- 1Department of Biochemistry and Molecular Biology, Cumming School of Medicine, University of Calgary, Calgary, Canada
- 2Department of Comparative Biology and Experimental Medicine, Faculty of Veterinary Medicine, University of Calgary, Calgary, Canada
- 3Department of Biological Sciences, Faculty of Science, University of Calgary, Calgary, Canada
An in vitro system to study testicular maturation in rats, an important model organism for reproductive toxicity, could serve as a platform for high-throughput drug and toxicity screening in a tissue specific context. In vitro maturation of somatic cells and spermatogonia in organ culture systems has been reported. However, this has been a challenge for organoids derived from dissociated testicular cells. Here, we report generation and maintenance of rat testicular organoids in microwell culture for 28 days. We find that rat organoids can be maintained in vitro only at lower than ambient O2 tension of 15% and organoids cultured at 34°C have higher somatic cell maturation and spermatogonial differentiation potential compared to cultures in 37°C. Upon exposure to known toxicants, phthalic acid mono-2-ethylhexyl ester and cadmium chloride, the organoids displayed loss of tight-junction protein Claudin 11 and altered transcription levels of somatic cell markers that are consistent with previous reports in animal models. Therefore, the microwell-derived rat testicular organoids described here can serve as a novel platform for the study of testicular cell maturation and reproductive toxicity in vitro.
Introduction
Male factor infertility is responsible for 40-50% of all cases of infertility worldwide (1) and around 9-16% of all men suffer from infertility (2). The causes of infertility are varied; some are due to pathophysiological conditions, while others may be caused by environmental toxicants such as exposure to phthalates and heavy metals (3–5).
Spermatogenesis is a highly orchestrated process that is dependent on a tightly regulated stem cell niche (6). This spermatogonial stem cell niche is primarily composed of spermatogonia and somatic cells, such as Sertoli cells, peritubular myoid cells and Leydig cells (6). The regulation of spermatogonial cell fate is tightly modulated by these somatic cells (7, 8). In vitro culture systems to model this niche have primarily been limited to conventional two-dimensional co-culture systems (9), which fail to mimic the cell-cell signaling seen in vivo (10, 11). A three-dimensional organotypic culture system can bridge the gap between cell cultures and whole animal models to better study developmental processes such as spermatogonial differentiation and reproductive toxicology in vitro (12, 13). Several three-dimensional testicular organoid systems have been developed with this goal in mind (11, 14–18).
Here, we describe the adaptation of microwell-derived testicular organoids, previously established in porcine, murine, human and primate models (11), to rat testicular organoids and highlight the species-specific challenges encountered during this endeavor. Rats have been extensively studied as an animal model for testicular maturation and reproductive toxicology (19–22). We report here that organotypic rat testicular organoids can be derived and maintained for 28 days only at lower than ambient (15%) O2 tension. We also characterize rat organoids cultured at both 37°C and 34°C and show that rat organoids cultured at lower temperature better support the differentiation of spermatogonia. Finally, we present proof of principle with known environmental toxicants to establish the utility of rat testicular organoids for the study of reproductive toxicity.
Materials and Methods
Preparation of Rat Starting Testicular Cell Population
SAS Sprague Dawley rats (Strain: code 400, Charles River), aged P4-P5, were euthanized and testes were removed. All animal procedures were performed as approved by the Animal Care Committee, University of Calgary. Using a pair of forceps, the tunica albuginea was removed to release the tubules, which were then washed in Hank’s Balanced Salt Solution (HBSS, Gibco, cat# 14025092) containing 1% penicillin-streptomycin (ThermoFisher Scientific, cat# 15070063). The tubules were digested using collagenase type IV (Worthington-Biochem, cat# LS004189) in HBSS (2 mg/mL) for 25 min at 37°C. The tubules were then sedimented by centrifugation at 90x g for 1.5 min and washed with 5 mL HBSS thrice. Finally, the tissue was digested with 0.25% trypsin–EDTA (Sigma-Aldrich, cat# T4049) and DNase I (Sigma-Aldrich, cat# DN25) in HBSS (10 mg/mL) for 5 min to obtain the starting cell population (11). All experiments were replicated using a minimum of three independently prepared cell suspensions.
Generation of Organoids
AggreWell 400 plates (STEMCELL Technologies Inc, Vancouver, Canada, cat# 34450) were treated with Anti-adherence Rinsing Solution (STEMCELL Technologies Inc, Vancouver, Canada, cat# 07010) according to the manufacturer’s instructions. Each well was filled with 500 μL of organoid formation medium (OFM) (Dulbecco Modified Eagle Medium F/12 (Gibco, cat# 11330-032) supplemented with insulin 10 μg/mL, transferrin 5.5 μg/mL, selenium 6.7 ng/mL (Gibco, cat#41400-045); 20 ng/mL epidermal growth factor (R & D Systems, cat# 236-EG); 1% Penicillin-Streptomycin) and the plates were then centrifuged at 2000x g for 2 minutes to release any trapped air bubbles (11). Each well was then seeded with 6 × 105 rat testicular cells suspended in 500 μL OFM. Finally, the plates were centrifuged at 500x g for 5 minutes to sediment the cells in the microwells. 500 μL of media were removed from each well and fresh media supplemented with Corning Matrigel Growth Factor Reduced (GFR) Basement Membrane Matrix (1:100 dilution; Life Sciences, cat# 354230) was used to replenish each well. Microwell plates were divided into three groups and placed in 3 incubators setup for three conditions: (i) ambient O2 tension (18.4% or 6.34 mM O2, 5% CO2, 37°C), (ii) 15% O2 tension at 37°C (15% or 5.18 mM O2, 5% CO2) and (iii) 15% O2 tension at 34°C (15% or 5.22 mM O2, 5% CO2). Culture in OFM was carried out for 3-5 days with 50% media changes every second day.
Maturation and Differentiation of Organoids
After culturing the organoids for 3 days, which were designated as day 0 undifferentiated organoids, the OFM media was completely removed, and the culture was continued in organoid differentiation medium (ODM). ODM was composed of Minimum Essential Medium α (ThermoFisher Scientific, cat# 12571063) supplemented with 10% KnockOut Serum Replacement (ThermoFisher Scientific, cat# 10828028), hepatocyte growth factor (5 ng/mL) (R&D Systems, cat# 294-HG), activin A (100 ng/mL) (Sigma-Aldrich, cat# a4941), follicle stimulating hormone (1 ng/mL) (Sigma-Aldrich, cat# F4021), luteinizing hormone (1 ng/mL) (Sigma-Aldrich, cat# L5259), testosterone (1 μM) (Steraloids, cat# A6950-000), recombinant human BMP-4 (20 ng/mL) (R&D Systems, cat# 314-BP), recombinant human BMP-7 (20 ng/mL) (R&D Systems, cat# 354-BP), 3,3’,5-triodo-L-thyronine sodium (2 ng/mL) (Sigma-Aldrich, cat# T6397), l-ascorbic acid-2-glucoside (1 mM) (Matrix Scientific, cat# 092375) and 1% Penicillin-Streptomycin (22, 23). Culture was carried out for an additional 28 days, with full media changes every second day. The organoids were sampled every 7 days, including day 0, for analysis.
Immunohistochemistry
Testes tissue from 43-day old rats were fixed in 4% paraformaldehyde, dehydrated with a gradient series of ethanol, and embedded in paraffin wax to prepare sections of 5 µm thickness. The rat starting cell populations and organoids were fixed using 2% paraformaldehyde and spun down on slides using cytospin centrifugation (1000 rpm for cells and 500 rpm for organoids) (Cytospin 4, Thermo Scientific). The samples were then permeabilized using a gradient series of methanol (24) and blocked with 10% donkey serum. The testes tissue was incubated with anti-γ-H2AX (Gamma H2A Histone Family X) (25) and anti-SYCP3 (Synaptonemal Complex Protein 3) (26) (Supplementary Table 1). For rat testicular cells, the slides were incubated with anti-GATA4 (GATA Binding Protein 4) (27), anti-VASA (DEAD-Box Helicase 4) (28), anti-α-SMA(alpha-Smooth Muscle Actin) (29), anti-3β-HSD (3 Beta-Hydroxysteroid Dehydrogenase) (28) (Supplementary Table 1). In addition to the antibodies mentioned above, rat organoids were also incubated with anti-Collagen IV (30), anti-Laminin (31), anti-Fibronectin (32), anti-Claudin 11 (33), anti-UCHL1 (Ubiquitin C-terminal Hydrolase L1), anti-TNP1 (Transition Protein 1) (34), anti-PRM1 (Protamine 1) (35), anti-ACR (Acrosin) (36) and anti-AR (Androgen Receptor) (37) antibodies overnight at 4°C (Supplementary Table 1). Fluorescence labelling was done with secondary antibodies conjugated with Alexa Fluor 488 and 555 (Supplementary Table 1). DAPI (4′,6-diamidino-2-phenylindole) (Vector, cat# H1200) was used for labelling the nuclei. The cells were analyzed using Zeiss Imager.M2 fluorescence microscopy and the percentages of testicular cell types were determined by counting the cells with ImageJ software. The organoids were analyzed using a Leica TCS-SP8 confocal laser scanning microscope with the Leica Las X software.
Cell Number Quantification Within Organoids
Immunohistochemistry for VASA was performed on day 7 and 28 organoids, while SYCP3 staining was performed on day 28 organoids. Using confocal microscopy, organoids were selected blindly based on only DAPI and scanned across the z-axis to quantify the number of VASA+ve and SYCP3+ve cells in each organoid. From each of the three independent experiments (n = 3) performed, a total of 30 organoids were analyzed for VASA+ve and 10 organoids were analyzed for SYCP3+ve cell counts.
Reverse Transcription Quantitative Polymerase Chain Reaction
RNA was isolated from 1200 organoids using RNeasy Micro Kit (QIAGEN, cat # 74004) and then reverse transcription was performed using SuperScript™ IV VILO™ Master Mix (Thermo Fisher Scientific, cat# 11756050). RT-qPCR with the primers listed in Supplementary Table 2 was performed with a 7.500 Fast Real-Time PCR System (Applied Biosystems) using SsoFast Eva Green Supermix with Low ROX (Bio-Rad laboratories, cat# 1725211). The expression levels were presented relative to Gapdh. Statistical analysis was performed on the mean of ΔΔCt.
MEHP and CdCl2 Treatment
Day 19 organoids were treated with 1 μM MEHP and 0.25 μM CdCl2. Controls were treated with equivalent volumes of DMSO. After 48 hours of treatment, at day 21, the organoids were harvested and analyzed with immunofluorescence and RT-qPCR.
Dose Determination
Day 5 organoids were treated with 0.5, 1 and 1.5 μM of MEHP (Sigma, Cat# 796832) and 0.01, 0.05, 0.25 and 1.25 μM of CdCl2 (Sigma, Cat# 202908). The control groups for MEHP and CdCl2 were treated with equivalent volumes of DMSO for 1.5 μM of MEHP and 1.25 μM of CdCl2, respectively. Controls of 1.5 μM and 1.25 μM DMSO were treated with equivalent volumes of phosphate buffered saline (Thermo Fisher Scientific, cat# 14190144). At day 7 (48 hours after treatment), the organoids were harvested and approximately 60 organoids suspended in 50 μL ODM were seeded in each well of a 96-well plate as duplicates to perform the MTT assay (Abcam, cat# ab211091) according to manufacturer’s instructions. Absorbance at OD 590 nm was measured using SpectraMax i3x plate reader (Molecular Devices). MTT assay was used to measure cellular metabolic activity as an indicator of cell viability.
Statistical Analysis
All the results described here are from at least three independent experiments performed with three separately prepared rat starting cell population (n = 3). Data were analyzed using the GraphPad Prism 8 software. Unpaired two-tailed t-tests were performed for single comparisons between two groups. For more than two groups, one-way ANOVA with Tukey’s multiple comparison tests were performed. A value of p < 0.05 was set as the limit of statistical significance.
Results
Rat Testicular Cells Generate and Maintain Organotypic Testicular Organoids at 15% O2 Tension
A rat (P4-P5) testicular starting cell population which contained 92.2 ± 1.28% GATA4+ve Sertoli cells (38), 1.7 ± 0.3% VASA+ve germ cells (a marker for spermatogonia, spermatocytes and round spermatids) (39), 2.6 ± 0.7% 3β-HSD+ve Leydig cells (40) and 19.97 ± 2.8% α-SMA+ve peritubular myoid cells (41) (n = 3) was used to generate rat testicular organoids with organoid formation media (OFM). Since lower O2 tension is known to support higher differentiation potential of rat testicular cells (22), initial cultures were carried out in incubators set up for ambient and 15% O2 tensions (37°C). Both culture conditions supported the initial generation of testicular organoids (72 hours), with organotypic morphology similar to our previously published porcine and murine model (11). However, unlike the porcine or murine models (11), the rat organoids cultured in ambient O2 tension underwent a loss of testis-specific tissue architecture at day 6 of culture while day 6 organoids cultured at 15% O2 tension had distinct internal-interstitial and external-seminiferous epithelial compartments. The two compartments were separated by a collagen IV+ve, fibronectin+ve and laminin+ve basement membrane (Figure 1A). The external compartment was composed of VASA+ve germ cells and GATA4+ve Sertoli cells. α-SMA+ve peritubular myoid cells were located lining the basement membrane in the interior compartment, while 3β-HSD+ve Leydig cells were distributed throughout the interior compartment (Figure 1A). In contrast, the organoids cultured at ambient O2 tension showed increased Sertoli cell numbers, generation of large Sertoli cell clusters and complete or partial separation of internal and external compartments (Figure 1B). Thus, subsequent experiments were carried out at 15% O2 tension.
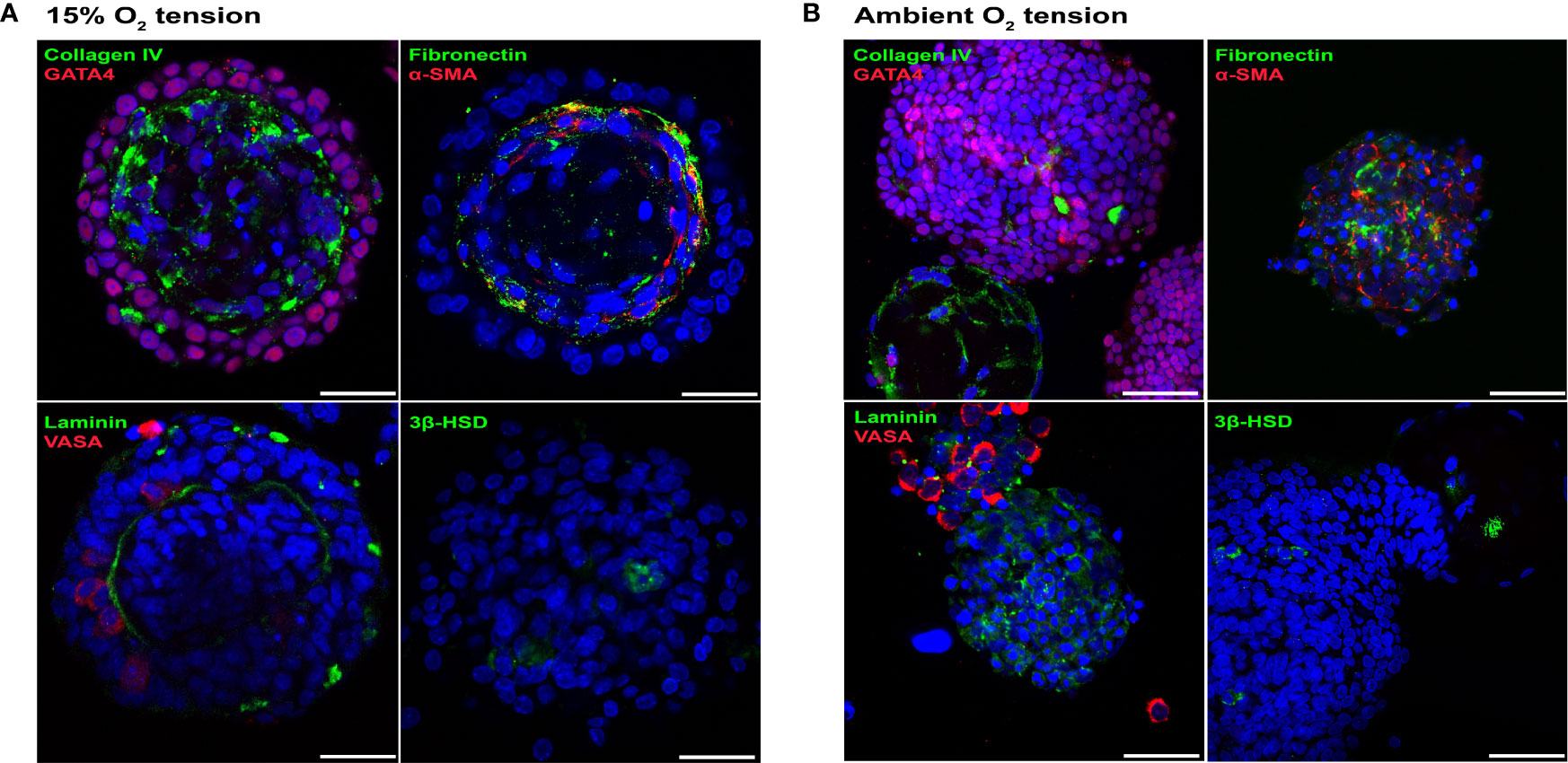
Figure 1 Rat testicular organoids cultured at 15% O2 tension maintained organotypic morphology. (A, B) Immunofluorescence images of day 6 rat testicular organoids at 15% (A) and ambient (B) O2 tensions showing the distribution of basement membrane (collagen IV, fibronectin, laminin), Sertoli cells (GATA4), peritubular myoid cells (α-SMA), germ cells (VASA) and Leydig cells (3β-HSD). Scale bars measure 25 μm.
Under Optimized Conditions, Rat Testicular Organoids Undergo Maturation and Support Spermatogonial Differentiation
Matsumura et al. (22) and Sato et al. (23) reported efficient spermatogenic differentiation of rodent testicular organ cultures at 34°C. To evaluate the effect of temperature on somatic cell and spermatogonial maturation, rat organoids were generated with OFM (72 hours: day 0 undifferentiated organoid) and then cultured with organoid differentiation medium (ODM) at 37°C and 34°C (22, 42) for up to 28 days. Immunofluorescence analysis revealed no morphological differences between the two groups at day 28 (Figure 2A) and both cultures showed expression of Claudin 11, a component of Sertoli cell tight junctions (Figure 2B) (43). Except for expression of Shbg (sex hormone binding globulin) (Sertoli and Leydig cells), which was increased 5.7-fold (n = 3, p < 0.05) in 34°C cultures, no significant differences in transcription levels were observed between the two groups for the somatic cell markers Fshr (follicle stimulating hormone receptor) (Sertoli cells), Star (steroidogenic acute regulatory protein), Cyp17a1 (cytochrome P450 family 17 subfamily a member 1) (Leydig cells) and Hsd17b3 (hydroxysteroid 17-beta dehydrogenase 3) (Sertoli and Leydig cells) (Supplementary Figure 1A) (n = 3, p > 0.05) (44–47). At day 7, rat testicular organoids contained UCHL1+ve undifferentiated spermatogonia at both temperatures (Supplementary Figure 1B). Both at day 7 and 28, there was no difference in the number of VASA+ve germ cells between 37°C and 34°C cultures (n = 3, p > 0.05) (Figures 2C, D). However, the number of VASA+ve germ cells was lower at day 28 compared to day 7 at both temperature conditions (n = 3, p < 0.05) (Figure 2D). SYCP3+ve spermatogenic cells were observed in both conditions, with a staining pattern similar to spermatocytes in 43-day old rat testes (Supplementary Figure 1E), starting from day 21. γ-H2AX, which is induced by the DNA double stranded break in leptotene and early zygotene, was also observed in both culture conditions with a staining pattern similar to 43-day old rat testes (Supplementary Figures 1D, E) (25). The number of SYCP3+ve was quantified and their number was found to be higher at day 28 in the organoids cultured at 34°C compared to 37°C (n = 3, p < 0.05) (Figures 2E, F) (48, 49). Therefore, the 37°C cultures were excluded from further analysis.
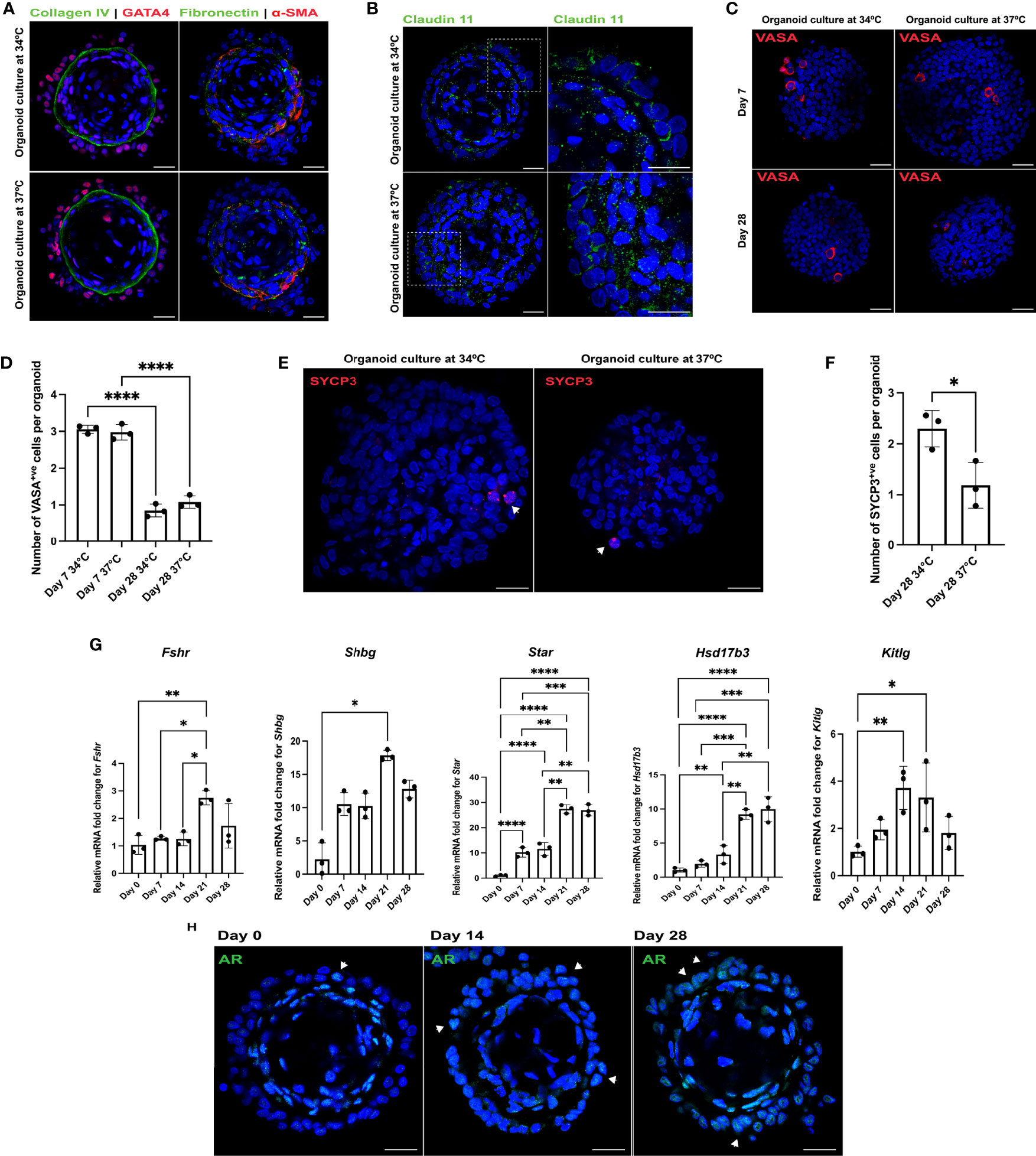
Figure 2 Rat testicular organoids support somatic cell maturation and spermatogonial differentiation at 34°C culture. (A–C, E) Immunofluorescent images of day 28 rat testicular organoids cultured at 34°C and 37°C showing (A) basement membrane (collagen IV, fibronectin), Sertoli cells (GATA4), peritubular myoid cells (α-SMA), (B) tight junction protein (Claudin 11) (inserts showing the magnified area on the right panel), (C) germ cells (VASA) and (E) meiotic cells (SYCP3) (indicated with white arrows). Scale bars measure 25 μm. (D) Number of germ cells adhered to each organoid. Bars indicate mean ± SD, n = 3. Analysis was performed using one-way ANOVA followed by Tukey’s multiple comparison test. (F) Number of meiotic cells adhered to each organoid. Bars indicate mean ± SD, n = 3. Analysis was performed using unpaired two-tailed t-test. (G) Relative mRNA fold change of Fshr, Shbg, Star, Hsd17b3 and Kitlg for organoids cultured at 34°C. Bars indicate mean ± SD, n = 3. Analysis for Fshr, Star, Hsd17b3 and Kitlg was performed using one-way ANOVA followed by Tukey’s multiple comparison test and analysis for Shbg was performed using Kruskal-Wallis test with Dunn’s multiple comparison test. (H) Immunofluorescent detection of AR (indicated with white arrows) in the 34°C culture at day 0, day 14 and day 28. Scale bars measure 25 μm. p ≤ 0.05 (*), p ≤ 0.01 (**), p ≤ 0.001 (***), p ≤ 0.0001 (****). Only significant differences are indicated with asterisks.
To characterize maturation during the 28-day long culture, transcription levels of the immature Sertoli cell marker Amh (anti-mullerian hormone) and Sertoli and Leydig cell markers Fshr, Shbg, Star, Hsd17b3 and Kitlg were analyzed for day 0, 7, 14, 21, 28 organoids. The transcription levels of Amh were undetectable within a week (n = 3, p < 0.05) while expression of Cyp17a1 showed no significant changes over the duration of the culture (n = 3, p > 0.05). Fshr, Shbg and Kitlg were upregulated by 2.7-, 17.9- and 3.3-fold at day 21 (n = 3, p < 0.05) (Figure 2G). Transcription levels of Star and Hsd17b3 increased 26.9- and 10-fold, respectively, by day 28 of culture (n = 3, p < 0.05) (Figure 2G). In addition, an increasing number of Sertoli cells in the organoids started to express AR with subsequent weeks of culture, indicating cell maturation (Figure 2H). Along with punctate SYCP3 staining (potentially leptotene spermatocytes) (Figure 2E), an elongated pattern of SYCP3 staining (as observed in early zygotene spermatocytes) (50) (Supplementary Figure 1C) was also detected at day 28. However, cells found with elongated SYCP3 expression were no longer adhered to the organoids at the time of analysis (Supplementary Figure 1C). In contrast, no PRM1+ve, TNP1+ve or ACR+ve cells were observed in the cultures.
Rat Testicular Organoids to Model Reproductive Toxicity: Proof of Principle
Initial dose-response experiments on monolayers of rat testicular cells (P4-P5) were used to select the dosages of 0.5, 1 and 1.5 μM for phthalic acid mono-2-ethylhexyl ester (MEHP); and 0.01, 0.05, 0.25 and 1.25 μM for the heavy metal cadmium chloride (CdCl2) to be tested on organoids. Then, relative cell viability assessments were performed on day 7 (treatment began on day 5) organoids treated with the aforementioned dosages with a 3-(4,5-dimethylthiazol-2-yl)-2,5-diphenyl-2H-tetrazolium bromide (MTT) assay, revealing that 1 μM MEHP and 0.25 μM CdCl2 were the highest doses without adverse effects on viability (n = 3, p < 0.05) (Figures 3A, B). Thus, all other dosages were excluded from further analyses. Since most of the maturation markers showed robust upregulation at day 21, toxicological effects of MEHP and CdCl2 were evaluated at day 21 by treating the organoids with MEHP and CdCl2 at day 19 and then harvesting and analyzing 48 hours later. MEHP treatment was associated with upregulation of Fshr and Star and downregulation of the expression of Cyp17a1 (n = 3, p < 0.05) (Figure 3D). Expression of Fshr, Shbg, Hsd17b3 and Cyp17a1 was drastically downregulated upon exposure to CdCl2 (n = 3, p < 0.05) (Figure 3D). Transcription of Star, in contrast, was upregulated 7-fold compared to DMSO controls (n = 3, p < 0.05) (Figure 3D). In contrast to MEHP which led to partial loss of tight-junction protein Claudin11, CdCl2 treatment caused a total loss of Claudin 11 (Figure 3C).
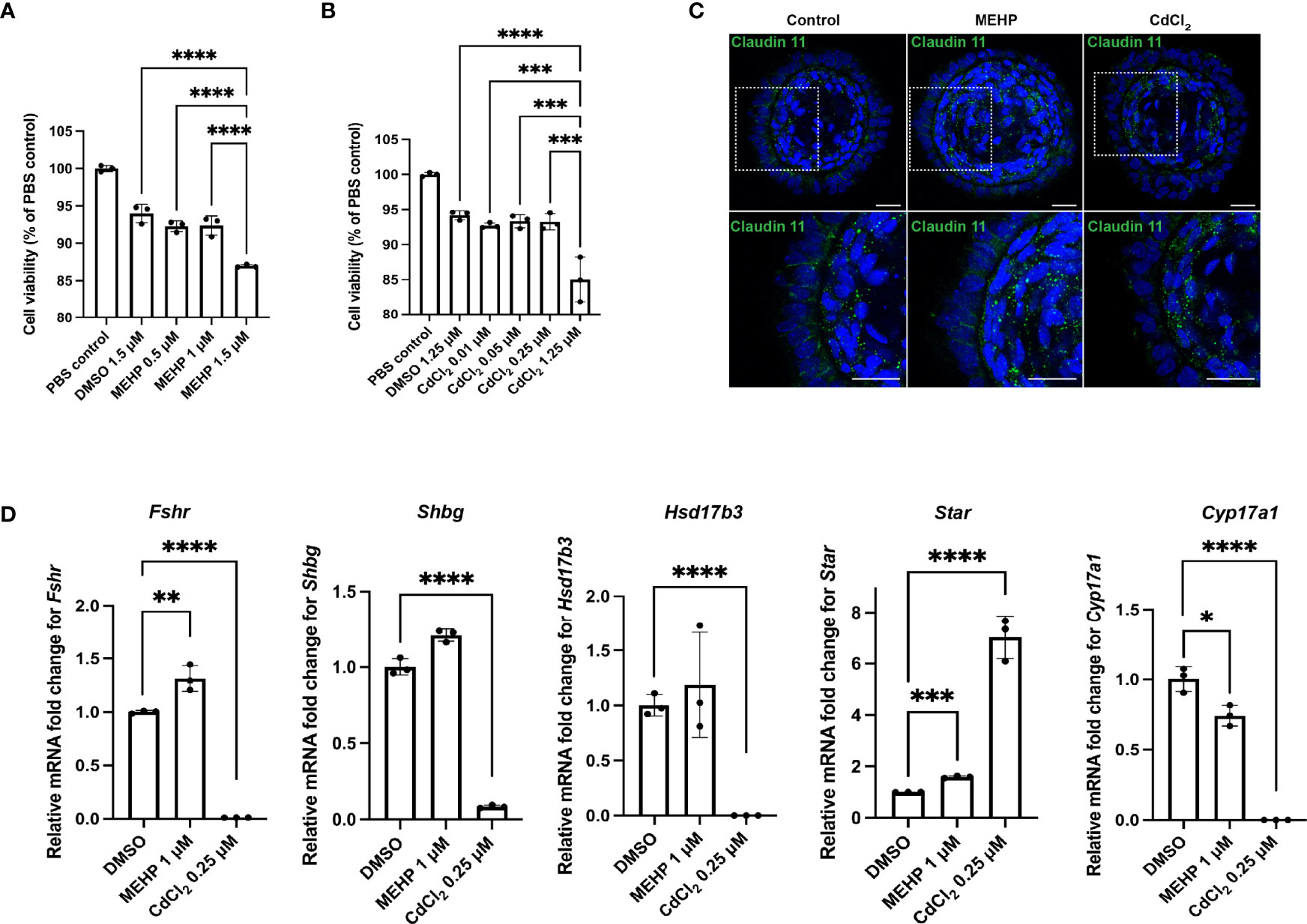
Figure 3 Rat testicular organoids allow for modeling reproductive toxicity. (A, B) Relative cell viability of organoids after MEHP and CdCl2 treatments. (C) Immunofluorescent images of day 21 rat testicular organoids treated with MEHP and CdCl2 showing tight junction protein (Claudin 11) (inserts on the top panels are showing the magnified area on the bottom panel). Scale bars measure 25 μm. (D) Relative mRNA fold change of Fshr, Shbg, Star, Hsd17b3 and Cyp17a1 in day 21 organoids after MEHP and CdCl2 treatment. Bars indicate mean ± SD, n = 3. Analysis was performed using one-way ANOVA followed by Tukey’s multiple comparison test. p ≤ 0.05 (*), p ≤ 0.01 (**), p ≤ 0.001 (***), p ≤ 0.0001 (****). Only significant differences are indicated with asterisks.
Discussion
In the last few years, we and others have reported the generation of testicular organoids from dissociated primary testicular cells (11, 14–18). In the current study, we adapted our previously established approach to generate porcine, murine, human and primate testicular organoids (11) to the formation of organotypic rat testicular organoids. Rats represent an important animal model for studying spermatogenesis and have been the main model for the study of reproductive toxicology (19–22). It was therefore necessary to establish the optimal conditions required for the generation and maturation of microwell-derived rat testicular organoids to support spermatogonial differentiation.
Rat testicular organoids presented some unique challenges that were not observed in our previously reported organoids (11). While porcine and murine organoids can be maintained for up to 45 days at ambient O2 tension, which translates to 18.4% or 6.34 mM O2 at 1084 m elevation in Calgary, Alberta, Canada (51, 52), rat organoids collapsed at day 6 by purging the interstitial compartment. This is likely due to the perturbation of the ratio of Sertoli cells to interstitial cells, caused by increased Sertoli cell proliferation. Sertoli cell proliferation levels are higher at high O2 tension compared to more hypoxic conditions (15%, 10%, 5% O2) (53). Unlike porcine and murine testicular organoids, where organoid formation leads to a contact inhibition effect on the cells, the Sertoli cells of rat testicular organoids seem to retain their ability to proliferate, which is exacerbated at ambient O2 tension. This increased number of Sertoli cells likely leads to a loss of their affinity for the basement membrane of the organoids. As a result, the Sertoli cells migrate to form separate aggregates which leads to complete or partial expulsion of the interstitial compartment.
Culture at 37°C can impair the glucose transport of spermatids and render spermatids and spermatozoa fragile (42, 54). It can also have deleterious effects on testis tissue in vitro (55). We observed higher expression of Shbg, increased numbers of early meiotic cells and the presence of cells with elongated SYCP3 staining pattern in 34°C culture conditions, indicating a positive effect on Sertoli cell maturation and spermatogonial differentiation, which is consistent with previous work (22, 45, 50, 54). As expected, number of germ cells were similar at both temperatures. This is consistent with previous reports which have found that the proliferation and survival of spermatogonia do not seem to be affected by temperature (54). However, the number of germ cells decreased over the duration of culture and zygotene spermatocytes, identified by the typical elongated staining pattern of SYCP3, were no longer adhered to the organoids. This gradual loss or dislodgement of loosely adhered germ cells is likely due to extensive media changes throughout the 28-day long culture. Such loss of a critical cell type may be mitigated by adapting the microwell system to support a continuous perfusion system (56). This would allow a slow and constant perfusion of media and reduce extensive handling for long-term cultures.
After establishing the optimal conditions for promoting maturation and early spermatogonial differentiation, we performed a proof of principle experiment to evaluate the utility of rat organoids for toxicological evaluation of drugs and environmental toxicants. Exposure to MEHP, a fairly common plasticizer (57), led to increased expression of Fshr and Star and decreased expression of Cyp17a1. This is consistent with previous reports, which have shown that phthalates can modulate basal steroidogenic machinery in both Sertoli and granulosa cells (58–60). Cadmium, a heavy metal that is often used as stabilizer in production of polymers and dyes, can cause endocrine disruption in the testis (61). We observed cadmium mediated disruption of Sertoli and Leydig cell function by downregulation of Fshr, Shbg, Hsd17b3 and Cyp17a1, and upregulation of Star, which has been reported previously (61, 62). Both MEHP and CdCl2 are known to disrupt the blood testes barrier by downregulation of tight-junction proteins such as Claudin 11 (63, 64). We witnessed a similar effect upon exposure of organoids to MEHP and CdCl2. Human exposure to CdCl2 and MEHP can depend on a number of factors such as cumulative effects, metabolism by the liver, accumulation due to continuous environmental and occupational exposure (65, 66). While all of these considerations are beyond the scope of this current study, the dosages used here show definite disruptive effects on the steroidogenic machinery. This proof of principle experiment shows that the rat testicular organoids can serve as viable platforms for modeling male reproductive toxicity.
In conclusion, we report a rat testicular organoid system that reflect testis specific morphology and can support early testicular maturation. In addition, this system supports germ cell development to early meiosis up to the zygotene stage. Further optimization of the differentiation conditions may be warranted to support full in vitro spermatogenesis.
Data Availability Statement
The original contributions presented in the study are included in the article/Supplementary Materials. Further inquiries can be directed to the corresponding author.
Ethics Statement
The animal study was reviewed and approved by University of Calgary Animal Care Committee.
Author Contributions
SS and ID conceived and designed the study. SS, NLML, and BH performed the data analysis, data interpretation and statistical analysis. SS prepared the manuscript. All authors contributed to the article and approved the submitted version.
Funding
This work was supported by NIH/NICHD HD091068-01 to ID; a graduate student scholarship to SS from the Alberta Children’s Hospital Research Institute (ACHRI); and a Canadian Institutes of Health Research (CIHR) fellowship (MFE-176542) and an ACHRI postdoctoral fellowship to NLML.
Conflict of Interest
The authors declare that the research was conducted in the absence of any commercial or financial relationships that could be construed as a potential conflict of interest.
Publisher’s Note
All claims expressed in this article are solely those of the authors and do not necessarily represent those of their affiliated organizations, or those of the publisher, the editors and the reviewers. Any product that may be evaluated in this article, or claim that may be made by its manufacturer, is not guaranteed or endorsed by the publisher.
Acknowledgments
We thank Dr. Mark Ungrin and his laboratory for their guidance and support.
Supplementary Material
The Supplementary Material for this article can be found online at: https://www.frontiersin.org/articles/10.3389/fendo.2022.892342/full#supplementary-material
References
1. Kumar N, Singh AK. Trends of Male Factor Infertility, an Important Cause of Infertility: A Review of Literature. J Hum Reprod Sci (2015) 8:191–6. doi: 10.4103/0974-1208.170370
2. Barratt CLR, Björndahl L, De Jonge CJ, Lamb DJ, Osorio Martini F, McLachlan R, et al. The Diagnosis of Male Infertility: An Analysis of the Evidence to Support the Development of Global WHO Guidance-Challenges and Future Research Opportunities. Hum Reprod Update (2017) 23:660–80. doi: 10.1093/humupd/dmx021
3. Cheng CY. Toxicants Target Cell Junctions in the Testis: Insights From the Indazole-Carboxylic Acid Model. Spermatogenesis (2014) 4:e981485. doi: 10.4161/21565562.2014.981485
4. Sharpe RM. Environmental/lifestyle Effects on Spermatogenesis. Philos Trans R Soc London Ser B Biol Sci (2010) 365:1697–712. doi: 10.1098/rstb.2009.0206
5. Siu ER, Mruk DD, Porto CS, Cheng CY. Cadmium-Induced Testicular Injury. Toxicol Appl Pharmacol (2009) 238:240–9. doi: 10.1016/j.taap.2009.01.028
6. Oatley JM, Brinster RL. The Germline Stem Cell Niche Unit in Mammalian Testes. Physiol Rev (2012) 92:577–95. doi: 10.1152/physrev.00025.2011
7. Huhtaniemi I, Toppari J. Endocrine, Paracrine and Autocrine Regulation of Testicular Steroidogenesis. Adv Exp Med Biol (1995) 377:33–54. doi: 10.1007/978-1-4899-0952-7_3
8. Hess RA, Renato de Franca L. Spermatogenesis and Cycle of the Seminiferous Epithelium. Adv Exp Med Biol (2008) 636:1–15. doi 10.1007/978-0-387-09597-4_1
9. Sousa M, Cremades N, Alves C, Silva J, Barros A. Developmental Potential of Human Spermatogenic Cells Co-Cultured With Sertoli Cells. Hum Reprod (2002) 17:161–72. doi: 10.1093/humrep/17.1.161
11. Sakib S, Uchida A, Valenzuela-Leon P, Yu Y, Valli-Pulaski H, Orwig K, et al. Formation of Organotypic Testicular Organoids in Microwell Culture†. Biol Reprod (2019) 100:1648–60. doi: 10.1093/biolre/ioz053
12. Staub C. A Century of Research on Mammalian Male Germ Cell Meiotic Differentiation In Vitro. J Androl (2001) 22:911–26. doi: 10.1002/j.1939-4640.2001.tb03430.x
13. Richer G, Baert Y. In-Vitro Spermatogenesis Through Testis Modelling: Toward the Generation of Testicular Organoids. Andrology (2020) 8:879–91. doi: 10.1111/andr.12741
14. Baert Y, Dvorakova-Hortova K, Margaryan H, Goossens E. Mouse In Vitro Spermatogenesis on Alginate-Based 3d Bioprinted Scaffolds. Biofabrication (2019) 11:035011. doi: 10.1088/1758-5090/ab1452
15. Alves-Lopes JP, Soder O, Stukenborg JB. Testicular Organoid Generation by a Novel In Vitro Three-Layer Gradient System. Biomaterials (2017) 130:76–89. doi: 10.1016/j.biomaterials.2017.03.025
16. Pendergraft SS, Sadri-Ardekani H, Atala A, Bishop CE. Three-Dimensional Testicular Organoid: A Novel Tool for the Study of Human Spermatogenesis and Gonadotoxicity In Vitro. Biol Reprod (2017) 96:720–32. doi: 10.1095/biolreprod.116.143446
17. Edmonds ME, Woodruff TK. Testicular Organoid Formation is a Property of Immature Somatic Cells, Which Self-Assemble and Exhibit Long-Term Hormone-Responsive Endocrine Function. Biofabrication (2020) 12:045002. doi: 10.1088/1758-5090/ab9907
18. Strange DP, Jiyarom B, Pourhabibi Zarandi N, Xie X, Baker C, Sadri-Ardekani H, et al. Axl Promotes Zika Virus Entry and Modulates the Antiviral State of Human Sertoli Cells. mBio (2019) 10:e01372-19. doi: 10.1128/mBio.01372-19
19. Hashway SA, Wilding LA. Translational Potential of Rats in Research. In: The Laboratory Rat. Elsevier (2020).
20. Miyake K, Yamamoto M, Mitsuya H. Pharmacological and Histological Evidence for Adrenergic Innervation of the Myoid Cells in the Rat Seminiferous Tubule. Tohoku J Exp Med (1986) 149:79–87. doi: 10.1620/tjem.149.79
21. Russell LD, Ettlin RA, Hikim APS, Clegg ED. Histological and Histopathological Evaluation of the Testis. In: Wiley Online Library (1993).
22. Matsumura T, Sato T, Abe T, Sanjo H, Katagiri K, Kimura H, et al. Rat In Vitro Spermatogenesis Promoted by Chemical Supplementations and Oxygen-Tension Control. Sci Rep (2021) 11:3458. doi: 10.1038/s41598-021-82792-2
23. Sato T, Katagiri K, Gohbara A, Inoue K, Ogonuki N, Ogura A, et al. In Vitro Production of Functional Sperm in Cultured Neonatal Mouse Testes. Nature (2011) 471:504–7. doi: 10.1038/nature09850
24. Uchida A, Sakib S, Labit E, Abbasi S, Scott RW, Underhill TM, et al. Development and Function of Smooth Muscle Cells is Modulated by Hic1 in Mouse Testis. Development (2020) 147:dev185884. doi: 10.1242/dev.185884
25. Mahadevaiah SK, Turner JM, Baudat F, Rogakou EP, de Boer P, Blanco-Rodríguez J, et al. Recombinational DNA Double-Strand Breaks in Mice Precede Synapsis. Nat Genet (2001) 27:271–6. doi: 10.1038/85830
26. Urriola-Muñoz P, Lagos-Cabré R, Moreno RD. A Mechanism of Male Germ Cell Apoptosis Induced by Bisphenol-A and Nonylphenol Involving ADAM17 and P38 MAPK Activation. PloS One (2014) 9:e113793–e113793. doi: 10.1371/journal.pone.0113793
27. Isotani A, Yamagata K, Okabe M, Ikawa M. Generation of Hprt-Disrupted Rat Through Mouse←Rat ES Chimeras. Sci Rep (2016) 6:24215. doi: 10.1038/srep24215
28. Di Lorenzo M, Winge SB, Svingen T, De Falco M, Boberg J. Intrauterine Exposure to Diethylhexyl Phthalate Disrupts Gap Junctions in the Fetal Rat Testis. Curr Res Toxicol (2020) 1:5–11. doi: 10.1016/j.crtox.2020.02.002
29. Wu Y, Johnson G, Zhao F, Wu Y, Zhao G, Brown A, et al. Features of Lipid Metabolism in Humanized ApoE Knockin Rat Models. Int J Mol Sci (2021) 22:8262. doi: 10.3390/ijms22158262
30. Kanazawa Y, Nagano M, Koinuma S, Sugiyo S, Shigeyoshi Y. Effects of Endurance Exercise on Basement Membrane in the Soleus Muscle of Aged Rats. Acta histochem cytochemica (2021) 54:167–75. doi: 10.1267/ahc.21-00057
31. Wu L, Zhang K, Sun L, Bai J, Zhang M, Zheng J. Laminin Degradation by Matrix Metalloproteinase 9 Promotes Ketamine-Induced Neuronal Apoptosis in the Early Developing Rat Retina. CNS Neurosci Ther (2020) 26:1058–68. doi: 10.1111/cns.13428
32. Li Y, Liu J, Liao G, Zhang J, Chen Y, Li L, et al. Early Intervention With Mesenchymal Stem Cells Prevents Nephropathy in Diabetic Rats by Ameliorating the Inflammatory Microenvironment. Int J Mol Med (2018) 41:2629–39. doi: 10.3892/ijmm.2018.3501
33. McCabe MJ, Tarulli GA, Meachem SJ, Robertson DM, Smooker PM, Stanton PG. Gonadotropins Regulate Rat Testicular Tight Junctions In Vivo. Endocrinology (2010) 151:2911–22. doi: 10.1210/en.2009-1278
34. Morohoshi A, Nakagawa T, Nakano S, Nagasawa Y, Nakayama K. The Ubiquitin Ligase Subunit β-TrCP in Sertoli Cells is Essential for Spermatogenesis in Mice. Dev Biol (2019) 445:178–88. doi: 10.1016/j.ydbio.2018.10.023
35. Liu J, Li X, Zhou G, Zhang Y, Sang Y, Wang J, et al. Silica Nanoparticles Inhibiting the Differentiation of Round Spermatid and Chromatin Remodeling of Haploid Period via MIWI in Mice. Environ Pollut (2021) 284:117446. doi: 10.1016/j.envpol.2021.117446
36. Bagdadi N, Sawaied A, AbuMadighem A, Lunenfeld E, Huleihel M. The Expression Levels and Cellular Localization of Pigment Epithelium Derived Factor (PEDF) in Mouse Testis: Its Possible Involvement in the Differentiation of Spermatogonial Cells. Int J Mol Sci (2021) 22:1–13. doi: 10.3390/ijms22031147
37. Hoffman JR, Zuckerman A, Ram O, Sadot O, Cohen H. Changes in Hippocampal Androgen Receptor Density and Behavior in Sprague-Dawley Male Rats Exposed to a Low-Pressure Blast Wave. Brain plast (Amsterdam Netherlands) (2020) 5:135–45. doi: 10.3233/BPL-200107
38. Chen S-R, Tang J-X, Cheng J-M, Li J, Jin C, Li X-Y, et al. Loss of Gata4 in Sertoli Cells Impairs the Spermatogonial Stem Cell Niche and Causes Germ Cell Exhaustion by Attenuating Chemokine Signaling. Oncotarget (2015) 6:37012–27. doi: 10.18632/oncotarget.6115
39. Kim JY, Jung HJ, Yoon MJ. VASA (DDX4) Is a Putative Marker for Spermatogonia, Spermatocytes and Round Spermatids in Stallions. Reprod Domest Anim (2015) 50:1032–8. doi: 10.1111/rda.12632
40. Teerds KJ, Rijntjes E, Veldhuizen-Tsoerkan MB, Rommerts FF, de Boer-Brouwer M. The Development of Rat Leydig Cell Progenitors In Vitro: How Essential is Luteinising Hormone? J Endocrinol (2007) 194:579–93. doi: 10.1677/JOE-06-0084
41. Tung PS, Fritz IB. Characterization of Rat Testicular Peritubular Myoid Cells in Culture: Alpha-Smooth Muscle Isoactin Is a Specific Differentiation Marker. Biol Reprod (1990) 42:351–65. doi: 10.1095/biolreprod42.2.351
42. Hall PF, Nakamura M. Influence of Temperature on Hexose Transport by Round Spermatids of Rats. J Reprod Fertil (1981) 63:373–9. doi: 10.1530/jrf.0.0630373
43. Mazaud-Guittot S, Meugnier E, Pesenti S, Wu X, Vidal H, Gow A, et al. Claudin 11 Deficiency in Mice Results in Loss of the Sertoli Cell Epithelial Phenotype in the Testis1. Biol Reprod (2010) 82:202–13. doi: 10.1095/biolreprod.109.078907
44. Gregory CW, DePhilip RM. Detection of Steroidogenic Acute Regulatory Protein (stAR) in Mitochondria of Cultured Rat Sertoli Cells Incubated With Follicle-Stimulating Hormone. Biol Reprod (1998) 58:470–4. doi: 10.1095/biolreprod58.2.470
45. Gassei K, Ehmcke J, Wood MA, Walker WH, Schlatt S. Immature Rat Seminiferous Tubules Reconstructed In Vitro Express Markers of Sertoli Cell Maturation After Xenografting Into Nude Mouse Hosts. Mol Hum Reprod (2010) 16:97–110. doi: 10.1093/molehr/gap081
46. Rebourcet D, Mackay R, Darbey A, Curley MK, Jørgensen A, Frederiksen H, et al. Ablation of the Canonical Testosterone Production Pathway via Knockout of the Steroidogenic Enzyme HSD17B3, Reveals a Novel Mechanism of Testicular Testosterone Production. FASEB J (2020) 34:10373–86. doi: 10.1096/fj.202000361R
47. Shima Y, Miyabayashi K, Haraguchi S, Arakawa T, Otake H, Baba T, et al. Contribution of Leydig and Sertoli Cells to Testosterone Production in Mouse Fetal Testes. Mol Endocrinol (2013) 27:63–73. doi: 10.1210/me.2012-1256
48. Yuan L, Liu JG, Zhao J, Brundell E, Daneholt B, Höög C. The Murine SCP3 Gene is Required for Synaptonemal Complex Assembly, Chromosome Synapsis, and Male Fertility. Mol Cell (2000) 5:73–83. doi: 10.1016/S1097-2765(00)80404-9
49. Eddy EM. Role of Heat Shock Protein HSP70-2 in Spermatogenesis. Rev Reprod (1999) 4:23–30. doi: 10.1530/ror.0.0040023
50. Medrano JV, Simon C, Pera RR. Human Germ Cell Differentiation From Pluripotent Embryonic Stem Cells and Induced Pluripotent Stem Cells. Methods Mol Biol (2014) 1154:563–78. doi: 10.1007/978-1-4939-0659-8_27
51. Sakib S, Voigt A, de Lima e Martins Lara N, Su L, Ungrin M, Rancourt D, et al. The Proliferation of Pre-Pubertal Porcine Spermatogonia in Stirred Suspension Bioreactors Is Partially Mediated by the Wnt/β-Catenin Pathway. Int J Mol Sci (2021) 22:13549. doi: 10.3390/ijms222413549
52. Al-Ani A, Toms D, Kondro D, Thundathil J, Yu Y. Oxygenation in Cell Culture: Critical Parameters for Reproducibility are Routinely Not Reported. Plos One (2018) 13:. doi: 10.1371/journal.pone.0204269
53. Hao WY, Shao CH, Feng YL, Hu JT, Li Q, Wang HQ, et al. Hypoxia Reduces the Proliferation and Occludin Expression of Primary Sertoli Cells. Zhonghua Nan Ke Xue (2013) 19:29–34.
54. Nakamura M, Namiki M, Okuyama A, Matsui T, Doi Y, Takeyama M, et al. Temperature Sensitivity of Human Spermatogonia and Spermatocytes In Vitro. Arch Androl (1987) 19:127–32. doi: 10.3109/01485018708986808
55. Medrano JV, Vilanova-Pérez T, Fornés-Ferrer V, Navarro-Gomezlechon A, Martínez-Triguero ML, García S, et al. Influence of Temperature, Serum, and Gonadotropin Supplementation in Short- and Long-Term Organotypic Culture of Human Immature Testicular Tissue. Fertil Steril (2018) 110:1045–1057.e3. doi: 10.1016/j.fertnstert.2018.07.018
56. Komeya M, Hayashi K, Nakamura H, Yamanaka H, Sanjo H, Kojima K, et al. Pumpless Microfluidic System Driven by Hydrostatic Pressure Induces and Maintains Mouse Spermatogenesis In Vitro. Sci Rep (2017) 7:15459. doi: 10.1038/s41598-017-15799-3
57. Howdeshell KL, Rider CV, Wilson VS, Gray LE Jr. Mechanisms of Action of Phthalate Esters, Individually and in Combination, to Induce Abnormal Reproductive Development in Male Laboratory Rats. Environ Res (2008) 108:168–76. doi: 10.1016/j.envres.2008.08.009
58. Svechnikov K, Svechnikova I, Soder O. Gender-Specific Adverse Effects of Mono-Ethylhexyl Phthalate on Steroidogenesis in Immature Granulosa Cells and Rat Leydig Cell Progenitors In Vitro. Front Endocrinol (2011) 2. doi: 10.3389/fendo.2011.00009
59. Li N, Liu T, Guo K, Zhu J, Yu G, Wang S, et al. Effect of Mono-(2-Ethylhexyl) Phthalate (MEHP) on Proliferation of and Steroid Hormone Synthesis in Rat Ovarian Granulosa Cells In Vitro. J Cell Physiol (2018) 233:3629–37. doi: 10.1002/jcp.26224
60. Chauvigné F, Plummer S, Lesné L, Cravedi JP, Dejucq-Rainsford N, Fostier A, et al. Mono-(2-Ethylhexyl) Phthalate Directly Alters the Expression of Leydig Cell Genes and CYP17 Lyase Activity in Cultured Rat Fetal Testis. PloS One (2011) 6:e27172. doi: 10.1371/journal.pone.0027172
61. Wu X, Guo X, Wang H, Zhou S, Li L, Chen X, et al. A Brief Exposure to Cadmium Impairs Leydig Cell Regeneration in the Adult Rat Testis. Sci Rep (2017) 7:6337–7. doi: 10.1038/s41598-017-06870-0
62. Park SY, Gomes C, Oh SD, Soh J. Cadmium Up-Regulates Transcription of the Steroidogenic Acute Regulatory Protein (StAR) Gene Through Phosphorylated CREB Rather Than SF-1 in K28 Cells. J Toxicol Sci (2015) 40:151–61. doi: 10.2131/jts.40.151
63. Chiba K, Kondo Y, Yamaguchi K, Miyake H, Fujisawa M. Inhibition of Claudin-11 and Occludin Expression in Rat Sertoli Cells by Mono-(2-Ethylhexyl) Phthalate Through P44/42 Mitogen-Activated Protein Kinase Pathway. J Andrology (2012) 33:368–74. doi: 10.2164/jandrol.111.013664
64. Yang Q, Hao J, Chen M, Li G. Dermatopontin Is a Novel Regulator of the CdCl2-Induced Decrease in Claudin-11 Expression. Toxicol Vitro (2014) 28:1158–64. doi: 10.1016/j.tiv.2014.05.013
65. Talsness CE, Andrade AJM, Kuriyama SN, Taylor JA, vom Saal FS. Components of Plastic: Experimental Studies in Animals and Relevance for Human Health. Philos Trans R Soc London Ser B Biol Sci (2009) 364:2079–96. doi: 10.1098/rstb.2008.0281
Keywords: testicular organoid, spermatogonia, MEHP, cadmium chloride, Sertoli cell
Citation: Sakib S, Lara NLM, Huynh BC and Dobrinski I (2022) Organotypic Rat Testicular Organoids for the Study of Testicular Maturation and Toxicology. Front. Endocrinol. 13:892342. doi: 10.3389/fendo.2022.892342
Received: 08 March 2022; Accepted: 02 May 2022;
Published: 09 June 2022.
Edited by:
Barry Zirkin, Johns Hopkins University, United StatesReviewed by:
Marie-Claude Hofmann, University of Texas MD Anderson Cancer Center, United StatesMartine Culty, University of Southern California, Los Angeles, United States
Copyright © 2022 Sakib, Lara, Huynh and Dobrinski. This is an open-access article distributed under the terms of the Creative Commons Attribution License (CC BY). The use, distribution or reproduction in other forums is permitted, provided the original author(s) and the copyright owner(s) are credited and that the original publication in this journal is cited, in accordance with accepted academic practice. No use, distribution or reproduction is permitted which does not comply with these terms.
*Correspondence: Ina Dobrinski, aWRvYnJpbnNAdWNhbGdhcnkuY2E=