- 1Raghavendra Institute of Pharmaceutical Education and Research, Anantapur, India
- 2Department of Pharmacology, Balaji College of Pharmacy, Anantapur, India
- 3College of Pharmacy, Riyadh ELM University, Riyadh, Saudi Arabia
- 4Faculty of Pharmacy, Dr. M.G.R. Educational and Research Institute, Chennai, India
- 5Vaccines and Bioprocessing Centre, King Abdulaziz City for Science and Technology (KACST), Riyadh, Saudi Arabia
- 6Department of Pharmaceutics, College of Pharmaceutical Sciences, Dayananda Sagar University, Bengaluru, India
- 7Faculty of Pharmaceutical Sciences, UCSI University, Kuala Lumpur, Malaysia
- 8School of Pharmacy, ITM University, Gwalior, India
- 9Amity Institute of Pharmacy, Amity University, Gwalior, India
- 10GITAM School of Pharmacy, GITAM University Hyderabad Campus, Rudraram, India
- 11Department of Biotechnology, School of Engineering and Technology, Sharda University, Greater Noida, India
- 12Department of Biotechnology, School of Applied & Life Sciences (SALS), Uttaranchal University, Dehradun, India
- 13School of Bioengineering & Biosciences, Lovely Professional University, Phagwara, India
- 14Department of Biotechnology Engineering and Food Technology, Chandigarh University, Mohali, India
- 15Faculty of Biotechnology and Food Sciences, Slovak University of Agriculture in Nitra, Nitra, Slovakia
- 16Laboratory of Animal Immunology and Biotechnology, Department of Animal Morphology, Physiology and Genetics, Faculty of AgriSciences, Mendel University in Brno, Brno, Czechia
- 17Department of Life Science and Bioinformatics, Assam University, Silchar, India
Colorectal cancer (CRC) is one of the most deaths causing diseases worldwide. Several risk factors including hormones like insulin and insulin like growth factors (e.g., IGF-1) have been considered responsible for growth and progression of colon cancer. Though there is a huge advancement in the available screening as well as treatment techniques for CRC. There is no significant decrease in the mortality of cancer patients. Moreover, the current treatment approaches for CRC are associated with serious challenges like drug resistance and cancer re-growth. Given the severity of the disease, there is an urgent need for novel therapeutic agents with ideal characteristics. Several pieces of evidence suggested that natural products, specifically medicinal plants, and derived phytochemicals may serve as potential sources for novel drug discovery for various diseases including cancer. On the other hand, cancer cells like colon cancer require a high basal level of reactive oxygen species (ROS) to maintain its own cellular functions. However, excess production of intracellular ROS leads to cancer cell death via disturbing cellular redox homeostasis. Therefore, medicinal plants and derived phytocompounds that can enhance the intracellular ROS and induce apoptotic cell death in cancer cells via modulating various molecular targets including IGF-1 could be potential therapeutic agents. Alkaloids form a major class of such phytoconstituents that can play a key role in cancer prevention. Moreover, several preclinical and clinical studies have also evidenced that these compounds show potent anti-colon cancer effects and exhibit negligible toxicity towards the normal cells. Hence, the present evidence-based study aimed to provide an update on various alkaloids that have been reported to induce ROS-mediated apoptosis in colon cancer cells via targeting various cellular components including hormones and growth factors, which play a role in metastasis, angiogenesis, proliferation, and invasion. This study also provides an individual account on each such alkaloid that underwent clinical trials either alone or in combination with other clinical drugs. In addition, various classes of phytochemicals that induce ROS-mediated cell death in different kinds of cancers including colon cancer are discussed.
1 Introduction
Globally, cancer is one of the highest mortality-causing diseases. Though there is a drastic improvement in the current treatment schedules followed for various cancers, there is no marked decrease of death rate (1, 2). Among the various dreadful cancers, colorectal cancer (CRC) stood in third and second globally regarding prevalence and death rates, respectively (3, 4). Moreover, a rapid rise in the cases and death rates of CRC has been predicted soon (5). By 2035, a global rise of 2.5 million cases has been estimated (6). However, the CRC incidence varies from country to country, and the maximum number of cases have been reported from the developed world (7). Colorectal tumors were more influenced by gender and sex, where male populations have been affected more significantly than the females (8). In addition, the other risk factors, such as age, consumption of alcohol, diet with high fat and low fruits, vegetables, and maintaining low physical activities, influences the level of progression of the disease (5). Growing evidence also suggests that mutations in the growth factors such as insulin-like growth factor 1 (IGF-1) also shows a massive impact on the development of colon cancer via activating rat sarcoma/mitogen-activated protein kinase/phosphatidylinositol-3 kinase/protein kinase B (RAS/MAPK/PI3K/AKT) pathways (4, 9). On the other hand, hormonal alterations also show significant role in production of ROS, which plays an important role in CRC development. Various studies have revealed that hormones like thyroid hormones, corticosteroids and catecholamines are also involved in the generation of ROS by modulating various signaling molecules and pathways like NADPH oxidase (NOX) pathway, mitochondrial electron transport chain (ETC), nitric oxide (NO), and nuclear factor erythroid 2-related factor 2 (Nrf2), apart from their normal functions (10, 11). In addition, some diseases like inflammatory bowel disorder (IBD) also serves as significant risk factors for colon cancer (12, 13).
Recently, there has been a huge advancement in the screening and treatment techniques of CRC. Among the various available treatments, surgery is the preferred treatment option for colon cancer patients (14). However, these treatments cannot effectively reach low-income patients and can only extend the survival time of CRC patients (4, 14). Furthermore, many effective chemotherapeutic drug candidates such as 5-fluorouracil, oxaliplatin, capecitabine, and irinotecan that are clinically recommended for colon cancer treatment either individually or in combination exhibit severe toxicity to normal cells (15, 16). Therefore, there is an urgent need for novel drug treatment with ideal characteristics to treat colorectal carcinoma. From ancient days natural products, specifically medicinal plants and herbal products, have played a significant role in identifying novel treatments against various diseases (17–24). Moreover, several studies have reported plant-derived components as safe to use, and they exhibited minimal toxicity towards non-cancerous cells (2, 25). Hence, these phytocompounds have also been suggested for use together with other clinically recommended chemotherapy drugs to reduce toxicity.
In general, cancer cells require a high level of reactive oxygen species (ROS) compared to normal cells due to elevated metabolic rate and mitochondrial dysfunction, and this makes the cancer cells more vulnerable to oxidative stress. Hence, an increased level of ROS than required can trigger the death-inducing signals in cancer cells via irreversible damage to various biomolecules. In contrast to cancer cells, normal cells develop potent antioxidant proteins that can nullify the toxic effects of ROS (26). Therefore, minimal elevation of intracellular ROS than the threshold limit via intervention with external agents can make the cancer cells more sensitive to oxidative stress than the normal cells (27–29). There are several phytocompounds, such as curcumin, capsaicin, sulforaphane, alpha-lipoic acid, and piperine belonging to the class of alkaloids, flavonoids, terpenoids, and phenolic compounds that are known to initiate ROS-mediated apoptotic cell death. Among the various chemical classes, alkaloids are the most divergent and vigorously investigated class of phytochemicals in different diseases including cancer. Various alkaloids like vincristine, vinblastine, evodiamine, sanguinarine, matrine, tetrandrine, camptothecin, and berberine have already proved their anti-cancer potentials via targeting different kinds of essential signaling molecules such as MAPK, extracellular signal-related kinases 1/2 (ERK1/2), tumor suppressor protein (p53), p38 mitogen-activated protein kinase, c-Jun N-terminal kinase (JNK), and PI3K/Akt. Hence, forced activation of ROS in the cancer cells through small molecules like alkaloids derived from medicinal plants and altering the signaling molecules that initiate the death process can improve the cancer condition. Moreover, several kinds of alkaloids like berberine, camptothecin and epigallocatechin as such or as derivatives have been under clinical trials at different stages (30; 31). Some of them have till now exhibited a good safety profile as compared to other recommended drugs (32, 33). Hence, this evidence-based study focused on the alkaloids and their ROS-mediated apoptotic signaling pathways. In addition, other classes of phytocompounds that induce ROS-mediated apoptosis and have been used in clinical trials either individually or in combination with other clinical drugs have also been looked at. Several studies have revealed that the synergetic or combination effect of medicinal plants or phytocompounds provides a novel therapeutic tool. Moreover, combinations of herbal compounds with existing clinical drugs have also been reported to increase the specificity and minimize the toxic effects of existing chemotherapy drugs. In this way, the current review acts as a standalone reference to all the classes of herbal compounds that involve ROS-mediated apoptotic cell death.
2 Methodology
The most relevant literature to conduct the study was extracted from electronic databases SCOPUS, and PubMed. The key words and the phrases used for collecting the highly relevant articles included colorectal cancer, alkaloids, ROS-mediated apoptosis, medicinal plants, phytochemicals, current therapies, side effects, hormones and growth factors, clinical trials, and related terms. Later, the findings from the selected studies were summarized by focusing on the alkaloids that induce ROS mediated apoptosis in colon cancer. The articles not published in English language were excluded.
3 ROS: sources and role in cancer development
ROS are highly active and most unstable oxygen containing metabolic products, generally produced by various endogenous and exogenous sources. Among the multiple endogenous sources, mitochondria-based metabolic pathways, p450 metabolism, endoplasmic reticulum, peroxisome, activated macrophages, and neutrophils mainly contribute to ROS generation (Figure 1). Besides, there are other resources such as monoamine oxidase, sirtuins, forkhead box O3 (FOXO3), alpha-ketoglutarate dehydrogenase (α-KGDH), nuclear factor erythroid 2-related factor 2 (Nrf2) and mitochondrial p66shc (proapoptotic protein) which also contribute to ROS generation (34). In addition, the innate immune response shown by the host against the pathogens also generates ROS as a defense mechanism. However, persistent or chronic inflammation leads to excessive production of ROS and develops various kinds of diseases including colon cancer. In a recent study, Sahoo and his group revealed that lipopolysaccharides (LPS)-induced chronic inflammation in the intestine may also lead to cancer in the gut. They observed that LPS treatment can enhance the levels of pro-cancer genes and help in cell proliferation in the colonoids obtained from the dogs suffering from IBD. Therefore, the persistent or chronic inflammatory diseases like IBD also play a part in the development of colon cancer though oxidative stress (13, 35).
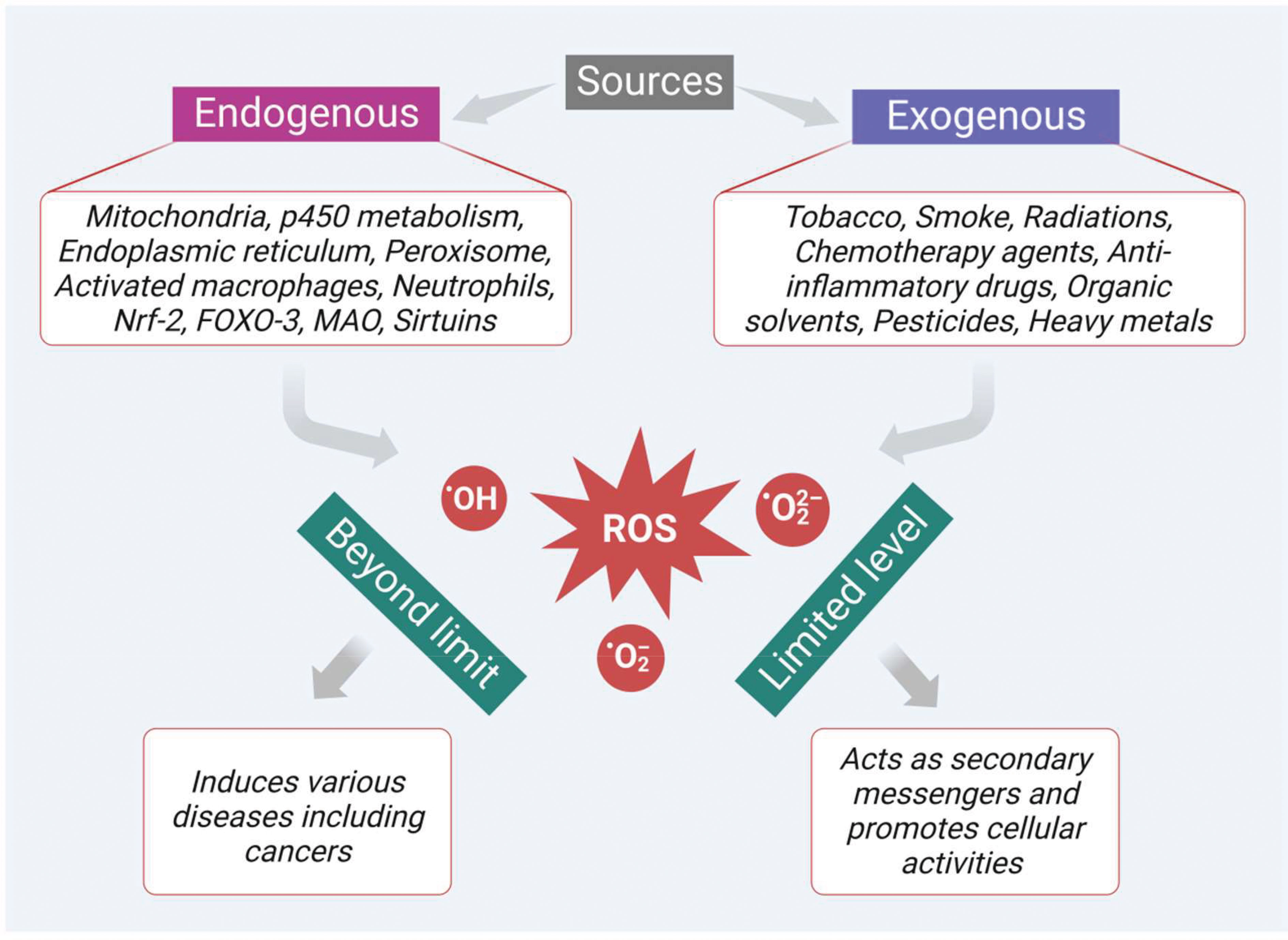
Figure 1 Sources of ROS generation which play dual role as secondary messengers that promote cellular activities at limited levels and as inducers of various diseases including cancer at excessive levels.
In addition, there are many exogenous sources like tobacco smoking, radiation, chemotherapeutic agents, anti-inflammatory drugs, chemicals containing quinones, organic solvents, pesticides, and other heavy metals (like cadmium, chromium, and arsenic) that also produce ROS intermediates by interacting with other molecules. In general, ROS like hydroxyl radical (•OH), superoxide radical (O2•–), and hydrogen peroxide (H2O2) at a limited level are crucial to cellular activities (Figure 1). They act as secondary messengers, promoting various essential cellular activities such as signal transduction, immune responses, and gene transcription. However, when the levels of ROS increase beyond the limit, it leads to oxidative stress and damages various vital biomolecules such as lipids, proteins, deoxyribonucleic acids (DNA), and ribonucleic acids (RNA). Moreover, ROS also initiate the development of various diseases, such as cardiovascular diseases, cataracts, neurodegenerative diseases, inflammation, diabetes mellitus, and cancer (Figure 1). Furthermore, ROS plays an important role in the initiation, development, and progression of cancer. Besides, the cancer cells themselves require a high level of ROS in contrast to the normal cells due to the high metabolic rate and increased energy demand. These increased levels of ROS via multiple sources interact with various essential signaling molecules thereby activating various growth factors like epidermal growth factor (EGF), and IGF-1, and inducing mutation in their receptors.
Mutated EGF receptor (EGFR) elevates the expression of its downstream signaling molecules such as rat sarcoma - rapidly accelerated fibrosarcoma (RAS-RAF), MAPK, AKT, PI3K and mammalian target of rapamycin (mTOR). In the initial stage of the pathway, the EGFR initiates son of sevenless homolog 1 (Sos 1) gene-mediated activation of RAS-RAF molecules. Then the activated RAS-RAF initiates the phosphorylation of MAPK, which later activates ERK1 and ERK2. The activated ERK immediately phosphorylates the various components of cytoskeleton like MAP 1, 2, and 4 in the cytoplasm, which controls the cell morphology and other functions. In addition, the activated ERK also shifts to the nucleus and helps in the transcription of various signaling molecules like proto-oncogene fos proto-oncogene (c-Fos), transcription factor Jun (c-Jun), cellular myelocytomatosis oncogene (c-Myc), erythroblast transformation specific (ETS) domain-containing protein ETS domain transcription factor ELK1 (Elk-1) and cyclic adenosine monophosphate (cAMP) dependent transcription factor ATF2 (activating transcription factor 2) (36). Mitogen-activated extracellular signal-regulated kinase (MEK) and ERK signaling molecules, proto-oncogene B-Raf (BRAF) (a downstream proto-oncogene of the RAF family) play a significant role in the activation of RAS. On the other hand, RAS also triggers the activation of PI3K signaling molecules, which promotes the conversion of PI-3, phosphatidylinositol 4,5-bisphosphate (PIP2) to phosphatidylinositol (3,4,5)-trisphosphate (PIP3). This PIP3 activates protein kinase B/Akt via phosphoinositide-dependent kinase 1 (PDK1) dependent phosphorylation. Then the activated Akt inactivates the transcription activity of FOXO and its interlinked downstream signals via phosphorylation and finally promotes the survival, proliferation, and growth of cancer cells (37). Besides Akt activation, it also inhibits glycogen synthase kinase-3 (GSK-3) via phosphorylation. This inactivation of GSK-3 protein leads to the activation of various signaling molecules such as (wingless gene) WNT/β-catenin, nuclear factor kappa B (NF-κB), mTOR and its respective downstream signals (Figure 2).
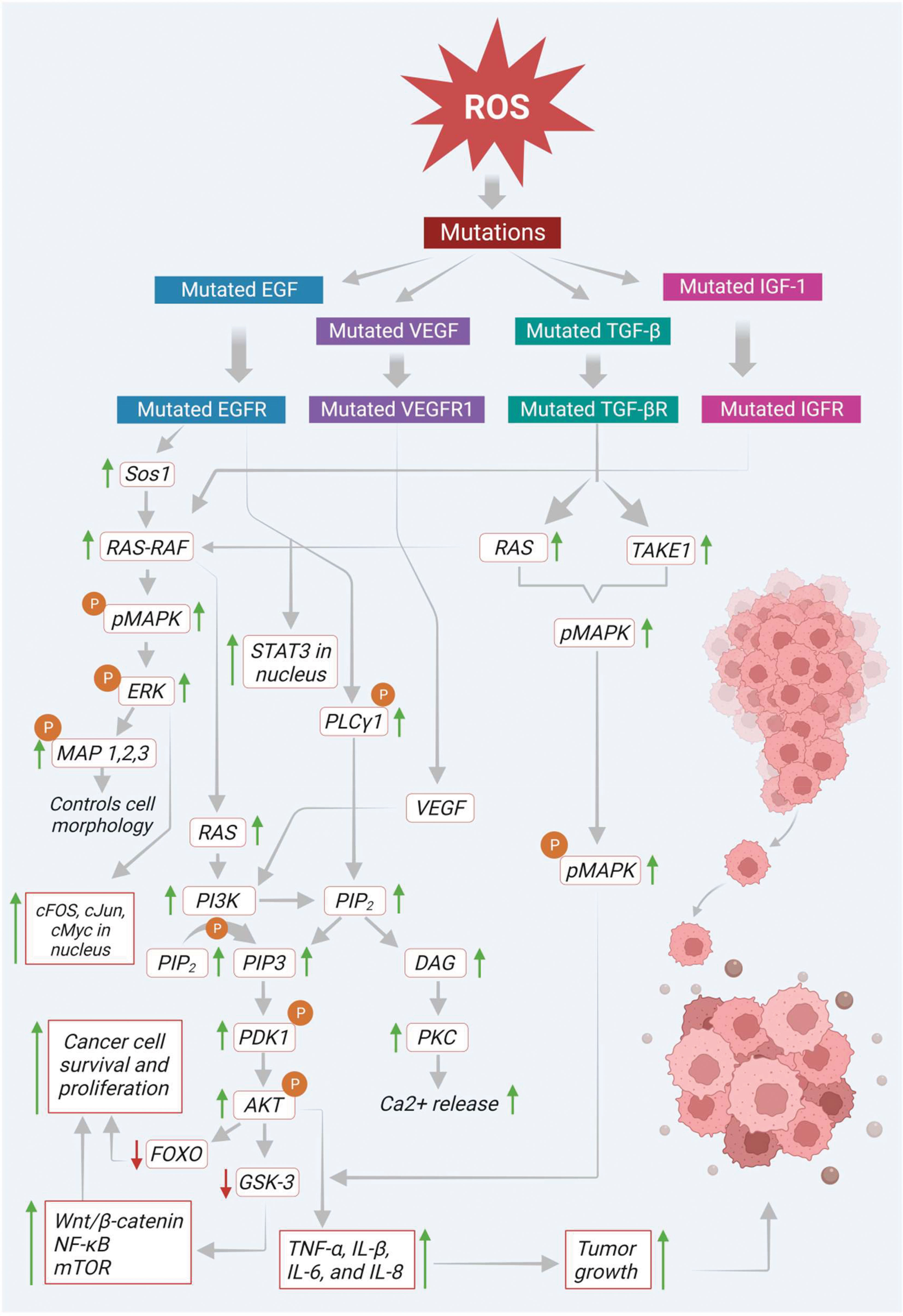
Figure 2 Basal levels of ROS initiate mutation in various transmembrane receptors thereby modulating multiple signaling molecules/pathways that involve tumor growth and progression.
In addition, activated EGFR directly phosphorylates and activates phosphoinositide-specific phospholipase C gamma 1 (PLC-γ1). This PLC-γ1 facilitates the generation of PIP3 and diacylglycerol (DAG) from PIP2. DAG further triggers PKC activation and enhances intracellular calcium (Ca+2) release, ultimately leading to carcinogenesis (38). Moreover, EGFR also promotes transcriptional activation of signal transducer and activator of transcription 3 (STAT3) in the nucleus via direct interaction and enhances its biological functions such as tumor growth, differentiation, and apoptosis (Figure 2). On the other hand, angiogenesis is another critical factor that plays a prominent role in the colon cancer progression.
Besides, ROS also initiate the activation of vascular endothelial growth factor (VEGF) via upregulation of the activity of hypoxia-inducible factor 1-alpha (HIF-1α). This activated VEGF binds to its respective receptor to further activate its downstream signaling molecules (39). There are three types of VEGF receptors such as VEGF receptors 1, 2, and 3 (VEGFR-1, -2, and -3). Moreover, they are all different in their activities and sites of action (40). Among the various VEGFR receptors, VEGFR-1 is found explicitly in various cancers, epithelial and inflammatory cells and promotes biological functions via interacting with VEGF. This receptor predominately helps the cancer cells to migrate during angiogenesis (41). Furthermore, it also shows a significant contribution to inflammatory disease conditions, including various cancers, through the activation of downstream signals such as PI3K/Akt/MAPK/ERK, resulting in the release of various inflammatory cytokines, including tumor necrosis factor alpha (TNF-α), and interleukins (IL-1β, IL-6, and IL-8). As shown in Figure 2, these cytokines finally promote tumor growth in respective tissues (42).
In addition, ROS also induce transforming growth factor-beta (TGF-β), which plays a vital role in various fibrotic diseases. Growing evidence suggests that TGF-β also shows impact on tumor growth and progression, which is mediated by ROS. In cancer cells, ROS initiated TGF-β was reported to induce the MAPK signaling pathway via activating Ras and TGF-β-activated kinase 1 (TAK1) (34). The activated MAPK further triggers its downstream signaling molecules like ERK1 and ERK2. Finally, this MAPK and TGF-β together with other cytokine molecules, recommend epithelial-mesenchymal transition (EMT), where the tumor epithelial cells adopt mesenchymal-like characteristics that can help in tumor progression and metastasis (Figure 2).
Furthermore, ROS also induce IGFs that play a pivotal role in cancer survival and progression. Several studies revealed that ROS-mediated IGF-I activation also activates its corresponding receptor IGF-1 via phosphorylation. As shown in Figure 2, this activated IGF-1 turns on its downstream signaling, such as MAPK, Akt, mTOR and NF-κB via induction of Ras-Raf proto-oncogenes (9). The pathways mentioned above are involved in ROS-mediated signaling in cancer development. The subsequent sections highlight growth factors and corresponding signaling in cancer progression.
4 ROS mediated apoptotic signaling
ROS are short-lived metabolic by-products that generally possess an unpaired electron in their respective outermost shells. Because of the unpaired electron, the ROS are highly reactive and show both dangerous and beneficial effects (43, 44). In fact, at the level or below the threshold limit, ROS assist in the development of various cancers via initiation of mutations in various signaling molecules like p53 as well as upregulation of oncogenes like Ras and c-Myc. However, cancer cells initiate apoptotic cell death mechanisms due to ROS accumulation beyond the threshold limit (45). Normally, ROS-associated apoptotic cell death can be triggered via activation of intrinsic or mitochondrial signaling and upregulation of extrinsic or death receptor signaling.
In the intrinsic pathway, excessive ROS production (e.g., by H2O2) modulates mitochondrial permeability transition (MPT) pore and mitochondrial membrane depolarization. As a result, two very essential groups that consist of inactive pro-apoptotic molecules are released in the cytoplasm. One such group contains signaling molecules like cytochrome c, second mitochondria-derived activator of caspase (Smac)/DIABLO (a direct IAP binding protein with low PI), and serine protease Htr (with high-temperature requirements) A2 (Omi) (nuclear-encoded mitochondrial serine protease). Significantly, all these molecules are involved in caspase-dependent apoptotic pathways (43). To be specific, cytochrome c facilitates the proteolytic maturation of both caspases-3 and 9 via allosteric activation of apoptotic peptidase activating factor 1 (APAF-1). This activation finally leads to the formation of apoptosomes (46, 47). In a similar manner, as shown in Figure 3, other pro-apoptotic proteins like Smac/DIABLO as well as HtrA2/Omi also initiate the apoptotic process via inhibition of IAP like XIAP (47, 48). The second group released from the mitochondrial membrane comprises signaling molecules like apoptosis-inducing factor (AIF), endonuclease G, and caspase-activated DNAse (CAD). After immediate release AIF, endonuclease G, and CAD proteins slide inside the nucleus and initiate caspase-dependent apoptotic features in the cells like DNA fragmentation as well as chromatin condensation (47, 49). Besides, the Bcl-2 family proteins also control the release of various apoptotic signaling molecules including cytochrome c. In the Bcl-2 family, proteins such as Bax, Bak, Bid, Bad, Bim, Bik, and Blk facilitate apoptosis. The other remaining proteins like B-cell leukemia/lymphoma proteins (Bcl-2, Bcl-x, Bcl-XL, Bcl-XS, Bcl-w) and BAG (Bcl-2–associated athanogene), favor inhibition of apoptosis signaling (50, 51). Interestingly, these Bcl-2 family proteins can be significantly tuned by tumor suppressor protein p53. Several studies revealed that p53 directly interacts with Bcl-2 family proteins and helps in mitochondrial membrane depolarization and increases the release of apoptotic signals. To be specific, p53 plays a role in promoting p53 upregulated modulator of apoptosis (Puma) and Bcl2 homology domain 3 (BH3) (Noxa), the two essential proteins of the Bcl2 family (Figure 3). A previous report reveals that Puma, in particular, enhances Bcl-2-associated X protein (BAX) and Noxa supports p53 mediated apoptotic signaling (3, 52). Additionally, excessive ROS upregulation influences apoptosis through the elevation of Ca2+ levels, which increases mitochondrial permeability (52).
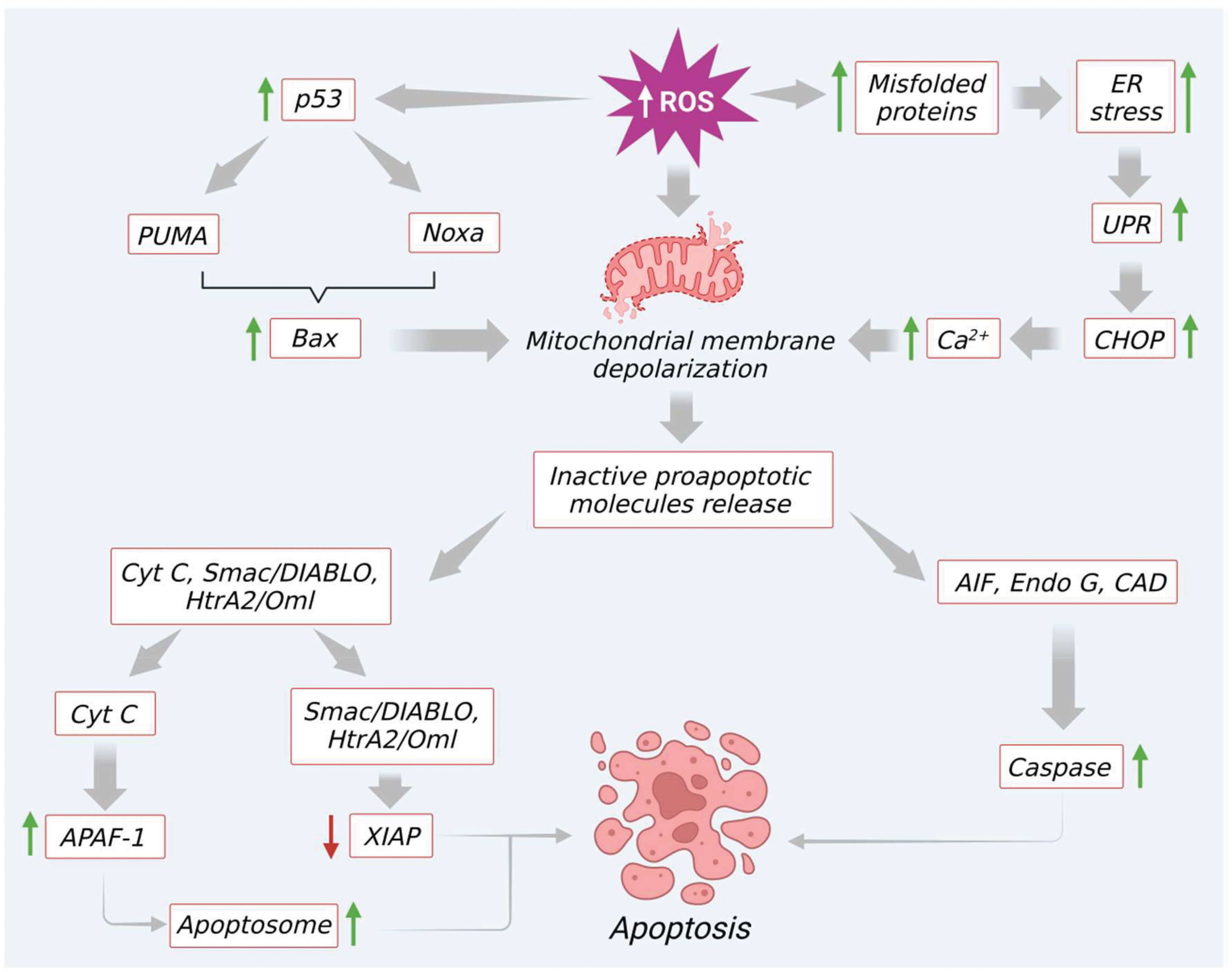
Figure 3 Excessive ROS generation induce mitochondrial mediated apoptosis through modulation of various signaling molecules.
Furthermore, an elevated level of ROS or prolonged oxidative stress induces endoplasmic reticulum (ER) stress via increasing protein misfolding. This intrinsic pathway promotes the unfolded protein response and activates C/EBP homologous protein (CHOP) by triggering the induction of IRE1α, ASK, and p38 MAPK proteins. This finally leads to apoptosis by upregulation of Bcl-2 apoptotic protein. At the same time, excess ROS also prompts the discharge of Ca2+ from the ER lumen. Furthermore, due to the proximity of mitochondria to the ER, a maximum amount of Ca2+ is absorbed into mitochondria. This condition leads to Ca2+ overload in mitochondria and initiates the opening of MPTs, and facilitates the release of molecules like ATP and cytochrome c. These molecules further enhance ROS production and apoptotic signaling, as shown in Figure 3.
On the other hand, ROS also induces apoptosis via the regulation of signaling molecules involved in the extrinsic pathway. In this pathway, several death receptors like death receptor 1 (DR1) or tumor necrosis factor receptor 1 (TNF-R1), death receptor 2 (DR2) or type-II transmembrane protein (Fas), death receptor 3 (DR3) or TNF receptor family (TRAMP), death receptor 4 (DR4) or TNF-related apoptosis-inducing ligand-receptor-1 (TRAIL-R1), death receptor 5 (DR5) or TNF-related apoptosis-inducing ligand-receptor-1(TRAIL-R2) and death receptor 6 (DR6) or TNF receptor superfamily member 21(TNFRSF21), that are derived from the TNF-R superfamily, have been found to be involved prominently in the apoptosis process. Generally, the extrinsic apoptotic pathway gets triggered via induction of transmembrane death receptors through interacting with various receptor-specific ligands such as Fas ligand (FasL), TNF-α, Apo3 ligand (Apo3L), and Apo2 ligand (Apo2L) (53). However, ligands like FasL, TNF-α, and Apo are mainly produced by activated macrophages and interact with specific death receptors, thereby initiating the apoptosis process in the cancer cells (54, 55). In the first step, the FasL and TNF-α interact with transmembrane proteins Fas or DR2 and TNFR1 or DR1 that consist of respective death domains. Both death receptors initiate apoptosis signaling in different manners.
In the TNF-α/TNFR1 pathway, the immediate interaction of TNF-α ligand to its receptor initiates the activation of TNFR1 via trimerization (Figure 4). This step engages other corresponding adapter molecules like TNFR type 1-associated DEATH domain protein (TRADD) and forms a complex in the cytoplasm. This complex activates and attracts a few more partners or signaling molecules, which decides the fate of the pathway (either cell survival or cell death). In this process, the TRADD complex attracts and activates other signaling molecules like tumor necrosis factor receptor 2 (TRAF2) and receptor-interacting protein kinases (RIP). This, in turn, triggers NFκB and MAPK-mediated cell survival process by increasing the levels of antioxidants as well as anti-apoptotic proteins such as Bcl-XL, X-linked inhibitor of apoptosis protein (XIAP), and inhibitors of apoptosis proteins (cIAP 1, 2) (53, 56) (Figure 4). On the other hand, the TRADD complex also stimulates apoptosis via recruiting FAS-associated death domain (FADD) protein. This complex promotes procaspases 8 and 10, which regulate the expression of caspases 3, 6 and 7 as shown in Figure 4.
Similarly, FasL activates apoptosis by binding to its corresponding receptor DR2 or Fas. This step activates the DR2 or Fas receptor via trimerization and recruits the other adaptor molecules like FADD. These linkages further recruit pro-caspase-8 through DED. This finally promotes the generation of DISC and triggers the downstream caspases such as caspases 3 and 6 and initiates the apoptotic signals in the cancer cells by inducing apoptotic features like nuclear condensation, DNA damage, and membrane blebbing (Figure 4).
In the same way, excessive ROS levels also initiate apoptosis via activation of PI3K/Akt/mTOR signaling pathway by altering the binding activity of various hormones and growth factors to their respective receptors. This evidence-based study specifically highlights the IGF-1 and its role in apoptosis. Several reports suggest that excessive intracellular ROS production trigger HIF-1α mediated apoptosis in cancer cells via activation of IGF-1 binding protein 3 (IGFBP-3) and inhibition of IGF-1 signaling. This step blocks the binding of IGF-1 to its receptor and subsequently inactivates the downstream signaling molecules like PI3K, Akt, and mTOR that help in tumor growth and progression, shown in Figure 5. Finally, this inhibits the NF-κB protein and promotes apoptosis. Therefore, the phytocompounds like alkaloids that induce ROS and modulate different kinds of signaling molecules, including hormone-related growth factors, can be exploited in colon cancer treatment (57).
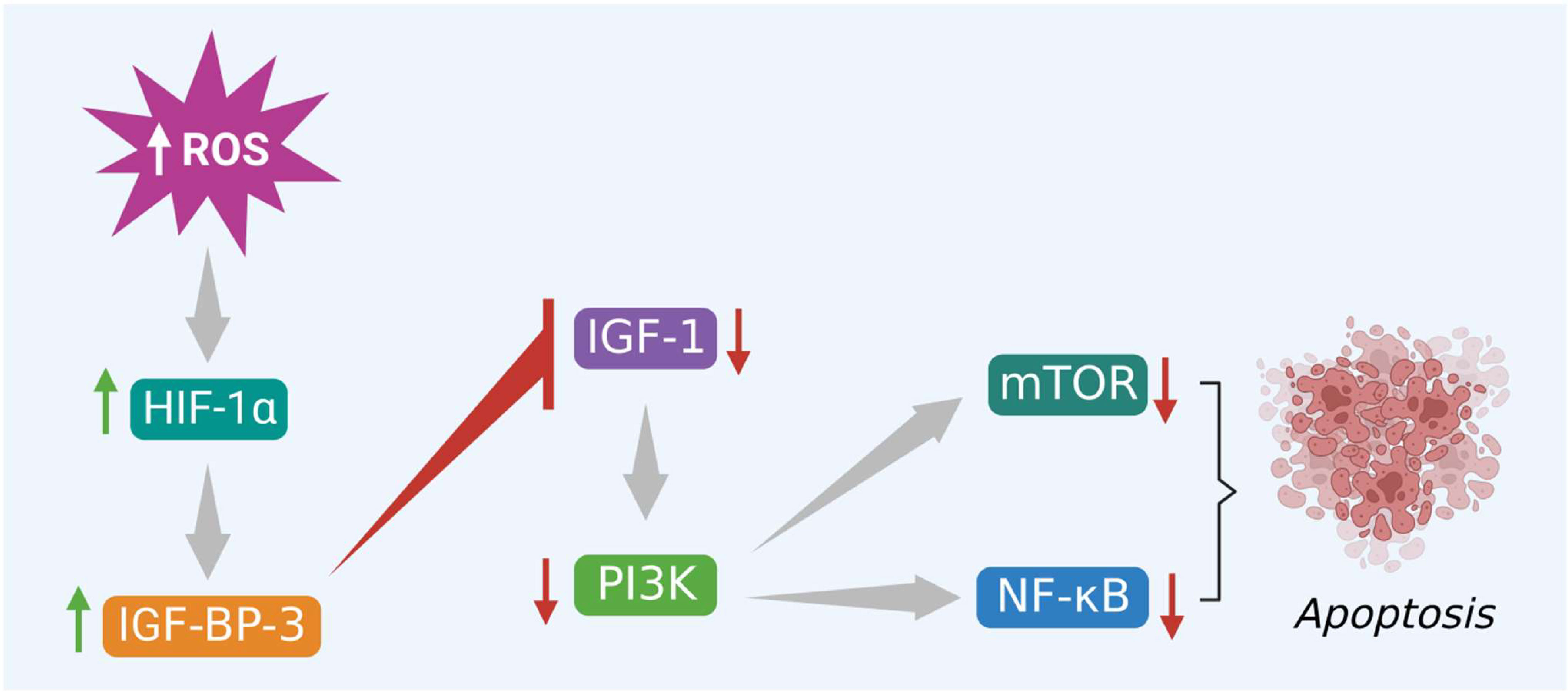
Figure 5 Excessive ROS levels induce apoptosis of cancer cells via activation of HIF-1α and its interlinked IGFBP-3 protein. IGFBP-3 inhibits PI3K/Akt/mTOR and triggers apoptosis.
5 Current treatment strategies of colon cancer and their limitations
Several studies revealed that colon cancer treatment depends on the tumor stage and location. In the early stage, a tumor can be surgically eliminated from the site. However, in the advanced stage, chemotherapy as a single drug or combined with other drugs or radiation is the only option. Among the chemotherapy drugs, fluoropyrimidines have been highly recommended for the treatment of colon cancer. However, fluoropyrimidine derivatives like 5-fluorouracil alone have not been found to be effective. In addition, it also exhibited multiple side effects such as neutropenia, stomatitis, nausea, alopecia, photosensitivity, cardiotoxicity, and sub-acute multifocal leukoencephalopathy. Therefore, its usage as a single drug is limited for colon cancer treatment (58). Subsequently, a platinum derivative named oxaliplatin has been introduced in the standard colon cancer treatment, and it was co-administered with 5-fluorouracil and leucovorin (folinic acid) to reduce the toxicity. This drug has specifically been used in the advanced stage of colon cancer. However, in clinical studies, oxaliplatin drug exhibited characteristic side effects as compared to other platinum derivatives including generation of neurotoxicity, hematotoxicity, gastrointestinal tract toxicity, neurolopathy, thrombocytopenia and nephrotoxicity. In addition to these toxicities, oxaliplatin increases CRC patients’ death rate when included in the treatment regimen (58, 59). Irinotecan hydrochloride, a camptothecin derivative, was introduced to improve the CRC treatment. This drug has been recommended in combination with 5-fluorouracil, leucovorin, and oxaliplatin to treat metastatic CRC. Interestingly, this combination increased the survival time by more than 30 months. However, the active metabolite of irinotecan (SN-38), an active metabolite of irinotecan hydrochloride showed severe toxicity, including neutropenia, by developing polymorphic glucuronidation. Additionally, two monoclonal antibodies such as cetuximab and bevacizumab, belonging to the class of EGFR and angiogenesis antagonists, respectively, have been added to the irinotecan hydrochloride treatment strategy. Cetuximab was also reported to specifically bind to and inhibit EGFR, whereas bevacizumab inhibited VEGF. Together with an irinotecan hydrochloride treatment regimen (comprising 5-fluorouracil/leucovorin), both cetuximab and bevacizumab are considered helpful in managing metastatic CRC patients. The inclusion of cetuximab can significantly reduce the progression of metastatic CRC. On the other hand, the inclusion of bevacizumab in the irinotecan hydrochloride treatment regimen can increase the survival rate of CRC patients by over 4 months as compared to other standard treatments. However, cetuximab, as a single drug or combined with irinotecan treatment, was associated with various adverse effects such as asthenia, leukopenia, abdominal pain, and neutropenia (60). Similarly, adverse effects like gastrointestinal hemorrhage and bowel ischemia have been associated with bevacizumab (61). Besides, panitumumab, another monoclonal antibody, and an EGFR inhibitor, have recently been introduced specifically to treat metastatic CRC patients with RAS wild type via interaction with PI3K/Akt/mTOR signaling molecules. This drug also showed severe toxicity related to skin including dermatitis, pruritus, paronychia, and erythema (62).
In addition, capecitabine, the first oral prodrug of 5-fluorouracil, was used in combination with intravenous irinotecan. However, this combination was administered with or without bevacizumab to treat metastatic CRC. Capecitabine administration was associated with toxicities such as hand-foot syndrome, leukopenia, and proteinuria. In addition, this drug has also shown toxicities like 5-fluorouracil (63, 64). Another prodrug of 5-fluorouracil, known as tegafur, is orally used to treat colorectal cancer liver metastases in combination with 5-fluorouracil. This combination blocks the crucial metabolizing enzyme of 5-fluorouracil and increases the serum concentration of 5-fluorouracil. Hence, in the current treatment regimen, this combination along with oral leucovorin has been included as a typical treatment for stage III colon cancer. Moreover, tegafur also shows serious side effects like neutropenia, thrombocytopenia, mucositis, and asthenia (65, 66). Recently, regorafenib, a kinase inhibitor has been approved for the treatment of metastatic CRC. This drug inhibits various kinases such as fms-related receptor tyrosine kinase 1 (FLT1), TEK receptor tyrosine kinase, rapidly accelerated fibrosarcoma (Raf-1) proto-oncogene, KIT proto-oncogene receptor tyrosine kinase, serine/threonine kinases, and kinase insert domain receptor (KDR). This drug has also proved its efficacy by increasing the survival time by 2.5 months. Moreover, this drug shows few side effects compared to other currently used chemotherapeutic drugs (64). Recently, another monoclonal antibody named dostarlimab, an anti-programmed cell death protein PD-1, has been introduced in colon cancer treatment regimen. The clinical study results show that dostarlimab can completely eradicate colon cancer. Moreover, this drug has also exhibited minimal toxicity in the CRC patients (67). Another monoclonal antibody, a PD-1 inhibitor, nivolumab was introduced to treat microsatellite instability high CRC. The study results revealed that nivolumab possess a better disease control rate of microsatellite instability high CRC (68). At the same time, when co-administrated with ipilimumab (a CTLA4 inhibitor), nivolumab improved cancer conditions and showed 80% disease control rate (69–71). Similarly, another monoclonal antibody named prembrolizumab was introduced to efficiently treat microsatellite instability high defective DNA mismatch repair CRC with a much higher rate of overall response and progression free survival rate. In the study, prembrolizumab has been found to increase the survival time by 8.3 months and the overall survival rate by 43.8% (72). However, almost all existing drugs, including immunotherapy, sooner or later have been becoming less effective, mainly due to resistance, associated toxicities, and immune escape (73, 74), as detailed in Table 1. Hence, there is an urgent need to develop a potential therapeutic tool against CRC. Among the various sources, medicinal plants and their derived compounds play essential roles in drug discovery. They show various pharmacological functions, including anti-cancer and immunomodulatory effects. Several plant derived compounds as such or combined with other clinical drugs have been used to treat various cancers. Phytocompounds like berberine, epigallocatechin, and lycopene have recently undergone clinical trials to manage colon cancer at different stages. Hence, this evidence-based study highlights phytochemicals, specifically the alkaloids that induce ROS-mediated apoptosis.
6 Role of phytocompounds in inducing ROS mediated apoptosis in colon cancer
Cancer is the second leading cause of mortality and a substantial global health concern. Despite significant advancements in treatment modalities, cases of various cancers and deaths have still been on the rise. In 2020, nearly 19.3 million new cancer cases and 10 million new deaths have been reported, accounting for the extreme increase of cases and deaths worldwide (75). Among the various cancers, CRC stood second in terms of cancer-related deaths (6, 75). This continuous rise in CRC cases is due to the lack of efficient screening and treatment methods. In addition, colon cancers were being detected at an advanced stage or at metastasis stage in most cases, which made it difficult to treat the patients. Moreover, currently recommended treatments such as surgery, radiation, chemotherapy, and combination treatments also exhibit serious challenges like non-specificity to cancer cells, side effects as well as drug resistance, thus limiting the efficacy of various treatment options (76).
Given the severity of the disease, there is an urgent need to find a novel treatment with minimal toxicity to the CRC patients. Due to the never-matched chemical library and other favorable factors, medicinal plants and derived phytochemicals act as potential resources for novel drug discovery against various diseases, including cancer (77, 78). In fact, plant secondary metabolites such as alkaloids, terpenoids, flavonoids, steroids, saponins, and phenolic compounds have been held responsible for their antitumor activity as well as other biological functions of the medicinal plants (76, 79, 80). For example, taxol, vincristine, vinblastine, and podophyllotoxin form the primary secondary metabolites with proven anticancer potentials via modulating various signaling molecules of death pathways (78, 81, 82). However, studies revealed that in most cases these anticancer phytochemicals also show toxicity towards non-cancerous cells, which is a serious challenge to the treatment (83). Hence, there is an urgent need for unique and ideal therapeutic tools that can selectively target cancer cells alone.
Generally, cancer cells require a high level of ROS to run normal biological functions such as differentiation and development and maintain an increased metabolic rate. In addition, ROS at the required level also help in tumor progression, metastasis, and angiogenesis. However, the level of ROS exceeds the limit, leading to oxidative damage to the cells, specifically in cancer cells (27). This discrete damage to cancer cells alone is due to the increased mitochondrial dysfunction, which makes the cancer cells more sensitive to oxidative stress than the normal cells. Hence, a slight increase in ROS levels by using external agents can induce cell death through various death mechanisms in cancer cells. Among the different kinds of programmed cell deaths, apoptosis is a well-studied and vital pathway involved in several diseases, including cancer. In cancer, the malignant cells maintain shallow levels of apoptosis, which result in tumor growth and progression. Hence, forced activation of apoptotic signaling molecules via up-regulation of ROS through introduction of small molecules from various sources can help to treat cancer better. Mounting evidence also suggests that medicinal plants and their derived components are better sources for novel drug discovery because of their minimal toxic effects.
Among the several classes of phytocompounds, alkaloids serve as a vital source for identification of novel drug candidates against various diseases including cancer. In addition, they can also induce ROS-mediated cell death by regulating various intrinsic and extrinsic apoptotic signaling molecules in different cancers, including colon cancer (24, 84). Alkaloid compounds that induce ROS-mediated apoptosis in colorectal cancer have been discussed in detail. Additionally, various classes of phytocompounds that initiate ROS-mediated apoptosis in different cancer cells are mentioned in Supplementary Tables 1–6. In this way, the current evidence-based review serves as a complete reference for all kinds of phytochemicals that promote ROS-associated apoptosis via targeting various signaling molecules, including IGF-1 in cancer cells.
6.1 Alkaloids as anti-colon cancer agent
Alkaloids are plant-derived naturally occurring secondary metabolites. These compounds mostly contain nitrogen as the heteroatom, which imparts basic nature to these molecules. There are several kinds of alkaloids based on heterocyclic rings that show numerous medicinal potentials against malaria, neurodegenerative diseases, viral infections, arrhythmia, hepatitis, asthma, bacterial infections, hypertension, eye infections as well as different kinds of cancers, including colon cancer (85). Various kinds of alkaloids that induce ROS-mediated apoptosis in colon cancer, either alone or in combination with other drugs, are discussed below.
6.1.1 Isoquinoline alkaloids
These compounds are known to show potential anti-colon cancer activity via targeting cancer cells alone through initiating ROS-dependent apoptosis. Evidence suggests that benzophenanthridine alkaloids belonging to the class of isoquinoline type of alkaloids can trigger ROS-mediated cell death in cancer cells. Among this class of compounds, sanguinarine is a naturally derived alkaloid isolated from Macleaya cordata, belonging to the family Papaveraceae. This compound killed the colon cancer cells like human colon adenocarcinoma (SW480) and human colon cancer (HCT116), as well as reduced the SW480 and HCT-116 cell-generated tumor growth in an orthotopic model of nude BALB/c mice. Sanguinarine also induced intrinsic apoptotic cell death in colon cancer cells via increasing ROS-mediated mitochondrial permeabilization and releasing important signaling molecules like cytochrome c and ATP. In fact, apoptosis was initiated through the activation of Bax (dependent), which disrupted the association of serine-threonine kinase receptor-associated protein (STRAP) and maternal embryonic leucine zipper kinase (MELK) proteins. Then ultimately activated the caspase 3 protein and its function (86). In another study, sanguinarine induced ROS mediated apoptotic cell death in colon cancer cells through DNA damage wherein the apoptotic activity was p53 independent (87). In addition, 6-methoxydihydrosanguinarine, a natural sanguinarine derivative, induces apoptotic cell death in various cancer cells via ROS production (88). Berberine, another isoquinoline type of alkaloid isolated from the Berberis genus, also produces an anti-colon cancer effect by upregulating ROS and apoptotic signals. In a study, this compound was reported to trigger apoptosis through ROS generation in colon cancer cells like human colorectal adenocarcinoma (HT-29) and HCT-116 cells via increasing the expression of lnc RNA cancer susceptibility candidate 2 (lncRNAs CASC2). lncRNA CASC2 later interacts with AU-rich element RNA-binding factor 1 (AUF1) to block the translation of Bcl-2 triggering apoptosis (89). This compound also initiated apoptotic mediated cell death in mouse conditionally immortalized epithelial (IMCE) cells that were inherited with adenomatous polyposis coli (Apc) multiple intestinal neoplasias (min) mutation. This investigation also revealed that berberine kills IMCE via inducing ROS, which initiates the release of AIF from mitochondria and triggers apoptosis in caspase-independent manner. Moreover, berberine did not show toxicity towards normal colon epithelial cells i.e., young adult mouse colon epithelial cells (90). In addition, berberine inhibits the migration of SW480 and HCT-116 cells via activation of AMPK through upregulation of ROS and downregulation of glucose levels. Thus activated AMPK decreases the activity of integrin β1 protein and initiates cell death (91). In another investigation, berberine showed ROS-mediated apoptosis in human colon carcinoma (SW620) cells by increasing JNK, c-Jun, and p38 MAPK phosphorylation. On the other hand, these compounds also enhance the release of cytochrome c and activate caspases 3 and 8 to support Fas-mediated apoptosis (92). Li and the team also studied the effect of berberine on azoxymethane or dextran sulfate sodium-induced colon tumors in mice. In their study, berberine reduced the tumor size to 60% via activation of AMPK and reduction of mTOR activities. They concluded that the tumor regression activity is associated with reduced activity of cyclin D1, nonhistone nuclear protein (Ki-67), cyclooxygenase-2 (COX-2), survivin, NF-κB, and increased activity of caspase-3 function. This compound also inhibits the migration and metastasis of SW620 and human colon cancer cell line (LoVo) cells via down-regulating the expression of COX-2, prostaglandin E2 (PGE2), Janus kinase 2 (JAK2), and STAT3 signaling molecules. Besides, it also suppresses the solid tumor growth in BALB/c mice and lung metastasis developed by SW620 and LoVo cells (93, 94). On the other hand, berberine inhibits colon tumor growth via targeting signaling molecules like TNF-α, EGFR, and Wnt/β-catenin signals (95–97). Furthermore, recent combination studies of berberine with Andrographis paniculata extract also showed an excellent anti-colon cancer effect, where they inhibited the growth of HT-29 cells and colon carcinoma cell line (RKO) cells and their corresponding in vivo tumor growth. This activity was initiated by an increase in the ROS levels, which finally altered the genes involved in DNA replication (98). Furthermore, when combined with evodamine an indole alkaloid, berberine enhances the anti-tumor activity. This combination promotes apoptosis in cancer coli-2 (Caco-2) and HT-29 cells via modulation of Nrf2-mediated pathways (99). Cepharanthine, a biscoclurine or bis benzylisoquinoline alkaloid extracted from Stephania cepharantha Hayanta, also significantly induced oxidative stress-mediated apoptosis in p53 mutated colon cancer cells HT-29 and SW620 cells. Cepharanthine initiated apoptosis in HT-29 cells, which is linked with cell cycle arrest at growth 1 (G1) phase and cyclin-dependent kinase inhibitor 1 or CDK-interacting protein 1 (p21Waf1/Cip1) level increase (100). Coptisine, another isoquinoline alkaloid extracted from Coptis chinensis Franch, reportedly triggered ROS-dependent apoptosis in HCT-116 cells. It reduced the growth and migration and finally promoted caspase-mediated apoptosis in HCT-116 cells by altering mitochondrial membrane potential as well as modulating various signaling molecules like Bcl-2, Bcl-XL, XIAP, Bax, Bad, cytochrome c, Apaf-1, AIF, caspase-3, PI3K and Akt. In addition, coptisine reduces the HCT-116 generated tumor growth by inducing apoptosis in nude BALB/c mice (101). In another study, this compound was reported to induce cell cycle arrest at G1 phase as well as inhibit HCT-116 xenograft tumor growth via targeting Milk Fat Globule EGF And Factor V/VIII Domain Containing (MFG-E8) gene and rat sarcoma- extracellular signal-regulated kinase (RAS-ERK) pathways (101–103). Moreover, alkaloids like neferine and isoliensinine isolated from Nelumbo nucifera (lotus) plants have shown significant anticancer potential by enhancing the chemo- sensitivity of colon cancer cells towards cisplatin treatment. These two alkaloids and cisplatin increased the ROS levels in human CRC (HCT-15) cells, leading to caspase-3 dependent apoptosis through the deactivation of MAPK/PI3K/Akt/mTOR signaling molecules. In addition, this combination was also found to decrease the resistance towards cisplatin and increase apoptotic markers in colon cancer stem cells via uplifting Ca2+ level and damaging mitochondrial membrane integrity (104, 105). Recent studies revealed that palmatine, another isoquinoline alkaloid, induces apoptosis in colon cancer cells like HCT-116, SW480, HT-29, and, in HCT-116 cell xenograft tumor via increasing ROS levels and decreasing mitochondrial membrane potential. This leads to release of cytochrome c protein. Palmatine is believed to target various signaling molecules such as aurora kinase A (AURKA), Bcl-xl, Bcl2, caspase 3 and 9 to induce apoptosis (106). Tetrandrine, a bisbenzylisoquinoline alkaloid derived from the roots of Stephania tetrandra, also triggers oxidative stress-mediated cell death in HCT-116 cells through modulation of E2F transcription factor 1 (E2F1) and p53/p21Cip1 levels (107, 108). Nano formulation of this alkaloid showed an enhanced anticancer effect against Lovo cells through ROS-mediated upregulation of JNK and caspase-3 activity (109). Similarly, xylopine, an isoquinoline alkaloid isolated from the stem of Xylopia laevigata, also induces oxidative stress-mediated apoptosis in HCT-116 cells by triggering apoptosis in p53 independent manner via induction of cell cycle arrest at growth 2 (G2)/mitosis and cytokinesis (M) phase, and caspase-3 activity (110). For more clarification, the above-mentioned compounds are presented in the Supplementary Table 7.
6.1.2 Indole alkaloids
Indole alkaloids also show a prominent anticancer effect. Camptothecin, a well-studied compound under the class of indole alkaloids, extracted from Camptotheca acuminata belonging to the family Nyssaceae showed potent anticancer effects (111). Evidence suggests that this molecule exhibits anti-tumor activity against various cancer cells, including colon cancer. Notably, the anticancer effect of camptothecin is associated with the apoptosis mechanism, which is mediated by ROS (112). A study by Park and colleagues reported that camptothecin kills the HCT-116 cells through activating apoptosis signaling associated with TNF-related apoptosis-inducing ligand (TRAIL). This study also found that camptothecin induces DNA damage and activates Bax, p53, and p21 proteins. This step finally leads to the death of colon cancer cells. Their investigation also proved that hypoxia condition reduces the apoptotic cell death of HCT 116 cells via decreasing Bax. The study concluded that ROS are essential for camptothecin’s anti-tumor activity (113). In addition, camptothecin, along with other chemotherapeutic drugs, initiates apoptosis in colorectal adenocarcinoma (DLD1) and HCT-116 colon cancer cells via increasing mitochondrial fission and decreasing complex I activity as well as mitochondrial size. This mitochondrial change provokes excess ROS production, which helps to activate apoptotic signaling (114). Furthermore, Wenzel and coworkers found that camptothecin at 50µM can induce apoptotic cell death in HT-29 colon cancer cells through upregulating caspase-3 activity via increasing mitochondrial superoxide ROS production. This caspase-3 activation triggers the apoptotic characteristics, like loss of plasma membrane integrity and nuclear fragmentation, in the HT-29 cells. In addition, this study also revealed that camptothecin modulates various signaling molecules like Bcl-2, Bax, Bak, p21, COX-2 and NF-κB in HT-29 cells, which promote apoptosis. However, ascorbic acid treatment at 50uM reverses the changes made by camptothecin in various signals and inhibits the apoptosis process via scavenging the superoxide radicals in HT-29 cells (115). In another study, Guo et al. showed that prodrug formulation of camptothecin via conjugating with lysine or arginine amino acids enhances water solubility, tumor penetration capacity as well as pharmacokinetic properties. This study also proved that prodrug formulation of camptothecin increases the mitochondrial ROS production much better than the camptothecin alone, thereby decreasing the murine colorectal carcinoma (CT-26) cells induced tumor size in Bagg albino (BALB/c) mice via initiating apoptosis process (116). Besides, the semi-synthetic derivative of camptothecin like irinotecan hydrochloride (IH) also shows anti-colon cancer activity. Moreover, SN-38, the active metabolite of IH, significantly improves the patients’ colon cancer condition and survival rate up to 30 months. Nowadays, IH is highly recommended to treat metastatic CRC in combination with other clinical drugs like 5-fluorouracil and oxaliplatin (117). A study conducted by Britten and his colleagues showed that irinotecan reduces the growth of HT-29 cells generated tumors drastically when co-administered with other compounds like 6-hydroxymethylacylfulvene and 5-fluorouracil (118). In another experiment, Allegrini and his team demonstrated that irinotecan, when co-administered with the antiangiogenic drug thrombospondin-1 (TS-1) to nude mice bearing HT-29 cells generated tumor, a significant reduction in the size of the tumor occurs as compared to the control as well as individual treatment. At the same time combination of TS-1 with irinotecan did not affect much growth of HT-29 cells (119, 120). Moreover, this combination is non-toxic to the normal cells. In another study, an anti-folate agent used in combination with SN-38, an active metabolite of irinotecan to treat 5-fluorouracil resistant HT-29 cells and found a significant growth inhibition of the cells. This combination also potentially reduced the HT-29 cell-oriented solid tumor in BALB/c nude mice (119, 120). Results from the clinical study also revealed that when irinotecan was given in combination with oxaliplatin, capecitabine, and bevacizumab for 8 cycles to a patient suffering from advanced tumor provided promising results by increasing the survival time. Similarly, irinotecan co-administered with 5-fluorouracil and oxaliplatin also showed a significant recovery in advanced colon cancer patients (121, 122). Additionally, all the indole alkaloids that induce ROS mediated apoptosis in colon cancer are presented in Supplementary Table 7 for detailed clarification.
6.1.3 Capsaicinoids
Capsaicinoids are another alkaloid class that induce ROS-mediated apoptosis in colon cancer cells. Capsaicin is a significant alkaloid that belongs to the class of capsaicinoids, reported to up-regulate apoptotic signals in colon cancer cells through increasing intercellular ROS (123). In a study conducted by Yang and his team, capsaicin was found to reduce the viability of colon cancer cells like human colon carcinoma (Colo320DM) and LoVo cells through initiating caspase-dependent apoptosis, which is linked with an increase in intracellular ROS production and altering mitochondrial membrane potentials (124). In another investigation, a group of researchers reported that capsaicin can trigger apoptosis in HT-29 colon cancer cells via activating peroxisome proliferator-activated receptor –γ (PPAR- γ) as well as AMPK but not the vanilloid receptor (125). Lu et al. showed that this compound induces apoptotic cell death in colo 205 cells as well as its corresponding tumor xenograft through targeting various signaling molecules like Fas, cytochrome c, Bax, Bcl-2 p53, p21, and caspases 3, 8 and 9. In addition, this apoptotic activity is strongly associated with increased intracellular ROS, Ca2+ production, and damage to mitochondrial membrane integrity (125–127). Furthermore, capsaicin in combination with 3,3′- diindolylmethane enhances the anti-cancer potential by inducing apoptosis in colon cancer cells like HCT116, SW480, LoVo, Caco-2, and HT-29 via targeting apoptotic markers like Fas, NF-κB, p53 and other proteins (128). Capsaicin, combined with resveratrol, have also been reported to induce apoptotic cell death in HCT-116 cells by increasing nitric oxide (NO) concentration and p53 level. The increase in p53 level decreases murine double minute 2 (Mdm2) and increases Bax expression to promote apoptosis (129). Supplementary Table 7 provides further information on capsaicinoids.
6.1.4 Carbazole alkaloids
Carbazole alkaloids constitute another plant-based alkaloid class, exhibiting an anti-colon cancer effect. Clausenidin is the one belonging to the carbazole alkaloid class, isolated from the roots of Clausena excavata and also reported to initiate caspase-9 dependent apoptosis in HT-29 cells through cell cycle arrest at quiescent phase (G0)/G1 phase, triggered by increasing ROS mediated mitochondrial membrane depolarization (130). More details can be found in Supplementary Table 7.
6.1.5 Diterpenoid alkaloids
Besides, diterpenoid alkaloids too exhibit anti-colon cancer effect (Supplementary Table 7). Lappaconitine hydrochloride (LH) is one such compound and a derivative of C 18-diterpenoid alkaloid. Song and colleagues suggested that this compound demonstrates an anti-colon cancer effect by inhibiting proliferation and increasing apoptosis in HCT-116 cells. Moreover, this activity is associated with increased ROS and decreased mitochondrial membrane potential. They also found that LH reduces the tumor volume of the HCT-116 cells-oriented xenograft model via altering the MAPK pathway (131).
6.1.6 Piperidine alkaloids
Piperine, a piperidine alkaloid obtained from black pepper also reported to trigger apoptotic cell death in HT-29 cells through cell cycle arrest at the G1 phase (Supplementary Table 7). This activity is achieved via targeting various markers, including cyclin D1, D2, and cyclin-dependent kinase inhibitor (p21WAF1 and p27KIP1) (132).
6.1.7 Amide alkaloids
Amide alkaloids are an essential group of alkaloids derived from plants. They also show potent anti-colon cancer effects (Supplementary Table 7). Piperlongumine is a critical compound from this class, extracted from the fruits of Piper species. Reports suggested this compound also induces apoptosis in HCT-116 via increasing ROS and decreasing antioxidant enzyme glutathione S-transferase. It targets JNK, MEK, and ERK proteins but not Bax, p21, and p53 to induce selective apoptosis in colon cancer cells. In addition, piperlongumine is also used in combination with oxaliplatin, a first-line drug of choice for metastatic colon cancer. This combination enhanced anti-colon cancer activity by increasing the level of ROS-mediated apoptosis, which is linked with upregulation of mitochondrial dysfunction and ER related apoptotic markers in HCT-116 and LoVo cells (133, 134).
6.1.8 Carboline alkaloids
Carboline alkaloids also show prominent anti-cancer potential (Supplementary Table 7). Kim et al. (135) reported another alkaloid harmine, a carboline derivative, extracted from Peganum harmala. This compound can induce ROS-dependent apoptosis in HCT-116 cells. In a study, they investigated the anti-colon cancer potential of harmine hydrochloride. This compound found found to activate apoptotic cell death in HCT-116 cells through increasing apoptotic [capases 3 and 9, poly (ADP-ribose) polymerases (PARP)] as well as pro-apoptotic proteins (Bax) and decreasing anti-apoptotic protein (Bcl-2) markers. These investigations also revealed that the harmine hydrochloride compound mainly alters ERK/PI3K/Akt/mTOR signaling pathway to initiate apoptosis. In addition, this compound also triggered apoptosis in SW620 cells by inducing arrest at the sub-G1 phase and decreasing mitochondrial membrane potential. Notably, harmine promotes caspase-dependent apoptotic cell death in SW620 cells through downregulation of ERK/PI3K/Akt/mTOR-related pathways (45, 135, 136).
6.1.9 Quinolizidine alkaloids
Likewise, a study revealed the anti-colon cancer effect of oxymatrine (Supplementary Table 7), a quinolizidine alkaloid extracted from the roots of Sophora flavescens – a Chinese traditional medicine. These compounds induce apoptosis in colon cancer cells via ROS generation. Moreover, earlier reports suggests that co-administration of oxymatrine with doxorubicin produces a better anti-colon cancer effect via enhancing apoptotic signals (cleaved caspase-3, cleaved caspase-9 and Bax/Bcl-2 ratio) in HT-29 and SW620 cells mediated by ROS generation. In addition, this combination also reduces the growth of HT-29 generated tumor xenograft in a dose dependent way via modulating E-cadherin and N-cadherin level. Besides, oxymatrine also increases the sensitivity of 5-fluorouracil resistant cells like HCT-8 and initiates apoptosis by promoting inhibition of EMT and NF-κB activity (137, 138).
6.1.10 Proto alkaloids
In a few studies, researchers reported colchicine, a proto alkaloid, to show an anti-colon cancer effect through up-regulation of ROS and its associated apoptotic markers. They showed that colchicine induces apoptosis in HT-29 cells by regulating ROS and decreasing mitochondrial membrane integrity. This compound was ultimately reported to trigger apoptosis by activating BAX, caspase 3, p38 and deactivating AKT signaling molecules. In another investigation, this compound reportedly initiated ROS-mediated apoptosis in HCT-116 and Colo-205 cells by depolymerizing microtubules and arresting the cell cycle at the G2/M transition state (139, 140).
6.2 Alkaloids and other phytocompounds in clinical trials against colorectal cancer
Existing drugs for colon cancer treatment have been showing various side effects, including resistance. Therefore, there is a rise in demand for novel therapeutics to overcome the toxicities and limitations (2). Multiple pieces of evidence support medicinal plants and their derived phytochemicals as a suitable and potent source of drug discovery against various diseases. In this evidence-based study, various classes of phytocompounds have been discussed, including the alkaloids that are of clinical value for colon cancer treatment. Such compounds include berberine, epigallocatechin-3-gallate (EGCG), curcumin, lycopene, fisetin, and resveratrol. These compounds have recently been used in different phases of clinical trials against CRC. In a clinical study, a patient suffering from CRC was advised to take 0.3 g of berberine daily two times, and there was no sign of a tumor in the later stage. Berberine was strongly recommended to decrease the chances of recurrence of colorectal adenoma and polypoid lesions. Moreover, this drug was safe and showed no severe side effect (33). In addition, EGCG is also in the early stage of CRC clinical trials. The chemopreventive effect of Teavigo (purified tea extract with 94% EGCG) has been evaluated. CRC patients with curative resections were administered orally with 450 mg of EGCG twice daily. Blood was drawn from the patients at 0, 3, 6, 9, 12, 15, and 18 months and a colonoscopy was done after a year (141). Furthermore, lycopene, a carotenoid, was also tested against metastatic CRC and was found to decrease skin toxicity associated disease as a single drug or in combination with panitumumab (31). Likewise, resveratrol, a stilbenoid compound, was also studied against metastatic CRC with liver metastases. A 5.0 g daily dose of the compounds administered for two weeks, could markedly induce caspase-dependent apoptosis in metastatic liver cells of CRC patients (142, 143).
Similarly, curcumin, a polyphenol compound, was also effective against various cancers, including CRC. In addition, some other phytocompounds that act as immune boosters or immune modulators help in blocking colon tumor progression. One such compound is fisetin, a flavonoid compound, evaluated in patients suffering from CRC for its anti-inflammatory effect. CRC patients under chemotherapy were fed with 100 mg of fisetin for 7 weeks. At the end of chemotherapy, various inflammatory markers were evaluated, and fisetin was found to reduce the levels of IL-8 and high-sensitivity C-reactive protein (hs-CRP) in CRC patients. Hence, fisetin may be suggested as an adjuvant treatment to the conventional therapies followed to CRC patients (32). Other details regarding the phytocompounds that are under clinical trials aere provided in Table 2.
7 Conclusions and future perspective
Colon cancer globally occupies the third and second positions regarding of prevalence and death rate, respectively. However, there is a vast improvement in the screening and early detection techniques as well as treatment schedules. Therefore, the CRC death rate has slightly decreased in recent times. Yet the incidence of the disease is increasing. This is due to the rise of multiple side effects and limitations in the existing therapies that are followed against CRC. Hence, there is an immediate need for typical, potential, and alternate treatment regimens that can overcome the existing limitations. Several documented evidence suggests that phytocompounds that induce ROS-mediated apoptosis in cancer cells could pose a better and alternative cancer treatment. Moreover, these compounds exhibit minimal toxicity and high specificity to cancer cells in most cases. Their selective cytotoxicity of ROS induced on cancer cells are due to the features like continuous mitochondrial dysfunction and compromised antioxidant defense mechanism. This leads the cancer cells to become more susceptible to oxidative stress. Therefore, slight increase of ROS than the threshold limit by using external agents like phytocompounds can lead the cancer cells to oxidative stress induced apoptotic cell death. However, normal cells can nullify the minimal ROS due to its proper antioxidant defense mechanism and escape from the ROS mediated cell death. Hence, researchers now-a-days focus on plants and their derived compounds that induce ROS for identifying novel drug candidates against various diseases including CRC.
This evidence-based study discusses important plant-based alkaloids that induce cell death via ROS-mediated apoptosis in colon cancer. Alkaloids can induce ROS-associated apoptosis via altering multiple signaling molecules that involve various vital characters like proliferation (Ki-67, STRAP, MELK, JNK, PI3K/Akt/mTOR/Wnt/β-catenin, ERK, MEK, MAPK-p38), cell cycle (cyclin D1, D2, surviving, c-Jun), inflammation (TNF-α, COX-2, NF-κB), oxidative stress (Nrf2, GSK-3β), tumor suppression (p53, E2F1, AMPK, PPAR- γ), metastasis or angiogenesis (IGF-1, MFG-E8, integrin β1, ERK, EGFR, E-cadherin, N-cadherin, PGE2) and apoptosis (cytochrome C, AIF, Bax, p21, p21WAF1, p27KIP1, p53/p21Cip1, caspases 3 and 9, XIAP, Bcl-2, IncRNA CASC2, AUF1, PARP) of colon cancer cells (Figure 6). Many of these alkaloids have shown promising results in various colon cancer preclinical studies. For example, alkaloids like coptisine, camptothecin, capsaicin, lappaconitine, oxymatrine, sophoridine, aleutianamine, and palmatine have shown positive results in different kinds of preclinical models and effectively reduced colon tumor growth. Similarly, preclinical and clinical data suggest berberine (an isoquinoline alkaloid) as a future drug candidate to treat colon cancer. Moreover, berberine works well against CRC and shows negligible toxicity. Furthermore, most alkaloids currently available in the market against various diseases are largely extracted from medicinal plants. Therefore, plant-derived alkaloids provide a potential and novel therapeutic source for effectively managing CRC.
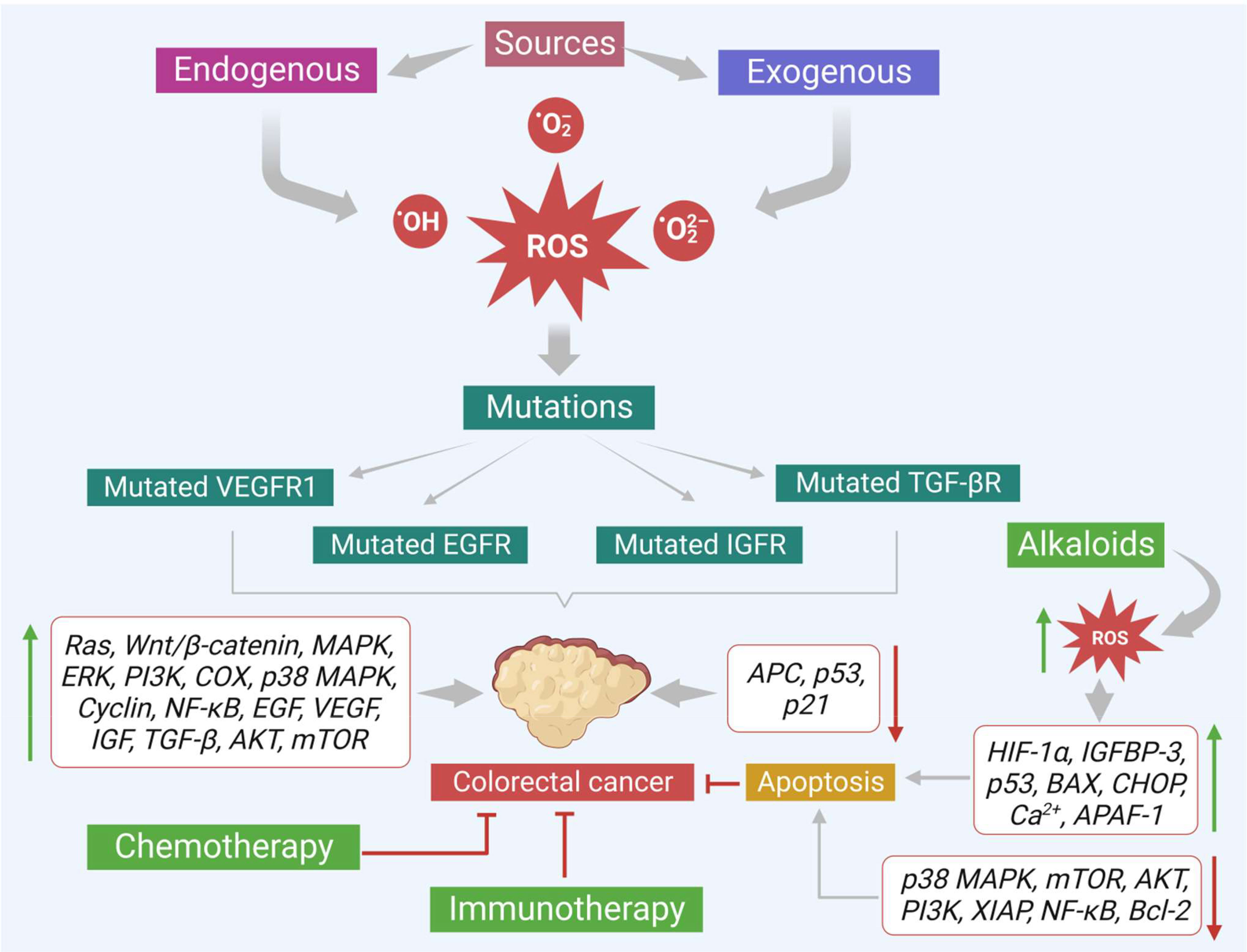
Figure 6 Various sources that promote colon cancer progression. Effects of alkaloids in reducing the colon cancer growth via increasing ROS production and modulating the important signals that trigger apoptosis are also shown.
Recent evidence suggests that diet and its related factors (hormonal alteration) are also the leading causes for the generation of 70 to 90% of CRC cases. Food material influences the gut microbiome composition (bacterial strains), which plays a vital role in the colon cancer progression. The gut microbiome is known to be occupied with two significant strains Bacteroides and Prevotella. However, a diet containing fruits and vegetables increases the Prevotella strain in the gut, which can break down the dietary phytochemicals in the lumen into tiny fatty acids like butyric acid. Multiple pieces of evidence revealed that butyric acid exhibits promising anti-cancer and anti-inflammatory effects. Taken together, dietary phytochemicals like alkaloids, which can modulate the gut microbiome, improve the host’s immunity, up regulate the intracellular ROS, and thus could be of promising therapeutic value against CRC. Hence, it may be concluded that consuming diet that contains fruits and vegetables that possess secondary metabolites like alkaloids can better control various kinds of cancers including colon cancer.
Author contributions
Conceptualization: VKN, SR; Writing—original draft: VKN, MN, JM, MS, NeKJ; Writing—review and editing: VKN, GB, YJ, OA, HS, AM, DN, AA, AJ, KKK, RRM, VM, NiKJ, AK, PS, SR; Illustrations: NiKJ; Supervision: PS, SR. All authors contributed to the article and approved the submitted version.
Acknowledgments
The authors thank Raghavendra Institute of Pharmaceutical Education & Research (Autonomous), Anantapuramu, Andhra Pradesh, India, and Assam University, Silchar, India for providing support. The authors would like to acknowledge BioRender (BioRender.com) for the illustrations.
Conflict of interest
The authors declare that the research was conducted in the absence of any commercial or financial relationships that could be construed as a potential conflict of interest.
Publisher’s note
All claims expressed in this article are solely those of the authors and do not necessarily represent those of their affiliated organizations, or those of the publisher, the editors and the reviewers. Any product that may be evaluated in this article, or claim that may be made by its manufacturer, is not guaranteed or endorsed by the publisher.
Supplementary material
The Supplementary Material for this article can be found online at: https://www.frontiersin.org/articles/10.3389/fendo.2023.1201198/full#supplementary-material
References
2. Nelson VK, Sahoo NK, Sahu M, Sudhan HH, Pullaiah CP, Muralikrishna KS. In vitro anticancer activity of eclipta alba whole plant extract on colon cancer cell HCT-116. BMC Complement Med Ther (2020) 20(1):355. doi: 10.1186/s12906-020-03118-9
3. Abane R, Mezger V. Roles of heat shock factors in gametogenesis and development. FEBS J (2010) 277(20):4150–72. doi: 10.1111/j.1742-4658.2010.07830.x
4. Zaytseva Y. Lipid metabolism as a targetable metabolic vulnerability in colorectal cancer. Cancers (Basel) (2021) 13(2):1–5. doi: 10.3390/cancers13020301
5. Dekker E, Tanis PJ, Vleugels JLA, Kasi PM, Wallace MB. Colorectal cancer. Lancet (2019) 394(10207):1467–80. doi: 10.1016/s0140-6736(19)32319-0
6. Sawicki T, Ruszkowska M, Danielewicz A, Niedźwiedzka E, Arłukowicz T, Przybyłowicz KE. A review of colorectal cancer in terms of epidemiology, risk factors, development, symptoms and diagnosis. Cancers (Basel) (2021) 13(9):1–23. doi: 10.3390/cancers13092025
7. Xi Y, Xu P. Global colorectal cancer burden in 2020 and projections to 2040. Transl Oncol (2021) 14(10):101174. doi: 10.1016/j.tranon.2021.101174
8. White A, Ironmonger L, Steele RJC, Ormiston-Smith N, Crawford C, Seims A. A review of sex-related differences in colorectal cancer incidence, screening uptake, routes to diagnosis, cancer stage and survival in the UK. BMC Cancer (2018) 18(1):906. doi: 10.1186/s12885-018-4786-7
9. Brahmkhatri VP, Prasanna C, Atreya HS. Insulin-like growth factor system in cancer: novel targeted therapies. BioMed Res Int (2015) 2015:538019. doi: 10.1155/2015/538019
10. Chainy GBN, Sahoo DK. Hormones and oxidative stress: an overview. Free Radic Res (2020) 54(1):1–26. doi: 10.1080/10715762.2019.1702656
11. Sahoo DK, Chainy GBN. Hormone-linked redox status and its modulation by antioxidants. Vitam Horm (2023) 121:197–246. doi: 10.1016/bs.vh.2022.10.007
12. Lukas M. Inflammatory bowel disease as a risk factor for colorectal cancer. Dig Dis (2010) 28(4-5):619–24. doi: 10.1159/000320276
13. Sahoo DK, Borcherding DC, Chandra L, Jergens AE, Atherly T, Bourgois-Mochel A, et al. Differential transcriptomic profiles following stimulation with lipopolysaccharide in intestinal organoids from dogs with inflammatory bowel disease and intestinal mast cell tumor. Cancers (Basel) (2022) 14(14):1–59. doi: 10.3390/cancers14143525
14. Gavrilas LI, Cruceriu D, Mocan A, Loghin F, Miere D, Balacescu O. Plant-derived bioactive compounds in colorectal cancer: insights from combined regimens with conventional chemotherapy to overcome drug-resistance. Biomedicines (2022) 10(8):1–14. doi: 10.3390/biomedicines10081948
15. Braun MS, Seymour MT. Balancing the efficacy and toxicity of chemotherapy in colorectal cancer. Ther Adv Med Oncol (2011) 3(1):43–52. doi: 10.1177/1758834010388342
16. Pardini B, Kumar R, Naccarati A, Novotny J, Prasad RB, Forsti A, et al. 5-fluorouracil-based chemotherapy for colorectal cancer and MTHFR/MTRR genotypes. Br J Clin Pharmacol (2011) 72(1):162–3. doi: 10.1111/j.1365-2125.2010.03892.x
17. Dutta N, Ghosh S, Nelson VK, Sareng HR, Majumder C, Mandal SC, et al. Andrographolide upregulates protein quality control mechanisms in cell and mouse through upregulation of mTORC1 function. Biochim Biophys Acta Gen Subj (2021) 1865(6):129885. doi: 10.1016/j.bbagen.2021.129885
18. Ghosh S, Hazra J, Pal K, Nelson VK, Pal M. Prostate cancer: therapeutic prospect with herbal medicine. Curr Res Pharmacol Drug Discovery (2021) 2:100034. doi: 10.1016/j.crphar.2021.100034
19. Pullaiah CP, Nelson VK, Rayapu S, Nk G V, Kedam T. Exploring cardioprotective potential of esculetin against isoproterenol induced myocardial toxicity in rats: in vivo and in vitro evidence. BMC Pharmacol Toxicol (2021) 22(1):43. doi: 10.1186/s40360-021-00510-0
20. Dutta N, Pemmaraju DB, Ghosh S, Ali A, Mondal A, Majumder C, et al. Alkaloid-rich fraction of ervatamia coronaria sensitizes colorectal cancer through modulating AMPK and mTOR signalling pathways. J Ethnopharmacol (2022) 283:114666. doi: 10.1016/j.jep.2021.114666
21. Nelson VK, Paul S, Roychoudhury S, Oyeyemi IT, Mandal SC, Kumar N, et al. Heat shock factors in protein quality control and spermatogenesis. Adv Exp Med Biol (2022) 1391:181–99. doi: 10.1007/978-3-031-12966-7_11
22. Nelson VK, Pullaiah CP, Saleem Ts M, Roychoudhury S, Chinnappan S, Vishnusai B, et al. Natural products as the modulators of oxidative stress: an herbal approach in the management of prostate cancer. Adv Exp Med Biol (2022) 1391:161–79. doi: 10.1007/978-3-031-12966-7_10
23. De S, Paul S, Manna A, Majumder C, Pal K, Casarcia N, et al. Phenolic phytochemicals for prevention and treatment of colorectal cancer: a critical evaluation of In vivo studies. Cancers (Basel) (2023) 15(3):1–66. doi: 10.3390/cancers15030993
24. Badavenkatappa Gari S, Nelson VK, Peraman R. Tinospora sinensis (Lour.) merr alkaloid rich extract induces colon cancer cell death via ROS mediated, mTOR dependent apoptosis pathway: “an in-vitro study”. BMC Complement Med Ther (2023) 23(1):33. doi: 10.1186/s12906-023-03849-5
25. Nelson VK, Ali A, Dutta N, Ghosh S, Jana M, Ganguli A, et al. Azadiradione ameliorates polyglutamine expansion disease in drosophila by potentiating DNA binding activity of heat shock factor 1. Oncotarget (2016) 7(48):78281–96. doi: 10.18632/oncotarget.12930
26. Liou GY, Storz P. Reactive oxygen species in cancer. Free Radic Res (2010) 44(5):479–96. doi: 10.3109/10715761003667554
27. Trachootham D, Alexandre J, Huang P. Targeting cancer cells by ROS-mediated mechanisms: a radical therapeutic approach? Nat Rev Drug Discovery (2009) 8(7):579–91. doi: 10.1038/nrd2803
28. Gorrini C, Harris IS, Mak TW. Modulation of oxidative stress as an anticancer strategy. Nat Rev Drug Discovery (2013) 12(12):931–47. doi: 10.1038/nrd4002
29. NavaneethaKrishnan S, Rosales JL, Lee KY. Targeting Cdk5 for killing of breast cancer cells via perturbation of redox homeostasis. Oncoscience (2018) 5(5-6):152–4. doi: 10.18632/oncoscience.431
30. Shimada Y, Yoshino M, Wakui A, Nakao I, Futatsuki K, Sakata Y, et al. Phase II study of CPT-11, a new camptothecin derivative, in metastatic colorectal cancer. CPT-11 gastrointestinal cancer study group. J Clin Oncol (1993) 11(5):909–13. doi: 10.1200/jco.1993.11.5.909
31. Kapała A, Szlendak M, Motacka E. The anti-cancer activity of lycopene: a systematic review of human and animal studies. Nutrients (2022) 14(23):1–44. doi: 10.3390/nu14235152
32. Farsad-Naeimi A, Alizadeh M, Esfahani A, Darvish Aminabad E. Effect of fisetin supplementation on inflammatory factors and matrix metalloproteinase enzymes in colorectal cancer patients. Food Funct (2018) 9(4):2025–31. doi: 10.1039/c7fo01898c
33. Jiang X, Jiang Z, Jiang M, Sun Y. Berberine as a potential agent for the treatment of colorectal cancer. Front Med (Lausanne) (2022) 9:886996. doi: 10.3389/fmed.2022.886996
34. Aggarwal V, Tuli HS, Varol A, Thakral F, Yerer MB, Sak K, et al. Role of reactive oxygen species in cancer progression: molecular mechanisms and recent advancements. Biomolecules (2019) 9(11):1–26. doi: 10.3390/biom9110735
35. Sahoo DK, Allenspach K, Mochel JP, Parker V, Rudinsky AJ, Winston JA, et al. Synbiotic-IgY therapy modulates the mucosal microbiome and inflammatory indices in dogs with chronic inflammatory enteropathy: a randomized, double-blind, placebo-controlled study. Vet Sci (2022) 10(1):1–18. doi: 10.3390/vetsci10010025
36. Guo YJ, Pan WW, Liu SB, Shen ZF, Xu Y, Hu LL. ERK/MAPK signalling pathway and tumorigenesis. Exp Ther Med (2020) 19(3):1997–2007. doi: 10.3892/etm.2020.8454
37. Zhang Y, Gan B, Liu D. & paik J FoxO family members in cancer. Cancer Biol Ther (2011) 12:4. doi: 10.4161/cbt.12.4.15954
38. Dai L, Zhuang L, Zhang B, Wang F, Chen X, Xia C, et al. DAG/PKCδ and IP3/Ca²⁺/CaMK IIβ operate in parallel to each other in PLCγ1-driven cell proliferation and migration of human gastric adenocarcinoma cells, through Akt/mTOR/S6 pathway. Int J Mol Sci (2015) 16(12):28510–22. doi: 10.3390/ijms161226116
39. Hwang AB, Lee SJ. Regulation of life span by mitochondrial respiration: the HIF-1 and ROS connection. Aging (2011), 304–10.
40. Guba M, Seeliger H, Kleespies A, Jauch KW, Bruns C. Vascular endothelial growth factor in colorectal cancer. Int J Colorectal Dis (2004) 19(6):510–7. doi: 10.1007/s00384-003-0576-y
41. Lee YJ, Karl DL, Maduekwe UN, Rothrock C, Ryeom S, D'Amore PA, et al. Differential effects of VEGFR-1 and VEGFR-2 inhibition on tumor metastases based on host organ environment. Cancer Res (2010) 70(21):8357–67. doi: 10.1158/0008-5472.CAN-10-1138
42. Lan T, Chen L, Wei X. Inflammatory Cytokines in cancer: comprehensive understanding and clinical progress in gene therapy. Cells (2021) 10(1):100. doi: 10.3390/cells10010100
43. NavaneethaKrishnan S, Rosales JL, Lee KY. ROS-mediated cancer cell killing through dietary phytochemicals. Oxid Med Cell Longev (2019) 2019:9051542. doi: 10.1155/2019/9051542
44. Sreevalsan S, Safe S. REACTIVE OXYGEN SPECIES AND COLORECTAL CANCER. Curr Colorectal Cancer Rep (2013) 9(4):350–7. doi: 10.1007/s11888-013-0190-5
45. Ahmed K, Zaidi SF, Cui ZG, Zhou D, Saeed SA, Inadera H. Potential proapoptotic phytochemical agents for the treatment and prevention of colorectal cancer. Oncol Lett (2019) 18(1):487–98. doi: 10.3892/ol.2019.10349
46. Hill MM, Adrain C, Duriez PJ, Creagh EM, Martin SJ. Analysis of the composition, assembly kinetics and activity of native apaf-1 apoptosomes. EMBO J (2004) 23(10):2134–45. doi: 10.1038/sj.emboj.7600210
47. Garrido C, Galluzzi L, Brunet M, Puig PE, Didelot C, Kroemer G. Mechanisms of cytochrome c release from mitochondria. Cell Death Differ (2006) 13(9):1423–33. doi: 10.1038/sj.cdd.4401950
48. Silke J, Meier P. Inhibitor of apoptosis (IAP) proteins-modulators of cell death and inflammation. Cold Spring Harb Perspect Biol (2013) 5(2). doi: 10.1101/cshperspect.a008730
49. Elmore S. Apoptosis: a review of programmed cell death. Toxicol Pathol (2007) 35(4):495–516. doi: 10.1080/01926230701320337
50. Bruckheimer EM, Cho SH, Sarkiss M, Herrmann J, McDonnell TJ. The bcl-2 gene family and apoptosis. Adv Biochem Eng Biotechnol (1998) 62:75–105. doi: 10.1007/BFb0102306
51. Hardwick JM, Soane L. Multiple functions of BCL-2 family proteins. Cold Spring Harb Perspect Biol (2013) 5(2). doi: 10.1101/cshperspect.a008722
52. Hempel N, Trebak M. Crosstalk between calcium and reactive oxygen species signaling in cancer. Cell Calcium (2017) 63:70–96. doi: 10.1016/j.ceca.2017.01.007
53. Redza-Dutordoir M, Averill-Bates DA. Activation of apoptosis signalling pathways by reactive oxygen species. Biochim Biophys Acta (2016) 1863(12):2977–92. doi: 1016/j.bbamcr.2016.09.012
54. Park DR, Thomsen AR, Frevert CW, Pham U, Skerrett SJ, Kiener PA, et al. Fas (CD95) induces proinflammatory cytokine responses by human monocytes and monocyte-derived macrophages. J Immunol (2003) 170(12):6209–16. doi: 10.4049/jimmunol.170.12.6209
55. Volpe E, Sambucci M, Battistini L, Borsellino G. Fas-fas ligand: checkpoint of T cell functions in multiple sclerosis. Front Immunol (2016) 7:382. doi: 10.3389/fimmu.2016.00382
56. Pobezinskaya YL, Liu Z. The role of TRADD in death receptor signaling. Cell Cycle (2012) 11(5):871–6. doi: 10.4161/cc.11.5.19300
57. Huang YT, Liu CH, Yang YC, Aneja R, Wen SY, Huang CY, et al. ROS- and HIF1α-dependent IGFBP3 upregulation blocks IGF1 survival signaling and thereby mediates high-glucose-induced cardiomyocyte apoptosis. J Cell Physiol (2019) 234(8):13557–70. doi: 10.1002/jcp.28034
58. Akhtar R, Chandel S, Sarotra P, Medhi B. Current status of pharmacological treatment of colorectal cancer. World J Gastrointest Oncol (2014) 6(6):177–83. doi: 10.4251/wjgo.v6.i6.177
59. Cassidy J, Misset JL. Oxaliplatin-related side effects: characteristics and management. Semin Oncol (2002) 29(5 Suppl 15):11–20. doi: 10.1053/sonc.2002.35524
60. Reynolds NA, Wagstaff AJ. Cetuximab: in the treatment of metastatic colorectal cancer. Drugs (2004) 64(1):109–18. doi: 10.2165/00003495-200464010-00007
61. Willems E, Gerne L, George C, D’Hondt M. Adverse effects of bevacizumab in metastatic colorectal cancer: a case report and literature review. Acta Gastroenterol Belg (2019) 82(2):322–5.
62. Battaglin F, Puccini A, Ahcene Djaballah S, Lenz HJ. The impact of panitumumab treatment on survival and quality of life in patients with RAS wild-type metastatic colorectal cancer. Cancer Manag Res (2019) 11:5911–24. doi: 10.2147/cmar.S186042
63. Hirsch BR, Zafar SY. Capecitabine in the management of colorectal cancer. Cancer Manag Res (2011) 3:79–89. doi: 10.2147/cmr.S11250
64. Van der Jeught K, Xu HC, Li YJ, Lu XB, Ji G. Drug resistance and new therapies in colorectal cancer. World J Gastroenterol (2018) 24(34):3834–48. doi: 10.3748/wjg.v24.i34.3834
65. Hasegawa K, Saiura A, Takayama T, Miyagawa S, Yamamoto J, Ijichi M, et al. Adjuvant oral uracil-tegafur with leucovorin for colorectal cancer liver metastases: a randomized controlled trial. PloS One (2016) 11(9):e0162400. doi: 10.1371/journal.pone.0162400
66. Hata T, Hagihara K, Tsutsui A, Akamatsu H, Ohue M, Shingai T, et al. Administration method of adjuvant tegafur-uracil and leucovorin calcium in patients with resected colorectal cancer: a phase III study. Oncologist (2021) 26(5):e735–41. doi: 10.1002/onco.13724
67. Singh V, Sheikh A, Abourehab MAS, Kesharwani P. Dostarlimab as a miracle drug: rising hope against cancer treatment. Biosensors (Basel) (2022) 12(8):1–14. doi: 10.3390/bios12080617
68. Xie YH, Chen YX, Fang JY. Comprehensive review of targeted therapy for colorectal cancer. Signal Transduct Target Ther (2020) 5(1):22. doi: 10.1038/s41392-020-0116-z
69. Rotte A. Combination of CTLA-4 and PD-1 blockers for treatment of cancer. J Exp Clin Cancer Res (2019) 38(1):255. doi: 10.1186/s13046-019-1259-z
70. Savoia P, Astrua C, Fava P. Ipilimumab (Anti-Ctla-4 mab) in the treatment of metastatic melanoma: effectiveness and toxicity management. Hum Vaccin Immunother (2016) 12(5):1092–101. doi: 10.1080/21645515.2015.1129478
71. Kooshkaki O, Derakhshani A, Hosseinkhani N, Torabi M, Safaei S, Brunetti O, et al. Combination of ipilimumab and nivolumab in cancers: from clinical practice to ongoing clinical trials. Int J Mol Sci (2020) 21(12):1–28. doi: 10.3390/ijms21124427
72. Wookey V, Grothey A. Update on the role of pembrolizumab in patients with unresectable or metastatic colorectal cancer. Therap Adv Gastroenterol (2021) 14:17562848211024460. doi: 10.1177/17562848211024460
73. Berg D. Managing the side effects of chemotherapy for colorectal cancer. Semin Oncol (1998) 25(5 Suppl 11):53–9.
74. Golshani G, Zhang Y. Advances in immunotherapy for colorectal cancer: a review. Therap Adv Gastroenterol (2020) 13:1756284820917527. doi: 10.1177/1756284820917527
75. Sung H, Ferlay J, Siegel RL, Laversanne M, Soerjomataram I, Jemal A, et al. Global cancer statistics 2020: GLOBOCAN estimates of incidence and mortality worldwide for 36 cancers in 185 countries. CA Cancer J Clin (2021) 71(3):209–49. doi: 10.3322/caac.21660
76. Hashem S, Ali TA, Akhtar S, Nisar S, Sageena G, Ali S, et al. Targeting cancer signaling pathways by natural products: exploring promising anti-cancer agents. BioMed Pharmacother (2022) 150:113054. doi: 10.1016/j.biopha.2022.113054
77. Choudhari AS, Mandave PC, Deshpande M, Ranjekar P, Prakash O. Phytochemicals in cancer treatment: from preclinical studies to clinical practice. Front Pharmacol (2019) 10:1614. doi: 10.3389/fphar.2019.01614
78. Rahman MA, Hannan MA, Dash R, Rahman MH, Islam R, Uddin MJ, et al. Phytochemicals as a complement to cancer chemotherapy: pharmacological modulation of the autophagy-apoptosis pathway. Front Pharmacol (2021) 12:639628. doi: 10.3389/fphar.2021.639628
79. Greenwell M, Rahman PK. Medicinal plants: their use in anticancer treatment. Int J Pharm Sci Res (2015) 6(10):4103–12. doi: 10.13040/ijpsr.0975-8232.6(10).4103-12
80. Seca AML, Pinto DCGA. Biological potential and medical use of secondary metabolites. Medicines (Basel) (2019) 6(2):1–6. doi: 10.3390/medicines6020066
81. Dhyani P, Quispe C, Sharma E, Bahukhandi A, Sati P, Attri DC, et al. Anticancer potential of alkaloids: a key emphasis to colchicine, vinblastine, vincristine, vindesine, vinorelbine and vincamine. Cancer Cell Int (2022) 22(1):206. doi: 10.1186/s12935-022-02624-9
82. Huang M, Lu JJ, Ding J. Natural products in cancer therapy: past, present and future. Nat Prod Bioprospect (2021) 11(1):5–13. doi: 10.1007/s13659-020-00293-7
83. Xu Y, Fang F, Miriyala S, Crooks PA, Oberley TD, Chaiswing L, et al. KEAP1 is a redox sensitive target that arbitrates the opposing radiosensitive effects of parthenolide in normal and cancer cells. Cancer Res (2013) 73(14):4406–17. doi: 10.1158/0008-5472.Can-12-4297
84. Khan H, Alam W, Alsharif KF, Aschner M, Pervez S, Saso L. Alkaloids and colon cancer: molecular mechanisms and therapeutic implications for cell cycle arrest. Molecules (2022) 27(3):1–26. doi: 10.3390/molecules27030920
85. Heinrich M, Mah J, Amirkia V. Alkaloids used as medicines: structural phytochemistry meets biodiversity-an update and forward look. Molecules (2021) 26(7):1–26. doi: 10.3390/molecules26071836
86. Gong X, Chen Z, Han Q, Chen C, Jing L, Liu Y, et al. Sanguinarine triggers intrinsic apoptosis to suppress colorectal cancer growth through disassociation between STRAP and MELK. BMC Cancer (2018) 18(1):578. doi: 10.1186/s12885-018-4463-x
87. Matkar SS, Wrischnik LA, Hellmann-Blumberg U. Sanguinarine causes DNA damage and p53-independent cell death in human colon cancer cell lines. Chem Biol Interact (2008) 172(1):63–71. doi: 10.1016/j.cbi.2007.12.006
88. Yin HQ, Kim YH, Moon CK, Lee BH. Reactive oxygen species-mediated induction of apoptosis by a plant alkaloid 6-methoxydihydrosanguinarine in HepG2 cells. Biochem Pharmacol (2005) 70(2):242–8. doi: 10.1016/j.bcp.2005.04.020
89. Dai W, Mu L, Cui Y, Li Y, Chen P, Xie H, et al. Berberine promotes apoptosis of colorectal cancer via regulation of the long non-coding RNA (lncRNA) cancer susceptibility candidate 2 (CASC2)/AU-binding factor 1 (AUF1)/B-cell CLL/Lymphoma 2 (Bcl-2) axis. Med Sci Monit (2019) 25:730–8. doi: 10.12659/msm.912082
90. Wang L, Liu L, Shi Y, Cao H, Chaturvedi R, Calcutt MW, et al. Berberine induces caspase-independent cell death in colon tumor cells through activation of apoptosis-inducing factor. PloS One (2012) 7(5):e36418. doi: 10.1371/journal.pone.0036418
91. Park JJ, Seo SM, Kim EJ, Lee YJ, Ko YG, Ha J, et al. Berberine inhibits human colon cancer cell migration via AMP-activated protein kinase-mediated downregulation of integrin β1 signaling. Biochem Biophys Res Commun (2012) 426(4):461–7. doi: 10.1016/j.bbrc.2012.08.091
92. Hsu WH, Hsieh YS, Kuo HC, Teng CY, Huang HI, Wang CJ, et al. Berberine induces apoptosis in SW620 human colonic carcinoma cells through generation of reactive oxygen species and activation of JNK/p38 MAPK and FasL. Arch Toxicol (2007) 81(10):719–28. doi: 10.1007/s00204-006-0169-y
93. Li W, Hua B, Saud SM, Lin H, Hou W, Matter MS, et al. Berberine regulates AMP-activated protein kinase signaling pathways and inhibits colon tumorigenesis in mice. Mol Carcinog (2015) 54(10):1096–109. doi: 10.1002/mc.22179
94. Liu X, Ji Q, Ye N, Sui H, Zhou L, Zhu H, et al. Berberine inhibits invasion and metastasis of colorectal cancer cells via COX-2/PGE2 mediated JAK2/STAT3 signaling pathway. PloS One (2015) 10(5):e0123478. doi: 10.1371/journal.pone.0123478
95. Wu K, Yang Q, Mu Y, Zhou L, Liu Y, Zhou Q, et al. Berberine inhibits the proliferation of colon cancer cells by inactivating wnt/β-catenin signaling. Int J Oncol (2012) 41(1):292–8. doi: 10.3892/ijo.2012.1423
96. Li D, Zhang Y, Liu K, Zhao Y, Xu B, Xu L, et al. Berberine inhibits colitis-associated tumorigenesis via suppressing inflammatory responses and the consequent EGFR signaling-involved tumor cell growth. Lab Invest (2017) 97(11):1343–53. doi: 10.1038/labinvest.2017.71
97. Samadi P, Sarvarian P, Gholipour E, Asenjan KS, Aghebati-Maleki L, Motavalli R, et al. Berberine: a novel therapeutic strategy for cancer. IUBMB Life (2020) 72(10):2065–79. doi: 10.1002/iub.2350
98. Zhao Y, Roy S, Wang C, Goel A. A combined treatment with berberine and andrographis exhibits enhanced anti-cancer activity through suppression of DNA replication in colorectal cancer. Pharm (Basel) (2022) 15(3):1–17. doi: 10.3390/ph15030262
99. Guan X, Zheng X, Vong CT, Zhao J, Xiao J, Wang Y, et al. Combined effects of berberine and evodiamine on colorectal cancer cells and cardiomyocytes in vitro. Eur J Pharmacol (2020) 875:173031. doi: 10.1016/j.ejphar.2020.173031
100. Rattanawong A, Payon V, Limpanasittikul W, Boonkrai C, Mutirangura A, Wonganan P. Cepharanthine exhibits a potent anticancer activity in p53-mutated colorectal cancer cells through upregulation of p21Waf1/Cip1. Oncol Rep (2018) 39(1):227–38. doi: 10.3892/or.2017.6084
101. Han B, Jiang P, Li Z, Yu Y, Huang T, Ye X, et al. Coptisine-induced apoptosis in human colon cancer cells (HCT-116) is mediated by PI3K/Akt and mitochondrial-associated apoptotic pathway. Phytomedicine (2018) 48:152–60. doi: 10.1016/j.phymed.2017.12.027
102. Huang T, Xiao Y, Yi L, Li L, Wang M, Tian C, et al. Coptisine from rhizoma coptidis suppresses HCT-116 cells-related tumor growth in vitro and in vivo. Sci Rep (2017) 7:38524. doi: 10.1038/srep38524
103. Cao Q, Hong S, Li Y, Chen H, Shen Y, Shao K, et al. Coptisine suppresses tumor growth and progression by down-regulating MFG-E8 in colorectal cancer. RSC Adv (2018) 8(54):30937–45. doi: 10.1039/c8ra05806g
104. Manogaran P, Beeraka NM, Huang CY, Vijaya Padma V. Neferine and isoliensinine enhance ‘intracellular uptake of cisplatin’ and induce ‘ROS-mediated apoptosis’ in colorectal cancer cells - a comparative study. Food Chem Toxicol (2019) 132:110652. doi: 10.1016/j.fct.2019.110652
105. Manogaran P, Somasundaram B, Viswanadha VP. Reversal of cisplatin resistance by neferine/isoliensinine and their combinatorial regimens with cisplatin-induced apoptosis in cisplatin-resistant colon cancer stem cells (CSCs). J Biochem Mol Toxicol (2022) 36(3):e22967. doi: 10.1002/jbt.22967
106. Liu X, Zhang Y, Wu S, Xu M, Shen Y, Yu M, et al. Palmatine induces G2/M phase arrest and mitochondrial-associated pathway apoptosis in colon cancer cells by targeting AURKA. Biochem Pharmacol (2020) 175:113933. doi: 10.1016/j.bcp.2020.113933
107. Meng LH, Zhang H, Hayward L, Takemura H, Shao RG, Pommier Y. Tetrandrine induces early G1 arrest in human colon carcinoma cells by down-regulating the activity and inducing the degradation of G1-s-specific cyclin-dependent kinases and by inducing p53 and p21Cip1. Cancer Res (2004) 64(24):9086–92. doi: 10.1158/0008-5472.Can-04-0313
108. Qin R, Shen H, Cao Y, Fang Y, Li H, Chen Q, et al. Tetrandrine induces mitochondria-mediated apoptosis in human gastric cancer BGC-823 cells. PloS One (2013) 8(10):e76486. doi: 10.1371/journal.pone.0076486
109. Li X, Zhen D, Lu X, Xu H, Shao Y, Xue Q, et al. Enhanced cytotoxicity and activation of ROS-dependent c-jun NH2-terminal kinase and caspase-3 by low doses of tetrandrine-loaded nanoparticles in lovo cells–a possible Trojan strategy against cancer. Eur J Pharm Biopharm (2010) 75(3):334–40. doi: 10.1016/j.ejpb.2010.04.016
110. Santos LS, Silva VR, Menezes LRA, Soares MBP, Costa EV, Bezerra DP. Xylopine induces oxidative stress and causes G(2)/M phase arrest, triggering caspase-mediated apoptosis by p53-independent pathway in HCT116 cells. Oxid Med Cell Longev (2017) 2017:7126872. doi: 10.1155/2017/7126872
111. Lorence A, Medina-Bolivar F, Nessler CL. Camptothecin and 10-hydroxycamptothecin from camptotheca acuminata hairy roots. Plant Cell Rep (2004) 22(6):437–41. doi: 10.1007/s00299-003-0708-4
112. Ha SW, Kim YJ, Kim W, Lee CS. Antitumor effects of camptothecin combined with conventional anticancer drugs on the cervical and uterine squamous cell carcinoma cell line SiHa. Korean J Physiol Pharmacol (2009) 13(2):115–21. doi: 10.4196/kjpp.2009.13.2.115
113. Park K, Abebe W, Fermin C, Reddy G, Habtemariam T, Chung J, et al. Hypoxia inhibition of camptothecin-induced apoptosis by bax loss. Biologia (2012) 67:616–21. doi: 10.2478/s11756-012-0037-6
114. Liskova V, Kajsik M, Chovancova B, Roller L, Krizanova O. Camptothecin, triptolide, and apoptosis inducer kit have differential effects on mitochondria in colorectal carcinoma cells. FEBS Open Bio (2022) 12(5):913–24. doi: 10.1002/2211-5463.13401
115. Wenzel U, Nickel A, Kuntz S, Daniel H. Ascorbic acid suppresses drug-induced apoptosis in human colon cancer cells by scavenging mitochondrial superoxide anions. Carcinogenesis (2004) 25(5):703–12. doi: 10.1093/carcin/bgh079
116. Guo Z, Wang Z, Liang R, Tian H, Chen X, Chen M. Reactive oxygen species activated by mitochondria-specific camptothecin prodrug for enhanced chemotherapy. Bosn J Basic Med Sci (2022) 22(6):934–48. doi: 10.17305/bjbms.2022.7194
117. Fujita K, Kubota Y, Ishida H, Sasaki Y. Irinotecan, a key chemotherapeutic drug for metastatic colorectal cancer. World J Gastroenterol (2015) 21(43):12234–48. doi: 10.3748/wjg.v21.i43.12234
118. Britten CD, Hilsenbeck SG, Eckhardt SG, Marty J, Mangold G, MacDonald JR, et al. Enhanced antitumor activity of 6-hydroxymethylacylfulvene in combination with irinotecan and 5-fluorouracil in the HT29 human colon tumor xenograft model. Cancer Res (1999) 59(5):1049–53.
119. Raymond E, Louvet C, Tournigand C, Coudray AM, Faivre S, De Gramont A, et al. Pemetrexed disodium combined with oxaliplatin, SN38, or 5-fluorouracil, based on the quantitation of drug interactions in human HT29 colon cancer cells. Int J Oncol (2002) 21(2):361–7. doi: 10.3892/ijo.21.2.361
120. Allegrini G, Goulette FA, Darnowski JW, Calabresi P. Thrombospondin-1 plus irinotecan: a novel antiangiogenic-chemotherapeutic combination that inhibits the growth of advanced human colon tumor xenografts in mice. Cancer Chemother Pharmacol (2004) 53(3):261–6. doi: 10.1007/s00280-003-0712-y
121. Di Bartolomeo M, Ciarlo A, Bertolini A, Barni S, Verusio C, Aitini E, et al. Capecitabine, oxaliplatin and irinotecan in combination, with bevacizumab (COI-b regimen) as first-line treatment of patients with advanced colorectal cancer. an Italian trials of medical oncology phase II study. Eur J Cancer (2015) 51(4):473–81. doi: 10.1016/j.ejca.2014.12.020
122. Cai Y, Deng R, Hu H, Zhang J, Ling J, Wu Z, et al. [Analysis on safety and preliminary efficacy of dose-modified regimen of 5-fluorouracil plus oxaliplatin and irinotecan (FOLFOXIRI) in advanced colorectal cancer]. Zhonghua Wei Chang Wai Ke Za Zhi (2018) 21(9):1045–50.
123. Reyes-Escogido Mde L, Gonzalez-Mondragon EG, Vazquez-Tzompantzi E. Chemical and pharmacological aspects of capsaicin. Molecules (2011) 16(2):1253–70. doi: 10.3390/molecules16021253
124. Yang KM, Pyo JO, Kim GY, Yu R, Han IS, Ju SA, et al. Capsaicin induces apoptosis by generating reactive oxygen species and disrupting mitochondrial transmembrane potential in human colon cancer cell lines. Cell Mol Biol Lett (2009) 14(3):497–510. doi: 10.2478/s11658-009-0016-2
125. Kim CS, Park WH, Park JY, Kang JH, Kim MO, Kawada T, et al. Capsaicin, a spicy component of hot pepper, induces apoptosis by activation of the peroxisome proliferator-activated receptor gamma in HT-29 human colon cancer cells. J Med Food (2004) 7(3):267–73. doi: 10.1089/jmf.2004.7.267
126. Kim YM, Hwang JT, Kwak DW, Lee YK, Park OJ. Involvement of AMPK signaling cascade in capsaicin-induced apoptosis of HT-29 colon cancer cells. Ann N Y Acad Sci (2007) 1095:496–503. doi: 10.1196/annals.1397.053
127. Lu HF, Chen YL, Yang JS, Yang YY, Liu JY, Hsu SC, et al. Antitumor activity of capsaicin on human colon cancer cells in vitro and colo 205 tumor xenografts in vivo. J Agric Food Chem (2010) 58(24):12999–3005. doi: 10.1021/jf103335w
128. Clark R, Lee J, Lee SH. Synergistic anticancer activity of capsaicin and 3,3’-diindolylmethane in human colorectal cancer. J Agric Food Chem (2015) 63(17):4297–304. doi: 10.1021/jf506098s
129. Kim MY, Trudel LJ, Wogan GN. Apoptosis induced by capsaicin and resveratrol in colon carcinoma cells requires nitric oxide production and caspase activation. Anticancer Res (2009) 29(10):3733–40.
130. Waziri PM, Abdullah R, Yeap SK, Omar AR, Kassim NK, Malami I, et al. Clausenidin induces caspase-dependent apoptosis in colon cancer. BMC Complement Altern Med (2016) 16:256. doi: 10.1186/s12906-016-1247-1
131. Song N, Ma J, Hu W, Guo Y, Hui L, Aamer M, et al. Lappaconitine hydrochloride inhibits proliferation and induces apoptosis in human colon cancer HCT-116 cells via mitochondrial and MAPK pathway. Acta Histochem (2021) 123(5):151736. doi: 10.1016/j.acthis.2021.151736
132. Yaffe PB, Power Coombs MR, Doucette CD, Walsh M, Hoskin DW. Piperine, an alkaloid from black pepper, inhibits growth of human colon cancer cells via G1 arrest and apoptosis triggered by endoplasmic reticulum stress. Mol Carcinog (2015) 54(10):1070–85. doi: 10.1002/mc.22176
133. DA Silva Machado F, Munari FM, Scariot FJ, Echeverrigaray S, Aguzzoli C, Pich CT, et al. Piperlongumine induces apoptosis in colorectal cancer HCT 116 cells independent of bax, p21 and p53 status. Anticancer Res (2018) 38(11):6231–6. doi: 10.21873/anticanres.12978
134. Chen W, Lian W, Yuan Y, Li M. The synergistic effects of oxaliplatin and piperlongumine on colorectal cancer are mediated by oxidative stress. Cell Death Dis (2019) 10(8):600. doi: 10.1038/s41419-019-1824-6
135. Kim GD. Harmine hydrochloride triggers G2/M cell cycle arrest and apoptosis in HCT116 cells through ERK and PI3K/AKT/mTOR signaling pathways. Prev Nutr Food Sci (2021) 26(4):445–52. doi: 10.3746/pnf.2021.26.4.445
136. Liu J, Li Q, Liu Z, Lin L, Zhang X, Cao M, et al. Harmine induces cell cycle arrest and mitochondrial pathway-mediated cellular apoptosis in SW620 cells via inhibition of the akt and ERK signaling pathways. Oncol Rep (2016) 35(6):3363–70. doi: 10.3892/or.2016.4695
137. Liang L, Wu J, Luo J, Wang L, Chen ZX, Han CL, et al. Oxymatrine reverses 5-fluorouracil resistance by inhibition of colon cancer cell epithelial-mesenchymal transition and NF-κB signaling in vitro. Oncol Lett (2020) 19(1):519–26. doi: 10.3892/ol.2019.11090
138. Pan D, Zhang W, Zhang N, Xu Y, Chen Y, Peng J, et al. Oxymatrine synergistically enhances doxorubicin anticancer effects in colorectal cancer. Front Pharmacol (2021) 12:673432. doi: 10.3389/fphar.2021.673432
139. Kumar A, Singh B, Sharma PR, Bharate SB, Saxena AK, Mondhe DM. A novel microtubule depolymerizing colchicine analogue triggers apoptosis and autophagy in HCT-116 colon cancer cells. Cell Biochem Funct (2016) 34(2):69–81. doi: 10.1002/cbf.3166
140. Huang Z, Xu Y, Peng W. Colchicine induces apoptosis in HT−29 human colon cancer cells via the AKT and c-jun n-terminal kinase signaling pathways. Mol Med Rep (2015) 12(4):5939–44. doi: 10.3892/mmr.2015.4222
141. Seufferlein T, Ettrich TJ, Menzler S, Messmann H, Kleber G, Zipprich A, et al. Green tea extract to prevent colorectal adenomas, results of a randomized, placebo-controlled clinical trial. Am J Gastroenterol (2022) 117(6):884–94. doi: 10.14309/ajg.0000000000001706
142. Singh AP, Singh R, Verma SS, Rai V, Kaschula CH, Maiti P, et al. Health benefits of resveratrol: evidence from clinical studies. Med Res Rev (2019) 39(5):1851–91. doi: 10.1002/med.21565
143. Wu XY, Zhai J, Huan XK, Xu WW, Tian J, Farhood B. A systematic review of the therapeutic potential of resveratrol during colorectal cancer chemotherapy. Mini Rev Med Chem (2022) 23(10):1137–52. doi: 10.2174/1389557522666220907145153
144. Sharma RA, Euden SA, Platton SL, Cooke DN, Shafayat A, Hewitt HR, et al. Phase I clinical trial of oral curcumin: biomarkers of systemic activity and compliance. Clin Cancer Res (2004) 10(20):6847–54. doi: 10.1158/1078-0432.Ccr-04-0744
Glossary
Keywords: oxidative stress, mutation, IGF-1, colon cancer progression, alkaloids, HIF-1α, IGFBP-3, apoptosis
Citation: Nelson VK, Nuli MV, Mastanaiah J, Saleem T. S. M, Birudala G, Jamous YF, Alshargi O, Kotha KK, Sudhan HH, Mani RR, Muthumanickam A, Niranjan D, Jain NK, Agrawal A, Jadon AS, Mayasa V, Jha NK, Kolesarova A, Slama P and Roychoudhury S (2023) Reactive oxygen species mediated apoptotic death of colon cancer cells: therapeutic potential of plant derived alkaloids. Front. Endocrinol. 14:1201198. doi: 10.3389/fendo.2023.1201198
Received: 06 April 2023; Accepted: 28 June 2023;
Published: 25 July 2023.
Edited by:
Dipak Kumar Sahoo, Iowa State University, United StatesReviewed by:
Ahmad Perwez, Case Western Reserve University, United StatesSuvranil Ghosh, University of Texas Southwestern Medical Center, United States
Copyright © 2023 Nelson, Nuli, Mastanaiah, Saleem T. S., Birudala, Jamous, Alshargi, Kotha, Sudhan, Mani, Muthumanickam, Niranjan, Jain, Agrawal, Jadon, Mayasa, Jha, Kolesarova, Slama and Roychoudhury. This is an open-access article distributed under the terms of the Creative Commons Attribution License (CC BY). The use, distribution or reproduction in other forums is permitted, provided the original author(s) and the copyright owner(s) are credited and that the original publication in this journal is cited, in accordance with accepted academic practice. No use, distribution or reproduction is permitted which does not comply with these terms.
*Correspondence: Vinod K. Nelson, dmlub2Qua3VtYXI0NTdAZ21haWwuY29t; Niraj Kumar Jha, bmlyYWouamhhQHNoYXJkYS5hYy5pbg==; Petr Slama, cGV0ci5zbGFtYUBtZW5kZWx1LmN6; Shubhadeep Roychoudhury, c2h1YmhhZGVlcDFAZ21haWwuY29t
†These authors have contributed equally to this work and share first authorship
‡These authors have contributed equally to this work and share second authorship