- Marine Science Institute, The University of Texas at Austin, Port Aransas, TX, United States
Parkinson’s disease (PD) is the second most common neurodegenerative disease worldwide, and current treatment options are unsatisfactory on the long term. Several studies suggest a potential neuroprotective action by female hormones, especially estrogens. The potential role of progestogens, however, is less defined, and no studies have investigated the potential involvement of membrane progesterone receptors (mPRs). In the present study, the putative neuroprotective role for mPRs was investigated in SH-SY5Y cells, using two established pharmacological treatments for cellular PD models, 6-hydroxydopamine (6-OHDA) and 1-methyl-4-phenylpyridinium (MPP+). Our results show that both the physiologic agonist progesterone and the specific mPR agonist Org OD 02-0 were effective in reducing SH-SY5Y cell death induced by 6-OHDA and MPP+, whereas the nuclear PR agonist promegestone (R5020) and the GABAA receptor agonist muscimol were ineffective. Experiments performed with gene silencing technology and selective pharmacological agonists showed that mPRα is the isoform responsible for the neuroprotective effects we observed. Further experiments showed that the PI3K-AKT and MAP kinase signaling pathways are involved in the mPRα-mediated progestogen neuroprotective action in SH-SY5Y cells. These findings suggest that mPRα could play a neuroprotective role in PD pathology and may be a promising target for the development of therapeutic strategies for PD prevention or management.
Introduction
Parkinson’s disease (PD) is the second most common neurodegenerative disorder. Due to aging of the world’s population, its prevalence is predicted to reach 9 million people worldwide by 2030 (1, 2). The disease is characterized by the progressive degeneration of dopaminergic neurons in the substantia nigra projecting to the striatum, the presence of Lewy bodies, and microgliosis (3, 4). Current treatments aim to control the motor symptoms, with no effects on the progression of the degenerative process, and lose efficacy with time (5). PD incidence and prevalence is higher in men than women (6–8). Moreover, based on epidemiological studies, it has been proposed that PD symptoms may have a later onset in women, possibly because of the neuroprotective effect of female sex hormones earlier in life (9–11). Therefore, it has been hypothesized that female sex hormones may play a neuroprotective role. Both epidemiological studies and animal models support a possible neuroprotective role of estrogens (9–15). Several in vivo animal studies, with different PD experimental models have also shown that progesterone (P4) may have neuroprotective action in dopaminergic neurons. In particular, several studies showed that P4 can be neuroprotective in the presence of 1-methyl-4-phenyl-1,2,3,6-tetrahydropyridine (MPTP), in a common in vivo model of PD damage, if administered before MPTP treatment (16–19). There is some evidence suggesting that P4 may also be potentially used as a treatment after the onset of PD symptoms. Indeed, P4 counteracts MPTP toxicity in male mice if administered within a short time window after the insult (20). Moreover, it bolsters dopaminergic differentiation in mouse embryonic stem cells undergoing an in vitro differentiation protocol, which may be beneficial in PD treatment (21). However, the putative neuroprotective activity of P4 in human cell models of PD and the progestogen receptors mediating this P4 action have not been investigated.
Membrane progesterone receptors (mPRs) are members of the progestin and adipoQ receptor (PAQR) family (22, 23), presenting five isoforms: mPRα/PAQR7, mPRβ/PAQR8, mPRγ/PAQR5 (24, 25), mPRδ/PAQR6 and mPRϵ/PAQR9 (26). These receptors were initially characterized in reproductive tissues, but are known to exert several activities also in non-reproductive tissues, including the nervous system (27). Moreover, mPRs have been reported to mediate progestogen neuroprotective actions in different neuronal cell line models (28–30). In particular, the specific mPR agonist Org OD 02-0 (02–0) (31) displayed neuroprotective activity in SH-SY5Y cells in a model of cell starvation (30). SH-SY5Y cells are a neuroblastoma cell line which show catecholaminergic characteristics. This cell line is widely used in PD research, with different protocols used for cell culture and different strategies employed to induce PD-like conditions (32). In this study we investigated the putative neuroprotective role of mPRα using two chemicals commonly used to mimic PD damage in cell cultures, 6-hydroxydopamine (6-OHDA) and MPTP’s active metabolite, 1-methyl-4-phenylpyridinium (MPP+) (32). mPRα neuroprotective activity to decrease cell death was examined following pharmacological activation of PD, and the specificity of mPRα’s action was confirmed with specific agonists and gene silencing studies. The intracellular mechanisms underlying the putative neuroprotective activity of mPRα were also investigated.
Materials and methods
Cell culture and pharmacological treatments
The SH-SY5Y neuronal cell line was obtained from American-type Cell Culture Collection (ATCC, Manassas, VA, USA). The medium used for cell culture was composed of 85% minimum essential medium, α modification (α-MEM, Lonza, Morristown, NJ, USA), with 2 nM L-glutamine (Gibco, Waltham, MA, USA) supplementation, and 15% fetal bovine serum (FBS, Corning Inc., Corning, NY, USA).
For the majority of experiments cells underwent overnight incubation in α-MEM supplemented with 2 nM L-glutamine (serum-free condition) before treatment for 24h with various pharmacological agents. For Western blot experiments requiring 6-OHDA pretreatment, 6-OHDA was added to the serum-free medium for the overnight incubation prior to progestogen treatment. Phosphorylation of ERK and AKT was assessed by Western blot analysis. Several time points were considered in preliminary experiments for 02-0 treatments (5,10 and 30 min, data not shown). The 10 min treatment was selected since it showed the stronger response. 6-OHDA (Tocris, Minneapolis, MN, USA) and MPP+ iodide (Sigma-Aldrich, St. Louis, MO, USA) were used to mimic PD damage in SH-SY5Y cells. The specific mPR agonist 02-0 (Axon Medchem, Groningen, Netherlands), the natural agonist P4 (Steraloids, Newport, RI, USA), the specific PR agonist R5020 (Steraloids) and the specific GABA-A receptor agonist muscimol (Tocris) were used to assess the contribution of different progestogen receptors. The specific ERK inhibitor AZD 6244 (Stemcell Technologies, Vancouver, BC, Canada), the specific PI3K inhibitor Wortmannin (EMD Millipore, Burlington, MA, USA) and the specific AKT inhibitor ML-9 (EMD Millipore) were used to investigate the activation of intracellular signaling pathways. The final concentrations to be used for treatments were either determined in a series of preliminary experiments or obtained from the literature. The different drugs were used at the following concentrations (when the concentration was obtained from the literature, a specific reference is provided in the following list): 6-OHDA 50 μM (33); MPP+ 750 nM; 02-0 100 nM (30, 34); P4 100 nM (30); R5020 100 nM (30, 34); muscimol 100 μM (35); AZD 6244 1 μM (36); Wortmannin 50 nM (34); ML-9 15 μM (34). A comparison between the concentrations used for these chemicals and their EC50/IC50 is presented in Table S1. Unless they were soluble in serum-free medium (Mus, 6-OHDA and MPP+), pharmacological treatment results were compared with those of their vehicle (Veh) solvents: EtOH (02-0, P4, R5020), DMSO (AZD 6244, Wortmannin, ML-9), or a combination of the two.
Hoechst assay
The Hoechst assay was performed as previously described (30). SH-SY-5Y cells were grown on coverslips inside multiwell plates and then underwent 20 min fixation with 4% formaldehyde (Thermo-Fisher Scientific, Waltham, MA, USA). After a rinse with phosphate buffer saline (PBS, Thermo-Fisher Scientific), cells were incubated with Hoechst 33342 (Tocris) 1 μg/mL for 5 min. The cells were then rinsed and observed with an Eclipse Ti2 microscope (Nikon, Tokyo, Japan). Cell death was assessed by changes in chromatin morphology, nuclei with compacted chromatin were counted as Hoechst positive. At least 20 images from different coverslips were acquired for each condition, with each acquired image representing a single sample. The determination of the percentage of Hoechst positive nuclei was performed with the ImageJ software (NIH, Bethesda, MD, USA).
RNA extraction and quantitative real time PCR (RT-qPCR)
The extraction of total RNA from samples was performed with TriReagent (Invitrogen, Carlsbad, CA, USA), following the manufacturer’s instructions, and quantification was performed with a Nanodrop 2000c UV spectrophotometer (Thermo-Fisher Scientific, Waltham, MA, USA). Samples were treated with the TURBO DNA-free™ kit (Thermo-Fisher Scientific) for the removal of any DNA contamination. Every sample in the RT-qPCR experiments was run in triplicate, with 10 ng of total RNA in each reaction. The RT-qPCR reaction was performed with a Mastercycler® RealPlex 2 (Eppendorf North America, Hauppauge, NY, USA), using the Luna Universal One-Step RT-qPCR kit (New England Biolabs, Ipswich, MA, USA). The primers used in these experiments have been previously published (34). Testing was performed to make sure every primer had an efficiency included between 90 and 105%. No-template controls were used as negative controls for all the experiments. The primers used are listed in Table S2.
Protein extraction and Western blot analysis
Cells were scraped in PBS, and then suspended in RIPA buffer (Sigma-Aldrich), with added protease and phosphatase inhibitors (EMD Millipore). They were then homogenized and centrifuged for 5 minutes at 800 g. The Western blot experiments were performed using the supernatant from this centrifugation. Protein samples were denatured at 100°C for 3 min. 20 μg of total protein samples were loaded into each well of SDS-PAGE 4-20% precast gels (Bio-Rad, Hercules, CA, USA) and underwent electrophoretic separation at 200 V for 50 minutes in running buffer. Proteins were then transferred to Hybond nitrocellulose membranes (Bio-Rad) by electro-blotting. The blocking phase was performed with 5% not-fat dry milk (Bio-Rad) in 0.1% PBS-Tween 20 (Sigma-Aldrich), followed by incubation with the primary antibodies diluted in the same solution. The following antibodies, all from Cell Signaling Technologies, were used: mouse anti-Akt 1:500; rabbit anti-pAkt Ser473 1:200; rabbit anti-pERK 1/2 1:500; mouse anti-ERK 1/2 1:200. After a rinse in PBS, membranes were incubated in PBS-Tween 20 0.1% containing suitable IRDye secondary antibodies. The antibodies, both from LI-COR Biosciences (Lincoln, NE, USA), were used at 1:7000 dilution: goat anti-rabbit IRDye 800; goat anti-mouse IRDye 680. After washing, the IR signal was detected with an Odyssey scanner (LI-COR Biosciences).
Silence RNA assay
Human Silencer Select PAQR7 siRNA, Silencer Select Negative Control #2 siRNA and Lipofectamine RNAiMAX were purchased from Invitrogen, and the silencing procedure was performed following the manufacturer’s instructions. Briefly, the day before the start of the silencing procedure, cells were plated at 50% confluence. The following day, the specific PAQR7 siRNA and the negative control (NC) oligo were dissolved in OptiMEM (Invitrogen). They were then mixed with Lipofectamine, previously dissolved in OptiMEM, and incubated for 20 minutes. The PAQR7 siRNA and the NC oligo were then transferred to the samples at a final concentration of 10 nM and incubated for 48 hours. The medium was then removed, and the cells were treated as described above. Silencing efficiency was tested by RT-qPCR.
Data analysis and statistics
All the described experiments were repeated at least three times. Statistical analyses was performed using Prism 5.0 (GraphPad, San Diego, CA, USA). Different statistical analyses were employed, depending on the experimental design: two-tailed unpaired Student’s t-test, one-way ANOVA, or two-way ANOVA. When a one-way ANOVA was used, single conditions were then compared among them with Tukey’s multiple comparison test. When two-way ANOVA was used, single conditions were compared by post-hoc multiple comparison of means, using the Bonferroni correction. P values < 0.05 were considered significant. All results are expressed as mean ± standard error of the mean (SEM).
Results
02-0 and P4 treatments are neuroprotective in SH-SY5Y cells
The gene expression of the nuclear P4 receptor (PR) and mPRα were determined by RT-qPCR. The average Ct value for PR was 33.82 ± 0.39, while the average Ct for mPRα was 25.90 ± 0.22. The calculated mPRα expression was 200 times higher than that of the PR in SH-SY5Y cells (Figure 1A).
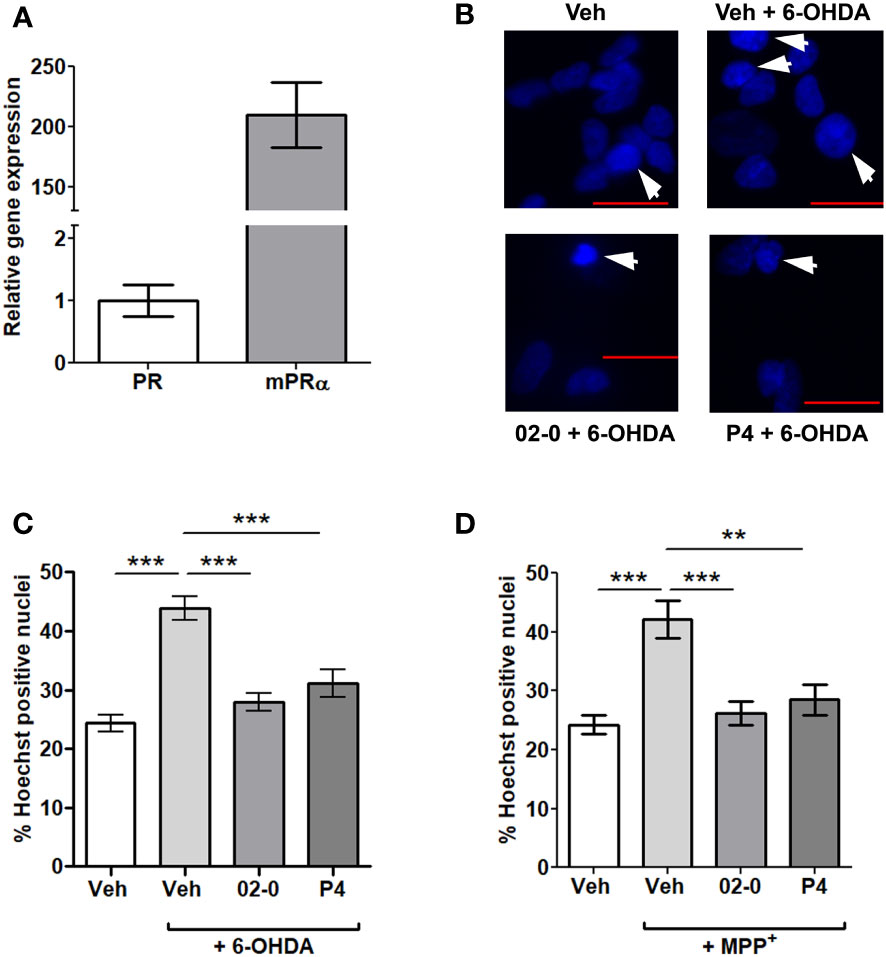
Figure 1 PR and mPRα expression and the effects of P4 and 02-0 treatments on 6-OHDA- and MPP+-mediated cell death in SH-SY5Y cells. (A) Gene expression of PR and mPRα in SH-SY5Y cells. N=6. (B) Representative images of SH-SY5Y cell nuclei labeled with Hoechst 33342 in the 6-OHDA experiment. Nuclei in which chromatin appears compacted and were counted as positive are indicated by an arrow. Scale bar: 20 μm. (C) Assessment of cell death in SH-SY5Y cells in the Hoechst assay following 24h Veh, 100 nM 02-0 and 100 nM P4 treatments in the presence or absence of 50µM 6-OHDA. N=18. One-way ANOVA results: p<0.0001. Tukey’s multiple comparisons test results: ***: p<0.001. (D) Assessment of cell death in SH-SY5Y cells following Veh, 100 nM 02-0 and 100 nM P4 24 h treatments in presence or absence of 750 nM MPP+. N=18. One-way ANOVA results: p<0.0001. Tukey’s multiple comparisons test results: **: p<0.01: ***: p<0.001.
The putative neuroprotective actions of the specific mPR agonist 02-0 and the physiological mPR ligand P4 to decrease cell death in SH-SY5Y cells were tested. 6-OHDA and MPP+ 24 h treatments significantly increased cell mortality, as determined by the Hoechst assay (Figures 1B–D). Both 100 nM 02-0 and P4 were effective in protecting SH-SY5Y cells from toxicity, significantly reducing the fraction of Hoechst- positive nuclei in cells treated with the neurotoxic chemicals to levels similar to those in Veh-treated cells not exposed to the chemicals (Figures 1C, D). Therefore, mPRs likely have a neuroprotective action in SH-SY5Y cells.
Progestogen neuroprotective activity is mediated through mPRα
We then investigated the possible contribution of different 02-0 and P4-binding receptors to the neuroprotective activity described above. Treatment with 100nM 02-0 significantly decreased cell death as observed previously. However, both the PR specific agonist R5020 (100nM) and the GABA-A receptor specific agonist muscimol (100µM) were ineffective and did not show a neuroprotective action during 6-OHDA or MPP+ 24 h incubations in the Hoechst assay, suggesting these two receptors are not involved in the neuroprotective action of P4 (Figures 2A, B). Once we determined that neuroprotection was mPR-mediated, we performed mPRα siRNA experiments to determine if the neuroprotection is mediated by this mPR isoform. The RT-qPCR assessment of silencing efficiency revealed that mPRα expression was reduced by 79.9% (Figure S1). The specific PAQR7 siRNA completely abolished the 02-0 protective effect on cell viability in presence of both neurotoxic chemicals (Figures 2C, D), which suggests that mPRα is the isoform promoting neuroprotection in SH-SY5Y cells.
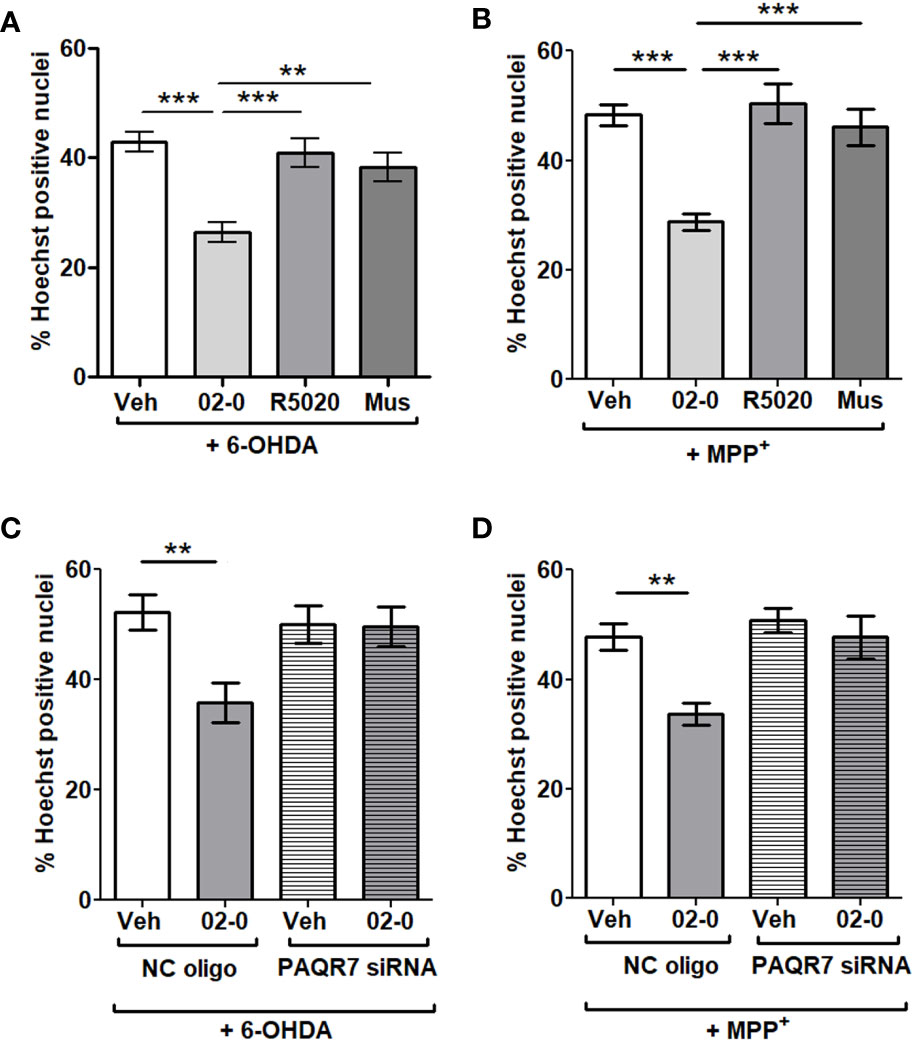
Figure 2 Investigation of the possible contribution of different progestogen receptors in P4 neuroprotection in SH-SY5Y cells (A) Assessment of cell death in SH-SY5Y cells following Veh, 100 nM 02-0, 100 nM R5020 and 100 μM Mus (muscimol) 24 h treatments in presence 50 μM 6-OHDA. N=18. One-way ANOVA results: p<0.0001. Tukey’s multiple comparisons test results: **: p<0.01: ***: p<0.001. (B) Assessment of cell death in SH-SY5Y cells following Veh, 100 nM 02-0, 100 nM R5020 and 100 μM Mus (muscimol) 24 h treatments in presence of 750 nM MPP+. N=18. ***: p<0.001. (C) Assessment of cell death in SH-SY5Y cells following Veh and 100 nM 02-0 24 h treatments in presence of 50 μM 6-OHDA and following PAQR7 (mPRα) gene silencing. N=18. Two-way ANOVA results: Interaction: p=0.0274, Treatment: p=0.0196, Silencing: p=0.1006. Bonferroni’s post-test results: **: p<0.01. (D) Assessment of cell death in SH-SY5Y cells following Veh and 100 nM 02-0 24 h treatments in presence of 750 nM MPP+ and following PAQR7 gene silencing. N=18. Two-way ANOVA results: Interaction: p=0.0514, Treatment: p=0.0033, Silencing: p=0.0036. Bonferroni’s post-test results: **: p<0.01.
mPRα differently activates the ERK and PI3K-AKT signaling pathways in SH-SY5Y cells
We next examined the signaling pathways involved in the neuroprotective activity of mPRα. We first tested the effects of 100nM 02-0 treatment of SH-SY5Y cells on two intracellular pathways often linked to mPRα activity in other human tissues (34, 37–40): the ERK and PI3K-AKT pathways. To this end, we analyzed by Western blot the effect of 02-0 treatments on the phosphorylation levels of ERK kinases 1/2 and the AKT kinase. Following 10 min treatment, 02-0 did not affect ERK phosphorylation (Figure 3A), but it significantly increased AKT phosphorylation (Figure 3B). We then repeated these experiments after pretreating SH-SY5Y cells overnight with 6-OHDA to model a PD condition. In this experiment, phosphorylation of both ERK and AKT was significantly increased after 02-0 treatment (Figures 3C, D). Therefore, both these signaling pathways can be activated by mPRα in these cells and may be involved in its neuroprotective action.
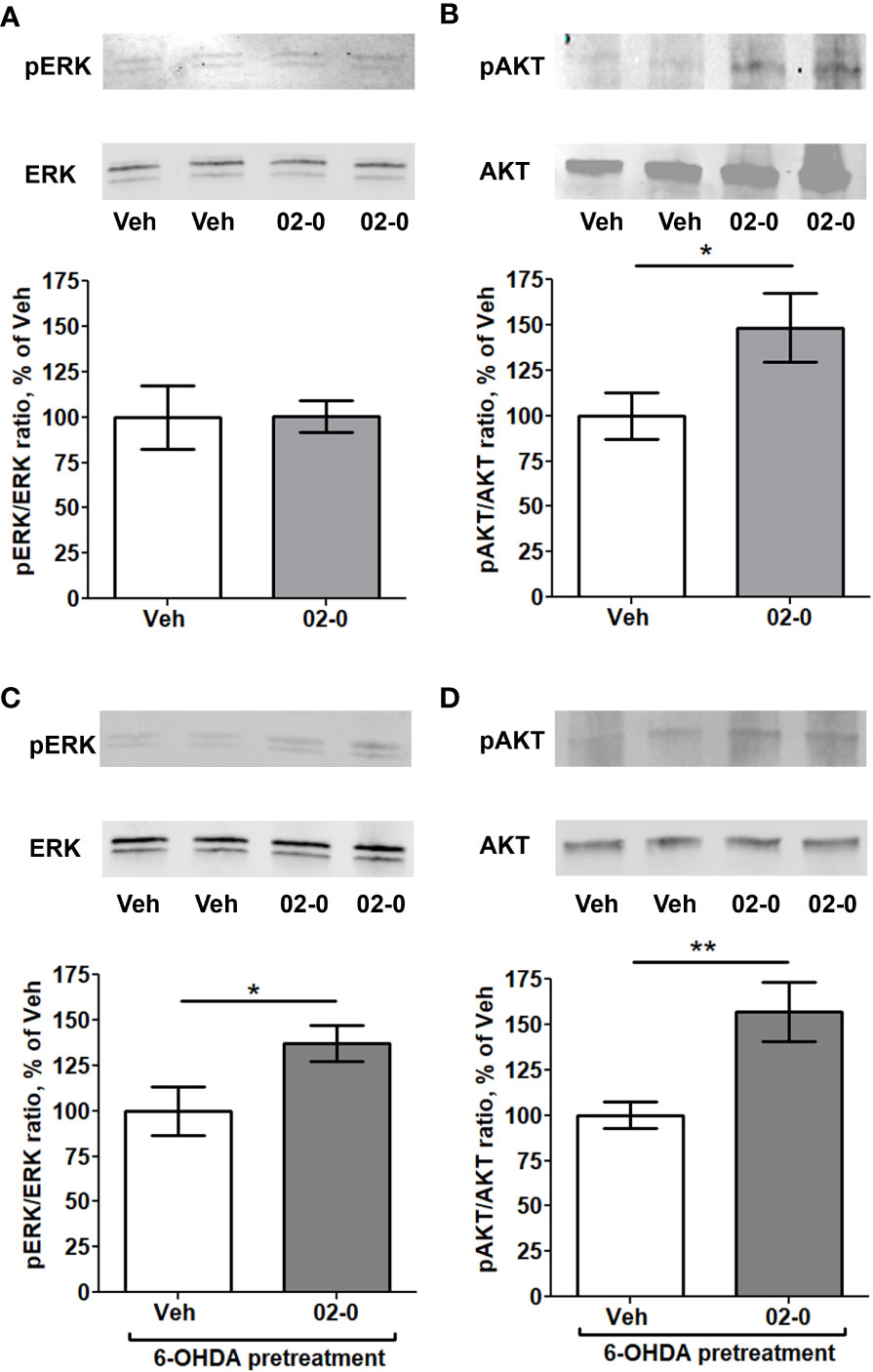
Figure 3 Activation of intracellular signaling pathways by 02-0 in SH-SY5Y cells (A) Western blot for ERK 1/2 and pERK 1/2 in SH-SY5Y cells after 10 min treatment with Veh or 100 nM 02-0, with representative blot showing bands at 42 and 44 KDa. N=8. Student’s t-test results: p>0.05. (B) Western blot for AKT and pAKT in SH-SY5Y cells after 10 min treatment with Veh or 100 nM 02-0, with representative blot showing bands at 62 KDa. N=8. Student’s t-test results: *: p<0.05. (C) Western blot for ERK 1/2 and pERK 1/2 in SH-SY5Y cells after 10 min treatment with Veh or 100 nM 02-0 following overnight pretreatment with 50 μM 6-OHDA, with representative blot showing bands at 42 and 44 KDa. N=8. Student’s t-test results: *: p<0.05. (D) Western blot for AKT and pAKT in SH-SY5Y cells after 10 min treatment with Veh or 100 nM 02-0 following overnight pretreatment with 50 μM 6-OHDA, with representative blot showing bands at 62 KDa. N=8. Student’s t-test results: **: p<0.01.
Both ERK and PI3K-AKT signaling pathways mediate mPRα neuroprotection
We performed studies with specific inhibitors of the above-mentioned signaling pathways to verify their roles in mPRα-mediated neuroprotective activity. The specific ERK inhibitor AZD 6244 (Figure 4A), the PI3K inhibitor Wortmannin (Figure 4B) and the AKT inhibitor ML-9 (Figure 4C) were used in a series of experiments when cells were treated for 24 h in the presence of 6-OHDA. All inhibitors proved effective in completely blocking the 02-0 protective effect on SH-SY5Y cell viability in the Hoechst assay. These results suggest both the ERK and the PI3K-AKT signaling pathways are involved in promoting mPRα-mediated neuroprotective activity.
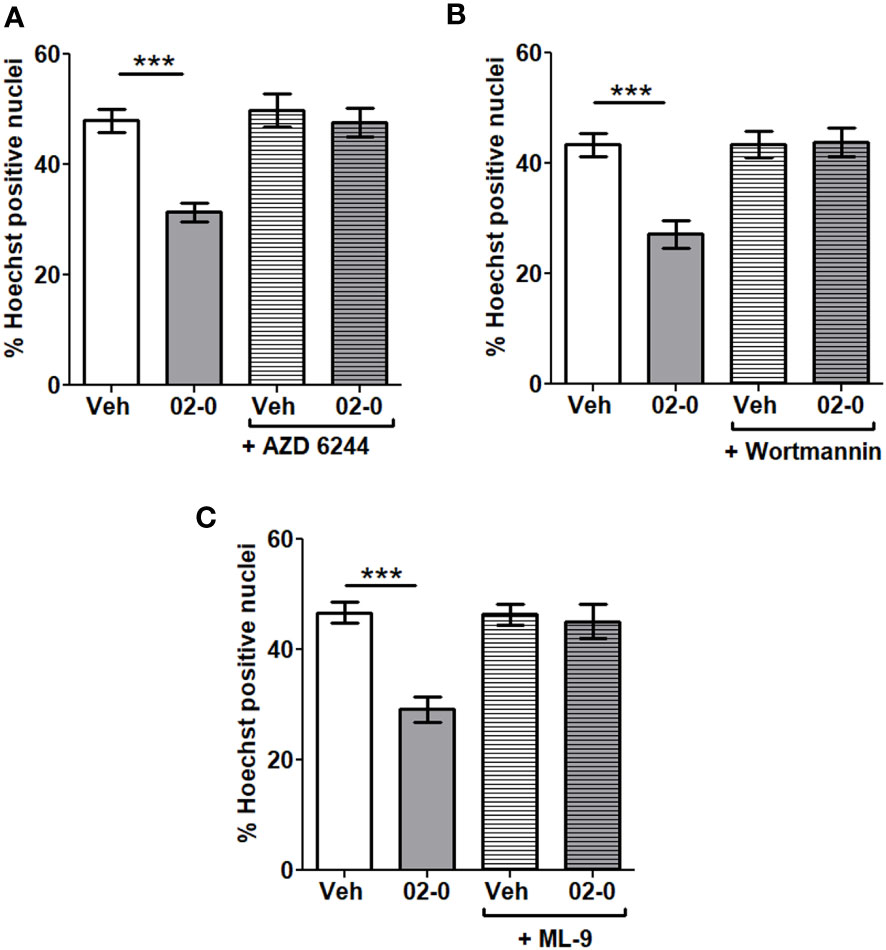
Figure 4 Involvement of signaling pathways in mPRα-mediated neuroprotection (A) Assessment of cell death in SH-SY5Y cells following Veh and 100 nM 02-0 24 h treatments in the presence of 50 μM 6-OHDA, with or without 1μM AZD 6244. N=18. Two-way ANOVA results: Interaction: p=0.0039, Treatment: p=0.0002, Inhibitor: p=0.0004. Bonferroni’s post-test results: ***: p<0.001. (B) Assessment of cell death in SH-SY5Y cells following Veh and 100 nM 02-0 24 h treatments in presence of 50 μM 6-OHDA, with or without 50 nM Wortmannin. N=18 Two-way ANOVA results: Interaction: p=0.0009, Treatment: p=0.0015, Inhibitor: p=0.0008. Bonferroni’s post-test results: ***: p<0.001. (C) Assessment of cell death in SH-SY5Y cells following Veh and 100 nM 02-0 24 h treatments in presence of 50 μM 6-OHDA, with or without 15 μM ML-9. N=18. Two-way ANOVA results: Interaction: p=0.0009, Treatment: p=0.0001, Inhibitor: p=0.0014. Bonferroni’s post-test results: ***: p<0.001.
Discussion
The results presented in this study show that P4 is neuroprotective in a cell model of PD through mPRα activation. The intracellular mechanism underlying mPRα activity involves the ERK and PI3K-AKT signaling pathways.
Non-differentiated SH-SY5Y cells were used for this study. Several differentiation protocols, mostly involving incubation with retinoic acid, have been proposed for this model in PD research. However, none of them is widely accepted, and a recent systematic review found that a no differentiation protocol was used in 81.5% of studies on SH-SY5Y cells as a PD model (32).
The comparison of mPRα and PR gene expression show that mPRα expression is 200 times higher than PR, likely making it the main mediator of P4 activity in this cell model. PR and mPRα were previously reported to be expressed in SH-SY5Y cells (30, 41, 42), but their expression levels were never compared. Our findings show that 02-0 and P4 are both effective in reducing SH-SY5Y cell death induced by 6-OHDA and MPP+ treatments. Both chemicals cause cell toxicity by causing oxidative stress. MPP+ increases the intracellular concentration of reactive oxygen species (ROS) by inhibiting complex I of the electron transport chain, thus impairing mitochondrial function. Moreover, it causes dopamine mobilization towards the cytoplasm, further increasing ROS production (43). 6-OHDA enters the cell through dopaminergic or catecholaminergic transporters. It then accumulates inside the cell, causing increased formation of ROS and catecholamine quinones, leading to cell death (44). P4 was previously shown to reduce ROS formation in ovarian and endometrial cancer cells (45) and to increase the expression and activity of the electron transport chain complex IV, thus reducing ROS formation, in the brain of ovariectomized rats (46). Therefore, even though mPRα influence on oxidative stress has not been characterized yet, it is reasonable to hypothesize that a modulation of ROS production may be involved in mPRα-mediated neuroprotection. This hypothesis requires further investigation.
In SH-SY5Y cells, 02-0 was previously shown to reduce cell death in a cell starvation model (30), and P4 was reported to be neuroprotective in models of pyroptosis (47) and neurological complications of HIV (41). Moreover, the P4 active metabolite allopregnanolone had protective activity in a model of Alzheimer’s disease following β-amyloid treatment (48). P4 was also neuroprotective in in vivo PD models, in particular following MPTP treatment (19, 20). Progestogens proved effective in eliciting neuroprotective effects also in other neuronal cell models. For example, P4 and allopregnanolone were neuroprotective, through mPR activation, in rodent GT1-7 and H19-7 neuronal cells in models of cell starvation (26, 28, 29). It should be noted that mPR activation was also shown to mediate neuroregenerative effects in peripheral glial cells (37, 49, 50). Since mPRs are also expressed in central nervous system glial cells (51, 52) and their expression in these cells in the brain is upregulated following traumatic brain injury (51), it can be hypothesized that mPRs in glial cells may be neuroprotective in PD. This hypothesis will need to be investigated in the future. Even taking in consideration the neuroprotective effects of progestogens described above, this is to our knowledge the first study showing P4 as a potential neuroprotective agent in an established human cell model of PD.
The pharmacological treatment and siRNA experiments show that mPRα is the receptor mediating progestogen neuroprotective action in SH-SY5Y cells following 6-OHDA and MPP+ treatments. As discussed above, mPRα and PR are expressed in SH-SY5Y cells, with the former being predominant. GABA-A receptor subunits were previously reported to be expressed at very low levels in SH-SY5Y cells, with the exception of the β3 subunit which was highly expressed (48). Muscimol treatments confirmed that the GABA-A receptor is not involved in the neuroprotective activity we observed. mPRβ was reported to be the most highly expressed mPR isoform in SH-SY5Y cells (30). However, the present results with PAQR7 siRNA clearly show that mPRα is the dominant isoform in mediating protective effects, as often observed in other human cell models (34, 39, 40).
Western blot and inhibitor studies clearly show that the ERK and PI3K-AKT signaling pathways are involved in the neuroprotective action mediated by mPRα. ERK activation only occurred following 6-OHDA pretreatment, suggesting it may be a specific response to cell damage. As mentioned above, both signaling pathways are known mediators of mPR activity in different human cell models (34, 37–40). Activation of both signaling pathways has been recently reported to be beneficial in different PD models (53–58). Our results also support the neuroprotective role of the ERK signaling pathway, whereas in several other studies the ERK pathway was also linked to possible worsening of PD damage by increasing oxidative stress and inflammation (59, 60). P4 treatment was not effective in activating these pathways in the striatum in an in vivo mouse model (20). This difference may be due to interspecies differences or involvement of other cell types, such as glial cells, in the mice striatum.
Taken together, our findings show that mPRα is neuroprotective in a human cell model of PD through the activation of the ERK and PI3K-AKT signaling pathways. These results will need to be confirmed in an in vivo model of PD to increase their translational potential. Nonetheless, these findings open the way for further investigation of mPRα as a potential target for progestogen therapies aimed at PD prevention or management.
Data availability statement
The raw data supporting the conclusions of this article will be made available by the authors, without undue reservation.
Author contributions
Both authors contributed to the study conception and design. L performed the experiments, wrote the original draft, and prepared the figures. P obtained funding, supervised the project, and revised the manuscript. Both authors approve the final version of the manuscript.
Funding
This research project was funded by The Morris L. Lichtenstein, Jr. Medical Research Foundation.
Conflict of interest
The authors declare that the research was conducted in the absence of any commercial or financial relationships that could be construed as a potential conflict of interest.
Publisher’s note
All claims expressed in this article are solely those of the authors and do not necessarily represent those of their affiliated organizations, or those of the publisher, the editors and the reviewers. Any product that may be evaluated in this article, or claim that may be made by its manufacturer, is not guaranteed or endorsed by the publisher.
Supplementary material
The Supplementary Material for this article can be found online at: https://www.frontiersin.org/articles/10.3389/fendo.2023.1125962/full#supplementary-material
References
1. Dorsey ER, Constantinescu R, Thompson JP, Biglan KM, Holloway RG, Kieburtz K, et al. Projected number of people with Parkinson disease in the most populous nations, 2005 through 2030. Neurology (2007) 68:384–6. doi: 10.1212/01.wnl.0000247740.47667.03
2. Wirdefeldt K, Adami H-O, Cole P, Trichopoulos D, Mandel J. Epidemiology and etiology of parkinson’s disease: A review of the evidence. Eur J Epidemiol (2011) 26 Suppl 1:S1–58. doi: 10.1007/s10654-011-9581-6
3. Hornykiewicz O. Ageing and neurotoxins as causative factors in idiopathic parkinson’s disease–a critical analysis of the neurochemical evidence. Prog Neuropsychopharmacol Biol Psychiatry (1989) 13:319–28. doi: 10.1016/0278-5846(89)90121-8
4. Dexter DT, Jenner P. Parkinson Disease: From pathology to molecular disease mechanisms. Free Radic Biol Med (2013) 62:132–44. doi: 10.1016/j.freeradbiomed.2013.01.018
5. Olanow CW, Stern MB, Sethi K. The scientific and clinical basis for the treatment of Parkinson disease (2009). Neurology (2009) 72:S1–136. doi: 10.1212/WNL.0b013e3181a1d44c
6. Wooten GF, Currie LJ, Bovbjerg VE, Lee JK, Patrie J. Are men at greater risk for parkinson’s disease than women? J Neurol Neurosurg Psychiatry (2004) 75:637–9. doi: 10.1136/jnnp.2003.020982
7. Van Den Eeden SK, Tanner CM, Bernstein AL, Fross RD, Leimpeter A, Bloch DA, et al. Incidence of parkinson’s disease: Variation by age, gender, and race/ethnicity. Am J Epidemiol (2003) 157:1015–22. doi: 10.1093/aje/kwg068
8. Shulman LM. Gender differences in parkinson’s disease. Gend Med (2007) 4:8–18. doi: 10.1016/s1550-8579(07)80003-9
9. Ragonese P, D’Amelio M, Salemi G, Aridon P, Gammino M, Epifanio A, et al. Risk of Parkinson disease in women: effect of reproductive characteristics. Neurology (2004) 62:2010–4. doi: 10.1212/wnl.62.11.2010
10. Saunders-Pullman R, Gordon-Elliott J, Parides M, Fahn S, Saunders HR, Bressman S. The effect of estrogen replacement on early parkinson’s disease. Neurology (1999) 52:1417–21. doi: 10.1212/wnl.52.7.1417
11. Shulman LM. Is there a connection between estrogen and parkinson’s disease? Parkinsonism Relat Disord (2002) 8:289–95. doi: 10.1016/s1353-8020(02)00014-7
12. Bourque M, Dluzen DE, Di Paolo T. Neuroprotective actions of sex steroids in parkinson’s disease. Front Neuroendocrinol (2009) 30:142–57. doi: 10.1016/j.yfrne.2009.04.014
13. Callier S, Morissette M, Grandbois M, Di Paolo T. Stereospecific prevention by 17beta-estradiol of MPTP-induced dopamine depletion in mice. Synapse (2000) 37:245–51. doi: 10.1002/1098-2396(20000915)37:4<245::AID-SYN1>3.0.CO;2-5
14. Morissette M, Al Sweidi S, Callier S, Di Paolo T. Estrogen and SERM neuroprotection in animal models of parkinson’s disease. Mol Cell Endocrinol (2008) 290:60–9. doi: 10.1016/j.mce.2008.04.008
15. Baraka AM, Korish AA, Soliman GA, Kamal H. The possible role of estrogen and selective estrogen receptor modulators in a rat model of parkinson’s disease. Life Sci (2011) 88:879–85. doi: 10.1016/j.lfs.2011.03.010
16. Grandbois M, Morissette M, Callier S, Di Paolo T. Ovarian steroids and raloxifene prevent MPTP-induced dopamine depletion in mice. Neuroreport (2000) 11:343–6. doi: 10.1097/00001756-200002070-00024
17. Yu L, Liao PC. Estrogen and progesterone distinctively modulate methamphetamine-induced dopamine and serotonin depletions in C57BL/6J mice. J Neural Transm (2000) 107:1139–47. doi: 10.1007/s007020070027
18. Callier S, Morissette M, Grandbois M, Pélaprat D, Di Paolo T. Neuroprotective properties of 17beta-estradiol, progesterone, and raloxifene in MPTP C57Bl/6 mice. Synapse (2001) 41:131–8. doi: 10.1002/syn.1067
19. Bourque M, Morissette M, Al Sweidi S, Caruso D, Melcangi RC, Di Paolo T. Neuroprotective effect of progesterone in MPTP-treated male mice. Neuroendocrinology (2016) 103:300–14. doi: 10.1159/000438789
20. Litim N, Morissette M, Di Paolo T. Effects of progesterone administered after MPTP on dopaminergic neurons of male mice. Neuropharmacology (2017) 117:209–18. doi: 10.1016/j.neuropharm.2017.02.007
21. Díaz NF, Díaz-Martínez NE, Velasco I, Camacho-Arroyo I. Progesterone increases dopamine neurone number in differentiating mouse embryonic stem cells. J Neuroendocrinol (2009) 21:730–6. doi: 10.1111/j.1365-2826.2009.01891.x
22. Thomas P, Pang Y, Dong J, Groenen P, Kelder J, de Vlieg J, et al. Steroid and G protein binding characteristics of the seatrout and human progestin membrane receptor alpha subtypes and their evolutionary origins. Endocrinology (2007) 148:705–18. doi: 10.1210/en.2006-0974
23. Kelder J, Pang Y, Dong J, Schaftenaar G, Thomas P. Molecular modeling, mutational analysis and steroid specificity of the ligand binding pocket of mPRα (PAQR7): Shared ligand binding with AdipoR1 and its structural basis. J Steroid Biochem Mol Biol (2022) 219:106082. doi: 10.1016/j.jsbmb.2022.106082
24. Zhu Y, Bond J, Thomas P. Identification, classification, and partial characterization of genes in humans and other vertebrates homologous to a fish membrane progestin receptor. Proc Natl Acad Sci USA (2003) 100:2237–42. doi: 10.1073/pnas.0436133100
25. Zhu Y, Rice CD, Pang Y, Pace M, Thomas P. Cloning, expression, and characterization of a membrane progestin receptor and evidence it is an intermediary in meiotic maturation of fish oocytes. Proc Natl Acad Sci USA (2003) 100:2231–6. doi: 10.1073/pnas.0336132100
26. Pang Y, Dong J, Thomas P. Characterization, neurosteroid binding and brain distribution of human membrane progesterone receptors δ and ϵ (mPRδ and mPRϵ) and mPRδ involvement in neurosteroid inhibition of apoptosis. Endocrinology (2013) 154:283–95. doi: 10.1210/en.2012-1772
27. Thomas P, Pang Y, Camilletti MA, Castelnovo LF. Functions of membrane progesterone receptors (mPRs, PAQRs) in nonreproductive tissues. Endocrinology (2022) 163:bqac147. doi: 10.1210/endocr/bqac147
28. Thomas P, Pang Y. Membrane progesterone receptors: Evidence for neuroprotective, neurosteroid signaling and neuroendocrine functions in neuronal cells. Neuroendocrinology (2012) 96:162–71. doi: 10.1159/000339822
29. Thomas P, Pang Y. Anti-apoptotic actions of allopregnanolone and ganaxolone mediated through membrane progesterone receptors (PAQRs) in neuronal cells. Front Endocrinol (Lausanne) (2020) 11:417. doi: 10.3389/fendo.2020.00417
30. Castelnovo LF, Thomas P. Membrane progesterone receptor α (mPRα/PAQR7) promotes survival and neurite outgrowth of human neuronal cells by a direct action and through schwann cell-like stem cells. J Mol Neurosci (2022) 72:2067–80. doi: 10.1007/s12031-022-02057-z
31. Kelder J, Azevedo R, Pang Y, de Vlieg J, Dong J, Thomas P. Comparison between steroid binding to membrane progesterone receptor α (mPRα) and to nuclear progesterone receptor: Correlation with physicochemical properties assessed by comparative molecular field analysis and identification of mPRα-specific agonists. Steroids (2010) 75:314–22. doi: 10.1016/j.steroids.2010.01.010
32. Xicoy H, Wieringa B, Martens GJM. The SH-SY5Y cell line in parkinson’s disease research: A systematic review. Mol Neurodegener (2017) 12:10. doi: 10.1186/s13024-017-0149-0
33. Chen Y, Shen J, Ma C, Cao M, Yan J, Liang J, et al. Skin-derived precursor schwann cells protect SH-SY5Y cells against 6-OHDA-induced neurotoxicity by PI3K/AKT/Bcl-2 pathway. Brain Res Bull (2020) 161:84–93. doi: 10.1016/j.brainresbull.2020.03.020
34. Castelnovo LF, Thomas P. Membrane progesterone receptor α (mPRα/PAQR7) promotes migration, proliferation and BDNF release in human schwann cell-like differentiated adipose stem cells. Mol Cell Endocrinol (2021) 531:111298. doi: 10.1016/j.mce.2021.111298
35. Faroni A, Terenghi G, Magnaghi V. Expression of functional γ-aminobutyric acid type a receptors in schwann-like adult stem cells. J Mol Neurosci (2012) 47:619–30. doi: 10.1007/s12031-011-9698-9
36. Pang Y, Thomas P. Involvement of sarco/endoplasmic reticulum Ca2+-ATPase (SERCA) in mPRα (PAQR7)-mediated progesterone induction of vascular smooth muscle relaxation. Am J Physiol Metab (2021) 320:E453–66. doi: 10.1152/ajpendo.00359.2020
37. Castelnovo LF, Caffino L, Bonalume V, Fumagalli F, Thomas P, Magnaghi V. Membrane progesterone receptors (mPRs/PAQRs) differently regulate migration, proliferation, and differentiation in rat schwann cells. J Mol Neurosci (2020) 70:433–48. doi: 10.1007/s12031-019-01433-6
38. Pang Y, Thomas P. Role of mPRα (PAQR7) in progesterone-induced Ca2+ decrease in human vascular smooth muscle cells. J Mol Endocrinol (2019) 63:199–213. doi: 10.1530/JME-19-0019
39. Pang Y, Thomas P. Progesterone induces relaxation of human umbilical cord vascular smooth muscle cells through mPRα (PAQR7). Mol Cell Endocrinol (2018) 474:20–34. doi: 10.1016/j.mce.2018.02.003
40. Pang Y, Dong J, Thomas P. Progesterone increases nitric oxide synthesis in human vascular endothelial cells through activation of membrane progesterone receptor-α. Am J Physiol Endocrinol Metab (2015) 308:E899–911. doi: 10.1152/ajpendo.00527.2014
41. Salahuddin MF, Qrareya AN, Mahdi F, Jackson D, Foster M, Vujanovic T, et al. Combined HIV-1 tat and oxycodone activate the hypothalamic-pituitary-adrenal and -gonadal axes and promote psychomotor, affective, and cognitive dysfunction in female mice. Horm Behav (2020) 119:104649. doi: 10.1016/j.yhbeh.2019.104649
42. Takahashi K, Piao S, Yamatani H, Du B, Yin L, Ohta T, et al. Estrogen induces neurite outgrowth via rho family GTPases in neuroblastoma cells. Mol Cell Neurosci (2011) 48:217–24. doi: 10.1016/j.mcn.2011.08.002
43. Vila M, Przedborski S. Targeting programmed cell death in neurodegenerative diseases. Nat Rev Neurosci (2003) 4:365–75. doi: 10.1038/nrn1100
44. Simola N, Morelli M, Carta AR. The 6-hydroxydopamine model of parkinson’s disease. Neurotox Res (2007) 11:151–67. doi: 10.1007/BF03033565
45. Nguyen H, Syed V. Progesterone inhibits growth and induces apoptosis in cancer cells through modulation of reactive oxygen species. Gynecol Endocrinol (2011) 27:830–6. doi: 10.3109/09513590.2010.538100
46. Irwin RW, Yao J, Hamilton RT, Cadenas E, Brinton RD, Nilsen J. Progesterone and estrogen regulate oxidative metabolism in brain mitochondria. Endocrinology (2008) 149:3167–75. doi: 10.1210/en.2007-1227
47. Cui C, Wang X, Zhang S, Wu H, Li M, Dong L, et al. Progesterone reduces ATP-induced pyroptosis of SH-SY5Y cells. BioMed Res Int (2022) 2022:4827444. doi: 10.1155/2022/4827444
48. Mendell AL, Chung BYT, Creighton CE, Kalisch BE, Bailey CDC, MacLusky NJ. Neurosteroid metabolites of testosterone and progesterone differentially inhibit ERK phosphorylation induced by amyloid β in SH-SY5Y cells and primary cortical neurons. Brain Res (2018) 1686:83–93. doi: 10.1016/j.brainres.2018.02.023
49. Castelnovo LF, Magnaghi V, Thomas P. Expression of membrane progesterone receptors (mPRs) in rat peripheral glial cell membranes and their potential role in the modulation of cell migration and protein expression. Steroids (2019) 142:6–13. doi: 10.1016/j.steroids.2017.09.009
50. Castelnovo LF, Thomas P, Magnaghi V. Membrane progesterone receptors (mPRs/PAQRs) in schwann cells represent a promising target for the promotion of neuroregeneration. Neural Regener Res (2021) 16:281–2. doi: 10.4103/1673-5374.290885
51. Meffre D, Labombarda F, Delespierre B, Chastre A, De Nicola AF, Stein DG, et al. Distribution of membrane progesterone receptor alpha in the male mouse and rat brain and its regulation after traumatic brain injury. Neuroscience (2013) 231:111–24. doi: 10.1016/j.neuroscience.2012.11.039
52. Labombarda F, Meffre D, Delespierre B, Krivokapic-Blondiaux S, Chastre A, Thomas P, et al. Membrane progesterone receptors localization in the mouse spinal cord. Neuroscience (2010) 166:94–106. doi: 10.1016/j.neuroscience.2009.12.012
53. Goyal A, Agrawal A, Verma A, Dubey N. The PI3K-AKT pathway: A plausible therapeutic target in parkinson’s disease. Exp Mol Pathol (2022) 129:104846. doi: 10.1016/j.yexmp.2022.104846
54. Ratih K, Lee Y-R, Chung K-H, Song DH, Lee K-J, Kim D-H, et al. L-theanine alleviates MPTP-induced parkinson’s disease by targeting wnt/β-catenin signaling mediated by the MAPK signaling pathway. Int J Biol Macromol (2022) 226:90–101. doi: 10.1016/j.ijbiomac.2022.12.030
55. Fu R-H, Tsai C-W, Liu S-P, Chiu S-C, Chen Y-C, Chiang Y-T, et al. Neuroprotective capability of narcissoside in 6-OHDA-exposed parkinson’s disease models through enhancing the MiR200a/Nrf-2/GSH axis and mediating MAPK/Akt associated signaling pathway. Antioxidants (Basel Switzerland) (2022) 11:2089. doi: 10.3390/antiox11112089
56. Mesa-Infante V, Afonso-Oramas D, Salas-Hernández J, Rodríguez-Núñez J, Barroso-Chinea P. Long-term exposure to GDNF induces dephosphorylation of ret, AKT, and ERK1/2, and is ineffective at protecting midbrain dopaminergic neurons in cellular models of parkinson’s disease. Mol Cell Neurosci (2022) 118:103684. doi: 10.1016/j.mcn.2021.103684
57. Ding Y, Zhou M, Zheng R, Ma R, Deng J, Hao W-Z, et al. Feruloylated oligosaccharides ameliorate MPTP-induced neurotoxicity in mice by activating ERK/CREB/BDNF/TrkB signalling pathway. Phytomedicine (2023) 108:154512. doi: 10.1016/j.phymed.2022.154512
58. Jiang Z, Wang J, Sun G, Feng M. BDNF-modified human umbilical cord mesenchymal stem cells-derived dopaminergic-like neurons improve rotation behavior of parkinson’s disease rats through neuroprotection and anti-neuroinflammation. Mol Cell Neurosci (2022) 123:103784. doi: 10.1016/j.mcn.2022.103784
59. Park J, Jang KM, Park K-K. Effects of apamin on MPP+-induced calcium overload and neurotoxicity by targeting CaMKII/ERK/p65/STAT3 signaling pathways in dopaminergic neuronal cells. Int J Mol Sci (2022) 23:15255. doi: 10.3390/ijms232315255
Keywords: Parkinson’s disease, membrane progesterone receptor α, neuroprotection, PI3K-AKT, ERK
Citation: Castelnovo LF and Thomas P (2023) Progesterone exerts a neuroprotective action in a Parkinson’s disease human cell model through membrane progesterone receptor α (mPRα/PAQR7). Front. Endocrinol. 14:1125962. doi: 10.3389/fendo.2023.1125962
Received: 16 December 2022; Accepted: 24 February 2023;
Published: 10 March 2023.
Edited by:
Rachida Geunnoun, Institut National de la Santé et de la Recherche Médicale (INSERM), FranceReviewed by:
Ignacio Camacho-Arroyo, National Autonomous University of Mexico, MexicoMeharvan Singh, Loyola University Chicago, United States
Hubert Vaudry, Université de Rouen, France
Copyright © 2023 Castelnovo and Thomas. This is an open-access article distributed under the terms of the Creative Commons Attribution License (CC BY). The use, distribution or reproduction in other forums is permitted, provided the original author(s) and the copyright owner(s) are credited and that the original publication in this journal is cited, in accordance with accepted academic practice. No use, distribution or reproduction is permitted which does not comply with these terms.
*Correspondence: Luca F. Castelnovo, Luca.Castelnovo@utexas.edu; Peter Thomas, Peter.Thomas@utexas.edu