- 1East Carolina Diabetes and Obesity Institute, East Carolina University, Greenville, NC, United States
- 2Department of Kinesiology, East Carolina University, Greenville, NC, United States
- 3Human Performance Laboratory, College of Human Performance and Health, East Carolina University, Greenville, NC, United States
- 4Department of Physiology, East Carolina University, Greenville, NC, United States
Emerging evidence identifies a potent role for aerobic exercise to modulate activity of neurons involved in regulating appetite; however, these studies produce conflicting results. These discrepancies may be, in part, due to methodological differences, including differences in exercise intensity and pre-exercise energy status. Consequently, the current study utilized a translational, well-controlled, within-subject, treadmill exercise protocol to investigate the differential effects of energy status and exercise intensity on post-exercise feeding behavior and appetite-controlling neurons in the hypothalamus. Mature, untrained male mice were exposed to acute sedentary, low (10m/min), moderate (14m/min), and high (18m/min) intensity treadmill exercise in a randomized crossover design. Fed and 10-hour-fasted mice were used, and food intake was monitored 48h. post-exercise. Immunohistochemical detection of cFOS was performed 1-hour post-exercise to determine changes in hypothalamic NPY/AgRP, POMC, tyrosine hydroxylase, and SIM1-expressing neuron activity concurrent with changes in food intake. Additionally, stains for pSTAT3tyr705 and pERKthr202/tyr204 were performed to detect exercise-mediated changes in intracellular signaling. Results demonstrated that fasted high intensity exercise suppressed food intake compared to sedentary trials, which was concurrent with increased anorexigenic POMC neuron activity. Conversely, fed mice experienced augmented post-exercise food intake, with no effects on POMC neuron activity. Regardless of pre-exercise energy status, tyrosine hydroxylase and SIM1 neuron activity in the paraventricular nucleus was elevated, as well as NPY/AgRP neuron activity in the arcuate nucleus. Notably, these neuronal changes were independent from changes in pSTAT3tyr705 and pERKthr202/tyr204 signaling. Overall, these results suggest fasted high intensity exercise may be beneficial for suppressing food intake, possibly due to hypothalamic POMC neuron excitation. Furthermore, this study identifies a novel role for pre-exercise energy status to differentially modify post-exercise feeding behavior and hypothalamic neuron activity, which may explain the inconsistent results from studies investigating exercise as a weight loss intervention.
Introduction
The hypothalamus is a critical nexus of neuron populations that interpret peripheral signals of energy status and deliver diverse efferent outputs to metabolically active tissues (1, 2). These neurons are critical to maintaining metabolic homeostasis, and disruption of their complex neurocircuitry is associated with disordered substrate metabolism and feeding behavior (1, 2). Additionally, emerging evidence identifies a potent role for aerobic exercise to modulate activity and synaptic organization of hypothalamic neurons, especially in the arcuate nucleus (ARC) (3–5). This hypothalamic region contains diverse neuron populations involved in regulating appetite; thus, the ARC presents an attractive target to investigate the regulation of post-exercise feeding behavior.
Identified ARC neurons modulated by acute treadmill exercise include the neuropeptide Y/agouti-related peptide (NPY/AgRPARC) and pro-opiomelanocortin (POMCARC) -expressing neuron populations (3–7). POMCARC neurons suppress food intake by releasing α-melanocyte-stimulating hormone (αMSH), which binds to melanocortin 4 receptors (MC4R’s) to excite satiety-inducing neurons in the paraventricular nucleus of the hypothalamus (PVN) (1, 8). NPY/AgRPARC neurons have opposite effects on MC4R-expressing neuron activity and feeding via co-release of gamma aminobutyric acid (GABA), NPY, and AgRP (1, 2, 9). NPY/AgRP neurons may also directly antagonize POMC neurons via GABAergic connections, but the relevance of this phenomenon in physiological conditions is unclear (10).
Both NPY/AGRPARC and POMCARC neurons are subject to intricate regulation by appetite-stimulating tyrosine hydroxylase neurons in the ARC (THARC) (11). TH is the rate-limiting enzyme in dopamine synthesis (12), and the effects of exercise on THARC neurons have yet to be investigated. THARC neurons exhibit direct excitatory dopaminergic innervation on NPY/AgRPARC neurons, as well as direct inhibitory dopaminergic and GABAergic innervation on POMCARC neurons (11). Moreover, another subpopulation of TH-expressing neurons resides in the PVN (THPVN) and receives presynaptic inputs from NPY/AgRPARC neurons to stimulate thermogenesis in brown adipose tissue (13). To date, the potential role of THPVN neurons in appetite regulation is unknown.
ARC neurons are located adjacent to the third ventricle and the median eminence, which provides convenient access to the cerebrospinal fluid (CSF) and a less selective blood-brain barrier, respectively, and allows for fine responsiveness to changes in energy status. For example, to promote feeding in response to fasting, ghrelin concentrations increase (14), while leptin and insulin levels decrease (15, 16), directly resulting in receptor-mediated increases in NPY/AgRP neuron activity and decreases in POMC neuron activity (17–20). Reduced glucose levels have similar effects on NPY/AgRP and POMC neurons; during which, glucose uptake by glucose transporters is reduced and activity of ATP-sensitive potassium channels is altered (21, 22). Glucose, insulin, leptin, and ghrelin concentrations also differentially fluctuate in response to exercise, depending on intensity and duration (4, 23–25); thus, insight into exercise-mediated changes in hypothalamic neuron activity could be beneficial to understanding of the complex physiological mechanisms regulating post-exercising feeding behavior.
Studies investigating exercise-mediated remodeling of hypothalamic neurocircuits produce conflicting results. For example, Bunner et al. (4) observed acute moderate intensity treadmill exercise (MIE) to increase NPY/AgRPARC neuron activity and subsequent food intake in fed mice, while He et al. (5) demonstrated opposite effects after fed high intensity interval training (HIIT). Results in POMCARC neurons are equally equivocal, with one report observing no changes in response to fasted MIE (4) and others observing increased activity after fasted high intensity exercise (HIE) (3) and fed HIIT (5). Notably, all these studies analyzed neuronal activity at a single timepoint immediately after exercise, and exercise-mediated changes in neuronal activity in the hypothalamus can be rapid and transient (5–7). The reported effects may reflect neuroendocrine responses during exercise, rather than changes after exercise, and may also miss critical changes in neuronal activity in the hours after exercise. Moreover, the studies observing appetite-suppressing, NPY/AgRP neuron inhibiting, and/or POMC activating effects after treadmill exercise used electric shock to motivate mice to run, which was not controlled for in sedentary trials (3, 5). This may be a confounding factor, since studies have demonstrated electric shock activates satiety-inducing neurons in the PVN and acute stress stimulates POMCARC-mediated hypophagia (26–28). Alternatively, He et al. (5) may have identified a unique appetite-suppressing effect specific to HIIT. Supporting this hypothesis, other studies have also observed decreased food intake in response to voluntary wheel running in rodents (29, 30), which typically is a more intermittent exercise model (31).
Another potential explanation for variability in investigations into exercise-mediated changes in hypothalamic neuron activity is that these studies contain methodological differences in exercise intensity and pre-exercise feeding (3–5). Considering glucose and metabolic hormone concentrations fluctuate with energy status and exercise intensity (19, 20, 22, 32), it is plausible that post-exercise changes in hypothalamic neuron activity vary depending on energy status and exercise intensity as well. For example, a recent report demonstrates that NPY/AgRP neurons are activated during fed HIIT, but exhibit opposite effects during fasted HIIT (6). Furthermore, the magnitude of these changes in neuronal activity is directly correlated with exercise intensity (6). Notably, these observations were made during exercise and it remains to be determined if post-exercise changes in hypothalamic neuron activity are similarly dynamic depending on energy status or exercise intensity. This potential phenomenon may explain why the effects of exercise on feeding behavior are inconsistent in the literature and why the success rates of exercise as a weight loss intervention are equally unpredictable (33–44). Overall, the potential confounding factors and methodological differences in studies investigating exercise-mediated modulation of hypothalamic neurons make drawing conclusions challenging; thus, the current study aimed to utilize a translational, well-controlled, within-subject, treadmill exercise protocol to determine the differential effects of fed vs. fasted exercise and exercise intensity on subsequent feeding behavior and hypothalamic neuron activity.
Materials and Methods
Animals
Male B6.Tg(NPY-hrGFP)1Lowl/J (NPY-GFP reporter) mice were cared for in accordance with the National Institutes of Health Guide for the Care and Use of Laboratory Animals, and experimental protocols were approved by Institutional Animal Care and Use Committee of East Carolina University. Mice were fed standard chow ad libitum (3.2kcal/g) and housed at 20–22°C with a 12-h light-dark cycle.
High-Fat Diet-Induced Obesity
5-6-week-old NPY-GFP reporter mice were given ad libitum access to a high-fat diet (5.56kcal/g) with a kilocalorie composition of 58%, 25%, and 17% of fat, carbohydrate, and protein, respectively, for 10 weeks (D12331; Research Diets, New Brunswick, NJ) before undergoing exercise trials.
Acute Treadmill Exercise
Exercise protocols were adapted from a previous study in our lab (4) and all mice were able to successfully complete all exercise trials. Briefly, using a randomized crossover design and commonly used treadmill speeds, untrained mice performed 1-hour sedentary, low (10m/min) (45), moderate (14m/min) (46), and high intensity (18m/min) (3) exercise with one week between bouts. On the day before experiments, mice were familiarized by running for 5 min at 5m/min followed by 5 min at 10m/min. On the day of exercise trials, mice ran at 5m/min for two minutes, 10m/min for two minutes, and then, when applicable, sped up 4m/min every 2 min until the target speed was reached. To minimize stress, a soft bristle brush or gentle puff of air was used to motivate mice, when needed. During sedentary trials, mice were placed in empty cages on top of the running treadmill for 1-hour to simulate stress during exercise bouts. For fasting exercise experiments, food was removed from cages 10-hours before exercise. All sedentary and exercise bouts were performed between 6:30 and 7:30pm., immediately before the dark-phase.
Food Intake and Body Weight Measurements
Food was weighed and added to individually-housed cages immediately after sedentary and exercise bouts (14-15g). Measurements were made 1, 2, 3, 6, 12, 24, and 48-hours post-exercise by subtracting from the total food. Bedding was inspected thoroughly for residual bits of food, which were included in measurements. All food intakes were normalized to body weight, which was measured immediately before sedentary and exercise bouts.
Immunohistochemistry
1-hour post-exercise, when differences in food intake were first observed, mice were intracardially perfused with PBS followed by 10% formalin before immunohistochemistry was performed as described previously (47). Briefly, brains were sliced into 20-μm coronal sections using a freezing microtome (VT1000 S; Leica, Wetzlar, Germany) and incubated overnight in antibody to cFOS (1:250; Santa Cruz Biotechnology, Santa Cruz, CA), POMC (1:6000; Phoenix Pharmaceuticals), tyrosine hydroxylase (TH) (1:100000; Millipore), and SIM1 (1:250; Millipore) followed by incubation with Alexa-fluorophore secondary antibody for 1 h (Abcam). To probe for two proteins with same-source antibodies DAB (3,3′-Diaminobenzidine) staining was performed prior to the immunohistochemistry protocols described above (4). Briefly, brain sections were incubated overnight in antibody to phosphorylated STAT3tyr705 (1:500; Cell Signaling Technology) or phosphorylated ERKthr202/tyr204 (1:500; Cell Signaling Technology) followed by biotinylated donkey anti-rabbit IgG (Vector; 1:1000) for 2 hours. Sections were then incubated in the avidin–biotin complex (ABC; Vector Elite Kit; 1:500) and incubated in 0.04% DAB, 0.02% cobalt chloride (Fisher Scientific), and 0.01% hydrogen peroxide. Note, pSTAT3tyr705 images were inverted (to white) for colocalization. All stains were photographed using an optical microscope (DM6000; Leica), followed by blind analysis using ImageJ. At least three anatomically matched images per mouse were quantified.
Statistical Analysis
For time course food intake experiments, differences among sedentary or exercise bouts were determined using 2-Way-ANOVA with Repeated Measures for time and treatment (n=11/group). Bonferroni corrections were used for multiple comparisons. For comparisons in neuronal activity between sedentary and high intensity exercise, Student’s T-tests were used (n=5-7/group for POMCARC neurons, 4-6/group for SIM1PVN neurons, 5-6/group for THARC neurons, 3-5/group for THPVN neurons, and 4-6/group for NPY/AgRPARC neurons). All analyses were performed using GraphPad Prism statistics software, and p<0.05 was considered statistically significant.
Data Availability Statement
The data sets generated during the current study are available from the corresponding author on reasonable request.
Results
Acute High Intensity Treadmill Exercise Suppresses Food Intake in Fasted Mice
To investigate the effects of varying aerobic exercise intensities on post-exercise food intake, 11 fasted male mice underwent acute sedentary, low intensity (LIE), MIE, and HIE bouts for 1-hour on the treadmill. Cumulative 24-hour food intake was unchanged after LIE and MIE; however, mice ate significantly less after HIE compared to sedentary bouts (5.3%), which persisted for at least 48 hours (6.7%) (Figures 1A, B). Further examination of food intake at specific time intervals revealed HIE-mediated appetite suppression occurred 1-2 hours post-exercise, followed by a compensatory increase in food intake at 6-12 hours, and another period of significantly reduced food intake at 12-24 hours (Figure 1C). MIE also elicited trends toward reduced food intake 1-2 hours post-exercise, but these effects were not statistically significant (p=0.16), and all exercise intensities elicited increases in food intake at 6-12 hours. Taken together, these data suggest HIE uniquely results in a suppression in appetite 1-2 and 12-24-hours post-exercise, resulting in reduced cumulative food intake for at least 48 hours.
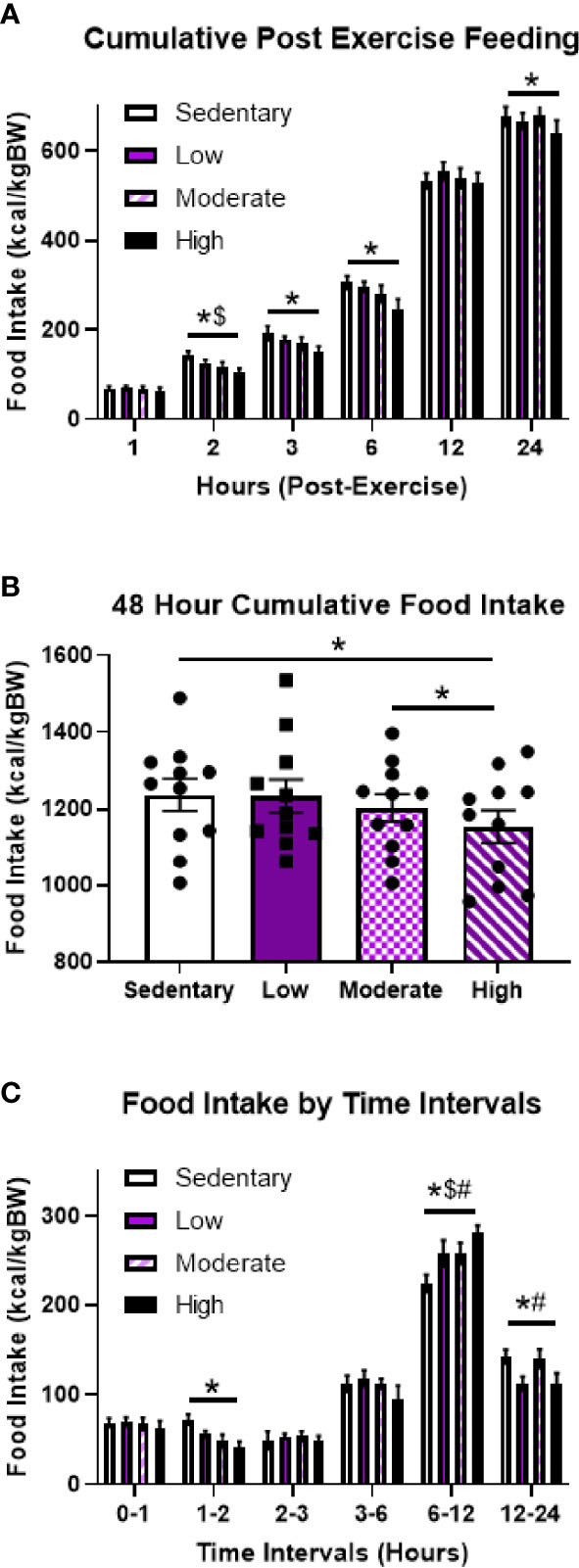
Figure 1 Acute high intensity treadmill exercise suppresses food intake in fasted male mice. (A) Timeline of cumulative food intake, (B) 48-hour cumulative food intake, and (C) food intake by time intervals in fasted male mice in response to different acute treadmill exercise intensities (n = 11). Data represented as mean ± SEM. *indicates p < 0.05 high intensity vs. sedentary; $indicates p < 0.05 moderate intensity vs. sedentary; #indicates p < 0.05 low intensity vs. sedentary.
Surprisingly LIE and HIE had no effects on food intake in DIO mice, but MIE significantly reduced 24-hour food intake 8.5% (Supplemental Figure 1A) and trended to decrease cumulative food intake at 48 hours (p=0.10; Supplemental Figure 1B). Specifically, the reductions in food intake in response to MIE were predominantly observed 2-3 hours post-exercise (Supplemental Figure 1C). In summary, these findings suggest, even in overweight mice, fasted aerobic exercise can have an appetite-suppressing effect.
High Intensity Exercise Increases Activity of POMCARC and SIM1PVN Neurons in Fasted Mice
Since HIE was the only bout that elicited effects on food intake, brains were removed 1-hour after HIE and immunohistochemical detection for cFOS (a marker of neuronal activity) was performed in the hypothalamus to investigate the associated changes in neuronal activity. Compared to sedentary controls, HIE significantly increased cFOS expression in the dorsomedial hypothalamus (1.8-fold) and ARC (4.4-fold), while trending to increase cFOS in the ventromedial hypothalamus (1.4-fold; p=0.08) (Figures 2A, B). Double-staining for cFOS and POMC-expressing neurons revealed that the robust increase in ARC cFOS post-exercise is, in part, due to POMCARC neuron excitation (2-fold) (Figures 2C–E), which are well-documented appetite-suppressing neurons (8). Additionally, HIE increased cFOS colocalization with SIM1PVN-expressing neurons 2.1-fold, which are also satiety-inducing neurons with presynaptic input from POMC neurons (Figures 2F–H) (2, 48). These data suggest that the POMCARC→ SIM1PVN neurocircuit may be activated by HIE.
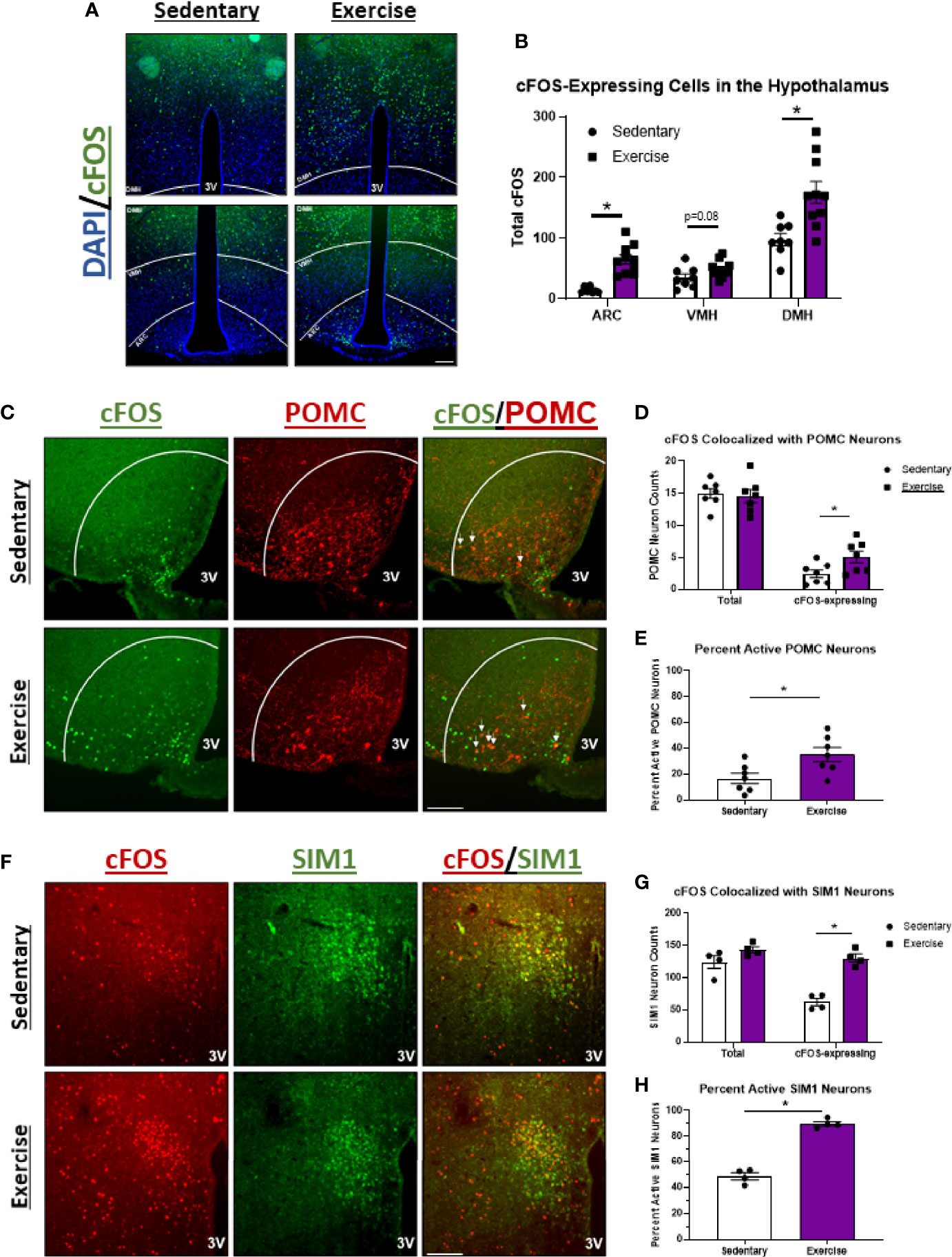
Figure 2 POMCARC and SIM1PVN neuron activity is elevated 1 hour after acute high intensity treadmill exercise in fasted male mice. (A) Representative images of cFOS (green) in the hypothalamus of fasted male mice 1 hour after sedentary trials or high intensity treadmill exercise (DAPI in blue). (B) Total cFOS-expressing cells in each region of the hypothalamus (n=10/group) DMH=dorsomedial hypothalamus; VMH=ventromedial hypothalamus; ARC=arcuate nucleus. (C) Representative images of cFOS (green) colocalized with POMCARC neurons (red) in fasted male mice 1 hour after sedentary trials or high intensity treadmill exercise. (D) Total and cFOS-expressing POMCARC neurons and (E) Percent active POMCARC neurons (n = 7/group). (F) Representative images of cFOS (red) colocalized with SIM1PVN neurons (green) in fasted male mice 1 hour after sedentary trials or high intensity treadmill exercise. (G) Total and cFOS-expressing SIM1PVN neurons and (H) Percent active SIM1PVN neurons (n = 4/group). 3V = Third ventricle; Scale bar = 50um. Data represented as mean ± SEM. *indicates p < 0.05 vs. sedentary trials.
High Intensity Exercise Also Increases Activity of NPY/AgRPARC and THPVN Neurons in Fasted Mice
We also investigated the effects of HIE on activity of appetite-inducing NPY/AgRPARC and THARC neurons. Surprisingly, cFOS colocalization with NPY/AgRPARC neurons was elevated 1.7-fold 1-hour post-HIE (Figures 3A–C). While these data are conflicting with the appetite-suppressing effects of HIE, previous reports suggest exercise-mediated increases in NPY/AgRP neuron activity may be critical to ensuring adequate post-exercise refueling and recovery (4). Moreover, HIE had no effects on cFOS colocalization with THARC neurons (Figures 3D–F); however, cFOS expression in THPVN neurons was increased 8.6-fold (Figures 3G–I).
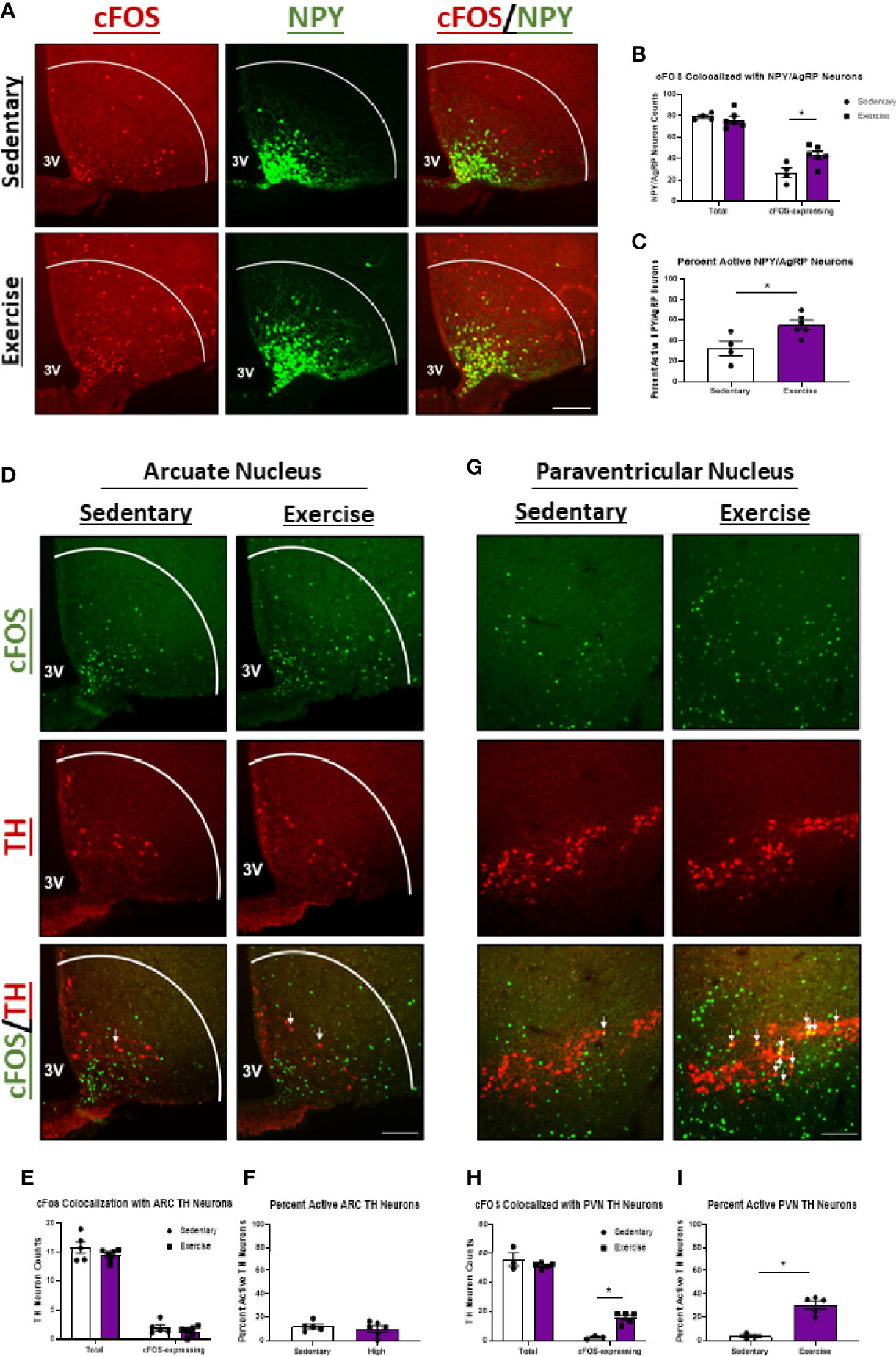
Figure 3 NPY/AgRPARC and THPVN neuron activity is elevated 1 hour after acute high intensity treadmill exercise in fasted male mice. (A) Representative images of cFOS (red) colocalized with NPY/AgRPARC neurons (green) in fasted male mice 1 hour after sedentary trials or high intensity treadmill exercise. (B) Total and cFOS-expressing NPY/AgRPARC neurons and (C) Percent active NPY/AgRPARC neurons (n = 4-6/group). (D) Representative images of cFOS (green) colocalized with THARC neurons (red) in fasted male mice 1 hour after sedentary trials or high intensity treadmill exercise. (E) Total and cFOS-expressing THARC neurons and (F) Percent active THARC neurons (n = 5-6/group). (G) Representative images of cFOS (green) colocalized with THPVN neurons (red) in fasted male mice 1 hour after sedentary trials or high intensity treadmill exercise. (H) Total and cFOS-expressing THPVN neurons and (I) Percent active THPVN neurons (n = 3-5/group). 3V = Third ventricle; Scale bar = 50um. Data represented as mean ± SEM. *indicates p < 0.05 vs. sedentary trials.
The diverse changes in neuronal activity in response to HIE occurred independent from changes in ARC pSTAT3tyr705 and pERKthr202/tyr204 intracellular signaling 1-hour post-exercise (Supplemental Figure 2). pSTAT3tyr705 and pERKthr202/tyr204 are downstream mediators of the anorexigenic hormone leptin to activate POMC neurons and inhibit NPY/AgRP neurons (17, 49); therefore, the appetite suppressing effects of HIE may be independent from leptin activity.
Aerobic Exercise in the Fed State Increases Post-Exercise Food Intake
While our data in fasted mice suggests HIE would be beneficial to creating a caloric deficit by increasing energy expenditure and decreasing food intake, recent studies investigating the effects of aerobic exercise on feeding behavior and appetite-controlling neurons produce conflicting results (3–5). Since the hypothalamic neurons affected by aerobic exercise are sensitive to changes in glucose and metabolic hormones (19, 20, 22, 32), we hypothesized that changes in pre-exercise energy status may differentially modulate the post-exercise feeding response. Compared to 10-hour-fasted sedentary mice, a separate cohort of ad libitum fed sedentary mice exhibited significantly elevated cFOS colocalization with POMCARC neurons (3.3-fold) (Supplemental Figure 3A) and trends toward decreased cFOS colocalization with NPY/AgRPARC neurons (p=0.08; 0.6-fold) (Supplemental Figure 3B). Moreover, both 1-hour and 24-hour cumulative food intake was decreased in fed mice, overall validating significant differences in pre-exercise energy status between fasted and fed mice (Supplemental Figure 3C).
Supporting the hypothesis that pre-exercise energy status dictates post-exercise feeding behavior, all exercise intensities resulted in 8-10% increases in 24-hour food intake compared to the sedentary bout (Figure 4A). Notably, these differences were no longer evident after 48 hours (Figure 4B). Analysis of specific time intervals revealed that LIE specifically elicited increased food intake between 12 and 24 hours post-exercise; however, there were no significant differences at any specific time intervals in response to MIE and HIE (Figure 4C). Overall, while time interval data suggests heterogeneity in the specific post-exercise feeding timelines among fed mice, these findings highlight drastic differences in post-exercise feeding behavior depending on energy status.
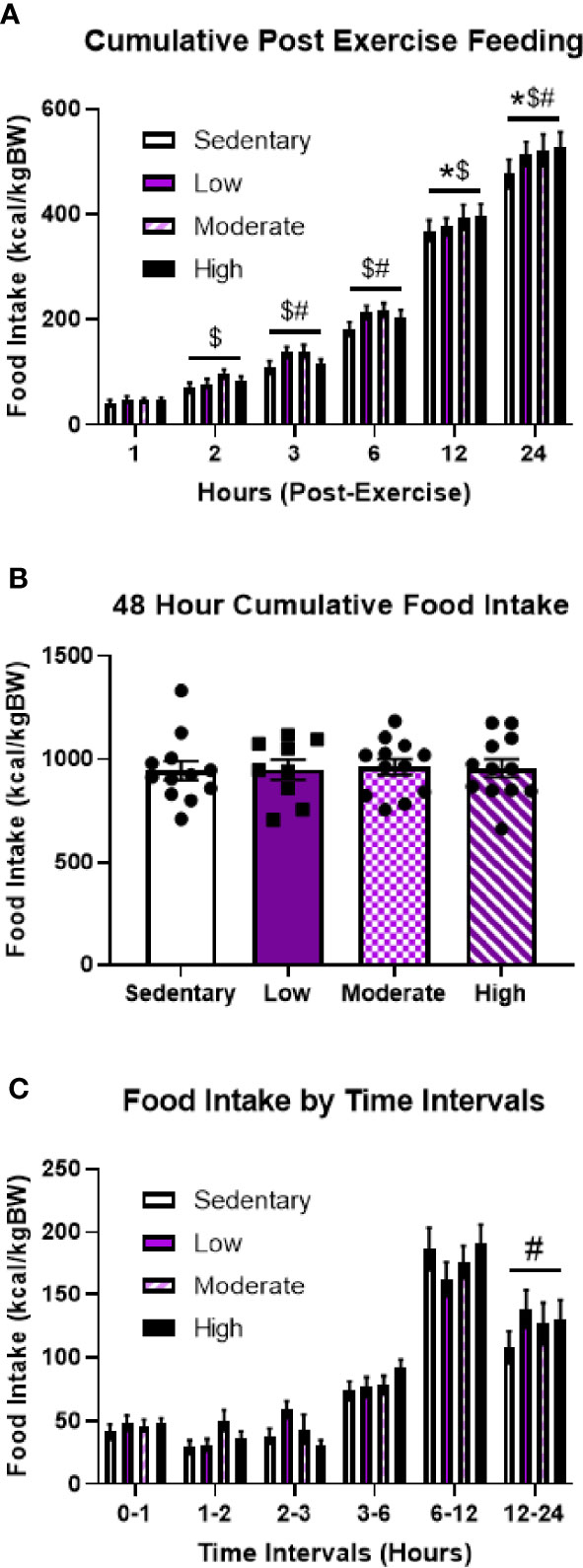
Figure 4 Regardless of intensity, acute treadmill exercise increases food intake in fed male mice. (A) Timeline of cumulative food intake, (B) 48-hour cumulative food intake, and (C) food intake by time intervals in fed male mice in response to different acute treadmill exercise intensities (n = 11). Data represented as mean ± SEM. *indicates p < 0.05 high intensity vs. sedentary; $indicates p < 0.05 moderate intensity vs. sedentary; #indicates p < 0.05 low intensity vs. sedentary.
POMCARC Neuron Excitation Is Specific to High Intensity Exercise in the Fasted Status
We next aimed to compare changes in hypothalamic neuron activity between exercise in the fasted and fed statuses to better understand the neuron populations responsible for energy status-dependent feeding behavior. Similar to HIE in the fasted status, HIE in fed mice resulted in increased cFOS expression in NPY/AgRPARC (1.8-fold; Figures 5A–C), THPVN (11.4-fold; Figures 5D–F), and SIM1PVN (2.2-fold; Figures 5G–I) neurons, with no effects in THARC neurons (Supplemental Figure 4). The similarities in hypothalamic NPY/AgRP, TH, and SIM1 neuron responses between fed and fasted exercise indicate these neuron populations may not be responsible for energy status-dependent effects of exercise on feeding behavior. Interestingly, unlike in fasted mice, fed HIE had no effects on cFOS colocalization with POMCARC neurons (Figure 6), suggesting POMC excitation may be, in part, responsible for energy status-dependent variations in post-exercise feeding.
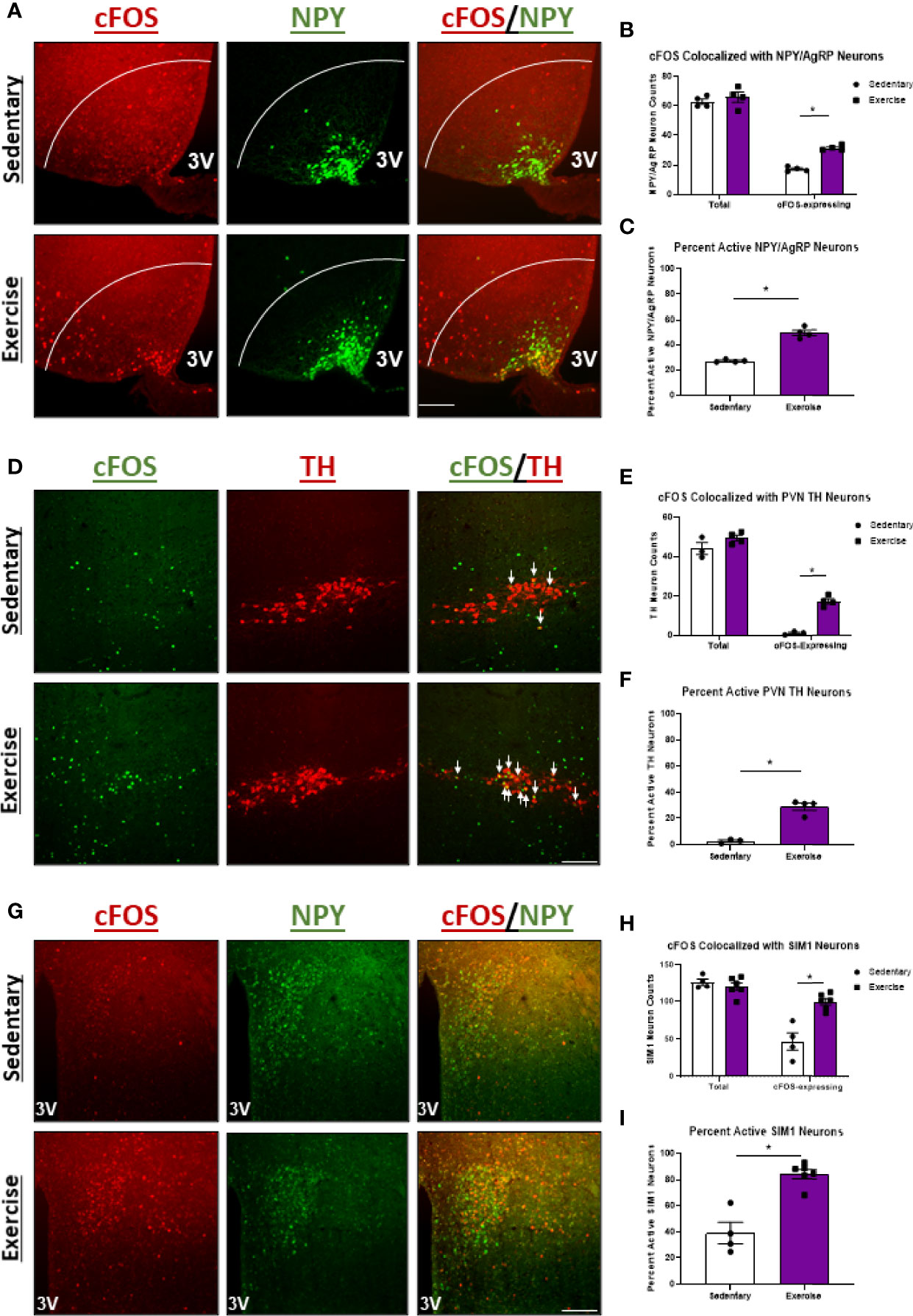
Figure 5 Regardless of energy status, high intensity treadmill exercise increases NPY/AgRPARC, THPVN, and SIM1PVN neuron activity. (A) Representative images of cFOS (red) colocalized with NPY/AgRPARC neurons (green) in male mice 1 hour after sedentary trials or high intensity treadmill exercise. (B) Total and cFOS-expressing NPY/AgRPARC neurons and (C) Percent active NPY/AgRPARC neurons (n=4/group). (D) Representative images of cFOS (green) colocalized with THPVN neurons (red) in fed male mice 1 hour after sedentary trials or high intensity treadmill exercise. (E) Total and cFOS-expressing THPVN neurons and (F) Percent active THPVN neurons (n = 3-4/group). (G) Representative images of cFOS (red) colocalized with SIM1PVN neurons (green) in fed male mice 1 hour after sedentary trials or high intensity treadmill exercise. (H) Total and cFOS-expressing SIM1PVN neurons and (I) Percent active SIM1PVN neurons (n = 4-6/group). 3V, Third ventricle; Scale bar = 50um. Data represented as mean ± SEM. *indicates p < 0.05 vs. sedentary trials.
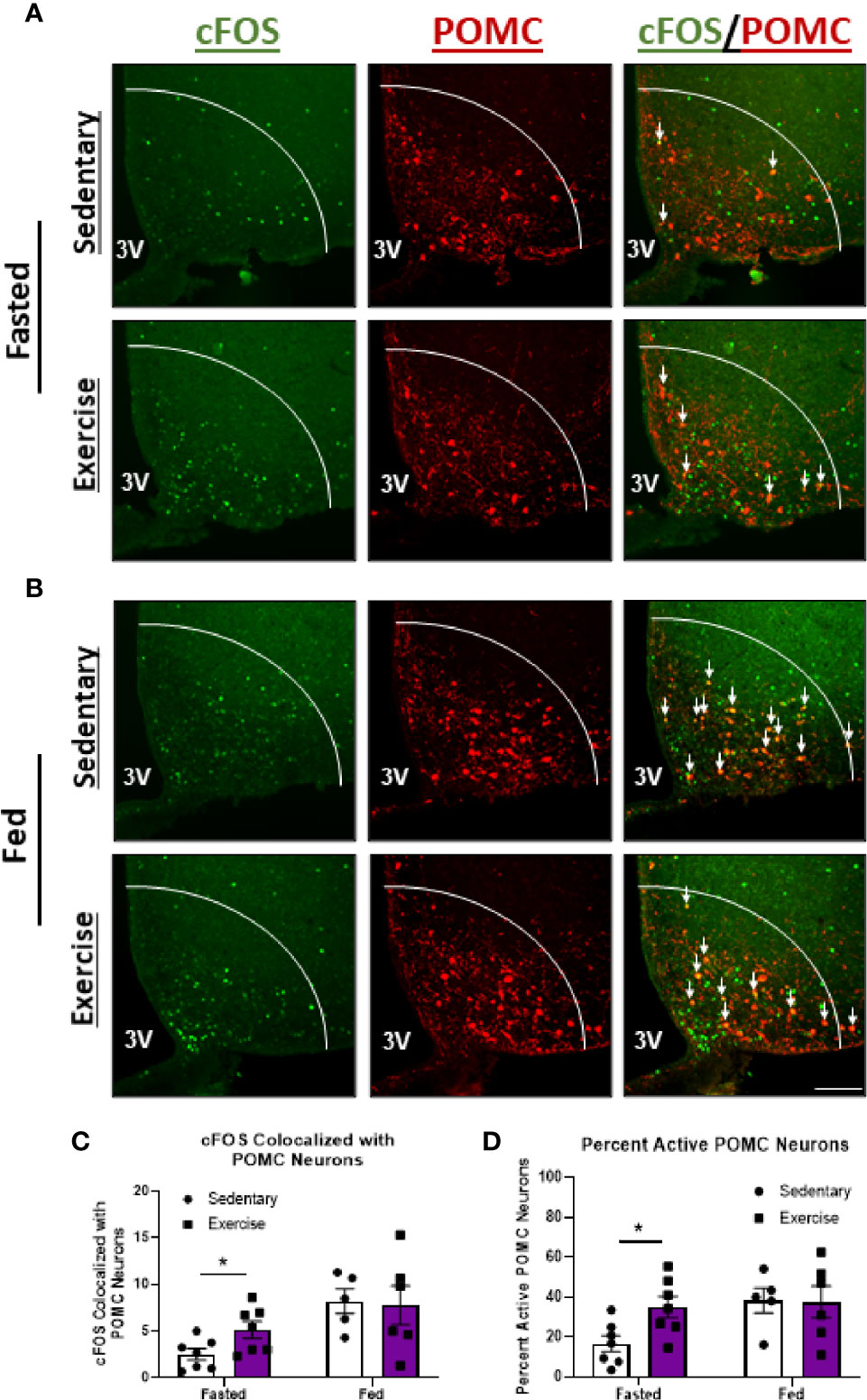
Figure 6 Exercise-mediated POMCARC activation is specific to the fasted status. Representative images of cFOS (green) colocalized with POMCARC neurons (red) in (A) fasted or (B) fed male mice 1 hour after sedentary trials or high intensity treadmill exercise. (C) cFOS-expressing POMCARC neurons and (D) Percent active POMCARC neurons (n = 5-7/group). 3V = Third ventricle; Scale bar = 50um. Data represented as mean ± SEM. *indicates p < 0.05 vs. sedentary trials.
Discussion
Recent studies identifying a potent ability for aerobic exercise to modulate hypothalamic neurons involved in appetite regulation produce conflicting results (3–5). These discrepancies may be explained by differences in methods utilized, including exercise intensity, pre-exercise energy status, and stimulus to promote the exercise. Considering this, the current study used a translational, within-subject, acute treadmill exercise protocol to determine that pre-exercise energy status and exercise intensity drastically modify post-exercise feeding behavior and concurrent hypothalamic neuron activity.
The current study identified a beneficial role of fasted aerobic exercise to suppress food intake in mice, which is consistent with some previous reports in rodents and humans (3, 35, 40, 50, 51). Interestingly, reduced food intake occurred only after HIE in lean mice, whereas in DIO mice, this effect was limited to the MIE bout. At first glance, this suggests DIO mice have a lower intensity threshold to elicit exercise-mediated reductions in food intake; however, intensities used in the current study are based on absolute treadmill speeds and do not account for intrinsic aerobic capacities or differences in body weight. Considering DIO mice have lower aerobic capacities compared to lean mice (52), and greater body mass requires more work to perform the same treadmill exercise, the relative intensity at which DIO mice are exercising is higher at any given absolute treadmill speed. Therefore, it is plausible that the HIE and MIE are eliciting similar relative intensities for the lean and DIO mice, respectively. Consequently, these findings suggest fasted aerobic exercise at higher intensities can reduce food intake regardless of obesity, and, coupled with the associated energy expenditure, may be optimal for creating a caloric deficit and inducing weight loss.
Despite our encouraging evidence suggesting higher intensity exercise can suppress appetite, the literature regarding post-exercise feeding produces inconsistent results (35, 36, 39–44, 50, 51, 53–55). The current study demonstrated a novel phenomenon in which post-exercise feeding behavior is dependent on pre-exercise energy status, which may explain these discrepancies. For example, fasted HIE suppressed food intake in lean mice, but fed exercise increased food intake regardless of intensity. While the reasons for these energy status-dependent differences in feeding behavior are unclear, glucose and metabolic hormone concentrations also fluctuate with energy status, modulating activity of neuron populations involved in regulating feeding behavior (19, 20, 22, 32). Consequently, recent studies have investigated the potential role for exercise to modulate activity of hypothalamic neurons involved in regulating appetite (3–7).
To elucidate the neuronal mechanisms underlying energy status-dependent post-exercise feeding, we compared the changes in hypothalamic neuron activity after fasted vs. fed HIE. The primary difference observed was an increase in anorexigenic POMCARC neuron activity 1-hour after fasted, but not fed, HIE. Since POMCARC neurons suppress food intake through excitatory synaptic connections with MC4RPVN-expressing neurons (1, 8), these results may suggest POMCARC neurons are a mediating factor in fasted HIE-mediated appetite suppression; although at this time it is unclear what is driving these energy status dependent changes. Feeding increases POMCARC neuron activity (1, 56); therefore it’s possible POMCARC neuron activity has plateaued before fed exercise, resulting in no additive effects of exercise on these neurons. Mechanistically, exercise has been shown to increase sensitivity to the POMCARC-stimulating hormone leptin (24, 57); however our data revealed no changes in the ARC in pSTAT3tyr705 or pERKthr202/tyr204, which are key mediators of leptin activity. Ropelle et al. (57) also observed no effects of exercise on hypothalamic pSTAT3tyr705 in lean mice, despite observing augmented appetite suppression in response to intracerebroventricular (ICV) leptin infusion. Since higher intensity exercise can decrease leptin levels (24), the absence of changes in downstream leptin signaling in the hypothalamus post-exercise may suggest compensatory increases in leptin sensitivity. Alternatively, exercise elevates IL-6 concentrations (58), improves insulin sensitivity (57), increases core temperature (3), and decreases ghrelin levels (59), which all can increase POMCARC neuron activity. Future studies investigating differential modulation of hormones and core temperature by fasted vs. fed exercise may provide further explanation into energy-status dependent POMCARC activation.
While our POMCARC results are consistent with past reports observing fasting HIE increases POMCARC neuron activity (3) and no effects of MIE in fed mice (4), one study discovered HIIT has excitatory effects on POMCARC neurons, even in the fed state (5). It is possible HIIT elicits additional appetite-suppressing benefits compared to steady-state HIE, independent from energy status. This hypothesis is supported by studies observing voluntary wheel running, which is a more intermittent model of exercise (31), to also suppress food intake (29, 30). Alternatively, He et al. (5) utilized electric shock as a stimulus to motivate mice during exercise, which may have been a confounding factor. Electric shock has been shown to activate satiety-inducing neurons in the hypothalamus (28), and acute stress in mice promotes POMCARC-mediated hypophagia (26, 27). These confounding factors highlight the importance of minimizing and controlling for stress in these studies.
Increased activity of many other neuron populations in the hypothalamus was observed in the present study in response to HIE. For example, NPY/AgRPARC and SIM1PVN neuron activity was elevated regardless of pre-exercise energy status, suggesting these neuron populations are not driving energy status-dependent feeding behavior after exercise. Activation of the orexigenic NPY/AgRPARC neuron population was surprising, although a previous study demonstrated NPY/AgRPARC activation post-exercise was essential to refeeding (4). Therefore, exercise-mediated NPY/AgRPARC neuron activation may be an evolutionarily preserved mechanism to ensure adequate refueling and recovery. Conversely, He et al., 2018 (5) observed inhibitory effects of HIIT on NPY/AgRPARC neurons, however, as previously mentioned, these effects may be unique to HIIT or a biproduct of electric shock-induced stress. While the importance of exercise-mediated increases in SIM1PVN neuron activity to feeding behavior is unclear, these findings were consistent with a previous study (4). Past reports using chemogenetic approaches determined exercise-induced SIM1PVN activity is independent from presynaptic NPY/AgRPARC neurons (4); however, recently a subpopulation of SIM1PVN neurons that exhibit excitatory synapses on NPY/AgRPARC neurons was identified (60). It is possible that aerobic exercise stimulates a SIM1PVN→NPY/AgRPARC neurocircuit to promote adequate refeeding, and future studies using chemogenetic inhibition of SIM1PVN neurons before aerobic exercise could help verify this hypothesis.
To our knowledge, the present study was the first to investigate the effects of HIE on hypothalamic TH-expressing neurons. THARC neurons stimulate food intake by releasing GABA and dopamine (11); however, no effects of exercise on THARC neuron activity were observed. Interestingly, a striking increase in THPVN neuron activity was observed in response to both fed and fasted exercise. THPVN neurons have been implicated in the regulation of brown adipose tissue thermogenesis (13), and they exhibit downstream synaptic connections in various areas of the hypothalamus (61), but their role in appetite regulation is unknown. Regardless, elevated THPVN neuron activity in response to both fed and fasted exercise suggests these neurons are also not driving factors in energy status-dependent feeding behavior post-exercise.
To summarize the neuronal phenotype in response to acute HIE, NPY/AgRPARC, THPVN, and SIM1PVN neuron activity are elevated 1-hour post-exercise, regardless of energy status, with no effects on THARC neurons. Surprisingly, POMCARC activity is only increased after fasted exercise, suggesting these neurons may possibly mediate suppression of food intake in response to fasted HIE (Figure 7). Notably, the current study only examined neuronal changes 1-hour post-exercise, the time point immediately before changes in food intake were first observed, and changes in neuronal activity after exercise are rapid and transient (5–7). For example, it is unclear what is driving compensatory increases in food intake 6-12-hours after fasted exercise. To better understand the driving factors in post-exercise feeding, future studies into the time course of neuronal activity changes would be valuable.
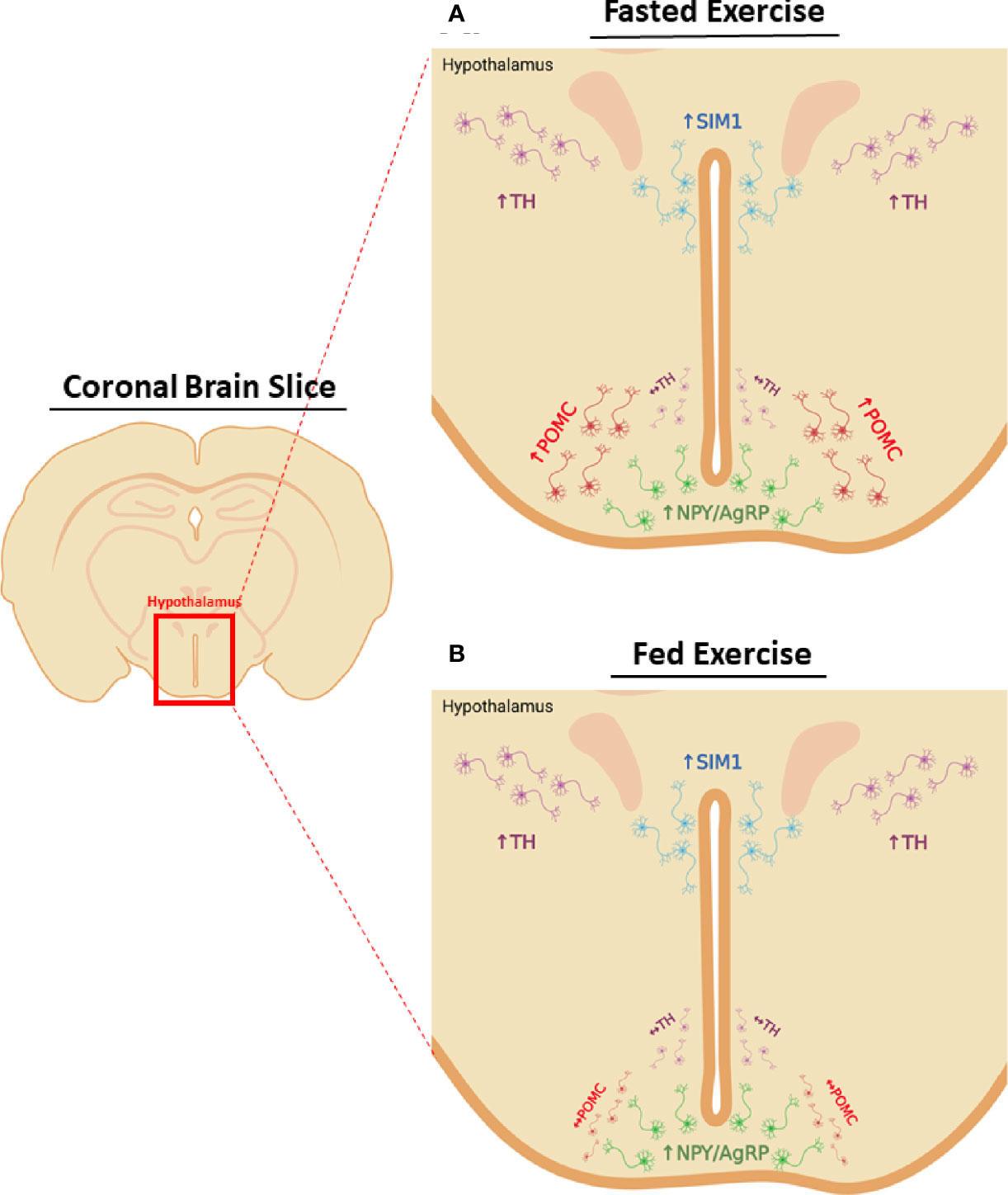
Figure 7 Energy status differentially modifies post-exercise neuronal activity in the hypothalamus. Changes in hypothalamic neuron activity 1-hour after HIE in the (A) fasted or (B) fed status. Regardless of energy status HIE increases NPY/AgRPARC, SIM1PVN, and TH PVN neuron activity, with no effects on THARC neurons. POMCARC neuron activity is only elevated 1-hour after fasted HIE.
Overall, our results identify a novel role for pre-exercise energy status to differentially modify post-exercise feeding behavior and hypothalamic neuron activity, which may explain the significant variability in results from studies investigating exercise as a weight loss intervention. Many other factors are also likely involved in mediating differences in post-exercise feeding, such as sex, training status, exercise volume, time of day, and type of exercise. For example, while our study utilized only male mice, female mice exhibit different regulatory mechanisms governing energy balance, including higher POMC expression (62), lower NPY expression (63), and a critical role for estrogen receptors in CNS control of feeding (64). Furthermore, since we investigated acute aerobic exercise, it is unclear if repeated aerobic exercise training or resistance training also have differential effects on CNS control of food intake. Lastly, to investigate the effects of exercise intensities on food intake, the current study did not control for total exercise volume or energy expenditure. It is possible that the observed effects of fasted HIE to decrease food intake and increase POMCARC neuron activity are due to increased exercise volume rather than intensity.
The effect of exercise on hypothalamic neuron activity is a relatively new focus of research and CNS control of feeding behavior is dynamic and complex. While many factors are likely involved, the current study identifies pre-exercise energy status as a novel variable that drastically modifies post-exercise food intake and appetite-controlling neurons. These findings could have implications when tailoring exercise programs to individual goals; for example, in clinical populations, fasted HIE may be beneficial to decreasing food intake and maximizing caloric deficit.
Guarantor Statement
HH is the guarantor of this work and, as such, has full access to all the data in the study and takes responsibility for its integrity and the accuracy of the analysis.
Data Availability Statement
The raw data supporting the conclusions of this article will be made available by the authors, without undue reservation.
Ethics Statement
The animal study was reviewed and approved by Institutional Animal Care and Use Committee of East Carolina University.
Author Contributions
TL conceptualization, methodology, validation, formal analysis, investigation, writing – original draft, writing – review and editing, and visualization. DS investigation, writing – review and editing. AC conceptualization, writing – review and editing. KF investigation. TN investigation. HH writing – review and editing, supervision, project administration, funding acquisition. All authors contributed to the article and approved the submitted version.
Funding
The funding for this project was provided by East Carolina University start up, the National Institute of Diabetes and Digestive and Kidney Disease (DK121215) to HH.
Conflict of Interest
The authors declare that the research was conducted in the absence of any commercial or financial relationships that could be construed as a potential conflict of interest.
Supplementary Material
The Supplementary Material for this article can be found online at: https://www.frontiersin.org/articles/10.3389/fendo.2021.705267/full#supplementary-material
Supplementary Figure 1 | Acute moderate intensity treadmill exercise suppresses food intake in fasted DIO male mice. (A) Timeline of cumulative food intake, (B) 48 hour cumulative food intake, and (C) food intake by time intervals in fasted male mice in response to different acute treadmill exercise intensities (n=11). Data represented as mean ± SEM. $ indicates p<0.05 moderate intensity vs. sedentary.
Supplementary Figure 2 | ARC pSTAT3tyr705 and pERKthr202/tyr204 are unchanged 1 hour after high intensity treadmill exercise in fasted male mice. (A) Representative inverted DAB images of pSTAT3tyr705 (white) colocalized with NPY/AgRPARC (green) and POMCARC neurons (red) in fasted male mice 1 hour after sedentary trials or high intensity treadmill exercise. (B) Colocalization with NPY/AgRPARC neurons and (C) Colocalization with POMC neurons. (D) Representative DAB images of pERKthr202/tyr204 (black) in the ARC. (E) Total ARC pERKthr202/tyr204 (n=4-5/group). 3V = Third ventricle; Scale bar = 50um. Data represented as mean ± SEM.
Supplementary Figure 3 | Fed mice exhibit elevated POMCARC neuron activity and reduced food intake. (A) Comparison of cFOS colocalized with POMCARC neurons in sedentary fasted mice vs. sedentary fed mice (original data presented in Figure 6). (B) Comparison of cFOS colocalized with NPY/AgRPARC neurons in sedentary fasted mice vs. sedentary fed mice (original data presented in Figures 3 and 6). (C) Comparison of cumulative food intake in sedentary fasted mice vs. sedentary fed mice (original data presented in Figures 1 and 4).
Supplementary Figure 4 | High intensity treadmill exercise has no effects on THARC neuron activity. (A) Representative images of cFOS (green) colocalized with THARC neurons (red) in fed male mice 1 hour after sedentary trials or high intensity treadmill exercise. (B) Total and cFOS-expressing THARC neurons and (C) Percent active THARC neurons (n=5-6/group).
References
1. Andermann ML, Lowell BB. Toward a Wiring Diagram Understanding of Appetite Control. Neuron (2017) 95:757–78. doi: 10.1016/j.neuron.2017.06.014
2. Atasoy D, Nicholas Betley J, Su HH, Sternson SM. Deconstruction of a Neural Circuit for Hunger. Nature (2012) 488:172–7. doi: 10.1038/nature11270
3. Jeong JH, Lee DK, Liu SM, Chua SC, Schwartz GJ, Jo YH. Activation of Temperature-Sensitive TRPV1-Like Receptors in ARC POMC Neurons Reduces Food Intake. PloS Biol (2018) 16:e2004399. doi: 10.1371/journal.pbio.2004399
4. Bunner W, Landry T, Laing BT, Li P, Rao Z, Yuan Y, et al. Arcagrp/NPY Neuron Activity Is Required for Acute Exercise-Induced Food Intake in Un-Trained Mice. Front Physiol (2020) 11:411. doi: 10.3389/fphys.2020.00411
5. He Z, Gao Y, Alhadeff AL, Castorena CM, Huang Y, Lieu L, et al. Cellular and Synaptic Reorganization of Arcuate NPY/AgRP and POMC Neurons After Exercise. Mol Metab (2018) 18:107–19. doi: 10.1016/j.molmet.2018.08.011
6. Lieu L, Chau D, Afrin S, Dong Y, Alhadeff AL, Betley JN, et al. Effects of Metabolic State on the Regulation of Melanocortin Circuits. Physiol Behav (2020) 224:113039. doi: 10.1016/j.physbeh.2020.113039
7. Miletta MC, Iyilikci O, Shanabrough M, Šestan-Peša M, Cammisa A, Zeiss CJ, et al. AgRP Neurons Control Compulsive Exercise and Survival in an Activity-Based Anorexia Model. Nat Metab (2020) 2:1204–11. doi: 10.1038/s42255-020-00300-8
8. Zhan C, Zhou J, Feng Q, Zhang J-E, Lin S, Bao J, et al. Acute and Long-Term Suppression of Feeding Behavior by POMC Neurons in the Brainstem and Hypothalamus, Respectively. J Neurosci (2013) 33:3624–32. doi: 10.1523/JNEUROSCI.2742-12.2013
9. Krashes MJ, Koda S, Ye C, Rogan SC, Adams AC, Cusher DS, et al. Rapid, Reversible Activation of AgRP Neurons Drives Feeding Behavior in Mice. J Clin Invest (2011) 121:1424–8. doi: 10.1172/JCI46229
10. Rau AR, Hentges ST. The Relevance of AgRP Neuron-Derived GABA Inputs to POMC Neurons Differs for Spontaneous and Evoked Release. J Neurosci (2017) 37:7362–72. doi: 10.1523/JNEUROSCI.0647-17.2017
11. Zhang X, Van Den Pol AN. Hypothalamic Arcuate Nucleus Tyrosine Hydroxylase Neurons Play Orexigenic Role in Energy Homeostasis. Nat Neurosci (2016) 19:1341–7. doi: 10.1038/nn.4372
12. Daubner SC, Le T, Wang S. Tyrosine Hydroxylase and Regulation of Dopamine Synthesis. Arch Biochem Biophys (2011) 508:1–12. doi: 10.1016/j.abb.2010.12.017
13. Shi YC, Lau J, Lin Z, Zhang H, Zhai L, Sperk G, et al. Arcuate NPY Controls Sympathetic Output and BAT Function Via a Relay of Tyrosine Hydroxylase Neurons in the PVN. Cell Metab (2013) 17:236–48. doi: 10.1016/j.cmet.2013.01.006
14. Toshinai K, Mondal MS, Nakazato M, Date Y, Murakami N, Kojima M, et al. Upregulation of Ghrelin Expression in the Stomach Upon Fasting, Insulin-Induced Hypoglycemia, and Leptin Administration. Biochem Biophys Res Commun (2001) 281:1220–5. doi: 10.1006/bbrc.2001.4518
15. Frederich RC, Lollmann B, Hamann A, Napolitano-Rosen A, Kahn BB, Lowell BB, et al. Expression of Ob mRNA and its Encoded Protein in Rodents. Impact of Nutrition and Obesity. J Clin Invest (1995) 96:1658–63. doi: 10.1172/JCI118206
16. Holmstrup ME, Owens CM, Fairchild TJ, Kanaley JA. Effect of Meal Frequency on Glucose and Insulin Excursions Over the Course of a Day. E-SPEN (2010) 5:e277–80. doi: 10.1016/j.eclnm.2010.10.001
17. Varela L, Horvath TL. Leptin and Insulin Pathways in POMC and AgRP Neurons That Modulate Energy Balance and Glucose Homeostasis. EMBO Rep (2012) 13:1079–86. doi: 10.1038/embor.2012.174
18. Chen S-R, Chen H, Zhou J-J, Pradhan G, Sun Y, Pan H-L, et al. Ghrelin Receptors Mediate Ghrelin-Induced Excitation of Agouti-Related Protein/Neuropeptide Y But Not Pro-Opiomelanocortin Neurons. J Neurochem (2017) 142:512–20. doi: 10.1111/jnc.14080
19. Mayer CM, Belsham DD. Insulin Directly Regulates NPY and AgRP Gene Expression Via the MAPK MEK/ERK Signal Transduction Pathway in mHypoE-46 Hypothalamic Neurons. Mol Cell Endocrinol (2009) 307:99–108. doi: 10.1016/j.mce.2009.02.031
20. Qiu J, Zhang C, Borgquist A, Nestor CC, Smith AW, Bosch MA, et al. Insulin Excites Anorexigenic Proopiomelanocortin Neurons Via Activation of Canonical Transient Receptor Potential Channels. Cell Metab (2014) 19:682–93. doi: 10.1016/j.cmet.2014.03.004
21. Belgardt BF, Okamura T, Brüning JC. Hormone and Glucose Signalling in POMC and AgRP Neurons. J Physiol (2009) 587:5305–14. doi: 10.1113/jphysiol.2009.179192
22. Claret M, Smith MA, Batterham RL, Selman C, Choudhury AI, Fryer LGD, et al. AMPK Is Essential for Energy Homeostasis Regulation and Glucose Sensing by POMC and AgRP Neurons. J Clin Invest (2007) 117:2325–36. doi: 10.1172/JCI31516
23. Mani BK, Castorena CM, Osborne-Lawrence S, Vijayaraghavan P, Metzger NP, Elmquist JK, et al. Ghrelin Mediates Exercise Endurance and the Feeding Response Post-Exercise. Mol Metab (2018) 9:114–30. doi: 10.1016/j.molmet.2018.01.006
24. Bouassida A, Zalleg D, Bouassida S, Zaouali M, Feki Y, Zbidi A, et al. Leptin, Its Implication in Physical Exercise and Training: A Short Review. J Sports Sci Med (2006) 5:172–81.
25. Borghouts LB, Keizer HA. Exercise and Insulin Sensitivity: A Review. Int J Sports Med (2000) 21:1–12. doi: 10.1055/s-2000-8847
26. Calvez J, Fromentin G, Nadkarni N, Darcel N, Even P, Tomé D, et al. Inhibition of Food Intake Induced by Acute Stress in Rats Is Due to Satiation Effects. Physiol Behav (2011) 104:675–83. doi: 10.1016/j.physbeh.2011.07.012
27. Qu N, He Y, Wang C, Xu P, Yang Y, Cai X, et al. A POMC-originated Circuit Regulates Stress-Induced Hypophagia, Depression, and Anhedonia. Mol Psychiatry (2020) 25:1006–21. doi: 10.1038/s41380-019-0506-1
28. Lin X, Itoga CA, Taha S, Li MH, Chen R, Sami K, et al. c-Fos Mapping of Brain Regions Activated by Multi-Modal and Electric Foot Shock Stress. Neurobiol Stress (2018) 8:92–102. doi: 10.1016/j.ynstr.2018.02.001
29. Obici S, Magrisso IJ, Ghazarian AS, Shirazian A, Miller JR, Loyd CM, et al. Moderate Voluntary Exercise Attenuates the Metabolic Syndrome in Melanocortin-4 Receptor-Deficient Rats Showing Central Dopaminergic Dysregulation. Mol Metab (2015) 4:692–705. doi: 10.1016/j.molmet.2015.07.003
30. O’Neal TJ, Friend DM, Guo J, Hall KD, Kravitz AV. Increases in Physical Activity Result in Diminishing Increments in Daily Energy Expenditure in Mice. Curr Biol (2017) 27:423–30. doi: 10.1016/j.cub.2016.12.009
31. Meijer JH, Robbers Y. Wheel Running in the Wild. Proc R Soc B Biol Sci (2014) 281:20140210. doi: 10.1098/rspb.2014.0210
32. Pocai A, Morgan K, Buettner C, Gutierrez-Juarez R, Obici S, Rossetti L. Central Leptin Acutely Reverses Diet-Induced Hepatic Insulin Resistance. Diabetes (2005) 54:3182–9. doi: 10.2337/diabetes.54.11.3182
33. Johnson NA, Sachinwalla T, Walton DW, Smith K, Armstrong A, Thompson MW, et al. Aerobic Exercise Training Reduces Hepatic and Visceral Lipids in Obese Individuals Without Weight Loss. Hepatology (2009) 50:1105–12. doi: 10.1002/hep.23129
34. Swift DL, Johannsen NM, Lavie CJ, Earnest CP, Church TS. The Role of Exercise and Physical Activity in Weight Loss and Maintenance. Prog Cardiovasc Dis (2014) 56:441–7. doi: 10.1016/j.pcad.2013.09.012
35. Katch VL, Martin R, Martin J. Effects of Exercise Intensity on Food Consumption in the Male Rat. Am J Clin Nutr (1979) 32:1401–7. doi: 10.1093/ajcn/32.7.1401
36. King N, Burley V, Blundell J. Exercise-Induced Suppression of Appetite: Effects on Food Intake and Implications for Energy Balance. Eur J Clin Nutr (1994) 48(10):715–24.
37. Thorogood A, Mottillo S, Shimony A, Filion KB, Joseph L, Genest J, et al. Isolated Aerobic Exercise and Weight Loss: A Systematic Review and Meta-Analysis of Randomized Controlled Trials. Am J Med (2011) 124:747–55. doi: 10.1016/j.amjmed.2011.02.037
38. Thomas DM, Bouchard C, Church T, Slentz C, Kraus WE, Redman LM, et al. Why do Individuals Not Lose More Weight From an Exercise Intervention at a Defined Dose? An Energy Balance Analysis. Obes Rev (2012) 13:835–47. doi: 10.1111/j.1467-789X.2012.01012.x
39. Donnelly JE, Smith BK. Is Exercise Effective for Weight Loss With Ad Libitum Diet? Energy Balance, Compensation, and Gender Differences. Exerc Sport Sci Rev (2005) 33:169–74. doi: 10.1097/00003677-200510000-00004
40. King NA, Hopkins M, Caudwell P, Stubbs RJ, Blundell JE. Individual Variability Following 12 Weeks of Supervised Exercise: Identification and Characterization of Compensation for Exercise-Induced Weight Loss. Int J Obes (2008) 32:177–84. doi: 10.1038/sj.ijo.0803712
41. Dorling J, Broom DR, Burns SF, Clayton DJ, Deighton K, James LJ, et al. Acute and Chronic Effects of Exercise on Appetite, Energy Intake, and Appetite-Related Hormones: The Modulating Effect of Adiposity, Sex, and Habitual Physical Activity. Nutrients (2018) 10:1140. doi: 10.3390/nu10091140
42. Douglas JA, King JA, McFarlane E, Baker L, Bradley C, Crouch N, et al. Appetite, Appetite Hormone and Energy Intake Responses to Two Consecutive Days of Aerobic Exercise in Healthy Young Men. Appetite (2015) 92:57–65. doi: 10.1016/j.appet.2015.05.006
43. Ebrahimi M, Rahmani-Nia F, Damirchi A, Mirzaie B, Pur SA. Effect of Short-Term Exercise on Appetite, Energy Intake and Energy-Regulating Hormones. Iran J Basic Med Sci (2013) 16:829–34. doi: 10.22038/ijbms.2013.1117
44. Flack KD, Hays HM, Moreland J, Long DE. Exercise for Weight Loss: Further Evaluating Energy Compensation With Exercise. Med Sci Sports Exerc (2020) 52:2466–75. doi: 10.1249/MSS.0000000000002376
45. Bobinski F, Teixeira JM, Sluka KA, Santos ARS. Interleukin-4 Mediates the Analgesia Produced by Low-Intensity Exercise in Mice With Neuropathic Pain. Pain (2018) 159:437–50. doi: 10.1097/j.pain.0000000000001109
46. Kim Y-A, Pitriani P, Park H-G, Lee W-L. Moderate Intensity Exercise Has More Positive Effects on The Gene Expression of Inflammasome, M1, M2 Macrophage Infiltration and Brown Adipocyte Markers Compared to High Intensity Exercise in Subcutaneous Adipose of Obese Mice Induced by High Fat Diet. J Life Sci (2019) 29:303–10. doi: 10.5352/JLS.2019.29.3.303
47. Landry T, Li P, Shookster D, Jiang Z, Li H, Laing BT, et al. Centrally Circulating α-Klotho Inversely Correlates With Human Obesity and Modulates Arcuate Cell Populations in Mice. Mol Metab (2021) 44:101136. doi: 10.1016/j.molmet.2020.101136
48. Millington GWM. The Role of Proopiomelanocortin (POMC) Neurones in Feeding Behaviour. Nutr Metab (2007) 4:1–16. doi: 10.1186/1743-7075-4-18
49. Rahmouni K, Sigmund CD, Haynes WG, Mark AL. Hypothalamic ERK Mediates the Anorectic and Thermogenic Sympathetic Effects of Leptin. Diabetes (2009) 58:536–42. doi: 10.2337/db08-0822
50. Nadermann N, Volkoff H. Effects of Short-Term Exercise on Food Intake and the Expression of Appetite-Regulating Factors in Goldfish. Peptides (2020) 123:170182. doi: 10.1016/j.peptides.2019.170182
51. Thompson DA, Wolfe LA, Eikelboom R. Acute Effects of Exercise Intensity on Appetite in Young Men. Med Sci Sport Exerc (1988) 20:222–7. doi: 10.1249/00005768-198806000-00002
52. Setty P, Padmanabha BV, Doddamani BR. Obesity and Cardio Respiratory Fitness. Int J Med Sci Public Heal (2013) 2:30–44. doi: 10.5455/ijmsph.2013.2.298-302
53. Staten MA. The Effect of Exercise on Food Intake in Men and Women. Am J Clin Nutr (1991) 53:27–31. doi: 10.1093/ajcn/53.1.27
54. Stensel D. Exercise, Appetite and Appetite-Regulating Hormones: Implications for Food Intake and Weight Control. Ann Nutr Metab (2010) 57:36–42. doi: 10.1159/000322702
55. Vatansever-Ozen S, Tiryaki-Sonmez G, Bugdayci G, Ozen G. The Effects of Exercise on Food Intake and Hunger: Relationship With Acylated Ghrelin and Leptin. J Sport Sci Med (2011) 10:283–91.
56. Mizuno TM, Kleopoulos SP, Bergen HT, Roberts JL, Priest CA, Mobbs CV. Hypothalamic Pro-Opiomelanocortin mRNA Is Reduced by Fasting in Ob/Ob and Db/Db Mice, But is Stimulated by Leptin. Diabetes (1998) 47:294–7. doi: 10.2337/diab.47.2.294
57. Ropelle ER, Flores MB, Cintra DE, Rocha GZ, Pauli JR, Morari J, et al. IL-6 and IL-10 Anti-Inflammatory Activity Links Exercise to Hypothalamic Insulin and Leptin Sensitivity Through Ikkβ and ER Stress Inhibition. PloS Biol (2010) 8:31–2. doi: 10.1371/journal.pbio.1000465
58. Fischer CP. Interleukin-6 in Acute Exercise and Training: What Is the Biological Relevance? Exerc Immunol Rev (2006) 12:6–33.
59. Broom DR, Stensel DJ, Bishop NC, Burns SF, Miyashita M. Exercise-Induced Suppression of Acylated Ghrelin in Humans. J Appl Physiol (2007) 102:2165–71. doi: 10.1152/japplphysiol.00759.2006
60. Krashes MJ, Shah BP, Madara JC, Olson DP, Strochlic DE, Garfield AS, et al. An Excitatory Paraventricular Nucleus to AgRP Neuron Circuit That Drives Hunger. Nature (2014) 507:238–42. doi: 10.1038/nature12956
61. Ogundele OM, Lee CC, Francis J. Thalamic Dopaminergic Neurons Projects to the Paraventricular Nucleus-Rostral Ventrolateral Medulla/C1 Neural Circuit. Anat Rec (2017) 300:1307–14. doi: 10.1002/ar.23528
62. Nohara K, Zhang Y, Waraich RS, Laque A, Tiano JP, Tong J, et al. Early-Life Exposure to Testosterone Programs the Hypothalamic Melanocortin System. Endocrinology (2011) 152:1661–9. doi: 10.1210/en.2010-1288
63. Urban JH, Bauer-Dantoin AC, Levine JE. Neuropeptide Y Gene Expression in the Arcuate Nucleus: Sexual Dimorphism and Modulation by Testosterone. Endocrinology (1993) 132:139–45. doi: 10.1210/en.132.1.139
Keywords: exercise, food intake, hypothalamus, POMC neuron, NPY/AgRP neuron, tyrosine hydroxylase (th), SIM1 neurons, energy balance
Citation: Landry T, Shookster D, Chaves A, Free K, Nguyen T and Huang H (2021) Energy Status Differentially Modifies Feeding Behavior and POMCARC Neuron Activity After Acute Treadmill Exercise in Untrained Mice. Front. Endocrinol. 12:705267. doi: 10.3389/fendo.2021.705267
Received: 05 May 2021; Accepted: 31 May 2021;
Published: 18 June 2021.
Edited by:
Hiroyuki Kaiya, National Cerebral and Cardiovascular Center, JapanReviewed by:
María Florencia Andreoli, Consejo Nacional de Investigaciones Científicas y Técnicas (CONICET), ArgentinaJoão Cavalcanti De Albuquerque, Federal University of Rio de Janeiro, Brazil
Copyright © 2021 Landry, Shookster, Chaves, Free, Nguyen and Huang. This is an open-access article distributed under the terms of the Creative Commons Attribution License (CC BY). The use, distribution or reproduction in other forums is permitted, provided the original author(s) and the copyright owner(s) are credited and that the original publication in this journal is cited, in accordance with accepted academic practice. No use, distribution or reproduction is permitted which does not comply with these terms.
*Correspondence: Hu Huang, huangh@ecu.edu