- Department of Endocrinology and Metabolism, The First Affiliated Hospital of Zhengzhou University, Zhengzhou, China
Diabetic kidney disease (DKD), one of the most severe complications of diabetes mellitus (DM), has received considerable attention owing to its increasing prevalence and contribution to chronic kidney disease (CKD) and end-stage kidney disease (ESRD). However, the use of drugs targeting DKD remains limited. Recent data suggest that long non-coding RNAs (lncRNAs) play a vital role in the development of DKD. The lncRNA H19 is the first imprinted gene, which is expressed in the embryo and down-regulated at birth, and its role in tumors has long been a subject of controversy, however, in recent years, it has received increasing attention in kidney disease. The LncRNA H19 is engaged in the pathological progression of DKD, including glomerulosclerosis and tubulointerstitial fibrosis via the induction of inflammatory responses, apoptosis, ferroptosis, pyroptosis, autophagy, and oxidative damage. In this review, we highlight the most recent research on the molecular mechanism and regulatory forms of lncRNA H19 in DKD, including epigenetic, post-transcriptional, and post-translational regulation, providing a new predictive marker and therapeutic target for the management of DKD.
1 Introduction
Diabetes mellitus (DM) is a heterogeneous group of disorders of glucose metabolism dysfunction (1), the global prevalence of which has risen dramatically in recent years, a figure projected to reach 783 million by 2045 (2). Excessive exposure to fluctuating glucose concentrations is increasingly recognized as an important pathological factor contributing to diabetic microvascular complications, which eventually lead to diabetic kidney disease, retinopathy, and neuropathy (3, 4). Among these, diabetic kidney disease (DKD) is of great concerns due to its high prevalence and harmful effects on the kidney. It is estimated that more than 40% of individuals with diabetes worldwide are affected by DKD, which is a leading cause of chronic kidney disease (CKD) and end-stage kidney disease (ESRD) (5). Moreover, DKD patients with ESRD have an alarmingly high mortality rate (6), and the therapy typically required for survival is renal replacement therapy (RRT), such as kidney dialysis or transplantation, both of which impose a huge financial burden on individuals and society (7). Hence, a deeper understanding of the molecular mechanisms underlying DKD is urgently required to assist in the discovery of effective therapeutics that can halt its progression and lower the associated risks.
Hyperglycemia is widely considered the primary etiological factor in the development of DKD, as it encourages metabolic and hemodynamic changes in the kidney, causing alterations in renal component structure and function such as glomerulosclerosis, tubulointerstitial inflammation, and fibrosis, which contribute to a decrease in glomerular filtration rate (GFR), ever-increasing albuminuria, and ultimately renal failure (8–10). Additionally, accumulating data indicate that the pathophysiology of DKD may be influenced by oxidative stress (OS), hypoxia, and overactivity of the renin-angiotensin-aldosterone system (RAAS) (11). Moreover, the available data suggest that long non-coding RNAs (lncRNAs) may be involved in the development of DKD, offering a novel avenue for the DKD therapy (12).
Long non-coding RNAs (LncRNAs), which are linear transcripts longer than 200 nucleotides that lack protein-translation potential, are of low abundance and stability making up the majority of non-coding RNAs (ncRNAs) in the genome (13, 14). Typically, lncRNAs are classified into multiple subtypes based on their positions, including sense, antisense, intronic, bidirectional, and intergenic (15). They exert biological functions by modulating various physiological processes, including cell differentiation, proliferation, and responses to various stresses (16). Recently, an expanding collection of studies have demonstrated differential expression of lncRNAs at the cellular and tissue levels in the kidney, ultimately leading to damage to cell masses that accelerate renal fibrosis and glomerulosclerosis (17).
Among them, lncRNA H19, the first imprinted and maternally expressed gene to be discovered in eukaryotes (18), is located close to the telomeric region of chromosome 11p15.5 and forms an imprinted domain with the gene insulin-like growth factor 2(IGF2), which is expressed from the paternal allele (19). Notably, during the development of kidney embryos, lncRNA H19 is extensively expressed in the ureteric bud branches and epithelial components of the metanephros, whereas its expression dramatically declines and fades in the postnatal period (20). Occasionally, lncRNA H19 is re-expressed during the activation of tumors, tissue repair, and intracellular stress, exhibiting its significance in aging, cancer, and liver diseases (21–23). Similarly, researchers found that H19 is upregulated in patients with DKD compared to that in the control group, and further studies have suggested the molecular mechanism of lncRNA H19 in regulating different processes of DKD (24). Therefore, in this review, we outline the latest developments in lncRNA H19 for modulating the pathophysiological processes of DKD and propose prospective future treatment strategies for DKD (Figure 1).
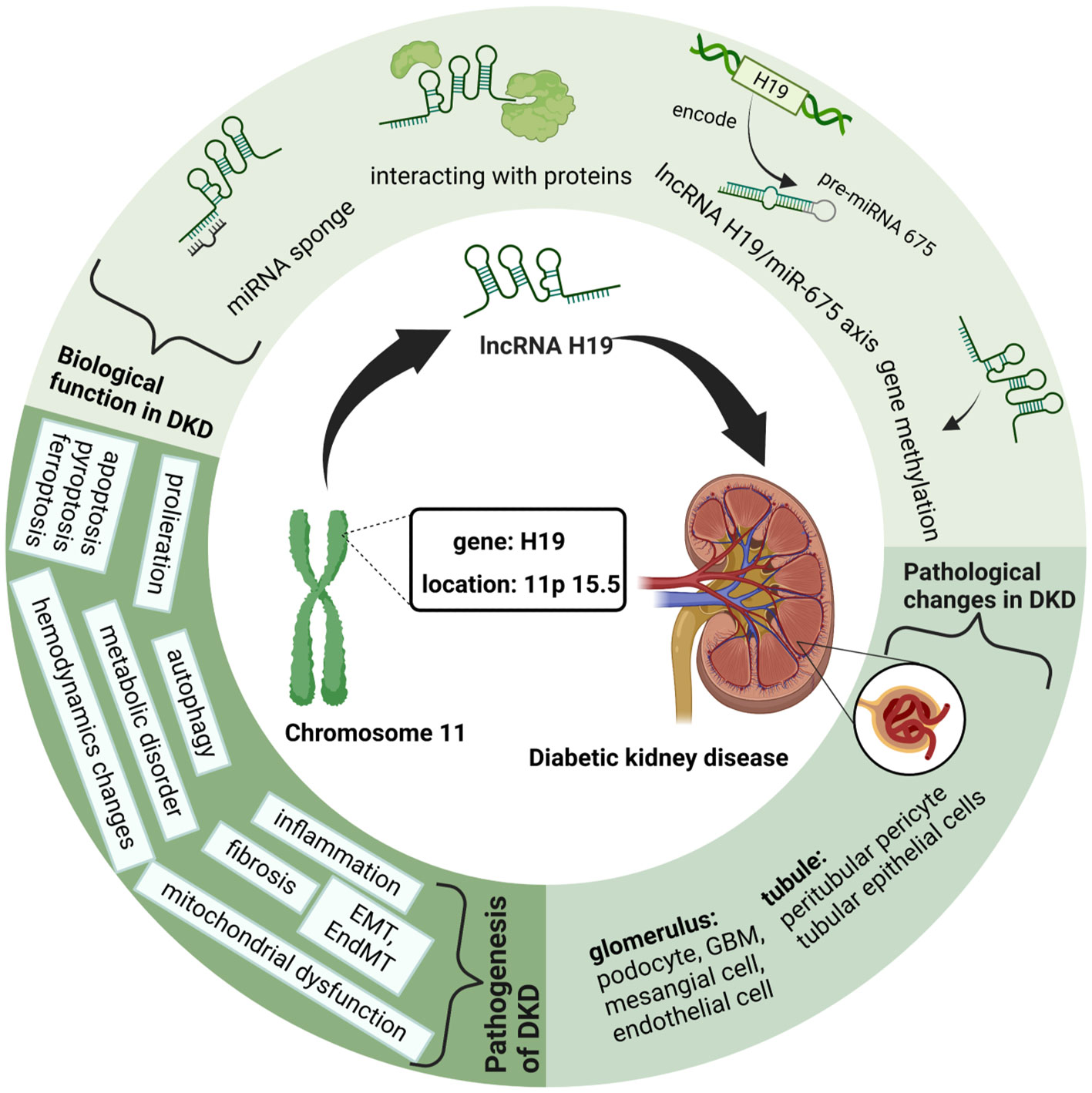
Figure 1 Graphical Abstract: LncRNA H19 in Diabetic kidney diseases. This figure is created with BioRender.com, and the authors have been granted a license to use the BioRender content.
2 ‘Sophisticated’ structure changes in DKD
2.1 Key changes in the diabetic glomerulus
Hyperglycemia affects nearly all renal components, including the glomeruli, tubules, and interstitium. However, the glomerulus is traditionally classified as the first step in DKD. The Renal Pathology Society established four categories of glomerular lesions in 2010: Class I, glomerular basement membrane (GBM) thickening; Class II, mesangial expansion; Class III, nodular sclerosis (Kimmelstiel-Wilson lesions); and Class IV, advanced diabetic glomerulosclerosis (25).
GBM thicking, glomerular hypertrophy, and glomerular filtration barrier (GFB) deficiencies are early signs of glomerular alterations (26). Among these, the initial and most typical change in glomeruli is GBM thickening, which is also a hallmark of podocytes and glomerular endothelial cells (GECs) dysfunction (27). GBM is composed of laminins (LM), nidogens, collagen IV (Col. IV), and heparan sulfate proteoglycans, all of which are required for glomerular capillary wall formation and kidney filtration (28). Along with GBM, GECs and podocytes are the other two main constituents of GFB; when they are in a dysfunctional state, they contribute to albuminuria and a decrease in GFR (29, 30).
The dominant alteration in the glomerulus is glomerular podocyte dysfunction or podocytopathy, which is marked by podocyte loss, cellular hypertrophy, and foot process effacement (31, 32). Reactive oxygen species (ROS) produced during hyperglycemia trigger podocyte detachment and apoptosis, ultimately resulting in podocyte loss (33). Due to the limited potential for podocyte regeneration, high glucose levels induce an adaptive response in the form of podocyte hypertrophy, which aims to maintain glomerular integrity and reduce glomerulosclerosis (34–36). As a result, both podocyte hypertrophy and deletion are responsible for the podocyte foot process effacement (37).
Additional glomerular lesions include deletion of endothelial fenestrations and expansion of the mesangial matrix. The negatively charged glycocalyx decorated on fenestrated endothelial cells is essential for maintaining fluidic equilibrium and controlling vascular permeability, as well as preventing blood cells from attaching to the vascular wall (38, 39). Hence, elimination of specific glycocalyx components such as keratan sulfate, chondroitin sulfate, dermatan sulfate, and hyaluronic acid, enhances the endothelial permeability and triggers microalbuminuria (40, 41). Similarly, the continual reduction in the surface area of the fenestrated endothelium is an outcome of the death and pyroptosis of podocytes brought on by high glucose levels (42, 43).
Furthermore, hyperglycemia accelerates matrix expansion and alterations in glomerular mesangial cells (GMCs), which are the central stalk of the glomerulus making up 30-40% of all glomerular cells and role as a functional unit with endothelial cells and podocytes (44, 45). The mechanism of GMCs alterations in DKD is convoluted. It is commonly acknowledged that elevated blood glucose promotes the formation of the extracellular matrix (ECM), which is connected to altered GBM remnants, and stimulates pro-apoptosis or pro-proliferation signaling, which boosts apoptosis, proliferation, and hypertrophy in mesangial cells, all of which ultimately leads to proteinuria and glomerular hyperfiltration (46–50). Notably, mesangial cells increase the levels of Col. IV, plasminogen activator inhibitor 1 (PAI-1), and fibronectin (FN) to induce glomerular fibrosis (51). Additionally, endothelial-to-mesenchymal transition (EndMT) and epithelial-to-mesenchymal transition (EMT) are involved in renal fibrosis (52, 53).
2.2 Renal tubular dysfunction and fibrogenesis
In contrast to careful studies on glomerular degeneration over the past few years, renal tubule interstitial impairment in DKD has largely been disregarded. Currently, the particular role of the renal tubule is of great interest in scientific research. Situated in the outer layer of the renal tubule, renal tubular epithelial cells (TECs) reabsorb chemicals such as amino acids and glucose from the urine, which is crucial for preserving the glomerulotubular balance. Additionally, TECs also influence the glomerular filtration rate by regulating concentrations of ions like Na+ and Cl-; this procedure is known as tubule-glomerular feedback (54, 55). In the early stages of DKD, renal tubular cells exhibit adaptive hypertrophy and elongation to maintain the glomerulotubular balance in response to glomerular hyperfiltration (56, 57). Subsequent histological alterations in the tubulointerstitium include tubular atrophy, peritubular capillary rarefaction, and inflammation, all of which contribute to the development of renal fibrosis (9, 58). Cell death functions as the main mechanism of renal tubular atrophy which is manifested by caspase-1-dependent pyroptosis, transforming growth factor-β(TGF-β1)-dependent ferroptosis, and caspase-3-dependent apoptosis (59–61). Moreover, increased diabetes-related inflammation encourages EMT in tubular epithelial cells and peritubular pericyte migration, both of which induce tubulointerstitial fibrosis (62, 63). Microvascular rarefaction refers to the peritubular pericyte moving out of the capillary and into the interstitial space (64), whereas EMT is marked by the acquisition of the mesenchymal markers smooth muscle actin (α-SMA) and the loss of the intracellular adhesion protein E-cadherin (65). As mentioned above, the hallmark pathological renal changes in DKD, such as GBM thickening, podocytopathy, loss of glomerular endothelium fenestrations, mesangial matrix expansion, and tubulointerstitial fibrosis, have all been well documented (Figure 2).
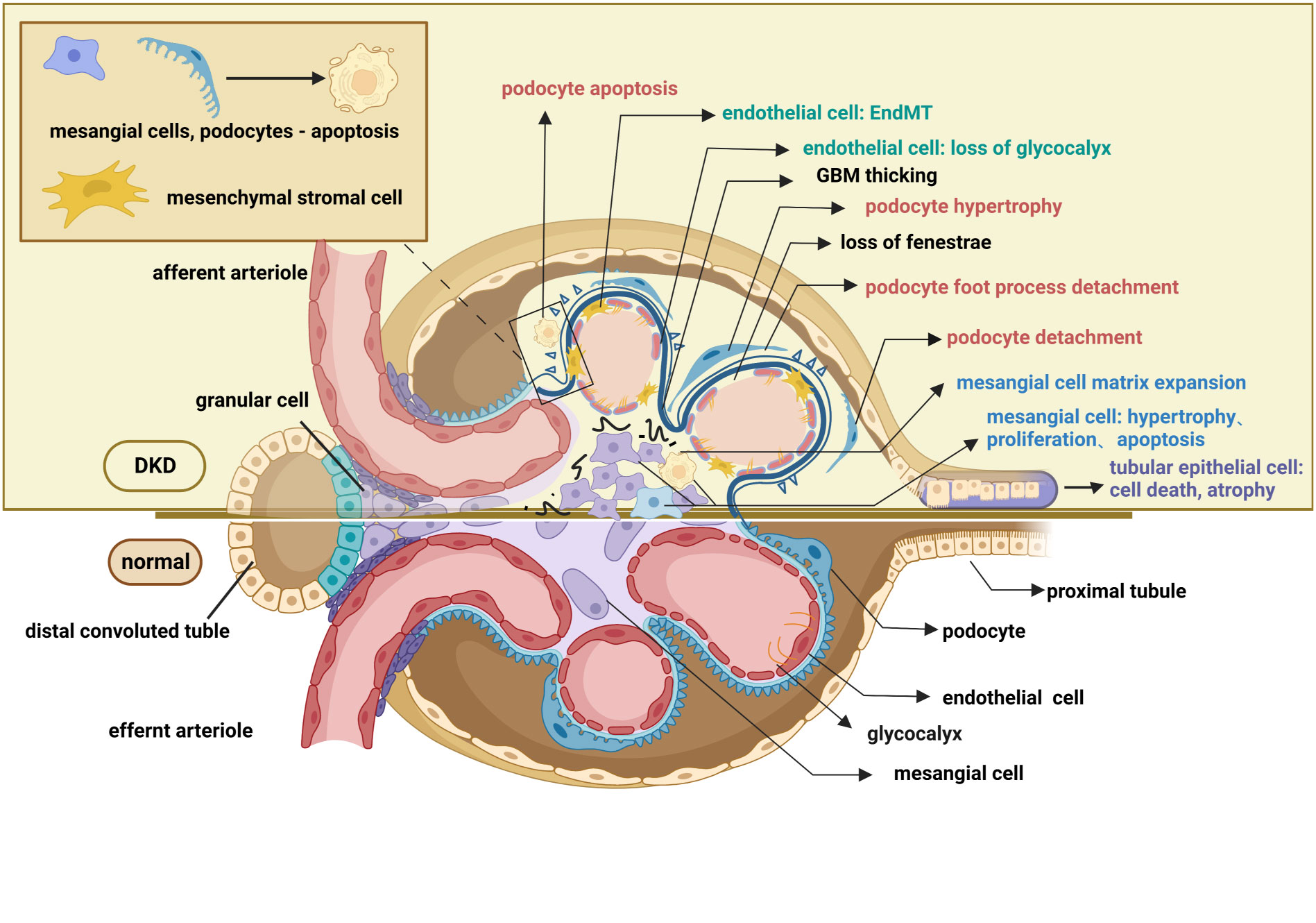
Figure 2 Pathological changes in the glomerulus and renal tubule of DKD. The pathological changes of the glomerulus mainly involve glomerular endothelial cells, mesangial cells, glomerular basement membrane, and podocytes. Endothelial cell changes include loss of glycocalyx, endothelial-mesenchymal transformation, apoptosis, and pyroptosis. Changes in podocyte include podocyte apoptosis, podocyte hypertrophy, podocyte detachment, podocyte loss, and podocyte foot process effacement. Mesangial cell changes include proliferation, hypertrophy, and apoptosis. Pathological changes in the renal tubules include tubular epithelial cell apoptosis ferroptosis, pyroptosis, autophagy, epithelial-mesenchymal transformation, and peritubular pericyte migration. These lesions, as well as the thickening of the basement membrane and the accumulation of extracellular matrix, contribute to the exacerbation of fibrosis in diabetic kidneys. This figure is created with BioRender.com, and the authors have been granted a license to use the BioRender content.
3 Pathogenesis of diabetic kidney disease
Considering that the underlying cellular processes resulting in the aforementioned histological presentations are considerably complex, continued attention to the pathophysiology of DKD is required. There is general agreement that critical metabolic derangements affect kidney hemodynamics and encourage immune dysregulation in early diabetes (66). Owing to the direct relevance of metabolic disorders, dyslipidemia, and intracellular stress, there is an increasing level of ROS, advanced glycation end products (AGEs), and lipid deposition (67–69). Moreover, intracellular stress includes hypoxia, endoplasmic reticulum (ER) stress, and OS (70), which generates ROS such as peroxides, superoxide, and hydroxyl radicals (71), which are linked to podocyte damage, focal segmental glomerulosclerosis (FSGS), and tubulointerstitial fibrosis (72). Activation of the RAAS is likely correlated with hemodynamic mechanisms, such as glomerular hyperfiltration and hypertension (73–75), which have long been known to be responsible for the initiation and propagation of DKD (76). Additionally, interactions between metabolic disorders and hemodynamic abnormalities lead to immunological dysfunction including inflammation and renal fibrosis by triggering the production of pro-inflammatory and pro-fibrotic mediators (77–79). Moreover, emerging evidence indicates that autophagy dysregulation, mitochondrial dysfunction, apoptosis, pyroptosis, and ferroptosis contribute to renal damage in DKD (78, 80–83).
4 LncRNA biology
LncRNAs are abundant as functional units in eukaryotes, performing precise expression patterns in their various subcellular localizations, which has recently been the focus of intense debate. Numerous lncRNAs are limited to and abundant in the nucleus, governing transcriptional programs via chromatin remodeling and interactions in trans or cis (84). However, some are present in the cytoplasm, where they mediate signal transduction pathways, translational programs, or post-transcriptional genetic regulation, whereas others are present in the mitochondria and ER, where their functions remain still obscure (85).
Depending on their roles, lncRNAs are commonly categorized as signals, decoys, scaffolds, and guides molecules that engage in diverse biological processes that affect gene expression, including epigenetic, transcriptional, post-transcriptional, translational, and post-translational regulation (16, 86). LncRNAs unlock the capabilities of epigenetic gene regulation by affecting histone modifications close to gene transcriptional start sites, boosting methylation of the gene promoter, and encouraging chromatin remodeling (87–89). Indeed, lncRNAs actively participate in transcriptional regulation by either trapping and regulating transcription factors (TF) or functioning as active enhancers to stimulate or suppress transcription (90–92). In addition to their roles in epigenetic and transcriptional regulation, lncRNAs also handle post-transcriptional regulation through several approaches, including the formation of specific lncRNA-protein complexes (lncRNPs) that operate as competitive endogenous RNAs (ceRNAs) or “sponges” of microRNAs (miRNAs), thereby regulating alternative splicing, mRNA degradation and diminishing mRNA stability (93–97). Furthermore, lncRNAs regulate several other aspects of gene expression, such as the disruption of translational processes by binding to eukaryotic translation initiation factor 4 gamma 2 (EIF4G2) and post-translational regulation via protein interactions (98, 99).
5 Classical lncRNAs in DKD
Thus, understanding lncRNAs in eukaryotes has broadened our understanding of the role of lncRNAs in regulating gene expression and other biological processes. Reports on the interactions between lncRNAs and DKD have emerged in recent years, suggesting their essential roles in the pathogenesis of DKD. LncRNAs regulate many crucial factors linked to the DKD progression in different cell masses (100).
5.1 Podocyte injury: autophagy, apoptosis, mitochondrial dysfunction
For example, a transcript called lncRNA AK044604 (Risa) is situated very close to the sirt1 gene, which is recognized as a modulator of autophagy and insulin sensitivity, contributing to the onset of DKD through the regulation of podocyte autophagy and the thickness of GBM (101). Furthermore, decreased levels of the lncRNA taurine-upregulated gene 1(Tug1) are observed in podocytes exposed to high glucose (HG) stimuli, exhibiting a reno-protective role in DKD through the lncRNA Tug1/PGC1α axis, which is essential for improving mitochondrial function (102). In contrast, lncRNA maternally expressed gene 3 (Meg3) is upregulated under the HG conditions, thereby increasing dynamin-related protein 1 (Drp1) expression and its phosphorylation or translocation, which triggers mitochondrial fission and podocyte damage in diabetic mice induced by streptozotocin (STZ) (103). Similarly, lncRNA PVT1 is up-regulated in DKD patients, and in vitro experiments explain that it encourages the recruitment of histone 3 lysine 27 trimethylation (H3K27me3) in the forkhead box A1(FOXA1) promoter region by recruiting enhancer of zeste homolog 2 (EZH2), which lessens FOXA1 expression, thereby increasing apoptosis and damage levels in podocytes (104).
5.2 Tubular epithelial cells: EMT and fibrosis
Overexpression of lncRNA NEAT1 is observed in the TECs which further promotes EMT and fibrosis through the ERK1/2 pathway resulting in the accumulation of critical cytokines like connective tissue growth factor (CTGF), TGF-β, vimentin, and α-SMA (105). Additionally, under the treatment of TGF-β1, cytoplasmic lncRNA growth arrest-specific 5 (GAS5) expression is significantly increased in the human proximal tubule cell line (HK-2) and exerts its biological effects by serving as a sponge for the miR-96-5p and enhancing the formation of fibronectin to exacerbate renal fibrosis (106). Furthermore, in the AGE-treated GECs and TECs, the expression of lncRNA Erbb4-IR is dramatically elevated in a Smad3-dependent way which can boost the production of Col. I and Col. IV, as well as worsen renal fibrosis by sponging miR-29b (107).
5.3 Mesangial cells: proliferation and ECM accumulation
LncRNA NR_033515, competitively binding to miR-743b-5p, is engaged in fibrosis, EMT, and proliferation in DKD as evidenced by the rise in fibrogenic proteins and epithelial cell markers such P38, α-SMA, FN, E-cadherin, as well as mesenchymal marker Vimentin (108). Moreover, in the HG-treated mesangial cells, lncRNA cyclin-dependent kinase inhibitor 2B antisense RNA 1(CDKN2B-AS1) is significantly elevated which binds to miR-424-5p to stimulate the production of high mobility group AT-hook 2(HMGA2). The levels of the proteins linked with PI3K/AKT signaling are increased through the DKN2B-AS1/miR-424-5p/HMGA2 axis, together with the stimulation of cell proliferation and ECM buildup (109).
6 Biological functions of lncRNA H19 in DKD pathogenesis
In addition to the aforementioned lncRNAs, H19 has received an avalanche of interest from researchers because of its unique biological characteristics. Because of its differential expression during the endocrine progenitor stage of pancreatic-islet development, H19 is crucial for pancreatic-islet development and function (110). LncRNA H19 is highly expressed under hyperglycemia and serves as a crucial regulator in many pathophysiological processes of DKD, including the inflammatory response, EMT, cellular proliferation, apoptosis, autophagy, and fibrosis. We outline the following four modes of action of lncRNA H19 in modulating DKD: its role as a miRNA sponge, role in gene methylation, role as a precursor of miR-675, and role in interacting with protein. Additionally, we illustrated the regulatory mechanism of lncRNA H19 in different renal cells (Table 1).
6.1 Role as a miRNA sponge
LncRNA H19 serves as a miRNA sponge to regulate EndMT, proliferation, inflammation, EMT, fibrosis, oxidative stress, ferroptosis, and pyroptosis (Figure 3). For example, a considerable increase in lncRNA H19 expression has been observed in mouse mesangial cells under HG conditions. Then, the luciferase reporter assay demonstrated that lncRNA H19 binds to miR-143-3p to boost the production of inflammatory molecules interleukin-6 (IL-6) and TNF-α as well as pro-fibrogenic molecules like TGF-β1, Col. IV, and FN, thereby advancing the inflammation and proliferation of GMCs (111). Similarly, in the serum of clinical patients with CKD, lncRNA H19 was positively linked with the expression of TNF-α and IL-6, the latter served as a risk factor for DKD (112, 113). Consistent with these findings, lncRNA H19 was up-regulated in both the STZ-induced animal model and the TGF-β2-induced human microvascular endothelial cells (HMVECs), blocking miR-29a expression to significantly increase the activity of the TGF-β/Smad3 pathway (114). In addition, miR-29a promotes nephrin acetylation to reduce the effects of hyperglycemia on podocytes (115). Further research has revealed that in diabetic kidneys, lncRNA H19 knockdown inhibits EndMT by upregulating the expression of mesothelial cell marker FSP-1 and downregulating the endothelial marker CD31 (114).
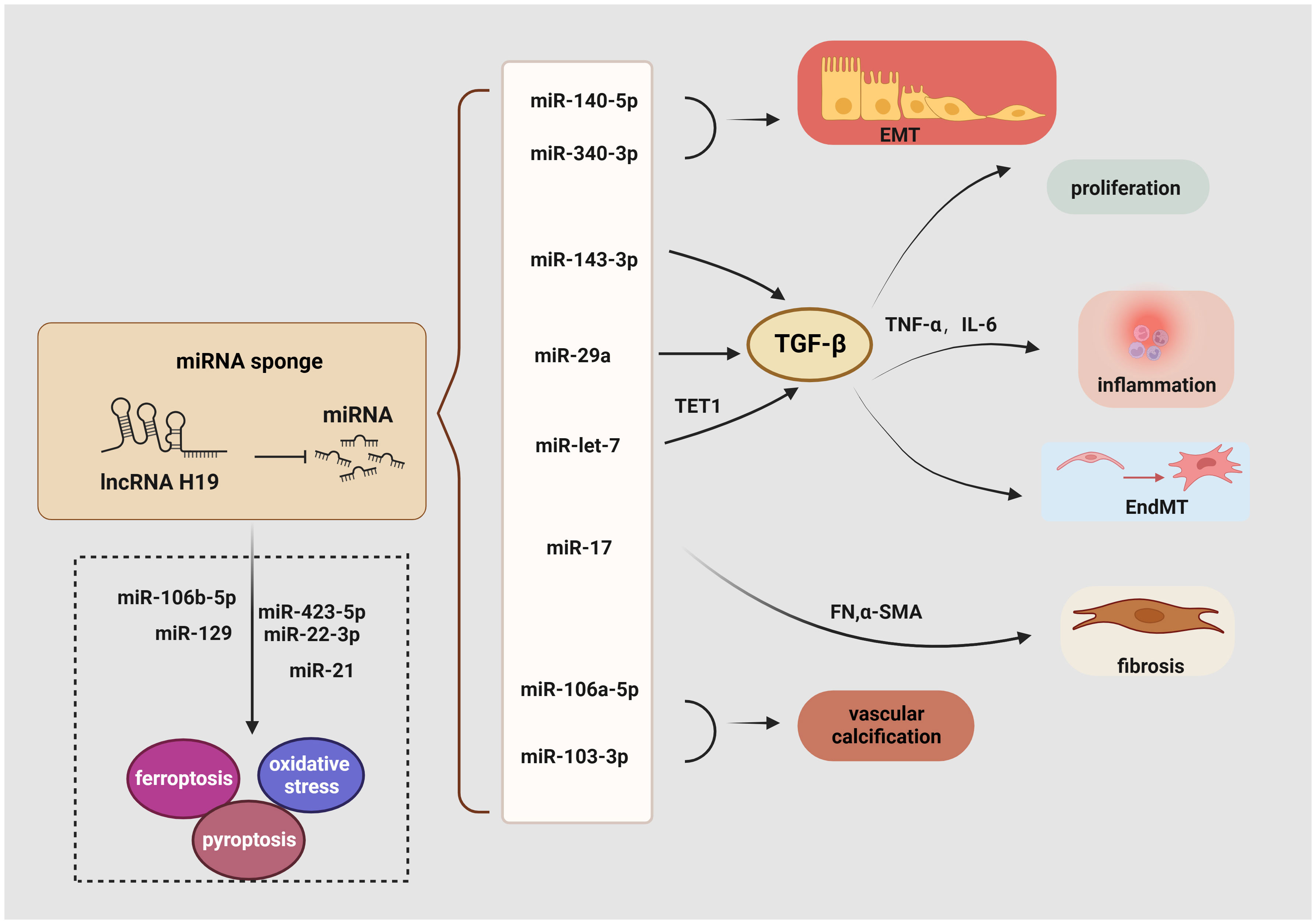
Figure 3 Role of lncRNA H19 as a miRNA sponge in DKD. LncRNA H19 could sponge miRNA inhibits its expression and engage in regulating the diverse pathogenesis of DKD, including EMT, EndMT, inflammation, proliferation, oxidative stress, ferroptosis, pyroptosis, autophagy, and fibrosis. →: promote, —: inhibit. This figure is created with BioRender.com, and the authors have been granted a license to use the BioRender content.
Another recent study demonstrated a favorable correlation between lncRNA H19 and renal fibrosis. Using TGF-β-induced HK-2 cells, StarBase 2.0 to identify miRNA recognition sites associated with lncRNA H19 during renal fibrosis, including miR-20, miR-93, miR-106, miR-18, and miR-17. It was shown that lncRNA H19 can function as an endogenous RNA to decrease miR-17 levels and boost the production of α-SMA, FN, Col. IV, and Col. I but reduce E-cadherin in unilateral ureteral obstruction mice. These findings collectively show that lncRNA H19 exacerbates renal fibrosis in DKD via the miR-17/fibronectin regulatory networks (116). Moreover, by serving as a miRNA sponge for let7, lncRNA H19 positively controlled the production of ten-eleven translocation (TET1), which in turn influences the epigenetic regulation of upstream TGF signaling genes including thrombospondin 1 (TSP1) and TGF‐β receptor 2 (TGFBR2). This increased the phosphorylation of the TGF signaling intermediate SMAD2 and the overexpression of the EndMT markers FN, vimentin, and smooth muscle 22 alpha (SM22‐α). Based on these findings, it suggests that the lncRNA H19/TET1 axis may contribute to phenotypic alterations during DKD progression (127). Besides, lncRNA H19 activates the PI3K/AKT pathway and regulates tyrosine 3-monooxygenase/tryptophan 5-monooxygenase activation protein zeta (YWHAZ) expression to promote EMT by working as an endogenous sponge for miR-340-3p and miR-140-5p (128, 129). Similarly, binding to and suppressing miRNAs like miR-138 and miR-148a, lncRNA H19 promoted the EMT markers along with stabilizing TGF-β through lncRNA H19/miR-138/SOX4 and lncRNA H19/miR-148a/ubiquitin-specific protease 4 (USP4) axes, which may further result in fibrosis (130, 131). Through sponging miR-106a-5p or miR-103-3p in a Runx2 dependent way, elevation of H19 contributes to increased vascular calcification (VC) in the kidney (132, 133).
Ferroptosis is another potential target of lncRNA H19 in DKD. Previous studies revealed that curcumin therapy markedly decreases the production of the lncRNA H19 in lung cancer cells to induce ferroptosis. Mechanistically, lncRNA H19, which works as a rival endogenous RNA bounding to miR-19b-3p, suppresses ferroptosis as shown by the increased transcriptional activity of ferritin heavy chain 1 (FTH1), a gene that is both an endogenous target of miR-19b-3p and a depressor of ferroptosis (134). Additionally, lncRNA H19 knockdown increased cell division and decreased ferroptosis in brain microvascular endothelial cells (BMVECs) by modulating the miR-106b-5p/acyl-CoA synthetase long-chain family member 4(ACSL4) axis (135). Intriguingly, an increase in the tubular pro-ferroptosis gene ACSL4 was linked to the renal function of acute kidney tubular injury patients, which triggered ferroptosis in TECs (136). Furthermore, lncRNA H19 has been shown to serve as a sponge for miR-129 (137), and, positively regulate ion of high-mobility group box 1 (HMGB1) which modulates oxidative stress and hyperglycemia-induced ferroptosis in mesangial cells through the nuclear-related factor 2 (Nrf2) pathway (117).
Given the crucial role of autophagy and pyroptosis in the progression of DKD, the regulatory relationship between lncRNA H19 and regulated cell death (RCD) cannot be neglected. Previous research has illustrated that lncRNA H19 also serves as a sponge for miR-22-3p and miR-423-5p to boost the activity of the Nod-like receptor family pyrin domain-containing 3 (NLRP3) (138, 139), a marker of pyroptosis and a factor in podocyte damage, thereby encouraging pyroptosis (118). Besides targeting miR-423-5p and miR-22-3p, lncRNA H19 also exhibits negative effects on miR-21, whereas the reduction of miR-21 enhances the expression of programmed cell death 4 (PDCD4), forming a competing endogenous RNA network (ceRNET) that significantly promotes an imbalance in the NLRP3/6 inflammasome, leading to pyroptosis (140). Another fascinating finding suggests that lncRNA H19 reverses mitochondrial damage and cell growth inhibition by sponging miR-93-5p under the condition of lipopolysaccharide (LPS) (141). Similarly, increased levels of lncRNA H19 trigger cellular autophagy via the lncRNA H19/miR-143/autophagy-related protein 7 (ATG7) signaling axis (142), while ATG7 is a hallmark of podocyte autophagy (119).
Apart from a miRNA sponge, lncRNA H19 exhibits its regulating effects in gene methylation, protein interaction, and transcription of small RNAs (Figure 4).
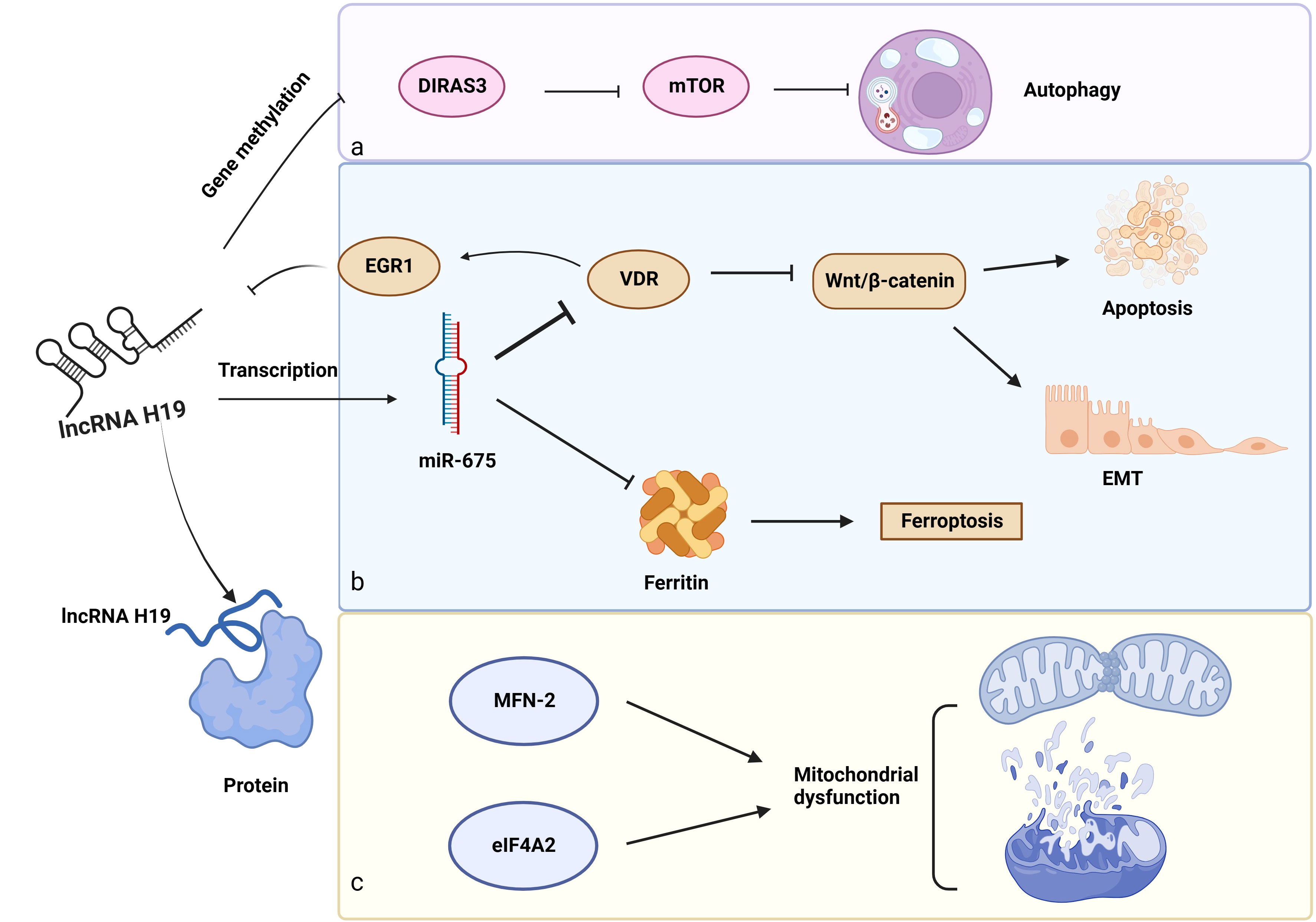
Figure 4 Other biological functions of lncRNA H19 in DKD. a: Gene methylation: LncRNA H19 regulates gene methylation of DIRAS3 to inhibit autophagy. b: Role of the lncRNA H19/miR-675 axis in DKD, c: LncRNA H19 interacts with proteins (MFN-2 and eIF4A2) to induce mitochondrial dysfunction. →: promote, —: inhibit. This figure is created with BioRender.com, and the authors have been granted a license to use the BioRender content.
6.2 Role in gene methylation
In addition to acting as a miRNA sponge, lncRNA H19 influences the gene methylation of downstream cytokines. These findings suggest that lncRNA H19 binds to and inhibits the enzyme S-adenosylhomocysteine hydrolase (SAHH), resulting in the build-up of SAH, which prevents the transcription factor DNA methyltransferase 3 B (DNMT3B) from methylating the Beclin1 promoter. As a consequence, lncRNA H19 encouraged Beclin1 transcription to trigger autophagy (143). However, the serum of patients with DKD has lower levels of Beclin-1, pointing to the probable involvement of lncRNA H19 and autophagy in this disease (120). Aplasia Ras homolog member I (DIRAS3), which is extensively expressed in epithelial cells, encodes a small GTP-binding protein (121). Interestingly, lncRNA H19 decreased the amounts of DIRAS3 and increased the mTOR-related proteins to inhibit podocyte autophagy (144), however, a high level of podocyte autophagy is essential to maintain renal homeostasis (145). Shensu IV, a well-known Chinese prescription, promotes lncRNA H19/DIRAS3-regulated autophagy to prevent kidney injuries in DKD (144).
6.3 Role as a precursor of small RNAs: lncRNA H19/miR-675 axis
Regarding the unique structures of miR-675 and H19, scientists have revealed some interesting findings. MiR-675, which is embedded in the first exon of H19 gene (146), is activated in response to increased lncRNA H19 synthesis, indicating that lncRNA H19 modulated the transcription of miR-675. Luciferase reporter assay further confirmed this combination. What’s more, 3′ UTR of vitamin D receptor (VDR) contains a complementary sequence of miR-675, providing a regulatory possibility for miR-675. The VDR is negatively linked to early growth response protein 1 (EGR1), a transcription factor of lncRNA H19. Furthermore, EGR1 stimulated the Wnt/β-catenin pathway, the classical signal of EMT, to accelerate renal fibrosis. These observations suggest a negative feedback loop for lncRNA H19/miR-675/EGR1 during the progression of DKD (24, 122). Notably, there might be a similar function of lncRNA H19/miR-675 in DKD because of its role in regulating EMT in hepatocytes, breast cells, and skin squamous cells (147–149), as well as its inhibitory effect on iron-stored protein ferritin (FHC), which is necessary to maintain iron metabolism in the kidney (150).
6.4 Role in interacting with protein
LncRNA H19 also influences downstream protein expression via direct binding. By binding to heterogeneous nuclear ribonucleoprotein A2B1(hnRNPA2B1), lncRNA H19 maintains and increases the expression of Raf-1 to activate the Raf-ERK signaling linked to EMT (151). In addition, overexpression of lncRNA H19 prevented Pink1 mRNA from binding to eukaryotic translation initiation factor 4A, isoform 2 (eIF4A2), blocking the translation of Pink1 and reducing mitophagy through the Pink1/Parkin pathway (152). Furthermore, in diabetic mice, H19 interacts with mitofusin-2 (MFN-2) mRNA. MFN-2 is a dynamin GTPase found on the outer mitochondrial membrane that is encoded by nuclear genes and is responsible for outer mitochondrial membrane fusion (123).
6.5 Role in other regulating factors related to DKD
However, some studies did not explain the specific working patterns of lncRNA H19 in DKD, leading to the following conclusions. One study showed that lncRNA H19 is highly expressed in both DKD rats and rat glomerular endothelial cells (rGEnCs). Further experiments demonstrated that lncRNA H19 knockdown reduced the GBM and ameliorated GEC impairment, as indicated by the high levels of the principal glycocalyx components WGA and Syndecan-1, as well as the essential tight junction proteins ZO-1 and Occludin. Additional research has demonstrated that lncRNA H19 has a deleterious effect on DKD by blocking Akt/eNOS signaling, which is essential for podocyte and GEC damage (124–126). According to a recent study, phospholipid hydroperoxide glutathione peroxidase (GPX4), a factor that negatively regulates ferroptosis, is favorably linked to lncRNA H19 levels during spontaneous abortion (153). Similarly, renal biopsy samples from patients with DKD show lower GPX4 expression than those from healthy controls, suggesting an independent predictor of ESKD development (154). These discoveries will make it possible to understand the relationships between lncRNA H19 and GPX4, which will help choose therapeutic targets to control ferroptosis and manage DKD progression.
In other disease models, lncRNA H19 plays a role in collective pathogenesis, providing more possibilities for the role of lncRNA H19 in DKD. For instance, lncRNA H19 promoted oxidative stress and released inflammatory factors IL-6, IL-1β, and TNF- by stimulating the NF-kB pathway in intracerebral hemorrhage (ICH) rats (155). Additionally, lncRNA H19 might encourage the translocation of β-catenin into the nucleus and trigger Wnt/β-catenin signaling, leading to EMT (156). In hepatocytes induced by IL-22, it is interesting to note that an elevation in lncRNA H19 influenced the expression of t AMPK and AKT proteins, which are upstream regulators of mTOR signaling, ultimately preserving mitochondrial function and integrity by activating the AMPK/AKT/mTOR axis (157). Besides, it has been proven that lncRNA H19 lowered the formation of ROS and lessened mitochondrial damage by suppressing the NF-kB activation driven on by ox-LDL (158).
7 Role of lncRNA H19 in nondiabetic kidney disease
Nondiabetic kidney disease (NDKD) refers to kidney diseases unrelated to diabetes, including glomerulonephritis, acute kidney injury (AKI), focal segmental glomerulosclerosis, and minimal change disease. Nevertheless, renal biopsy in diabetic patients with chronic kidney diseases may indicate the following three possibilities: DKD, NDKD, or a mixture of both DKD and NDKD (159). Therefore, additional investigations of lncRNA H19 in NDKD are helpful to fully understand its role in DKD.
DKD patients are more susceptible to AKI, which has been shown to deteriorate kidney function and is challenging to recover from when it occurs in DKD (160). In AKI model mice, the elevated expression of lncRNA H19 has been shown to stimulate the synthesis of Wnt and β-catenin through sponging miR-196a-5p, promoting Wnt/β-catenin signaling pathway, which in turn promotes renal fibrosis (161). Meanwhile, another study verifies that lncRNA H19 regulates the apoptosis, proliferation, and inflammation of TECs in a miR-130 manner. The pro-apoptotic proteins are elevated whereas the anti-apoptotic protein Bcl-2 is decreased in HK-2 cells due to the lncRNA H19/miR-130a axis. Additionally, the lncRNA H19/miR-130a/BCL2L11 axis stimulates the production of IL-6, IL-1, and TNF-α while suppressing IL-10, resulting in inflammation in the TECs (162). Notably, inconsistent outcomes are observed in the renal adeno-associated virus 2 (AAV2)-mediated mouse model. LncRNA H19 is observed to upregulate in the kidney biopsies of AKI patients. In vivo experiments then confirm that lncRNA H19, which sponges miR-30a-5p, could attenuate apoptosis and inflammation while also stimulating pro-angiogenic signaling, suggesting a protective role against kidney injury. It proves that capillary density and tubular epithelial integrity can be preserved in the lncRNA H19/miR-30a-5p axis (163). Altogether, these findings imply that lncRNA H19 might be crucial in AKI.
Researchers found that lncRNA H19 was overexpression in the glyoxylate-induced Calcium oxalate (CaOx) nephrocalcinosis mouse models. Their additional findings that lncRNA H19 accelerates renal epithelial cell injury in a miR-216b-5p manner, thereby up-regulating the expression of HMGB1, another target of miR-216b-5p that is negatively linked with it. Then HMGB1 interacted with toll-like receptor 4 (TLR4) to promote the transcription and expression of several chemokines and proinflammatory cytokines like IL-6, IL-1β, and TNF-α via NF-kB or NADPH oxidase ways (164). It is recognized that TLRs are endogenous danger-associated molecular patterns produced in DM that allow for the stimulation of the NF-kB signaling to trigger a sterile tubulointerstitial inflammatory response (77). These results collectively revealed a possible involvement for the lncRNA H19/mir-216b-5p/HMGB1 axis in the pathology of TEC injury through the modulation of OS and inflammatory response (164). It was recently discovered in nephroblastoma cells that lncRNA H19 significantly influences the miR-675/TGFBI axis to enhance proliferation and prevent apoptosis (165). Intriguingly, it has been shown that IGF2 and lncRNA H19 compete for the same binding enhancer, and the lncRNA H19-IGF2 imprinted gene area is likely linked to an increased risk of having compromised renal function (IRF) (166). Otherwise, renal clear cell carcinoma, Wilms tumors, and renal cell carcinoma are all influenced by the interaction between lncRNA H19 and IGF2 (167–169).
8 Conclusion and perspectives
This review focuses on the primary pathogenic alterations and mechanisms of DKD, as well as the functions of lncRNAs in DKD, highlighting lncRNA H19. Substantial evidence supports the essential role of lncRNA H19 in the physiology and pathology of the diabetic kidney. As a result, we outlined how lncRNA H19 might affect the phenotypes associated with DKD, including inflammation, oxidative stress, autophagy, mitochondrial dysfunction, EMT, EndMT, ECM buildup, and fibrosis. We divided the mechanisms of lncRNA H19 in controlling DKD into four groups at the molecular level: regulation of gene methylation, protein interactions, miRNA sponges, and small RNA precursors. Among these, we find that serving as miRNA sponges is more common for lncRNA H19, which sponge miRNA to regulate downstream factors to modulate fibrosis-related progressions and hastens the development of DKD. Previous research revealed that lncRNA H19 is an independent risk factor for T2DM. Increased renal indicators (urea, creatinine, and UACR) and nephropathy are more frequently linked to H19 expression (170, 171). In addition, the discovery that metformin, a first-line therapy for type 2 diabetes, might lessen kidney damage through downregulating lncRNA H19, and therefore DKD, offers another direction for our investigation of the potential of metformin for treating DKD. Despite these developments, many unresolved problems persist. Drugs targeting lncRNA H19 are limited. We can focus on some Chinese traditional medicine such as Shensu IV to deeply explore the mechanisms of lncRNA H19, providing novel therapy for DKD patients. Hence, in-depth research on lncRNA H19 is required. More mechanistic and translational studies with tissue- and cell-type-specific H19−/− animal models are needed. Additionally, studies are required to validate preclinical findings in human samples and to examine the effects of compounds that modulate lncRNA H19 in DKD clinical trials. In the future, we propose a hypothesis that we can identify a functional motif of H19 and deliver the functional motif to kidney cells to alleviate kidney injuries, which is similar to some lncRNA-targeted therapy in pathological cardiac hypertrophy and tumor disorders. Furthermore, given the imprinted properties of lncRNA H19, we may utilize its expression variations to investigate the prevalence of DKD in various sexes, offering novel approaches for illuminating the genetic landscape and the early detection of DKD. In a word, lncRNA H19 provides more possibilities for us to diagnose and treat DKD.
Author contributions
QW wrote and revised the manuscript. FH critically revised the manuscript. All authors contributed to the article and approved the submitted version.
Funding
The authors’ work cited in this review was supported by grants from the National Natural Science Foundation of China (Grant number: 82000787), Henan Medical Science and Technology Research Program Project (Grant number: SBGJ202102142), and the Postdoctoral Research Foundation of China (Grant number: 2021M692949).
Acknowledgments
We would like to thank Editage (www.editage.cn) for English language editing.
Conflict of interest
The authors declare that the research was conducted in the absence of any commercial or financial relationships that could be construed as a potential conflict of interest.
Publisher’s note
All claims expressed in this article are solely those of the authors and do not necessarily represent those of their affiliated organizations, or those of the publisher, the editors and the reviewers. Any product that may be evaluated in this article, or claim that may be made by its manufacturer, is not guaranteed or endorsed by the publisher.
References
1. A.D. Association. Diagnosis and classification of diabetes mellitus. Diabetes Care (2014) 37:S81–90. doi: 10.2337/dc14-S081
2. Sun H, Saeedi P, Karuranga S, Pinkepank M, Ogurtsova K, Duncan BB, et al. IDF Diabetes Atlas: Global, regional and country-level diabetes prevalence estimates for 2021 and projections for 2045. Diabetes Res Clin Pract (2022) 183. doi: 109119.10.1016/j.diabres.2021.109119
3. Pettus JH, Zhou FL, Shepherd L, Preblick R, Hunt PR, Paranjape S, et al. Incidences of severe hypoglycemia and diabetic ketoacidosis and prevalence of microvascular complications stratified by age and glycemic control in U.S. Adult patients with type 1 diabetes: A real-world study. Diabetes Care (2019) 42:2220–7. doi: 10.2337/dc19-0830
4. Bjerg L, Hulman A, Carstensen B, Charles M, Jørgensen ME, Witte. DR. Development of microvascular complications and effect of concurrent risk factors in type 1 diabetes: A multistate model from an observational clinical cohort study. Diabetes Care (2018) 41:2297–305. doi: 10.2337/dc18-0679
5. Jager KJ, Kovesdy C, Langham R, Rosenberg M, Jha V, Zoccali. C. A single number for advocacy and communication-worldwide more than 850 million individuals have kidney diseases. Nephrol Dial Transplant (2019) 34:1803–5. doi: 10.1093/ndt/gfz174
6. González-Pérez A, Saez M, Vizcaya D, Lind M, Garcia Rodriguez. L. Incidence and risk factors for mortality and end-stage renal disease in people with type 2 diabetes and diabetic kidney disease: a population-based cohort study in the UK. BMJ Open Diabetes Res Care (2021) 9. doi: 10.1136/bmjdrc-2021-002146
7. Lok CE, Perazella MA, Choi. MJ. American society of nephrology quiz and questionnaire 2015: ESRD/RRT. Clin J Am Soc Nephrol (2016) 11:1313–20. doi: 10.2215/cjn.01280216
8. Pichaiwong W, Homsuwan W, Leelahavanichkul. A. The prevalence of normoalbuminuria and renal impairment in type 2 diabetes mellitus. Clin Nephrol (2019) 92:73–80. doi: 10.5414/cn109606
9. Oshima M, Shimizu M, Yamanouchi M, Toyama T, Hara A, Furuichi K, et al. Trajectories of kidney function in diabetes: a clinicopathological update. Nat Rev Nephrol (2021) 17:740–50. doi: 10.1038/s41581-021-00462-y
10. Mori Y, Ajay AK, Chang JH, Mou S, Zhao H, Kishi S, et al. KIM-1 mediates fatty acid uptake by renal tubular cells to promote progressive diabetic kidney disease. Cell Metab (2021) 33:1042–1061.e7. doi: 10.1016/j.cmet.2021.04.004
11. Thomas MC, Brownlee M, Susztak K, Sharma K, Jandeleit-Dahm KA, Zoungas S, et al. Diabetic kidney disease. Nat Rev Dis Primers (2015) 1:15018. doi: 10.1038/nrdp.2015.18
12. Guo J, Liu Z, Gong. R. Long noncoding RNA: an emerging player in diabetes and diabetic kidney disease. Clin Sci (Lond) (2019) 133:1321–39. doi: 10.1042/cs20190372
13. Derrien T, Johnson R, Bussotti G, Tanzer A, Djebali S, Tilgner H, et al. The GENCODE v7 catalog of human long noncoding RNAs: analysis of their gene structure, evolution, and expression. Genome Res (2012) 22:1775–89. doi: 10.1101/gr.132159.111
14. Nojima T, Proudfoot. NJ. Mechanisms of lncRNA biogenesis as revealed by nascent transcriptomics. Nat Rev Mol Cell Biol (2022) 23:389–406. doi: 10.1038/s41580-021-00447-6
15. Mattick JS, Rinn. JL. Discovery and annotation of long noncoding RNAs. Nat Struct Mol Biol (2015) 22:5–7. doi: 10.1038/nsmb.2942
16. Statello L, Guo CJ, Chen LL, Huarte. M. Gene regulation by long non-coding RNAs and its biological functions. Nat Rev Mol Cell Biol (2021) 22:96–118. doi: 10.1038/s41580-020-00315-9
17. Srivastava SP, Goodwin JE, Tripathi P, Kanasaki K, Koya. D. Interactions among Long Non-Coding RNAs and microRNAs Influence Disease Phenotype in Diabetes and Diabetic Kidney Disease. Int J Mol Sci (2021) 22. doi: 10.3390/ijms22116027
18. Brannan CI, Dees EC, Ingram RS, Tilghman. SM. The product of the H19 gene may function as an RNA. Mol Cell Biol (1990) 10:28–36. doi: 10.1128/mcb.10.1.28-36.1990
19. Hurst LD, Smith. NG. Molecular evolutionary evidence that H19 mRNA is functional. Trends Genet (1999) 15:134–5. doi: 10.1016/s0168-9525(99)01696-0
20. Kanwar YS, Pan X, Lin S, Kumar A, Wada J, Haas CS, et al. Imprinted mesodermal specific transcript (MEST) and H19 genes in renal development and diabetes. Kidney Int (2003) 63:1658–70. doi: 10.1046/j.1523-1755.2003.00905.x
21. Raveh E, Matouk IJ, Gilon M, Hochberg. A. The H19 Long non-coding RNA in cancer initiation, progression and metastasis - a proposed unifying theory. Mol Cancer (2015) 14:184. doi: 10.1186/s12943-015-0458-2
22. Wang WT, Ye H, Wei PP, Han BW, He B, Chen ZH, et al. LncRNAs H19 and HULC, activated by oxidative stress, promote cell migration and invasion in cholangiocarcinoma through a ceRNA manner. J Hematol Oncol (2016) 9:117. doi: 10.1186/s13045-016-0348-0
23. Yang Z, Zhang T, Han S, Kusumanchi P, Huda N, Jiang Y, et al. Long noncoding RNA H19 - a new player in the pathogenesis of liver diseases. Transl Res (2021) 230:139–50. doi: 10.1016/j.trsl.2020.11.010
24. Fan W, Peng Y, Liang Z, Yang Y, Zhang. J. A negative feedback loop of H19/miR-675/EGR1 is involved in diabetic nephropathy by downregulating the expression of the vitamin D receptor. J Cell Physiol (2019) 234:17505–13. doi: 10.1002/jcp.28373
25. Tervaert TW, Mooyaart AL, Amann K, Cohen AH, Cook HT, Drachenberg CB, et al. Pathologic classification of diabetic nephropathy. J Am Soc Nephrol (2010) 21:556–63. doi: 10.1681/asn.2010010010
26. Wiggenhauser LM, Metzger L, Bennewitz K, Soleymani S, Boger M, Tabler CT, et al. pdx1 knockout leads to a diabetic nephropathy- like phenotype in zebrafish and identifies phosphatidylethanolamine as metabolite promoting early diabetic kidney damage. Diabetes (2022) 71:1073–80. doi: 10.2337/db21-0645
27. Abrahamson DR. Role of the podocyte (and glomerular endothelium) in building the GBM. Semin Nephrol (2012) 32:342–9. doi: 10.1016/j.semnephrol.2012.06.005
28. Naylor RW, Morais M, Lennon R. Complexities of the glomerular basement membrane. Nat Rev Nephrol (2021) 17:112–27. doi: 10.1038/s41581-020-0329-y
29. Siddiqi FS, Advani A. Endothelial-podocyte crosstalk: the missing link between endothelial dysfunction and albuminuria in diabetes. Diabetes (2013) 62:3647–55. doi: 10.2337/db13-0795
30. Casalena GA, Yu L, Gil R, Rodriguez S, Sosa S, Janssen W, et al. The diabetic microenvironment causes mitochondrial oxidative stress in glomerular endothelial cells and pathological crosstalk with podocytes. Cell Commun Signal (2020) 18:105. doi: 10.1186/s12964-020-00605-x
31. Pagtalunan ME, Miller PL, Jumping-Eagle S, Nelson RG, Myers BD, Rennke HG, et al. Podocyte loss and progressive glomerular injury in type II diabetes. J Clin Invest (1997) 99:342–8. doi: 10.1172/jci119163
32. Yin L, Yu L, He JC, Chen A. Controversies in podocyte loss: death or detachment? Front Cell Dev Biol (2021) 9. doi: 10.3389/fcell.2021.771931
33. Susztak K, Raff AC, Schiffer M, Böttinger EP. Glucose-induced reactive oxygen species cause apoptosis of podocytes and podocyte depletion at the onset of diabetic nephropathy. Diabetes (2006) 55:225–33. doi: 10.2337/diabetes.55.01.06.db05-0894
34. Romagnani P, Remuzzi G. Renal progenitors in non-diabetic and diabetic nephropathies. Trends Endocrinol Metab (2013) 24:13–20. doi: 10.1016/j.tem.2012.09.002
35. Zhou J, Yang J, Wang YM, Ding H, Li TS, Liu ZH, et al. IL-6/STAT3 signaling activation exacerbates high fructose-induced podocyte hypertrophy by ketohexokinase-A-mediated tristetraprolin down-regulation. Cell Signal (2021) 86:110082. doi: 10.1016/j.cellsig.2021.110082
36. Puelles VG, van der Wolde JW, Wanner N, Scheppach MW, Cullen-McEwen LA, Bork T, et al. mTOR-mediated podocyte hypertrophy regulates glomerular integrity in mice and humans. JCI Insight (2019) 4. doi: 10.1172/jci.insight.99271
37. Saga N, Sakamoto K, Matsusaka T, Nagata M. Glomerular filtrate affects the dynamics of podocyte detachment in a model of diffuse toxic podocytopathy. Kidney Int (2021) 99:1149–61. doi: 10.1016/j.kint.2020.12.034
38. Jourde-Chiche N, Fakhouri F, Dou L, Bellien J, Burtey S, Frimat M, et al. Endothelium structure and function in kidney health and disease. Nat Rev Nephrol (2019) 15:87–108. doi: 10.1038/s41581-018-0098-z
39. Satchell S. The role of the glomerular endothelium in albumin handling. Nat Rev Nephrol (2013) 9:717–25. doi: 10.1038/nrneph.2013.197
40. Jiang L, Zhou J, Zhang L, Du Y, Jiang M, Xie L, et al. The association between serum interleukin-1 beta and heparin sulphate in diabetic nephropathy patients. Glycoconj J (2021) 38:697–707. doi: 10.1007/s10719-021-10035-7
41. Ebefors K, Khramova A, Nyström J. The role of proteoglycans in glomerular physiology and pathophysiology. FASEB J (2020) 34:1–1. doi: 10.1096/fasebj.2020.34.s1.09398
42. Zheng F, Ma L, Li X, Gao R, Peng C, Kang B, et al. Neutrophil extracellular traps induce glomerular endothelial cell dysfunction and pyroptosis in diabetic kidney disease. Diabetes (2022) 71:2739–50. doi: 10.2337/db22-0153
43. Tang LX, Wei B, Jiang LY, Ying YY, Li K, Chen TX, et al. Intercellular mitochondrial transfer as a means of revitalizing injured glomerular endothelial cells. World J Stem Cells (2022) 14:729–43. doi: 10.4252/wjsc.v14.i9.729
44. Schlöndorff D, Banas B. The mesangial cell revisited: no cell is an island. J Am Soc Nephrol (2009) 20:1179–87. doi: 10.1681/asn.2008050549
45. ScIndia YM, Deshmukh US, Bagavant H. Mesangial pathology in glomerular disease: targets for therapeutic intervention. Adv Drug Delivery Rev (2010) 62:1337–43. doi: 10.1016/j.addr.2010.08.011
46. Yin J, Wang K, Zhu X, Lu G, Jin D, Qiu J, et al. Procyanidin B2 suppresses hyperglycemia-induced renal mesangial cell dysfunction by modulating CAV-1-dependent signaling. Exp Ther Med (2022) 24:496. doi: 10.3892/etm.2022.11423
47. Kriz W, Löwen J, Federico G, Born Jvd, Gröne E, Gröne HJ. Accumulation of worn-out GBM material substantially contributes to mesangial matrix expansion in diabetic nephropathy. Am J Physiol Renal Physiol (2017) 312:F1101–f1111. doi: 10.1152/ajprenal.00020.2017
48. Lei C-T, Tang H, Ye C, You C-Q, Zhang J, Zhang C-Y, et al. MDM2 contributes to high glucose-induced glomerular mesangial cell proliferation and extracellular matrix accumulation via Notch1. Sci Rep (2017) 7:1–12. doi: 10.1038/s41598-017-10927-5
49. Nilsson LM, Zhang L, Bondar A, Svensson D, Wernerson A, Brismar H, et al. Prompt apoptotic response to high glucose in SGLT-expressing renal cells. Am J Physiol-Renal Physiol (2019) 316:F1078–89. doi: 10.1152/ajprenal.00615.2018
50. Chen K, Yu B, Liao J. LncRNA SOX2OT alleviates mesangial cell proliferation and fibrosis in diabetic nephropathy via Akt/mTOR-mediated autophagy. Mol Med (2021) 27:71. doi: 10.1186/s10020-021-00310-6
51. Wang L, Yuan X, Lian L, Guo H, Zhang H, Zhang M. Knockdown of lncRNA NORAD inhibits the proliferation, inflammation and fibrosis of human mesangial cells under high-glucose conditions by regulating the miR-485/NRF1 axis. Exp Ther Med (2021) 22:874. doi: 10.3892/etm.2021.10306
52. Xie L, Zhai R, Chen T, Gao C, Xue R, Wang N, et al. Panax notoginseng ameliorates podocyte EMT by targeting the wnt/β-catenin signaling pathway in STZ-induced diabetic rats. Drug Des Devel Ther (2020) 14:527–38. doi: 10.2147/dddt.S235491
53. Li L, Chen L, Zang J, Tang X, Liu Y, Zhang J, et al. C3a and C5a receptor antagonists ameliorate endothelial-myofibroblast transition via the Wnt/β-catenin signaling pathway in diabetic kidney disease. Metabolism (2015) 64:597–610. doi: 10.1016/j.metabol.2015.01.014
54. Mihevc M, Petreski T, Maver U, Bevc S. Renal proximal tubular epithelial cells: review of isolation, characterization, and culturing techniques. Mol Biol Rep (2020) 47:9865–82. doi: 10.1007/s11033-020-05977-4
55. Monu SR, Ren Y, Masjoan-Juncos JX, Kutskill K, Wang H, Kumar N, et al. Connecting tubule glomerular feedback mediates tubuloglomerular feedback resetting after unilateral nephrectomy. Am J Physiol Renal Physiol (2018) 315:F806–f811. doi: 10.1152/ajprenal.00619.2016
56. Thomas MC. Targeting the pathobiology of diabetic kidney disease. Adv Chronic Kidney Dis (2021) 28:282–9. doi: 10.1053/j.ackd.2021.07.001
57. Grahammer F, Haenisch N, Steinhardt F, Sandner L, Roerden M, Arnold F, et al. mTORC1 maintains renal tubular homeostasis and is essential in response to ischemic stress. Proc Natl Acad Sci U.S.A. (2014) 111:E2817–26. doi: 10.1073/pnas.1402352111
58. Anders HJ, Huber TB, Isermann B, Schiffer M. CKD in diabetes: diabetic kidney disease versus nondiabetic kidney disease. Nat Rev Nephrol (2018) 14:361–77. doi: 10.1038/s41581-018-0001-y
59. Kumar D, Robertson S, Burns KD. Evidence of apoptosis in human diabetic kidney. Mol Cell Biochem (2004) 259:67–70. doi: 10.1023/b:mcbi.0000021346.03260.7e
60. Shahzad K, Bock F, Al-Dabet MM, Gadi I, Kohli S, Nazir S, et al. Caspase-1, but not caspase-3, promotes diabetic nephropathy. J Am Soc Nephrol (2016) 27:2270–5. doi: 10.1681/asn.2015060676
61. Kim S, Kang SW, Joo J, Han SH, Shin H, Nam BY, et al. Characterization of ferroptosis in kidney tubular cell death under diabetic conditions. Cell Death Dis (2021) 12:160. doi: 10.1038/s41419-021-03452-x
62. Wang WW, Liu YL, Wang MZ, Li H, Liu BH, Tu Y, et al. Inhibition of renal tubular epithelial mesenchymal transition and endoplasmic reticulum stress-induced apoptosis with Shenkang injection attenuates diabetic tubulopathy. Front Pharmacol (2021) 12:662706. doi: 10.3389/fphar.2021.662706
63. Chen Q, Gao C, Wang M, Fei X, Zhao N. TRIM18-regulated STAT3 signaling pathway via PTP1B promotes renal epithelial-mesenchymal transition, inflammation, and fibrosis in diabetic kidney disease. Front Physiol (2021) 12:709506. doi: 10.3389/fphys.2021.709506
64. Humphreys BD. Targeting pericyte differentiation as a strategy to modulate kidney fibrosis in diabetic nephropathy. Semin Nephrol (2012) 32:463–70. doi: 10.1016/j.semnephrol.2012.07.009
65. Xu Z, Jia K, Wang H, Gao F, Zhao S, Li F, et al. METTL14-regulated PI3K/Akt signaling pathway via PTEN affects HDAC5-mediated epithelial-mesenchymal transition of renal tubular cells in diabetic kidney disease. Cell Death Dis (2021) 12:32. doi: 10.1038/s41419-020-03312-0
66. Tuttle KR, Agarwal R, Alpers CE, Bakris GL, Brosius FC, Kolkhof P, et al. Molecular mechanisms and therapeutic targets for diabetic kidney disease. Kidney Int (2022) 102:248–60. doi: 10.1016/j.kint.2022.05.012
67. Opazo-Ríos L, Mas S, Marín-Royo G, Mezzano S, Gómez-Guerrero C, Moreno JA, et al. Lipotoxicity and diabetic nephropathy: novel mechanistic insights and therapeutic opportunities. Int J Mol Sci (2020) 21. doi: 10.3390/ijms21072632
68. Beisswenger PJ, Bebu I, Howell S, Lachin J. Advanced Glycation End products (AGEs)-role in development and progression of kidney disease in type 1 diabetes in DCCT/EDIC. Diabetes (2018) 67. doi: 10.2337/db18-521-P
69. Jha JC, Banal C, Chow BS, Cooper ME, Jandeleit-Dahm K. Diabetes and kidney disease: role of oxidative stress. Antioxid Redox Signal (2016) 25:657–84. doi: 10.1089/ars.2016.6664
70. Kroemer G, Mariño G, Levine B. Autophagy and the integrated stress response. Mol Cell (2010) 40:280–93. doi: 10.1016/j.molcel.2010.09.023
71. Sedeek M, Nasrallah R, Touyz RM, Hébert RL. NADPH oxidases, reactive oxygen species, and the kidney: friend and foe. J Am Soc Nephrol (2013) 24:1512–8. doi: 10.1681/asn.2012111112
72. Duni A, Liakopoulos V, Roumeliotis S, Peschos D, Dounousi E. Oxidative stress in the pathogenesis and evolution of chronic kidney disease: untangling Ariadne's thread. Int J Mol Sci (2019) 20. doi: 10.3390/ijms20153711
73. Mora-Fernández C, Domínguez-Pimentel V, de Fuentes MM, Górriz JL, Martínez-Castelao A, Navarro-González JF. Diabetic kidney disease: from physiology to therapeutics. J Physiol (2014) 592:3997–4012. doi: 10.1113/jphysiol.2014.272328
74. Wysocki J, Goodling A, Burgaya M, Whitlock K, Ruzinski J, Batlle D, et al. Urine RAS components in mice and people with type 1 diabetes and chronic kidney disease. Am J Physiol Renal Physiol (2017) 313:F487–f494. doi: 10.1152/ajprenal.00074.2017
75. Huang X, Hamza SM, Zhuang W, Cupples WA, Braam B. Angiotensin II and the renal hemodynamic response to an isolated increased renal venous pressure in rats. Front Physiol (2021) 12:753355. doi: 10.3389/fphys.2021.753355
76. Alicic RZ, Rooney MT, Tuttle KR. Diabetic kidney disease: challenges, progress, and possibilities. Clin J Am Soc Nephrol (2017) 12:2032–45. doi: 10.2215/cjn.11491116
77. Tang SCW, Yiu WH. Innate immunity in diabetic kidney disease. Nat Rev Nephrol (2020) 16:206–22. doi: 10.1038/s41581-019-0234-4
78. Huang F, Wang Q, Guo F, Zhao Y, Ji L, An T, et al. FoxO1-mediated inhibition of STAT1 alleviates tubulointerstitial fibrosis and tubule apoptosis in diabetic kidney disease. EBioMedicine (2019) 48:491–504. doi: 10.1016/j.ebiom.2019.09.002
79. Jha JC, Dai A, Holterman CE, Cooper ME, Touyz RM, Kennedy CR, et al. Endothelial or vascular smooth muscle cell-specific expression of human NOX5 exacerbates renal inflammation, fibrosis and albuminuria in the Akita mouse. Diabetologia (2019) 62:1712–26. doi: 10.1007/s00125-019-4924-z
80. Lin J, Cheng A, Cheng K, Deng Q, Zhang S, Lan Z, et al. New insights into the mechanisms of pyroptosis and implications for diabetic kidney disease. Int J Mol Sci (2020) 21:7057. doi: 10.3390/ijms21197057
81. Wei PZ, Szeto CC. Mitochondrial dysfunction in diabetic kidney disease. Clin Chim Acta (2019) 496:108–16. doi: 10.1016/j.cca.2019.07.005
82. Yang XD, Yang YY. Ferroptosis as a novel therapeutic target for diabetes and its complications. Front Endocrinol (Lausanne) (2022) 13:853822. doi: 10.3389/fendo.2022.853822
83. Wu M, Zhang M, Zhang Y, Li Z, Li X, Liu Z, et al. Relationship between lysosomal dyshomeostasis and progression of diabetic kidney disease. Cell Death Dis (2021) 12:958. doi: 10.1038/s41419-021-04271-w
84. Kopp F, Mendell JT. Functional classification and experimental dissection of long noncoding RNAs. Cell (2018) 172:393–407. doi: 10.1016/j.cell.2018.01.011
85. Bridges MC, Daulagala AC, Kourtidis A. LNCcation: lncRNA localization and function. J Cell Biol (2021) 220. doi: 10.1083/jcb.202009045
86. Quinn JJ, Chang HY. Unique features of long non-coding RNA biogenesis and function. Nat Rev Genet (2016) 17:47–62. doi: 10.1038/nrg.2015.10
87. Wang Z, Zhang S, Li K. LncRNA NEAT1 induces autophagy through epigenetic regulation of autophagy-related gene expression in neuroglial cells. J Cell Physiol (2022) 237:824–32. doi: 10.1002/jcp.30556
88. Jiang Y, Zhu H, Chen H, Yu YC, Xu YT, Liu F, et al. Elevated Expression of lncRNA MEG3 Induces Endothelial Dysfunction on HUVECs of IVF Born Offspring via Epigenetic Regulation. Front Cardiovasc Med (2021) 8:717729. doi: 10.3389/fcvm.2021.717729
89. Jarroux J, Foretek D, Bertrand C, Gabriel M, Szachnowski U, Saci Z, et al. HOTAIR lncRNA promotes epithelial-mesenchymal transition by redistributing LSD1 at regulatory chromatin regions. EMBO Rep (2021) 22:e50193. doi: 10.15252/embr.202050193
90. Zhang Y, Huang YX, Wang DL, Yang B, Yan HY, Lin LH, et al. LncRNA DSCAM-AS1 interacts with YBX1 to promote cancer progression by forming a positive feedback loop that activates FOXA1 transcription network. Theranostics (2020) 10:10823–37. doi: 10.7150/thno.47830
91. Shi T, Guo D, Xu H, Su G, Chen J, Zhao Z, et al. HOTAIRM1, an enhancer lncRNA, promotes glioma proliferation by regulating long-range chromatin interactions within HOXA cluster genes. Mol Biol Rep (2020) 47:2723–33. doi: 10.1007/s11033-020-05371-0
92. Guo ZW, Xie C, Li K, Zhai XM, Cai GX, Yang XX, et al. SELER: a database of super-enhancer-associated lncRNA- directed transcriptional regulation in human cancers. Database (Oxford) (2019) 2019. doi: 10.1093/database/baz027
93. Zhou L, Jiang J, Huang Z, Jin P, Peng L, Luo M, et al. Hypoxia-induced lncRNA STEAP3-AS1 activates Wnt/β-catenin signaling to promote colorectal cancer progression by preventing m(6)A-mediated degradation of STEAP3 mRNA. Mol Cancer (2022) 21:168. doi: 10.1186/s12943-022-01638-1
94. Wang W, Zhao Z, Xu C, Li C, Ding C, Chen J, et al. LncRNA FAM83A-AS1 promotes lung adenocarcinoma progression by enhancing the pre-mRNA stability of FAM83A. Thorac Cancer (2021) 12:1495–502. doi: 10.1111/1759-7714.13928
95. Ghosh A, Pandey SP, Ansari AH, Sundar JS, Singh P, Khan Y, et al. Alternative splicing modulation mediated by G-quadruplex structures in MALAT1 lncRNA. Nucleic Acids Res (2022) 50:378–96. doi: 10.1093/nar/gkab1066
96. Feng Y, Ge Y, Wu M, Xie Y, Wang M, Chen Y, et al. Long Non−Coding RNAs Regulate Inflammation in Diabetic Peripheral Neuropathy by Acting as ceRNAs Targeting miR-146a-5p. Diabetes Metab Syndr Obes (2020) 13:413–22. doi: 10.2147/dmso.S242789
97. Wu M, Yang LZ, Chen LL. Long noncoding RNA and protein abundance in lncRNPs. Rna (2021) 27:1427–40. doi: 10.1261/rna.078971.121
98. Zhang J, Zhang R, Ye Y. Long non-coding RNA (LncRNA) SNHG7/ Eukaryotic translation initiation factor 4 gamma 2 (EIF4G2) involves in the Malignant events of ovarian cancer cells with paclitaxel resistant. Bioengineered (2021) 12:10541–52. doi: 10.1080/21655979.2021.1999555
99. Wang P, Xue Y, Han Y, Lin L, Wu C, Xu S, et al. The STAT3-binding long noncoding RNA lnc-DC controls human dendritic cell differentiation. Science (2014) 344:310–3. doi: 10.1126/science.1251456
100. Kato M, Natarajan R. Epigenetics and epigenomics in diabetic kidney disease and metabolic memory. Nat Rev Nephrol (2019) 15:327–45. doi: 10.1038/s41581-019-0135-6
101. Su PP, Liu DW, Zhou SJ, Chen H, Wu XM, Liu ZS. Down-regulation of Risa improves podocyte injury by enhancing autophagy in diabetic nephropathy. Mil Med Res (2022) 9:23. doi: 10.1186/s40779-022-00385-0
102. Li L, Long J, Mise K, Galvan DL, Overbeek PA, Tan L, et al. PGC1α is required for the renoprotective effect of lncRNA Tug1 in vivo and links Tug1 with urea cycle metabolites. Cell Rep (2021) 36:109510. doi: 10.1016/j.celrep.2021.109510
103. Deng Q, Wen R, Liu S, Chen X, Song S, Li X, et al. Increased long noncoding RNA maternally expressed gene 3 contributes to podocyte injury induced by high glucose through regulation of mitochondrial fission. Cell Death Dis (2020) 11:814. doi: 10.1038/s41419-020-03022-7
104. Liu DW, Zhang JH, Liu FX, Wang XT, Pan SK, Jiang DK, et al. Silencing of long noncoding RNA PVT1 inhibits podocyte damage and apoptosis in diabetic nephropathy by upregulating FOXA1. Exp Mol Med (2019) 51:1–15. doi: 10.1038/s12276-019-0259-6
105. Yang YL, Xue M, Jia YJ, Hu F, Zheng ZJ, Wang L, et al. Long noncoding RNA NEAT1 is involved in the protective effect of Klotho on renal tubular epithelial cells in diabetic kidney disease through the ERK1/2 signaling pathway. Exp Mol Med (2020) 52:266–80. doi: 10.1038/s12276-020-0381-5
106. Wang W, Jia YJ, Yang YL, Xue M, Zheng ZJ, Wang L, et al. LncRNA GAS5 exacerbates renal tubular epithelial fibrosis by acting as a competing endogenous RNA of miR-96-5p. BioMed Pharmacother (2020) 121:109411. doi: 10.1016/j.biopha.2019.109411
107. Sun SF, Tang PMK, Feng M, Xiao J, Huang XR, Li P, et al. Novel lncRNA Erbb4-IR Promotes Diabetic Kidney Injury in db/db Mice by Targeting miR-29b. Diabetes (2018) 67:731–44. doi: 10.2337/db17-0816
108. Gao J, Wang W, Wang F, Guo C. LncRNA-NR_033515 promotes proliferation, fibrogenesis and epithelial-to-mesenchymal transition by targeting miR-743b-5p in diabetic nephropathy. BioMed Pharmacother (2018) 106:543–52. doi: 10.1016/j.biopha.2018.06.104
109. Li Y, Zheng LL, Huang DG, Cao H, Gao YH, Fan ZC. LNCRNA CDKN2B-AS1 regulates mesangial cell proliferation and extracellular matrix accumulation via miR-424-5p/HMGA2 axis. BioMed Pharmacother (2020) 121:109622. doi: 10.1016/j.biopha.2019.109622
110. Nair AK, Traurig M, Sutherland JR, Muller YL, Grellinger ED, Saporito L, et al. Generation of Isogenic hiPSCs with Targeted Edits at Multiple Intronic SNPs to Study the Effects of the Type 2 Diabetes Associated KCNQ1 Locus in American Indians. Cells (2022) 11. doi: 10.3390/cells11091446
111. Xu J, Xiang P, Liu L, Sun J, Ye S. Metformin inhibits extracellular matrix accumulation, inflammation and proliferation of mesangial cells in diabetic nephropathy by regulating H19/miR-143-3p/TGF-β1 axis. J Pharm Pharmacol (2020) 72:1101–9. doi: 10.1111/jphp.13280
112. Okuyan HM, Dogan S, Terzi MY, Begen MA, Turgut FH. Association of serum lncRNA H19 expression with inflammatory and oxidative stress markers and routine biochemical parameters in chronic kidney disease. Clin Exp Nephrol (2021) 25:522–30. doi: 10.1007/s10157-021-02023-w
113. Sanchez-Alamo B, Shabaka A, Cachofeiro V, Cases-Corona C, Fernandez-Juarez G. Serum interleukin-6 levels predict kidney disease progression in diabetic nephropathy. Clin Nephrol (2022) 97:1–9. doi: 10.5414/cn110223
114. Shi S, Song L, Yu H, Feng S, He J, Liu Y, et al. Knockdown of lncRNA-H19 ameliorates kidney fibrosis in diabetic mice by suppressing miR-29a-mediated endMT. Front Pharmacol (2020) 11:586895. doi: 10.3389/fphar.2020.586895
115. Lin CL, Lee PH, Hsu YC, Lei CC, Ko JY, Chuang PC, et al. MicroRNA-29a promotion of nephrin acetylation ameliorates hyperglycemia-induced podocyte dysfunction. J Am Soc Nephrol (2014) 25:1698–709. doi: 10.1681/asn.2013050527
116. Xie H, Xue JD, Chao F, Jin YF, Fu Q. Long non-coding RNA-H19 antagonism protects against renal fibrosis. Oncotarget (2016) 7:51473–81. doi: 10.18632/oncotarget.10444
117. Wu Y, Zhao Y, Yang HZ, Wang YJ, Chen Y. HMGB1 regulates ferroptosis through Nrf2 pathway in mesangial cells in response to high glucose. Biosci Rep (2021) 41. doi: 10.1042/bsr20202924
118. Liu BH, Tu Y, Ni GX, Yan J, Yue L, Li ZL, et al. Total Flavones of Abelmoschus manihot Ameliorates Podocyte Pyroptosis and Injury in High Glucose Conditions by Targeting METTL3-Dependent m(6)A Modification-Mediated NLRP3-Inflammasome Activation and PTEN/PI3K/Akt Signaling. Front Pharmacol (2021) 12:667644. doi: 10.3389/fphar.2021.667644
119. Liu XQ, Jiang L, Li YY, Huang YB, Hu XR, Zhu W, et al. Wogonin protects glomerular podocytes by targeting Bcl-2-mediated autophagy and apoptosis in diabetic kidney disease. Acta Pharmacol Sin (2022) 43:96–110. doi: 10.1038/s41401-021-00721-5
120. Naguib M, Rashed LA. Serum level of the autophagy biomarker Beclin-1 in patients with diabetic kidney disease. Diabetes Res Clin Pract (2018) 143:56–61. doi: 10.1016/j.diabres.2018.06.022
121. Qiu J, Li X, He Y, Sun D, Li W, Xin Y. Distinct subgroup of the Ras family member 3 (DIRAS3) expression impairs metastasis and induces autophagy of gastric cancer cells in mice. J Cancer Res Clin Oncol (2018) 144:1869–86. doi: 10.1007/s00432-018-2708-3
122. Zeng J, Bao X. Tanshinone IIA attenuates high glucose-induced epithelial-to-mesenchymal transition in HK-2 cells through VDR/Wnt/β-catenin signaling pathway. Folia Histochem Cytobiol (2021) 59:259–70. doi: 10.5603/FHC.a2021.0025
123. Sultan HK, El-Ayat WM, AbouGhalia AH, Lasheen NN, Moustafa AS. Study of long non-coding RNA and mitochondrial dysfunction in diabetic rats. Tissue Cell (2021) 71:101516. doi: 10.1016/j.tice.2021.101516
124. Liu X, Li MH, Zhao YY, Xie YL, Yu X, Chen YJ, et al. LncRNA H19 deficiency protects against the structural damage of glomerular endothelium in diabetic nephropathy via Akt/eNOS pathway. Arch Physiol Biochem (2022) 1–10. doi: 10.1080/13813455.2022.2102655
125. Yang R, Xu S, Zhang X, Zheng X, Liu Y, Jiang C, et al. Cyclocarya paliurus triterpenoids attenuate glomerular endothelial injury in the diabetic rats via ROCK pathway. J Ethnopharmacol (2022) 291:115127. doi: 10.1016/j.jep.2022.115127
126. Veron D, Aggarwal PK, Li Q, Moeckel G, Kashgarian M, Tufro A. Podocyte VEGF-A knockdown induces diffuse glomerulosclerosis in diabetic and in eNOS knockout mice. Front Pharmacol (2021) 12:788886. doi: 10.3389/fphar.2021.788886
127. Cao T, Jiang Y, Li D, Sun X, Zhang Y, Qin L, et al. H19/TET1 axis promotes TGF-β signaling linked to endothelial-to-mesenchymal transition. FASEB J (2020) 34:8625–40. doi: 10.1096/fj.202000073RRRRR
128. Xu H, Ding Y, Yang X. Overexpression of long noncoding RNA H19 downregulates miR-140-5p and activates PI3K/AKT signaling pathway to promote invasion, migration and epithelial-mesenchymal transition of ovarian cancer cells. BioMed Res Int (2021) 2021:6619730. doi: 10.1155/2021/6619730
129. Yan L, Yang S, Yue CX, Wei XY, Peng W, Dong ZY, et al. Long noncoding RNA H19 acts as a miR-340-3p sponge to promote epithelial-mesenchymal transition by regulating YWHAZ expression in paclitaxel-resistant breast cancer cells. Environ Toxicol (2020) 35:1015–28. doi: 10.1002/tox.22938
130. Zhu J, Luo Z, Pan Y, Zheng W, Li W, Zhang Z, et al. H19/miR-148a/USP4 axis facilitates liver fibrosis by enhancing TGF-β signaling in both hepatic stellate cells and hepatocytes. J Cell Physiol (2019) 234:9698–710. doi: 10.1002/jcp.27656
131. Si H, Chen P, Li H, Wang X. Long non-coding RNA H19 regulates cell growth and metastasis via miR-138 in breast cancer. Am J Transl Res (2019) 11:3213–25. doi: 10.1007/s10719-021-10035-7
132. Zhou W, Feng Q, Cheng M, Zhang D, Jin J, Zhang S, et al. LncRNA H19 sponges miR-103-3p to promote the high phosphorus-induced osteoblast phenotypic transition of vascular smooth muscle cells by upregulating Runx2. Cell Signal (2022) 91:110220. doi: 10.1016/j.cellsig.2021.110220
133. Li XZ, Xiong ZC, Zhang SL, Hao QY, Liu ZY, Zhang HF, et al. Upregulated lncRNA H19 sponges miR-106a-5p and contributes to aldosterone-induced vascular calcification via activating the runx2-dependent pathway. Arterioscler Thromb Vasc Biol (2023) 43:1684–99. doi: 10.1161/atvbaha.123.319308
134. Zhang R, Pan T, Xiang Y, Zhang M, Xie H, Liang Z, et al. Curcumenol triggered ferroptosis in lung cancer cells via lncRNA H19/miR-19b-3p/FTH1 axis. Bioact Mater (2022) 13:23–36. doi: 10.1016/j.bioactmat.2021.11.013
135. Chen B, Wang H, Lv C, Mao C, Cui Y. Long non-coding RNA H19 protects against intracerebral hemorrhage injuries via regulating microRNA-106b-5p/acyl-CoA synthetase long chain family member 4 axis. Bioengineered (2021) 12:4004–15. doi: 10.1080/21655979.2021.1951070
136. Zhao Z, Wu J, Xu H, Zhou C, Han B, Zhu H, et al. XJB-5-131 inhibited ferroptosis in tubular epithelial cells after ischemia-reperfusion injury. Cell Death Dis (2020) 11:629. doi: 10.1038/s41419-020-02871-6
137. Zhang YY, Bao HL, Dong LX, Liu Y, Zhang GW, An FM. Silenced lncRNA H19 and up-regulated microRNA-129 accelerates viability and restrains apoptosis of PC12 cells induced by Aβ(25-35) in a cellular model of Alzheimer's disease. Cell Cycle (2021) 20:112–25. doi: 10.1080/15384101.2020.1863681
138. Ye Y, Feng Z, Tian S, Yang Y, Jia Y, Wang G, et al. HBO Alleviates Neural Stem Cell Pyroptosis via lncRNA-H19/miR-423-5p/NLRP3 Axis and Improves Neurogenesis after Oxygen Glucose Deprivation. Oxid Med Cell Longev (2022) 2022:9030771. doi: 10.1155/2022/9030771
139. Sun J, Mao S, Ji W. LncRNA H19 activates cell pyroptosis via the miR-22-3p/NLRP3 axis in pneumonia. Am J Transl Res (2021) 13:11384–98.
140. Wan P, Su W, Zhang Y, Li Z, Deng C, Li J, et al. LncRNA H19 initiates microglial pyroptosis and neuronal death in retinal ischemia/reperfusion injury. Cell Death Differ (2020) 27:176–91. doi: 10.1038/s41418-019-0351-4
141. Shan B, Li JY, Liu YJ, Tang XB, Zhou Z, Luo LX. LncRNA H19 Inhibits the Progression of Sepsis-Induced Myocardial Injury via Regulation of the miR-93-5p/SORBS2 Axis. Inflammation (2021) 44:344–57. doi: 10.1007/s10753-020-01340-8
142. Lv XW, Wang MJ, Qin QY, Lu P, Qin GW. 6-Gingerol relieves myocardial ischaemia/reperfusion injury by regulating lncRNA H19/miR-143/ATG7 signaling axis-mediated autophagy. Lab Invest (2021) 101:865–77. doi: 10.1038/s41374-021-00575-9
143. Wang J, Xie S, Yang J, Xiong H, Jia Y, Zhou Y, et al. The long noncoding RNA H19 promotes tamoxifen resistance in breast cancer via autophagy. J Hematol Oncol (2019) 12:81. doi: 10.1186/s13045-019-0747-0
144. Huang Y, Huang Y, Zhou Y, Cheng J, Wan C, Wang M, et al. Shensu IV prevents glomerular podocyte injury in nephrotic rats via promoting lncRNA H19/DIRAS3-mediated autophagy. Biosci Rep (2021) 41. doi: 10.1042/bsr20203362
145. Tang C, Livingston MJ, Liu Z, Dong Z. Autophagy in kidney homeostasis and disease. Nat Rev Nephrol (2020) 16:489–508. doi: 10.1038/s41581-020-0309-2
146. Keniry A, Oxley D, Monnier P, Kyba M, Dandolo L, Smits G, et al. The H19 lincRNA is a developmental reservoir of miR-675 that suppresses growth and Igf1r. Nat Cell Biol (2012) 14:659–65. doi: 10.1038/ncb2521
147. Zhang W, Zhou K, Zhang X, Wu C, Deng D, Yao Z. Roles of the H19/microRNA−675 axis in the proliferation and epithelial−mesenchymal transition of human cutaneous squamous cell carcinoma cells. Oncol Rep (2021) 45. doi: 10.3892/or.2021.7990
148. Xu Y, Liu Y, Li Z, Li H, Li X, Yan L, et al. Long non−coding RNA H19 is involved in sorafenib resistance in hepatocellular carcinoma by upregulating miR−675. Oncol Rep (2020) 44:165–73. doi: 10.3892/or.2020.7608
149. Peperstraete E, Lecerf C, Collette J, Vennin C, Raby L, Völkel P, et al. Enhancement of Breast Cancer Cell Aggressiveness by lncRNA H19 and its Mir-675 Derivative: Insight into Shared and Different Actions. Cancers (Basel) (2020) 12. doi: 10.3390/cancers12071730
150. Di Sanzo M, Chirillo R, Aversa I, Biamonte F, Santamaria G, Giovannone ED, et al. shRNA targeting of ferritin heavy chain activates H19/miR-675 axis in K562 cells. Gene (2018) 657:92–9. doi: 10.1016/j.gene.2018.03.027
151. Zhang Y, Huang W, Yuan Y, Li J, Wu J, Yu J, et al. Long non-coding RNA H19 promotes colorectal cancer metastasis via binding to hnRNPA2B1. J Exp Clin Cancer Res (2020) 39:141. doi: 10.1186/s13046-020-01619-6
152. Wang SH, Zhu XL, Wang F, Chen SX, Chen ZT, Qiu Q, et al. LncRNA H19 governs mitophagy and restores mitochondrial respiration in the heart through Pink1/Parkin signaling during obesity. Cell Death Dis (2021) 12:557. doi: 10.1038/s41419-021-03821-6
153. Bai RX, Tang ZY. Long non-coding RNA H19 regulates Bcl-2, Bax and phospholipid hydroperoxide glutathione peroxidase expression in spontaneous abortion. Exp Ther Med (2021) 21:41. doi: 10.3892/etm.2020.9473
154. Wang YH, Chang DY, Zhao MH, Chen M. Glutathione peroxidase 4 is a predictor of diabetic kidney disease progression in type 2 diabetes mellitus. Oxid Med Cell Longev (2022) 2022:2948248. doi: 10.1155/2022/2948248
155. Mao S, Huang H, Chen X. lncRNA H19 Aggravates Brain Injury in Rats following Experimental Intracerebral Hemorrhage via NF-κB Pathway. Comput Math Methods Med (2022) 2022:3017312. doi: 10.1155/2022/3017312
156. Liu J, Wang G, Zhao J, Liu X, Zhang K, Gong G, et al. LncRNA H19 promoted the epithelial to mesenchymal transition and metastasis in gastric cancer via activating wnt/β-catenin signaling. Dig Dis (2022) 40:436–47. doi: 10.1159/000518627
157. Chen W, Zai W, Fan J, Zhang X, Zeng X, Luan J, et al. Interleukin-22 drives a metabolic adaptive reprogramming to maintain mitochondrial fitness and treat liver injury. Theranostics (2020) 10:5879–94. doi: 10.7150/thno.43894
158. Liu S, Xu DS, Ma JL, Huang P, Wu D, Ren LQ. LncRNA H19 mitigates oxidized low-density lipoprotein induced pyroptosis via caspase-1 in raw 264.7 cells. Inflammation (2021) 44:2407–18. doi: 10.1007/s10753-021-01511-1
159. Tong X, Yu Q, Ankawi G, Pang B, Yang B, Yang H. Insights into the role of renal biopsy in patients with T2DM: A literature review of global renal biopsy results. Diabetes Ther (2020) 11:1983–99. doi: 10.1007/s13300-020-00888-w
160. Li Z, Liu Z, Lu H, Dai W, Chen J, He L. RvD1 attenuated susceptibility to ischemic AKI in diabetes by downregulating nuclear factor-κ B signal and inhibiting apoptosis. Front Physiol (2021) 12:651645. doi: 10.3389/fphys.2021.651645
161. Dong X, Cao R, Li Q, Yin L. The long noncoding RNA-H19 mediates the progression of fibrosis from acute kidney injury to chronic kidney disease by regulating the miR-196a/wnt/β-catenin signaling. Nephron (2022) 146:209–19. doi: 10.1159/000518756
162. Yuan Y, Li X, Chu Y, Ye G, Yang L, Dong Z. Long non-coding RNA H19 augments hypoxia/reoxygenation-induced renal tubular epithelial cell apoptosis and injury by the miR-130a/BCL2L11 pathway. Front Physiol (2021) 12:632398. doi: 10.3389/fphys.2021.632398
163. Haddad G, Kölling M, Wegmann UA, Dettling A, Seeger H, Schmitt R, et al. Renal AAV2-mediated overexpression of long non-coding RNA H19 attenuates ischemic acute kidney injury through sponging of microRNA-30a-5p. J Am Soc Nephrol (2021) 32:323–41. doi: 10.1681/asn.2020060775
164. Liu H, Ye T, Yang X, Liu J, Jiang K, Lu H, et al. H19 promote calcium oxalate nephrocalcinosis-induced renal tubular epithelial cell injury via a ceRNA pathway. EBioMedicine (2019) 50:366–78. doi: 10.1016/j.ebiom.2019.10.059
165. Liu HC, Zhu WY, Ren LY. LncRNA H19 inhibits proliferation and enhances apoptosis of nephroblastoma cells by regulating the miR-675/TGFBI axis. Eur Rev Med Pharmacol Sci (2022) 26:3800–6. doi: 10.26355/eurrev_202206_28947
166. Fukuzawa R, Breslow NE, Morison IM, Dwyer P, Kusafuka T, Kobayashi Y, et al. Epigenetic differences between Wilms' tumours in white and east-Asian children. Lancet (2004) 363:446–51. doi: 10.1016/s0140-6736(04)15491-3
167. Oda H, Kume H, Shimizu Y, Inoue T, Ishikawa T. Loss of imprinting of igf2 in renal-cell carcinomas. Int J Cancer (1998) 75:343–6. doi: 10.1002/(sici)1097-0215(19980130)75:3<343::aid-ijc3>3.0.co;2-2
168. Schuster AE, Schneider DT, Fritsch MK, Grundy P, Perlman EJ. Genetic and genetic expression analyses of clear cell sarcoma of the kidney. Lab Invest (2003) 83:1293–9. doi: 10.1097/01.lab.0000087850.69363.59
169. He L, Wang H, He P, Jiang Y, Ma F, Wang J, et al. Serum long noncoding RNA H19 and CKD progression in igA nephropathy. J Nephrol (2023) 36:397–406. doi: 10.1007/s40620-022-01536-1
170. Alrefai AA, Khader HF, Elbasuony HA, Elzorkany KM, Saleh AA. Evaluation of the expression levels of lncRNAs H19 and MEG3 in patients with type 2 diabetes mellitus. Mol Biol Rep (2023) 50:6075–85. doi: 10.1007/s11033-023-08569-0
Keywords: LncRNA H19, diabetic kidney disease (DKD), pathogenesis, pathological changes, kidney cells
Citation: Wu Q and Huang F (2023) LncRNA H19: a novel player in the regulation of diabetic kidney disease. Front. Endocrinol. 14:1238981. doi: 10.3389/fendo.2023.1238981
Received: 12 June 2023; Accepted: 16 October 2023;
Published: 27 October 2023.
Edited by:
Mohamed El-Sherbiny, Almaarefa University, Saudi ArabiaReviewed by:
Akira Sugawara, Tohoku University, JapanJiankang Fang, University of Pennsylvania, United States
Gurudeeban Selvaraj, Concordia University, Canada
Copyright © 2023 Wu and Huang. This is an open-access article distributed under the terms of the Creative Commons Attribution License (CC BY). The use, distribution or reproduction in other forums is permitted, provided the original author(s) and the copyright owner(s) are credited and that the original publication in this journal is cited, in accordance with accepted academic practice. No use, distribution or reproduction is permitted which does not comply with these terms.
*Correspondence: Fengjuan Huang, Zmh1YW5nNjY2QDEyNi5jb20=