eels of both sexes, while T strongly stimulated pituitary Lh level only in males (75). Similar sex-specific effects were observed for fshb transcripts in coho salmon (Oncorhynchus kisutch), where E2 was found to inhibit fshb in males but not in females (74). Dose-specific responses were reported in medaka, where high concentrations of E2 decreased lhb mRNA levels (90, 91) while more physiological levels stimulated lhb synthesis (9). Interestingly, in coho salmon, pituitary Fsh levels increased following administration of the non-aromatizable androgen 11-ketoandrostenedione (11-KA, a precursor of 11-KT), whereas T suppressed pituitary Fsh in summer but stimulated it in autumn (113), suggesting season-specific functions of T and an important role of aromatase in mediating negative feedback.
Secretion of Lh and Fsh are also affected by sex steroids, with differential effects depending on the species, sex, and/or maturational stage of the animal (Table 1). For instance, in goldfish, Lh release was stimulated by T in mature females and by E2 in early recrudescence in males (80). Studies in rainbow trout reported that T increased Fsh plasma levels in immature fish (103) but decreased in mature fish (104–106). Opposite effects were found in mature male Atlantic salmon (Salmo salar), in which T inhibited Fsh release in summer during gonadal maturation, but stimulated Fsh release during the autumn spawning period (68). Sex steroids can also influence gonadotrope cell activity by modulating gonadotrope response to Gnrh. For instance, in Atlantic croaker (Micropogonias undulates), a study found no effect of E2 on basal plasma Lh levels but an inhibitory effect on Gnrh-induced Lh release in mixed-sex adults (64). In goldfish, E2 and T potentiated the Gnrh agonist (Gnrh-a) effect on Lh secretion in vivo in a season-dependent manner (83)
Gonadotropin synthesis and release can be differentially affected by sex steroids within the same organism, as shown for example in rainbow trout where E2 stimulated Lh synthesis but did not affect Lh release (93, 99, 101–103). Finally, Lh and Fsh can be oppositely regulated within in the same species, both at the secretion and expression levels. For instance, in previtellogenic female rainbow trout, E2 implants increased plasma Lh level but decreased plasma Fsh (57), and in male coho salmon, E2 increased pituitary mRNA levels of lhb but decreased fshb (74).
Effects on Gonadotrope Cell Populations
In addition to changes in gonadotrope cell activity, plasticity also results from changes in gonadotrope numbers and pituitary reorganization (Figure 2). For example, in European sea bass, while gonadotropes are only located in the PPD in immature fish, they tend to also colonize the periphery of the pars intermedia (PI) during maturation (46). Such population-level plasticity can make it difficult to discern whether steroid-induced changes in hormone mRNA or protein levels, detected by quantitative approaches such as ELISA or qPCR on the whole tissue, are due to changes in cell activity or cell number. Gonadotrope population changes in the pituitary can be due to proliferation of gonadotropes (Figure 2A), differentiation of progenitor cells, (Figure 2B) transdifferentiation (Figure 2C), and cell death (Figure 2D). These mechanisms underlie many changes in the rates of synthesis and release of Fsh and Lh by the pituitary, and there is increasing evidence that the sex steroids may play a role in these processes.
Proliferation
In teleosts, the pituitary grows throughout the lifespan. Evidence that pituitary endocrine cells are mitotically active has been illustrated at the electron microscope level in the mosquito fish (Gambusia affinis) (114). More specifically, the number of gonadotropes can change according to life stage or other factors, such as social status. For example, Lh cell number increased in juvenile male African catfish as spermatogenesis progressed (61, 115), and in both male and female medaka, the numbers of both Lh and Fsh cells increased between juvenile and adult stages (91, 116). In Nile tilapia, Fsh cell numbers were higher in dominant than subordinate males (117).
Furthermore, some studies demonstrate that gonadotrope proliferation can be controlled by sex steroids. In medaka, for instance, gonadotrope proliferation following steroid treatment was documented using double staining with proliferation markers Pcna and BrdU (91, 116). Exposure to T or E2, but not 11-KT, stimulated Lh and Fsh cell proliferation in both males and females, suggesting a positive effect of E2 on gonadotrope cell proliferation. In contrast, in zebrafish larvae, the number of Fsh cells was significantly lower in E2-treated fish than in controls (118), suggesting that E2 inhibits Fsh cell proliferation during early development in zebrafish. An earlier study in juvenile male African catfish showed that the number of Lh gonadotropes with numerous Lh-containing granules increased following T treatment (61). However, the authors did not detect differences in pituitary cell proliferation between androgen-treated and control fish and therefore speculated that androgens might activate quiescent gonadotropes. Whether these divergent results are due to species or stage differences remains to be investigated.
Differentiation of Progenitor Cells
Differentiation of progenitor cells may also increase gonadotrope cell numbers, but the evidence of a role of steroids in this process is not conclusive. In mammals, Sox2-expressing cells were shown to comprise a pool of pluripotent progenitor cells that proliferate and differentiate to either replenish pituitary cell populations or increase the absolute numbers of cells, including gonadotropes (119–122). These multipotent progenitor cells have been found in the pituitary cleft of mice (123–125) and rats (125, 126), lining the intraglandular structure bordering the adenohypophysis and neurohypophysis. While E2 treatment inhibited SOX2 expression in human embryonic stem cells in vitro (127), other studies suggest that SOX2+ cell proliferation is stimulated by E2 treatment (128). This indicates that progenitor stem cell proliferation, and perhaps differentiation, may be regulated by sex steroids in mammals. In teleosts, however, multipotent sox2-expressing cells have only been detected in the brain (129–132) and retina (133). Pituitary sox2-immunoreactive cells have, to our knowledge, only been identified in one teleost study, where they were localized at the junction of the adenohypophysis and neurohypophysis in medaka (91). However, evidence that these cells are pluripotent and contribute to endocrine tissue renewal in teleosts is lacking.
Another marker, S-100, has been widely used to identify follicular stellate (FS) cells, which are non-endocrine cells networked by gap junctions throughout the anterior pituitary in mammals [for review, see (134, 135)]. FS cells are thought to be progenitor cells and thus involved in pituitary cell renewal and plasticity (136, 137). Interestingly, mammalian FS cells have been found to be sex steroid sensitive. Indeed, in male rats, GDX decreased the number of gap junctions, but T replacement maintained their numbers (138, 139). Similar observations were made in females where OVX reduced the gap junction number while E2, and to a lesser extent T, partly restored it (140). However, the role of sex steroids in FS cell proliferation or differentiation remains unknown in mammals.
Pituitary non-secretory (agranular) cells have been described in several teleost species, including the southern mouth-brooder (Pseudocrenilabrus philander) (141), sailfin molly (Poecilia latipinna) (142), ironfish (hybrid between the Funa (Carassius carassius) and goldfish) (143), stickleback (Pungitius pungitius L) (144), European eel (145), Mediterranean yellowtail (Seriola dumerilii) (146), grey mullet (Mugil cephalus) (147), Arabian toothcarp (Aphanius dispar) (148), and white seabream (Diplodus sargus) (149). In Nile tilapia, FS cells were observed to network via gap junctions (150). In the Japanese eel (Anguilla japonica), it was demonstrated that aromatase-positive cells, most likely corresponding to FS cells, express the proliferation marker PCNA (151). Such cells are suspected to have the same origin as the aromatase positive radial glial cells in the brain that act as progenitors throughout life (152). These results suggest that the proliferation of such cells could be sex steroid dependent, but this remains to be systematically investigated.
Transdifferentiation
The third mechanism that remodels gonadotrope populations is known as transdifferentiation, defined by (153–155) as the change from one hormone producing cell type into another. Transdifferentiation may allow the pituitary to appropriately respond to certain physiological and pathological conditions (156). While experiments have generated evidence of the phenomenon, the molecular mechanisms mediating such transformations are still enigmatic.
In teleosts, a recent study in medaka demonstrated that Fsh cells commenced lhb production in vitro, indicating the capability of a fully differentiated cell to transdifferentiate into another cell type (116). However, transdifferentiation between other pituitary cell types has not been reported in teleosts, and the role of sex steroids in transdifferentiation has not been investigated to date.
In contrast, several examples of pituitary endocrine cell transdifferentiation have been described in mammals. For instance, a study in adult mice found that stem-somatotropes can populate the pituitary with both somatotropes and lactotropes (157, 158). Similarly, studies in rats (159, 160) and humans (161) suggest that the proportions of somatotropes, lactotropes and mammosomatotropes (GH+/PRL+) in the adenohypophysis vary among non-pregnant, pregnant and lactating females due to cell transdifferentiation. More direct evidence of transdifferentiation of somatotropes into lactotropes is provided by in vitro studies (162, 163). In mammalian species, reversible interconversion has also been observed between somatotropes and lactotropes (164), somatotropes and thyrotropes (130, 153, 165–167), and somatotropes and gonadotropes (168).
While direct evidence for the role of sex steroids in cell phenotypic interconversion is also limited in mammals, there is some indirect evidence. In female dogs, estrogen deficiency due to ovarian dysfunction leads to an increase in gonadotropes, attributed in part to transdifferentiation (169). In rats, the production of Fshb and Lhb by somatotropes was coincident with a dramatic increase in Esrβ expression (168), suggesting that estrogen may regulate gonadotrope population remodeling via transdifferentiation.
Cell Death
Pituitary cell apoptosis is considered necessary to ensure the balance between cell renewal and cell loss and permit optimal response to physiological demands (170). Although experimental evidence of sex steroids regulating endocrine pituitary cell apoptosis is lacking in teleosts, there is ample evidence in mammals of both androgens and estrogens modulating apoptosis in such cells, including gonadotropes (170, 171). For instance, in proestrus rats, E2 has been reported to increase anterior pituitary cell apoptosis, predominately in gonadotropes, both in vitro and in vivo (172, 173). Another study in female rats reported that gonadotrope proliferation is lowest during diestrus (when E2 is lowest) before rising gradually until the estrus phase (174), suggesting that E2 may exert either anti-proliferative or apoptotic action to maintain an appropriate gonadotrope population in mammals. Whether apoptosis regulates the number of endocrine cells in teleosts remains to be elucidated.
Conclusion
As shown above, it is now well established that sex steroids participate in the regulation of gonadotrope plasticity. The effects mainly occur at the cellular level by modulating the sensitivity of the endocrine cells to ligands through the regulation of the number of receptors, or by regulating endocrine cell activity (hormone synthesis and release). It also appears that in at least some species, increased gonadotropin production can result from gonadotrope cell division or progenitor cell differentiation. However, these and other population-level processes remain poorly characterized in teleosts. Therefore, there is a need for further studies to elucidate the underlying mechanisms regulating gonadotrope cell number and the potential role of sex steroids.
Sex Steroids Mediate Gonadotrope Plasticity Directly and via the Brain
Because of the strong regulation of gonadotropes by the brain, it can be difficult to distinguish whether signaling molecules act directly at the pituitary, or instead modulate the gonadotrope regulatory systems in the brain. For example, in ex vivo medaka brain and pituitary preparations, Fsh cells exhibited a calcium response to Gnrh stimulation (175), but in dissociated cell cultures Fsh cells did not (176), suggesting an indirect effect of Gnrh on Fsh cells. For sex steroids, it is particulary complicated as sex steroids can simultaneously act at all levels of the BPG axis, making it difficult to determine whether the observed effects are directly on gonadotropes, mediated through the brain or another pituitary cell type, or both. Therefore, a combination of in vivo or ex vivo and in vitro techniques can help discriminate direct from indirect effects. In addition, investigation of Esr and Ar expression in brain and pituitary cells in vivo have identified some direct targets of sex steroids and thus have helped to decipher the pathways used for the regulation of gonadotrope plasticity, although much work remains.
Brain Mediated Effects
ESRs, and to a lesser extent ARs, have been found to be widely expressed in the brains of vertebrates (177, 178), including teleosts (Table 2). While esrs and ars are expressed in many different brain areas, numerous teleost studies have demonstrated that both esrs and ars are highly expressed in the classical neuroendocrine regions of the brain such as the POA and the mediobasal and caudal hypothalamus (221). The high expression of esrs and ars in the brain provides histological support for the sex steroid feedback control on gonadotropin synthesis/secretion and gonadotrope proliferation which may be relayed through regulators from the brain, notably Gnrh, DA, and kisspeptins. Therefore, any change in neuronal activity (synthesis or secretion) or cell number (neurogenesis or neurodegeneration) in the populations producing such factors might affect gonadotrope activity.
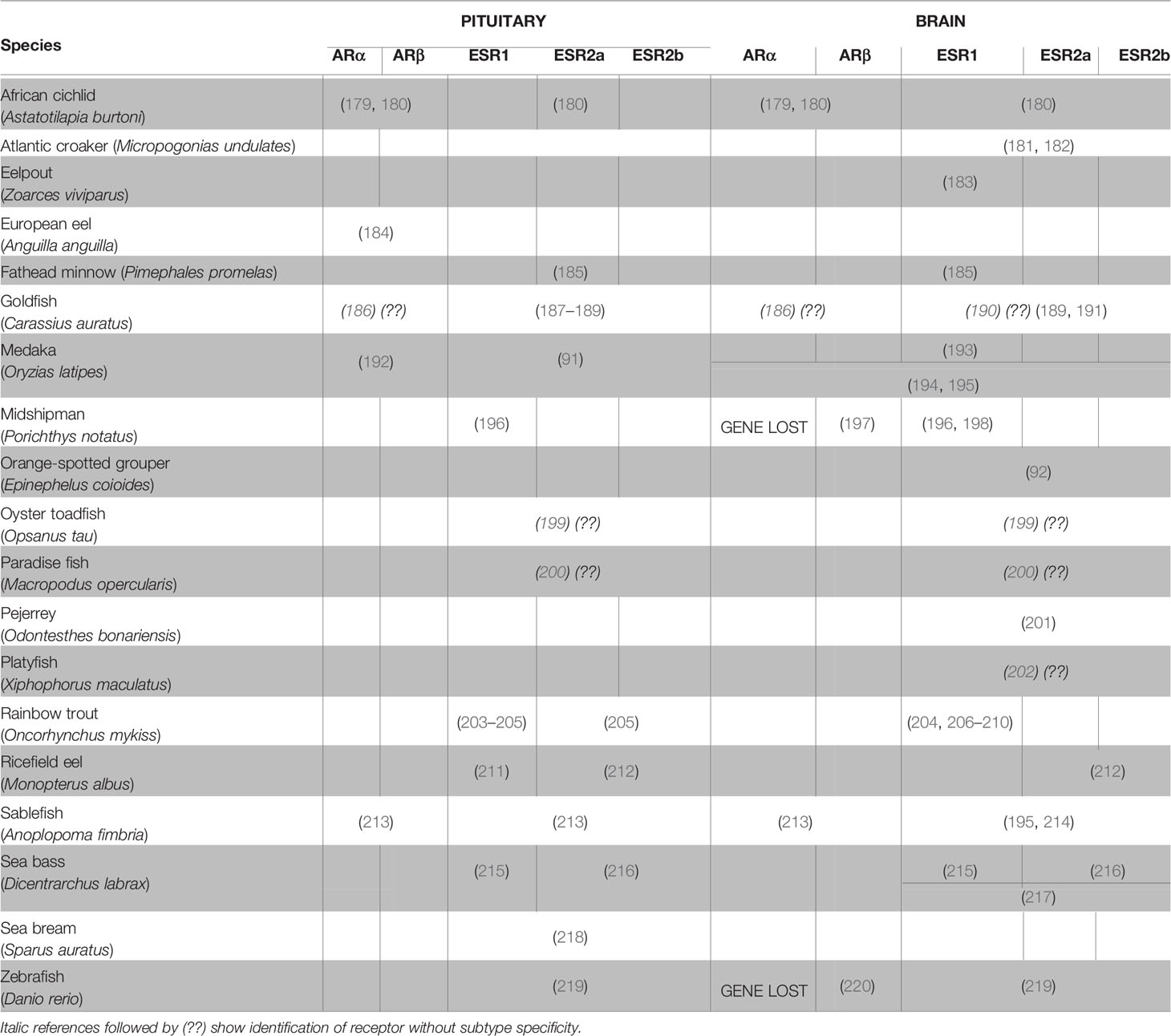
Table 2 Literature on the expression of androgen and estrogen receptors in the brain and the pituitary of teleosts.
Gnrh System
The Gnrh system is the main stimulator of gonadotrope cell activity. Gnrh exerts its effects through three Gnrh paralogs, classified according to lineage: Gnrh1, Gnrh2, and Gnrh3 (222, 223). While both Gnrh receptors and Gnrh3 or Gnrh2 fibers have been observed in the retina and the pineal gland respectively, hypophysiotropic Gnrh1, or Gnrh3 in those teleost species lacking Gnrh1, serves as the main stimulator of gonadotropes by projecting in close proximity to gonadotrope cells in the pituitary [for review, see (15)].
Gnrh is regulated by sex steroids in both mammals [for review, see (224) and (225)] and fishes. In teleosts, sex steroids can stimulate or inhibit the activity of Gnrh neurons, thus indirectly regulate gonadotrope function, as shown in Table 3 and previously discussed in (240). The effects of sex steroids seem to depend on the specific Gnrh cell population and the maturation stage of the fish. For instance, in yearling masu salmon (Oncorhynchus masou), castration in under-yearling precocious males increased gnrh3 mRNA levels in the ventral telencephalon but not in the POA, suggesting that the Gnrh3 cell populations are differentially regulated by gonadal steroids (89). In medaka, E2 significantly suppressed gnrh expression in embryos (233) but not in adults (90), suggesting that the effect might be stage-specific in some species. Interestingly, in the spotted scat (Scatophagus argus), E2 inhibited gnrh1 expression in a dose-dependent manner and this effect was abolished by a broad spectrum Esr antagonist or an Esr1-specific antagonist, but not by an Esr2 antagonist (238), which suggests that Esr1 mediates the inhibitory effect of E2 in this species.
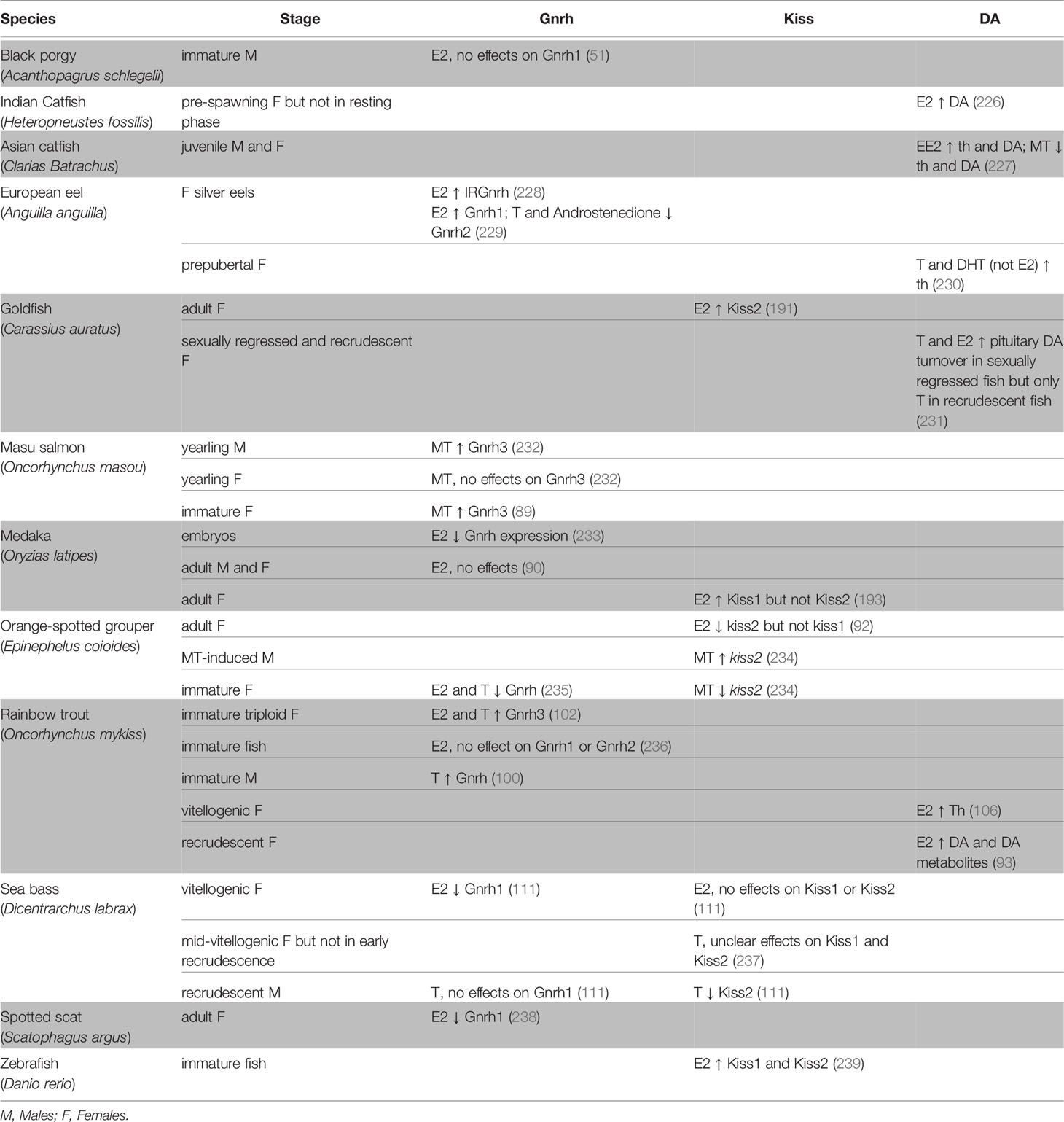
Table 3 Effects of sex steroids on the brain and the main neuroendocrine factors involved the regulation of gonadotrope function: Gonadotropin-releasing hormone (Gnrh), Kiss and Dopamine (DA).
In mammals the limited expression of Esrs in Gnrh neurons indicates that steroids do not exert significant feedback directly to these neurons (for review, see 149 and 150). Similarly, esrs were not found in Gnrh neurons in the rainbow trout (241). However, a few studies have reported the presence of Esrs and Ars in teleost Gnrh neurons, suggesting the possibility of a direct sex steroid feedback. The erα paralog was found to be expressed in Gnrh3 neurons in medaka (195) and in Gnrh1, 2, and 3 neurons in Nile tilapia (242), while both arα and arβ were found in Gnrh1 neurons in the cichlid Astatotilapia burtoni (179). Thus, it is possible that sex steroids might regulate Gnrh activity and proliferation directly in teleosts, although as described below there is more evidence of indirect pathways via effects on Gnrh regulatory factors (e.g., kisspeptin and dopamine), as seen in mammals.
Kiss System
Kisspeptin (Kiss), a member of the (RF)-amide peptide family, has been recognized as an important regulator of reproduction in vertebrates. In mammals, Kiss neurons in the POA and the mediobasal hypothalamus are believed to stimulate the synthesis and secretion of Gnrh and mediate feedback by sex steroids (243–245). However, in teleosts, a study in striped bass showed that Kiss regulates gonadotropes in a Gnrh-independent manner (246). Recently, a study in zebrafish reported that Kiss directly stimulates lhb and fshb expression in pituitary in vitro culture, therefore suggesting that Kiss more directly regulates gonadotropes in teleosts than in mammals [(247) and reviewed in (15)].
While Kiss expression is stimulated by E2 in rodents (248, 249), the existence of kiss paralogs (kiss1 and kiss2) in teleosts (250, 251) substantially increases the complexity of E2 regulation of kiss genes in these species (Table 3). Furthermore, which of the paralogs plays a role in the regulation of the BPG axis and steroid feedback may vary by species. For instance, in female medaka, kiss2 expression in POA does not vary with reproductive state or after OVX (193). However, kiss1 cell number was higher in reproductive fish compared to that in non-reproductive fish, and decreased significantly after OVX, which suggests sex steroids may exert positive feedback on kiss1 in this species. In contrast, in the orange-spotted grouper (Epinephelus coioides), the expression of kiss2, but not kiss1, significantly increased in OVX females, which was reversed with E2 treatment (92). However, in situ hybridization showed that both kiss1 and kiss2 neurons express esr1, esr2a, and esr2b, indicating that E2 may potentially regulate both kiss1 and kiss2 in this species. Esrs have also been described in kisspeptin neurons in other teleost species: erα in medaka (193) and European seabass (217), and esr1, esr2a and esr2b in goldfish (191, 252), suggesting that Kiss neurons may be directly regulated by estrogens in teleosts as in mammals.
Interestingly, androgens also modulate kiss expression with different effects depending on the species, sex, or reproductive stage (Table 3). For instance, a study in female European seabass reported that during mid-vitellogenesis, but not during early recrudescence, both GDX and T treatment after GDX significantly lowered kiss1 expression, but that kiss2 expression decreased only after T treatment in GDX animals (237). In the orange-spotted grouper, during MT-induced sex reversal from female to male, hypothalamic kiss2 transcript levels were significantly lower 1 week after methyltestosterone (MT) implantation in females (234). Levels remained low in the 2nd and 3rd weeks, but increased significantly in the 4th week, compared to controls. Interestingly, a second MT implant at the 3rd week significantly enhanced kiss2 expression. These results suggest that MT may stimulate kiss2 in males but suppress it in females in this species.
Dopaminergic System
Dopamine (DA), a catecholamine, is also known to modulate the levels of Gnrh through D2 type DA receptors (253), which subsequently regulate the activity and proliferation of gonadotropes as well as gonadotropin synthesis (17, 254). In teleosts, neurons that produce the tyrosine hydroxylase (Th) enzyme (the rate-limiting enzyme in catecholamine biosynthesis) and project to the pituitary have been localized in the POA, in close proximity of Gnrh neurons, in several species including goldfish (255), rainbow trout (206), European eel (256), zebrafish (257), and the cichlid A. burtoni (253).
Several studies have demonstrated the effects of estrogens and androgens on th expression or DA levels in teleosts (Table 3). The effects of sex steroids on the dopaminergic system seem to also depend on the maturation state. For instance, in the Asian catfish (Heteropneustes fossilis), OVX or E2 replacement in 4-week OVX fish did not significantly affect the DA system during the resting phase, but in the pre-spawning phase, OVX significantly decreased while E2 replacement increased DA levels (226). However, due to the limited number of studies and species investigated, it remains unknown whether the sex steroid regulation of DA is sex- or species-dependent.
Nevertheless, there is evidence that esrs are expressed in DA neurons in the POA of rainbow trout brain (206) which suggests a direct role of estrogens on the DA system. However, it is difficult to know whether the observed effects of DA on gonadotropes result from a direct action of DA or if they are indirectly mediated through the Gnrh system, as Gnrh neurons are also controlled by DA.
Apoptosis and Proliferation
Sex steroids have been shown to play important roles regulating certain neuronal cell populations in the brain through neurogenesis (29) and neurodegeneration (258) in vertebrates. Published research on the role of sex steroids on cell survival and apoptosis in the teleost brain is limited to a single study in adult male zebrafish, which found that cell survival was slightly reduced in several brain areas after E2 treatment (132).
There is more evidence of the effects of sex steroids on cell proliferation in the teleost brain. For instance, in adult male zebrafish, E2 treatment inhibited cell proliferation in several brain areas, whereas an aromatase inhibitor treatment tended to stimulate cell proliferation, although the effect was not significant in all regions studied (133). However, the same authors reported that fish treated with a high affinity Esr antagonist had higher numbers of proliferative cells in several brain regions, suggesting that E2 inhibits, rather than stimulates, neuronal cell proliferation in this species. An anti-proliferative effect of E2 was also seen in adult female zebrafish, where the number of proliferating cells labelled with BrdU decreased in several neurogenic brain regions, including the POA (214). However, opposite effects were observed in juvenile black porgy treated with E2, where levels of brain aromatase and numbers of proliferative cells increased (259). A significant reduction in brain cell proliferation was observed after treatment with an aromatase inhibitor, while castration did not affect the number of brain cells. This suggests that T itself may inhibit neurogenesis, but local E2 synthesis from aromatization of T may promote neurogenesis in this species.
Changes in specific neuroendocrine cell populations in teleosts due to sex steroids was first investigated in sex‐reversing fishes. In bluehead wrasse (Thalassoma bifasciatum), the number of Gnrh neurons in the POA was higher in males at the terminal phase of sex transformation than females or initial-phase males (260, 261). Additionally, the number of Gnrh neurons was shown to increase in females and initial-phase males, but not in terminal phase males, following 11-KT treatment, demonstrating a role of sex steroids on Gnrh neuron number (260, 261). However, whether this increase was due to cell proliferation or recruitment was not investigated. In Mozambique tilapia, Gnrh3 neurons are more numerous in males, and treatment with 11-KT or methyltestosterone (MT, a potent synthetic androgen), but not E2, increased the number of Gnrh3 neurons in females to a level similar to that in males, and modified the fish behavior (262). Recently, the same group showed that this phenomenon was due to proliferation by identifying newly formed Gnrh3 neurons after androgen treatment (263). In larval zebrafish, treatment with the synthetic estrogen 17α-ethinylestradiol (EE2) increased the numbers of forebrain Gnrh3 cells (264). The authors suggest that EE2 accelerated Gnrh3 neuron development as 5 dpf larvae treated with EE2 had similar numbers of Gnrh3 neurons as 20 dpf control fish. Similar effects of sex steroids were seen in immature African catfish, where testosterone increased the number of Gnrh1 neurons (265).
There is also some evidence that sex steroids may also affect kiss neuron number in teleosts. In medaka, the number of kiss1 neurons was observed to decrease after OVX in some brain regions, but was maintained with E2 treatment (193). However, it is not known whether this was due to a decrease of kiss1 expression in some neurons after OVX or if E2 treatment had a positive effect on cell survival. Finally, information on the effects of sex steroids on DA cell number is to date still lacking.
Direct Effects on Gonadotropes
Because no direct effects of sex steroids have been demonstrated on gonadotrope proliferation, transdifferentiation or cell death in vitro, this section will address the question of gonadotrope plasticity by considering changes in their activity only.
The Pituitary: A Target of Sex Steroids
As in other vertebrates, Esrs have been identified in the pituitary of many teleost species while Ars have been described in only a few species (Table 2).
Interestingly, in male wild sablefish (Anoplopoma fimbria), pituitary esr1 and ara mRNA levels were positively correlated with those of lhb, whereas esr2a and esr2b were correlated with fshb transcripts during gametogenesis (213). However, whether the alterations in esr or ar levels occurred in gonadotropes remains to be determined. Indeed, the specific cell types within the pituitary that express these receptors have only been investigated in a few teleost species. In European sea bass, high expression of esr1, esr2a, and esr2b mRNAs was localized to the PPD and PI, and double label in situ hybridization demonstrated that both Fsh and Lh cells express esr1, esr2a, and esr2b transcripts (215, 216). In ricefield eel (Monopterus albus), esr1, but not esr2, was expressed in Lh cells (211, 212). In medaka, Lh cells expressed all three isoforms, with esr1 and esr2b most predominately expressed (91). In contrast, nothing is known regarding the presence of ars in teleost gonadotropes.
In summary, the localization of both Esrs and Ars in the pituitary, and the presence of Esr in gonadotropes support a direct effect of sex steroids on gonadotropes. Yet, further studies are needed to identify which pituitary cell types express which receptors and thereby provide stronger evidence of direct signaling, and to determine whether expression patterns vary among species and with stage of sexual maturity.
Aromatization of Androgens in the Pituitary: A Production Site of Estrogens
Using aromatase inhibitors (51, 211, 266) or a combination of aromatizable and non-aromatizable androgens (62, 79, 91, 116), several studies have clearly demonstrated that aromatase, by converting T into E2, plays a role in the cellular responses to T observed in teleosts.
Aromatase has been identified in the pituitary of all major vertebrate groups from fishes to mammals. However, experiments in goldfish, toadfish (Opsanus tau), and sculpin (Myoxocephalus octadecimspinosus) showed that teleost pituitaries have aromatase levels l00 –1000 times greater than those in mammals and other vertebrates (27, 267, 268). Since then, aromatase expression or activity has been demonstrated in the pituitary of many other teleost species such as African catfish (269), Atlantic salmon (270), Mozambique tilapia (Oreochromis mossambicus) (271), rainbow trout (210), channel catfish (Ictalurus punctatus), and zebrafish (272), midshipman fish (Porichthys notatus) (196), Atlantic cod (273), killifish (Fundulus heteroclitus) (274), black sea bass (Centropristis striata) (275), a neotropical cichlid fish (Cichlasoma dimerus) (276), sablefish (213), brown ghost knifefish [Apteronotus leptorhynchus) (277)], and black porgy (51).
Interestingly, in pejerrey (Odontesthes bonariensis), aromatase (cyp19a1b) expressing cells labeled by immunohistochemistry or in situ hybridization were found close to blood vessels in the pituitary (278). Moreover, aromatase has been located in pituitary cells in the Japanese eel (152) and larval zebrafish (264), in Lh cells, but not Fsh cells, in ricefield eels (211), and in both Lh and Fsh cells in medaka (91, 116). While no sex differences were detected in pituitary aromatase mRNA levels or enzyme activity in the pejerrey (278) and the Japanese eel (279), respectively, aromatase activity in European seabass (280), and cyp19a1b expression in the yellow perch (281) and a South American catfish (282), were higher in male pituitaries. This might explain the differential sex responses of gonadotropes observed following steroid treatment in some species. In larval zebrafish, EE2 exposure did not affect cyp19a1b levels in the pituitary, despite increasing levels in the forebrain (264).
Direct Effects on Hormone Synthesis and Release
In 1983, pituitary grafts (transplantation of pituitaries to another tissue) in rainbow trout revealed positive effects of T on pituitary and plasma Lh levels, indicating a stimulatory role of T on Lh synthesis and release (283). Stimulatory effects of androgens and estrogens have also been observed in studies using whole pituitary or fragments in cultures from rainbow trout (99) and ricefield eel (211). However, as paracrine signaling occurs between pituitary cells in vertebrates (284), including in teleosts as shown for instance in goldfish (285) and Grass carp (Ctenopharyngodon idella) (286), and pituitary endocrine cells can be stimulated by neuroendocrine factors despite the absence of cognate receptors, through cell-cell communication (176), using whole pituitary or fragments could mask potential direct effects from the steroids. Therefore, we argue that dissociated cell culture is the only suitable technique to investigate the direct effect of sex steroids (or other factors) on gonadotropin synthesis and secretion.
Studies using dissociated pituitary cell cultures from several teleost species have yielded convincing evidence for a direct role of sex steroids in the regulation of gonadotropes, mostly by stimulating lhb and fshb expression (Table 4). However, divergent effects of treatment duration have reported in juvenile eel pituitary cells for example, where no effects on lhb mRNA levels were observed after 24 h E2 treatment while a 72 h E2 treatment decreased it (288). In African catfish, both T and E2 inhibited lhb transcription after 24 h treatment but simulated lhb levels after 48 h treatment (287).
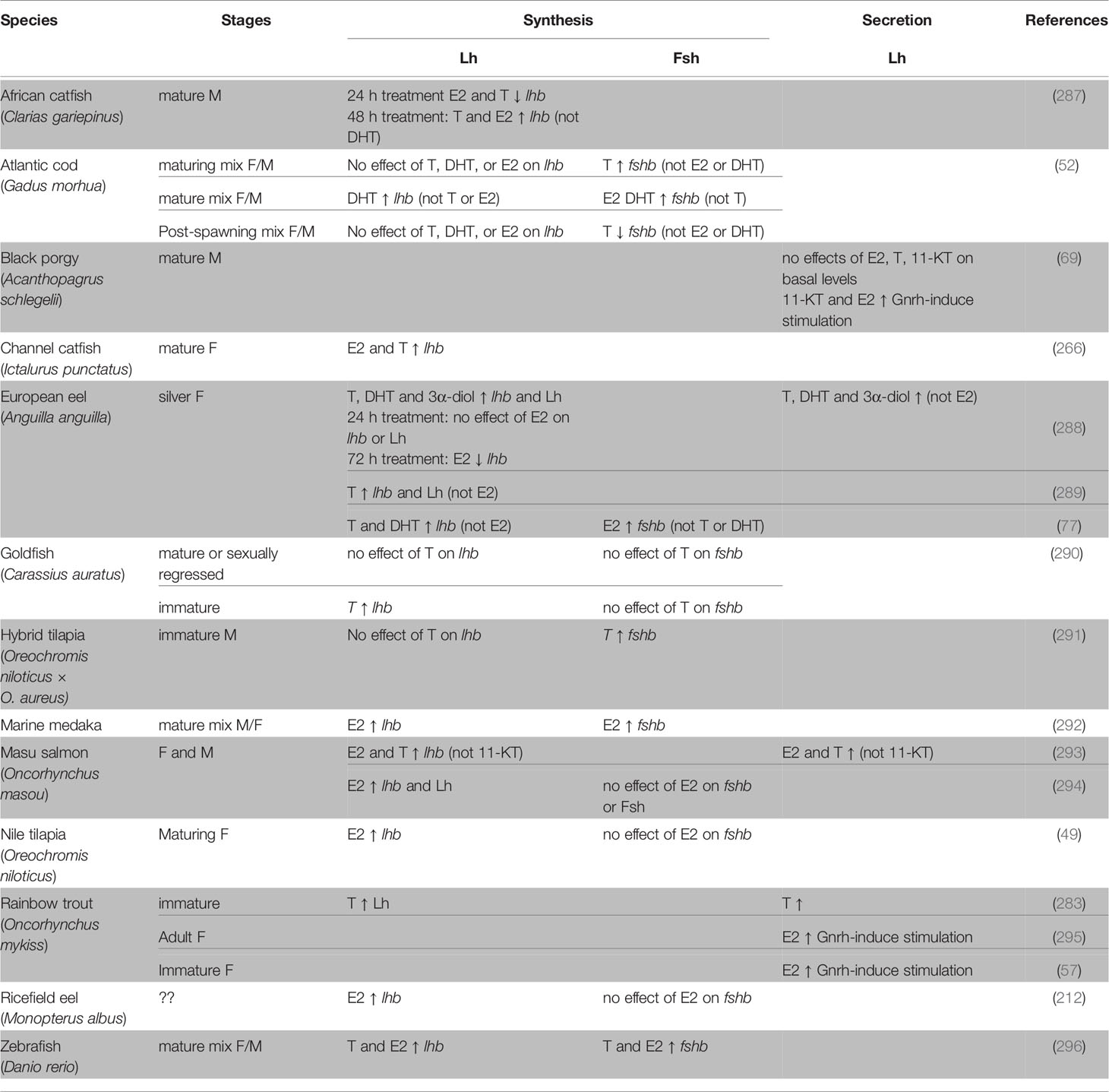
Table 4 Direct effects of sex steroids demonstrated by in vitro studies using dissociated pituitary cells from teleosts.
Interestingly, in the channel catfish, both E2 and T enhanced the expression of lhb, but the effect of T was abolished by an aromatase inhibitor (266), indicating an important role of aromatase and E2. This result is supported by other studies where T, but not non-aromatizable androgens, gave a similar effect on gonadotropin gene expression as did E2 treatment (77, 293). Several studies using dissociated pituitary cell cultures have also demonstrated a direct effect of sex steroids on Gnrh-induced Lh release (Table 4). For instance, E2 treatment increased Gnrh3-stimulated Lh release from female rainbow trout pituitary cells (57, 295).
Again, sex steroid effects on gonadotropes were found to vary with stage of sexual maturity and sex. For example, T stimulated fshb in cells from maturing Atlantic cod, had no effect in cells from mature fish and decreased fshb from post-spawning fish (52). In cells from masu salmon, the combination of Gnrh3 and E2 increased lhb mRNA levels and decreased those of fshb in males, but had no effect on lhb or fshb in females (297). These results indicate that E2 and Gnrh3 signaling differentially modulate gonadotropin synthesis and that effects might be sex-specific in this species.
Further evidence of the ability of sex steroids to directly modulate gonadotropin transcription is provided by the identification of steroid response elements (SRE) in gonadotropin promoters and by in vitro reporter assays. SRE are short, palindromic nucleotide sequences in target genes where steroid receptors bind to regulate transcription of those genes. Full-site and half-site estrogen response elements (ERE) have been identified upstream of the lhb gene in chinook salmon (298, 299), but only half-site EREs have been found in both fshb and lhb genes in Nile tilapia [lhb (300); fshb (301)] and goldfish [lhb (80); fshb (302)], and the fshb gene in chinook salmon (303) and sea bass (304). A half-site androgen response element (ARE) was also been identified upstream of fhb in sea bass (304). Using an in vitro reporter assay, the ricefield eel lhb promoter was activated highly by E2, and to a lesser extent by T and 11-KT, indicating the presence of functionally active ERE and ARE in the lhb promoter. Conversely, neither E2 or androgens activated the fshb promoter (211).
Conclusion
As shown above, it is clear that sex steroids act at both the brain and gonadotrope levels. However, there is still a need to further decipher direct from indirect effects of sex steroids. So far, the use of dispersed cell cultures has been the only way to confirm a direct effect of sex steroids on gonadotropes. However, precaution should be taken as gonadotropes have been shown to change phenotype after dissociation and seeding. Indeed, in a medaka study, Fsh cells which were shown to not possess Gnrh receptors in vivo and to not respond to Gnrh treatment in vitro 24 h after dissociation, did respond to Gnrh after 3 days in culture (116), suggesting that they begin to express Gnrh receptors during incubation. Therefore, the direct effects of sex steroids in dissociated cell cultures should be confirmed by the localization of sex steroid receptors in vivo in the cells of interest.
A lot of work remains to identify the cell types that express esr and ar, in both the brain and the pituitary which will help to elucidate the regulatory pathway. While Gnrh, Kiss and DA are the primary gonadotrope regulators, they are complemented by, and themselves controlled by, other factors including sex steroids. New techniques such as multicolor in situ hybridization and single cell transcriptomics will accelerate such research.
Finally, most of the direct effects observed on gonadotrope plasticity are about the activity (cell sensitivity and hormone synthesis and release). No data exist on the direct effect of sex steroids on the regulation of cell proliferation or cell death, which are difficult to address in in vitro work. Therefore, new approaches are needed to address these questions in the future.
General Conclusion and Future Perspectives
To conclude, any attempt to draw generalizations in teleosts, or to propose hypotheses on evolutionarily conserved mechanisms or ecological, developmental or sex-specific adaptations, is difficult due to an insufficient body of evidence. Indeed, although many studies have been performed on several species, the information is often conflicting, with differential effects of sex steroids among species, sexes, developmental stages, and reproductive states. Because effects can vary between males and females, or over the reproductive cycle within one species, it is evident that the signaling pathways can change according to the unique molecular environment. It must be recognized that teleost fish diverged over their 300 MY evolutionary history, and there is enormous variability among its nearly 30,000 species, in terms of both the environmental parameters of their habitats (salinity, light, temperature, etc.) and their reproductive strategies (iteroparity vs. semelparity, daily spawning vs. seasonal spawning, etc.). Thus, it is not surprising to find diversity among teleosts regarding the roles of sex steroids in mediating gonadotrope plasticity and the pathways by which they exert these effects. This high complexity of sex steroid signaling necessitates further research addressing the molecular mechanisms behind the observed responses. One mechanism underlying such variation is likely the differences in steroid levels across stages and between sexes, which has been extensively reviewed [e.g. (18, 305–307)]. A second mechanism is likely due to variation in the steroid modifying enzymes (e.g., aromatase) in the pituitary, leading to local changes in levels of specific steroids. A third mechanism is likely differences in the receptor subtypes and their numbers in target tissues, which might be differentially regulated by environmental and internal factors.
Fortunately, we have a wealth of powerful tools available to investigate the role of gonadal sex steroids. For instance, GDX, which is still used today in teleosts and adapted to new species of interest (308), is a powerful technique when combined with steroid replacement to investigate the role sex steroids play in tissue plasticity. However, GDX does not remove all sex steroids, as some can be produced by interrenal cells and adipose tissue (309), as well as in the brain and pituitary as described above. Therefore, future studies are needed to investigate the potential roles of the extra-gonadal sources of sex steroids. Administration with exogenous hormones is certainly an appropriate approach to evaluate physiological effects, but the method of administration, time and duration, and concentration can influence response. For example, recent studies in medaka showed that E2 may be best administered through feeding as it is convenient and effectively mimics the diurnal E2 changes in this species, whereas fish exposed to E2 in tank water exhibited blood E2 concentrations exceeding those of environmental water, suggesting that E2 bioconcentrates (310). In addition, as shown in the female ricefield eel for example (211), when testing for effects of steroids in vivo, the use of a non-aromatizable androgen such as 11-KT and an aromatase inhibitor in addition to androgen and estrogen treatments will help to clearly identify the roles of estrogens vs. androgens, which is still unclear.
Finally, because teleosts possess many paralogous sex steroid receptors, it is important that future studies investigate the role of each. Recently, transgenesis techniques such as TALEN and CRISPR/Cas9 have provided new approaches for such investigations. In medaka, for example, TALEN was used to develop an Esr1 knockout (KO) fish (311). Using these animals, the authors demonstrated the dispensable role of Esr1 for development and reproduction in medaka. Using the CRISPR/Cas9 technique, three mutant transgenic zebrafish lines have been created for each of the esr present in the zebrafish genome, as well as all possible double and triple knockouts of the three esrs (312). The authors did not observe any reproductive dysfunction for the three single esr mutant fish lines, which suggests functional redundancy among Esrs. However, double and triple knockouts showed that esr2a and esr2b were essential for reproduction in females and maintenance of the female sex phenotype as the double mutant sex-change from female to male. While these techniques show a great number of benefits, some limitations still exist. Knocking out gene expression from the one cell stage, as it is currently performed in most teleost experiments, may activate compensatory mechanisms (312). Also, as sex steroid receptors, aromatase, and other steroidogenic enzymes are widely expressed in the brain, pituitary and gonads, it is impossible to identify the precise origin of the effects observed after a KO is made. However, techniques allowing spatial and/or temporal control of the KO have recently been established in fish (313). Such techniques might be a promising tool for future investigations of the molecular, cellular and physiological roles of specific sex steroid receptors, and thus their roles in gonadotrope plasticity.
Thus, we are hopeful that more light will be shed on these topics as they will provide important information to better understand the role of sex steroids and the pathway they use to regulate gonadotrope plasticity.
Author Contributions
RF, DB, and F-AW planned the manuscript. RF and DB wrote the paper with the help of MR and KK. All authors contributed to the article and approved the submitted version.
Funding
This study was funded by the Norwegian University of Life Sciences. DB was supported by the Waple Professorship at the University of Mary Washington.
Conflict of Interest
The authors declare that the research was conducted in the absence of any commercial or financial relationships that could be construed as a potential conflict of interest.
Acknowledgments
We thank Dr. Olivier Kah for the interesting discussion and comments which have helped us to write this review, and Anthony Peltier for the illustrations used in the figures.
Abbreviations
11- HA, 11β-hydroxyandrosterone; 11-KT, 11-ketotestosterone; Acth, Adrenocorticotropin; Ar, Androgen receptor; BPG, Brain-pituitary-gonad; D1, Type 1 dopamine receptors; D2, Type 2 dopamine receptors; DA, Dopamine; DHT, 5α-dihydrotestosterone; E2, 17β-estradiol; EE2, 17α-ethinylestradiol; Esr, Estrogen receptor; Fsh, Follicle-stimulating hormone; GDX, Gonadectomy; Gh, Growth hormone; Gnrh, Gonadotropin-releasing hormone; Lh, Luteinizing hormone; Msh, Melanocyte-stimulating hormone; MT, Methyltestosterone; OVX, Ovariectomy; PI, Pars intermedia; POA, Preoptic area; Pomc, Pro-opiomelanocortin; PPD, Proximal pars distalis; Prl, Prolactin; RPD, Rostral pars distalis; Sl, Somatolactin; T, Testosterone; Th, Tyrosine hydroxylase; Tsh, Thyrotropin.
References
2. Shima A, Mitani H. Medaka as a research organism: past, present and future. Mech Dev (2004) 121(7-8):599–604. doi: 10.1016/j.mod.2004.03.011
3. Meyers JR. Zebrafish: Development of a vertebrate model organism. Curr Protoc Essential Lab Techniq (2018) 16(1):e19. doi: 10.1002/cpet.19
4. Steinke D, Hoegg S, Brinkmann H, Meyer A. Three rounds (1R/2R/3R) of genome duplications and the evolution of the glycolytic pathway in vertebrates. BMC Biol (2006) 4:16. doi: 10.1186/1741-7007-4-16
5. Lien S, Koop BF, Sandve SR, Miller JR, Kent MP, Nome T, et al. The Atlantic salmon genome provides insights into rediploidization. Nature (2016) 533(6020):200–5. doi: 10.1038/nature17164
6. Weltzien FA, Andersson E, Andersen O, Shalchian-Tabrizi K, Norberg B. The brain-pituitary-gonad axis in male teleosts, with special emphasis on flatfish (Pleuronectiformes). Comp Biochem Physiol A Mol Integr Physiol (2004) 137(3):447–77. doi: 10.1016/j.cbpb.2003.11.007
7. Zohar Y, Munoz-Cueto JA, Elizur A, Kah O. Neuroendocrinology of reproduction in teleost fish. Gen Comp Endocrinol (2010) 165(3):438–55. doi: 10.1016/j.ygcen.2009.04.017
8. Pierce JG, Parsons TF. Glycoprotein Hormones: Structure and Function. Annu Rev Biochem (1981) 50(1):465–95. doi: 10.1146/annurev.bi.50.070181.002341
9. Kanda S, Okubo K, Oka Y. Differential regulation of the luteinizing hormone genes in teleosts and tetrapods due to their distinct genomic environments–insights into gonadotropin beta subunit evolution. Gen Comp Endocrinol (2011) 173(2):253–8. doi: 10.1016/j.ygcen.2011.05.015
10. Weltzien FA, Hildahl J, Hodne K, Okubo K, Haug TM. Embryonic development of gonadotrope cells and gonadotropic hormones–lessons from model fish. Mol Cell Endocrinol (2014) 385(1-2):18–27. doi: 10.1016/j.mce.2013.10.016
11. Pogoda HM, Hammerschmidt M. Molecular genetics of pituitary development in zebrafish. Semin Cell Dev Biol (2007) 18(4):543–58. doi: 10.1016/j.semcdb.2007.04.004
12. Fontaine R, Ciani E, Haug TM, Hodne K, Ager-Wick E, Baker DM, et al. Gonadotrope plasticity at cellular, population and structural levels: A comparison between fishes and mammals. Gen Comp Endocrinol (2020) 287:113344. doi: 10.1016/j.ygcen.2019.113344
13. Jena BP. Secretion machinery at the cell plasma membrane. Curr Opin Struc Biol (2007) 17(4):437–43. doi: 10.1016/j.sbi.2007.07.002
14. Yaron Z, Levavi-Sivan B. Fish Reproduction. In: Evans DH, editor. Physiology of Fishes. 3rd ed. New York: CRC (2006). p. 343–86.
15. Somoza GM, Mechaly AS, Trudeau VL. Kisspeptin and GnRH interactions in the reproductive brain of teleosts. Gen Comp Endocrinol (2020) 298:113568. doi: 10.1016/j.ygcen.2020.113568
16. Roa J, Aguilar E, Dieguez C, Pinilla L, Tena-Sempere M. New frontiers in kisspeptin/GPR54 physiology as fundamental gatekeepers of reproductive function. Front Neuroendocrinol (2008) 29(1):48–69. doi: 10.1016/j.yfrne.2007.07.002
17. Dufour S, Sebert ME, Weltzien FA, Rousseau K, Pasqualini C. Neuroendocrine control by dopamine of teleost reproduction. J Fish Biol (2010) 76(1):129–60. doi: 10.1111/j.1095-8649.2009.02499.x
18. Tokarz J, Moller G, Hrabe de Angelis M, Adamski J. Steroids in teleost fishes: A functional point of view. Steroids (2015) 103:123–44. doi: 10.1016/j.steroids.2015.06.011
19. Devlin RH, Nagahama Y. Sex determination and sex differentiation in fish: an overview of genetic, physiological, and environmental influences. Aquaculture (2002) 208(3):191–364. doi: 10.1016/S0044-8486(02)00057-1
20. Diotel N, Page YL, Mouriec K, Tong SK, Pellegrini E, Vaillant C, et al. Aromatase in the brain of teleost fish: Expression, regulation and putative functions. Front Neuroendocrinol (2010) 31(2):172–92. doi: 10.1016/j.yfrne.2010.01.003
21. Zhang Y, Zhang S, Lu H, Zhang L, Zhang W. Genes encoding aromatases in teleosts: evolution and expression regulation. Gen Comp Endocrinol (2014) 205:151–8. doi: 10.1016/j.ygcen.2014.05.008
22. Borg B. Androgens in teleost fishes. Comp Biochem Physiol Part C: Pharmacol Toxicol Endocrinol (1994) 109(3):219–45. doi: 10.1016/0742-8413(94)00063-G
23. Rege J, Garber S, Conley AJ, Elsey RM, Turcu AF, Auchus RJ, et al. Circulating 11-oxygenated androgens across species. J Steroid Biochem (2019) 190:242–9. doi: 10.1016/j.jsbmb.2019.04.005
24. Margiotta-Casaluci L, Sumpter JP. 5α-Dihydrotestosterone is a potent androgen in the fathead minnow (Pimephales promelas). Gen Comp Endocrinol (2011) 171(3):309–18. doi: 10.1016/j.ygcen.2011.02.012
25. Margiotta-Casaluci L, Courant F, Antignac JP, Le Bizec B, Sumpter JP. Identification and quantification of 5α-dihydrotestosterone in the teleost fathead minnow (Pimephales promelas) by gas chromatography-tandem mass spectrometry. Gen Comp Endocrinol (2013) 191:202–9. doi: 10.1016/j.ygcen.2013.06.017
26. Asahina K, Suzuki K, Aida K, Hibiya T, Tamaoki B. Relationship between the structures and steroidogenic functions of the testes of the urohaze-goby (Glossogobius olivaceus). Gen Comp Endocrinol (1985) 57(2):281–92. doi: 10.1016/0016-6480(85)90273-4
27. Pasmanik M, Callard GV. Aromatase and 5 alpha-reductase in the teleost brain, spinal cord, and pituitary gland. Gen Comp Endocrinol (1985) 60(2):244–51. doi: 10.1016/0016-6480(85)90320-x
28. Schmidt KL, Pradhan DS, Shah AH, Charlier TD, Chin EH, Soma KK. Neurosteroids, immunosteroids, and the Balkanization of endocrinology. Gen Comp Endocrinol (2008) 157(3):266–74. doi: 10.1016/j.ygcen.2008.03.025
29. Diotel N, Charlier TD, Lefebvre d’Hellencourt C, Couret D, Trudeau VL, Nicolau JC, et al. Steroid transport, local synthesis, and signaling within the brain: roles in neurogenesis, neuroprotection, and sexual behaviors. Front Neurosci (2018) 12:84. doi: 10.3389/fnins.2018.00084
30. Piferrer F, Blazquez M. Aromatase distribution and regulation in fish. Fish Physiol Biochem (2005) 31(2-3):215–26. doi: 10.1007/s10695-006-0027-0
31. Diotel N, Do-Rego JL, Anglade I, Vaillant C, Pellegrini E, Vaudry H, et al. The brain of teleost fish, a source, and a target of sexual steroids. Front Neurosci (2011) 5:137. doi: 10.3389/fnins.2011.00137
32. Wierman ME. Sex steroid effects at target tissues: mechanisms of action. Adv Physiol Educ (2007) 31(1):26–33. doi: 10.1152/advan.00086.2006
33. Guerriero G. Vertebrate sex steroid receptors: evolution, ligands, and neurodistribution. Ann New Y Acad Sci (2009) 1163(1):154–68. doi: 10.1111/j.1749-6632.2009.04460.x
34. Contró V, Basile J, Proia P. Sex steroid hormone receptors, their ligands, and nuclear and non-nuclear pathways. AIMS Mol Sci (2015) 2:294–310. doi: 10.3934/molsci.2015.3.294
35. Douard V, Brunet F, Boussau B, Ahrens-Fath I, Vlaeminck-Guillem V, Haendler B, et al. The fate of the duplicated androgen receptor in fishes: a late neofunctionalization event? BMC Evolution Biol (2008) 8:336–6. doi: 10.1186/1471-2148-8-336
36. Ogino Y, Tohyama S, Kohno S, Toyota K, Yamada G, Yatsu R, et al. Functional distinctions associated with the diversity of sex steroid hormone receptors ESR and AR. J Steroid Biochem Mol Biol (2018) 184:38–46. doi: 10.1016/j.jsbmb.2018.06.002
37. Hapgood JP, Sadie H, van Biljon W, Ronacher K. Regulation of expression of mammalian gonadotrophin-releasing hormone receptor genes. J Neuroendocrinol (2005) 17(10):619–38. doi: 10.1111/j.1365-2826.2005.01353.x
38. Ciani E, Fontaine R, Maugars G, Nourizadeh-Lillabadi R, Andersson E, Bogerd J, et al. Gnrh receptor gnrhr2bbalpha is expressed exclusively in lhb-expressing cells in Atlantic salmon male parr. Gen Comp Endocrinol (2020) 285:113293. doi: 10.1016/j.ygcen.2019.113293
39. Schoenemann HM, Humphrey WD, Crowder ME, Nett TM, Reeves JJ. Pituitary luteinizing hormone-releasing hormone receptors in ovariectomized cows after challenge with ovarian steroids. Biol Reprod (1985) 32(3):574–83. doi: 10.1095/biolreprod32.3.574
40. Vizcarra JA, Wettemann RP, Braden TD, Turzillo AM, Nett TM. Effect of gonadotropin-releasing hormone (GnRH) pulse frequency on serum and pituitary concentrations of luteinizing hormone and follicle-stimulating hormone, GnRH receptors, and messenger ribonucleic acid for gonadotropin subunits in cows. Endocrinology (1997) 138(2):594–601. doi: 10.1210/endo.138.2.4938
41. Bauer-Dantoin AC, Weiss J, Jameson JL. Roles of estrogen, progesterone, and gonadotropin-releasing hormone (GnRH) in the control of pituitary GnRH receptor gene expression at the time of the preovulatory gonadotropin surges. Endocrinology (1995) 136(3):1014–9. doi: 10.1210/endo.136.3.7867555
42. Yasin M, Dalkin AC, Haisenleder DJ, Kerrigan JR, Marshall JC. Gonadotropin-releasing hormone (GnRH) pulse pattern regulates GnRH receptor gene expression: augmentation by estradiol. Endocrinology (1995) 136(4):1559–64. doi: 10.1210/endo.136.4.7895666
43. Cowley MA, Rao A, Wright PJ, Illing N, Millar RP, Clarke IJ. Evidence for differential regulation of multiple transcripts of the gonadotropin releasing hormone receptor in the ovine pituitary gland; effect of estrogen. Mol Cell Endocrinol (1998) 146(1):141–9. doi: 10.1016/S0303-7207(98)00162-2
44. Turzillo AM, Clapper JA, Moss GE, Nett TM. Regulation of ovine GnRH receptor gene expression by progesterone and oestradiol. J Reprod Fertil (1998) 113(2):251–6. doi: 10.1530/jrf.0.1130251
45. Tibolt RE, Childs GV. Cytochemical and cytophysiological studies of gonadotropin-releasing hormone (GnRH) target cells in the male rat pituitary: Differential effects of androgens and corticosterone on gnrh binding and gonadotropin release. Endocrinology (1985) 117(1):396–404. doi: 10.1210/endo-117-1-396
46. González-Martínez D, Madigou T, Mañanos E, Cerdá-Reverter JM, Zanuy S, Kah O, et al. Cloning and Expression of Gonadotropin-Releasing Hormone Receptor in the Brain and Pituitary of the European Sea Bass: An In Situ Hybridization Study1. Biol Reprod (2004) 70(5):1380–91. doi: 10.1095/biolreprod.103.022624
47. Habibi HR, De Leeuw R, Nahorniak CS, Goos HJT, Peter RE. Pituitary gonadotropin-releasing hormone (GnRH) receptor activity in goldfish and catfish: seasonal and gonadal effects. Fish Physiol Biochem (1989) 7(1):109–18. doi: 10.1007/BF00004696
48. Trudeau VL, Murthy CK, Habibi HR, Sloley BD, Peter RE. Effects of sex steroid treatments on gonadotropin-releasing hormone-stimulated gonadotropin secretion from the goldfish pituitary. Biol Reprod (1993) 48(2):300–7. doi: 10.1095/biolreprod48.2.300
49. Levavi-Sivan B, Biran J, Fireman E. Sex Steroids Are Involved in the Regulation of Gonadotropin-Releasing Hormone and Dopamine D2 Receptors in Female Tilapia Pituitary. Biol Reprod (2006) 75(4):642–50. doi: 10.1095/biolreprod.106.051540
50. Lin CJ, Wu GC, Lee MF, Lau EL, Dufour S, Chang CF. Regulation of two forms of gonadotropin-releasing hormone receptor gene expression in the protandrous black porgy fish, Acanthopagrus schlegeli. Mol Cell Endocrinol (2010) 323(2):137–46. doi: 10.1016/J.MCE.2010.04.003
51. Lin CJ, Wu GC, Dufour S, Chang CF. Activation of the brain-pituitary-gonadotropic axis in the black porgy Acanthopagrus schlegelii during gonadal differentiation and testis development and effect of estradiol treatment. Gen Comp Endocrinol (2019) 281:17–29. doi: 10.1016/j.ygcen.2019.05.008
52. von Krogh K, Bjorndal GT, Nourizadeh-Lillabadi R, Hodne K, Ropstad E, Haug TM, et al. Sex steroids differentially regulate fshb, lhb and gnrhr expression in Atlantic cod (Gadus morhua). Reproduction (2017) 154(5):581–94. doi: 10.1530/REP-17-0208
53. Ali SF, Peck EJ Jr. Modulation of anterior pituitary dopamine receptors by estradiol 17-beta: dose-response relationship. J Neurosci Res (1985) 13(4):497–507. doi: 10.1002/jnr.490130404
54. Di Paolo T, Falardeau P. Modulation of brain and pituitary dopamine receptors by estrogens and prolactin. Prog Neuropsychopharmacol Biol Psychiatry (1985) 9(5-6):473–80. doi: 10.1016/0278-5846(85)90004-1
55. Pasqualini C, Bojda F, Kerdelhue B. Direct effect of estradiol on the number of dopamine receptors in the anterior pituitary of ovariectomized rats. Endocrinology (1986) 119(6):2484–9. doi: 10.1210/endo-119-6-2484
56. Yamamoto K, Fontaine R, Pasqualini C, Vernier P. Classification of dopamine receptor genes in vertebrates: nine subtypes in osteichthyes. Brain Behav Evol (2015) 86(3-4):164–75. doi: 10.1159/000441550
57. Vacher C, Ferrière F, Marmignon MH, Pellegrini E, Saligaut C. Dopamine D2 receptors and secretion of FSH and LH: role of sexual steroids on the pituitary of the female rainbow trout. Gen Comp Endocrinol (2002) 127(2):198–206. doi: 10.1016/S0016-6480(02)00046-1
58. Fontaine R, Affaticati P, Yamamoto K, Jolly C, Bureau C, Baloche S, et al. Dopamine inhibits reproduction in female zebrafish (Danio rerio) via three pituitary D2 receptor subtypes. Endocrinology (2013) 154(2):807–18. doi: 10.1210/en.2012-1759
59. Vacher C, Mananos EL, Breton B, Marmignon MH, Saligaut C. Modulation of pituitary dopamine D1 or D2 receptors and secretion of follicle stimulating hormone and luteinizing hormone during the annual reproductive cycle of female rainbow trout. J Neuroendocrinol (2000) 12(12):1219–26. doi: 10.1046/j.1365-2826.2000.00585.x
60. Pasqualini C, Weltzien FA, Vidal B, Baloche S, Rouget C, Gilles N, et al. Two distinct dopamine D2 receptor genes in the European eel: molecular characterization, tissue-specific transcription, and regulation by sex steroids. Endocrinology (2009) 150(3):1377–92. doi: 10.1210/en.2008-0578
61. Cavaco JE, van Baal J, van Dijk W, Hassing GA, Goos HJ, Schulz RW. Steroid hormones stimulate gonadotrophs in juvenile male African catfish (Clarias gariepinus). Biol Reprod (2001) 64(5):1358–65. doi: 10.1095/biolreprod64.5.1358
62. De Leeuw R, Wurth YA, Zandbergen MA, Peute J, Goos HJT. The effects of aromatizable androgens, non-aromatizable androgens, and estrogens on gonadotropin release in castrated African catfish, Clarias gariepinus (Burchell). Cell Tissue Res (1986) 243(3):587–94. doi: 10.1007/BF00218066
63. Rebers FEM, Tensen CP, Schulz RW, Goos HJT, Bogerd J. Modulation of glycoprotein hormone α- and gonadotropin IIβ-subunit mRNA levels in the pituitary gland of mature male African catfish, Clarias gariepinus. Fish Physiol Biochem (1997) 17(1):99–108. doi: 10.1023/A:1007725321061
64. Banerjee A, Khan IA. Molecular cloning of FSH and LH β subunits and their regulation by estrogen in Atlantic croaker. Gen Comp Endocrinol (2008) 155(3):827–37. doi: 10.1016/j.ygcen.2007.09.016
65. Khan IA, Hawkins MB, Thomas P. Gonadal stage-dpendent effects of gonadal steroids on gonadotropin II secretion in the atlantic croaker (Micropogonias undulatus). Biol Reprod (1999) 61(3):834–41. doi: 10.1095/biolreprod61.3.834
66. Crim L W, Peter RE. The influence of testosterone implantation in the brain and pituitary on pituitary gonadotropin levels in Atlantic Salmon parr. Ann Biol Anim Bioch Biophys (1978) 18(3):689–94. doi: 10.1051/rnd:19780405
67. Antonopoulou E, Swanson P, Mayer I, Borg B. Feedback control of gonadotropins in Atlantic salmon, Salmo salar, male parr.II. Aromatase inhibitor and androgen effects. Gen Comp Endocrinol (1999) 114(1):142–50. doi: 10.1006/gcen.1998.7248
68. Borg B, Antonopoulou E, Mayer I, Andersson E, Berglund I, Swanson P. Effects of gonadectomy and androgen treatments on pituitary and plasma levels of gonadotropins in mature male Atlantic salmon, Salmo salar, parr–positive feedback control of both gonadotropins. Biol Reprod (1998) 58(3):814–20. doi: 10.1095/biolreprod58.3.814
69. Yen FP, Lee YH, He CL, Huang JD, Sun LT, Dufour S, et al. Estradiol-17β triggers luteinizing hormone release in the protandrous black porgy (Acanthopagrus schlegeli Bleeker) through multiple interactions with gonadotropin-releasing hormone control. Biol Reprod (2002) 66(1):251–7. doi: 10.1095/biolreprod66.1.251
70. Du JL, Lee CY, Tacon P, Lee YH, Yen FP, Tanaka H, et al. Estradiol-17β stimulates gonadotropin II expression and release in the protandrous male black porgy Acanthopagrus schlegeli Bleeker: a possible role in sex change. Gen Comp Endocrinol (2001) 121(2):135–45. doi: 10.1006/gcen.2000.7583
71. Lee YH, Du JL, Yueh WS, Lee FY, Tanaka H, Chang CF. 17β-Estradiol, but not testosterone stimulates gonadotropin II concentrations in the protandrous black porgy, Acanthopagrus schlegeli Bleeker. Fish Physiol Biochem (1999) 21(4):345–51. doi: 10.1023/A:1007830019846
72. Lee YH, Lee FY, Yueh WS, Tacon P, Du JL, Chang CN, et al. Profiles of gonadal development, sex steroids, aromatase activity, and gonadotropin II in the controlled sex change of protandrous black porgy, Acanthopagrus schlegeli Bleeker. Gen Comp Endocrinol (2000) 119(1):111–20. doi: 10.1006/gcen.2000.7499
73. Lee YH, Du JL, Yen FP, Lee CY, Dufour S, Huang JD, et al. Regulation of plasma gonadotropin II secretion by sex steroids, aromatase inhibitors, and antiestrogens in the protandrous black porgy, Acanthopagrus schlegeli Bleeker. Comp Biochem Physiol B Biochem Mol Biol (2001) 129(2):399–406. doi: 10.1016/S1096-4959(01)00337-2
74. Dickey JT, Swanson P. Effects of sex steroids on gonadotropin (FSH and LH) regulation in coho salmon (Oncorhynchus kisutch). J Mol Endocrinol (1998) 21(3):291–306. doi: 10.1677/jme.0.0210291
75. Dufour S, Delerue-Le Belle N, Fontaine YA. Effects of steroid hormones on pituitary immunoreactive gonadotropin in European freshwater eel, Anguilla anguilla. Gen Comp Endocrinol (1983) 52(2):190–7. doi: 10.1016/0016-6480(83)90112-0
76. Querat B, Hardy A, Fontaine YA. Regulation of the type-II gonadotrophin α and β subunit mRNAs by oestradiol and testosterone in the European eel. J Mol Endocrinol (1991) 7(1):81–6. doi: 10.1677/jme.0.0070081
77. Aroua S, Weltzien FA, Belle NL, Dufour S. Development of real-time RT-PCR assays for eel gonadotropins and their application to the comparison of in vivo and in vitro effects of sex steroids. Gen Comp Endocrinol (2007) 153(1):333–43. doi: 10.1016/j.ygcen.2007.02.027
78. Huggard D, Khakoo Z, Kassam G, Seyed Mahmoud S, Habibi HR. Effect of testosterone on maturational gonadotropin subunit messenger ribonucleic acid levels in the goldfish Pituitary1. Biol Reprod (1996) 54(6):1184–91. doi: 10.1095/biolreprod54.6.1184
79. Kobayashi MA, Sohn YOCH, Yoshiura YA, Aida KA. Effects of sex steroids on the mRNA levels of gonadotropin subunits in juvenile and ovariectomized goldfish Carassius auratus. Fisheries Sci (2000) 66(2):223–31. doi: 10.1046/j.1444-2906.2000.00038.x
80. Sohn YC, Suetake H, Yoshiura Y, Kobayashi M, Aida K. Structural and expression analyses of gonadotropin Ibeta subunit genes in goldfish (Carassius auratus). Gene (1998) 222(2):257–67. doi: 10.1016/s0378-1119(98)00505-8
81. Billard R, Peter RE. Gonadotropin release after implantation of anti-estrogens in the pituitary and hypothalamus of goldfish Carassius auratus. Gen Comp Endocrinol (1977) 32(2):213–20. doi: 10.1016/0016-6480(77)90154-x
82. Kobayashi M, Stacey NE. Effect of ovariectomy and steroid hormone implantation on serum gonadotropin levels in female goldfish. Zoological Sci (1990) 7(4):715–21.
83. Trudeau VL, Peter RE, Sloley BD. Testosterone and estradiol potentiate the serum gonadotropin response to gonadotropin-releasing hormone in goldfish. Biol Reprod (1991) 44(6):951–60. doi: 10.1095/biolreprod44.6.951
84. Huggard-Nelson DL, Nathwani PS, Kermouni A, Habibi HR. Molecular characterization of LH-β and FSH-β subunits and their regulation by estrogen in the goldfish pituitary. Mol Cell Endocrinol (2002) 188(1):171–93. doi: 10.1016/S0303-7207(01)00716-X
85. Klenke U, Zohar Y. Gonadal regulation of gonadotropin subunit expression and pituitary Lh protein content in female hybrid striped bass. Fish Physiol Biochem (2003) 28(1):25–7. doi: 10.1023/B:FISH.0000030465.56876.97
86. Acharjee A, Chaube R, Joy KP. Estrogen regulation of gonadotropin subunit (GPα, FSHβ, LHβ) mRNA expression during sexually quiescent and breeding phases in the female catfish heteropneustes fossilis. J Trans Neurosci (2016) 1:2.
87. Senthilkumaran B, Joy KP. Effects of administration of some monoamine-synthesis blockers and precursors on ovariectomy-induced rise in plasma Gonadotropin II in the CatfishHeteropneustes fossilis. Gen Comp Endocrinol (1996) 101(2):220–6. doi: 10.1006/gcen.1996.0024
88. Lin HR, Zhang ML, Zhang SM, Van der Kraak G, Peter RE. Effects of sex steroids, [d-Ala6, Pro9-N-ethylamide]-LHRH (LHRH-A) and domperidone (DOM) on gonadotropin secretion in female silver eel, Anguilla japonica Temminck and Schlegel. In: LHER Hirano, editor. The Second Asian Fisheries Forum. Asian Fisheries Society (1990). p. 591.
89. Amano M, Ikuta K, Kitamura S, Aida K. The maturation of the salmon GnRH system and its regulation by gonadal steroids in masu salmon. Fish Physiol Biochem (1997) 17(1):63–70. doi: 10.1023/A:1007797631403
90. Zhang X, Hecker M, Park JW, Tompsett AR, Newsted J, Nakayama K, et al. Real-time PCR array to study effects of chemicals on the Hypothalamic-Pituitary-Gonadal axis of the Japanese medaka. Aquat Toxicol (2008) 88(3):173–82. doi: 10.1016/j.aquatox.2008.04.009
91. Fontaine R, Ager-Wick E, Hodne K, Weltzien FA. Plasticity of Lh cells caused by cell proliferation and recruitment of existing cells. J Endocrinol (2019) 240(2):361–77. doi: 10.1530/JOE-18-0412
92. Guo Y, Wang Q, Li G, He M, Tang H, Zhang H, et al. Molecular mechanism of feedback regulation of 17β-estradiol on two kiss genes in the protogynous orange-spotted grouper (Epinephelus coioides). Mol Rep Dev (2017) 84(6):495–507. doi: 10.1002/mrd.22800
93. Saligaut C, Garnier DH, Bennani S, Salbert G, Bailhache T, Jego P. Effects of estradiol on brain aminergic turnover of the female rainbow trout (Oncorhynchus mykiss) at the beginning of vitellogenesis. Gen Comp Endocrinol (1992) 88(2):209–16. doi: 10.1016/0016-6480(92)90252-F
94. Billard R, Reinaud P. Testicular feed back on the hypothalamo-pituitary axis in rainbow trout (Salmo gairdneri R.). Ann Biol Anim Bioch Biophys (1978) 18:813–18. doi: 10.1051/rnd:19780509
95. Crim LW, Evans DM. Influence of testosterone and/or luteinizing hormone releasing hormone analogue on precocious sexual development in the juvenile rainbow trout. Biol Reprod (1983) 29(1):137–42. doi: 10.1095/biolreprod29.1.137
96. Trinh KYY, Wang NC, Hew CL, Crim LW. Molecular cloning and sequencing of salmon gonadotropin β subunit. Eur J Biochem (1986) 159(3):619–24. doi: 10.1111/j.1432-1033.1986.tb09930.x
97. Bommelaer MC, Billard R, Breton B. Changes in plasma gonadotropin after ovariectomy and estradiol supplementation at different stages at the end of the reproductive cycle in the rainbow trout (Salmo gairdneri R.). Reprod Nutr Develop (1981) 21(6A):989–97. doi: 10.1051/rnd:19810711
98. Crim LW, Peter RE, Billard R. Onset of gonadotropic hormone accumulation in the immature trout pituitary gland in response to estrogen or aromatizable androgen steroid hormones. Gen Comp Endocrinol (1981) 44(3):374–81. doi: 10.1016/0016-6480(81)90015-0
99. Fahraeus-van Ree GE, van Vlaardingen M, Gielen JT. Effect of 17 alpha-methyltestosterone, estradiol-17 beta and synthetic LHRH on production of gonadotropic hormone in pituitaries of rainbow trout (organ culture). Cell Tissue Res (1983) 232(1):157–76. doi: 10.1007/BF00222381
100. Goos HJT, De Leeuw R, Cook H, van Oordt PGWJ. Gonadotropic hormone-releasing hormone (GnRH) bioactivity in the brain of the immature rainbow trout, Salmo gairdneri: the effect of testosterone. Gen Comp Endocrinol (1986) 64(1):80–4. doi: 10.1016/0016-6480(86)90031-6
101. Linard B, Bennani S, Saligaut C. Involvement of estradiol in a catecholamine inhibitory tone of gonadotropin release in the rainbow trout (Oncorhynchus mykiss). Gen Comp Endocrinol (1995) 99(2):192–6. doi: 10.1006/gcen.1995.1101
102. Breton B, Sambroni E. Steroid activation of the brain–pituitary complex gonadotropic function in the triploid rainbow trout Oncorhynchus mykiss. Gen Comp Endocrinol (1996) 101(2):155–64. doi: 10.1006/gcen.1996.0017
103. Breton B, Sambroni É, Govoroun M, Weil C. Effects of steroids on GTH I and GTH II secretion and pituitary concentration in the immature rainbow trout Oncorhynchus mykiss. Comptes Rendus Acad Des Sci Serie III (1997) 320(10):783–9. doi: 10.1016/S0764-4469(97)85013-5
104. Saligaut C, Linard B, Mañanos EL, Kah O, Breton B, Govoroun M. Release of pituitary gonadotrophins GtH I and GtH II in the rainbow trout (Oncorhynchus mykiss): modulation by estradiol and catecholamines. Gen Comp Endocrinol (1998) 109(3):302–9. doi: 10.1006/gcen.1997.7033
105. Chyb J, Mikolajczyk T, Breton B. Post-ovulatory secretion of pituitary gonadotropins GtH I and GtH II in the rainbow trout (Oncorhynchus mykiss): regulation by steroids and possible role of non-steroidal gonadal factors. J Endocrinol (1999) 163(1):87–97. doi: 10.1677/joe.0.1630087
106. Vetillard A, Atteke C, Saligaut C, Jego P, Bailhache T. Differential regulation of tyrosine hydroxylase and estradiol receptor expression in the rainbow trout brain. Mol Cell Endocrinol (2003) 199(1-2):37–47. doi: 10.1016/s0303-7207(02)00305-2
107. Yamaguchi S, Gen K, Okuzawa K, Matsuyama M, Kagawa H. Influence of estradiol-17β, testosterone, and 11-ketotestosterone on testicular development, serum steroid hormone, and gonadotropin secretion in male red sea bream Pagrus major. Fisheries Sci (2006) 72(4):835–45. doi: 10.1111/j.1444-2906.2006.01225.x
108. Guzmán J, Luckenbach A, Goetz F, Fairgrieve W, Middleton M, Swanson P. Reproductive dysfunction in cultured sablefish (Anoplopoma fimbria). Bull Fish Res Agen (2015) 40:111–9.
109. Guzmán JM, Luckenbach JA, da Silva DA, Ylitalo GM, Swanson P. Development of approaches to induce puberty in cultured female sablefish (Anoplopoma fimbria). Gen Comp Endocrinol (2015) 221:101–13. doi: 10.1016/j.ygcen.2015.02.024
110. Mateos J, Mañanos E, Carrillo M, Zanuy S. Regulation of follicle-stimulating hormone (FSH) and luteinizing hormone (LH) gene expression by gonadotropin-releasing hormone (GnRH) and sexual steroids in the Mediterranean Sea bass. Comp Biochem Physiol B Biochem Mol Biol (2002) 132(1):75–86. doi: 10.1016/S1096-4959(01)00535-8
111. Alvarado MV, Servili A, Moles G, Gueguen MM, Carrillo M, Kah O, et al. Actions of sex steroids on kisspeptin expression and other reproduction-related genes in the brain of the teleost fish European sea bass. J Exp Biol (2016) 219(21):3353–65. doi: 10.1242/jeb.137364
112. Holland MCH, Hassin S, Zohar Y. Effects of long-term testosterone, gonadotropin-releasing hormone agonist, and pimozide treatments on gonadotropin II levels and ovarian development in juvenile female striped bass (Morone saxatilis). Biol Reprod (1998) 59(5):1153–62. doi: 10.1095/biolreprod59.5.1153
113. Mayer I, Borg B, Schulz R. Conversion of 11-ketoandrostenedione to 11-ketotestosterone by blood cells of six fish species. Gen Comp Endocrinol (1990) 77(1):70–4. doi: 10.1016/0016-6480(90)90207-3
114. Kah O, Chambolle P, Olivereau M, Dubourg P, Surleve-Bazeille JE. Pituitary ultrastructure in Gambusia sp. (teleostean fish) in situ and after long-term graft. 1. Rostral pars distalis. Gen Comp Endocrinol (1979) 38(2):253–63. doi: 10.1016/0016-6480(79)90214-4
115. Schulz RW, Zandbergen MA, Peute J, Bogerd J, van Dijk W, Goos HJ. Pituitary gonadotrophs are strongly activated at the beginning of spermatogenesis in African catfish, Clarias gariepinus. Biol Reprod (1997) 57(1):139–47. doi: 10.1095/biolreprod57.1.139
116. Fontaine R, Ager-Wick E, Hodne K, Weltzien FA. Plasticity in medaka gonadotropes via cell proliferation and phenotypic conversion. J Endocrinol (2020) 245(1):21–37. doi: 10.1530/JOE-19-0405
117. Golan M, Levavi-Sivan B. Social dominance in tilapia is associated with gonadotroph hyperplasia. Gen Comp Endocrinol (2013) 192:126–35. doi: 10.1016/j.ygcen.2013.04.032
118. Golan M, Biran J, Levavi-Sivan B. A novel model for development, organization, and function of gonadotropes in fish pituitary. Front Endocrinol (2014) 5:182. doi: 10.3389/fendo.2014.00182
119. Kelberman D, Rizzoti K, Lovell-Badge R, Robinson IC, Dattani MT. Genetic regulation of pituitary gland development in human and mouse. Endocr Rev (2009) 30(7):790–829. doi: 10.1210/er.2009-0008
120. Drouin J, Bilodeau S, Roussel-Gervais A. Stem cells, differentiation and cell cycle control in pituitary. In: Arzt E, Bronstein M, Guitelman M, editors. Pituitary Today II: New Molecular, Physiological and Clinical Aspects, vol. 38. Basel, Karger: Front Horm Res (2010). p. 15–24. doi: 10.1159/000318490
121. Kim GL, Wang X, Chalmers JA, Thompson DR, Dhillon SS, Koletar MM, et al. Generation of immortal cell lines from the adult pituitary: role of camp on differentiation of SOX2-expressing progenitor cells to mature gonadotropes. PloS One (2011) 6(11):e27799. doi: 10.1371/journal.pone.0027799
122. Vankelecom H, Chen J. Pituitary stem cells: where do we stand? Mol Cell Endocrinol (2014) 385(1):2–17. doi: 10.1016/j.mce.2013.08.018
123. Fauquier DA, Mazet JAK, Gulland FMD, Spraker TR, Christopher MM. Distribution of tissue enzymes in three species of Pinnipeds. J Zoo Wild Med (2008) 39(1):1–5. doi: 10.1638/2006-0012.1
124. Fauquier T, Rizzoti K, Dattani M, Lovell-Badge R, Robinson ICAF. SOX2-expressing progenitor cells generate all of the major cell types in the adult mouse pituitary gland. Proc Natl Acad Sci U S A (2008) 105(8):2907. doi: 10.1073/pnas.0707886105
125. Gremeaux L, Fu Q, Chen J, Vankelecom H. Activated phenotype of the pituitary stem/progenitor cell compartment during the early-postnatal maturation phase of the gland. Stem Cells Dev (2012) 21: (5):801–13. doi: 10.1089/scd.2011.0496
126. Chen M, Kato T, Higuchi M, Yoshida S, Yako H, Kanno N, et al. Coxsackievirus and adenovirus receptor-positive cells compose the putative stem/progenitor cell niches in the marginal cell layer and parenchyma of the rat anterior pituitary. Cell Tissue Res (2013) 354(3):823–36. doi: 10.1007/s00441-013-1713-8
127. Jeon SY, Hwang KA, Kim CW, Jeung EB, Choi KC. Altered expression of epithelial mesenchymal transition and pluripotent associated markers by sex steroid hormones in human embryonic stem cells. Mol Med Rep (2017) 16(1):828–36. doi: 10.3892/mmr.2017.6672
128. Rizzoti K. Adult pituitary progenitors/stem cells: from in vitro characterization to in vivo function. Eur J Neurosci (2010) 32(12):2053–62. doi: 10.1111/j.1460-9568.2010.07524.x
129. Okuda Y, Yoda H, Uchikawa M, Furutani-Seiki M, Takeda H, Kondoh H, et al. Comparative genomic and expression analysis of group B1 sox genes in zebrafish indicates their diversification during vertebrate evolution. Dev Dyn (2006) 235(3):811–25. doi: 10.1002/dvdy.20678
130. Alunni A, Hermel JM, Heuzé A, Bourrat F, Jamen F, Joly JS. Evidence for neural stem cells in the medaka optic tectum proliferation zones. Dev Neurobiol (2010) 70(10):693–713. doi: 10.1002/dneu.20799
131. Germanà A, Montalbano G, Guerrera MC, Amato V, Laurà R, Magnoli D, et al. Developmental changes in the expression of sox2 in the zebrafish brain. Microsc Res Tech (2011) 74(4):347–54. doi: 10.1002/jemt.20915
132. Diotel N, Vaillant C, Gabbero C, Mironov S, Fostier A, Gueguen MM, et al. Effects of estradiol in adult neurogenesis and brain repair in zebrafish. Hormones Behav (2013) 63(2):193–207. doi: 10.1016/j.yhbeh.2012.04.003
133. Lust K, Wittbrodt J. Activating the regenerative potential of Müller glia cells in a regeneration-deficient retina. eLife (2018) 7:e32319. doi: 10.7554/eLife.32319
134. Morand I, Fonlupt P, Guerrier A, Trouillas J, Calle A, Remy C, et al. Cell-to-cell communication in the anterior pituitary: evidence for gap junction-mediated exchanges between endocrine cells and folliculostellate cells. Endocrinology (1996) 137(8):3356–67. doi: 10.1210/endo.137.8.8754762
135. Fauquier T, Guerineau NC, McKinney RA, Bauer K, Mollard P. Folliculostellate cell network: a route for long-distance communication in the anterior pituitary. Proc Natl Acad Sci U S A (2001) 98(15):8891–6. doi: 10.1073/pnas.151339598
136. Horvath E, Kovacs K. Folliculo-stellate cells of the human pituitary: a type of adult stem cell? Ultrastruct Pathol (2002) 26(4):219–28. doi: 10.1080/01913120290104476
137. Inoue K, Mogi C, Ogawa S, Tomida M, Miyai S. Are folliculo-stellate cells in the anterior pituitary gland supportive cells or organ-specific stem cells? Arch Physiol Biochem (2002) 110(1-2):50–3. doi: 10.1076/apab.110.1.50.911
138. Soji T, Herbert DC. Intercellular communication within the rat anterior pituitary gland. II. Castration effects and changes after injection of luteinizing hormone-releasing hormone (LH-RH) or testosterone. Anat Rec (1990) 226(3):342–6. doi: 10.1002/ar.1092260311
139. Sakuma E, Herbert DC, Soji T. The effects of sex steroids on the formation of gap junctions between folliculo-stellate cells; a study in castrated male rats and ovariectomized female rats. Arch Histol Cytol (2003) 66(3):229–38. doi: 10.1679/aohc.66.229
140. Kurono C. Intercellular communication within the rat anterior pituitary gland: VI. Development of gap junctions between folliculo-stellate cells under the influence of ovariectomy and sex steroids in the female rat. Anat Rec (1996) 244(3):366–73. doi: 10.1002/(SICI)1097-0185(199603)244:3<366::AID-AR8>3.0.CO;2-W
141. Rawdon B. Ultrastructure of the Non-granulated Hypophysial Cells in the Teleost Pseudocrenilabrus philander (Hemihaplochromis philander), with particular reference to cytological changes in culture. Acta Zoologica (1978) 59:25–33. doi: 10.1111/j.1463-6395.1978.tb00108.x
142. Batten T, Ball JN, Benjamin M. Ultrastructure of the adenohypophysis in the teleost Poecilia latipinna. Cell Tissue Res (1975) 161(2):239–61. doi: 10.1007/BF00220372
143. Yamamoto T, Kataoka K, Ochi J, Honma Y. The fine structure of the follicle-forming cells in the adenohypophysis of the ironfish, a natural hybrid between the Funa and the goldfish (Teleostei, Cyprinidae). Arch Histol Jpn (1982) 45(4):355–64. doi: 10.1679/aohc.45.355
144. Benjamin M. The origin of pituitary cysts in the rostral pars distalis of the nine-spined stickleback, Pungitius pungitius L. Cell Tissue Res (1981) 214(2):417–30. doi: 10.1007/BF00249222
145. Olivereau M, Dubourg P, Chambolle P, Olivereau J. Effects of estradiol and mammalian LHRH on the ultrastructure of the pars distalis of the eel. Cell Tissue Res (1986) 246(2):425–37. doi: 10.1007/BF00215905
146. Garcia-Ayala A, Garcia-Hernandez MP, Quesada JA, Agulleiro B. Immunocytochemical and ultrastructural characterization of prolactin, growth hormone, and somatolactin cells from the Mediterranean yellowtail (Seriola dumerilii, Risso 1810). Anat Rec (1997) 247(3):395–404. doi: 10.1002/(SICI)1097-0185(199703)247:3<395::AID-AR11>3.0.CO;2-K
147. Abraham M. The ultrastructure of the cell types and of the neurosecretory innervation in the pituitary of Mugil cephalus L. from fresh water, the sea, and a hypersaline lagoon. I. The rostral pars distalis. Gen Comp Endocrinol (1971) 17(2):324–50. doi: 10.1016/0016-6480(71)90144-4
148. Abraham M, Dinari-Lavie V, Lotan R. The pituitary of Aphanius dispar (Ruppell) from hypersaline marshes and freshwater. II. Ultrastructure of the rostral pars distalis. Cell Tissue Res (1977) 179(3):317–30. doi: 10.1007/BF00221103
149. Ferrandino I, Grimaldi MC. Ultrastructural study of the pituicytes in the pituitary gland of the teleost Diplodus sargus. Brain Res Bull (2008) 75(1):133–7. doi: 10.1016/j.brainresbull.2007.08.006
150. Golan M, Hollander-Cohen L, Levavi-Sivan B. Stellate cell networks in the teleost pituitary. Sci Rep (2016) 6:24426. doi: 10.1038/srep24426
151. Jeng SR, Yueh WS, Pen YT, Gueguen MM, Pasquier J, Dufour S, et al. Expression of aromatase in radial glial cells in the brain of the japanese eel provides insight into the evolution of the cyp191a gene in actinopterygians. PloS One (2012) 7(9):e44750. doi: 10.1371/journal.pone.0044750
152. Pellegrini E, Mouriec K, Anglade I, Menuet A, Le Page Y, Gueguen MM, et al. Identification of aromatase-positive radial glial cells as progenitor cells in the ventricular layer of the forebrain in zebrafish. J Comp Neurol (2007) 501(1):150–67. doi: 10.1002/cne.21222
153. Horvath E, Lloyd RV, Kovacs K. Propylthiouracyl-induced hypothyroidism results in reversible transdifferentiation of somatotrophs into thyroidectomy cells. A morphologic study of the rat pituitary including immunoelectron microscopy. Lab Invest (1990) 63(4):511–20.
154. Cova L, Ratti A, Volta M, Fogh I, Cardin V, Corbo M, et al. Stem cell therapy for neurodegenerative diseases: the issue of transdifferentiation. Stem Cells Dev (2004) 13(1):121–31. doi: 10.1089/154732804773099326
155. Vankelecom H. Non-hormonal cell types in the pituitary candidating for stem cell. Semin Cell Dev Biol (2007) 18(4):559–70. doi: 10.1016/j.semcdb.2007.04.006
156. Willems C, Vankelecom H. Pituitary cell differentiation from stem cells and other cells: toward restorative therapy for hypopituitarism? Regener Med (2014) 9(4):513–34. doi: 10.2217/rme.14.19
157. Borrelli E, Heyman RA, Arias C, Sawchenko PE, Evans RM. Transgenic mice with inducible dwarfism. Nature (1989) 339(6225):538–41. doi: 10.1038/339538a0
158. Fu Q, Vankelecom H. Regenerative capacity of the adult pituitary: multiple mechanisms of lactotrope restoration after transgenic ablation. Stem Cells Dev (2012) 21(18):3245–57. doi: 10.1089/scd.2012.0290
159. Frawley LS, Boockfor FR. Mammosomatotropes: presence and functions in normal and neoplastic pituitary tissue. Endocr Rev (1991) 12(4):337–55. doi: 10.1210/edrv-12-4-337
160. Porter TE, Wiles CD, Frawley LS. Evidence for bidirectional interconversion of mammotropes and somatotropes: rapid reversion of acidophilic cell types to pregestational proportions after weaning. Endocrinology (1991) 129(3):1215–20. doi: 10.1210/endo-129-3-1215
161. Stefaneanu L, Kovacs K, Lloyd RV, Scheithauer BW, Young WF, Sano T, et al. Pituitary lactotrophs and somatotrophs in pregnancy: a correlative in situ hybridization and immunocytochemical study. Virchows Archiv B (1992) 62(1):291. doi: 10.1007/BF02899695
162. Inoue K, Sakai T. Conversion of growth hormone-secreting cells into prolactin-secreting cells and its promotion by insulin and insulin-like growth factor-1 in vitro. Exp Cell Res (1991) 195(1):53–8. doi: 10.1016/0014-4827(91)90499-K
163. Kakeya T, Takeuchi S, Takahashi S. Epidermal growth factor, insulin, and estrogen stimulate development of prolactin-secreting cells in cultures of GH3 cells. Cell Tissue Res (2000) 299(2):237–43. doi: 10.1007/s004419900147
164. Vidal S, Horvath E, Kovacs K, Lloyd RV, Smyth HS. Reversible transdifferentiation: interconversion of somatotrophs and lactotrophs in pituitary hyperplasia. Mod Pathol (2001) 14(1):20–8. doi: 10.1038/modpathol.3880252
165. Childs GV, Yang HY, Tobin RB, Wilber JF, Kubek M. Effects of thyroidectomy, propylthiouracil, and thyroxine on pituitary content and immunocytochemical staining of thyrotropin (TSH) and thyrotropin releasing hormone (TRH). J Histochem Cytochem (1981) 29(3):357–63. doi: 10.1177/29.3.6787114
166. Vidal S, Horvath E, Kovacs K, Cohen SM, Lloyd RV, Scheithauer BW. Transdifferentiation of somatotrophs to thyrotrophs in the pituitary of patients with protracted primary hypothyroidism. Virchows Archiv (2000) 436(1):43–51. doi: 10.1007/PL00008197
167. Radian S, Coculescu M, Morris JF. Somatotroph to thyrotroph cell transdifferentiation during experimental hypothyroidism - a light and electron-microscopy study. J Cell Mol Med (2003) 7(3):297–306. doi: 10.1111/j.1582-4934.2003.tb00230.x
168. Childs GV. Development of gonadotropes may involve cyclic transdifferentiation of growth hormone cells. Arch Physiol Biochem (2002) 110(1-2):42–9. doi: 10.1076/apab.110.5.5.42.19864
169. Buijtels JJCWM, de Gier J, Kooistra HS, Kroeze EJBV, Okkens AC. Alterations of the pituitary-ovarian axis in dogs with a functional granulosa cell tumor. Theriogenology (2010) 73(1):11–9. doi: 10.1016/j.theriogenology.2009.06.031
170. Seilicovich A. Cell life and death in the anterior pituitary gland: role of oestrogens. J Neuroendocrinol (2010) 22(7):758–64. doi: 10.1111/j.1365-2826.2010.02010.x
171. Vasconsuelo A, Pronsato L, Ronda AC, Boland R, Milanesi L. Role of 17β-estradiol and testosterone in apoptosis. Steroids (2011) 76(12):1223–31. doi: 10.1016/j.steroids.2011.08.001
172. Yin P, Arita J. Proestrous surge of gonadotropin-releasing hormone secretion inhibits apoptosis of anterior pituitary cells in cycling female rats. Neuroendocrinology (2002) 76(5):272–82. doi: 10.1159/000066626
173. Zaldivar V, Magri ML, Zárate S, Jaita G, Eijo G, Radl D, et al. Estradiol increases the Bax/Bcl-2 ratio and induces apoptosis in the anterior pituitary gland. Neuroendocrinology (2009) 90(3):292–300. doi: 10.1159/000235618
174. Oishi Y, Okuda M, Takahashi H, Fujii T, Morii S. Cellular proliferation in the anterior pituitary gland of normal adult rats: influences of sex, estrous cycle, and circadian change. Anat Rec (1993) 235(1):111–20. doi: 10.1002/ar.1092350111
175. Karigo T, Aikawa M, Kondo C, Abe H, Kanda S, Oka Y. Whole brain-pituitary in vitro preparation of the transgenic medaka (Oryzias latipes) as a tool for analyzing the differential regulatory mechanisms of LH and FSH release. Endocrinology (2014) 155(2):536–47. doi: 10.1210/en.2013-1642
176. Hodne K, Fontaine R, Ager-Wick E, Weltzien FA. Gnrh1-induced responses are indirect in female medaka Fsh cells, generated through cellular networks. Endocrinology (2019) 160(12):3018–32. doi: 10.1210/en.2019-00595
177. Weiser MJ, Foradori CD, Handa RJ. Estrogen receptor beta in the brain: From form to function. Brain Res Rev (2008) 57(2):309–20. doi: 10.1016/j.brainresrev.2007.05.013
178. Coumailleau P, Pellegrini E, Adrio F, Diotel N, Cano-Nicolau J, Nasri A, et al. Aromatase, estrogen receptors and brain development in fish and amphibians. Biochim Biophys Acta (2015) 1849(2):152–62. doi: 10.1016/j.bbagrm.2014.07.002
179. Harbott LK, Burmeister SS, White RB, Vagell M, Fernald RD. Androgen receptors in a cichlid fish, Astatotilapia burtoni: structure, localization, and expression levels. J Comp Neurol (2007) 504(1):57–73. doi: 10.1002/cne.21435
180. Munchrath LA, Hofmann HA. Distribution of sex steroid hormone receptors in the brain of an African cichlid fish, Astatotilapia burtoni. J Comp Neurol (2010) 518(16):3302–26. doi: 10.1002/cne.22401
181. Hawkins MB, Thornton JW, Crews D, Skipper JK, Dotte A, Thomas P. Identification of a third distinct estrogen receptor and reclassification of estrogen receptors in teleosts. Proc Natl Acad Sci USA (2000) 97(20):10751–6. doi: 10.1073/pnas.97.20.10751
182. Hawkins MB, Godwin J, Crews D, Thomas P. The distributions of the duplicate oestrogen receptors ER-βa and ER-βb in the forebrain of the Atlantic croaker (Micropogonias undulatus): evidence for subfunctionalization after gene duplication. Proc R Soc B: Biol Sci (2005) 272(1563):633–41. doi: 10.1098/rspb.2004.3008
183. Andreassen TK, Skjoedt K, Anglade I, Kah O, Korsgaard B. Molecular cloning, characterisation, and tissue distribution of oestrogen receptor alpha in eelpout (Zoarces viviparus). Gen Comp Endocrinol (2003) 132(3):356–68. doi: 10.1016/S0016-6480(03)00101-1
184. Peñaranda DS, Mazzeo I, Gallego V, Hildahl J, Nourizadeh-Lillabadi R, Pérez L, et al. The regulation of aromatase and androgen receptor expression during gonad development in male and female European eel. Reprod Domest Anim (2014) 49(3):512–21. doi: 10.1111/rda.12321
185. Filby AL, Tyler CR. Molecular characterization of estrogen receptors 1, 2a, and 2b and their tissue and ontogenic expression profiles in Fathead Minnow (Pimephales promelas). Biol Reprod (2005) 73(4):648–62. doi: 10.1095/biolreprod.105.039701
186. Pasmanik M, Callard GV. A high abundance androgen receptor in goldfish brain: characteristics and seasonal changes. Endocrinology (1988) 123(2):1162–71. doi: 10.1210/endo-123-2-1162
187. Tchoudakova A, Pathak S, Callard GV. Molecular cloning of an estrogen receptor β subtype from the goldfish, Carassius auratus. Gen Comp Endocrinol (1999) 113(3):388–400. doi: 10.1006/gcen.1998.7217
188. Ma CH, Dong KW, Yu KL. cDNA cloning and expression of a novel estrogen receptor β-subtype in goldfish (Carassius auratus). Biochim Biophys Acta (BBA) Gene Struct Expression (2000) 1490(1):145–52. doi: 10.1016/S0167-4781(99)00235-3
189. Choi CY, Habibi HR. Molecular cloning of estrogen receptor alpha and expression pattern of estrogen receptor subtypes in male and female goldfish. Mol Cell Endocrinol (2003) 204(1-2):169–77. doi: 10.1016/s0303-7207(02)00182-x
190. Kim YS, Stumpf WE, Sar M. Topography of estrogen target cells in the forebrain of goldfish, Carassius auratus. J Comp Neurol (1978) 182(4):611–20. doi: 10.1002/cne.901820404
191. Kanda S, Karigo T, Oka Y. Steroid sensitive kiss2 neurones in the goldfish: evolutionary insights into the duplicate Kisspeptin gene-expressing neurones. J Neuroendocrinol (2012) 24(6):897–906. doi: 10.1111/j.1365-2826.2012.02296.x
192. Kawabata-Sakata Y, Nishiike Y, Fleming T, Kikuchi Y, Okubo K. Androgen-dependent sexual dimorphism in pituitary tryptophan hydroxylase expression: relevance to sex differences in pituitary hormones. Proc R Soc B: Biol Sci (2020) 287(1928):20200713. doi: 10.1098/rspb.2020.0713
193. Mitani Y, Kanda S, Akazome Y, Zempo B, Oka Y. Hypothalamic Kiss1 but not Kiss2 neurons are involved in estrogen feedback in Medaka (Oryzias latipes). Endocrinology (2010) 151(4):1751–9. doi: 10.1210/en.2009-1174
194. Hiraki T, Takeuchi A, Tsumaki T, Zempo B, Kanda S, Oka Y, et al. Female-specific target sites for both oestrogen and androgen in the teleost brain. Proc Biol Sci (2012) 279(1749):5014–23. doi: 10.1098/rspb.2012.2011
195. Zempo B, Kanda S, Okubo K, Akazome Y, Oka Y. Anatomical distribution of sex steroid hormone receptors in the brain of female medaka. J Comp Neurol (2013) 521(8):1760–80. doi: 10.1002/cne.23255
196. Forlano PM, Deitcher DL, Bass AH. Distribution of estrogen receptor alpha mRNA in the brain and inner ear of a vocal fish with comparisons to sites of aromatase expression. J Comp Neurol (2005) 483(1):91–113. doi: 10.1002/cne.20397
197. Forlano PM, Marchaterre M, Deitcher DL, Bass AH. Distribution of androgen receptor mRNA expression in vocal, auditory, and neuroendocrine circuits in a teleost fish. J Comp Neurol (2010) 518(4):493–512. doi: 10.1002/cne.22233
198. Fergus DJ, Bass AH. Localization and divergent profiles of estrogen receptors and aromatase in the vocal and auditory networks of a fish with alternative mating tactics. J Comp Neurol (2013) 521(12):2850–69. doi: 10.1002/cne.23320
199. Fine ML, Keefer DA, Russel-Mergenthal H. Autoradiographic localization of estrogen-concentrating cells in the brain and pituitary of the oyster toadfish. Brain Res (1990) 536(1):207–19. doi: 10.1016/0006-8993(90)90027-9
200. Davis RE, Morrell JI, Pfaff DW. Autoradiographic localization of sex steroid-concentrating cells in the brain of the teleost Macropodus opercularis (Osteichthyes: Belontiidae). Gen Comp Endocrinol (1977) 33(4):496–505. doi: 10.1016/0016-6480(77)90108-3
201. Strobl-Mazzulla PH, Lethimonier C, Gueguen MM, Karube M, Fernandino JI, Yoshizaki G, et al. Brain aromatase (Cyp19A2) and estrogen receptorslarvae and adult pejerrey fish Odontesthes bonariensis: Neuroanatomical and functional relations. Gen Comp Endocrinol (2008) 158(2):191–201. doi: 10.1016/j.ygcen.2008.07.006
202. Kim YS, Stumpf WE, Sar M. Topographical distribution of estrogen target cells in the forebrain of platyfish, Xiphophorus maculatus, studied by autoradiography. Brain Res (1979) 170(1):43–59. doi: 10.1016/0006-8993(79)90939-9
203. Pakdel F, Gac FL, Goff PL, Valotaire Y. Full-length sequence and in vitro expression of rainbow trout estrogen receptor cDNA. Mol Cell Endocrinol (1990) 71(3):195–204. doi: 10.1016/0303-7207(90)90025-4
204. Menuet A, Anglade I, Flouriot G, Pakdel F, Kah O. Tissue-specific expression of two structurally different estrogen receptor alpha isoforms along the female reproductive axis of an oviparous species, the rainbow trout. Biol Reprod (2001) 65(5):1548–57. doi: 10.1095/biolreprod65.5.1548
205. Nagler JJ, Cavileer T, Sullivan J, Cyr DG, Rexroad C3. The complete nuclear estrogen receptor family in the rainbow trout: discovery of the novel ERalpha2 and both ERbeta isoforms. Gene (2007) 392(1-2):164–73. doi: 10.1016/j.gene.2006.12.030
206. Linard B, Anglade I, Corio M, Navas JM, Pakdel F, Saligaut C, et al. Estrogen receptors are expressed in a subset of tyrosine hydroxylase-positive neurons of the anterior preoptic region in the rainbow trout. Neuroendocrinology (1996) 63(2):156–65. doi: 10.1159/000126952
207. Salbert G, Bonnec G, Le Goff P, Boujard D, Valotaire Y, Jego P. Localization of the estradiol receptor mRNA in the forebrain of the rainbow trout. Mol Cell Endocrinol (1991) 76(1):173–80. doi: 10.1016/0303-7207(91)90271-S
208. Anglade I, Pakdel F, Bailhache T, Petit F, Salbert G, Jego P, et al. Distribution of estrogen receptor-lmmunoreactive cells in the brain of the rainbow trout (Oncorhynchus mykiss). J Neuroendocrinol (1994) 6(5):573–83. doi: 10.1111/j.1365-2826.1994.tb00621.x
209. Pakdel F, Petit F, Anglade I, Kah O, Delaunay F, Bailhache T, et al. Overexpression of rainbow trout estrogen receptor domains in Escherichia coli: characterization and utilization in the production of antibodies for immunoblotting and immunocytochemistry. Mol Cell Endocrinol (1994) 104(1):81–93. doi: 10.1016/0303-7207(94)90054-X
210. Menuet A, Anglade I, Le Guevel R, Pellegrini E, Pakdel F, Kah O. Distribution of aromatase mRNA and protein in the brain and pituitary of female rainbow trout: comparison with estrogen receptor α. J Comp Neurol (2003) 462(2):180–93. doi: 10.1002/cne.10726
211. Zhang S, Zhang Y, Chen W, Wu Y, Ge W, Zhang L, et al. Aromatase (Cyp19a1b) in the pituitary is dynamically involved in the upregulation of lhb but not fshb in the vitellogenic female ricefield eel Monopterus albus. Endocrinology (2014) 155(11):4531–41. doi: 10.1210/en.2014-1069
212. Zheng Z, Liu M, Meng F, Zhang W, Zhang L. Differential distribution and potential regulatory roles of estrogen receptor 2a and 2b in the pituitary of ricefield eel Monopterus albus. Gen Comp Endocrinol (2020) 298:113554. doi: 10.1016/j.ygcen.2020.113554
213. Guzmán JM, Luckenbach JA, Da Silva DAM, Hayman ES, Ylitalo GM, Goetz FW, et al. Seasonal variation of pituitary gonadotropin subunit, brain-type aromatase and sex steroid receptor mRNAs, and plasma steroids during gametogenesis in wild sablefish. Comp Biochem Physiol Part A Mol Integr Physiol (2018) 219-220:48–57. doi: 10.1016/J.CBPA.2018.02.010
214. Makantasi P, Dermon CR. Estradiol treatment decreases cell proliferation in the neurogenic zones of adult female zebrafish (Danio rerio) brain. Neuroscience (2014) 277:306–20. doi: 10.1016/j.neuroscience.2014.06.071
215. Muriach B, Cerdá-Reverter JM, Gómez A, Zanuy S, Carrillo M. Molecular characterization and central distribution of the estradiol receptor alpha (ERα) in the sea bass (Dicentrarchus labrax). J Chem Neuroanat (2008) 35(1):33–48. doi: 10.1016/j.jchemneu.2007.05.010
216. Muriach B, Carrillo M, Zanuy S, Cerdá-Reverter JM. Distribution of estrogen receptor 2 mRNAs (Esr2a and Esr2b) in the brain and pituitary of the sea bass (Dicentrarchus labrax). Brain Res (2008) 1210:126–41. doi: 10.1016/j.brainres.2008.02.053
217. Escobar S, Felip A, Gueguen MM, Zanuy S, Carrillo M, Kah O, et al. Expression of kisspeptins in the brain and pituitary of the European sea bass (Dicentrarchus labrax). J Comp Neurol (2013) 521(4):933–48. doi: 10.1002/cne.23211
218. Pinto PIS, Passos AL, Martins DMPRS, Canário AVM. Characterization of estrogen receptor βb in sea bream (Sparus auratus): phylogeny, ligand-binding, and comparative analysis of expression. Gen Comp Endocrinol (2006) 145(2):197–207. doi: 10.1016/j.ygcen.2005.08.010
219. Menuet A, Pellegrini E, Anglade I, Blaise O, Laudet V, Kah O, et al. Molecular characterization of three estrogen receptor forms in zebrafish: binding characteristics, transactivation properties, and tissue distributions. Biol Reprod (2002) 66(6):1881–92. doi: 10.1095/biolreprod66.6.1881
220. Gorelick DA, Watson W, Halpern ME. Androgen receptor gene expression in the developing and adult zebrafish brain. Dev Dyn (2008) 237(10):2987–95. doi: 10.1002/dvdy.21700
221. Pellegrini E, Menuet A, Lethimonier C, Adrio F, Gueguen MM, Tascon C, et al. Relationships between aromatase and estrogen receptors in the brain of teleost fish. Gen Comp Endocrinol (2005) 14(1):60–6. doi: 10.1016/j.ygcen.2004.12.003
222. Kah O, Lethimonier C, Somoza G, Guilgur LG, Vaillant C, Lareyre JJ. GnRH and GnRH receptors in metazoa: a historical, comparative, and evolutive perspective. Gen Comp Endocrinol (2007) 153(1-3):346–64. doi: 10.1016/j.ygcen.2007.01.030
223. Okubo K, Nagahama Y. Structural and functional evolution of gonadotropin-releasing hormone in vertebrates. Acta Physiol (Oxf) (2008) 193(1):3–15. doi: 10.1111/j.1748-1716.2008.01832.x
224. Herbison AE. Estrogen positive feedback to gonadotropin-releasing hormone (GnRH) neurons in the rodent: the case for the rostral periventricular area of the third ventricle (RP3V). Brain Res Rev (2008) 57(2):277–87. doi: 10.1016/j.brainresrev.2007.05.006
225. Radovick S. Estrogenic regulation of the GnRH neuron. Front Endocrinol (2012) 3:52. doi: 10.3389/fendo.2012.00052
226. Senthilkumaran B, Joy KP. Changes in hypothalamic catecholamines, dopamine-beta-hydroxylase, and phenylethanolamine-N-methyltransferase in the catfish Heteropneustes fossilis in relation to season, raised photoperiod and temperature, ovariectomy, and estradiol-17 beta replacement. Gen Comp Endocrinol (1995) 97(1):121–34. doi: 10.1006/gcen.1995.1012
227. Mamta SK, Raghuveer K, Sudhakumari CC, Rajakumar A, Basavaraju Y, Senthilkumaran B. Cloning and expression analysis of tyrosine hydroxylase and changes in catecholamine levels in brain during ontogeny and after sex steroid analogues exposure in the catfish, Clarias batrachus. Gen Comp Endocrinol (2014) 197:18–25. doi: 10.1016/j.ygcen.2013.11.022
228. Dufour S, Fontaine YA, Kerdelhue B. Increase in brain and pituitary radioimmunoassayable gonadotropin releasing hormone (GnRH) in the European silver eel treated with sexual steroid or human chorionic gonadotropin. Neuropeptides (1985) 6(6):495–502. doi: 10.1016/0143-4179(85)90111-8
229. Montero M, Lebelle N, Kingb JA, Millarb RP, Dufour S. Differential regulation of the two forms of gonadotropin-releasing hormone (mGnRH and cGnRH-II) by sex steroids in the european female silver eel (anguilla anguilla). Neuroendocrinology (1995) 61(5):525–35. doi: 10.1159/000126876
230. Weltzien FA, Pasqualini C, Sebert ME, Vidal B, Le Belle N, Kah O, et al. Androgen-dependent stimulation of brain dopaminergic systems in the female European eel (Anguilla anguilla). Endocrinology (2006) 147(6):2964–73. doi: 10.1210/en.2005-1477
231. Trudeau VL, Sloley BD, Wong AO, Peter RE. Interactions of gonadal steroids with brain dopamine and gonadotropin-releasing hormone in the control of gonadotropin-II secretion in the goldfish. Gen Comp Endocrinol (1993) 89(1):39–50. doi: 10.1006/gcen.1993.1007
232. Amano M, Hyodo S, Urano A, Okumoto N, Kitamura S, Ikuta K, et al. Activation Of salmon gonadotropin-releasing hormone synthesis by 17α-methyltestosterone administration in yearling masu salmon, Oncorhynchus Masou. Genl Comp Endocrinol (1994) 95(3):374–80. doi: 10.1006/gcen.1994.1136
233. Lee W, Kang CW, Su CK, Okubo K, Nagahama Y. Screening estrogenic activity of environmental contaminants and water samples using a transgenic medaka embryo bioassay. Chemosphere (2012) 88(8):945–52. doi: 10.1016/j.chemosphere.2012.03.024
234. Shi Y, Zhang Y, Li S, Liu Q, Lu D, Liu M, et al. Molecular identification of the Kiss2/Kiss1ra system and its potential function during 17alpha-methyltestosterone-induced sex reversal in the orange-spotted grouper, Epinephelus coioides. Biol Reprod (2010) 83(1):63–74. doi: 10.1095/biolreprod.109.080044
235. Cui M, Li W, Liu W, Yang K, Pang Y, Haoran L. Production of recombinant orange-spotted grouper (Epinephelus coioides) luteinizing hormone in insect cells by the baculovirus expression system and its biological effect. Biol Reprod (2007) 76(1):74–84. doi: 10.1095/biolreprod.105.050484
236. Vetillard A, Bailhache T. Effects of 4-n-nonylphenol and tamoxifen on salmon gonadotropin-releasing hormone, estrogen receptor, and vitellogenin gene expression in juvenile rainbow trout. Toxicol Sci (2006) 92(2):537–44. doi: 10.1093/toxsci/kfl015
237. Klenke U, Zmora N, Stubblefield J, Zohar Y. Expression patterns of the kisspeptin system and gnrh1 correlate in their response to gonadal feedback in female striped bass. Indian J Sci Technol (2011) 4:33–4. doi: 10.17485/ijst/2011/v4is.29
238. Chen HP, Cui XF, Wang YR, Li ZY, Tian CX, Jiang DN, et al. Identification, functional characterization, and estrogen regulation on gonadotropin-releasing hormone in the spotted scat, Scatophagus argus. Fish Physiol Biochem (2020) 46:1743–57. doi: 10.1007/s10695-020-00825-5
239. Servili A, Le Page Y, Leprince J, Caraty A, Escobar S, Parhar IS, et al. Organization of two independent kisspeptin systems derived from evolutionary-ancient kiss genes in the brain of zebrafish. Endocrinology (2011) 152(4):1527–40. doi: 10.1210/en.2010-0948
240. Amano M, Urano A, Aida K. Distribution and function of gonadotropin-releasing hormone (GnRH) in the teleost brain. Zoological Sci (1997) 14(1):1–11. doi: 10.2108/zsj.14.1
241. Navas JM, Anglade I, Bailhache T, Pakdel F, Breton B, Jégo P, et al. Do gonadotrophin-releasing hormone neurons express estrogen receptors in the rainbow trout? A double immunohistochemical study. J Comp Neurol (1995) 363(3):461–74. doi: 10.1002/cne.903630309
242. Ogawa S, Parhar IS. Single-cell gene profiling reveals social status-dependent modulation of nuclear hormone receptors in GnRH neurons in a male cichlid fish. Int J Mol Sci (2020) 21:8. doi: 10.3390/ijms21082724
243. De Roux N, Genin E, Carel JC, Matsuda F, Chaussain JL, Milgrom E. Hypogonadotropic hypogonadism due to loss of function of the KiSS1-derived peptide receptor GPR54. Proc Natl Acad Sci USA (2003) 100(19):10972–6. doi: 10.1073/pnas.1834399100
244. Seminara SB, Messager S, Chatzidaki EE, Thresher RR, Acierno JS Jr, Shagoury JK, et al. The GPR54 gene as a regulator of puberty. N Engl J Med (2003) 349(17):1614–27. doi: 10.1056/NEJMoa035322
245. Dungan HM, Clifton DK, Steiner RA. Minireview: Kisspeptin neurons as central processors in the regulation of gonadotropin-releasing hormone secretion. Endocrinology (2006) 147(3):1154–8. doi: 10.1210/en.2005-1282
246. Zmora N, Stubblefield J, Golan M, Servili A, Levavi-Sivan B, Zohar Y. The medio-basal hypothalamus as a dynamic and plastic reproduction-related kisspeptin-gnrh-pituitary center in fish. Endocrinology (2014) 155(5):1874–86. doi: 10.1210/en.2013-1894
247. Song Y, Chen J, Tao B, Luo D, Zhu Z, Hu W. Kisspeptin2 regulates hormone expression in female zebrafish (Danio rerio) pituitary. Mol Cell Endocrinol (2020) 513:110858. doi: 10.1016/j.mce.2020.110858
248. Smith JT, Cunningham MJ, Rissman EF, Clifton DK, Steiner RA. Regulation of kiss1 gene expression in the brain of the female mouse. Endocrinology (2005) 146(9):3686–92. doi: 10.1210/en.2005-0488
249. Smith JT, Dungan HM, Stoll EA, Gottsch ML, Braun RE, Eacker SM, et al. Differential regulation of kiSS-1 mRNA expression by sex steroids in the brain of the male mouse. Endocrinology (2005) 146(7):2976–84. doi: 10.1210/en.2005-0323
250. Felip A, Zanuy S, Pineda R, Pinilla L, Carrillo M, Tena-Sempere M, et al. Evidence for two distinct KiSS genes in non-placental vertebrates that encode kisspeptins with different gonadotropin-releasing activities in fish and mammals. Mol Cell Endocrinol (2009) 312(1-2):61–71. doi: 10.1016/j.mce.2008.11.017
251. Lee YR, Tsunekawa K, Moon MJ, Um HN, Hwang JI, Osugi T, et al. Molecular evolution of multiple forms of kisspeptins and GPR54 receptors in vertebrates. Endocrinology (2009) 150:2837–46. doi: 10.1210/en.2008-1679
252. Wang Q, Sham KWY, Ogawa S, Li S, Parhar IS, Cheng CHK, et al. Regulation of the two kiss promoters in goldfish (Carassius auratus) by estrogen via different ERα pathways. Mol Cell Endocrinol (2013) 375(1):130–9. doi: 10.1016/j.mce.2013.04.023
253. Bryant AS, Greenwood AK, Juntti SA, Byrne AE, Fernald RD. Dopaminergic inhibition of gonadotropin-releasing hormone neurons in the cichlid fish Astatotilapia burtoni. J Exp Biol (2016) 219(24):3861–5. doi: 10.1242/jeb.147637
254. Liu X, Herbison AE. Dopamine regulation of gonadotropin-releasing hormone neuron excitability in male and female mice. Endocrinology (2013) 154(1):340–50. doi: 10.1210/en.2012-1602
255. Kah O, Dulka JG, Dubourg P, Thibault J, Peter RE. Neuroanatomical substrate for the inhibition of gonadotrophin secretion in goldfish: existence of a dopaminergic preoptico-hypophyseal pathway. Neuroendocrinology (1987) 45(6):451–8. doi: 10.1159/000124774
256. Vidal B, Pasqualini C, Le Belle N, Holland MC, Sbaihi M, Vernier P, et al. Dopamine inhibits luteinizing hormone synthesis and release in the juvenile European eel: a neuroendocrine lock for the onset of puberty. Biol Reprod (2004) 71:1491–500. doi: 10.1095/biolreprod.104.030627
257. Fontaine R, Affaticati P, Bureau C, Colin I, Demarque M, Dufour S, et al. Dopaminergic neurons controlling anterior pituitary functions: anatomy and ontogenesis in zebrafish. Endocrinology (2015) 156(8):2934–48. doi: 10.1210/en.2015-1091
258. Zarate S, Stevnsner T, Gredilla R. Role of estrogen and other sex Hormones in brain aging. Neuroprotection and DNA repair. Front Aging Neurosci (2017) 9:430. doi: 10.3389/fnagi.2017.00430
259. Lin CJ, Fan-Chiang YC, Dufour S, Chang CF. Activation of brain steroidogenesis and neurogenesis during the gonadal differentiation in protandrous black porgy, Acanthopagrus schlegelii. Dev Neurobiol (2016) 76(2):121–36. doi: 10.1002/dneu.22303
260. Grober MS, Bass AH. Neuronal correlates of sex/role change in labrid fishes: LHRH-like immunoreactivity. Brain Behav Evol (1991) 38(6):302–12. doi: 10.1159/000114396
261. Grober MS, Jackson IMD, Bass AH. Gonadal steroids affect LHRH preoptic cell number in a sex/role changing fish. J Neurobiol (1991) 22(7):734–41. doi: 10.1002/neu.480220708
262. Asami K, Atsuhiro T, Toyoji K, Ritsuko OK. Sexual dimorphism of gonadotropin-releasing hormone type-III (GnRH3) neurons and hormonal sex reversal of male reproductive behavior in mozambique tilapia. Zoological Sci (2011) 28(10):733–9. doi: 10.2108/zsj.28.733
263. Narita Y, Tsutiya A, Nakano Y, Ashitomi M, Sato K, Hosono K, et al. Androgen induced cellular proliferation, neurogenesis, and generation of GnRH3 neurons in the brain of mature female Mozambique tilapia. Sci Rep (2018) 8(1):16855. doi: 10.1038/s41598-018-35303-9
264. Vosges M, Le Page Y, Chung BC, Combarnous Y, Porcher JM, Kah O, et al. 17alpha-ethinylestradiol disrupts the ontogeny of the forebrain GnRH system and the expression of brain aromatase during early development of zebrafish. Aquat Toxicol (2010) 99(4):479–91. doi: 10.1016/j.aquatox.2010.06.009
265. Dubois EA, Florijn MA, Zandbergen MA, Peute J, Goos H. Testosterone accelerates the development of the catfish GnRH system in the brain of immature African catfish (Clarias gariepinus). Gen Comp Endocrinol (1998) 112(3):383–93. doi: 10.1006/gcen.1998.7141
266. Kazeto Y, Trant JM. Molecular biology of channel catfish brain cytochrome P450 aromatase (CYP19A2): cloning, preovulatory induction of gene expression, hormonal gene regulation and analysis of promoter region. J Mol Endocrinol (2005) 35(3):571–83. doi: 10.1677/jme.1.01805
267. Callard GV, Petro Z, Ryan KJ. Biochemical evidence for aromatization of androgen to estrogen in the pituitary. Gen Comp Endocrinol (1981) 44(3):359–64. doi: 10.1016/0016-6480(81)90013-7
268. Olivereau M, Callard G. Distribution of cell types and aromatase activity in the sculpin (Myoxocephalus) pituitary. Gen Comp Endocrinol (1985) 58(2):280–90. doi: 10.1016/0016-6480(85)90344-2
269. De Leeuw R, Smit-van Dijk W, Zigterman JW, van der Loo JC, Lambert JG, Goos HJ. Aromatase, estrogen 2-hydroxylase, and catechol-O-methyltransferase activity in isolated, cultured gonadotropic cells of mature African catfish, Clarias gariepinus (Burchell). Gen Comp Endocrinol (1985) 60(2):171–7. doi: 10.1016/0016-6480(85)90311-9
270. Andersson E, Borg B, Lambert JG. Aromatase activity in brain and pituitary of immature and mature Atlantic salmon (Salmo salar L.) parr. Gen Comp Endocrinol (1988) 72(3):394–401. doi: 10.1016/0016-6480(88)90161-x
271. Callard GV, Specker JL, Knapp J, Nishioka RS, Bern HA. Aromatase is concentrated in the proximal pars distalis of tilapia pituitary. Gen Comp Endocrinol (1988) 71(1):70–9. doi: 10.1016/0016-6480(88)90296-1
272. Kazeto Y, Goto-Kazeto R, Place AR, Trant JM. Aromatase expression in zebrafish and channel catfish brains: changes in transcript abundance associated with the reproductive cycle and exposure to endocrine disrupting chemicals. Fish Physiol Biochem (2003) 28(1-4):29–32. doi: 10.1023/B:Fish.0000030466.23085.94
273. Mittelholzer C, Andersson E, Consten D, Hirai T, Nagahama Y, Norberg B. 20β-hydroxysteroid dehydrogenase and CYP19A1 are differentially expressed during maturation in Atlantic cod (Gadus morhua). J Mol Endocrinol (2007) 39(4):319–28. doi: 10.1677/JME-07-0070
274. Dong W, Willett KL. Local expression of CYP19A1 and CYP19A2 in developing and adult killifish (Fundulus heteroclitus). Gen Comp Endocrinol (2008) 155(2):307–17. doi: 10.1016/j.ygcen.2007.05.018
275. Breton TS, DiMaggio MA, Sower SA, Berlinsky DL. Brain aromatase (cyp19a1b) and gonadotropin releasing hormone (gnrh2 and gnrh3) expression during reproductive development and sex change in black sea bass (Centropristis striata). Comp Biochem Physiol Part A Mol Integr Physiol (2015) 181:45–53. doi: 10.1016/j.cbpa.2014.11.020
276. Ramallo MR, Morandini L, Birba A, Somoza GM, Pandolfi M. From molecule to behavior: Brain aromatase (cyp19a1b) characterization, expression analysis and its relation with social status and male agonistic behavior in a Neotropical cichlid fish. Hormones Behav (2017) 89:176–88. doi: 10.1016/j.yhbeh.2017.02.005
277. Shaw K, Krahe R. Pattern of aromatase mRNA expression in the brain of a weakly electric fish, Apteronotus leptorhynchus. J Chem Neuroanat (2018) 90:70–9. doi: 10.1016/j.jchemneu.2017.12.009
278. Strobl-Mazzulla PH, Moncaut NP, López GC, Miranda LA, Canario AVM, Somoza GM. Brain aromatase from pejerrey fish (Odontesthes bonariensis): cDNA cloning, tissue expression, and immunohistochemical localization. Gen Comp Endocrinol (2005) 143(1):21–32. doi: 10.1016/j.ygcen.2005.02.026
279. Jeng SR, Dufour S, Chang CF. Differential expression of neural and gonadal aromatase enzymatic activities in relation to gonadal development in Japanese eel, Anguilla japonica. J Exp Zool A Comp Exp Biol (2005) 303A(9):802–12. doi: 10.1002/jez.a.194
280. Gonzalez A, Piferrer F. Aromatase activity in the European sea bass (Dicentrarchus labrax L.) brain. Distribution and changes in relation to age, sex, and the annual reproductive cycle. Gen Comp Endocrinol (2003) 132(2):223–30. doi: 10.1016/s0016-6480(03)00086-8
281. Lynn SG, Birge WJ, Shepherd BS. Molecular characterization and sex-specific tissue expression of estrogen receptor α (esr1), estrogen receptor βa (esr2a) and ovarian aromatase (cyp19a1a) in yellow perch (Perca flavescens). Comp Biochem Physiol B Biochem Mol Biol (2008) 149(1):126–47. doi: 10.1016/j.cbpb.2007.09.001
282. Silva de Assis HC, Navarro-Martín L, Fernandes LSP, Cardoso CC, Pavoni DP, Trudeau VL. Cloning, partial sequencing and expression analysis of the neural form of P450 aromatase (cyp19a1b) in the South America catfish Rhamdia quelen. Comp Biochem Physiol B Biochem Mol Biol (2018) 221-222:11–7. doi: 10.1016/j.cbpb.2018.04.001
283. Gielen JT, Goos HJ. The brain-pituitary-gonadal axis in the rainbow trout, Salmo gairdneri. II. Direct effect of gonadal steroids on the gonadotropic cells. Cell Tissue Res (1983) 233(2):377–88. doi: 10.1007/BF00238304
284. Denef C. Paracrinicity: the story of 30 years of cellular pituitary crosstalk. J Neuroendocrinol (2008) 20(1):1–70. doi: 10.1111/j.1365-2826.2007.01616.x
285. Yuen CW, Ge W. Follistatin suppresses FSHβ but increases LHβ expression in the goldfish - evidence for an activin-mediated autocrine/paracrine system in fish pituitary. Gen Comp Endocrinol (2004) 135:108–15. doi: 10.1016/j.ygcen.2003.08.012
286. Zhou H, Wang X, Ko WKW, Wong AOL. Evidence for a novel intrapituitary autocrine/paracrine feedback loop regulating growth hormone synthesis and secretion in grass carp pituitary cells by functional interactions between gonadotrophs and somatotrophs. Endocrinology (2004) 145(12):5548–59. doi: 10.1210/en.2004-0362
287. Rebers FE, Hassing GA, Zandbergen MA, Goos HJ, Schulz RW. Regulation of steady-state luteinizing hormone messenger ribonucleic acid levels, de novo synthesis, and release by sex steroids in primary pituitary cell cultures of male African catfish, Clarias gariepinus. Biol Reprod (2000) 62(4):864–72. doi: 10.1095/biolreprod62.4.864
288. Huang YS, Schmitz M, Le Belle N, Chang CF, Quérat B, Dufour S. Androgens stimulate gonadotropin-II β-subunit in eel pituitary cells in vitro. Mol Cell Endocrinol (1997) 131(2):157–66. doi: 10.1016/S0303-7207(97)00100-7
289. Huang YS, Rousseau K, Sbaihi M, Le Belle N, Schmitz M, Dufour S. Cortisol selectively stimulates pituitary gonadotropin beta-subunit in a primitive teleost, Anguilla anguilla. Endocrinology (1999) 140(3):1228–35. doi: 10.1210/endo.140.3.6598
290. Sohn YC, Kobayashi M, Aida K. Regulation of gonadotropin β subunit gene expression by testosterone and gonadotropin-releasing hormones in the goldfish, Carassius auratus. Comp Biochem Physiol B Biochem Mol Biol (2001) 129(2):419–26. doi: 10.1016/S1096-4959(01)00342-6
291. Melamed P, Gur G, Rosenfeld H, Elizur A, Yaron Z. The mRNA levels of GtH Iß , GtH IIß and GH in relation to testicular development and testosterone treatment in pituitary cells of male tilapia. Fish Physiol Biochem (1997) 17(1/6):93–8. doi: 10.1023/A:1007737414565
292. Tse AC, Lau KY, Ge W, Wu RS. A rapid screening test for endocrine disrupting chemicals using primary cell culture of the marine medaka. Aquat Toxicol (2013) 144-145:50–8. doi: 10.1016/j.aquatox.2013.09.022
293. Ando H, Swanson P, Urano A. Regulation of LH synthesis and release by GnRH and gonadal steroids in masu salmon. Fish Physiol Biochem (2003) 28(1):61–3. doi: 10.1023/B:FISH.0000030477.87920.2d
294. Ando H, Swanson P, Kitani T, Koide N, Okada H, Ueda H, et al. Synergistic effects of salmon gonadotropin-releasing hormone and estradiol-17β on gonadotropin subunit gene expression and release in masu salmon pituitary cells in vitro. Gen Compe Endocrinol (2004) 137(1):109–21. doi: 10.1016/J.YGCEN.2004.02.012
295. Weil C, Marcuzzi O. Cultured pituitary cell GtH response to GnRH at different stages of rainbow trout oogenesis and influence of steroid hormones. Gen Comp Endocrinol (1990) 79(3):483–91. doi: 10.1016/0016-6480(90)90079-2
296. Lin SW, Ge W. Differential regulation of gonadotropins (FSH and LH) and growth hormone (GH) by neuroendocrine, endocrine, and paracrine factors in the zebrafish—an in vitro approach. Gen Comp Endocrinol (2009) 160(2):183–93. doi: 10.1016/J.YGCEN.2008.11.020
297. Ando H, Swanson P, Kitani T, Koide N, Okada H, Ueda H, et al. Synergistic effects of salmon gonadotropin-releasing hormone and estradiol-17beta on gonadotropin subunit gene expression and release in masu salmon pituitary cells in vitro. Gen Comp Endocrinol (2004) 137(1):109–21. doi: 10.1016/j.ygcen.2004.02.012
298. Xiong F. The chinook salmon gonadotropin II beta subunit gene contains a strong minimal promoter with a proximal negative element. Mol Endocrinol (1994) 8(6):771–81. doi: 10.1210/me.8.6.771
299. Liu D. Functional analysis of estrogen-responsive elements in chinook salmon (Oncorhynchus tschawytscha) gonadotropin II beta subunit gene. Endocrinology (1995) 136(8):3486–93. doi: 10.1210/en.136.8.3486
300. Rosenfeld H, Levavi-Sivan B, Gur G, Melamed P, Meiri I, Yaron Z, et al. Characterization of tilapia FSHβ gene and analysis of its 5′ flanking region. Comp Biochem Physiol B Biochem Mol Biol (2001) 129(2):389–98. doi: 10.1016/S1096-4959(01)00331-1
301. Yaron Z, Gur G, Melamed P, Rosenfeld H, Levavi-Sivan B, Elizur A. Regulation of gonadotropin subunit genes in tilapia. Comp Biochem Physiol B Biochem Mol Biol (2001) 129(2):489–502. doi: 10.1016/S1096-4959(01)00345-1
302. Sohn YC, Yoshiura Y, Suetake H, Kobayashi M, Aida K. Nucleotide sequence of gonadotropin IIβ subunit gene in goldfish. Fisheries Sci (1999) 65(5):800–1. doi: 10.2331/fishsci.65.800
303. Chong KL, Wang S, Melamed P. Isolation and characterization of the follicle-stimulating hormone beta subunit gene and 5’ flanking region of the Chinook salmon. Neuroendocrinology (2004) 80(3):158–70. doi: 10.1159/000082357
304. Muriach B, Carrillo M, Zanuy S, Cerdá-Reverter JM. Characterization of sea bass FSHβ 5′ flanking region: transcriptional control by 17β-estradiol. Fish Physiol Biochem (2014) 40(3):849–64. doi: 10.1007/s10695-013-9891-6
305. Fostier A, Jalabert B, Billard R, Breton B, Zohar Y. The gonadal steroids. Fish Physiol A (1983) 9:277–372. doi: 10.1016/S1546-5098(08)60291-5
306. Kime DE. Classical’ and ‘non-classical’ reproductive steroids in fish. Rev Fish Biol Fish (1993) 3:160–80. doi: 10.1007/BF00045230
307. Cowan M, Azpeleta C, López-Olmeda JF. Rhythms in the endocrine system of fish: a review. J Comp Physiol B (2017) 187:1057–89. doi: 10.1007/s00360-017-1094-5
308. Royan MR, Kanda S, Kayo D, Song W, Ge W, Weltzien FA, et al. Gonadectomy and blood sampling procedures in small size teleost models. bioRxiv (2020). doi: 10.1101/2020.08.30.271478
309. Rajakumar A, Senthilkumaran B. Steroidogenesis and its regulation in teleost-a review. Fish Physiol Biochem (2020) 46(3):803–18. doi: 10.1007/s10695-019-00752-0
310. Kayo D, Oka Y, Kanda S. Examination of methods for manipulating serum 17β-Estradiol (E2) levels by analysis of blood E2 concentration in medaka (Oryzias latipes). Gen Comp Endocrinol (2020) 285:113272. doi: 10.1016/j.ygcen.2019.113272
311. Tohyama S, Ogino Y, Lange A, Myosho T, Kobayashi T, Hirano Y, et al. Establishment of estrogen receptor 1 (ESR1)-knockout medaka: ESR1 is dispensable for sexual development and reproduction in medaka, Oryzias latipes. Dev Growth Differ (2017) 59(6):552–61. doi: 10.1111/dgd.12386
312. Lu H, Cui Y, Jiang L, Ge W. Functional analysis of nuclear estrogen receptors in zebrafish reproduction by genome editing approach. Endocrinology (2017) 158(7):2292–308. doi: 10.1210/en.2017-00215
Keywords: estrogen, androgen, adenohypophysis, brain, gonads, plasticity, pituitary, steroids
Citation: Fontaine R, Royan MR, von Krogh K, Weltzien F-A and Baker DM (2020) Direct and Indirect Effects of Sex Steroids on Gonadotrope Cell Plasticity in the Teleost Fish Pituitary. Front. Endocrinol. 11:605068. doi: 10.3389/fendo.2020.605068
Received: 11 September 2020; Accepted: 12 October 2020;
Published: 07 December 2020.
Edited by:
Gustavo M. Somoza, CONICET Instituto Tecnológico de Chascomús (INTECH), ArgentinaReviewed by:
Kataaki Okubo, The University of Tokyo, JapanPaula Gabriela Vissio, University of Buenos Aires, Argentina
Copyright © 2020 Fontaine, Royan, von Krogh, Weltzien and Baker. This is an open-access article distributed under the terms of the Creative Commons Attribution License (CC BY). The use, distribution or reproduction in other forums is permitted, provided the original author(s) and the copyright owner(s) are credited and that the original publication in this journal is cited, in accordance with accepted academic practice. No use, distribution or reproduction is permitted which does not comply with these terms.
*Correspondence: Romain Fontaine, cm9tYWluLmZvbnRhaW5lQG5tYnUubm8=