- 1Instituto Tecnológico Chascomús (INTECH), CONICET-EByNT-UNSAM, Chascomús, Argentina
- 2Centro Oceanográfico de Vigo, Instituto Español de Oceanografía - Consejo Superior de Investigaciones Científicas (IEO-CSIC), Vigo, Spain
Fish body growth is a trait of major importance for individual survival and reproduction. It has implications in population, ecology, and evolution. Somatic growth is controlled by the GH/IGF endocrine axis and is influenced by nutrition, feeding, and reproductive-regulating hormones as well as abiotic factors such as temperature, oxygen levels, and salinity. Global climate change and anthropogenic pollutants will modify environmental conditions affecting directly or indirectly fish growth performance. In the present review, we offer an overview of somatic growth and its interplay with the feeding regulatory axis and summarize the effects of global warming and the main anthropogenic pollutants on these endocrine axes.
1 Introduction
Fish growth rate is an important trait with ecological, evolutionary, and conservation implications (1, 2). Anthropogenic stressors such as global climate change, which imply temperature rise, salinity change, and ocean acidification; ecosystem disturbance; and selective pressure from capture have shown a rapid effect on the growth rate of several fish species (2–10). During the last century, the mean global temperatures have risen approximately 1.1°C concurrently with increasing anthropogenic greenhouse gas emissions (11). Temperature is one of the main abiotic factors affecting body size in fish (12–14). It has been described that increasing temperature induces a reduction in body size of ectotherm organisms, such as fish (8, 15–18). Beyond the passionate discussion about the underlying mechanism to explain this fact (19, 20), it is forecasted that body size will be reduced in any scenario of global warming. Reduction of body size might have important deleterious effects not only in the reduction of species fitness but also perturbation of energy and nutrient web, ecosystem, and economic losses (1, 2, 7, 21, 22). In this regard, it would be necessary to understand the effects of anthropogenic stressors on somatic growth endocrine regulation.
Body growth is promoted by growth hormone–insulin‐like growth factors, the so-called GH/IGF axis or somatic growth axis (23–27). This endocrine axis is integrated and influenced by reproduction, feeding, and energy balance (23, 25, 28–30). Therefore, fish growth is affected by a wide range of biotic and abiotic factors, such as temperature (12, 13), nutritional state and energy metabolism (30–32), intra- and interspecies interactions (33, 34), and sex and reproduction (35–37). Thus, pollutants could interfere with this hormonal assembly that impacts the growth rate and performance of fish, potentially affecting their populations and the entire ecosystem (Figure 1).
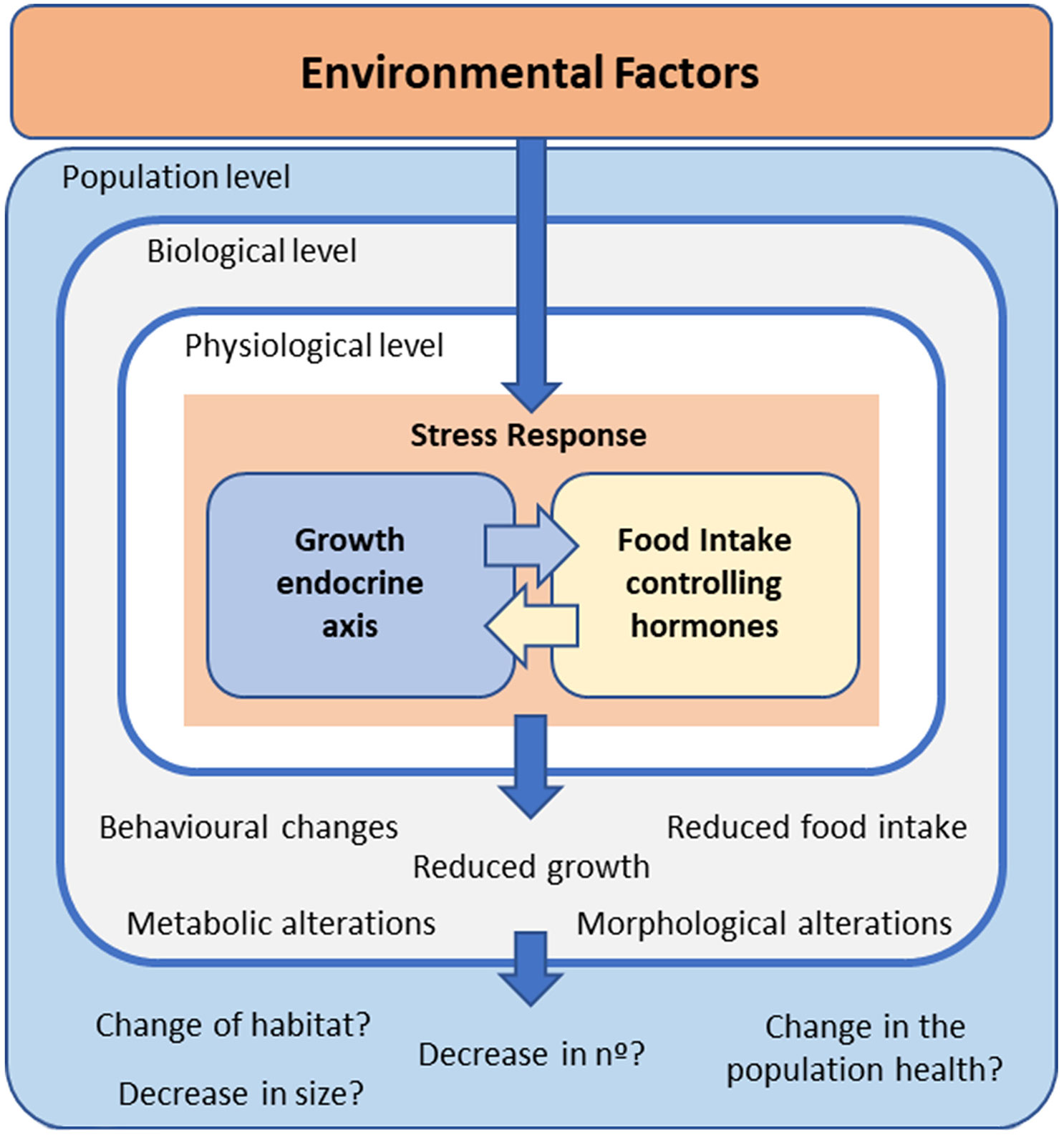
Figure 1 Schematic representation of how environmental factors activate a stress response in fish, which includes modifications in the interrelated growth – and food intake –endocrine axes. The possible consequences at different levels of biological organization are also mentioned.
The main goal of this review is to provide an overview and update on the effects of several abiotic anthropogenic factors on GH and food intake regulation. By summarizing the current knowledge on this topic, this review aims to serve as a resource for those involved in research related to understanding the effects of global warming and pollutants on individuals and population growth and the energy web.
2 Interrelationship between growth and food intake in fish
The endocrine and neuroendocrine regulation of somatic growth in teleost fish has been extensively reviewed (23, 24, 26–29, 38–40). The main factors regulating growth are growth hormone (GH), the insulin-like growth factors (IGFs), and their respective receptors (GHRs and IGFRs). Systemic IGFs, derived mainly from liver sources, in turn, act in a negative feedback fashion to suppress GH. GH belongs to the GH/prolactin (PRL)/somatolactin (SL) family (23, 39, 41). GH, produced in and released by the pituitary gland, is largely responsible for endocrine growth promotion during postnatal growth. Once in the circulation, according to the classical somatomedin hypothesis (42–44), the main endocrine action of GH is stimulating hepatic IGF production, which is the primary inducer of cell proliferation and differentiation (45, 46). IGFs act on target tissues in either an autocrine, paracrine, or endocrine manner (43, 47–49) stimulating many biological processes, including protein synthesis and turnover and cell proliferation and differentiation, among others (44, 50).
Furthermore, GH acts directly on different target tissues including the muscle and adipose tissue, to regulate physiological processes associated with protein synthesis and metabolism (40). GH also induces the local production of IGFs to regulate, in a paracrine way, cell proliferation and tissue growth, as shown in mammals (51). In addition to growth promotion, GH is a pleiotropic hormone also involved in several important processes such as nutrition, metabolism, reproduction, neuroprotection, immunity, osmoregulation, and social behavior (23, 28, 39, 40, 45, 49, 52–55). Consequently, the neuroendocrine regulation of GH is influenced by several peptides and neurotransmitters also involved in the regulation of either food intake or reproduction [for review, see (23, 28, 29, 53)], suggesting a close integration of different endocrine axes (Table 1).
The regulation of feeding behavior is based in the ventral tuberal hypothalamus where several brain nuclei produce either feeding-stimulating (orexigenic) peptides, particularly, agouti-related protein (AgRP) and neuropeptide Y (NPY), or feeding-inhibiting (anorexigenic) peptides, mainly melanocortin- stimulating hormone (aMSH), a pro-opiomelanocortin (POMC) derived peptide, and cocaine and amphetamine- related transcript (CART), which act in a coordinated manner to maintain food intake levels according to the requirements. As has been extensively studied in mammalian models, the primary hypothalamic nuclei involved in the regulation of food intake are the arcuate and the paraventricular nuclei (108). Teleost fish present the same neuropeptide control of food intake as mammals and have equivalent brain areas involved in it [for review, see (28, 29, 74, 83, 103, 108)].
The neuroendocrine control of the GH/IGF axis includes several stimulatory and inhibitory factors [for review, see (28, 29)]. The main stimulatory factors are the growth hormone-releasing hormone (GHRH) (109, 110) and pituitary adenyl cyclase-activating polypeptide (PACAP) (109, 111–114). In addition, other hypothalamic or gastrointestinal peptides and catecholamines also stimulate GH secretion. Among them that are worthy to mention are gonadotropin-releasing hormone (GnRH) (115–123), neuropeptide Y (NPY) (104–106), corticotropin-releasing hormone (CRH) (124), thyrotropin-releasing hormone (TRH) (125–127), ghrelin (84–87, 128), cholecystokinin (CCK) (84, 99, 100), gastrin-releasing peptide (GRP) (84, 99, 100, 107), nesfatin-1 (90, 129, 130), and dopamine (DA) (123, 131–135).
On the other hand, somatostatin (SS) is the main hypothalamic factor that inhibits both basal and stimulated GH secretion (112, 120, 123, 124, 136–141). In addition, norepinephrine inhibits GH secretion as well (142).
Several of the abovementioned neuropeptides regulating GH secretion are also involved in the control of feeding and energy balance (Table 1). For example, neuropeptides such as NPY, PACAP, SS, GnRH, TRH, and CRH and gastrointestinal peptides like CCK, ghrelin, GRP, and nesfatin-1, among others, have a role in feeding and energy balance regulation [for review, see (28, 29, 83, 88, 89, 103, 143, 144)].
This intricate regulatory network with many points of contact suggests a strong interrelationship between these two physiological processes. Furthermore, the GH itself, IGFs, and their regulatory network are involved in the regulation of feeding, energy balance, and metabolism [for review, see (53, 74)].
3 External regulation of endocrine factors controlling growth and food intake in fish
As mentioned earlier, the somatotropic axis is highly coordinated with the regulation of food intake as well as the reproductive endocrine axis. Thus, the somatotropic axis involves a great number of controlling factors briefly summarized in the previous sections. Therefore, the somatic endocrine axis would be highly informative in a wide range of growth-disturbing and stressful stimuli, and its study would represent a tool for assessing nutritional and environmental limitations (39). In this context, we will present the available information about abiotic factors affecting growth and the somatic endocrine axis.
3.1 Photoperiod
Photoperiod has been shown to alter somatic growth as well as the synthesis, secretion, and responsiveness of growth-related hormones (145–148). As a general plot, increasing light duration conducts to a higher growth rate and increased plasma IGF-1 levels (146, 149). Nonetheless, growth shows seasonal variation being increased during the summer and spring with non-parallel variations among GH and IGF serum levels and growth rates (149–155). For instance, a longer photoperiod results in higher GH levels or expression in Atlantic salmon (Salmo salar) (150, 152, 156), gilthead sea bream (Sparus aurata) (157), goldfish (Carassius auratus) (138), and coho salmon (Oncorhynchus kisutch) (158). Moreover, studies on Atlantic salmon suggest that the photoperiod may modify the effects of temperature on GH levels (159, 160). Thus, temperature and photoperiod should not be considered as independent controlling factors of fish endocrine growth axis (146). Furthermore, the individual effects of the two factors are difficult to dissect because fish are subjected to seasonal cycles and both photoperiod and temperature vary under natural conditions. Additionally, the relationship among GH, IGFs, and growth rates is affected by a wide range of endogenous and exogenous factors, such as gender, developmental and maturity state, nutritional state, temperature, salinity, and stress, in addition to photoperiod (39, 161, 162). Moreover, several of these factors, particularly those related to reproduction and feeding, are also affected by photoperiod and temperature.
3.2 Temperature
Fish, as aquatic ectotherms, must be able to contend with continuous fluctuations in water temperature, which, in turn, influences gene expression and the activity of metabolic enzymes (163–167). In fact, environmental temperature is one of the most important ecological factors, which influences the behavior and physiological processes of aquatic animals (145, 168).
Seasonal variations of somatic growth as well as in GH levels are associated with water temperature changes. Thus, it has been shown that pituitary GH content or plasma GH levels and also IGF-1 levels were increased during spring and summer in several teleost species such as perch (Perca fluviatilis) (169), brown bullhead (Ictalurus nebulosus) (170), coho salmon (171), goldfish (138), rainbow trout (Oncorhynchus mykiss) (172), gilthead sea bream (157, 173), chinook salmon (Oncorhynchus tshawytscha) (174), and common carp (Cyprinus carpio) (175). Consequently, it is likely that water temperature regulates the plasma levels of GH and IGF-1.
Although GH has been detected at the early stages of embryo development and post-hatching (176–178), it seems that water temperature does not affect GH cell differentiation or GH expression (179, 180) at these early stages of life. Even so, the expression levels of IGF-2 and IGF-1 receptors were upregulated when incubated in elevated temperatures in correlation with the enhanced embryonic growth rate found in this condition (181). However, at hatching, the expression levels of IGF-1 and IGF-2 were higher at the lowest temperature (4°C) (179, 181). These findings indicate that embryonic growth is GH independent and there is a shift in growth regulation and temperature affects it upon hatching.
The effect of temperature, independent of photoperiod, on GH serum levels can be experimentally addressed. For example, when Nile tilapia, Oreochromis niloticus, was maintained in increased temperature, higher circulating GH levels were observed (182). Rainbow trout reared at increasing temperatures shows that growth rate correlates with temperature (183). In this experiment, higher temperatures increased IGF-1 plasma levels and hepatic IGF-1 mRNA levels (183) stimulated by GH. Increasing water temperature during winter (short photoperiod) stimulates growth rates and increases plasma GH levels in sea bream. Conversely, decreasing water temperature during summer (long photoperiod) decreases growth rates and circulating GH levels (138, 157). Thus, it seems that temperature, independent of photoperiod, induces higher GH and IGF secretion, which positively correlates with increased growth rates (184).
However, the positive correlation between increased temperature and increased plasma GH could not translate into enhanced fish growth because of stress or undernutrition. For instance, Atlantic salmon reared at increasing temperatures shows a biphasic growth rate increasing from 4.6°C to 14.4°C and decreasing between 14.4°C and 18.9°C while reaching the highest amounts of plasma GH at 18.9°C (185). Moreover, increasing water temperature close to the maximum of the tolerable range elevates GH levels regardless of feed conditions (166, 183, 186). However, IGF-1 concomitantly increases only in ad libitum-fed fish, while fish-restricted feeding regimens showed reduced IGF-1 levels and reduced growth rates (183). It can be concluded that fish under ad libitum feeding regimen had an increase in GH which is translated into an increase in IGF-1 levels and somatic growth regardless of the rearing temperature, while fish under restricted feeding and high temperature exhibited a reduced GH receptors’ response, with reduced IGF-1 and growth rates and increased GH levels which may reflect a GH resistance (see discussion in Section 3.3 below). Thus, the positive effect of temperature on hepatic IGF-1 synthesis was avoided (166, 183). Therefore, nutritional condition interferes with the somatic growth endocrine axis. Additionally, higher temperatures increase feed consumption (149, 166), which indeed stimulated somatic growth (145).
3.3 Nutrition
Nutrient and diet composition strongly influence somatic growth in fish (28, 187). The synthesis and release of hepatic IGF-1 are largely affected by food restriction or deprivation as shown by studies in several salmonid species (166, 183, 188–191) as well as in gilthead sea bream (192, 193) and hybrid striped bass (Morone chrysops x Morone saxatilis) (194, 195). Given that GH stimulates lipolysis independently of IGFs and growth promotion, it plays an important role in energy mobilization in the context of undernutrition or nutrient deficiency. Thus, elevated circulating levels of GH along with low IGF-1 confer a metabolic advantage under this condition (39). Therefore, a state of GH resistance, a reduced hepatic responsiveness to the anabolic GH action, is highly conserved through the evolution of fishes and higher vertebrates (39). The GH resistance may be the result of reduced GH receptor expression or post-receptor defects in GH signaling (39). In this context, it was shown that the hepatic transcript of ghr-1 correlates with changes in growth rates, plasma IGF-1 level, and hepatic igf-1 expression (39, 196–198). In addition, deficiencies of nutrients as a consequence of unbalanced diets downregulate hepatic ghr-1 expression (197–199), indicating the prominent role of GHR-1 in the systemic growth-promoting action of GH.
It has been shown that, in teleost, nutrients like glucose, amino acids, and lipids influence hormones and peptides that regulate feeding such as nesfatin-1, ghrelin, CCK, and leptin, as well as the GH/IGF axis [for review, see (28)]. The way nutrients influence feeding growth and metabolism is not fully understood, but it would depend on nutrient- sensing mechanisms located in several tissues such as the gut, liver, pancreas, and hypothalamus (200–202). These nutrient- sensing mechanisms are capable of determining directly or indirectly the levels of nutrients and stored energy [for review, see (28, 103)]. Thus, nutrients in the luminal surface of the gastrointestinal tract (GIT) evoke changes in the expression and activity of digestive enzymes and the synthesis and secretion of GIT peptides and hormones such as CCK, GLP-1, GIP, PYY, secretin, ghrelin, or nesfatin-1 (28). Therefore, the GIT conveys information about the type and levels of nutrients through the vagal enteric afferents and/or an endocrine pathway to the brain as shown for mammalian species (203). Furthermore, circulating levels of nutrients modulate the responses of hypothalamic neurons that express either AgRP and NPY (AgRP/NPY neurons) or CART and POMC (CART/POMC neurons). As a general pattern, higher nutrient levels activate the CART/POMC neurons and inhibit the AgRP/NPY neurons (201) inducing satiation. Although it was described that these peptides affect the GH/IGF axis (29, 83), it is not clear at this point if GH is concomitantly affected. Nevertheless, nutrients such as proteins, amino acids, and lipids modulate the GH/IGF axis [for review, see (28)]. For instance, it was shown in rainbow trout that in well-fed fish, GH induced hepatic IGF expression through a signaling pathway involving JAK-STAT, PI3K-Akt, and ERK. However, under fasting conditions, GH stimulated lipolysis through PKC/phospholipase C (PLC) and MAPK/ERK pathways (40). Furthermore, two GH receptor (GHR) isoforms have been described in teleost fish named GHR-I and GHR-II with additional variants in salmonids (GHR2a, GHR2b) (40, 204, 205). If the receptor isoforms are involved in switching signal transductions is not yet established. Nevertheless, according to tissue expression patterns and changes in the expression levels of the isoforms depending on nutritional status, it is suggested that GHR-I could be involved in growth promotion, while GHR-II could be involved in the metabolic response to GH (40). However, experimental confirmation is still required. Thus, nutrient availability is sensed, and a coordinated response by the central and peripheral systems is executed to provoke either somatic growth or internal maintenance processes.
3.4 Salinity
The relationship between the somatotrophic axis and osmoregulation has been extensively demonstrated (206–211). In this regard, GH and IGF- 1 improve acclimation and survival to high salinity water (212–220). Conversely, water salinity modulates growth rates, food intake, and feed efficiency ratio in several species. When euryhaline or freshwater fish species were transferred to high salinity water, they showed a higher growth rate and improved feed efficiency ratio (221–225). In marine fish species, however, intermedia salinities improve growth rates and feed conversion (226–230). The mechanisms by which water salinity influences growth are not fully understood; although, a reduction of energy expenditure related to osmotic regulation would be involved (207). Additionally, increasing water salinity stimulates GH and IGF production. For example, plasma GH and IGF- 1 levels were increased in catfish and trout when transferred to high salinities (231, 232). Moreover, changes in water salinity modulate the expression levels of genes of the somatic growth axis in salmonids (213), blackhead sea bream (Acanthopagrus schlegelii) (233), gilthead sea bream (234), tilapia (Oreochromis mossambicus) (235), and pejerrey (Odontesthes bonariensis) (236). Furthermore, high salinity increases food intake (237, 238), perhaps stimulated directly by GH or indirectly through the metabolic effects of GH (75, 239, 240). Moreover, GH improves nutrient absorption at the gut level (241) which can explain the observed increase in feed efficiency.
3.5 Oxygen
The concentration of dissolved oxygen (DO) in water would affect the performance of fish as oxygen is required for respiration and metabolism. Environmental conditions regarding oxygen partial pressure, water temperature, and salinity as well as oxygen consumption by aquatic organisms (biological oxygen demand) would affect the amount of DO in the water column (242). Low levels of DO or hypoxia could be defined as the values of DO that negatively affect the physiology or behavior of fish (242–245). Hypoxia conditions reduce respiration, feeding activities, and growth rate and increase the chances of disease in fish (243, 246–248). Furthermore, hypoxia induces behavioral, physiological, immunological, and metabolic adjustment [for review, see (243)] including swimming behavior, declined metabolic rate, high ventilation and anaerobic respiration, and high Hb-O2 affinity to cope with low oxygen stress. In addition, hypoxia inhibits gonadal development and reproduction as was shown in goldfish exposed to different diel hypoxia cycles (249). Furthermore, hypoxia provokes a reduction in egg production and spawning, delays embryo development, and increases embryo and larvae mortality (242).
In terms of somatic growth and feeding, it has been shown that low DO level negatively impacts growth, feed intake, and overall fish performance (242, 244, 245). In Nile tilapia reared at high, medium, and low DO, the fastest rate of growth was at high DO and the slowest growth was at low DO (250). In the same study, the authors found that the food conversion ratio (FCR) was inversely related to the dissolved oxygen level. Thus, exposure to hypoxia reduced growth and feed utilization, as well as respiration and feeding activities (251–253). It has been shown in several fish species that hypoxia inhibited weight gain, probably due to low feed intake (252, 254–260).
Reduced food intake at low DO has been found in several fish species such as channel catfish (Ictalurus punctatus) (261, 262), rainbow trout (263), blue tilapia (Oreochromis aureus) (264), European sea bass (Dicentrarchus labrax) (265), juvenile turbot (Scophthalmus maximus) (252), and Nile tilapia (250, 257–260).
Nevertheless, how hypoxic conditions affect the regulatory endocrine axis for both somatic growth and feeding is not clear. Somatic growth inhibition and lowered plasma IGF-1 occur in hypoxic conditions as well as in crowded fish (266, 267). Thus, activation of the stress response of the hypothalamus–pituitary–interrenal (HPI) axis could be responsible for the inhibition of hepatic response to GH (266, 267). However, gh-transgenic fishes show a reduced capacity to cope with a hypoxic environment (268, 269), suggesting some interaction between hypoxia and the GH/IGF axis. In this regard, it was shown that knocking down insulin-like growth factor binding protein (igfbp)-1, a known IGF- binding protein with growth inhibition effect (270–272), moderates the hypoxia effects on growth and development in zebrafish (Danio rerio) (273). On the contrary, overexpression of igfbp-1 mimics hypoxia- induced growth and developmental retardation, even under normoxia (273). Furthermore, sirtuins, which are involved in conveying energy level information and modulating the anabolic action of GH by inhibiting GH receptor signaling (28, 39), could act as links between oxygen availability, energy status, and the somatotropic axis (39).
4 Effect of different pollutants on the fish endocrine network governing growth and feeding
Fish are particularly vulnerable to pollution because they are heavily exposed to contaminants as they feed and live in the aquatic environment. Therefore, fish are more sensitive to many toxicants in comparison with other vertebrates, making them an important subject of experimentation and good bioindicators of ecosystem health. In this section, we will cover the knowledge about the impact of pollutants on the endocrine axes that regulate growth and food intake behavior in fish.
A key role in the integration of stress response is played by the steroid hormone cortisol. Cortisol is usually released into circulation when fish are under conditions of stress by the activation of the vertebrate hypothalamus–pituitary–adrenal (HPA) axis, so- called the “stress axis.” Cortisol is one of the downstream main effectors of the HPA axis, playing essential roles in development, energy balance, and behavior (274). One notorious effect of cortisol is an increase in the plasmatic levels of GH (217, 275, 276). Aside from a possible direct effect at the pituitary level increasing GH synthesis and release (277), cortisol decreases the hepatic expression of the GH receptors ghr1 and ghr2 and decreases the serum levels of IGF-1 and the liver expression of igf-1 (162). Thus, corticosteroids could induce a state of “GH resistance” characterized by high serum GH levels concomitant to low serum IGF- 1 levels. Moreover, in chronic treatments, cortisol led to a reduction of somatic growth in fish. Concerning food intake regulation, it has been reported that chronic and acute stress treatments exert a potent anorexigenic effect in fish involving several hypothalamic neuropeptide s such as CRH and aMSH, along with an increase in cortisol levels (278). This steroid hormone seems also to be involved in the maintenance of the anorectic response in teleost (279–281). The synthesis and release of cortisol in response to contaminants in fish have been very well documented. However, the specific connection between each pollutant and the expression of factors governing growth and or food intake, mediated by cortisol, is not always evaluated. Therefore, in those cases in which the effect of pollutants on cortisol as mediating their effects on growth and food intake was not studied, we will only mention it to point out the need for additional studies.
4.1 Heavy metals
Heavy metal pollution in aquatic environments is the result of anthropogenic and natural activities, such as atmospheric deposition, geological weathering, and the discharge of agricultural, municipal, residential, or industrial waste products. When heavy metals occur in high concentrations, they are a serious threat because of their toxicity, long persistence, bioaccumulation, and biomagnification in the food chain. Low levels of pollution may have no apparent impact on fish, but it may decrease the fecundity of fish populations, leading to a long-term decline and eventual extinction of species (282). Heavy metals negatively affect various metabolic processes in fish embryos, resulting in developmental retardation, morphological and functional anomalies, and ultimately premature death. Additionally, heavy metals activate energy-consuming detoxification processes resulting in less energy available for growth (283). Cortisol is usually released when fish are under conditions of stress, and one of the consequences observed is an increase in the plasmatic levels of growth hormone and a concomitant reduction in IGF levels (217, 275, 276). In chronic treatments, this led to a reduction of somatic growth.
Concerning the impact of heavy metals at the endocrine level, it was found that cadmium delays the expression of GH during the early development of rainbow trout, demonstrating an endocrine-disrupting capacity in vivo of cadmium in teleost fish (284). Lead was also found to inhibit the expression of GH in roho labeo (Labeo rohita). Concentrations of Pb from around the LC50 were found to reduce the growth and consistently the plasmatic levels of GH while increasing cortisol, compared with the control group during 5 weeks (285). When Prussian carp (Carassius auratus gibelio) were exposed to Cd at 1, 2, and 4 mg/l per 30 days, the gene expression levels of several neuropeptides in the brain were consistent with the observed changes in food intake behavior (286). In all the Cd-exposed groups, the expression levels of NPY, apelin, and metallothionein increased significantly, while those of POMC, ghrelin, and CRH decreased significantly. The authors suggested that low doses of Cd might increase food intake, as well as weight and length gains, but high doses of Cd might have the opposite effect. The observed changes in food intake seem to be a result of neurohumoral regulation in response to Cd exposure. The expression levels of three genes (gh, ghr, and igf-1) related to the growth endocrine axis were shown to be altered in grass carp (Ctenopharyngodon idella) under mercury chloride (HgCl2) and temperature stress. According to the authors, this result indicated that the combined stress of temperature and HgCl2 affected the growth performance of grass carp at the molecular level. In this study, the expression of gh and ghr has increased, while that of igf-1 has decreased, indicating that there was a subtle interaction between the two experimental factors (287). These results show that the binding ability of GH and its receptor decreases in fish under physiological stress, resulting in the decrease of signal from GHR, leading to the inhibition of IGF-1 synthesis and ultimately decreasing fish growth (236, 288). Arsenic is a contaminant commonly present in water and crops from several parts of the world, and exposure to arsenic is linked to decreased birth weight, decreased weight gain, and improper muscle function. The effects of embryonic arsenic exposure on muscle growth and on the IGF pathway were tested in killifish (Fundulus heteroclitus) by Szymkowicz et al. (289). The authors found significant reductions in condition factors of fish exposed to arsenic after 16, 28, and 40 weeks. Additionally, they found that exposure of killifish embryos to arsenic has long-term effects, reducing growth and increasing both igf-1 and igf-1r levels in skeletal muscle even after 1 year. Since fish presented reduced growth, the higher expression levels of igf-1 and its receptor in skeletal muscle might be related to a dysregulation in their expression originated by the early exposition to AsIII. In common carp, a decreased expression of gh mRNA was detected in the pituitary in response to Zn (290). In rainbow trout, a decreased expression of gh, igf-1, and igf- 2 was detected in an exposure time-dependent response to Zn and Co (291). The authors conclude that igf- 1 is the most resistant and gh is the most sensitive component against cobalt and zinc exposure. The effect of Zn and Cu on the action of CCK on the enzymes peptidases and glycosidases in goldfish intestine was examined. Interestingly, both metals affect the activity of glycosidases induced by CCK. This means that the action of CCK is influenced by the presence of Zn and Cu, which could affect other processes involving the action of CCK (apart from nutrient assimilation) such as food intake regulation (292).
The effect of a mixture of heavy metals was studied in Nile tilapia (293). Adults chronically exposed (5 weeks) to a mixture of Pb, Cu, and Zn at sublethal concentrations showed a decrease in all parameters related to growth and body weight compared with control. Consistently, the expression of igf-1 and ghrelin in liver and stomach, respectively, decreased in the exposed group compared with the control group (293).
4.2 Persistent organic pollutants
Persistent organic pollutants (POPs) are ubiquitous environmental contaminants that are not easily degraded and can biomagnify in aquatic and marine food webs (294). The list of POPs includes a wide range of halogenated contaminants such as polychlorinated biphenyls (PCBs), dichlorodiphenyltrichloroethane (DDT), chlordanes, and hexachlorobenzene (HCB) as well as chemicals of emerging concern such as polybrominated diphenyl ethers (PBDEs) or perfluorinated compounds. Because of their lipophilic or proteophilic nature, they can be found at high concentrations in the tissues of aquatic organisms (295, 296). POPs could originate as industrial compounds or pesticides, being produced during natural disasters (e.g., forest fires, volcanic eruptions), as a by-product during wood pulp processing or in the synthesis of chlorinated chemicals, or as a result of incineration of chlorine-containing compounds (297). Although POPs have relatively low hormonal activity, they are persistent in animal tissues and, thus, pose a serious risk as endocrine disruptors. There is a wide range of molecular interactions between POPs and the endocrine system of fish. The effects can vary depending on the chemical, the fish species, sex, reproductive stage, and exposure conditions, among other factors. The action of POPs as endocrine disruptors could be at different levels, such as hormone synthesis, transport and secretion, transformation, excretion or clearance, receptor recognition/binding, and post-receptor response (298). Thus, the mechanisms of POP-induced toxicity at the endocrine level can be complex, with limited information on the molecular interaction between these toxicants and the hormonal system. In zebrafish, the developmental effects of lifelong exposure to environmentally relevant concentrations of two natural mixtures of POP s were investigated by Lyche and colleagues (299). The mixtures emulate those found in freshwater systems in Norway, with high and background levels of PBDE s, PCB s, and DDT metabolites. The phenotypic effects observed in both exposure groups included differences in body weight at 5 months of age. Genome-wide transcription profiling showed changes in the regulation of genes involved in endocrine signaling and growth. Consistent with the changes observed at the growth level, the transcriptomic changes include key regulator genes for steroid hormone functions (ncoa3) and growth (c/ebp, ncoa3) (299).
Although POPs have been highlighted as endocrine disruptors of special concern, as far as we know, their impact has been studied on the thyroid and reproductive axes (300). Only the abovementioned study reports an effect on the growth endocrine axis, highlighting a clear gap of knowledge since it was widely demonstrated that POPs generated several alterations of growth in fish (301). Additionally, and to the best of our knowledge, no studies have been carried out to cover the effects of POPs on the endocrine regulation of food intake in fish.
4.3 Agrochemicals
The existence of several agrochemicals, popularly known as pesticides, in water bodies such as dams, lakes, streams, and rivers generates a multifarious exposure of these chemicals to aquatic organisms (302). Pesticides are known as elements released into the environment to kill fungi, weeds, insects, rodents, etc. Despite their advantages for the human economy, pesticides might have harmful effects on humans and other organisms (303). Based on their harmfulness, the World Health Organization (WHO) grouped them into four categories, namely, exceedingly hazardous, greatly hazardous, abstemiously dangerous, and considerably harmful (304). Although there are a few reports indicating the effects of agrochemicals on growth and metabolism in fish (305–307), there are very few reports on the effect on the endocrine growth axis. A possible alteration of the growth endocrine axis by agrochemicals was found in a study by Singh and colleagues (308). In this study, high levels of pesticides (hexachlorocyclohexane, dichlorodiphenyl-trichloroethane, and chlorpyrifos) were co-related with low levels of cortisol in wild populations of catfish. It is inferred that low levels of cortisol lead to an increase in the expression of GH, but the real effect on fish growth was not determined. The effect of two widely used insecticides, chlorpyrifos and esfenvalerate, was evaluated on the expression of igf-1 in juveniles of Chinook salmon. A decreased expression of igf-1 was found in the spleen and related by the authors to immune system response and growth effects (309). Clearly, there is a knowledge gap about the impact of agrochemicals on the endocrine growth axis and the endocrine factors regulating food intake in fish. In the search for molecular biomarkers suitable for use in aquatic toxicology, knowing the effect of agrochemicals on the expression of genes of the abovementioned axes would be a great advantage.
4.4 Pharmaceutical and personal care products
Pharmaceutical and personal care products (PPCPs) are widely used by individuals for health or cosmetic reasons or by industries to promote growth or protect the health of production animals. These chemicals include a huge variety of therapeutic and veterinary drugs, fragrances, and cosmetics. The number of PPCPs has increased exponentially during the past decades, with most of them being synthetic compounds. Therefore, fish do not have a completely evolved tolerance to these chemicals. Thus, the large number of PPCPs and their diversity in chemical structures and toxicology currently pose a significant challenge in understanding the adverse environmental and health impacts of these products on animals and humans. Moreover, in the environment, organisms are exposed to mixtures of chemicals which exert their effects through complex chemical, toxicological, and physiological interactions (310). Therefore, in this section, we will describe as far as possible the interactions between PPCPs with the endocrine growth axis and hormones governing food intake behavior.
The effect of environmental estrogens (EEs) such as 17β-estradiol (E2), β-sitosterol (βS), and 4-n-nonylphenol (NP) on the synthesis of IGFs on rainbow trout was studied by Hanson et al. (311). The authors found that E2, βS, and NP significantly inhibited the expression of igf-1 and igf-2 mRNAs in the liver and gill in a time- and concentration-related manner. The mechanism through which EEs inhibit igfs mRNA expression was investigated in vitro in isolated liver cells. The authors determined that EE treatment deactivated JAK, STAT, ERK, and AKT. Also, the blockade of GH-stimulated igf expression by EEs was accompanied by the deactivation of JAK, STAT, ERK, and AKT. Overall, the results indicate that EEs directly inhibit the expression of igf mRNAs by disrupting GH post-receptor signaling pathways (311). Embryos of rainbow trout were exposed to graded concentrations of E2 (measured: 0, 1.13, 1.57, 6.22, 16.3, 55.1, and 169 ng/L) from hatching to 4 and up to 60 days post-hatch (dph) to assess molecular and apical responses (312). Whole proteome analyses of alevins did not show clear estrogenic effects, but among other results, some terms were overrepresented, including regulation of IGF transport and uptake by IGFBPs, apolipoprotein A1/A4/E domain, and lipid transport. Although all these factors have been previously associated (by other authors) with endocrine functions such as growth, this apical response was not observed in this work. The effects of 17a-ethinylestradiol (EE2), 17β-estradiol, and 4-nonylphenol on igfbps were studied in two stages of Atlantic salmon by Breves et al. (313). In fry and smolts, hepatic ghr, igf-1, and igf-2 were diminished by exposure to endocrine disruptor chemicals (EDCs). In fry, EDCs diminished igfbp1b1, igfbp2a, igfbp2b1, igfbp4, igfbp5b2, and igfbp6b1. In smolts, EDCs diminished igfbp1b1, igfbp4, and igfbp6b1. Igfbp5a was stimulated by EDCs in both fry and smolts. The authors conclude that hepatic igfbps directly or indirectly respond to environmental estrogens during two key life stages of Atlantic salmon and, thus, might modulate the growth and development of exposed individuals. Shved and coworkers (314) investigated whether estrogen exposure during early development affects growth and the IGF- 1 system, both at the systemic and tissue levels in Nile tilapia. Developmental exposure to 17a-ethinylestradiol had persistent effects on sex ratio and growth. Serum IGF-1, hepatic igf-1 mRNA, and the number of igf-1 mRNA-containing hepatocytes were significantly decreased at 75 days post-fertilization (DPF), while liver receptor alpha mRNA was significantly induced. In both sexes, pituitary GH mRNA was significantly suppressed. A transient downregulation of igf-1 mRNA occurred in the ovaries (75 DPF) and testes (90 DPF). These results clearly show that estrogen treatment impairs GH/IGF- 1 expression in fish and also that the effects persist during development. These long-lasting effects seem to be exerted indirectly via inhibition of pituitary GH and directly by suppression of local IGF-1 in organ-specific cells (314). These authors also demonstrated in a related work that environmentally relevant concentrations of EE2 interfere with the GH/IGF- 1 system, at the endocrine, paracrine, and autocrine levels in developing Nile tilapia (315). These results at the molecular level were also accompanied by diminished growth and weight gain in male fish. All these results demonstrated that estrogens released into the environment interact with several endocrine functions in fish, related not only to reproduction but also to growth and metabolism.
The influence of PPCPs on endocrine factors regulating food intake in fish was not yet determined. This is an interesting topic to address particularly in the case of EEs since they can affect the intrinsic relationship between reproduction, growth, and food intake in fish (23, 316, 317). As an antecedent, a study carried out by Bertucci et al. (318) aimed to determine whether estradiol (E2) and testosterone (T) affect the expression of several components of this axis: preproghrelin, ghrelin/growth hormone secretagogue receptor (GHS-R), ghrelin O-acyl transferase (GOAT), and NUCB2 in goldfish. RT- qPCR analyses at 2.5 days post-administration show that gut preproghrelin and GOAT expression was upregulated by both E2 and T treatments, while the same effect was observed for GHS-R only in the pituitary. Both treatments also reduced hypothalamic preproghrelin mRNA expression. NUCB2 expression was increased in the forebrain of the T-treated group and reduced in the gut and pituitary under both treatments. These results showed modulation by sex steroids of genes implicated in both food intake behavior and reproduction that might help to explain the reproduction- dependent energy demands in fish and highlight a possible negative impact of PPCPs in wild fish.
4.5 Micro- and nano plastics
There are several works studying how microplastics and, to a lower extent, nanoplastics can affect fish growth [for review, see (319–321)]. Although most of these works were carried out on model fish species and with concentrations of plastic particles that are several orders of magnitude above the range found in the environment (322), they provide novelty data about the problem of plastics in the aquatic environment. A few of these articles studied the effect of micro- or nanoplastics on the endocrine growth axis. Some works investigated the effect of microplastics and their combinations with other pollutants at the transcriptomic level, but no reports of genes involved in growth were found (323–325). Zheng and colleagues (326) investigated the effects of embryo–larvae exposure to 500 μg/L of polystyrene microplastic (MP) (5 µm) alone and in combination with ZnO nanoparticles (NPs) on exposed F0 and unexposed F1 larvae of zebrafish. The authors found that growth inhibition, oxidative stress, apoptosis, and disturbance of the GH/IGF axis were induced by MPs alone in F0 larvae. Reduced growth and antioxidant capacity and downregulated GH/IGF axis were merely observed in F1 larvae from F0 parents exposed to MPs + ZnO. In another work carried out by Pedersen and colleagues (327), the early growth and development of zebrafish was not impacted by NP, but the endocrine system, disease rate, and organismal development were significantly altered by NP exposure.
Microplastics can be ingested by fish and accumulated in the stomach, generating a sensation of satiety or damage that could inhibit food intake. This was previously reported (328–331) although the effect of MP on factors that regulate food intake has not been deeply studied yet. We found only one report, by Im and coworkers (332), that investigated the neurodevelopmental toxicity of polystyrene microplastics (PSMPs) in zebrafish. Fish were exposed to PSMPs during the embryonic stage and then allowed to recover. Among other results, the authors reported an increased expression of NPY, a peptide involved among other functions in the increase of appetite. However, no relations with food intake were found in that work. On the other hand, the ingestion of polyethylene microplastics by larvae and juveniles of medaka (Oryzias latipes) does not affect the expression of molecular markers involved in glucose metabolism, energy homeostasis, weight gain, and proteolysis (ISN, GLP, PYY, and TRP) (333).
As a conclusion, there are few reports indicating that MP or NP can trigger a stress response inducing mechanisms that involved the GH–IGF axis. Moreover, their very well-documented accumulation in the stomach of fish might alter the response of peripheral factors regulating food intake, but surprisingly, this has not been deeply studied yet. These two points represent a knowledge gap that would be interesting to address to do a proper estimation of the potential risk of MP and NP present in the marine environment.
5 Effects of global climate change factors on growth and feeding endocrine axis
Fish are severely exposed to all changes produced by global climate change that currently impacts the oceans. There are predictions that indicate an increase in the mean values of water temperature, an increase in dissolved CO2 with the concomitant decrease in water pH, and higher variations in salinity, among others (334). In this section, we will summarize the data on the effects of ocean acidification and ocean warming on fish endocrine axis regulating growth and food intake.
5.1 Ocean acidification
During the last 150 years, the burning of fossil fuels has contributed to an increase in atmospheric CO2 from approximately 280 to 410 ppm, with a predicted increase of 730 to 1,020 ppm by the end of 2100 (335). The absorption of atmospheric CO2 by oceans generates rapid changes in the seawater carbonate system ultimately decreasing the pH (among other effects), a process termed ocean acidification (OA). Exposure to these conditions can severely impact marine organisms as the acclimation to a suboptimal environment requires physiological adaptive responses that are energetically costly. Consequently, fish experience environmental stress, which may impact them at the biological and physiological levels (336). A comparison of the effects of OA on the growth and development of the early life stages of marine fish indicates that these are species-specific, and thus, generalizing the impact of climate-driven ocean acidification is not warranted. The effect will ultimately depend on the capacity of the specie to balance the energy costs of acid–base homeostasis versus that contributing to somatic growth.
An interesting case study that related growth with food intake was carried out in black sea bream by Tegomo et al. (337). Although the authors did not analyze the endocrine response of the growth axis, they observed that under two projected scenarios of OA, with pH values of 7.80-pCO2 = 749.12 ± 27.03 and 7.40-pCO2 = 321.37 ± 11.48 μatm, the growth, feeding efficiency, protein efficiency ratio, and crude protein content were significantly decreased in juveniles. The authors conclude that, because of an elevated pCO2 in seawater, the fish eat more than normal but grow less than normal. These results evidence that ocean acidification provokes changes at the endocrine level inducing an increase in feeding behavior while generating diminished growth. Interestingly, Carney Almroth et al. (338) found that the enzyme BChE was decreased at elevated CO2 treatment in the Atlantic halibut, Hippoglossus hippoglossus. The BChE enzyme was inversely correlated with circulating levels of the orexigenic peptide ghrelin in mice (339). Therefore, these decreases in BChE levels under OA conditions could explain the endocrine source of the increase in food intake observed by Tegomo et al. (337) in black sea bream.
A study of the complete transcriptomic response in Asian sea bass (Lates calcarifer) juveniles reared for 7 days under OA conditions showed tissue-specific differentially expressed genes (340) involving many molecular processes in the brain, gill, and kidney. The results indicate that although short-term ocean acidification does not cause obvious phenotypic and behavioral changes, it causes substantial changes in gene expression in the analyzed tissues. These changes are related to organ and muscle development, growth, and nervous system development, as well as behavior related to memory (e.g., hunting, homing, and escaping from predators). The authors conclude that these changes in gene expressions may eventually affect the physiological fitness of fish (340).
In the Atlantic salmon, the physiological adaptations which take place during the early phase of salmon ocean migration (high hypo-osmoregulatory capacity, growth, and energy metabolism) are all processes that are under the control of the growth endocrine axis (341). The osmoregulatory development that allows smolts to have high seawater tolerance while still in freshwater may make them more susceptible to external stressors, particularly those that affect ion regulation, such as water acidification. The smolt stage of Atlantic salmon is then particularly sensitive to acidification, and it was observed that moderate conditions can lead to increased mortality and less severe conditions result in loss of salinity tolerance and reduced adult return rates (342).
Shao et al. (343) found that OA suppresses the mRNA expression of insulin-like growth factor 1 and reduces the growth rate in juveniles of orange-spotted groupers (Epinephelus coioides). In this study, the authors exposed fish to different levels of acidification: a condition predicted by the Intergovernmental Panel on Climate Change (IPCC, pH 7.8 - 8.0) and an extreme condition (pH 7.4 - 7.6) that may occur in coastal waters in the near future. After 6 weeks, the growth rates of fish reared at pH 7.4 - 7.6 were less than those raised in control water (pH 8.1 - 8.3). Additionally, exposure to pH 7.4 - 7.6 resulted in lower levels of hepatic igf-1 mRNA but did not affect the levels of pituitary gh or hypothalamic pre-pro-somatostatin II and III (psst2 and psst3, respectively). Overall, these results show that ocean acidification conditions suppress the growth of juvenile grouper, which may be a consequence of reduced levels of IGF-1, but not due to diminished growth hormone release (343). To test the effects of climate change on juvenile blue rockfish (Sebastes mystinus), Bruzzio (344) measured the endocrine response to single and combined stressors of OA and hypoxia after 1 week of exposure. Assays of cortisol and IGF-1 hormone responses served as proxies for stress and growth, respectively. There was no observable difference in IGF-1 in juvenile blue rockfish after a week of exposure to stressors such as low pH or low DO. The author also found a high peak of cortisol with low pH presumably associated with the role of cortisol in acid–base regulation. Interestingly, when cortisol levels were high, the same fish had low levels of IGF-1, but when cortisol levels were lower, the same fish had highly variable levels of IGF-1. Overall, the results indicated that pH levels influenced hormonal stress physiology, while DO levels contributed to differences in metabolism, body condition, and behavioral anxiety in juvenile blue rockfish.
All these reports highlight the necessity to investigate the effects of climate change on fish endocrine response related to growth and food intake behavior to find interrelationships with physiological and biological effects. It will be important to obtain more knowledge on molecular markers that can be used for fish population management in a rapidly changing ocean.
5.2 Ocean warming
The ocean absorbs most of the excess heat from greenhouse gas emissions, leading to rising ocean temperatures in a phenomenon known as ocean warming (OW). Increasing temperatures affect marine species causing a stunning impact on ecosystems, such as coral bleaching and the loss of breeding grounds for marine fishes and mammals. The effects of OW are being widely studied in marine invertebrates although not much information is available for fish, neither at the biological nor at the endocrinological level. At a physiological level, temperature has been proven to affect metabolic rate, aerobic metabolism, growth, reproduction, and survival with potential effects on population sustainability (345). Moreover, changes at the individual level are dependent on the capacity to modulate gene expression under environmental variation (346, 347). Changes in cellular stress proteins (heat shock proteins, antioxidant enzymes, etc.) have been detected in marine organisms exposed to acute and chronic heat stress (348, 349). Nevertheless, these studies have only focused on a few protein biomarkers and therefore do not explore all the possible changes caused by OW. In this section, we will summarize the knowledge about the influence of environmental temperature increase on the endocrine growth axis and, if available, on the endocrine regulation of feeding behavior.
The effect of water temperature increase seems to be specie-specific. For example, no changes in the mRNA expression of igf-1 with the temperature increase were found in European sea bass after 15 and 60 days of exposure, but a decrease in the myog gene (associated with muscle growth) was found at 60 days (350). In European eel (Anguilla Anguilla), the impact of temperature on thermally induced phenotypic variability from larval hatch to first feeding was studied by Politis et al. (351). The results showed that increasing temperature from 16°C to 22°C accelerated larval development, while gene expression of hsp70, hsp90, gh, and igf-1 was also accelerated at warm temperatures. On the contrary, larval gene expression patterns (hsp70, hsp90, gh, and igf-1) were delayed at cold temperatures. Moreover, the expression of gh was the highest at 16°C during the jaw/teeth formation and the first-feeding developmental stages. These results highlight the differences in the effect provoked by the increase of water temperature through developmental stages. In conclusion, the authors suggest a plausible vulnerability of this critically endangered species in future scenarios of increasing ocean temperature. The larvae of the gilthead sea bream exposed to three temperatures —18°C (control), 24°C (warm), and 30°C (heat wave)—for 7 days showed a survival decrease with increasing temperature, with no larvae surviving at 30°C. A proteomic analysis (that was only carried out at 18°C and 24°C) showed that larvae upregulated, among other proteins, the growth hormone. These results might indicate that although the temperature increase could generate a proteome modulation, gilthead sea bream larvae would not be able to fully acclimate to higher temperatures. This conclusion was supported by the observed low survival rates at high temperature (352).
Overall, as it was mentioned before, the effect of ocean warming seems to be dependent on the fish specie and on the developmental stage, leading to changes in larvae that could result in mortality or malformations altering the future stages of these fish. Independent of the specie, as mentioned in Section 3.2, temperature induces changes in the growth endocrine axis that seems to be more related to changes in metabolism, stress resistance, or energy mobilization, than directly to growth (146). However, overexpression of gh or igfs can indirectly lead to an increment of growth as a side effect. This increment in growth should be taken carefully because, instead of a positive effect, it could mask developmental problems in fish with later repercussions at a population level.
6 Concluding remarks
The main factors that influence somatic growth are nutrition, temperature, photoperiod, salinity, and reproduction. Food restriction reduces growth, as well as changes in temperature or photoperiod and reproduction modify feeding, influencing the GH/IGF axis (23, 29, 161, 353). The temperature–size response found in aquatic ectotherms (16) that may involve the animal’s capacity of capturing oxygen and the adjustments in respiratory surfaces have received high attention, leading to an intense debate (19, 20), and several mechanisms have been purposed to explain this fact (18, 20, 354–357). However, these proposals overlook the endocrine regulation of growth and the effects of hypoxia on the GH/IGF axis as well as in feeding and metabolism which will affect somatic growth as well, as described in early sections. Nevertheless, there is an intricate relationship between the abovementioned environmental factors, growth, feeding, and the endocrine processes governing them. These relationships were molded over millions of years through fish evolution and currently are being altered as a consequence of, for example, global climate change phenomena.
The effects of reproduction on somatic growth cannot be explained only as a mere allocation of energy in gonads, instead of somatic growth (20, 161), as many times simplistically illustrated [see (20) and references therein]. The relationship between growth and reproduction would not depend solely on the amount of energy devoted to gonadal development. Instead, it will depend on the hormonal control of each process and the crosstalk between the endocrine axes. Thus, sex steroid hormones may have divergent effects on the GH/IGF axis depending on specie and gender (161, 358–364). Nevertheless, in a general view, they contribute by reducing somatic growth while inducing gonadal development and sexual behavior that may reduce energy partitioning to somatic growth processes. Both GH and IGF have been implicated in gonadal development as well (23, 29, 365, 366); therefore, gender and sexual development produced a discordant variation of somatic growth and GH/IGF axis regulation (161). Sex steroids, particularly estrogens, increase pituitary GH content and release and reduce GH responsiveness and IGF production in the liver [for review, see (29)]. Thus, estrogens uncouple GH levels with somatic growth in a similar fashion to fasting and stress (29). The presence of steroid hormones and its derivates in aquatic environments as a consequence of anthropogenic activity is provoking disruptions in fish that have been mostly studied at the reproductive level. However, taking into consideration all the evidence exposed in this review, it would be important to look at their impact on growth and feeding behavior as well.
Although the available information on the effects of pollutants on growth and feeding is scarce, the few studies that address the issue clearly point out that pollutants would affect growth, metabolism, and development, affecting the animal’s performance. Thus, some heavy metals, EEs, and micro- and nanoplastics have been shown to affect growth and/or the GH/IGF axis. Nevertheless, more studies are required to analyze pollutants’ effects on growth and feeding and their regulatory endocrine network. It is possible, however, to hypothesize that most of the effects of abiotic factors that influence growth and feeding would have a common effector in the HPI axis and in the stress response. The physiological result due to stress and activation of the HPI axis is an increase in serum levels of cortisol. As such, the magnitude and duration of cortisol variation may be used to define the response to a stressful condition (161, 367). There is extensive research on cortisol response to stress, as well as cortisol effects on growth and feeding in fishes (367–371). In a general plot, stress and cortisol reduce somatic growth and appetite and decrease IGF plasma levels or mRNA levels (23, 29).
Future work analyzing the effects of pollutants and other abiotic factors not only on size, condition factors, growth rates, and food intake but also on some key regulatory hormones of somatic growth (GH, IGF), feeding (ghrelin, nesfatin-1), metabolism (insulin), and stress (cortisol, catecholamines) will help to understand the mechanistic control and its perturbation evoked by environmental factors in fishes. All this information would be very useful to integrate the early signals of endocrine disruption with the apical effects provoked by pollutants. This will generate new approaches for the study of the effect of contaminants on fish and the possibility to add a predictive value to the results.
Author contributions
Both authors, LFC and JIB, contributed to the idea and design of the manuscript, wrote sections of the MS, revised the drafts, and approved the submitted version.
Funding
This paper was partially funded by Agencia Nacional de Promoción Científica y Tecnológica (ANPCyT, Argentina; PICT 2010-1493, PICT 2019-1234) and Consejo Nacional de Investigaciones Científicas y Tecnológicas (CONICET, Argentina; PIP 2014/0595), Ministerio de Ciencia, Tecnología e Innovación Productiva (MINCyT, CyTCH-C 038) to LFC, and the European Union’s Horizon 2020 research and innovation programme under the Marie Sklodowska-Curie grant agreement No. 101027681 to JIB. This study was also supported by the Emerging Leaders of the Americas Program (ELAP, Canada).
Acknowledgments
The authors would like to thank Dr. María Corvi for the critical and grammar revision of the MS.
Conflict of interest
The authors declare that the research was conducted in the absence of any commercial or financial relationships that could be construed as a potential conflict of interest.
Publisher’s note
All claims expressed in this article are solely those of the authors and do not necessarily represent those of their affiliated organizations, or those of the publisher, the editors and the reviewers. Any product that may be evaluated in this article, or claim that may be made by its manufacturer, is not guaranteed or endorsed by the publisher.
References
1. Lorenzen K. Toward a new paradigm for growth modeling in fisheries stock assessments: Embracing plasticity and its consequences. Fisheries Res (2016) 180:4–22. doi: 10.1016/j.fishres.2016.01.006
2. Dijoux S, Boukal DS. Community structure and collapses in multichannel food webs: Role of consumer body sizes and mesohabitat productivities. Ecol Letters (2021) 24(8):1607–18. doi: 10.1111/ele.13772
3. Hendry AP, Gotanda KM, Svensson EI. Human influences on evolution, and the ecological and societal consequences. Philos Trans R Soc B: Biol Sci (2017) 372(1712):20160028. doi: 10.1098/rstb.2016.0028
4. Laufkötter CA-O, Zscheischler JA-O, Frölicher TA-O. High-impact marine heatwaves attributable to human-induced global warming. Science (2020) 369:1621–5. doi: 10.1126/science.aba0690
5. Silvy Y, Guilyardi E, Sallée J-B, Durack PJ. Human-induced changes to the global ocean water masses and their time of emergence. Nat Climate Change (2020) 10(11):1030–6. doi: 10.1038/s41558-020-0878-x
6. Bowles E, Marin K, Mogensen S, MacLeod P, Fraser DJ. Size reductions and genomic changes within two generations in wild walleye populations: Associated with harvest? Evolutionary Appl (2020) 13(6):1128–44. doi: 10.1111/eva.12987
7. Denechaud C, Smoliński S, Geffen AJ, Godiksen JA, Campana SE. A century of fish growth in relation to climate change, population dynamics and exploitation. Global Change Biol (2020) 26(10):5661–78. doi: 10.1111/gcb.15298
8. Oke KB, Cunningham CJ, Westley PAH, Baskett ML, Carlson SM, Clark J, et al. Recent declines in salmon body size impact ecosystems and fisheries. Nat Commun (2020) 11(1):4155. doi: 10.1038/s41467-020-17726-z
9. Pinsky ML, Eikeset AM, Helmerson C, Bradbury IR, Bentzen P, Morris C, et al. Genomic stability through time despite decades of exploitation in cod on both sides of the Atlantic. P Natl Acad Sci USA (2021) 118(15):e2025453118. doi: 10.1073/pnas.2025453118
10. Andersen KH, Jacobsen NS, Farnsworth KD. The theoretical foundations for size spectrum models of fish communities. Can J Fisheries Aquat Sci (2016) 73(4):575–88. doi: 10.1139/cjfas-2015-0230
11. IPCC. Climate change 2021: The physical science basis. In: Contribution of working group I to the sixth assessment report of the intergovernmental panel on climate change. Cambridge, United Kingdom and New York, NY, USA: Cambridge University Press (2021).
12. Boltaña S, Sanhueza N, Aguilar A, Gallardo-Escarate C, Arriagada G, Valdes JA, et al. Influences of thermal environment on fish growth. Ecol Evolution (2017) 7(17):6814–25. doi: 10.1002/ece3.3239
13. Wellenreuther M, Le Luyer J, Cook D, Ritchie PA, Bernatchez L. Domestication and temperature modulate gene expression signatures and growth in the Australasian snapper chrysophrys auratus. G3 (Bethesda) (2019) 9(1):105–16. doi: 10.1534/g3.118.200647
14. Besson M, Vandeputte M, van Arendonk JAM, Aubin J, de Boer IJM, Quillet E, et al. Influence of water temperature on the economic value of growth rate in fish farming: The case of sea bass (Dicentrarchus labrax) cage farming in the Mediterranean. Aquaculture (2016) 462:47–55. doi: 10.1016/j.aquaculture.2016.04.030
15. Gardner JL, Peters A, Kearney MR, Joseph L, Heinsohn R. Declining body size: A third universal response to warming? Trends Ecol Evol (2011) 26(6):285–91. doi: 10.1016/j.tree.2011.03.005
16. Daufresne M, Lengfellner K, Sommer U. Global warming benefits the small in aquatic ecosystems. Proc Natl Acad Sci (2009) 106(31):12788–93. doi: 10.1073/pnas.0902080106
17. Angilletta JMichael J, Dunham Arthur E. The temperature-size rule in ectotherms: Simple evolutionary explanations may not be general. Am Naturalist (2003) 162(3):332–42. doi: 10.1086/377187
18. Verberk WCEP, Atkinson D, Hoefnagel KN, Hirst AG, Horne CR, Siepel H. Shrinking body sizes in response to warming: Explanations for the temperature–size rule with special emphasis on the role of oxygen. Biol Rev (2021) 96(1):247–68. doi: 10.1111/brv.12653
19. Lefevre S, McKenzie DJ, Nilsson GE. Models projecting the fate of fish populations under climate change need to be based on valid physiological mechanisms. Global Change Biol (2017) 23(9):3449–59. doi: 10.1111/gcb.13652
20. Pauly D. The gill-oxygen limitation theory (GOLT) and its critics. Sci Adv (2021) 7(2):eabc6050. doi: 10.1126/sciadv.abc6050
21. Peters RH. The ecological implications of body size. Cambridge: Cambridge University Press (1983).
22. Hildrew AG, Raffaelli DG, Edmonds-Brown R. Body size: the structure and function of aquatic ecosystems. Hildrew A, Raffaelli D, Edmonds-Brown R, editors. Cambridge ;: Cambridge University Press (2007).
23. Canosa LF, Chang JP, Peter RE. Neuroendocrine control of growth hormone in fish. Gen Comp Endocrinol (2007) 151(1):1–26. doi: 10.1016/j.ygcen.2006.12.010
24. Cerdá-Reverter JM, Canosa LF. Neuroendocrine systems of the fish brain. In: Bernier NJ, van der Kraak G, Farrell AP, Brauner CJ, editors. Fish neruoendocrinology. fish physiology, 1st ed. London, UK: Elsevier (2009). p. 1–75.
25. Chang JP, Wong AO. Growth hormons regulation in fish: A multifactorial model with hypothalamic, peripheral and local Autocrine/Paracrine signals. In: Bernier NJ, van der Kraak G, Farrell AP, Brauner CJ, editors. Fish neuroendocrinology. fish physiology, 1st ed. London, UK: Elsevier (2009). p. 152–95.
26. Gahete MD, Durán-Prado M, Luque RM, Martínez-Fuentes AJ, Quintero A, Gutiérrez-Pascual E, et al. Understanding the multifactorial control of growth hormone release by somatotropes: Lessons from comparative endocrinology. Ann Ny Acad Sci (2009) 1163:137–53. doi: 10.1111/j.1749-6632.2008.03660.x
27. Vélez EJ, Unniappan S. A comparative update on the neuroendocrine regulation of growth hormone in vertebrates. Front Endocrinol (2021) 11:614981. doi: 10.3389/fendo.2020.614981
28. Canosa LF, Bertucci JI. Nutrient regulation of somatic growth in teleost fish The interaction between somatic growth, feeding and metabolism. Mol Cell Endocrinol (2020) 518:111029. doi: 10.1016/j.mce.2020.111029
29. Canosa LF, Gómez-Requeni P, Cerdá-Reverter JM. Integrative cross-talk between food intake and growth function. In: Polakof S, Moon TW, editors. Trout: From physiology to conservation. New York: Nova Science Publishers (2013). p. 179–239.
30. Bertucci JI, Blanco AM, Sundarrajan L, Rajeswari JJ, Velasco C, Unniappan S. Nutrient regulation of endocrine factors influencing feeding and growth in fish. Front Endocrinol (2019) 10(83). doi: 10.3389/fendo.2019.00083
31. Escalante-Rojas M, Martínez-Brown JM, Ibarra-Castro L, Llera-Herrera R, García-Gasca A. Effects of feed restriction on growth performance, lipid mobilization, and gene expression in rose spotted snapper (Lutjanus guttatus). J Comp Physiol B (2020) 190(3):275–86. doi: 10.1007/s00360-020-01268-3
32. Gómez-Requeni P, Kraemer NM, Canosa FL. The dietary lipid content affects the tissue gene expression of muscle growth biomarkers and the GH/IGF system of pejerrey (Odontesthes bonariensis) juveniles. Fishes (2019) 4(3):37. doi: 10.3390/fishes4030037
33. Mallard F, Le Bourlot V, Le Coeur C, Avnaim M, Péronnet R, Claessen D, et al. From individuals to populations: How intraspecific competition shapes thermal reaction norms. Funct Ecol (2020) 34(3):669–83. doi: 10.1111/1365-2435.13516
34. Korman J, Yard MD, Dzul MC, Yackulic CB, Dodrill MJ, Deemer BR, et al. Changes in prey, turbidity, and competition reduce somatic growth and cause the collapse of a fish population. Ecol Monographs (2021) 91(1):e01427. doi: 10.1002/ecm.1427
35. Shan B, Liu Y, Yang C, Li Y, Wang L, Sun D. Comparative transcriptome analysis of female and male fine-patterned puffer: Identification of candidate genes associated with growth and sex differentiation. Fishes (2021) 6(4):79. doi: 10.3390/fishes6040079
36. Ma Y, Ladisa C, Chang JP, Habibi HR. Multifactorial control of reproductive and growth axis in male goldfish: Influences of GnRH, GnIH and thyroid hormone. Mol Cell Endocrinol (2020) 500:110764. doi: 10.1016/j.mce.2019.110629
37. Taylor JF, Porter MJR, Bromage NR, Migaud H. Relationships between environmental changes, maturity, growth rate and plasma insulin-like growth factor-I (IGF-I) in female rainbow trout. Gen Comp Endocrinol (2008) 155(2):257–70. doi: 10.1016/j.ygcen.2007.05.014
38. Ranke MB, Wit JM. Growth hormone - past, present and future. Nat Rev Endocrinol (2018) 14(5):285–300. doi: 10.1038/nrendo.2018.22
39. Pérez-Sánchez J, Simó-Mirabet P, Naya-Català F, Martos-Sitcha JA, Perera E, Bermejo-Nogales A, et al. Somatotropic axis regulation unravels the differential effects of nutritional and environmental factors in growth performance of marine farmed fishes. Front Endocrinol (2018) 9:687. doi: 10.3389/fendo.2018.00687
40. Bergan-Roller HE, Sheridan MA. The growth hormone signaling system: Insights into coordinating the anabolic and catabolic actions of growth hormone. Gen Comp Endocrinol (2018) 258:119–33. doi: 10.1016/j.ygcen.2017.07.028
41. Pérez-Sánchez J, Calduch-Giner JA, Mingarro M, Vega-Rubín de Celis S, Gómez-Requeni P, Saera-Vila A, et al. Overview of fish growth hormone family. new insights in genomic organization and heterogeneity of growth hormone receptors. Fish Physiol Biochem (2002) 27(3-4):243–58. doi: 10.1023/B:FISH.0000032729.72746.C8
42. Daughaday WH. Growth hormone axis overview - somatomedin hypothesis. Pediatr Nephrol (2000) 14(7):537. doi: 10.1007/s004670000334
43. Kaplan SA, Cohen P. Review: The somatomedin hypothesis 2007: 50 years later. J Clin Endocrinol Metab (2007) 92(12):4529–35. doi: 10.1210/jc.2007-0526
44. Le Roith D, Bondy C, Yakar S, Liu JL, Butler A. The somatomedin hypothesis: 2001. Endocr Rev (2001) 22(1):53–74. doi: 10.1210/edrv.22.1.0419
45. Vélez EJ, Lutfi E, Azizi S, Perelló M, Salmerón C, Riera-Codina M, et al. Understanding fish muscle growth regulation to optimize aquaculture production. Aquaculture (2017) 467:28–40. doi: 10.1016/j.aquaculture.2016.07.004
46. Duan C, Ren H, Gao S. Insulin-like growth factors (IGFs), IGF receptors, and IGF-binding proteins: Roles in skeletal muscle growth and differentiation. Gen Comp Endocrinol (2010) 167(3):344–51. doi: 10.1016/j.ygcen.2010.04.009
47. Yakar S, Liu J, Stannard B, Butler A, Accili D, Sauer B, et al. Normal growth and development in the absence of hepatic insulin-like growth factor I. Proc Natl Acad Sci USA (1999) 96(13):324–7329. doi: 10.1073/pnas.96.13.7324
48. Yakar S, Adamo ML. Insulin-like growth factor 1 physiology: Lessons from mouse models. Endocrinol Metab Clinics North America (2012) 41(2):231–47. doi: 10.1016/j.ecl.2012.04.008
49. Björnsson BT, Johansson V, Benedet S, Einarsdottir IE, Hildahl J, Agustsson T, et al. Growth hormone endocrinology of salmonids: Regulatory mechanisms and mode of action. Fish Physiol Biochem (2002) 27(3-4):227–42. doi: 10.1023/B:FISH.0000032728.91152.10
50. Le Roith D, Scavo L, Butler A. What is the role of circulating IGF-I? Trends Endocrinol metabolism: TEM (2001) 12(2):48–52. doi: 10.1016/S1043-2760(00)00349-0
51. Butler AA, Le Roith D. Control of growth by the somatropic axis: Growth hormone and the insulin-like growth factors have related and independent roles. Annu Rev Physiol (2001) 63:141–64. doi: 10.1146/annurev.physiol.63.1.141
52. Arámburo C, Alba-Betancourt C, Luna M, Harvey S. Expression and function of growth hormone in the nervous system: A brief review. Gen Comp Endocrinol (2014) 203:35–42. doi: 10.1016/j.ygcen.2014.04.035
53. Blanco AM. Hypothalamic- and pituitary-derived growth and reproductive hormones and the control of energy balance in fish. Gen Comp Endocrinol (2020) 287:113322. doi: 10.1016/j.ygcen.2019.113322
54. Pérez-Sánchez J. The involvement of growth hormone in growth regulation, energy homeostasis and immune function in the gilthead sea bream (Sparus aurata): A short review. Fish Physiol Biochem (2000) 22(2):135–44. doi: 10.1023/A:1007816015345
55. Yada T, Azuma T, Takagi Y. Stimulation of non-specific immune functions in seawater-acclimated rainbow trout, oncorhynchus mykiss, with reference to the role of growth hormone. Comp Biochem Physiol B Biochem Mol Biol (2001) 129(2-3):695–701. doi: 10.1016/S1096-4959(01)00370-0
56. Matsuda K, Maruyama K. Regulation of feeding behavior by pituitary adenylate cyclase-activating polypeptide (PACAP) and vasoactive intestinal polypeptide (VIP) in vertebrates. Peptides (2007) 28(9):1761–6. doi: 10.1016/j.peptides.2007.03.007
57. Matsuda K, Maruyama K, Nakamachi T, Miura T, Shioda S. Effects of pituitary adenylate cyclase-activating polypeptide and vasoactive intestinal polypeptide on food intake and locomotor activity in the goldfish, carassius auratus. Ann Ny Acad Sci (2006) 1070:417–21. doi: 10.1196/annals.1317.054
58. Matsuda K, Maruyama K, Nakamachi T, Miura T, Uchiyama M, Shioda S. Inhibitory effects of pituitary adenylate cyclase-activating polypeptide (PACAP) and vasoactive intestinal peptide (VIP) on food intake in the goldfish, carassius auratus. Peptides (2005) 26(9):1611–6. doi: 10.1016/j.peptides.2005.02.022
59. Lugo JM, Oliva A, Morales A, Reyes O, Garay HE, Herrera F, et al. The biological role of pituitary adenylate cyclase-activating polypeptide (PACAP) in growth and feeding behavior in juvenile fish. J Pept Sci (2010) 16(11):633–43. doi: 10.1002/psc.1275
60. Olsson C, Holmgren S. PACAP and nitric oxide inhibit contractions in the proximal intestine of the atlantic cod, gadus morhua. J Exp Biol (2000) 203(3):575–83. doi: 10.1242/jeb.203.3.575
61. Matsuda K, Kashimoto K, Higuchi T, Yoshida T, Uchiyama M, Shioda S, et al. Presence of pituitary adenylate cyclase-activating polypeptide (PACAP) and its relaxant activity in the rectum of a teleost, the stargazer, uranoscopus japonicus. Peptides (2000) 21(6):821–7. doi: 10.1016/S0196-9781(00)00215-1
62. Abbott M, Volkoff H. Thyrotropin releasing hormone (TRH) in goldfish (Carassius auratus): Role in the regulation of feeding and locomotor behaviors and interactions with the orexin system and cocaine- and amphetamine regulated transcript (CART). Hormones Behavior (2011) 59(2):236–45. doi: 10.1016/j.yhbeh.2010.12.008
63. Maruyama K, Miura T, Uchiyama M, Shioda S, Matsuda K. Relationship between anorexigenic action of pituitary adenylate cyclase-activating polypeptide (PACAP) and that of corticotropin-releasing hormone (CRH) in the goldfish, carassius auratus. Peptides (2006) 27(7):1820–6. doi: 10.1016/j.peptides.2006.01.013
64. Matsuda K, Kojima K, Shimakura S-I, Wada K, Maruyama K, Uchiyama M, et al. Corticotropin-releasing hormone mediates [alpha]-melanocyte-stimulating hormone-induced anorexigenic action in goldfish. Peptides (2008) 29(11):1930–6. doi: 10.1016/j.peptides.2008.06.028
65. Kang KS, Yahashi S, Azuma M, Matsuda K. The anorexigenic effect of cholecystokinin octapeptide in a goldfish model is mediated by the vagal afferent and subsequently through the melanocortin- and corticotropin-releasing hormone-signaling pathways. Peptides (2010) 31(11):2130–4. doi: 10.1016/j.peptides.2010.07.019
66. Kumar U, Singh S. Role of somatostatin in the regulation of central and peripheral factors of satiety and obesity. Int J Mol Sci (2020) 21(1422-0067(1422-0067 (Electronic):2568. doi: 10.3390/ijms21072568
67. Fuessl HS, Carolan G, Williams G, Bloom SR. Effect of a long-acting somatostatin analogue (SMS 201–995) on postprandial gastric emptying of 99mTc-tin colloid and mouth-to-Caecum transit time in man. Digestion (1987) 36(2):101–7. doi: 10.1159/000199407
68. Heini AF, Kirk KA, Lara-Castro C, Weinsier RL. Relationship between hunger-satiety feelings and various metabolic parameters in women with obesity during controlled weight loss. Obes Res (1998) 6(3):225–30. doi: 10.1002/j.1550-8528.1998.tb00341.x
69. Lieverse RJ, Jansen JBMJ, Masclee AAM, Lamers CBHW. Effects of somatostatin on human satiety. Neuroendocrinology (1995) 61(2):112–6. doi: 10.1159/000126831
70. Lembcke B, Creutzfeldt W, Schleser S, Ebert R, Shaw C, Koop I. Effect of the somatostatin analogue sandostatin (SMS 201–995) on gastrointestinal, pancreatic and biliary function and hormone release in normal men. Digestion (1987) 36(2):108–24. doi: 10.1159/000199408
71. Eilertson CD, Sheridan MA. Differential-effects of somatostatin-14 and somatostatin-25 on carbohydrate and lipid-metabolism in rainbow-trout oncorhynchus-mykiss. Gen Comp Endocrinol (1993) 92(1):62–70. doi: 10.1006/gcen.1993.1143
72. Klein SE, Sheridan MA. Somatostatin signaling and the regulation of growth and metabolism in fish. Mol Cell Endocrinol (2008) 286(1-2):148–54. doi: 10.1016/j.mce.2007.08.010
73. Sheridan MA, Kittilson JD. The role of somatostatins in the regulation of metabolism in fish. Comp Biochem Physiol B-Biochem Mol Biol (2004) 138(4):323–30. doi: 10.1016/j.cbpc.2004.04.006
74. White SL, Volkoff H, Devlin RH. Regulation of feeding behavior and food intake by appetite-regulating peptides in wild-type and growth hormone-transgenic coho salmon. Hormones Behavior (2016) 84:18–28. doi: 10.1016/j.yhbeh.2016.04.005
75. Leggatt RA, Raven PA, Mommsen TP, Sakhrani D, Higgs D, Devlin RH. Growth hormone transgenesis influences carbohydrate, lipid and protein metabolism capacity for energy production in coho salmon (Oncorhynchus kisutch). Comp Biochem Physiol Part B: Biochem Mol Biol (2009) 154(1):121–33. doi: 10.1016/j.cbpb.2009.05.010
76. Johnsson JI, Björnsson BT. Growth hormone increases growth rate, appetite and dominance in juvenile rainbow trout, oncorhynchus mykiss. Anim Behaviour (1994) 48(1):177–86. doi: 10.1006/anbe.1994.1224
77. Silverstein JT, Wolters WR, Shimizu M, Dickhoff WW. Bovine growth hormone treatment of channel catfish: strain and temperature effects on growth, plasma IGF-I levels, feed intake and efficiency and body composition. Aquaculture (2000) 190(1):77–88. doi: 10.1016/S0044-8486(00)00387-2
78. Zhong C, Song Y, Wang Y, Zhang T, Duan M, Li Y, et al. Increased food intake in growth hormone-transgenic common carp (Cyprinus carpio l.) may be mediated by upregulating agouti-related protein (AgRP). Gen Comp Endocrinol (2013) 192:81–8. doi: 10.1016/j.ygcen.2013.03.024
79. Dalmolin C, Almeida DV, Figueiredo MA, Marins LF. Food intake and appetite control in a GH-transgenic zebrafish. Fish Physiol Biochem (2015) 41(5):1131–41. doi: 10.1007/s10695-015-0074-5
80. Kim JH, Chatchaiphan S, Crown MT, White SL, Devlin RH. Effect of growth hormone overexpression on gastric evacuation rate in coho salmon. Fish Physiol Biochem (2018) 44(1):119–35. doi: 10.1007/s10695-017-0418-4
81. Foster AR, Houlihan DF, Gray C, Medale F, Fauconneau B, Kaushikj SJ, et al. The effects of ovine growth hormone on protein turnover in rainbow trout. Gen Comp Endocrinol (1991) 82(1):111–20. doi: 10.1016/0016-6480(91)90302-M
82. Leung TC, Ng TB, Woo NYS. Metabolic effects of bovine growth hormone in the tilapia oreochromis mossambicus. Comp Biochem Physiol Part A: Physiol (1991) 99(4):633–6. doi: 10.1016/0300-9629(91)90142-Y
83. Volkoff H. The neuroendocrine regulation of food intake in fish: A review of current knowledge. Front Neurosci (2016) 10(540). doi: 10.3389/fnins.2016.00540
84. Canosa LF, Unniappan S, Peter RE. Periprandial changes in growth hormone release in goldfish: role of somatostatin, ghrelin, and gastrin-releasing peptide. Am J Physiol Regul Integr Comp Physiol (2005) 289(1):R125–R33. doi: 10.1152/ajpregu.00759.2004
85. Kaiya H, Small BC, Bilodeau AL, Shepherd BS, Kojima M, Hosoda H, et al. Purification, cDNA cloning, and characterization of ghrelin in channel catfish, ictalurus punctatus. Gen Comp Endocrinol (2005) 143(3):201–10. doi: 10.1016/j.ygcen.2005.03.012
86. Unniappan S, Peter RE. In vitro and in vivo effects of ghrelin on luteinizing hormone and growth hormone release in goldfish. Am J Physiol Regul Integr Comp Physiol (2004) 286(6):R1093–101. doi: 10.1152/ajpregu.00669.2003
87. Unniappan S, Peter RE. Structure, distribution and physiological functions of ghrelin in fish. Comp Biochem Physiol - A Mol Integr Physiol (2005) 140(4):396–408. doi: 10.1016/j.cbpb.2005.02.011
88. Volkoff H, Canosa LF, Unniappan S, Cerdá-Reverter JM, Bernier NJ, Kelly SP, et al. Neuropeptides and the control of food intake in fish. Gen Comp Endocrinol (2005) 142(1-2):3–19. doi: 10.1016/j.ygcen.2004.11.001
89. Blanco AM, Bertucci JI, Valenciano AI, Delgado MJ, Unniappan S. Ghrelin suppresses cholecystokinin (CCK), peptide YY (PYY) and glucagon-like peptide-1 (GLP-1) in the intestine, and attenuates the anorectic effects of CCK, PYY and GLP-1 in goldfish (Carassius auratus). Hormones Behavior (2017) 93:62–71. doi: 10.1016/j.yhbeh.2017.05.004
90. Blanco AM, Pemberton JG, Gonzalez R, Hatef A, Pham V, Chang JP, et al. Nesfatin-1 is an inhibitor of the growth hormone-insulin-like growth factor axis in goldfish (Carassius auratus). J Neuroendocrinol (2021) 33(9):e13010. doi: 10.1111/jne.13010
91. Gonzalez R, Kerbel B, Chun A, Unniappan S. Molecular, cellular and physiological evidences for the anorexigenic actions of nesfatin-1 in goldfish. PloS One (2010) 5(12):e15201. doi: 10.1371/journal.pone.0015201
92. Gonzalez R, Perry RLS, Gao X, Gaidhu MP, Tsushima RG, Ceddia RB, et al. Nutrient responsive nesfatin-1 regulates energy balance and induces glucose-stimulated insulin secretion in rats. Endocrinology (2011) 152(10):3628–37. doi: 10.1210/en.2010-1471
93. Gonzalez R, Reingold BK, Gao X, Gaidhu MP, Tsushima RG, Unniappan S. Nesfatin-1 exerts a direct, glucose-dependent insulinotropic action on mouse islet β- and MIN6 cells. J Endocrinol (2011) 208(3):R9–R16. doi: 10.1530/JOE-10-0492
94. Kerbel B, Unniappan S. Nesfatin-1 suppresses energy intake, Co-localises ghrelin in the brain and gut, and alters ghrelin, cholecystokinin and orexin mRNA expression in goldfish. J Neuroendocrinol (2012) 24(2):366–77. doi: 10.1111/j.1365-2826.2011.02246.x
95. Blanco AM, Velasco C, Bertucci JI, Soengas JL, Unniappan S. Nesfatin-1 regulates feeding, glucosensing and lipid metabolism in rainbow trout. Front Endocrinol (2018) 9(AUG). doi: 10.3389/fendo.2018.00484
96. Li Z, Gao L, Tang H, Yin Y, Xiang X, Li Y, et al. Peripheral effects of nesfatin-1 on glucose homeostasis. PloS One (2013) 8(8):e71513. doi: 10.1371/journal.pone.0071513
97. Dong J, Xu H, Wang PF, Cai GJ, Song HF, Wang CC, et al. Nesfatin-1 stimulates fatty-acid oxidation by activating AMP-activated protein kinase in STZ-induced type 2 diabetic mice. PloS One (2013) 8(12):e83397. doi: 10.1371/journal.pone.0083397
98. Yin Y, Li Z, Gao L, Li Y, Zhao J, Zhang W. AMPK-dependent modulation of hepatic lipid metabolism by nesfatin-1. Mol Cell Endocrinol (2015) 417:20–6. doi: 10.1016/j.mce.2015.09.006
99. Himick BA, Golosinski AA, Jonsson AC, Peter RE. Cck/Gastrin-like immunoreactivity in the goldfish pituitary - regulation of pituitary-hormone secretion by cck-like peptides in-vitro. Gen Comp Endocrinol (1993) 92(1):88–103. doi: 10.1006/gcen.1993.1146
100. Canosa LF, Peter RE. Effects of cholecystokinin and bombesin on the expression of preprosomatostatin-encoding genes in goldfish forebrain. Regul Pept (2004) 121(1-3):99–105. doi: 10.1016/j.regpep.2004.04.011
101. Volkoff H. The role of neuropeptide y, orexins, cocaine and amphetamine-related transcript, cholecystokinin, amylin and leptin in the regulation of feeding in fish. Comp Biochem Physiol - A Mol Integr Physiol (2006) 144(3):325–31. doi: 10.1016/j.cbpa.2005.10.026
102. Himick BA, Peter RE. Cck/Gastrin-like immunoreactivity in brain and gut, and cck suppression of feeding in goldfish. Am J Physiol (1994) 267(3):R841–R51. doi: 10.1152/ajpregu.1994.267.3.R841
103. Soengas JL, Cerdá-Reverter JM, Delgado MJ. Central regulation of food intake in fish: An evolutionary perspective. J Mol Endocrinol (2018) 60(4):R171–R99. doi: 10.1530/JME-17-0320
104. Li S, Zhao L, Xiao L, Liu Q, Zhou W, Qi X, et al. Structural and functional characterization of neuropeptide y in a primitive teleost, the Japanese eel (Anguilla japonica). Gen Comp Endocrinol (2012) 179(1):99–106. doi: 10.1016/j.ygcen.2012.07.018
105. Peng C, Chang JP, Yu KL, Wong AO, Van Goor F, Peter RE, et al. Neuropeptide-y stimulates growth hormone and gonadotropin-II secretion in the goldfish pituitary: Involvement of both presynaptic and pituitary cell actions. Endocrinology (1993) 132(4):1820–9. doi: 10.1210/endo.132.4.8462479
106. Peng C, Humphries S, Peter RE, Rivier JE, Blomqvist AG, Larhammar D. Actions of goldfish neuropeptide y on the secretion of growth hormone and gonadotropin-II in female goldfish. Gen Comp Endocrinol (1993) 90(3):306–17. doi: 10.1006/gcen.1993.1086
107. Himick BA, Peter RE. Bombesin acts to suppress feeding-behavior and alter serum growth-hormone in goldfish. Physiol Behavior (1994) 55(1):65–72. doi: 10.1016/0031-9384(94)90011-6
108. Schwartz MW. Central nervous system regulation of food intake. Obes (Silver Spring) (2006) 14(Suppl 1):1S–8S. doi: 10.1038/oby.2006.275
109. Lee LTO, Siu FKY, Tam JKV, Lau ITY, Wong AOL, Lin MCM, et al. Discovery of growth hormone-releasing hormones and receptors in nonmammalian vertebrates. P Natl Acad Sci USA (2007) 104(7):2133–8. doi: 10.1073/pnas.0611008104
110. Nam BH, Moon JY, Kim YO, Kong HJ, Kim WJ, Kim KK, et al. Molecular and functional analyses of growth hormone-releasing hormone (GHRH) from olive flounder (Paralichthys olivaceus). Comp Biochem Physiol - B Biochem Mol Biol (2011) 159(2):84–91. doi: 10.1016/j.cbpb.2011.02.006
111. Parker DB, Power ME, Swanson P, Rivier J, Sherwood NM. Exon skipping in the gene encoding pituitary adenylate cyclase-activating polypeptide in salmon alters the expression of two hormones that stimulate growth hormone release. Endocrinology (1997) 138(1):414–23. doi: 10.1210/endo.138.1.4830
112. Rousseau K, Le Belle N, Pichavant K, Marchelidon J, Chow BKC, Boeuf G, et al. Pituitary growth hormone secretion in the turbot, a phylogenetically recent teleost, is regulated by a species-specific pattern of neuropeptides. Neuroendocrinology (2001) 74(6):375–85. doi: 10.1159/000054704
113. Wong AOL, Leung MY, Shea WLC, Tse LY, Chang JP, Chow BKC. Hypophysiotropic action of pituitary adenylate cyclase-activating polypeptide (PACAP) in the goldfish: Immunohistochemical demonstration of PACAP in the pituitary, PACAP stimulation of growth hormone release from pituitary cells, and molecular cloning of pituitary type I PACAP receptor. Endocrinology (1998) 139(8):3465–79. doi: 10.1210/endo.139.8.6145
114. Xiao D, Chu MMS, Lee EKY, Lin HR, Wong AOL. Regulation of growth hormone release in common carp pituitary cells by pituitary adenylate cyclase-activating polypeptide: Signal transduction involves cAMP- and calcium-dependent mechanisms. Neuroendocrinology (2002) 76(5):325–38. doi: 10.1159/000066627
115. Adams BA, Vickers ED, Warby C, Park M, Fischer WH, Craig AG, et al. Three forms of gonadotropin-releasing hormone, including a novel form, in a basal salmonid, coregonus clupeaformis. Biol Of Reproduction (2002) 67(1):232–9. doi: 10.1095/biolreprod67.1.232
116. Blaise O, Le Bail PY, Weil C. Permissive effect of insulin-like growth factor I (IGF-I) on gonadotropin releasing-hormone action on in vitro growth hormone release, in rainbow trout (Oncorhynchus mykiss). Comp Biochem Physiol - A Physiol (1997) 116(1):75–81. doi: 10.1016/S0300-9629(96)00119-3
117. Holloway AC, Leatherland JF. The effects of n-methyl-D, l-aspartate and gonadotropin-releasing hormone on in vitro growth hormone release in steroid-primed immature rainbow trout, oncorhynchus mykiss. Gen Comp Endocrinol (1997) 107(1):32–43. doi: 10.1006/gcen.1997.6907
118. Li WS, Lin HR, Wong AOL. Effects of gonadotropin-releasing hormone on growth hormone secretion and gene expression in common carp pituitary. Comp Biochem Physiol - B Biochem Mol Biol (2002) 132(2):335–41. doi: 10.1016/S1096-4959(02)00039-8
119. Lin HR, Lu M, Lin XW, Zhang WM, Sun Y, Chen LX. Effects of gonadotropin-releasing hormone (GnRH) analogs and sex steroids on growth hormone (GH) secretion and growth in common carp (Cyprinus carpio) and grass carp (Ctenopharyngodon idellus). Aquaculture (1995) 135(1-3):173–84. doi: 10.1016/0044-8486(95)01012-2
120. Lin XW, Lin HR, Peter RE. Growth hormone and gonadotropin secretion in the common carp (Cyprinus carpio l.): In vitro interactions of gonadotropin-releasing hormone, somatostatin, and the dopamine agonist apomorphine. Gen Comp Endocrinol (1993) 89(1):62–71. doi: 10.1006/gcen.1993.1009
121. Marchant TA, Chang JP, Nahorniak CS, Peter RE. Evidence that gonadotropin-releasing hormone also functions as a growth hormone-releasing factor in the goldfish. Endocrinology (1989) 124(5):2509–18. doi: 10.1210/endo-124-5-2509
122. Melamed P, Gur G, Elizur A, Rosenfeld H, Sivan B, RentierDelrue F, et al. Differential effects of gonadotropin-releasing hormone, dopamine and somatostatin and their second messengers on the mRNA levels of gonadotropin II beta subunit and growth hormone in the teleost fish, tilapia. Neuroendocrinology (1996) 64(4):320–8. doi: 10.1159/000127135
123. Wong AOL, Ng S, Lee EKY, Leung RCY, Ho WKK. Somatostatin inhibits (d-Arg6, Pro9-NEt) salmon gonadotropin-releasing hormone- and dopamine D1-stimulated growth hormone release from perifused pituitary cells of Chinese grass Carp,Ctenopharyngodon idellus. Gen Comp Endocrinol (1998) 110(1):29–45. doi: 10.1006/gcen.1997.7045
124. Rousseau K, Le Belle N, Marchelidon J, Dufour S. Evidence that corticotropin-releasing hormone acts as a growth hormone-releasing factor in a primitive teleost, the European eel (Anguilla anguilla). J Neuroendocrinol (1999) 11(5):385–92. doi: 10.1046/j.1365-2826.1999.00334.x
125. Lin XW, Lin HR, Peter RE. The regulatory effects of thyrotropin-releasing hormone on growth hormone secretion from the pituitary of common carp in vitro. Fish Physiol Biochem (1993) 11(1-6):71–6. doi: 10.1007/BF00004552
126. Batten TF, Wigham T. Effects of TRH and somatostatin on releases of prolactin and growth hormone in vitro by the pituitary of poecilia latipinna. II. electron-microscopic morphometry using automatic image analysis. Cell Tissue Res (1984) 237(3):595–603. doi: 10.1007/BF00228444
127. Kagabu Y, Mishiba T, Okino T, Yanagisawa T. Effects of thyrotropin-releasing hormone and its metabolites, Cyclo(His-pro) and TRH-OH, on growth hormone and prolactin synthesis in primary cultured pituitary cells of the common Carp,Cyprinus carpio. Gen Comp Endocrinol (1998) 111(3):395–403. doi: 10.1006/gcen.1998.7124
128. Picha ME, Strom CN, Riley LG, Walker AA, Won ET, Johnstone WM, et al. Plasma ghrelin and growth hormone regulation in response to metabolic state in hybrid striped bass: Effects of feeding, ghrelin and insulin-like growth factor-I on in vivo and in vitro GH secretion. Gen Comp Endocrinol (2009) 161(3):365. doi: 10.1016/j.ygcen.2009.01.026
129. Devesa J. The complex world of regulation of pituitary growth hormone secretion: The role of ghrelin, klotho, and nesfatins in it. Front Endocrinol (2021) 12. doi: 10.3389/fendo.2021.636403
130. Vélez EJ, Unniappan S. Nesfatin-1 and nesfatin-1-like peptide suppress growth hormone synthesis via the AC/PKA/CREB pathway in mammalian somatotrophs. Sci Rep (2020) 10(1):16686. doi: 10.1038/s41598-020-73840-4
131. Ágústsson T, Ebbesson LOE, Björnsson BT. Dopaminergic innervation of the rainbow trout pituitary and stimulatory effect of dopamine on growth hormone secretion in vitro. Comp Biochem Physiol - A Mol Integr Physiol (2000) 127(3):355–64. doi: 10.1016/S1095-6433(00)00265-8
132. Melamed P, Eliahu N, Levavi-Sivan B, Ofir M, Farchi-Pisanty O, Rentier-Delrue F, et al. Hypothalamic and thyroidal regulation of growth hormone in tilapia. Gen Comp Endocrinol (1995) 97(1):13–30. doi: 10.1006/gcen.1995.1002
133. Melamed P, Eliahu N, Ofir M, Levavi-Sivan B, Smal J, Rentier-Delrue F, et al. The effects of gonadal development and sex steroids on growth hormone secretion in the male tilapia hybrid (Oreochromis niloticus X o. aureus). Fish Physiol Biochem (1995) 14(4):267–77. doi: 10.1007/BF00004065
134. Wong AO, Chang JP, Peter RE. Dopamine stimulates growth hormone release from the pituitary of goldfish, carassius auratus, through the dopamine dl receptors. Endocrinology (1992) 130(3):1201–10. doi: 10.1210/endo.130.3.1347006
135. Wong AO, Chang JP, Peter RE. Characterization of D1 receptors mediating dopamine-stimulated growth hormone release from pituitary cells of the goldfish, carassius auratus. Endocrinology (1993) 133(2):577–84. doi: 10.1210/endo.133.2.8102094
136. Kwong P, Chang JP. Somatostatin inhibition of growth hormone release in goldfish: Possible targets of intracellular mechanisms of action. Gen Comp Endocrinol (1997) 108(3):446–56. doi: 10.1006/gcen.1997.6995
137. Marchant TA, Fraser RA, Andrews PC, Peter RE. The influence of mammalian and teleost somatostatins on the secretion of growth-hormone from goldfish (Carassius-auratus l) pituitary fragments in vitro. Regul Peptides (1987) 17(1):41–52. doi: 10.1016/0167-0115(87)90031-0
138. Marchant TA, Peter RE. Seasonal variations in body growth rates and circulating levels of growth hormone in the goldfish, carassius auratus. J Exp Zool (1986) 237(2):231–9. doi: 10.1002/jez.1402370209
139. Yunker WK, Chang JP. Somatostatin actions on a protein kinase c-dependent growth hormone secretagogue cascade. Mol Cell Endocrinol (2001) 175(1-2):193–204. doi: 10.1016/S0303-7207(01)00386-0
140. Yunker WK, Chang JP. Somatostatin-14 actions on dopamine- and pituitary adenylate cyclase-activating polypeptide-evoked Ca2+ signals and growth hormone secretion. J Neuroendocrinol (2004) 16(8):684–94. doi: 10.1111/j.1365-2826.2004.01218.x
141. Yunker WK, Smith S, Graves C, Davis PJ, Unniappan S, Rivier JE, et al. Endogenous hypothalamic somatostatins differentially regulate growth hormone secretion from goldfish pituitary somatotropes in vitro. Endocrinology (2003) 144(9):4031–41. doi: 10.1210/en.2003-0439
142. Yunker L, Wong C. Norepinephrine regulation of growth hormone release from goldfish pituitary cells. II. intracellular sites of action. J Neuroendocrinol (2000) 12(4):323–33. doi: 10.1046/j.1365-2826.2000.00456.x
143. Kamijo M, Kojima K, Maruyama K, Konno N, Motohashi E, Ikegami T, et al. Neuropeptide y in tiger puffer (takifugu rubripes): Distribution, cloning, characterization, and mRNA expression responses to prandial condition. Zool Sci (2011) 28(12):882–90. doi: 10.2108/zsj.28.882
144. Zhou Y, Liang XF, Yuan X, Li J, He Y, Fang L, et al. Neuropeptide y stimulates food intake and regulates metabolism in grass carp, ctenopharyngodon idellus. Aquaculture (2013) 380-383:52–61. doi: 10.1016/j.aquaculture.2012.11.033
145. Triantaphyllopoulos KA, Cartas D, Miliou H. Factors influencing GH and IGF-I gene expression on growth in teleost fish: How can aquaculture industry benefit? Rev Aquaculture (2020) 12(3):1637–62. doi: 10.1111/raq.12402
146. Deane EE, Woo NYS. Modulation of fish growth hormone levels by salinity, temperature, pollutants and aquaculture related stress: A review. Rev Fish Biol Fisheries (2009) 19(1):97–120. doi: 10.1007/s11160-008-9091-0
147. Boeuf G, Falcon J. Photoperiod and growth in fish. Vie Milieu/Life Environment (2001) 51(4):247–66.
148. Boeuf G, Le Bail P-Y. Does light have an influence on fish growth? Aquaculture (1999) 177(1):129–52. doi: 10.1016/S0044-8486(99)00074-5
149. Taylor JF, Migaud H, Porter MJR, Bromage NR. Photoperiod influences growth rate and plasma insulin-like growth factor-I levels in juvenile rainbow trout, oncorhynchus mykiss. Gen Comp Endocrinol (2005) 142(1):169–85. doi: 10.1016/j.ygcen.2005.02.006
150. Björnsson BT. The biology of salmon growth hormone: From daylight to dominance. Fish Physiol Biochem (1997) 17:9–24. doi: 10.1023/A:1007712413908
151. Björnsson BT, Taranger GL, Hansen T, Stefansson SO, Haux C. The interrelation between photoperiod, growth-hormone, and sexual-maturation of adult Atlantic salmon (Salmo-salar). Gen Comp Endocrinol (1994) 93(1):70–81. doi: 10.1006/gcen.1994.1009
152. Nordgarden U, Björnsson BT, Hansen T. Developmental stage of Atlantic salmon parr regulates pituitary GH secretion and parr–smolt transformation. Aquaculture (2007) 264(1):441–8. doi: 10.1016/j.aquaculture.2006.12.040
153. Nordgarden U, Hansen T, Hemre G-I, Sundby A, Björnsson BT. Endocrine growth regulation of adult Atlantic salmon in seawater: The effects of light regime on plasma growth hormone, insulin-like growth factor-I, and insulin levels. Aquaculture (2005) 250(3):862–71. doi: 10.1016/j.aquaculture.2005.05.030
154. Imsland AK, Foss A, Roth B, Stefansson SO, Vikingstad E, Pedersen S, et al. Plasma insulin-like growth factor-I concentrations and growth in juvenile halibut (Hippoglossus hippoglossus): Effects of photoperiods and feeding regimes. Comp Biochem Physiol - A Mol Integr Physiol (2008) 151(1):66–70. doi: 10.1016/j.cbpa.2008.05.179
155. Falcón J, Migaud H, Muñoz-Cueto JA, Carrillo M. Current knowledge on the melatonin system in teleost fish. Gen Comp Endocrinol (2010) 165(3):469–82. doi: 10.1016/j.ygcen.2009.04.026
156. Björnsson BT, Hemre G-I, Bjørnevik M, Hansen T. Photoperiod regulation of plasma growth hormone levels during induced smoltification of underyearling Atlantic salmon. Gen Comp Endocrinol (2000) 119(1):17–25. doi: 10.1006/gcen.2000.7439
157. Pérez-Sánchez J, Marti-Palanca H, Le Bail PY. Seasonal-changes in circulating growth-hormone (Gh), hepatic gh-binding and plasma insulin-like growth-Factor-I immunoreactivity in a marine fish, gilthead Sea bream, sparus-aurata. Fish Physiol Biochem (1994) 13(3):199–208. doi: 10.1007/BF00004358
158. Young G, Björnsson BT, Prunet P, Lin RJ, Bern HA. Smoltification and seawater adaptation in coho salmon (Oncorhynchus kisutch): Plasma prolactin, growth hormone, thyroid hormones, and cortisol. Gen Comp Endocrinol (1989) 74(3):335–45. doi: 10.1016/S0016-6480(89)80029-2
159. McCormick SD, Moriyama S, Björnsson BT. Low temperature limits photoperiod control of smolting in Atlantic salmon through endocrine mechanisms. Am J Physiology-Regulatory Integr Comp Physiol (2000) 278(5):R1352–R61. doi: 10.1152/ajpregu.2000.278.5.R1352
160. McCormick SD, Shrimpton JM, Moriyama S, Bjoürnsson BRT. Effects of an advanced temperature cycle on smolt development and endocrinology indicate that temperature is not a zeitgeber for smolting in Atlantic salmon. J Exp Biol (2002) 205(22):3553–60. doi: 10.1242/jeb.205.22.3553
161. Beckman BR. Perspectives on concordant and discordant relations between insulin-like growth factor 1 (IGF1) and growth in fishes. Gen Comp Endocrinol (2011) 170(2):233–52. doi: 10.1016/j.ygcen.2010.08.009
162. Reindl KM, Sheridan MA. Peripheral regulation of the growth hormone-insulin-like growth factor system in fish and other vertebrates. Comp Biochem Physiol - A Mol Integr Physiol (2012) 163(3-4):231–45. doi: 10.1016/j.cbpa.2012.08.003
163. Crawford DL, Powers DA. Molecular basis of evolutionary adaptation at the lactate dehydrogenase-b locus in the fish fundulus heteroclitus. Proc Natl Acad Sci (1989) 86(23):9365–9. doi: 10.1073/pnas.86.23.9365
164. Lin JJ, Somero GN. Temperature-dependent changes in expression of thermostable and thermolabile isozymes of cytosolic malate dehydrogenase in the eurythermal goby fish gillichthys mirabilis. Physiol Zool (1995) 68(1):114–28. doi: 10.1086/physzool.68.1.30163921
165. Seddon WL. Mechanisms of temperature acclimation of the channel catfish ictalurus punctatus: Isozymes and quantitative changes. Comp Biochem Physiol A. (1997) 118(3):813–20. doi: 10.1016/S0300-9629(97)87356-2
166. Gabillard JC, Yao K, Vandeputte M, Guttierez J, Le Bail PY. Differential expression of two GH receptor mRNAs following temperature change in rainbow trout (Oncorhynchus mykiss). J Endocrinol (2006) 190(1):29–37. doi: 10.1677/joe.1.06695
167. Purohit GK, Mahanty A, Suar M, Sharma AP, Mohanty BP, Mohanty S. Investigating hsp gene expression in liver of Channa striatus under heat stress for understanding the upper thermal acclimation. BioMed Res Int (2014) 2014:381719. doi: 10.1155/2014/381719
168. Mizanur RM, Yun H, Moniruzzaman M, Ferreira F, K-w K, Bai SC. Effects of feeding rate and water temperature on growth and body composition of juvenile Korean rockfish, sebastes schlegeli (Hilgendorf 1880). Asian-Australas J Anim Sci (2014) 27(5):690–9. doi: 10.5713/ajas.2013.13508
169. Swift DR, Pickford GE. Seasonal variations in the hormone content of the pituitary gland of the perch, perca fluviatilis l. Gen Comp Endocrinol (1965) 5(3):354–65. doi: 10.1016/0016-6480(65)90060-2
170. Farbridge KJ, Burke MG, Leatherland JF. Seasonal changes in the structure of the adenohypophysis of the brown bullhead (Ictalurus nebulosus LeSeur). Cytobios (1985) 44:49–66.
171. Duan C, Plisetskaya EM, Dickhoff WW. Expression of insulin-like growth factor I in normally and abnormally developing coho salmon (Oncorhynchus kisutch). Endocrinology (1995) 136(2):446–52. doi: 10.1210/endo.136.2.7835275
172. Barrett BA, McKeown BA. Plasma growth hormone levels in salmo gairdneri: Studies on temperature and the exercise intensity/duration relationship. Comp Biochem Physiol Part A: Physiol (1989) 94(4):791–4. doi: 10.1016/0300-9629(89)90635-X
173. Mingarro M, de Celis SVR, Astola A, Pendon C, Valdivia MM, Perez-Sanchez J. Endocrine mediators of seasonal growth in gilthead sea bream (Sparus aurata): The growth hormone and somatolactin paradigm. Gen Comp Endocrinol (2002) 128(2):102–11. doi: 10.1016/S0016-6480(02)00042-4
174. Silverstein JT, Shearer KD, Dickhoff WW, Plisetskaya EM. Effects of growth and fatness on sexual development of chinook salmon (Oncorhynchus tshawytscha) parr. Can J Fisheries Aquat Sci (1998) 55(11):2376–82. doi: 10.1139/f98-111
175. Figueroa J, Martín RS, Flores C, Grothusen H, Kausel G. Seasonal modulation of growth hormone mRNA and protein levels in carp pituitary: Evidence for two expressed genes. J Comp Physiol B (2005) 175(3):185–92. doi: 10.1007/s00360-005-0474-4
176. Arakawa E, Kaneko T, Tsukamoto K, Hirano T. Immunocytochemical detection of prolactin and growth hormone cells in the pituitary during early development of the Japanese eel, Anguilla japonica. Zool Sci (1992) 9:1061–6.
177. Ayson FG, Kaneko T, Hasegawa S, Hirano T. Differential expression of two prolactin and growth hormone genes during early development of tilapia (Oreochromis mossambicus) in fresh water and seawater: Implications for possible involvement in osmoregulation during early life stages. Gen Comp Endocrinol (1994) 95(1):143–52. doi: 10.1006/gcen.1994.1111
178. Deane EE, Kelly SP, Collins PM, Woo NYS. Larval development of silver Sea bream (Sparus sarba): Ontogeny of RNA-DNA ratio, GH, IGF-I, and na+-K+-ATPase. Mar Biotechnol (2003) 5(1):79–91. doi: 10.1007/s10126-002-0052-7
179. Gabillard JC, Weil C, Rescan PY, Navarro I, Gutierrez J, Le Bail PY. Does the GH/IGF system mediate the effect of water temperature on fish growth? a review. Cybium (2005) 29(2):107–17. doi: 10.26028/cybium/2005-292-001
180. Gabillard JC, Weil C, Rescan PY, Navarro I, Gutiérrez J, Le Bail PY. Environmental temperature increases plasma GH levels independently of nutritional status in rainbow trout (Oncorhynchus mykiss). Gen Comp Endocrinol (2003) 133(1):17–26. doi: 10.1016/S0016-6480(03)00156-4
181. Gabillard J-C, Rescan P-Y, Fauconneau B, Weil C, Le Bail P-Y. Effect of temperature on gene expression of the Gh/Igf system during embryonic development in rainbow trout (Oncorhynchus mykiss). J Exp Zool Part A: Comp Exp Biol (2003) 298A(2):134–42. doi: 10.1002/jez.a.10280
182. Ricordel M-J, Smal J, Le Bail P-Y. Application of a recombinant cichlid growth hormone radioimmunoassay to measure native GH in tilapia (Oreochromis niloticus) bred at different temperatures. Aquat Living Resour (1995) 8(2):153–60. doi: 10.1051/alr:1995012
183. Gabillard JC, Weil C, Rescan PY, Navarro I, Gutiérrez J, Le Bail PY. Effects of environmental temperature on IGF1, IGF2, and IGF type I receptor expression in rainbow trout (Oncorhynchus mykiss). Gen Comp Endocrinol (2003) 133(2):233–42. doi: 10.1016/S0016-6480(03)00167-9
184. Pérez-Sánchez J, Le Bail P-Y. Growth hormone axis as marker of nutritional status and growth performance in fish. Aquaculture (1999) 177(1):117–28. doi: 10.1016/S0044-8486(99)00073-3
185. Handeland SO, Berge Å, Björnsson BT, Lie Ø, Stefansson SO. Seawater adaptation by out-of-season Atlantic salmon (Salmo salar l.) smolts at different temperatures. Aquaculture (2000) 181(3):377–96. doi: 10.1016/S0044-8486(99)00241-0
186. Collins GM, Ball AS, Qin JG, Bowyer JN, Stone DAJ. Effect of alternative lipids and temperature on growth factor gene expression in yellowtail kingfish (Seriola lalandi). Aquaculture Res (2014) 45(7):1236–45. doi: 10.1111/are.12067
187. Moriyama S, Ayson FG, Kawauchi H. Growth regulation by insulin-like growth factor-I in fish. Biosci Biotech Biochem (2000) 64(8):1553–62. doi: 10.1271/bbb.64.1553
188. Shamblott MJ, Chen TT. Age-related and tissue-specific levels of five forms of insulin-like growth factor mRNA in a teleost. Mol Mar Biol Biotechnol (1993) 2(6):351–61.
189. Duan CM. Nutritional and developmental regulation of insulin-like growth factors in fish. J Nutr (1998) 128(2):306S–14S. doi: 10.1093/jn/128.2.306S
190. Duguay SJ, Swanson P, Dickhoff WW. Differential expression and hormonal regulation of alternatively spliced IGF-I mRNA transcripts in salmon. J Mol Endocrinol (1994) 12(1):25–37. doi: 10.1677/jme.0.0120025
191. Niu PD, Perez-Sanchez J, Le Bail PY. Development of a protein binding assay for teleost insulin-like growth factor (IGF)-like: Relationship between growth hormone (GH) and IGF-like in the blood of rainbow trout (Oncorhynchus mykiss). Fish Physiol Biochem (1993) 11:381–91. doi: 10.1007/BF00004588
192. Pérez-Sánchez J, Marti-Palanca H, Le Bail PY. Homologous growth-hormone (Gh) binding in gilthead Sea bream (Sparus-aurata) - effect of fasting and refeeding on hepatic gh-binding and plasma somatomedin-like immunoreactivity. J Fish Biol (1994) 44(2):287–301. doi: 10.1111/j.1095-8649.1994.tb01206.x
193. Duguay SJ, Lai-Zhang J, Steiner DF, Funkenstein B, Chan SJ. Developmental and tissue-regulated expression of IGF-I and IGF-II mRNAs in sparus aurata. J Mol Endocrinol (1996) 16(2):123–32. doi: 10.1677/jme.0.0160123
194. Picha ME, Silverstein JT, Borski RJ. Discordant regulation of hepatic IGF-I mRNA and circulating IGF-I during compensatory growth in a teleost, the hybrid striped bass (Morone chrysops × morone saxatilis). Gen Comp Endocrinol (2006) 147(2):196–205. doi: 10.1016/j.ygcen.2005.12.020
195. Picha ME, Turano MJ, Tipsmark CK, Borski RJ. Regulation of endocrine and paracrine sources of igfs and gh receptor during compensatory growth in hybrid striped bass (Morone chrysops×Morone saxatilis). J Endocrinol (2008) 199(1):81–94. doi: 10.1677/JOE-07-0649
196. Saera-Vila A, Calduch-Giner JA, Perez-Sanchez J. Co-Expression of IGFs and GH receptors (GHRs) in gilthead sea bream (Sparus aurata l.): Sequence analysis of the GHR-flanking region. J Endocrinol (2007) 194(2):361–72. doi: 10.1677/JOE-06-0229
197. Benedito-Palos L, Ballester-Lozano GF, Simó P, Karalazos V, Ortiz Á, Calduch-Giner J, et al. Lasting effects of butyrate and low FM/FO diets on growth performance, blood haematology/biochemistry and molecular growth-related markers in gilthead sea bream (Sparus aurata). Aquaculture (2016) 454:8–18. doi: 10.1016/j.aquaculture.2015.12.008
198. Benedito-Palos L, Saera-Vila A, Calduch-Giner J-A, Kaushik S, Pérez-Sánchez J. Combined replacement of fish meal and oil in practical diets for fast growing juveniles of gilthead sea bream (Sparus aurata l.): Networking of systemic and local components of GH/IGF axis. Aquaculture (2007) 267(1):199–212. doi: 10.1016/j.aquaculture.2007.01.011
199. Ballester-Lozano GF, Benedito-Palos L, Estensoro I, Sitjà-Bobadilla A, Kaushik S, Pérez-Sánchez J. Comprehensive biometric, biochemical and histopathological assessment of nutrient deficiencies in gilthead sea bream fed semi-purified diets. Br J Nutr (2015) 114(5):713–26. doi: 10.1017/S0007114515002354
200. Comesaña S, Velasco C, Conde-Sieira M, Míguez JM, Soengas JL, Morais S. Feeding stimulation ability and central effects of intraperitoneal treatment of l-leucine, l-valine, and l-proline on amino acid sensing systems in rainbow trout: Implication in food intake control. Front Physiol (2018) 9(AUG). doi: 10.3389/fphys.2018.01209
201. Conde-Sieira M, Soengas JL. Nutrient sensing systems in fish: Impact on food intake regulation and energy homeostasis. Front Neurosci (2017) 10:603. doi: 10.3389/fnins.2016.00603
202. Delgado MJ, Cerdá-Reverter JM, Soengas JL. Hypothalamic integration of metabolic, endocrine, and circadian signals in fish: Involvement in the control of food intake. Front Neurosci (2017) 11(354). doi: 10.3389/fnins.2017.00354
203. Raka F, Farr S, Kelly J, Stoianov A, Adeli K. Metabolic control via nutrient-sensing mechanisms: role of taste receptors and the gut-brain neuroendocrine axis. Am J Physiol Endocrinol Metab (2019) 317(4):E559–E72. doi: 10.1152/ajpendo.00036.2019
204. Ozaki Y, Fukada H, Kazeto Y, Adachi S, Hara A, Yamauchi K. Molecular cloning and characterization of growth hormone receptor and its homologue in the Japanese eel (Anguilla japonica). Comp Biochem Physiol Part B: Biochem Mol Biol (2006) 143(4):422–31. doi: 10.1016/j.cbpb.2005.12.016
205. Saera-Vila A, Calduch-Giner J-A, Pérez-Sánchez J. Duplication of growth hormone receptor (GHR) in fish genome: Gene organization and transcriptional regulation of GHR type I and II in gilthead sea bream (Sparus aurata). Gen Comp Endocrinol (2005) 142(1):193–203. doi: 10.1016/j.ygcen.2004.11.005
206. Sakamoto T, McCormick SD. Prolactin and growth hormone in fish osmoregulation. Gen Comp Endocrinol (2006) 147(1):24–30. doi: 10.1016/j.ygcen.2005.10.008
207. Boeuf G, Payan P. How should salinity influence fish growth? Comp Biochem Physiol Part C: Toxicol Pharmacol (2001) 130(4):411–23. doi: 10.1016/S1532-0456(01)00268-X
208. Wood AW, Duan CM, Bern HA. Insulin-like growth factor signaling in fish. Int Rev Cytol - Survey Cell Biol (2005) 243:215–85. doi: 10.1016/S0074-7696(05)43004-1
209. Yada T, McCormick SD, Hyodo S. Effects of environmental salinity, biopsy, and GH and IGF-I administration on the expression of immune and osmoregulatory genes in the gills of Atlantic salmon (Salmo salar). Aquaculture (2012) 362-363:177–83. doi: 10.1016/j.aquaculture.2010.12.029
210. McCormick SD. Endocrine control of osmoregulation in teleost fish. Am Zoologist (2001) 41(4):781–94. doi: 10.1093/icb/41.4.781
211. Reinecke M. Influences of the environment on the endocrine and paracrine fish growth hormone–insulin-like growth factor-I system. J Fish Biol (2010) 76(6):1233–54. doi: 10.1111/j.1095-8649.2010.02605.x
212. Bolton JP, Collie NL, Kawauchi H, Hirano T. Osmoregulatory actions of growth hormone in rainbow trout (Salmo gairdneri). J Endocrinol (1987) 112(1):63–8. doi: 10.1677/joe.0.1120063
213. Sakamoto T, Hirano T. Expression of insulin-like growth factor I gene in osmoregulatory organs during seawater adaptation of the salmonid fish: Possible mode of osmoregulatory action of growth hormone. Proc Natl Acad Sci (1993) 90(5):1912–6. doi: 10.1073/pnas.90.5.1912
214. Sakamoto T, Shepherd BS, Madsen SS, Nishioka RS, Siharath K, Richman NH 3rd, et al. Osmoregulatory actions of growth hormone and prolactin in an advanced teleost. Gen Comp Endocrinol (1997) 106(1):95–101. doi: 10.1006/gcen.1996.6854
215. Mancera JM, McCormick SD. Evidence for growth hormone/insulin-like growth factor I axis regulation of seawater acclimation in the euryhaline teleost fundulus heteroclitus. Gen Comp Endocrinol (1998) 111(2):103–12. doi: 10.1006/gcen.1998.7086
216. Mancera JM, McCormick SD. Influence of cortisol, growth hormone, insulin-like growth factor I and 3,3′,5-triiodo-l-thyronine on hypoosmoregulatory ability in the euryhaline teleost fundulus heteroclitus. Fish Physiol Biochem (1999) 21(1):25–33. doi: 10.1023/A:1007737924339
217. McCormick SD. Effects of growth hormone and insulin-like growth factor I on salinity tolerance and gill na+, k+-ATPase in Atlantic salmon (Salmo salar): Interaction with cortisol. Gen Comp Endocrinol (1996) 101(1):3–11. doi: 10.1006/gcen.1996.0002
218. Xu B, Miao H, Zhang P, Li D. Osmoregulatory actions of growth hormone in juvenile tilapia (Oreochromis niloticus). Fish Physiol Biochem (1997) 17(1):295–301. doi: 10.1023/A:1007750022878
219. Shrimpton JM, Devlin RH, McLean E, Byatt JC, Donaldson EM, Randall DJ. Increases in gill cytosolic corticosteroid receptor abundance and saltwater tolerance in juvenile coho salmon (Oncorhynchus kisutch) treated with growth hormone and placental lactogen. Gen Comp Endocrinol (1995) 98(1):1–15. doi: 10.1006/gcen.1995.1039
220. Sangiao-Alvarellos S, Miguez JM, Soengas JL. Actions of growth hormone on carbohydrate metabolism and osmoregulation of rainbow trout (Oncorhynchus mykiss). Gen Comp Endocrinol (2005) 141(3):214–25. doi: 10.1016/j.ygcen.2005.01.007
221. Watanabe WO, Ellingson LJ, Wicklund RI, Olla BL. Effects of salinity on growth, food consumption and conversion in juvenile, monosex male Florida red tilapia. the second international symposium on tilapia in aquaculture. ICLARM Conf Proc (Philippines) (1988), 515–23.
222. Watanabe WO, Ernst DH, Chasar MP, Wicklund RI, Olla BL. The effects of temperature and salinity on growth and feed utilization of juvenile, sex-reversed male Florida red tilapia cultured in a recirculating system. Aquaculture (1993) 112(4):309–20. doi: 10.1016/0044-8486(93)90392-C
223. Liu B, Guo HY, Zhu KC, Guo L, Liu BS, Zhang N, et al. Growth, physiological, and molecular responses of golden pompano trachinotus ovatus (Linnaeus, 1758) reared at different salinities. Fish Physiol Biochem (2019) 45:1879–93. doi: 10.1007/s10695-019-00684-9
224. Zhang YT, Huang S, Qiu HT, Li Z, Mao Y, Hong WS, et al. Optimal salinity for rearing Chinese black sleeper (Bostrychus sinensis) fry. Aquaculture (2017) 476:37–43. doi: 10.1016/j.aquaculture.2017.04.004
225. Mattioli CC, Takata R, de Oliveira Paes Leme F, Costa DC, Melillo Filho R, de Souza e Silva W, et al. The effects of acute and chronic exposure to water salinity on juveniles of the carnivorous freshwater catfish lophiosilurus alexandri. Aquaculture (2017) 481:255–66. doi: 10.1016/j.aquaculture.2017.08.016
226. Lambert Y, Dutil J-D, Munro JL. Effects of intermediate and low salinity conditions on growth rate and food conversion of Atlantic cod (Gadus morhua). Can J Fisheries Aquat Sci (1994) 51:1569–76. doi: 10.1139/f94-155
227. Gaumet F, Boeuf G, Severe A, Le Roux A, Mayer-Gostan N. Effects of salinity on the ionic balance and growth of juvenile turbot. J Fish Biol (1995) 47(5):865–76. doi: 10.1111/j.1095-8649.1995.tb06008.x
228. Dutil JD, Lambert Y, Boucher E. Does higher growth rate in Atlantic cod (Gadus morhua) at low salinity result from lower standard metabolic rate or increased protein digestibility? Can J Fisheries Aquat Sci (1997) 54(S1):99–103. doi: 10.1139/f96-148
229. Imsland AKD, Foss A, Gunnarsson S, Berntssen MHG, Fitzgerald RD, Bonga SEW, et al. The interaction of temperature and salinity on growth and food conversion in juvenile turbot (Scophthalmus maximus). Aquaculture (2001) 198:353–67. doi: 10.1016/S0044-8486(01)00507-5
230. da Hora MDSC, Joyeux J-C, Rodrigues RV, de Sousa-Santos LP, Gomes LC, Tsuzuki MY. Tolerance and growth of the longsnout seahorse hippocampus reidi at different salinities. Aquaculture (2016) 463:1–6. doi: 10.1016/j.aquaculture.2016.05.003
231. Drennon K, Moriyama S, Kawauchi H, Small B, Silverstein J, Parhar I, et al. Development of an enzyme-linked immunosorbent assay for the measurement of plasma growth hormone (GH) levels in channel catfish (Ictalurus punctatus): Assessment of environmental salinity and GH secretogogues on plasma GH levels. Gen Comp Endocrinol (2003) 133(3):314–22. doi: 10.1016/S0016-6480(03)00194-1
232. Shepherd BS, Drennon K, Johnson J, Nichols JW, Playle RC, Singer TD, et al. Salinity acclimation affects the somatotropic axis in rainbow trout. Am J Physiology-Regulatory Integr Comp Physiol (2005) 288(5):R1385–R95. doi: 10.1152/ajpregu.00443.2004
233. Tomy S, Chang Y-M, Chen Y-H, Cao J-C, Wang T-P, Chang C-F. Salinity effects on the expression of osmoregulatory genes in the euryhaline black porgy acanthopagrus schlegeli. Gen Comp Endocrinol (2009) 161(1):123–32. doi: 10.1016/j.ygcen.2008.12.003
234. Laiz-Carrión R, Fuentes J, Redruello B, Guzmán JM, Martín del Río MP, Power D, et al. Expression of pituitary prolactin, growth hormone and somatolactin is modified in response to different stressors (salinity, crowding and food-deprivation) in gilthead sea bream sparus auratus. Gen Comp Endocrinol (2009) 162(3):293–300. doi: 10.1016/j.ygcen.2009.03.026
235. Link K, Berishvili G, Shved N, D’Cotta H, Baroiller J-F, Reinecke M, et al. Seawater and freshwater challenges affect the insulin-like growth factors IGF-I and IGF-II in liver and osmoregulatory organs of the tilapia. Mol Cell Endocrinol (2010) 327(1):40–6. doi: 10.1016/j.mce.2010.05.011
236. Bertucci JI, Tovar MO, Blanco AM, Gomez-Requeni P, Unniappan S, Canosa LF. Influence of water salinity on genes implicated in somatic growth, lipid metabolism and food intake in pejerrey (Odontesthes bonariensis). Comp Biochem Physiol B Biochem Mol Biol (2017) 210:29–38. doi: 10.1016/j.cbpb.2017.05.005
237. Nguyen PTH, Do HTT, Mather PB, Hurwood DA. Experimental assessment of the effects of sublethal salinities on growth performance and stress in cultured tra catfish (Pangasianodon hypophthalmus). Fish Physiol Biochem (2014) 40(6):1839–48. doi: 10.1007/s10695-014-9972-1
238. Guan B, Hu W, Zhang T, Wang Y, Zhu Z. Metabolism traits of a’ll-fish’ growth hormone transgenic common carp (Cyprinus carpio l.). Aquaculture (2008) 284(1):217–23. doi: 10.1016/j.aquaculture.2008.06.028
239. Higgs DA, Sutton JN, Kim H, Oakes JD, Smith J, Biagi C, et al. Influence of dietary concentrations of protein, lipid and carbohydrate on growth, protein and energy utilization, body composition, and plasma titres of growth hormone and insulin-like growth factor-1 in non-transgenic and growth hormone transgenic coho salmon, oncorhynchus kisutch (Walbaum). Aquaculture (2009) 286(1-2):127–37. doi: 10.1016/j.aquaculture.2008.08.036
240. Leggatt RA, Devlin RH, Farrell AP, Randall DJ. Oxygen uptake of growth hormone transgenic coho salmon during starvation and feeding. J Fish Biol (2003) 62(5):1053–66. doi: 10.1046/j.1095-8649.2003.00096.x
241. Meirelles MG, Nornberg BF, da Silveira TLR, Kütter MT, Castro CG, Ramirez JRB, et al. Growth hormone overexpression induces hyperphagia and intestinal morphophysiological adaptations to improve nutrient uptake in zebrafish. Front Physiol (2021) 12:723853. doi: 10.3389/fphys.2021.723853
242. Pollock MS, Clarke LMJ, Dubé MG. The effects of hypoxia on fishes: From ecological relevance to physiological effects. Environ Rev (2007) 15(NA):1–14. doi: 10.1139/a06-006
243. Abdel-Tawwab M, Monier MN, Hoseinifar SH, Faggio C. Fish response to hypoxia stress: Growth, physiological, and immunological biomarkers. Fish Physiol Biochem (2019) 45(3):997–1013. doi: 10.1007/s10695-019-00614-9
244. Thorarensen H, Gústavsson A, Mallya Y, Gunnarsson S, Árnason J, Arnarson I, et al. The effect of oxygen saturation on the growth and feed conversion of Atlantic halibut (Hippoglossus hippoglossus l.). Aquaculture (2010) 309(1):96–102. doi: 10.1016/j.aquaculture.2010.08.019
245. Mallya YJ. The effects of dissolved oxygen on fish growth in aquaculture. Reykjavik, Iceland: Fisheries Training Programme Final project The United Nations University (2007). p. 30.
246. Kestemont P, Baras E. Environmental Factors and Feed Intake: Mechanisms and Interactions. In: Houlihan D., Boujard T. M. J editors. Food Intake in Fish. Oxford, UK: Blackwell Science Ltd (2001). p. 131–56.
247. Fitzgibbon QP, Strawbridge A, Seymour RS. Metabolic scope, swimming performance and the effects of hypoxia in the mulloway, argyrosomus japonicus (Pisces: Sciaenidae). Aquaculture (2007) 270(1):358–68. doi: 10.1016/j.aquaculture.2007.04.038
248. Pörtner HO. Oxygen- and capacity-limitation of thermal tolerance: A matrix for integrating climate-related stressor effects in marine ecosystems. J Exp Biol (2010) 213(6):881–93. doi: 10.1242/jeb.037523
249. Bera A, Sawant PB, Dasgupta S, Chadha NK, Sawant BT, Pal AK. Diel cyclic hypoxia alters plasma lipid dynamics and impairs reproduction in goldfish (Carassius auratus). Fish Physiol Biochem (2017) 43(6):1677–88. doi: 10.1007/s10695-017-0401-0
250. Tsadik G, Kutty M. Influence of ambient oxygen on feeding and growth of the tilapia oreochromis niloticus (Linnaeus). Port Harcourt, Nigeria: FAO (1987).
251. Pichavant K, Person-Le-Ruyet J, Le Bayon N, Sévère A, Le Roux A, Quéméner L, et al. Effects of hypoxia on growth and metabolism of juvenile turbot. Aquaculture (2000) 188(1):103–14. doi: 10.1016/S0044-8486(00)00316-1
252. Pichavant K, Person-Le-Ruyet J, Bayon NL, Severe A, Roux AL, Boeuf G. Comparative effects of long-term hypoxia on growth, feeding and oxygen consumption in juvenile turbot and European sea bass. J Fish Biol (2001) 59(4):875–83. doi: 10.1111/j.1095-8649.2001.tb00158.x
253. Foss A, Evensen TH, Øiestad V. Effects of hypoxia and hyperoxia on growth and food conversion efficiency in the spotted wolffish anarhichas minor (Olafsen). Aquaculture Res (2002) 33(6):437–44. doi: 10.1046/j.1365-2109.2002.00693.x
254. Remen M, Oppedal F, Torgersen T, Imsland AK, Olsen RE. Effects of cyclic environmental hypoxia on physiology and feed intake of post-smolt Atlantic salmon: Initial responses and acclimation. Aquaculture (2012) 326-329:148–55. doi: 10.1016/j.aquaculture.2011.11.036
255. Brett JR, Blackburn JM. Oxygen requirements for growth of young coho (Oncorhynchus kisutch) and sockeye (O. nerka) salmon at 15 °C. Can J Fisheries Aquat Sci (1981) 38(4):399–404. doi: 10.1139/f81-056
256. Person-Le Ruyet J, Lacut A, Le Bayon N, Le Roux A, Pichavant K, Quéméner L. Effects of repeated hypoxic shocks on growth and metabolism of turbot juveniles. Aquat Living Resources (2003) 16(1):25–34. doi: 10.1016/S0990-7440(02)00002-5
257. Tran-Duy A, Schrama JW, van Dam AA, Verreth JAJ. Effects of oxygen concentration and body weight on maximum feed intake, growth and hematological parameters of Nile tilapia, oreochromis niloticus. Aquaculture (2008) 275(1):152–62. doi: 10.1016/j.aquaculture.2007.12.024
258. Abdel-Tawwab M, Hagras AE, Elbaghdady HAM, Monier MN. Dissolved oxygen level and stocking density effects on growth, feed utilization, physiology, and innate immunity of Nile tilapia, oreochromis niloticus. J Appl Aquaculture (2014) 26(4):340–55. doi: 10.1080/10454438.2014.959830
259. Abdel-Tawwab M, Hagras AE, Elbaghdady HAM, Monier MN. Effects of dissolved oxygen and fish size on Nile tilapia, oreochromis niloticus (L.): growth performance, whole-body composition, and innate immunity. Aquaculture Int (2015) 23(5):1261–74. doi: 10.1007/s10499-015-9882-y
260. Li M, Wang X, Qi C, Li E, Du Z, Qin JG, et al. Metabolic response of Nile tilapia (Oreochromis niloticus) to acute and chronic hypoxia stress. Aquaculture (2018) 495:187–95. doi: 10.1016/j.aquaculture.2018.05.031
261. Randolph KN, Clemens HP. Some factors influencing the feeding behavior of channel catfish in culture ponds. Trans Am Fisheries Society (1976) 105(6):718–24. doi: 10.1577/1548-8659(1976)105<718:SFITFB>2.0.CO;2
262. Buentello JA, Gatlin DM, Neill WH. Effects of water temperature and dissolved oxygen on daily feed consumption, feed utilization and growth of channel catfish (Ictalurus punctatus). Aquaculture (2000) 182(3):339–52. doi: 10.1016/S0044-8486(99)00274-4
263. Jobling M. he influence of environmental temperature on growth and conversion efficiency in fish. Causes of observed variations in fish growth. ICES (1995) 4:1–25.
264. Papoutsoglou SE, Tziha G. Blue tilapia (Oreochromis aureus) growth rate in relation to dissolved oxygen concentration under recirculated water conditions. Aquacultural Eng (1996) 15(3):181–92. doi: 10.1016/0144-8609(95)00013-5
265. Thetmeyer H, Waller U, Black KD, Inselmann S, Rosenthal H. Growth of European sea bass (Dicentrarchus labrax l.) under hypoxic and oscillating oxygen conditions. Aquaculture (1999) 174(3):355–67. doi: 10.1016/S0044-8486(99)00028-9
266. Pickering AD, Pottinger TG, Sumpter JP, Carragher JF, Le Bail PY. Effects of acute and chronic stress on the levels of circulating growth hormone in the rainbow trout, oncorhynchus mykiss. Gen Comp Endocrinol (1991) 83(1):86–93. doi: 10.1016/0016-6480(91)90108-I
267. Martos-Sitcha JA, Bermejo-Nogales A, Calduch-Giner JA, Pérez-Sánchez J. Gene expression profiling of whole blood cells supports a more efficient mitochondrial respiration in hypoxia-challenged gilthead sea bream (Sparus aurata). Front Zool (2017) 14(1):34. doi: 10.1186/s12983-017-0220-2
268. Almeida DV, Bianchini A, Marins LF. Growth hormone overexpression generates an unfavorable phenotype in juvenile transgenic zebrafish under hypoxic conditions. Gen Comp Endocrinol (2013) 194:102–9. doi: 10.1016/j.ygcen.2013.08.017
269. McKenzie DJ, Martínez R, Morales A, Acosta J, Morales R, Taylor EW, et al. Effects of growth hormone transgenesis on metabolic rate, exercise performance and hypoxia tolerance in tilapia hybrids. J Fish Biol (2003) 63(2):398–409. doi: 10.1046/j.1095-8649.2003.00162.x
270. Maures TJ, Duan C. Structure, developmental expression, and physiological regulation of zebrafish IGF binding protein-1. Endocrinology (2002) 143(7):2722–31. doi: 10.1210/endo.143.7.8905
271. Breves JP, Tipsmark CK, Stough BA, Seale AP, Flack BR, Moorman BP, et al. Nutritional status and growth hormone regulate insulin-like growth factor binding protein (igfbp) transcripts in Mozambique tilapia. Gen Comp Endocrinol (2014) 207:66–73. doi: 10.1016/j.ygcen.2014.04.034
272. Garcia de la Serrana D, Macqueen DJ. Insulin-like growth factor-binding proteins of teleost fishes. Front Endocrinol (2018) 9. doi: 10.3389/fendo.2018.00080
273. Kajimura S, Aida K, Duan C. Insulin-like growth factor-binding protein-1 (IGFBP-1) mediates hypoxia-induced embryonic growth and developmental retardation. Proc Natl Acad Sci (2005) 102(4):1240–5. doi: 10.1073/pnas.0407443102
274. Denver RJ. Structural and functional evolution of vertebrate neuroendocrine stress systems. Ann Ny Acad Sci (2009) 1163(1):1–16. doi: 10.1111/j.1749-6632.2009.04433.x
275. Pierce AL, Breves JP, Moriyama S, Hirano T, Grau EG. Differential regulation of Igf1 and Igf2 mRNA levels in tilapia hepatocytes: Effects of insulin and cortisol on GH sensitivity. J Endocrinol (2011) 211(2):201–10. doi: 10.1530/JOE-10-0456
276. Faught E, Vijayan MM. Mechanisms of cortisol action in fish hepatocytes. Comp Biochem Physiol Part B: Biochem Mol Biol (2016) 199:136–45. doi: 10.1016/j.cbpb.2016.06.012
277. Uchida K, Yoshikawa-Ebesu JS, Kajimura S, Yada T, Hirano T, Gordon Grau E. In vitro effects of cortisol on the release and gene expression of prolactin and growth hormone in the tilapia, oreochromis mossambicus. Gen Comp Endocrinol (2004) 135(1):116–25. doi: 10.1016/j.ygcen.2003.08.010
278. Conde-Sieira M, Chivite M, Míguez JM, Soengas JL. Stress effects on the mechanisms regulating appetite in teleost fish. Front Endocrinol (2018) 9(OCT):631. doi: 10.3389/fendo.2018.00631
279. Picha ME, Turano MJ, Beckman BR, Borski RJ. Endocrine biomarkers of growth and applications to aquaculture: A minireview of growth hormone, insulin-like growth factor (IGF)-I, and IGF-binding proteins as potential growth indicators in fish. North Am J Aquaculture (2008) 70(2):196–211. doi: 10.1577/A07-038.1
280. Madison BN, Tavakoli S, Kramer S, Bernier NJ. Chronic cortisol and the regulation of food intake and the endocrine growth axis in rainbow trout. J Endocrinol (2015) 226(2):103–19. doi: 10.1530/JOE-15-0186
281. Janzen WJ, Duncan CA, Riley LG. Cortisol treatment reduces ghrelin signaling and food intake in tilapia, oreochromis mossambicus. Domest Anim Endocrinol (2012) 43(3):251–9. doi: 10.1016/j.domaniend.2012.04.003
282. Authman MM. Use of fish as bio-indicator of the effects of heavy metals pollution. J Aquaculture Res Dev (2015) 06:328. doi: 10.4172/2155-9546.1000328
283. Jezierska B, Ługowska K, Witeska M. The effects of heavy metals on embryonic development of fish (a review). Fish Physiol Biochem (2008) 35:625–40. doi: 10.1007/s10695-008-9284-4
284. Jones I, Kille P, Sweeney G. Cadmium delays growth hormone expression during rainbow trout development. J Fish Biol (2001) 59:1015–22. doi: 10.1111/j.1095-8649.2001.tb00168.x
285. Sajjad S, Malik H, Saeed L, Chaudhary A. Changes in growth hormone and cortisol profile due to lead induced toxicity in labeo rohita. Turkish J Fisheries Aquat Sci (2018) 18:921–6. doi: 10.4194/1303-2712-v18_7_10
286. Cai Y, Yin Y, Li Y, Guan L, Zhang P, Qin Y, et al. Cadmium exposure affects growth performance, energy metabolism, and neuropeptide expression in carassius auratus gibelio. Fish Physiol Biochem (2020) 46:187–97. doi: 10.1007/s10695-019-00709-3
287. Li ZH, Li P, Wu Y. Effects of temperature fluctuation on endocrine disturbance of grass carp ctenopharyngodon idella under mercury chloride stress. Chemosphere (2021) 263:128137. doi: 10.1016/j.chemosphere.2020.128137
288. Saera-Vila A, Calduch-Giner JA, Prunet P, Pérez-Sánchez J. Dynamics of liver GH/IGF axis and selected stress markers in juvenile gilthead sea bream (Sparus aurata) exposed to acute confinement: Differential stress response of growth hormone receptors. Comp Biochem Physiol Part A: Mol Integr Physiol (2009) 154(2):197–203. doi: 10.1016/j.cbpa.2009.06.004
289. Szymkowicz DB, Sims KC, Castro NM, Bridges WC, Bain LJ. Embryonic-only arsenic exposure in killifish (Fundulus heteroclitus) reduces growth and alters muscle IGF levels one year later. Aquat Toxicol (2017) 186:1–10. doi: 10.1016/j.aquatox.2017.02.020
290. Valenzuela GE, Perez A, Navarro M, Romero A, Figueroa J, Kausel G. Differential response of two somatolactin genes to zinc or estrogen in pituitary of cyprinus carpio. Gen Comp Endocrinol (2015) 215:98–105. doi: 10.1016/j.ygcen.2014.09.015
291. Ekinci D, Ceyhun SB, Aksakal E, Erdoğan O. IGF and GH mRNA levels are suppressed upon exposure to micromolar concentrations of cobalt and zinc in rainbow trout white muscle. Comp Biochem Physiol Part C: Toxicol Pharmacol (2011) 153(3):336–41. doi: 10.1016/j.cbpc.2010.12.004
292. Kuz’mina VV, Kulivatskaya EA. Activity of peptidases and glycosidases: Effect of light deprivation and heavy metals on the effects of serotonin and cholecystokinin in the carp cyprinus carpio. J Ichthyol (2018) 58(2):269–73. doi: 10.1134/S0032945218010083
293. Saleh HA, Gaber HS, El-Khayat HMM, Abdel-Motleb A, Mohammed WAA, Okasha H. Influences of dietary supplementation of chlorella vulgaris and spirulina platensis on growth-related genes expression and antioxidant enzymes in oreochromis niloticus fish exposed to heavy metals. Aquaculture Stud (2021) 22:AQUAST793. doi: 10.4194/AQUAST793
294. Rodrigues JP, Duarte AC, Santos-Echeandía J, Rocha-Santos T. Significance of interactions between microplastics and POPs in the marine environment: A critical overview. TrAC Trends Analytical Chem (2019) 111:252–60. doi: 10.1016/j.trac.2018.11.038
295. Ge M, Wang X, Yang G, Wang Z, Li Z, Zhang X, et al. Persistent organic pollutants (POPs) in deep-sea sediments of the tropical western pacific ocean. Chemosphere (2021) 277:130267. doi: 10.1016/j.chemosphere.2021.130267
296. Wenning RJ, Martello L. POPs in marine and freshwater environments. In O’Sullivan G., Sandau C. editors. Environmental Forensics for Persistent Organic Pollutants Amsterdam, The Netherlands: Elsevier (2014), 357–90. doi: 10.1016/B978-0-444-59424-2.00008-6
297. Olisah C, Adams JB, Rubidge G. The state of persistent organic pollutants in south African estuaries: A review of environmental exposure and sources. Ecotoxicology Environ Safety (2021) 219:112316. doi: 10.1016/j.ecoenv.2021.112316
298. Kendall M, Hamilton RS, Watt J, Williams ID. Characterisation of selected speciated organic compounds associated with particulate matter in London. Atmospheric Environment (2001) 35:2483–95. doi: 10.1016/S1352-2310(00)00431-3
299. Lyche JL, Nourizadeh-Lillabadi R, Almaas C, Stavik B, Berg V, Skare JU, et al. Natural mixtures of persistent organic pollutants (POP) increase weight gain, advance puberty, and induce changes in gene expression associated with steroid hormones and obesity in female zebrafish. J Toxicol Environ Health - Part A: Curr Issues (2010) 73:1032–57. doi: 10.1080/15287394.2010.481618
300. Vasseur P, Cossu-Leguille C. Linking molecular interactions to consequent effects of persistent organic pollutants (POPs) upon populations. Chemosphere (2006) 62:1033–42. doi: 10.1016/j.chemosphere.2005.05.043
301. Johnson LL, Anulacion BF, Arkoosh MR, Burrows DG, da Silva DAM, Dietrich JP, et al. Effects of legacy persistent organic pollutants (POPs) in fish–current and future challenges. Fish Physiol (2013) 33:53–140. doi: 10.1016/B978-0-12-398254-4.00002-9
302. Covert SA, Shoda ME, Stackpoole SM, Stone WW. Pesticide mixtures show potential toxicity to aquatic life in U.S. streams, water years 2013–2017. Sci Total Environ (2020) 745:141285. doi: 10.1016/j.scitotenv.2020.141285
303. Gyawali K. Pesticide uses and its effects on public health and environment. J Health Promotion (2018) 6:28–36. doi: 10.3126/jhp.v6i0.21801
304. Al-Mamun A. Pesticide degradations, residues and environmental concerns. In: Khan MS, Rahman MS, editors. Pesticide residue in foods: Sources, management, and control. Cham: Springer (2017). p. 87–102.
305. Huang T, Jiang H, Zhao Y, He J, Cheng H, Martyniuk CJ. A comprehensive review of 1, 2, 4-triazole fungicide toxicity in zebrafish (Danio rerio): A mitochondrial and metabolic perspective. Sci Total Environ (2022) 809:151177. doi: 10.1016/j.scitotenv.2021.151177
306. Huang T, Zhao Y, He J, Cheng H, Martyniuk CJ. Endocrine disruption by azole fungicides in fish: A review of the evidence. Sci Total Environment (2022) 822:153412. doi: 10.1016/j.scitotenv.2022.153412
307. Martyniuk CJ, Mehinto AC, Denslow ND. Organochlorine pesticides: Agrochemicals with potent endocrine-disrupting properties in fish. Mol Cell Endocrinol (2020) 507:110764. doi: 10.1016/j.mce.2020.110764
308. Singh PB, Nigam SK, Singh R, Singh HK. Tissue concentration of organochlorines, organophosphate and plasma cortisol in captured fish of polluted river gomti atJaunpur during post-monsoon season. J Ecophysiol Occup Health (2011) 11:181–97. doi: 10.18311/jeoh/2011/2263
309. Eder KJ, Clifford MA, Hedrick RP, Köhler H-R, Werner I. Expression of immune-regulatory genes in juvenile Chinook salmon following exposure to pesticides and infectious hematopoietic necrosis virus (IHNV). Fish Shellfish Immunol (2008) 25(5):508–16. doi: 10.1016/j.fsi.2008.07.003
310. Hontela A, Habibi HR. Personal care products in the aquatic environment: A case study on the effects of triclosan in fish. Fish Physiol (2013) 33:411–37. doi: 10.1016/B978-0-12-398254-4.00008-X
311. Hanson AM, Kittilson JD, Sheridan MA. Environmental estrogens inhibit the expression of insulin-like growth factor mRNAs in rainbow trout in vitro by altering activation of the JAK-STAT, AKT-PI3K, and ERK signaling pathways. Gen Comp Endocrinol (2021) 309:113792. doi: 10.1016/j.ygcen.2021.113792
312. Alcaraz AJG, Mikulás K, Pote D, Park B, Shekh K, Ewald J, et al. Assessing the toxicity of 17α-ethinylestradiol in rainbow trout using a 4-day transcriptomics benchmark dose (BMD) embryo assay. Environ Sci Technol (2021) 55:10608–18. doi: 10.1021/acs.est.1c02401
313. Breves JP, Duffy TA, Einarsdottir IE, Björnsson BT, McCormick SD. In vivo effects of 17α-ethinylestradiol, 17β-estradiol and 4-nonylphenol on insulin-like growth-factor binding proteins (igfbps) in Atlantic salmon. Aquat Toxicol (2018) 203:28–39. doi: 10.1016/j.aquatox.2018.07.018
314. Shved N, Berishvili G, D'Cotta H, Baroiller JF, Segner H, Eppler E, et al. Ethinylestradiol differentially interferes with IGF-I in liver and extrahepatic sites during development of male and female bony fish. J Endocrinol (2007) 195:513–23. doi: 10.1677/JOE-07-0295
315. Shved N, Berishvili G, Baroiller JF, Segner H, Reinecke M. Environmentally relevant concentrations of 17alpha-ethinylestradiol (EE2) interfere with the growth hormone (GH)/insulin-like growth factor (IGF)-I system in developing bony fish. Toxicological Sci (2008) 106:93–102. doi: 10.1093/toxsci/kfn150
316. Lin XW, Volkoff H, Narnaware Y, Bernier NJ, Peyon P, Peter RE. Brain regulation of feeding behavior and food intake in fish. Comp Biochem Physiol a-Molecular Integr Physiol (2000) 126(4):415–34. doi: 10.1016/S1095-6433(00)00230-0
317. Volkoff H, Unniappan S, Kelly SP. The endocrine regulation of food intake. In: Bernier NJ, van der Kraak G, Farrell AP, Brauner CJ, editors. Fish neuroendocrinology. fish physiology, 1st ed. London, UK: Elsevier (2009). p. 421–65.
318. Bertucci JI, Blanco AM, Canosa LF, Unniappan S. Estradiol and testosterone modulate the tissue-specific expression of ghrelin, ghs-r, goat and nucb2 in goldfish. Gen Comp Endocrinol (2016) 228:17–23. doi: 10.1016/j.ygcen.2016.01.006
319. Barría C, Brandts I, Tort L, Oliveira M, Teles M. Effect of nanoplastics on fish health and performance: A review. Mar pollut Bulletin (2020) 151:110791. doi: 10.1016/j.marpolbul.2019.110791
320. Chen G, Li Y, Wang J. Occurrence and ecological impact of microplastics in aquaculture ecosystems. Chemosphere (2021) 274:129989. doi: 10.1016/j.chemosphere.2021.129989
321. Zolotova N, Kosyreva A, Dzhalilova D, Fokichev N, Makarova O. Harmful effects of the microplastic pollution on animal health: a literature review. Peer J (2022) 10:e13503. doi: 10.7717/peerj.13503
322. Burns EE, Boxall ABA. Microplastics in the aquatic environment: Evidence for or against adverse impacts and major knowledge gaps. Environ Toxicol Chem (2018) 37:2776–96. doi: 10.1002/etc.4268
323. Chatterjee A, Maity S, Banerjee S, Dutta S, Adhikari M, Guchhait R, et al. Toxicological impacts of nanopolystyrene on zebrafish oocyte with insight into the mechanism of action: An expression-based analysis. Sci Total Environ (2022) 830:154796. doi: 10.1016/j.scitotenv.2022.154796
324. Hoseini SM, Khosraviani K, Hosseinpour Delavar F, Arghideh M, Zavvar F, Hoseinifar SH, et al. Hepatic transcriptomic and histopathological responses of common carp, cyprinus carpio, to copper and microplastic exposure. Mar pollut Bull (2022) 175:113401. doi: 10.1016/j.marpolbul.2022.113401
325. Wang J, Zheng M, Lu L, Li X, Zhang Z, Ru S. Adaptation of life-history traits and trade-offs in marine medaka (Oryzias melastigma) after whole life-cycle exposure to polystyrene microplastics. J Hazardous Materials (2021) 414:125537. doi: 10.1016/j.jhazmat.2021.125537
326. Zheng JL, Chen X, Peng LB, Wang D, Zhu QL, Li J, et al. Particles rather than released Zn2+ from ZnO nanoparticles aggravate microplastics toxicity in early stages of exposed zebrafish and their unexposed offspring. J Hazardous Materials (2022) 424:127589. doi: 10.1016/j.jhazmat.2021.127589
327. Pedersen AF, Meyer DN, Petriv AMV, Soto AL, Shields JN, Akemann C, et al. Nanoplastics impact the zebrafish (Danio rerio) transcriptome: Associated developmental and neurobehavioral consequences. Environ Pollution (2020) 266:115090. doi: 10.1016/j.envpol.2020.115090
328. Kim SA, Kim L, Kim TH, An YJ. Assessing the size-dependent effects of microplastics on zebrafish larvae through fish lateral line system and gut damage. Mar pollut Bulletin (2022) 185:114279. doi: 10.1016/j.marpolbul.2022.114279
329. Luo H, Du Q, Zhong Z, Xu Y, Peng J. Protein-coated microplastics corona complex: An underestimated risk of microplastics. Sci Total Environ (2022) 851:157948. doi: 10.1016/j.scitotenv.2022.157948
330. Siddiqui S, Dickens JM, Cunningham BE, Hutton SJ, Pedersen EI, Harper B, et al. Internalization, reduced growth, and behavioral effects following exposure to micro and nano tire particles in two estuarine indicator species. Chemosphere (2022) 296:133934. doi: 10.1016/j.chemosphere.2022.133934
331. Zhao Y, Qiao R, Zhang S, Wang G. Metabolomic profiling reveals the intestinal toxicity of different length of microplastic fibers on zebrafish (Danio rerio). J Hazardous Materials (2021) 403:123663. doi: 10.1016/j.jhazmat.2020.123663
332. Im J, Eom HJ, Choi J. Effect of early-life exposure of polystyrene microplastics on behavior and DNA methylation in later life stage of zebrafish. Arch Environ Contamination Toxicol (2022) 82:558–68. doi: 10.1007/s00244-022-00924-9
333. DiBona E, Pinnell LJ, Heising-Huang A, Geist S, Turner JW, Seemann FA. Holistic assessment of polyethylene fiber ingestion in larval and juvenile Japanese medaka fish. Front Physiol (2021) 12. doi: 10.3389/fphys.2021.668645
334. FAO. Deep-ocean climate change impacts on habitat, fish and fisheries. Levin L, Baker M, Thompson A, editors. Rome: FAO (2019). p. 186.
335. Cominassi L, Moyano M, Claireaux G, Howald S, Mark FC, Zambonino-Infante J-L, et al. Combined effects of ocean acidification and temperature on larval and juvenile growth, development and swimming performance of European sea bass (Dicentrarchus labrax). PLOS ONE (2019) 14(9):e0221283. doi: 10.1371/journal.pone.0221283
336. Servili A, Lévêque E, Mouchel O, Devergne J, Lebigre C, Roussel S, et al. Ocean acidification alters the acute stress response of a marine fish. Sci Total Environment (2023) 858:159804. doi: 10.1016/j.scitotenv.2022.159804
337. Tegomo FA, Zhong Z, Njomoue AP, Okon SU, Ullah S, Gray NA, et al. Experimental studies on the impact of the projected ocean acidification on fish survival, health, growth, and meat quality; black Sea bream (Acanthopagrus schlegelii), physiological and histological studies. Animals (2021) 11:3119. doi: 10.3390/ani11113119
338. Carney Almroth B, Bresolin de Souza K, Jönsson E, Sturve J. Oxidative stress and biomarker responses in the Atlantic halibut after long term exposure to elevated CO2 and a range of temperatures. Comp Biochem Physiol Part - B: Biochem Mol Biol (2019) 238:110321. doi: 10.1016/j.cbpb.2019.110321
339. Brimijoin S, Chen VP, Pang YP, Geng L, Gao Y. Physiological roles for butyrylcholinesterase: A BChE-ghrelin axis. Chemico-Biological Interactions (2016) 259:271–5. doi: 10.1016/j.cbi.2016.02.013
340. Wang L, Sun F, Wen Y, Yue GH. Effects of ocean acidification on transcriptomes in Asian seabass juveniles. Mar Biotechnol (2021) 23:445–55. doi: 10.1007/s10126-021-10036-5
341. Reinecke M, Bjornsson BT, Dickhoff WW, McCormick SD, Navarro I, Power DM, et al. Growth hormone and insulin-like growth factors in fish: Where we are and where to go. Gen Comp Endocrinol (2005) 142(1-2):20–4. doi: 10.1016/j.ygcen.2005.01.016
342. Björnsson BT, Stefansson SO, McCormick SD. Environmental endocrinology of salmon smoltification. Gen Comp Endocrinol (2011) 170(2):290–8. doi: 10.1016/j.ygcen.2010.07.003
343. Shao YT, Chang FY, Fu WC, Yan HY. Acidified seawater suppresses insulin-like growth factor I mRNA expression and reduces growth rate of juvenile orange-spotted groupers, epinephelus coioides (Hamilton, 1822). Aquaculture Res (2016) 47:721–31. doi: 10.1111/are.12533
344. Bruzzio H. Effects of ocean acidification and hypoxia on stress and growth hormone responses in juvenile blue rockfish (Sebastes mystinus). [Master's Thesis]. Monterey Bay: California State University (2022).
345. Madeira D, Araújo JE, Vitorino R, Costa PM, Capelo JL, Vinagre C, et al. Molecular plasticity under ocean warming: Proteomics and fitness data provides clues for a better understanding of the thermal tolerance in fish. Front Physiol (2017) 8:825. doi: 10.3389/fphys.2017.00825
346. Logan CA, Buckley BA, Podrabsky JE, Stillman JH, Tomanek L. Transcriptomic responses to environmental temperature in eurythermal and stenothermal fishes. J Exp Biol (2015) 218:1915–24. doi: 10.1242/jeb.114397
347. Logan CA, Somero GN. Effects of thermal acclimation on transcriptional responses to acute heat stress in the eurythermal fish gillichthys mirabilis (Cooper). Am J Physiol - Regul Integr Comp Physiol (2011) 300:1373–83. doi: 10.1152/ajpregu.00689.2010
348. Madeira D, Narciso L, Cabral HN, Vinagre C, Diniz MS. Influence of temperature in thermal and oxidative stress responses in estuarine fish. Comp Biochem Physiol Part A: Mol Integr Physiol (2013) 166:237–43. doi: 10.1016/j.cbpa.2013.06.008
349. Tomanek L. Variation in the heat shock response and its implication for predicting the effect of global climate change on species' biogeographical distribution ranges and metabolic costs. J Exp Biol (2010) 213:971–9. doi: 10.1242/jeb.038034
350. Anastasiadi D, Díaz N, Piferrer F. Small ocean temperature increases elicit stage-dependent changes in DNA methylation and gene expression in a fish, the European sea bass. Sci Rep (2017) 7:12401. doi: 10.1038/s41598-017-10861-6
351. Politis SN, Mazurais D, Servili A, Zambonino-Infante J-L, Miest JJ, Sørensen SR, et al. Temperature effects on gene expression and morphological development of European eel, Anguilla anguilla larvae. PLOS ONE (2017) 12(8):e0182726. doi: 10.1371/journal.pone.0182726
352. Madeira D, Araújo JE, Vitorino R, Capelo JL, Vinagre C, Diniz MS. Ocean warming alters cellular metabolism and induces mortality in fish early life stages: A proteomic approach. Environ Res (2016) 148:164–76. doi: 10.1016/j.envres.2016.03.030
353. Yada T. Growth hormone and fish immune system. Gen Comp Endocrinol (2007) 152(2-3):353–8. doi: 10.1016/j.ygcen.2007.01.045
354. Pörtner H-O, Bock C, Mark FC. Oxygen- and capacity-limited thermal tolerance: bridging ecology and physiology. J Exp Biol (2017) 220(15):2685–96. doi: 10.1242/jeb.134585
355. Deutsch C, Ferrel A, Seibel B, Pörtner H-O, Huey Raymond B. Climate change tightens a metabolic constraint on marine habitats. Science (2015) 348(6239):1132–5. doi: 10.1126/science.aaa1605
356. Clarke TM, Wabnitz CCC, Striegel S, Frölicher TL, Reygondeau G, Cheung WWL. Aerobic growth index (AGI): An index to understand the impacts of ocean warming and deoxygenation on global marine fisheries resources. Prog Oceanogr (2021) 195:102588. doi: 10.1016/j.pocean.2021.102588
357. Lavin CP, Gordó-Vilaseca C, Costello MJ, Shi Z, Stephenson F, Grüss A. Warm and cold temperatures limit the maximum body length of teleost fishes across a latitudinal gradient in Norwegian waters. Environ Biol Fish. (2022) 105:1415–29. doi: 10.1007/s10641-022-01270-4
358. Riley LG, Hirano T, Grau EG. Disparate effects of gonadal steroid hormones on plasma and liver mRNA levels of insulin-like growth factor-I and vitellogenin in the tilapia, oreochromis mossambicus. Fish Physiol Biochem (2002) 26(3):223–30. doi: 10.1023/A:1026209502696
359. Riley LG, Richman NH 3rd, Hirano T, Gordon Grau E. Activation of the growth hormone/insulin-like growth factor axis by treatment with 17 alpha-methyltestosterone and seawater rearing in the tilapia, oreochromis mossambicus. Gen Comp Endocrinol (2002) 127(3):285–92. doi: 10.1016/S0016-6480(02)00051-5
360. Higgs DA, Fagerlund UHM, Eales JG, Mcbride JR. Application of thyroid and steroid-hormones as anabolic agents in fish culture. Comp Biochem Physiol B-Biochem Mol Biol (1982) 73(1):143–76. doi: 10.1016/0305-0491(82)90206-1
361. Larsen DA, Beckman BR, Cooper KA, Barrett D, Johnston M, Swanson P, et al. Assessment of high rates of precocious Male maturation in a spring Chinook salmon supplementation hatchery program. Trans Am Fisheries Society (2004) 133(1):98. doi: 10.1577/T03-031
362. Larsen DA, Shimizu M, Cooper KA, Swanson P, Dickhoff WW. Androgen effects on plasma GH, IGF-I, and 41-kDa IGFBP in coho salmon (Oncorhynchus kisutch). Gen Comp Endocrinol (2004) 139(1):29–37. doi: 10.1016/j.ygcen.2004.07.007
363. Holloway AC, Leatherland JF. Effect of gonadal steroid hormones on plasma growth hormone concentrations in sexually immature rainbow trout, oncorhynchus mykiss. Gen Comp Endocrinol (1997) 105(2):246–54. doi: 10.1006/gcen.1996.6826
364. Reinecke M. Insulin-like growth factors and fish reproduction. Biol Reproduction (2010) 82(4):656–61. doi: 10.1095/biolreprod.109.080093
365. Hull KL, Harvey S. GH as a co-gonadotropin: The relevance of correlative changes in GH secretion and reproductive state. J Endocrinol (2002) 172(1):1–19. doi: 10.1677/joe.0.1720001
366. Hull KL, Harvey S. Growth hormone and reproduction: A review of endocrine and Autocrine/Paracrine interactions. Int J Endocrinol (2014) 2014:234014. doi: 10.1155/2014/234014
367. Wendelaar Bonga SE. The stress response in fish. Physiol Rev (1997) 77(3):591–625. doi: 10.1152/physrev.1997.77.3.591
368. Barton BA. Stress in fishes: A diversity of responses with particular reference to changes in circulating Corticosteroids1. Integr Comp Biol (2002) 42(3):517–25. doi: 10.1093/icb/42.3.517
369. Mommsen TP, Vijayan MM, Moon TW. Cortisol in teleosts: Dynamics, mechanisms of action, and metabolic regulation. Rev Fish Biol Fisheries (1999) 9(3):211–68. doi: 10.1023/A:1008924418720
370. Peterson BC, Small BC. Effects of exogenous cortisol on the GH/IGF-I/IGFBP network in channel catfish. Domest Anim Endocrinol (2005) 28(4):391–404. doi: 10.1016/j.domaniend.2005.01.003
Keywords: climate change, environmental pollutants, growth hormone, IGF, somatic growth, feeding, teleost
Citation: Canosa LF and Bertucci JI (2023) The effect of environmental stressors on growth in fish and its endocrine control. Front. Endocrinol. 14:1109461. doi: 10.3389/fendo.2023.1109461
Received: 27 November 2022; Accepted: 13 March 2023;
Published: 30 March 2023.
Edited by:
Takayoshi Ubuka, International Cancer Laboratory Co., Ltd., JapanReviewed by:
José Luis Soengas, University of Vigo, SpainRussell J Borski, North Carolina State University, United States
Copyright © 2023 Canosa and Bertucci. This is an open-access article distributed under the terms of the Creative Commons Attribution License (CC BY). The use, distribution or reproduction in other forums is permitted, provided the original author(s) and the copyright owner(s) are credited and that the original publication in this journal is cited, in accordance with accepted academic practice. No use, distribution or reproduction is permitted which does not comply with these terms.
*Correspondence: Luis Fabián Canosa, bGNhbm9zYUBpbnRlY2guZ292LmFy; Juan Ignacio Bertucci, aWduYWNpb2JlcnR1Y2NpQGhvdG1haWwuY29t