- 1Institute of Immunology, Faculty of Medicine, RWTH Aachen University, Aachen, Germany
- 2Department of Hematology, Oncology, Hemostaseology and Stem Cell Transplantation, Faculty of Medicine, RWTH Aachen University, Aachen, Germany
During the current corona pandemic, new therapeutic options against this viral disease are urgently desired. Due to the rapid spread and immense number of affected individuals worldwide, cost-effective, globally available, and safe options with minimal side effects and simple application are extremely warranted. This review will therefore discuss the potential of zinc as preventive and therapeutic agent alone or in combination with other strategies, as zinc meets all the above described criteria. While a variety of data on the association of the individual zinc status with viral and respiratory tract infections are available, study evidence regarding COVID-19 is so far missing but can be assumed as was indicated by others and is detailed in this perspective, focusing on re-balancing of the immune response by zinc supplementation. Especially, the role of zinc in viral-induced vascular complications has barely been discussed, so far. Interestingly, most of the risk groups described for COVID-19 are at the same time groups that were associated with zinc deficiency. As zinc is essential to preserve natural tissue barriers such as the respiratory epithelium, preventing pathogen entry, for a balanced function of the immune system and the redox system, zinc deficiency can probably be added to the factors predisposing individuals to infection and detrimental progression of COVID-19. Finally, due to its direct antiviral properties, it can be assumed that zinc administration is beneficial for most of the population, especially those with suboptimal zinc status.
Introduction
The importance of the trace element zinc for the development and function of the immune system across all kinds of species has been proven in numerous studies (1–3). As zinc deficiency results in altered numbers and dysfunction of all immune cells, subjects with suboptimal zinc state have an increased risk for infectious diseases, autoimmune disorders, and cancer (3–6). In addition to malnutrition, risk groups for zinc deficiency include the elderly and patients with various inflammatory and autoimmune diseases, which will be discussed in detail later in the article (7, 8). As mild zinc deficiency is largely sub-clinical, it is unnoticed in most people. However, the World Health Organization (WHO) assumes that at least one third of the world population is affected by zinc deficiency (9). The fact that zinc deficiency is responsible for 16% of all deep respiratory infections world-wide (9) provides a first strong hint on a link of zinc deficiency with the risk of infection and severe progression of COVID-19 and suggests potential benefits of zinc supplementation.
The most common symptoms of COVID-19 are impaired smell and taste, fever, cough, sore throat, general weakness, pain as aching limbs, runny nose, and in some cases diarrhea (10). In the subsequent chapters, we will associate most of those symptoms with altered zinc homeostasis and explain how zinc might prevent or attenuate those symptoms, as summarized in Figure 1, and thus should be regarded as promising cost-effective, globally available therapeutic approach for COVID-19 patients, for which minimal to no side effects are known.
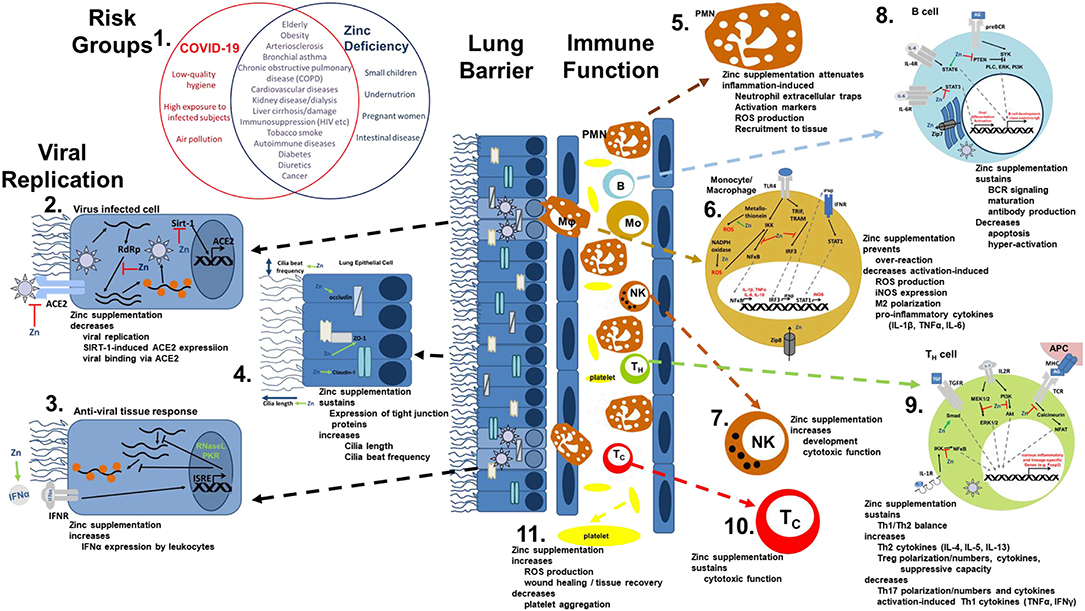
Figure 1. Viral mechanism of COVID-19 and how they might be opposed by zinc data. (1) There is an impressive intersection of known risk factors for zinc deficiency (blue circle) and the predisposition for a severe COVID-19 infection (red circle). (2,3) Zinc (Zn) supplementation might already prevent the viral entry and also suppresses its replication, while it supports the anti-viral response of the host cells. (4) As zinc is known to increase ciliary length and movements and also sustains tissue integrity, entrance of the virus is impeded. (5−10) The importance of zinc on the development and function of the immune cells is manifold. It should be underlined, that zinc's effects should not generally be described as activating or inhibiting, as zinc in various cases normalizes overshooting immune reactions and balances the ratios of the various immune cell types. Zinc thus prevents for example that high levels of inflammatory mediators including reactive oxygen and nitrogen species destroy the host tissue. (11) On first view it appears contradicting, that zinc increases activation induced production of reactive oxygen species in platelets, while it is generally considered as anti-oxidative. However, in case of platelets, up to a certain threshold, ROS production is essential, as it can prevent the formation of platelet aggregates. In summary, zinc therefore might be able to prevent vascular complications observed in COVID-19 patients. Details for each point can be found in the text. ACE2, angiotensin converting enzyme 2; AG, antigen; IFN, interferon; IFNR, interferon receptor; ISRE, interferon-sensitive response element; APC, antigen presenting cell; IKK, IκB kinase; IL, interleukin; iNOS, inducible nitric oxide synthase; IRF3, IFN regulatory factor 3; MHC, major histocompatibility complex; MEK1/2, mitogen-activated protein kinase kinase 1/2; NADPH oxidase, nicotinamide adenine dinucleotide phosphate oxidase; NFAT, nuclear factor of activated T-cells; NF-κB, nuclear factor kappa B; PKR, protein kinase R; Akt, protein kinase B; PI3K, phosphatidylinositol-3 kinases; ROS, reactive oxygen species; RdRP, RNA-dependent RNA polymerase; RNase L, ribonuclease L; Sirt-, Sirtuin 1; STAT, signal transducer and activators of transcription; TCR, T cell receptor; Tc, cytotoxic T cell; TH, helper T cell; TGF, transforming growth factor; TRAM, TRIF-related adaptor molecule; TRIF, TIR-domain-containing adapter-inducing interferon-β; TLR, toll-like receptor; TNF, tumor necrosis factor; Zip, Zrt- and Irt-like protein; ZO-1, zona occludens.
Zinc Protects the Human Body From Entering of the Virus
The entry of infectious agents into the human body is prevented by tissue barriers equipped with cilia and mucus, anti-microbial peptides like lysozymes and interferons. Regarding SARS-CoV2, the angiotensin-converting enzyme 2 (ACE2) and the cellular protease TMPRSS2 are the major mechanism for entering the cells (11).
a) Mucociliar clearance of viruses is affected by zinc
Infections with coronaviruses go along with damage of the ciliated epithelium and ciliary dyskinesia consecutively impairing the mucociliar clearance (12). It was shown that physiological concentrations of zinc increase ciliary beat frequency (13). Moreover, zinc supplementation in zinc deficient rats had a positive effect on the number and the length of bronchial cilia (14) (Figure 1.4). Improved ciliary clearance does not only improve the removal of virus particle, it also reduces the risk of secondary bacterial infections, as discussed later. Alterations of the extracellular matrix, as monitored by increased epidermal growth factor and proliferating cell nuclear antigen (PCNA) immunostaining of rat lungs during zinc deficiency have also been described (15).
b) Zinc is essential for preserving tissue barriers
Disturbances in the integrity of the respiratory epithelia facilitate the entry of the virus as well as co-infecting pathogens and can lead to pathogens entering the blood stream. An ex-vivo model of the chronic obstructive pulmonary disease (COPD) showed that decreasing zinc levels raised the leakage of the epithelium of the respiratory tract (16), while zinc supplementation improved lung integrity in a murine model of acute lung injury in vivo (17). Increased apoptosis and E-cadherin/beta-catenin proteolysis were amongst the underlying mechanisms (17–19). The expression of tight junction proteins like Claudin-1 and ZO-1 was found to be zinc-dependent, offering another explanation for zinc's positive effects on lung integrity (16). In addition, an inhibitory effect of zinc on LFA-1/ICAM-1 interaction weakened inflammation in the respiratory tract via reduction of leukocyte recruitment (20). Furthermore, high zinc levels improved the tolerance of the lung towards damage induced by mechanical ventilation (21) (Figure 1.4).
c) Zinc-dependent alterations in gene expression by pneumocytes could affect viral entering
ACE-2, mainly expressed on pneumocytes type 2, is a zinc-metalloenzyme. Zinc binds to its active center and is thus essential for its enzymatic activity. If zinc binding also affects the molecular structure of ACE-2 and thereby its binding affinity to the virus, remains to be tested (22, 23). However, this is likely as zinc is important for stabilizing protein structures and altering substrate affinity of various metalloproteins (24, 25). Finally, zinc homeostasis might affect ACE-2 expression, as zinc-dependent expression was reported for other zinc-metalloenzyme such as metallothionein and matrix metalloproteinases (26). This suggestion is strengthened by the finding that ACE-2 expression is regulated by Sirt-1 (27, 28). As zinc decreases Sirt-1 activity (27), it might decrease ACE-2 expression and thus viral entry into the cell (Figure 1.2).
A lack of adequate secretion of type I and type II interferons was reported for COVID-19 patients (29). For human interferon alpha (IFN-α) it was shown that zinc supplementation can reconstitute its expression by leukocytes and potentiates its anti-viral effect via JAK/STAT1 signaling as observed for rhinovirus-infected cells (30, 31). However, as it was suggested that SARS-CoV2 might take advantage of the interferon-dependent expression of ACE2, which was recently addressed by Ziegler et al. (32), the overall effects of zinc need to be carefully evaluated in future studies.
Zinc Directly Inhibits Viral Replication
As a virus, SARS-CoV2 is highly dependent on the metabolism of the host cell. Direct antiviral effects of zinc have been demonstrated in various cases, which was reviewed in great detail (33–37). Examples include coronaviridae, picornavirus, papilloma virus, metapneumovirus, rhinovirus, herpes simplex virus, varicella-zoster virus, respiratory syncytial virus, human immunodeficiency virus (HIV), and the hepatitis C virus (34, 35, 37–39). It was suggested that zinc can prevent fusion with the host membrane, decreases the viral polymerase function, impairs protein translation and processing, blocks viral particle release, and destabilizes the viral envelope (35, 37, 40). Low-dose zinc supplementation together with small concentrations of the zinc ionophores pyrithione or hinokitol decreased RNA synthesis in influenza, poliovirus, picornavirus, the equine arteritis virus, and SARS-CoV by directly inhibiting the RNA-dependent RNA polymerase of the virus (34, 41). There is evidence that zinc can enhance the effect of chloroquine, another known zinc ionophore, while zinc ionophores like epigallocatechin-gallate or quercetin remain to be tested (42–45). There are close similarities of SARS-CoV2 and other coronaviridae like SARS-CoV and Middle East respiratory syndrome-related coronavirus (MERS-CoV) (46). Also, the alcohol-aversive drug disulfiram can bind the papain-like proteases of SARS-CoV and MERS-CoV resulting in release of cysteine-bound zinc that results in protein destabilization (47). Detailed studies on zinc's effect specifically on SARS-CoV2 are highly required (Figure 1.3).
Zinc Balances the Immune Response During Infectious Diseases
One of the hallmarks of COVID-19 is an imbalanced immune response (48). Due to hyper-inflammation, immune products including pro-inflammatory cytokines like interleukin (IL)-6, C-reactive protein (CRP), tumor necrosis factor (TNF)α and IL-1β (summarized as cytokine storm or cytokine release syndrome), reactive oxygen, and nitrogen species in connection with the recruitment of high numbers of strongly activated immune cells to the lungs, the destruction of the tissue, permanent lung damage and death due to systemic inflammation, and organ failure are expected, while the anti-inflammatory response is insufficient (48–52). A high number of patients develop an acute respiratory distress syndrome (ARDS) accompanied by high alveolar leakage leading to alveolar and interstitial edema with severely limited oxygen exchange (53). Advanced SARS-CoV2 infections are characterized by a systemic involvement with organ complications and accompanying cytokine storm (52, 54).
There is no doubt on the anti-inflammatory and anti-oxidative properties of zinc and underlying mechanisms have been the focus of numerous studies (1–3, 6, 55–60). A detailed description of zinc metabolism in airway epithelium and during inflammation of the airways has been published by Zalewski et al. (61). On the other hand, zinc deficiency was associated with elevated levels of pro-inflammatory mediators, increased reactive oxygen species (ROS) levels and pre-disposing for severe progression of inflammatory diseases, especially those affecting the lung, often reversible by zinc supplementation (6, 17, 56, 62–66) (Figures 1.5,1.6). As one example, exposure to organic dust increased lung damage, inflammation and macrophage hyper-activation in animals with zinc deficiency, predisposing these animals to pulmonary fibrosis, while zinc supplementation 24 h before induction of acute lung injury significantly attenuated the inflammatory reaction and tissue damage (17, 67). Regarding systemic inflammatory diseases the number of studies showing benefits of especially preventive zinc supplementation is constantly increasing (17, 18, 58, 65, 68). Amongst the underlying mechanism, zinc's role as second messenger and importance in regulating intracellular signaling as detailed in Figure 1 were described as well as zinc's effects on the epigenome (56, 57, 69–74).
Furthermore, leukocytosis with neutrophilia and lymphopenia, especially affecting CD8+ T cells, were associated with poor prognosis of COVID-19 and the recovery of lymphocyte counts lead to clinical recovery (75, 76). Similar changes in lymphopoiesis and myelopoiesis have been described in zinc deficient rodents, which were normalized when zinc was supplemented (17, 19). Circulating and lung-resident T cells from COVID-19 patients showed increased expression of markers for T cell exhaustion like Tim-3 and PD-1 (77). The extent of these changes had an impact on the patient's prognosis (50). During the past decades, an immense literature was generated on the need of zinc for lymphocyte development and function and that zinc supplementation (6, 19, 63, 64, 78, 79) can reverse lymphopenia. Enumerating all findings and underlying mechanisms is beyond the scope of this article, and a lot of aspects have been discussed in related publications (36) but as one of the many key roles of zinc in the context of T cell function, zinc is indispensable in the signal cascade of the T cell receptor and IL-2 as a second messenger (78, 80) (Figure 1.9). The B cell compartment also strongly benefits from a balanced zinc homeostasis, as zinc is required for B cell maturation and function (72, 81) (Figure 1.8). Also important to mention, but neglected by previous related articles, is that there is evidence (82, 83) that SARS-CoV2 can directly infect T cells as well as B cells and impair their cell specific function. This could explain the impact of SARS-CoV2 infection on lymphoid tissues like the human spleen and lymph nodes (84). However, as data are limited to in vitro experiments, this needs to be verified in vivo as well as if zinc affects the virus-induced changes in T and B cells.
Additionally, granulocytes play a vital role during the inflammation-induced destruction of the lung (85). Recent evidence suggests that lipopolysaccharide-induced hyper-activation, recruitment and formation of neutrophil extracellular traps are attenuated by zinc supplementation in vivo and that cytokine expression, phagocytosis and burst, chemotaxis and degranulation, and intracellular signaling are zinc regulated (17, 86, 87) (Figure 1.5). Important defense mechanisms of the innate immunity include the toll like receptors. For instance, in silico data suggest that toll-like receptor (TLR)-4 can potentially recognize outer components of SARS-CoV2's like the viral spikes (88), while intracellular receptors including TLR3, TLR7/8, and TLR9 can recognize viral dsRNA, ssRNA, and unmethylated CpG DNA respectively (89–92). Intranasal pretreatment with a TLR3 agonist and, to a lesser extent, with TLR9, TLR7/8, or TLR4 agonists, provided a high level of protection against infections by SARS coronavirus and influence virus in mice, suggesting that TLR signaling can induce protective antiviral immunity (93). This might be a completely novel approach to consider regarding COVID-19 as well. Zinc is an essential regulator in TLR-4- and TLR-3-induced signaling in innate immune cells (94). Thus, zinc deficiency potentially disturbs the innate immune response toward SARS-CoV2, enabling the virus to easily spread throughout the host without an adequate immune response (Figure 1.6).
Clinical improvement of COVID-19 patients was correlated to an increase of CD14+ monocytes and NK cells in the recovery phase (48). For a physiological inflammatory response and phagocytic activity macrophages need sufficient intracellular zinc levels (1). In addition, for NK cells and cytotoxic T cells it was shown that zinc supplementation increased their cytotoxicity toward target cells (1, 2, 95) (Figures 1.7,1.10).
In summary zinc's (re-)balancing power regarding immune cell numbers and functions might be highly beneficial in regard to therapy of COVID-19.
Zinc Supplementation in Respiratory Infections
Our suggested benefits of zinc supplementation to prevent and treat COVID-19 are supported by a row of successful supplementation studies focusing on respiratory tract infection, of which we listed some selected publications in Table 1. In most cases, prophylactic zinc supplementation was more effective than therapeutic proceedings (106–108, 111). Up to 30% of the everyday respiratory infections, briefly named “common cold,” are due to infections with coronaviruses (112). Studies showed reduced symptom severity, reduced frequency, and duration of the common cold after zinc administration (99, 100, 113, 114) depending on dosage, zinc compound and the start time after initial symptoms (115). Most importantly, zinc supplementation of children revealed significant benefits in various studies (96, 106) and reduced 15% pneumonia-specific morality and 19% of pneumonia morbidity in developing countries (116).
Risk Groups and Symptoms of COVID-19 and Zinc Deficiency Reveal a Large Overlap
As illustrated in Figure 1.1, the intersection between risk groups of COVID-19 and zinc deficiency is impressive. In patients with chronic obstructive pulmonary disease (COPD), bronchial asthma, cardiovascular diseases, autoimmune diseases, kidney diseases, dialysis, obesity, diabetes, cancer, atherosclerosis, liver cirrhosis, immunosuppression, and known liver damage low serum zinc levels are regularly observed (4, 117). At the same time, these groups are particular at risk for COVID-19 (10, 51, 118, 119). For example 57.5% elderly and nursing home residents in the U.S., for which high incidence of respiratory tract infections is described, showed significantly decreased zinc intake levels and are considered subjects with high risk regarding COVID-19 (120). Moreover, other studies showed that serum zinc levels were inversely correlated with pneumonia and cystic fibrosis (121, 122). On the other hand, zinc supplementation was able to reconstitute immune function in elderly and zinc deficient individuals (107, 123), which remains to be addressed for SARS-CoV2 infections (36). In this regard, the low response of older patients with low serum zinc to a 23-valent pneumococcal polysaccharide vaccination compared to those with higher zinc level (124), should be mentioned. However, zinc's role in the response to vaccination is generally discussed controversially and no data are available for vaccination against any corona virus.
Several studies indicate that there is an association between chemosensory dysfunctions and COVID-19 (125–133). Smell or taste is largely decreased, which might be a good disease biomarker (133). It was suggested that this might either be due to direct destruction of sensory cells by the virus, as ACE-2 is highly expressed by the oral mucosa, or by viral entry into the brain and neuronal pathologies as was described for other SARS-CoV (133, 134). Zinc deficiency was related to significantly reduced taste sensitivity and impaired saliva secretion in humans and animals, which might involve zinc's importance for the action of carbonic anhydrase (135–140). Results from supplementation studies largely describe improvements in chemosensory functions (140, 141), but some studies did not find any effects (142) or even more severe disturbances when very high zinc concentrations were used (143). This is possibly due to investigating olfactory diseases of various origins, the lack of controlled trials and inclusion of observable studies. Thus, the benefits of zinc supplementation alone or in combination with common medical strategies should be tested for taste and smell diseases according to the available guidelines (144).
About 50% of patients that died of COVID-19 had bacterial or fungal co-infections (145), underlining the importance of sustaining the immune function by a sufficient zinc supply (1, 2, 36). In animal experiments it was shown that zinc restriction made mice highly susceptible to bacterial infection with streptococcus pneumoniae (146). As mentioned earlier, marginal zinc deficiency affects one third of the worldwide population and most subjects with COVID-19 are at risk of zinc deficiency (Figure 1). During physiological inflammatory responses, zinc is additionally redistributed to the tissues, resulting in serum hypozincemia (1, 65). In combination with the pre-existing suboptimal zinc supply, this will decrease serum zinc levels to critically low values and thereby significantly increase the susceptibility for co-infections with detrimental progression. In critically ill patients persistent low serum zinc was associated with recurrent sepsis and serum zinc levels were inversely correlated with mortality from sepsis (62), underlining the potential benefits of monitoring the zinc status of the patients and implementing zinc supplementation into therapy of COVID-19.
Vascular complications resulting from atherosclerosis development, microangiopathic organ failure, and venous thromboembolism were found as a major cause of death in COVID-19 patients (147–149), suggesting an important role of disease-induced coagulopathy, which, however, needs further investigation. Zinc influences thrombocyte aggregation and coagulation (150). Recently, a functional association between zinc and ROS production in platelets was described, indicating that zinc could decrease thrombus formation in a clinical context (151). Complications of SARS-CoV2 infections also include tissue damage affecting the gastrointestinal system (152), the liver (153), heart (154), nervous system (155), kidneys (156), blood vessels (149), and the skin (157). In this regard it should be mentioned that a balanced zinc homeostasis is essential for wound healing and tissue recovery after mechanical and inflammation-mediated damage (158, 159), adding more potential benefits of zinc supplementation of COVID-19 patients (Figure 1.11).
Conclusion
In this perspective, we reviewed the most important literature on the role of zinc homeostasis during viral infections, focusing on the potential benefits of zinc supplementation to prevent and treat SARS-CoV2 infections. Although data specifically on SARS-CoV2 are unfortunately still pending and randomized controlled studies have not been conducted, the enumerated evidence from the literature strongly suggests great benefits of zinc supplementation. Zinc supplementation improves the mucociliary clearance, strengthens the integrity of the epithelium, decreases viral replication, preserves antiviral immunity, attenuates the risk of hyper-inflammation, supports anti-oxidative effects and thus reduces lung damage and minimized secondary infections. Especially older subjects, patients with chronic diseases and most of the remaining COVID-19 risk groups would most likely benefit. Although studies are needed testing the effect of zinc as therapeutic option for established disease, preventive supplementation of subjects from risk groups should begin now, as zinc is a cost-efficient, globally available and simple to use option with little to no side effects. The first clinical trials on zinc supplementation alone and in combination with other drugs such as chloroquine have been registered (124, 160–162). Thus, first results and treatment regimens regarding zinc supplementation for COVID-19 risk groups and patients can be anticipated soon.
Data Availability Statement
The original contributions presented in the study are included in the article/supplementary material, further inquiries can be directed to the corresponding author/s.
Author's Note
LR is a member of ZINC Net.
Author Contributions
IW, BR, and LR drafted and corrected the text, table, and figure. All authors contributed to the article and approved the submitted version.
Conflict of Interest
The authors declare that the research was conducted in the absence of any commercial or financial relationships that could be construed as a potential conflict of interest.
References
1. Wessels I, Maywald M, Rink L. Zinc as a gatekeeper of immune function. Nutrients. (2017) 9:1286. doi: 10.3390/nu9121286
2. Gammoh NZ, Rink L. Zinc in infection and inflammation. Nutrients. (2017) 9:624. doi: 10.20944/preprints201705.0176.v1
3. Roohani N, Hurrell R, Kelishadi R, Schulin R. Zinc and its importance for human health: an integrative review. J Res Med Sci. (2013) 18:144–57.
4. Wessels I, Rink L. Micronutrients in autoimmune diseases: possible therapeutic benefits of zinc and vitamin D. J Nutr Biochem. (2020) 77:108240. doi: 10.1016/j.jnutbio.2019.108240
5. Haase H, Schomburg L. You'd better zinc-trace element homeostasis in infection and inflammation. Nutrients. (2019) 11:2078. doi: 10.3390/nu11092078
6. Prasad AS. Effects of zinc deficiency on Th1 and Th2 cytokine shifts. J Infect Dis. (2000) 182(Suppl. 1):S62–8. doi: 10.1086/315916
7. Suita S, Ikeda K, Nagasaki A, Hayashida Y. Zinc deficiency during total parenteral nutrition in childhood. J Pediatric Surg. (1978) 13:5–9. doi: 10.1016/S0022-3468(78)80202-4
8. Hotz C, Brown KH. Identifying populations at risk of zinc deficiency: the use of supplementation trials. Nutr Rev. (2001) 59:80–4. doi: 10.1111/j.1753-4887.2001.tb06992.x
9. World Health Organization. The World Health report 2002. Midwifery. (2003) 19:72–3. doi: 10.1054/midw.2002.0343
10. Eurosurveillance ET. Updated rapid risk assessment from ECDC on coronavirus disease 2019 (COVID-19) pandemic: increased transmission in the EU/EEA and the UK. Euro Surveill. (2020) 25:2003121. doi: 10.2807/1560-7917.ES.2020.25.10.2003121
11. Hoffmann M, Kleine-Weber H, Krüger N, Müller M, Drosten C, Pöhlmann S. The novel coronavirus 2019 (2019-nCoV) uses the SARS-coronavirus receptor ACE2 and the cellular protease TMPRSS2 for entry into target cells. bioRxiv. (2020). doi: 10.1101/2020.01.31.929042. [Epub ahead of print].
12. Chilvers MA, McKean M, Rutman A, Myint BS, Silverman M, O'Callaghan C. The effects of coronavirus on human nasal ciliated respiratory epithelium. Eur Respir J. (2001) 18:965–70. doi: 10.1183/09031936.01.00093001
13. Woodworth BA, Zhang S, Tamashiro E, Bhargave G, Palmer JN, Cohen NA. Zinc increases ciliary beat frequency in a calcium-dependent manner. Am J Rhinol Allergy. (2010) 24:6–10. doi: 10.2500/ajra.2010.24.3379
14. Darma A, Athiyyah AF, Ranuh RG, Merbawani W, Setyoningrum RA, Hidajat B, et al. Zinc supplementation effect on the bronchial cilia length, the number of cilia, and the number of intact bronchial cell in zinc deficiency rats. Indones Biomed J. (2020) 12:78–84. doi: 10.18585/inabj.v12i1.998
15. Biaggio VS, Salvetti NR, Pérez Chaca MV, Valdez SR, Ortega HH, Gimenez MS, et al. Alterations of the extracellular matrix of lung during zinc deficiency. Br J Nutr. (2012) 108:62–70. doi: 10.1017/S0007114511005290
16. Roscioli E, Jersmann HP, Lester S, Badiei A, Fon A, Zalewski P, et al. Zinc deficiency as a codeterminant for airway epithelial barrier dysfunction in an ex vivo model of COPD. Int J Chron Obstruct Pulmon Dis. (2017) 12:3503–10. doi: 10.2147/COPD.S149589
17. Wessels I, Pupke JT, Trotha K-T von, Gombert A, Himmelsbach A, Fischer HJ, et al. Zinc supplementation ameliorates lung injury by reducing neutrophil recruitment and activity. Thorax. (2020) 75:253–61. doi: 10.1136/thoraxjnl-2019-213357
18. Bao S, Knoell DL. Zinc modulates cytokine-induced lung epithelial cell barrier permeability. Am J Physiol Lung Cell Mol Physiol. (2006) 291:L1132–41. doi: 10.1152/ajplung.00207.2006
19. Fraker PJ, King LE. Reprogramming of the immune system during zinc deficiency. Annu Rev Nutr. (2004) 24:277–98. doi: 10.1146/annurev.nutr.24.012003.132454
20. Novick SG, Godfrey JC, Pollack RL, Wilder HR. Zinc-induced suppression of inflammation in the respiratory tract, caused by infection with human rhinovirus and other irritants. Med Hypotheses. (1997) 49:347–57. doi: 10.1016/S0306-9877(97)90201-2
21. Boudreault F, Pinilla-Vera M, Englert JA, Kho AT, Isabelle C, Arciniegas AJ, et al. Zinc deficiency primes the lung for ventilator-induced injury. JCI Insight. (2017) 2:e86507. doi: 10.1172/jci.insight.86507
22. Speth R, Carrera E, Jean-Baptiste M, Joachim A, Linares A. Concentration-dependent effects of zinc on angiotensin-converting enzyme-2 activity (1067.4). FASEB J. (2014) 28(1_supplement):1067.4.
23. Reeves PG, O'Dell BL. Effects of dietary zinc deprivation on the activity of angiotensin-converting enzyme in serum of rats and guinea pigs. J Nutr. (1986) 116:128–34. doi: 10.1093/jn/116.1.128
24. Christianson DW, Alexander RS. Carboxylate-histidine-zinc interactions in protein structure and function. J. Am. Chem. Soc. (1989) 111:6412–9. doi: 10.1021/ja00198a065
25. Cox E. Zinc-dependent protein folding. Curr Opin Chem Biol. (2000) 4:162–5. doi: 10.1016/S1367-5931(99)00070-8
26. Cao J-W, Duan S-Y, Zhang H-X, Chen Y, Guo M. Zinc deficiency promoted fibrosis via ROS and TIMP/MMPs in the myocardium of mice. Biol Trace Elem Res. (2019) 196:145–52. doi: 10.1007/s12011-019-01902-4
27. Rosenkranz E, Metz CH, Maywald M, Hilgers R-D, Weßels I, Senff T, et al. Zinc supplementation induces regulatory T cells by inhibition of Sirt-1 deacetylase in mixed lymphocyte cultures. Mol Nutr Food Res. (2016) 60:661–71. doi: 10.1002/mnfr.201500524
28. Patel VB, Zhong J-C, Grant MB, Oudit GY. Role of the ACE2/Angiotensin 1-7 axis of the renin-angiotensin system in heart failure. Circ Res. (2016) 118:1313–26. doi: 10.1161/CIRCRESAHA.116.307708
29. Blanco-Melo D, Nilsson-Payant BE, Liu W-C, Møller R, Panis M, Sachs D, et al. SARS-CoV-2 launches a unique transcriptional signature from in vitro, ex vivo, and in vivo systems. bioRxiv. (2020). doi: 10.1101/2020.03.24.004655. [Epub ahead of print].
30. Berg K, Bolt G, Andersen H, Owen TC. Zinc potentiates the antiviral action of human IFN-alpha tenfold. J Interferon Cytokine Res. (2001) 21:471–4. doi: 10.1089/10799900152434330
31. Cakman I, Kirchner H, Rink L. Zinc supplementation reconstitutes the production of interferon-alpha by leukocytes from elderly persons. J Interferon Cytokine Res. (1997) 17:469–72. doi: 10.1089/jir.1997.17.469
32. Ziegler CGK, Allon SJ, Nyquist SK, Mbano IM, Miao VN, Tzouanas CN, et al. SARS-CoV-2 receptor ACE2 is an interferon-stimulated gene in human airway epithelial cells and is detected in specific cell subsets across tissues. Cell. (2020) 181:1016–35.e19. doi: 10.1016/j.cell.2020.04.035
33. Ishida T. Review on the role of Zn2+ ions in viral pathogenesis and the effect of Zn2+ Ions for host cell-virus growth inhibition. AJBSR. (2019) 2:28–37. doi: 10.34297/AJBSR.2019.02.000566
34. Krenn BM, Gaudernak E, Holzer B, Lanke K, van Kuppeveld FJ, Seipelt J. Antiviral activity of the zinc ionophores pyrithione and hinokitiol against picornavirus infections. J Virol. (2009) 83:58–64. doi: 10.1128/JVI.01543-08
35. Read SA, Obeid S, Ahlenstiel C, Ahlenstiel G. The role of zinc in antiviral immunity. Adv Nutr. (2019) 10:696–710. doi: 10.1093/advances/nmz013
36. Skalny AV, Rink L, Ajsuvakova OP, Aschner M, Gritsenko VA, Alekseenko SI, et al. Zinc and respiratory tract infections: perspectives for COVID19 (Review). Int J Mol Med. (2020) 46:17–26. doi: 10.3892/ijmm.2020.4575
37. Suara RO, Crowe JE. Effect of zinc salts on respiratory syncytial virus replication. Antimicrob Agents Chemother. (2004) 48:783–90. doi: 10.1128/AAC.48.3.783-790.2004
38. Cai H, Zhang Y, Ma Y, Sun J, Liang X, Li J. Zinc binding activity of human metapneumovirus M2-1 protein is indispensable for viral replication and pathogenesis in vivo. J Virol. (2015) 89:6391–405. doi: 10.1128/JVI.03488-14
39. Lazarczyk M, Favre M. Role of Zn2+ ions in host-virus interactions. J Virol. (2008) 82:11486–94. doi: 10.1128/JVI.01314-08
40. Kümel G, Schrader S, Zentgraf H, Daus H, Brendel M. The mechanism of the antiherpetic activity of zinc sulphate. J Gen Virol. (1990) 71:2989–97. doi: 10.1099/0022-1317-71-12-2989
41. te Velthuis AJ, van den Worm SH, Sims AC, Baric RS, Snijder EJ, van Hemert MJ. Zn(2+) inhibits coronavirus and arterivirus RNA polymerase activity in vitro and zinc ionophores block the replication of these viruses in cell culture. PLoS Pathog. (2010) 6:e1001176. doi: 10.1371/journal.ppat.1001176
42. Shittu MO, Afolami OI. Improving the efficacy of chloroquine and hydroxychloroquine against SARS-CoV-2 may require zinc additives - a better synergy for future COVID-19 clinical trials. Infez Med. (2020) 28:192–7.
43. Dabbagh-Bazarbachi H, Clergeaud G, Quesada IM, Ortiz M, O'Sullivan CK, Fernández-Larrea JB. Zinc ionophore activity of quercetin and epigallocatechin-gallate: from Hepa 1-6 cells to a liposome model. J Agric Food Chem. (2014) 62:8085–93. doi: 10.1021/jf5014633
44. Wang M, Cao R, Zhang L, Yang X, Liu J, Xu M, et al. Remdesivir and chloroquine effectively inhibit the recently emerged novel coronavirus (2019-nCoV) in vitro. Cell Res. (2020) 30:269–71. doi: 10.1038/s41422-020-0282-0
45. Xue J, Moyer A, Peng B, Wu J, Hannafon BN, Ding W-Q. Chloroquine is a zinc ionophore. PLoS ONE. (2014) 9:e109180. doi: 10.1371/journal.pone.0109180
46. Liu J, Zheng X, Tong Q, Li W, Wang B, Sutter K, et al. Overlapping and discrete aspects of the pathology and pathogenesis of the emerging human pathogenic coronaviruses SARS-CoV, MERS-CoV, and 2019-nCoV. J Med Virol. (2020) 92:491–4. doi: 10.1002/jmv.25709
47. Lin M-H, Moses DC, Hsieh C-H, Cheng S-C, Chen Y-H, Sun C-Y, et al. Disulfiram can inhibit MERS and SARS coronavirus papain-like proteases via different modes. Antiviral Res. (2018) 150:155–63. doi: 10.1016/j.antiviral.2017.12.015
48. Wen W, Su W, Tang H, Le W, Zhang X, Zheng Y, et al. Immune cell profiling of COVID-19 patients in the recovery stage by single-cell sequencing. Cell Discov. (2020) 6:31. doi: 10.1038/s41421-020-00187-5
49. Cheng ZJ, Shan J. 2019 Novel coronavirus: where we are and what we know. Infection. (2020) 48:155–63. doi: 10.1007/s15010-020-01401-y
50. Chen G, Di Wu, Guo W, Cao Y, Huang D, Wang H, et al. Clinical and immunological features of severe and moderate coronavirus disease 2019. J Clin Invest. (2020) 130:2620–9. doi: 10.1172/JCI137244
51. Guan W-J, Ni Z-Y, Hu Y, Liang W-H, Ou C-Q, He J-X, et al. Clinical characteristics of coronavirus disease 2019 in China. N Engl J Med. (2020) 382:1708–20. doi: 10.1056/NEJMoa2002032
52. Mehta P, McAuley DF, Brown M, Sanchez E, Tattersall RS, Manson JJ. COVID-19: consider cytokine storm syndromes and immunosuppression. Lancet. (2020) 395:1033–4. doi: 10.1016/S0140-6736(20)30628-0
53. Matthay MA, Zemans RL, Zimmerman GA, Arabi YM, Beitler JR, Mercat A, et al. Acute respiratory distress syndrome. Nat Rev Dis Primers. (2019) 5:18. doi: 10.1038/s41572-019-0069-0
54. Wichmann D, Sperhake J-P, Lütgehetmann M, Steurer S, Edler C, Heinemann A, et al. Autopsy findings and venous thromboembolism in patients with COVID-19: a prospective cohort study. Ann Intern Med. (2020). doi: 10.7326/M20-2003. [Epub ahead of print].
55. Beck FW, Prasad AS, Kaplan J, Fitzgerald JT, Brewer GJ. Changes in cytokine production and T cell subpopulations in experimentally induced zinc-deficient humans. Am J Physiol. (1997) 272:E1002–7. doi: 10.1152/ajpendo.1997.272.6.E1002
56. Wessels I, Haase H, Engelhardt G, Rink L, Uciechowski P. Zinc deficiency induces production of the proinflammatory cytokines IL-1β and TNFα in promyeloid cells via epigenetic and redox-dependent mechanisms. J Nutr Biochem. (2013) 24:289–97. doi: 10.1016/j.jnutbio.2012.06.007
57. Liu M-J, Bao S, Gálvez-Peralta M, Pyle CJ, Rudawsky AC, Pavlovicz RE, et al. ZIP8 regulates host defense through zinc-mediated inhibition of NF-κB. Cell Rep. (2013) 3:386–400. doi: 10.1016/j.celrep.2013.01.009
58. Ganatra HA, Varisco BM, Harmon K, Lahni P, Opoka A, Wong HR. Zinc supplementation leads to immune modulation and improved survival in a juvenile model of murine sepsis. Innate Immun. (2017) 23:67–76. doi: 10.1177/1753425916677073
59. Choi S, Liu X, Pan Z. Zinc deficiency and cellular oxidative stress: prognostic implications in cardiovascular diseases. Acta Pharmacol Sin. (2018) 39:1120–32. doi: 10.1038/aps.2018.25
60. Prasad AS, Beck FW, Bao B, Fitzgerald JT, Snell DC, Steinberg JD, et al. Zinc supplementation decreases incidence of infections in the elderly: effect of zinc on generation of cytokines and oxidative stress. Am J Clin Nutr. (2007) 85:837–44. doi: 10.1093/ajcn/85.3.837
61. Zalewski PD, Truong-Tran AQ, Grosser D, Jayaram L, Murgia C, Ruffin RE. Zinc metabolism in airway epithelium and airway inflammation: basic mechanisms and clinical targets. a review. Pharmacol Ther. (2005) 105:127–49. doi: 10.1016/j.pharmthera.2004.09.004
62. Hoeger J, Simon T-P, Beeker T, Marx G, Haase H, Schuerholz T. Persistent low serum zinc is associated with recurrent sepsis in critically ill patients - a pilot study. PLoS ONE. (2017) 12:e0176069. doi: 10.1371/journal.pone.0176069
63. Ibs K-H, Rink L. Zinc-altered immune function. J Nutr. (2003) 133:1452S−6S. doi: 10.1093/jn/133.5.1452S
64. Kahmann L, Uciechowski P, Warmuth S, Plümäkers B, Gressner AM, Malavolta M, et al. Zinc supplementation in the elderly reduces spontaneous inflammatory cytokine release and restores T cell functions. Rejuvenation Res. (2008) 11:227–37. doi: 10.1089/rej.2007.0613
65. Wessels I, Cousins RJ. Zinc dyshomeostasis during polymicrobial sepsis in mice involves zinc transporter Zip14 and can be overcome by zinc supplementation. Am J Physiol Gastrointest Liver Physiol. (2015) 309:G768–78. doi: 10.1152/ajpgi.00179.2015
66. Besecker BY, Exline MC, Hollyfield J, Phillips G, Disilvestro RA, Wewers MD, et al. A comparison of zinc metabolism, inflammation, and disease severity in critically ill infected and noninfected adults early after intensive care unit admission. Am J Clin Nutr. (2011) 93:1356–64. doi: 10.3945/ajcn.110.008417
67. Knoell DL, Smith DA, Sapkota M, Heires AJ, Hanson CK, Smith LM, et al. Insufficient zinc intake enhances lung inflammation in response to agricultural organic dust exposure. J Nutr Biochem. (2019) 70:56–64. doi: 10.1016/j.jnutbio.2019.04.007
68. Bao S, Liu M-J, Lee B, Besecker B, Lai J-P, Guttridge DC, et al. Zinc modulates the innate immune response in vivo to polymicrobial sepsis through regulation of NF-kappaB. Am J Physiol Lung Cell Mol Physiol. (2010) 298:L744–54. doi: 10.1152/ajplung.00368.2009
69. Gruber K, Maywald M, Rosenkranz E, Haase H, Plumakers B, Rink L. Zinc deficiency adversely influences interleukin-4 and interleukin-6 signaling. J Biol Regul Homeost Agents. (2013) 27:661–71.
70. Maywald M, Wessels I, Rink L. Zinc signals and immunity. Int J Mol Sci. (2017) 18:2222. doi: 10.3390/ijms18102222
71. Maywald M, Meurer SK, Weiskirchen R, Rink L. Zinc supplementation augments TGF-beta1-dependent regulatory T cell induction. Mol Nutr Food Res. (2017) 61:1–11. doi: 10.1002/mnfr.201600493
72. Ollig J, Kloubert V, Taylor KM, Rink L. B cell activation and proliferation increase intracellular zinc levels. J Nutr Biochem. (2019) 64:72–9. doi: 10.1016/j.jnutbio.2018.10.008
73. Reiber C, Brieger A, Engelhardt G, Hebel S, Rink L, Haase H. Zinc chelation decreases IFN-beta-induced STAT1 upregulation and iNOS expression in RAW 264.7 macrophages. J Trace Elem Med Biol. (2017) 44:76–82. doi: 10.1016/j.jtemb.2017.05.011
74. Kulik L, Maywald M, Kloubert V, Wessels I, Rink L. Zinc deficiency drives Th17 polarization and promotes loss of Treg cell function. J Nutr Biochem. (2019) 63:11–8. doi: 10.1016/j.jnutbio.2018.09.011
75. Chen X, Ling J, Mo P, Zhang Y, Jiang Q, Ma Z, et al. Restoration of leukomonocyte counts is associated with viral clearance in COVID-19 hospitalized patients. medRxiv. (2020). doi: 10.1101/2020.03.03.20030437. [Epub ahead of print].
76. Liu J, Li S, Liu J, Liang B, Wang X, Wang H, et al. Longitudinal characteristics of lymphocyte responses and cytokine profiles in the peripheral blood of SARS-CoV-2 infected patients. EBioMedicine. (2020) 55:102763. doi: 10.1016/j.ebiom.2020.102763
77. Diao B, Wang C, Tan Y, Chen X, Liu Y, Ning L, et al. Reduction and functional exhaustion of t cells in patients with Coronavirus Disease 2019 (COVID-19). Front Immunol. (2020) 11:827. doi: 10.3389/fimmu.2020.00827
78. Hönscheid A, Rink L, Haase H. T-lymphocytes: a target for stimulatory and inhibitory effects of zinc ions. Endocr Metab Immune Disord Drug Targets. (2009) 9:132–44. doi: 10.2174/187153009788452390
79. Prasad AS, Meftah S, Abdallah J, Kaplan J, Brewer GJ, Bach JF, et al. Serum thymulin in human zinc deficiency. J Clin Invest. (1988) 82:1202–10. doi: 10.1172/JCI113717
80. Kaltenberg J, Plum LM, Ober-Blöbaum JL, Hönscheid A, Rink L, Haase H. Zinc signals promote IL-2-dependent proliferation of T cells. Eur J Immunol. (2010) 40:1496–503. doi: 10.1002/eji.200939574
81. King LE, Fraker PJ. Variations in the cell cycle status of lymphopoietic and myelopoietic cells created by zinc deficiency. J Infect Dis. (2000) 182(Suppl 1):S16–22. doi: 10.1086/315923
82. Kam YW, Kien F, Roberts A, Cheung YC, Lamirande EW, Vogel L, et al. Antibodies against trimeric S glycoprotein protect hamsters against SARS-CoV challenge despite their capacity to mediate FcgammaRII-dependent entry into B cells in vitro. Vaccine. (2007) 25:729–40. doi: 10.1016/j.vaccine.2006.08.011
83. Jaume M, Yip MS, Cheung CY, Leung HL, Li PH, Kien F, et al. Anti-severe acute respiratory syndrome coronavirus spike antibodies trigger infection of human immune cells via a pH- and cysteine protease-independent FcγR pathway. J Virol. (2011) 85:10582–97. doi: 10.1128/JVI.00671-11
84. Chen Y, Feng Z, Diao B, Wang R, Wang G, Wang C, et al. The novel severe acute respiratory syndrome coronavirus 2 (SARS-CoV-2) directly decimates human spleens and lymph nodes. bioRxiv. (2020). doi: 10.1101/2020.03.27.20045427. [Epub ahead of print].
85. Grommes J, Soehnlein O. Contribution of neutrophils to acute lung injury. Mol Med. (2011) 17:293–307. doi: 10.2119/molmed.2010.00138
86. Hasan R, Rink L, Haase H. Zinc signals in neutrophil granulocytes are required for the formation of neutrophil extracellular traps. Innate Immun. (2013) 19:253–64. doi: 10.1177/1753425912458815
87. Hasan R, Rink L, Haase H. Chelation of free Zn(2)(+) impairs chemotaxis, phagocytosis, oxidative burst, degranulation, and cytokine production by neutrophil granulocytes. Biol Trace Elem Res. (2016) 171:79–88. doi: 10.1007/s12011-015-0515-0
88. Choudhury A, Mukherjee S. In silico studies on the comparative characterization of the interactions of SARS-CoV-2 spike glycoprotein with ACE-2 receptor homologs and human TLRs. J Med Virol. (2020) 1–9. doi: 10.1002/jmv.25987. [Epub ahead of print].
89. Bieback K, Lien E, Klagge IM, Avota E, Schneider-Schaulies J, Duprex WP, et al. Hemagglutinin protein of wild-type measles virus activates toll-like receptor 2 signaling. J Virol. (2002) 76:8729–36. doi: 10.1128/JVI.76.17.8729-8736.2002
90. Kurt-Jones EA, Popova L, Kwinn L, Haynes LM, Jones LP, Tripp RA, et al. Pattern recognition receptors TLR4 and CD14 mediate response to respiratory syncytial virus. Nat Immunol. (2000) 1:398–401. doi: 10.1038/80833
91. Alexopoulou L, Holt AC, Medzhitov R, Flavell RA. Recognition of double-stranded RNA and activation of NF-kappaB by Toll-like receptor 3. Nature. (2001) 413:732–8. doi: 10.1038/35099560
92. Heil F, Hemmi H, Hochrein H, Ampenberger F, Kirschning C, Akira S, et al. Species-specific recognition of single-stranded RNA via toll-like receptor 7 and 8. Science. (2004) 303:1526–9. doi: 10.1126/science.1093620
93. Zhao J, Wohlford-Lenane C, Zhao J, Fleming E, Lane TE, McCray PB, et al. Intranasal treatment with poly(I∙C) protects aged mice from lethal respiratory virus infections. J Virol. (2012) 86:11416–24. doi: 10.1128/JVI.01410-12
94. Brieger A, Rink L, Haase H. Differential regulation of TLR-dependent MyD88 and TRIF signaling pathways by free zinc ions. J Immunol. (2013) 191:1808–17. doi: 10.4049/jimmunol.1301261
95. Rolles B, Maywald M, Rink L. Influence of zinc deficiency and supplementation on NK cell cytotoxicity. J Funct Foods. (2018) 48:322–8. doi: 10.1016/j.jff.2018.07.027
96. Rerksuppaphol S, Rerksuppaphol L. A randomized controlled trial of zinc supplementation in the treatment of acute respiratory tract infection in Thai children. Pediatr Rep. (2019) 11:7954. doi: 10.4081/pr.2019.7954
97. Mahalanabis D, Lahiri M, Paul D, Gupta S, Gupta A, Wahed MA, et al. Randomized, double-blind, placebo-controlled clinical trial of the efficacy of treatment with zinc or vitamin A in infants and young children with severe acute lower respiratory infection. Am J Clin Nutr. (2004) 79:430–6. doi: 10.1093/ajcn/79.3.430
98. Shah UH, Abu-Shaheen AK, Malik MA, Alam S, Riaz M, Al-Tannir MA. The efficacy of zinc supplementation in young children with acute lower respiratory infections: a randomized double-blind controlled trial. Clin Nutr. (2013) 32:193–9. doi: 10.1016/j.clnu.2012.08.018
99. Hulisz D. Efficacy of zinc against common cold viruses: an overview. J Am Pharm Assoc. (2003). (2004) 44:594–603. doi: 10.1331/1544-3191.44.5.594.Hulisz
100. Hemilä H. Zinc lozenges and the common cold: a meta-analysis comparing zinc acetate and zinc gluconate, and the role of zinc dosage. JRSM Open. (2017) 8:2054270417694291. doi: 10.1177/2054270417694291
101. Abdulhamid I, Beck FW, Millard S, Chen X, Prasad A. Effect of zinc supplementation on respiratory tract infections in children with cystic fibrosis. Pediatr Pulmonol. (2008) 43:281–7. doi: 10.1002/ppul.20771
102. Malik A, Taneja DK, Devasenapathy N, Rajeshwari K. Zinc supplementation for prevention of acute respiratory infections in infants: a randomized controlled trial. Indian Pediatr. (2014) 51:780–4. doi: 10.1007/s13312-014-0503-z
103. Khera D, Singh S, Purohit P, Sharma P, Singh K. Prevalence of zinc deficiency and effect of zinc supplementation on prevention of acute respiratory infections. Turk Thorac J. (2019) 1–14. doi: 10.2139/ssrn.3273670
104. Martinez-Estevez NS, Alvarez-Guevara AN, Rodriguez-Martinez CE. Effects of zinc supplementation in the prevention of respiratory tract infections and diarrheal disease in Colombian children: A 12-month randomised controlled trial. Allergol Immunopathol. (2016) 44:368–75. doi: 10.1016/j.aller.2015.12.006
105. Sazawal S, Black RE, Jalla S, Mazumdar S, Sinha A, Bhan MK. Zinc supplementation reduces the incidence of acute lower respiratory infections in infants and preschool children: a double-blind, controlled trial. Pediatrics. (1998) 102:1–5. doi: 10.1542/peds.102.1.1
106. Roth DE, Richard SA, Black RE. Zinc supplementation for the prevention of acute lower respiratory infection in children in developing countries: meta-analysis and meta-regression of randomized trials. Int J Epidemiol. (2010) 39:795–808. doi: 10.1093/ije/dyp391
107. Meydani SN, Barnett JB, Dallal GE, Fine BC, Jacques PF, Leka LS, et al. Serum zinc and pneumonia in nursing home elderly. Am J Clin Nutr. (2007) 86:1167–73. doi: 10.1093/ajcn/86.4.1167
108. Hasanzadeh Kiabi F, Alipour A, Darvishi-Khezri H, Aliasgharian A, Emami Zeydi A. Zinc supplementation in adult mechanically ventilated trauma patients is associated with decreased occurrence of ventilator-associated pneumonia: a secondary analysis of a prospective, observational study. Indian J Crit Care Med. (2017) 21:34–9. doi: 10.4103/0972-5229.198324
109. Al-Nakib W, Higgins PG, Barrow I, Batstone G, Tyrrell DA. Prophylaxis and treatment of rhinovirus colds with zinc gluconate lozenges. J Antimicrob Chemother. (1987) 20:893–901. doi: 10.1093/jac/20.6.893
110. Kurugöl Z, Akilli M, Bayram N, Koturoglu G. The prophylactic and therapeutic effectiveness of zinc sulphate on common cold in children. Acta Paediatr. (2006) 95:1175–81. doi: 10.1080/08035250600603024
111. Singh M, Das RR. Zinc for the common cold. Cochrane Database Syst Rev. (2013) 18:CD001364. doi: 10.1002/14651858.CD001364.pub4
112. Isaacs D, Flowers D, Clarke JR, Valman HB, MacNaughton MR. Epidemiology of coronavirus respiratory infections. Arch Dis Child. (1983) 58:500–3. doi: 10.1136/adc.58.7.500
113. Eby GA, Davis DR, Halcomb WW. Reduction in duration of common colds by zinc gluconate lozenges in a double-blind study. Antimicrob Agents Chemother. (1984) 25:20–4. doi: 10.1128/AAC.25.1.20
114. Godfrey JC, Conant Sloane B, Smith DS, Turco JH, Mercer N, Godfrey NJ. Zinc gluconate and the common cold: a controlled clinical study. J Int Med Res. (1992) 20:234–46. doi: 10.1177/030006059202000305
115. Wang MX, Win SS, Pang J. Zinc supplementation reduces common cold duration among healthy adults: a systematic review of randomized controlled trials with micronutrients supplementation. Am J Trop Med Hyg. (2020). doi: 10.4269/ajtmh.19-0718
116. Yakoob MY, Theodoratou E, Jabeen A, Imdad A, Eisele TP, Ferguson J, et al. Preventive zinc supplementation in developing countries: impact on mortality and morbidity due to diarrhea, pneumonia and malaria. BMC Public Health. (2011) 11(Suppl. 3):S23. doi: 10.1186/1471-2458-11-S3-S23
117. Ackland ML, Michalczyk A. Zinc deficiency and its inherited disorders -a review. Genes Nutr. (2006) 1:41–9. doi: 10.1007/BF02829935
118. Petrilli CM, Jones SA, Yang J, Rajagopalan H, O'Donnell LF, Chernyak Y, et al. Factors associated with hospitalization and critical illness among 4,103 patients with COVID-19 disease in New York city. medRxiv [Preprint]. (2020). doi: 10.1101/2020.04.08.20057794. [Epub ahead of print].
119. Vishnevetsky A, Levy M. Rethinking high-risk groups in COVID-19. Mult Scler Relat Disord. (2020) 42:102139. doi: 10.1016/j.msard.2020.102139
120. Briefel RR, Bialostosky K, Kennedy-Stephenson J, McDowell MA, Ervin RB, Wright JD. Zinc intake of the U.S. population: findings from the third National Health and Nutrition Examination Survey, 1988-1994. J Nutr. (2000) 130:1367S−73S. doi: 10.1093/jn/130.5.1367S
121. Bhat MH, Mudassir Rather AB, Dhobi GN, Koul AN, Bhat FA, et al. Zinc levels in community acquired pneumonia in hospitalized patients; a case control study. Egypt J Chest Dis Tubercul. (2016) 65:485–9. doi: 10.1016/j.ejcdt.2015.12.020
122. Kamei S, Fujikawa H, Nohara H, Ueno-Shuto K, Maruta K, Nakashima R, et al. Zinc deficiency via a splice switch in zinc importer ZIP2/SLC39A2 causes cystic fibrosis-associated MUC5AC hypersecretion in airway epithelial cells. EBioMedicine. (2018) 27:304–16. doi: 10.1016/j.ebiom.2017.12.025
123. Haase H, Mocchegiani E, Rink L. Correlation between zinc status and immune function in the elderly. Biogerontology. (2006) 7:421–8. doi: 10.1007/s10522-006-9057-3
124. ClinicalTrials.gov. A Study of Hydroxychloroquine and Zinc in the Prevention of COVID-19 Infection in Military Healthcare Workers. (2020). Available online at: https://ClinicalTrials.gov/show/ (accessed May 15, 2020).
125. Yan CH, Faraji F, Prajapati DP, Boone CE, DeConde AS. Association of chemosensory dysfunction and Covid-19 in patients presenting with influenza-like symptoms. Int Forum Allergy Rhinol. (2020) 10:806–13. doi: 10.1002/alr.22579
126. Yan CH, Faraji F, Prajapati DP, Ostrander BT, DeConde AS. Self-reported olfactory loss associates with outpatient clinical course in Covid-19. Int Forum Allergy Rhinol. (2020) 10:821–31. doi: 10.1002/alr.22592
127. Soler ZM, Patel ZM, Turner JH, Holbrook EH. A primer on viral-associated olfactory loss in the era of COVID-19. Int Forum Allergy Rhinol. (2020) 10:814–20. doi: 10.1002/alr.22578
128. Roland LT, Gurrola JG, Loftus PA, Cheung SW, Chang JL. Smell and taste symptom-based predictive model for COVID-19 diagnosis. Int Forum Allergy Rhinol. (2020) 10:832–8. doi: 10.1002/alr.22602
129. Moein ST, Hashemian SM, Mansourafshar B, Khorram-Tousi A, Tabarsi P, Doty RL. Smell dysfunction: a biomarker for COVID-19. Int Forum Allergy Rhinol. (2020). doi: 10.1002/alr.22587. [Epub ahead of print].
130. Lechien JR, Hopkins C, Saussez S. Sniffing out the evidence; It's now time for public health bodies recognize the link between COVID-19 and smell and taste disturbance. Rhinology. (2020). doi: 10.4193/Rhin20.159. [Epub ahead of print].
131. Lechien JR, Chiesa-Estomba CM, Siati DR de, Horoi M, Le Bon SD, Rodriguez A, et al. Olfactory and gustatory dysfunctions as a clinical presentation of mild-to-moderate forms of the coronavirus disease (COVID-19): a multicenter European study. Eur Arch Otorhinolaryngol. (2020) 1–11. doi: 10.1007/s00405-020-05965-1
132. Russell B, Moss C, Rigg A, Hopkins C, Papa S, van Hemelrijck M. Anosmia and ageusia are emerging as symptoms in patients with COVID-19: What does the current evidence say? Ecancermedicalscience. (2020) 14:ed98. doi: 10.3332/ecancer.2020.ed98
133. Giacomelli A, Pezzati L, Conti F, Bernacchia D, Siano M, Oreni L, et al. Self-reported olfactory and taste disorders in SARS-CoV-2 patients: a cross-sectional study. Clin Infect Dis. (2020). doi: 10.1093/cid/ciaa330. [Epub ahead of print].
134. Netland J, Meyerholz DK, Moore S, Cassell M, Perlman S. Severe acute respiratory syndrome coronavirus infection causes neuronal death in the absence of encephalitis in mice transgenic for human ACE2. J Virol. (2008) 82:7264–75. doi: 10.1128/JVI.00737-08
135. Goto T, Komai M, Bryant BP, Furukawa Y. Reduction in carbonic anhydrase activity in the tongue epithelium and submandibular gland in zinc-deficient rats. Int J Vitam Nutr Res. (2000) 70:110–8. doi: 10.1024/0300-9831.70.3.110
136. Goto T, Komai M, Suzuki H, Furukawa Y. Long-term zinc deficiency decreases taste sensitivity in rats. J Nutr. (2001) 131:305–10. doi: 10.1093/jn/131.2.305
137. Goto T, Shirakawa H, Furukawa Y, Komai M. Decreased expression of carbonic anhydrase isozyme II, rather than of isozyme VI, in submandibular glands in long-term zinc-deficient rats. Br J Nutr. (2008) 99:248–53. doi: 10.1017/S0007114507801565
138. Tanaka M. Secretory function of the salivary gland in patients with taste disorders or xerostomia: correlation with zinc deficiency. Acta Otolaryngol Suppl. (2002) 122:134–41. doi: 10.1080/00016480260046526
139. Russell RM, Cox ME, Solomons N. Zinc and the special senses. Ann Intern Med. (1983) 99:227–39. doi: 10.7326/0003-4819-99-2-227
140. Sandstead HH, Henriksen LK, Greger JL, Prasad AS, Good RA. Zinc nutriture in the elderly in relation to taste acuity, immune response, and wound healing. Am J Clin Nutr. (1982) 36:1046–59. doi: 10.1093/ajcn/36.5.1046
141. Heckmann SM, Hujoel P, Habiger S, Friess W, Wichmann M, Heckmann JG, et al. Zinc gluconate in the treatment of dysgeusia–a randomized clinical trial. J Dent Res. (2005) 84:35–8. doi: 10.1177/154405910508400105
142. Sturniolo GC, D'Inca R, Parisi G, Giacomazzi F, Montino MC, D'Odorico A, et al. Taste alterations in liver cirrhosis: are they related to zinc deficiency? J Trace Elem Electrolytes Health Dis. (1992) 6:15–9.
143. Lyckholm L, Heddinger SP, Parker G, Coyne PJ, Ramakrishnan V, Smith TJ, et al. A randomized, placebo controlled trial of oral zinc for chemotherapy-related taste and smell disorders. J Pain Palliat Care Pharmacother. (2012) 26:111–4. doi: 10.3109/15360288.2012.676618
144. Arbeitsgemeinschaft der Wissenschaftlichen Medizinischen Fachgesellschaften. Riech- und Schmeckstörungen (017/050). (2016). Available online at: https://www.awmf.org/leitlinien/detail/ll/017-050.html
145. Cox MJ, Loman N, Bogaert D, O'Grady J. Co-infections: potentially lethal and unexplored in COVID-19. Lancet Microbe. (2020) 1:e11. doi: 10.1016/S2666-5247(20)30009-4
146. Eijkelkamp BA, Morey JR, Neville SL, Tan A, Pederick VG, Cole N, et al. Dietary zinc and the control of Streptococcus pneumoniae infection. PLoS Pathog. (2019) 15:e1007957. doi: 10.1371/journal.ppat.1007957
147. Levi M, Thachil J, Iba T, Levy JH. Coagulation abnormalities and thrombosis in patients with COVID-19. The Lancet Haematology. (2020) 7:e438–40. doi: 10.1016/S2352-3026(20)30145-9
148. Gavriilaki E, Brodsky RA. Severe COVID-19 infection and thrombotic microangiopathy: success doesn't come easily. Br J Haematol. (2020) 189:e227–30. doi: 10.1111/bjh.16783
149. Varga Z, Flammer AJ, Steiger P, Haberecker M, Andermatt R, Zinkernagel AS, et al. Endothelial cell infection and endotheliitis in COVID-19. Lancet. (2020) 395:1417–8. doi: 10.1016/S0140-6736(20)30937-5
150. Taylor KA, Pugh N. The contribution of zinc to platelet behaviour during haemostasis and thrombosis. Metallomics. (2016) 8:144–55. doi: 10.1039/C5MT00251F
151. Lopes-Pires ME, Ahmed NS, Vara D, Gibbins JM, Pula G, Pugh N. Zinc regulates reactive oxygen species generation in platelets. Platelets. (2020). doi: 10.1080/09537104.2020.1742311. [Epub ahead of print].
152. Xiao F, Tang M, Zheng X, Liu Y, Li X, Shan H. Evidence for gastrointestinal infection of SARS-CoV-2. Gastroenterology. (2020) 158:1831–3.e3. doi: 10.1101/2020.02.17.20023721
153. Zhang C, Shi L, Wang F-S. Liver injury in COVID-19: management and challenges. Lancet Gastroenterol Hepatol. (2020) 5:428–30. doi: 10.1016/S2468-1253(20)30057-1
154. Inciardi RM, Lupi L, Zaccone G, Italia L, Raffo M, Tomasoni D, et al. Cardiac involvement in a patient with coronavirus disease 2019 (COVID-19). JAMA Cardiol. (2020). doi: 10.1001/jamacardio.2020.1096. [Epub ahead of print].
155. Baig AM. Updates on what ACS reported: emerging evidences of COVID-19 with nervous system involvement. ACS Chem Neurosci. (2020) 11:1204–5. doi: 10.1021/acschemneuro.0c00181
156. Diao B, Wang C, Wang R, Feng Z, Tan Y, Wang H, et al. Human kidney is a target for novel severe acute respiratory syndrome coronavirus 2 (SARSCoV-2) infection. medRxiv [Preprint]. (2020). doi: 10.1101/2020.03.04.20031120
157. Galván Casas C, Català A, Carretero Hernández G, Rodríguez-Jiménez P, Fernández Nieto D, Rodríguez-Villa Lario A, et al. Classification of the cutaneous manifestations of COVID-19: a rapid prospective nationwide consensus study in Spain with 375 cases. Br J Dermatol. (2020) 183:71–7. doi: 10.1111/bjd.19163
158. Lin P-H, Sermersheim M, Li H, Lee PH, Steinberg SM, Ma J. Zinc in wound healing modulation. Nutrients. (2017) 10:16. doi: 10.3390/nu10010016
159. Aydemir TB, Sitren HS, Cousins RJ. The zinc transporter Zip14 influences c-Met phosphorylation and hepatocyte proliferation during liver regeneration in mice. Gastroenterology. (2012) 142:1536–46.e5. doi: 10.1053/j.gastro.2012.02.046
160. ClinicalTrials.gov. Coronavirus 2019 (COVID-19)- Using Ascorbic Acid and Zinc Supplementation. (2020). Available online at: https://ClinicalTrials.gov/show/NCT04342728 (accessed May 15, 2020).
161. ClinicalTrials.gov. Hydroxychloroquine and Zinc With Either Azithromycin or Doxycycline for Treatment of COVID-19 in Outpatient Setting. (2020). Available from: https://ClinicalTrials.gov/show/NCT04370782 (accessed May 15, 2020).
162. ANZCTR.org.au. High-Dose Intravenous Zinc (HDIVZn) As Adjunctive Therapy in COVID-19 Positive Critically Ill Patients: A Pilot Randomized Controlled Trial. (2020). Available online at: https://www.anzctr.org.au/Trial/Registration/TrialReview.aspx?id=379579&isReview=true (accessed May 15, 2020).
Keywords: zinc, COVID-19, SARS-CoV2, 2019-nCoV, coronaviridae, zinc deficiency, impaired immune system
Citation: Wessels I, Rolles B and Rink L (2020) The Potential Impact of Zinc Supplementation on COVID-19 Pathogenesis. Front. Immunol. 11:1712. doi: 10.3389/fimmu.2020.01712
Received: 18 May 2020; Accepted: 26 June 2020;
Published: 10 July 2020.
Edited by:
Francisco José Pérez-Cano, University of Barcelona, SpainReviewed by:
Anastasia N. Vlasova, The Ohio State University, United StatesAlberto Finamore, Council for Agricultural and Economics Research (CREA), Italy
Copyright © 2020 Wessels, Rolles and Rink. This is an open-access article distributed under the terms of the Creative Commons Attribution License (CC BY). The use, distribution or reproduction in other forums is permitted, provided the original author(s) and the copyright owner(s) are credited and that the original publication in this journal is cited, in accordance with accepted academic practice. No use, distribution or reproduction is permitted which does not comply with these terms.
*Correspondence: Lothar Rink, bHJpbmtAdWthYWNoZW4uZGU=
†These authors have contributed equally to this work