- 1Department of Microbiology, Faculty of Medicine, Chulalongkorn University, Bangkok, Thailand
- 2Program in Bioinformatics and Computational Biology, Graduate School, Chulalongkorn University, Bangkok, Thailand
- 3Department of Biochemistry, Faculty of Medicine, Chulalongkorn University, Bangkok, Thailand
- 4Center of Excellence in Systems Biology, Chulalongkorn University, Bangkok, Thailand
- 5Medical Microbiology, Interdisciplinary Program, Graduate School, Chulalongkorn University, Bangkok, Thailand
- 6Translational Research in Inflammation and Immunology Research Unit, Department of Microbiology, Chulalongkorn University, Bangkok, Thailand
Obesity induces gut leakage and elevates serum lipopolysaccharide (LPS), a major cell wall component of Gram-negative bacteria, through gut translocation. Because Candida albicans is prominent in human gut but not in mouse, C. albicans, a source of (1→3)-β-D-glucan (BG) in gut contents, was administered in high-fat diet (HFD)–induced obese mice at 1 week before sepsis induction by cecal ligation and puncture (CLP). As such, sepsis in Candida-administered obese mice was more severe than obese mice without Candida as determined by mortality, organ injury (liver and kidney), serum cytokines, gut leakage, endotoxemia, serum BG, and fecal Gram-negative bacteria (microbiome analysis). Mice subjected to CLP and fed a HFD, but not treated with Candida demonstrated a similar mortality to non-obese mice with more severe gut leakage and higher serum cytokines. In vitro experiments demonstrated that LPS plus BG (LPS + BG) induced higher supernatant cytokines from hepatocytes (HepG2) and macrophages (RAW264.7), compared with the activation by each molecule alone, and were amplified by palmitic acid, a representative saturated fatty acid. The energy production capacity of HepG2 cells was also decreased by LPS + BG compared with LPS alone as evaluated by extracellular flux analysis. However, Lactobacillus rhamnosus L34 (L34) improved sepsis, regardless of Candida administration, through the attenuation of gut leakage and gut dysbiosis. In conclusion, an impact of gut Candida was demonstrated by Candida pretreatment in obese mice that worsened sepsis through (1) gut dysbiosis–induced gut leakage and (2) amplified systemic inflammation due to LPS, BG, and saturated fatty acid.
Introduction
Both sepsis, a syndrome of imbalance immune responses to pathogens, and obesity are major healthcare problems worldwide (1–4). Whereas obesity induces several chronic conditions such as diabetes, dyslipidemia, and cardiovascular disease (5), sepsis is a major cause of death in critically ill patients, mostly with chronic underlying diseases (3). Indeed, obesity is categorized as a sepsis comorbidity and an independent risk factor for death of patients in the intensive care unit (6, 7), at least in part, due to the enhanced inflammation caused by adipocytes and immune cells (8, 9). In addition, obesity and high-fat diet (HFD) cause gut dysbiosis, an alteration of bacteria and fungi in gut (10), that induces gut-permeability defect (gut leakage) leading to spontaneous endotoxemia (11). The impact of obesity on sepsis remains a controversy as obesity worsens sepsis severity through the induction of several metabolic abnormalities but beneficially restores energy preserve in the moribund stage of sepsis (12, 13). However, endotoxemia increased systemic inflammation and enhanced sepsis severity (14). Although endotoxemia from obesity implies the importance of intestinal Gram-negative bacteria as a source of intestinal endotoxin (lipopolysaccharide; LPS), the impact of Candida albicans which is the second most predominant gut organism (15) on obesity is still not clear. On the contrary, the impact of intestinal C. albicans in other models has been mentioned. For example, increased abundance of C. albicans in alcohol ingestion model and in patients enhances liver cirrhosis through direct activation of intestinal (1→3)-β-D-glucan (BG) which is a major component of fungal cell wall against hepatocytes (16–18). In addition, intestinal C. albicans is a source of BG in gut contents. In addition, BG from gut translocation amplifies the inflammatory property of LPS through the synergy of Dectin-1 and Toll-like receptor (TLR)-4 which are receptors of BG and LPS, respectively, in sepsis and several inflammatory models (19–24).
Interestingly, HFD also increases the abundance of Candida spp. in mouse feces, but the abundance of fecal fungi in mouse feces is not high enough to be detectable by culture (10). Indeed, C. albicans in mouse intestine are lesser than human intestine (25) as fungi in human stool are easily detectable by culture in comparison with mouse feces (26). Hence, oral administration of C. albicans is necessary to increase mouse fecal fungi. Mouse models with fecal C. albicans more closely resemble human conditions, at least in part, because of the interaction between gut organisms (gut dysbiosis) (27). As such, C. albicans induce gut dysbiosis in sepsis (28) and sepsis with obesity (29, 30) that might be associated with gut leakage (31). In addition, gut leakage in obesity (11), and sepsis (14) is attenuated by probiotics (32–36), including Lactobacillus spp. that could interfere with Candida growth (37). Moreover, Lactobacillus spp. attenuated gut dysbiosis in several animal models (27, 38). Hence, an obesity mouse model was performed with C. albicans pretreatment before cecal ligation and puncture (CLP) sepsis with an evaluation on a probiotic. Understanding the influence of gut fungi in sepsis with obesity might be beneficial in sepsis treatment.
Materials and Methods
Animals and Animal Model
The animal care and use protocol prepared according to the US National Institutes of Health standards was approved by the Institutional Animal Care and Use Committee of the Faculty of Medicine, Chulalongkorn University, Bangkok, Thailand (SST 04/2561). Male, 8-week-old C57BL/6 mice were purchased from the National Laboratory Animal Center, Nakhorn Pathom, Thailand. Mice in the regular diet group received standard laboratory chow containing fat (4.5% w/w), with energy content calculated at 3.04 kcal/g (Mouse Feed Food No. 082; C.P. Company, Bangkok, Thailand). Mice in the obese group were fed for 5 months with HFD containing fat, mostly from lard (60% w/w), with energy content calculated at 8.64 kcal/g following a publication (39). Schema of the experiments is demonstrated in Figure 1. At 3 months of the experiment, Lactobacillus rhamnosus L34 (L34) (38) at 1 × 109 colony-forming units (CFU) in 0.5 ml phosphate buffer solution (PBS) or PBS alone were administered daily for 2 months before Candida administration. At 1 week before sepsis induction, C. albicans from American Type Culture Collection (ATCC90028; Fisher Scientific, Waltham, MA, United States) at 1 × 106 CFU in 0.5 ml PBS or PBS alone were orally administered every 2 days to induce Candida in gut. At 24 h from the last dose of Candida or PBS, CLP or sham was performed following a publication with 10-mm cecal ligation and a 21-gage needle under isoflurane anesthesia (21). Fentanyl, 0.03 mg/kg in 0.5 ml of normal saline solution (NSS), was subcutaneously administered at post-operation and at 6 h later. Mice were sacrificed at 24 h post-surgery under isoflurane anesthesia with blood and organ collection. Ascending colon 1 cm from colon–cecal junction was snap frozen in liquid nitrogen and kept at -80°C before use. Feces from all parts of colon were combined and collected for microbiome analysis and fecal fungal burdens.
Mouse Blood Sample Analysis and Gut Leakage Measurement
Several obesity parameters were determined after fasting for 12 h with free access to drinking water. Fasting glucose and triglyceride were measured by glucose colorimetric assay (Cayman Chemical, Ann Arbor, MI, United States) and triglyceride quantification kit (Sigma-Aldrich, St. Louis, MO, United States), respectively. The lipid profile was evaluated using assays of total cholesterol quantitation (Sigma-Aldrich), low-density lipoprotein cholesterol (LDL; Crystal Chem, Downners Grove, IL, United States), and high-density lipoprotein cholesterol (HDL; Crystal Chem). Renal injury and liver damage were determined by QuantiChrom Creatinine Assay (DICT-500; Bioassay, Hayward, CA, United States) and EnzyChrom Alanine Transaminase assay (EALT-100; BioAssay), respectively. Serum cytokine levels were determined by ELISA for mouse cytokines [tumor necrosis factor (TNF)-α, interleukin (IL)-6, and IL-10] (Invitrogen, Carlsbad, CA, United States). Gut leakage was determined by detection of fluorescein isothiocyanate (FITC)–dextran, a non-absorbable high molecular weight molecule, in serum after oral administration as mentioned in previous publications (40). Serum BG was determined by Fungitell (Associates of Cape Cod, East Falmouth, MA, United States) and serum endotoxin (LPS) was measured by HEK-Blue LPS Detection (InvivoGen, San Diego, CA, United States). When values of BG and LPS at <7.8 and at <0.01 EU/ml, respectively, were beyond the lower range of the standard curve, data were recorded as 0.
Liver Histology, Intestinal Cytokines, Fecal Fungal Burden, and Fecal pH
Paraffin-embedded liver sections (4 μm thick) stained by H&E from 10% formalin-fixed samples were evaluated with a scoring system of obesity-induced liver damage as the following: steatosis (0–3), lobular inflammation (0–3), and hepatocellular ballooning degeneration (0–2) (41). For intestinal cytokine detection, intestinal tissues were weighed, cut, thoroughly sonicated (15 s with pulse off 5 s for 5 times; High Intensity Ultrasonic Processor, Newtown, CT, United States) in 500 μl of ice-cold PBS containing protease inhibitor Cocktail (I3786; Sigma-Aldrich) and measured cytokines from the supernatant by ELISA (Invitrogen). For analysis of fungal burdens in feces, feces were suspended with PBS at a ratio of 100 μg per 1 μl and serially diluted before plating onto 0.1% chloramphenicol in Sabouraud Dextrose Agar (SDA; Thermo Scientific, Waltham, MA, United States) and aerobically incubated at 35°C for 72 h before colony enumeration. For fecal pH evaluation, 1 g of feces was thoroughly mixed with 2 ml of water before centrifugation at 4000 rpm for 3 min. Then, the pH of the supernatant was measured by a pH meter (Orion’4 star, pH Conductivity Benchtop; Thermo Scientific).
Fecal Microbiome Analysis
Feces from nine mice (0.25 g per mouse) from different cages in each experimental group were divided into three samples per group (three mice per sample) before performing microbiota analysis. Total DNA from feces was extracted by GenUP gDNA extraction kit (Biotechrabbit, Germany) followed by 16S rDNA amplification for next-generation sequencing (NGS) with Illumina platform as previously published (42). For data analysis, the raw data were de-multiplexed by miSeq reporter software (version 2.6.2.3). Paired-end FASTQ sequences were then analyzed with QIIME2 pipeline (version 2018.8) (43). After that, joined reads were de-duplicated and clustered with 97% similarity by VSEARCH (44). Chimeric sequences were filtered out by UCHIME algorithm (45). The filtered reads were classified based on 99% operational taxonomic units (OTUs) clustered 16S Greengene database (2013.8) (46) using vsearch algorithm.
Hepatocyte Cell-Line Experiments
HepG2, a human hepatoma cell line (ATCC HB-8065; Fisher Scientific), was maintained in Dulbecco’s Modified Eagle Medium (DMEM) with 10% fetal bovine serum (FBS), 1% penicillin/streptomycin antibiotics, and 1% sodium pyruvate in a humidified atmosphere of 5% CO2 at 37°C. HepG2 at 2 × 105 cells/ml in a 96-well plate were incubated with or without 0.5 mM of palmitic acid (PA; Sigma-Aldrich), a saturated free fatty acid, in DMEM at 37°C for 48 h before further incubation with purified LPS (1 μg/ml) from Escherichia coli 026:B6 (Sigma-Aldrich) alone or in combination with CM-Pachyman (100 μg/ml; Megazyme, Bray, Ireland) as a representative of BG. After PA incubation for 24 h, intracellular lipid was determined by 0.3% Oil Red O solution (Sigma-Aldrich) and evaluated by Image J (NIH, Bethesda, MD, United States) in 10 randomized fields from each well as previously mentioned (47). Supernatant cytokine were determined using ELISA for human cytokines (TNF-α, IL-8, and IL-10; R&D Systems, Minneapolis, MN, United States). In addition, a neutral soluble glucan, a competitive Dectin-1 binding agent (at 150 μg/ml; InvivoGen), was incubated simultaneously with BG as a Dectin-1 inhibitor to explore the impact of Dectin-1, a BG receptor, on hepatocytes. Moreover, energy metabolism profiles of hepatocytes activated by PA simultaneously with LPS or with LPS + BG with glycolysis estimation through extracellular acidification rate and mitochondrial oxidative phosphorylation by oxygen consumption rate were performed using Seahorse XFp Analyzers (Agilent, Santa Clara, CA, United States) upon HepG2 at 1 × 104 cells/well (47, 48).
Macrophage Cell-Line Experiments
RAW264.7, a mouse macrophage cell line, at 1 × 105 cells per well was incubated with 0.2 mM PA (Sigma-Aldrich) alone or in combination with LPS (1 μg/ml; Sigma-Aldrich) or BG, CM-Pachyman (100 μg/ml; Megazyme), or LPS + BG, similar to hepatocyte experiments, for 6 h before determination of Oil Red O staining. In parallel, supernatant cytokines were measured by ELISA for mouse cytokines (TNF-α, IL-6, and IL-10; Invitrogen). In addition, a Dectin-1 inhibitor (150 μg/ml; InvivoGen) was incubated with BG to explore the impact of Dectin-1 in macrophages.
Statistical Analysis
Mean ± SE was used for data presentation. The differences between groups were examined for statistical significance by one-way ANOVA followed by Tukey’s analysis or Student’s t-test for comparisons of multiple groups or two groups, respectively. Survival analysis was performed by log-rank test. All statistical analyses were performed with SPSS 11.5 software (SPSS, IL, United States) and GraphPad Prism version 7.0 software (La Jolla, CA, United States). A p value of < 0.05 was considered statistically significant.
Results
As expected, HFD-induced obesity in mice led to increased body weight, peri-renal fat, liver weight, fatty liver score, fasting blood glucose, and altered lipid profiles (Figure 2).
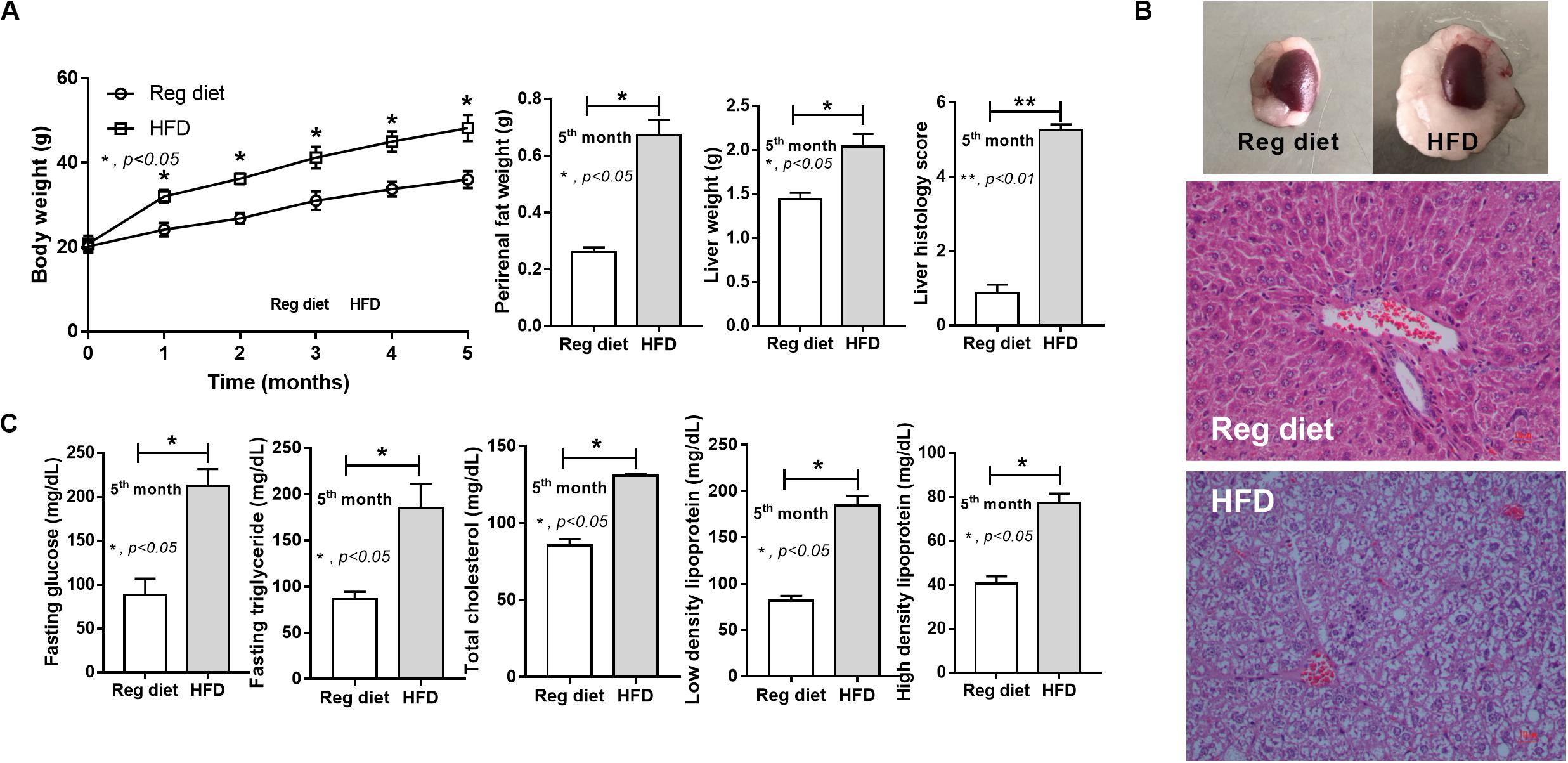
Figure 2. Characteristics of mice fed with a regular diet and a high-fat diet (HFD) as demonstrated by body weight, peri-renal fat weight, liver weight, and liver histology score (A; n = 7–8/time point and n = 6–8/group) with representative pictures of peri-renal fat and H&E-stained liver histology (B) together with metabolic parameters (C; n = 6–8/group) are demonstrated. *p < 0.05; **p < 0.01.
Sepsis Severity of Obese Mice With and Without Candida Administration, an Impact of Gut-Permeability Defect and Gut Dysbiosis
In mice subjected to CLP and fed a HFD, but not treated with Candida, sepsis were more severe than regular diet mice as determined by survival, organ injury (serum creatinine and alanine transaminase), serum cytokines (TNF-α and IL-6), gut leakage by FITC–dextran and endotoxemia, but not serum BG (Figures 3A–I). In mice that were not subjected to CLP, there were slightly elevated serum endotoxin (Figure 3H) along with gut dysbiosis in HFD mice compared with regular diet mice as demonstrated by fecal total Gram-negative bacteria (Proteobacteria in Halomonas spp.) and reduced Ruminococcaceae, beneficial cellulolytic Gram-positive anaerobes (49) (Figure 4). Mice in the HFD-CLP group that were not treated with Candida demonstrated lower total fecal Gram-negative bacteria (Desulfovibrionaceae, Bacteroides) with higher Firmicutes, beneficial Gram-positive anaerobes, and Clostridiales family, a group of bacteria including mucosal invasive Clostridium spp. (50), in comparison with mice in the regular diet–CLP group (Figure 4). On the other hand, Candida administration did not induce diarrhea (data not shown) and did not alter obesity parameters (Figure 5), but it enhanced CLP mortality in both regular diet and HFD mice (Figure 6A).
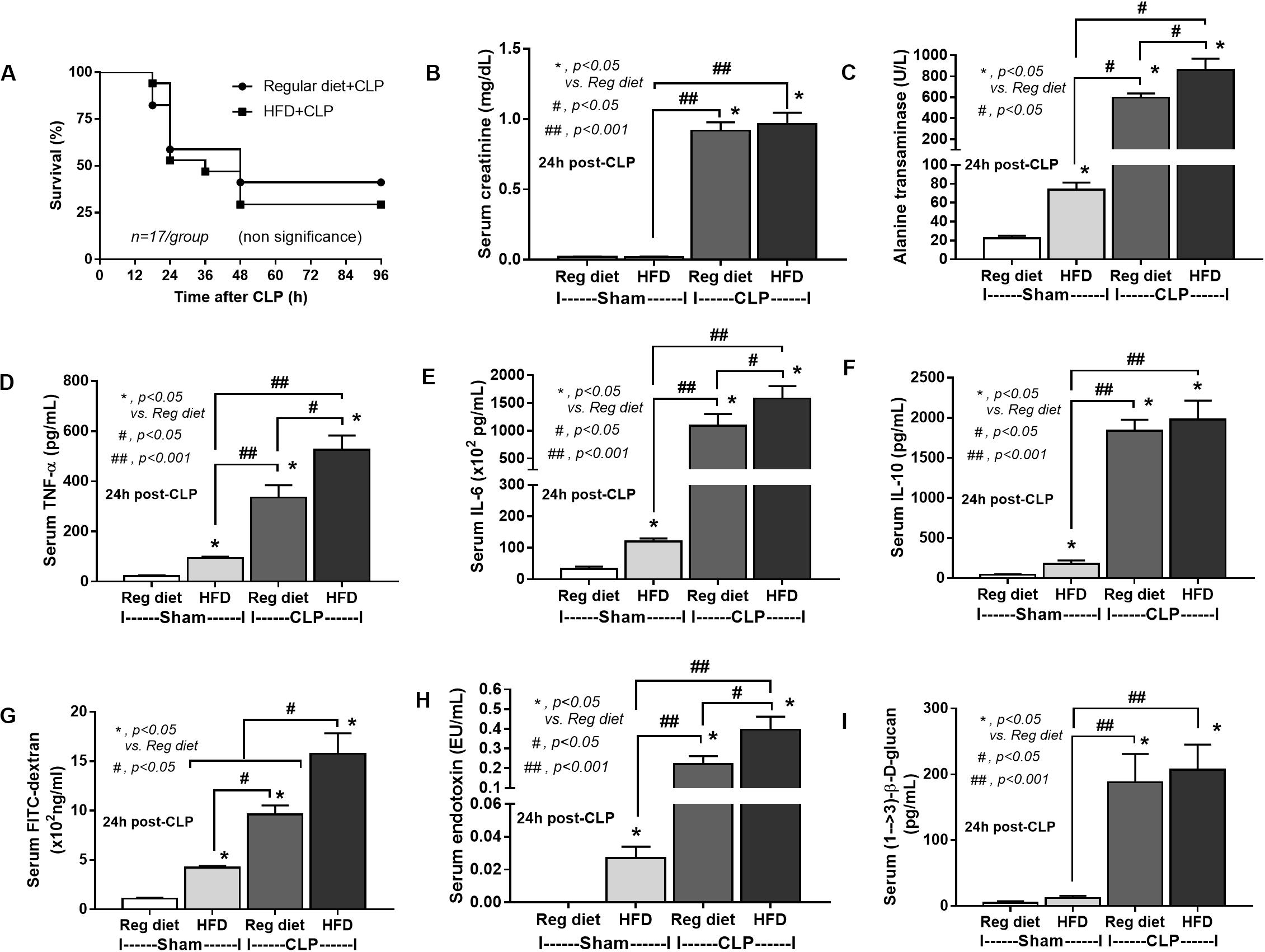
Figure 3. Characteristics of mice fed a regular diet or a high-fat diet (HFD) with sham or cecal ligation and puncture (CLP) surgery as determined by survival analysis (A), kidney and liver injury (B,C), serum cytokines (D–F), gut leakage by FITC–dextran (G), endotoxemia (H), and serum (1→3)-β-D-glucan (I; n = 6–8/group for B–I) are demonstrated. *p < 0.05; #p < 0.05; ##p < 0.001.
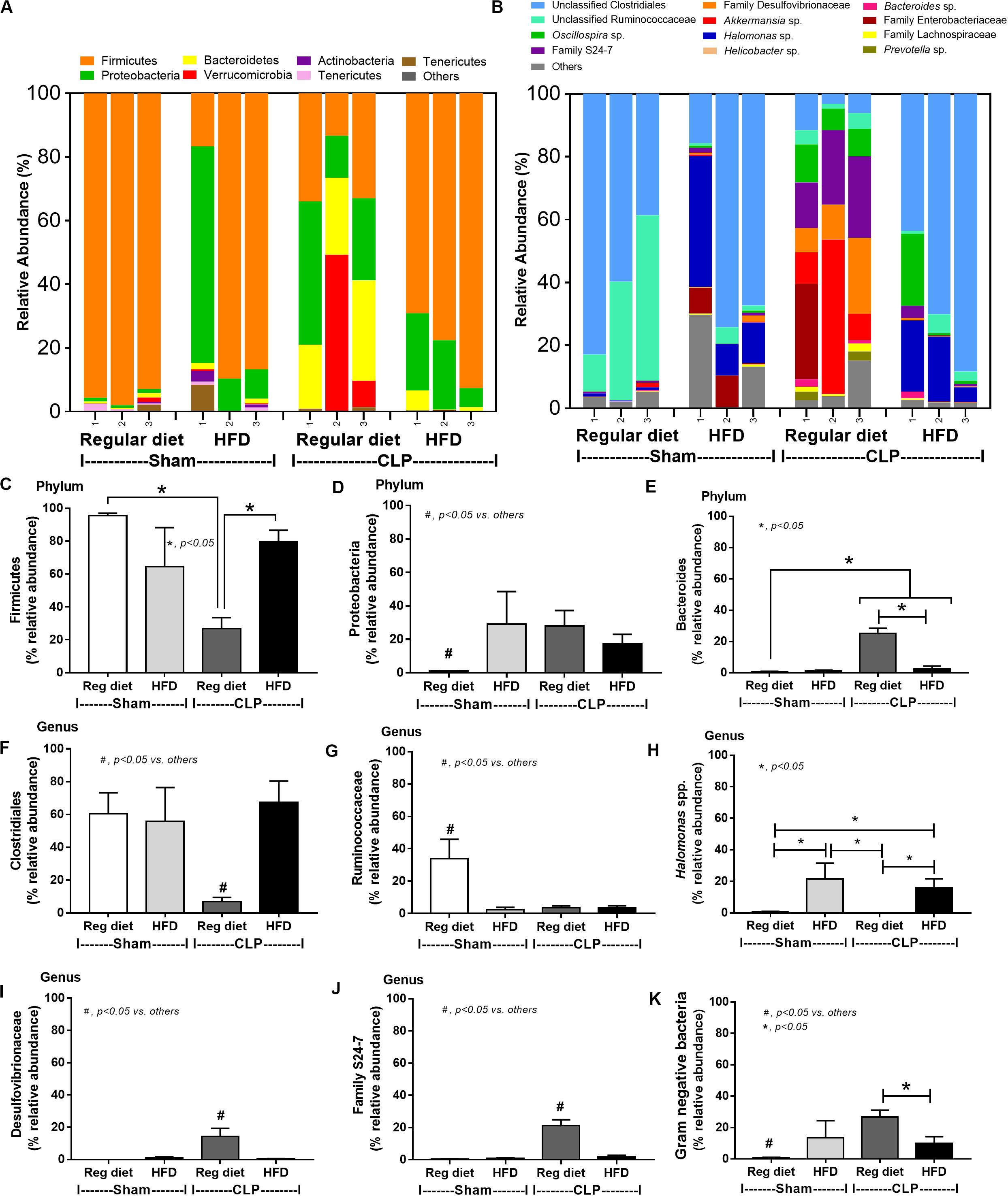
Figure 4. Gut microbiota analysis from feces of mice fed with a regular diet or a high-fat diet (HFD) with sham or cecal ligation and puncture (CLP) surgery by relative abundance of bacterial diversity at phylum (A) and at genus (B) with the better visualization (C–J) and abundance of total Gram-negative bacteria determined from phylum (K) are demonstrated. *p < 0.05; #p < 0.05.
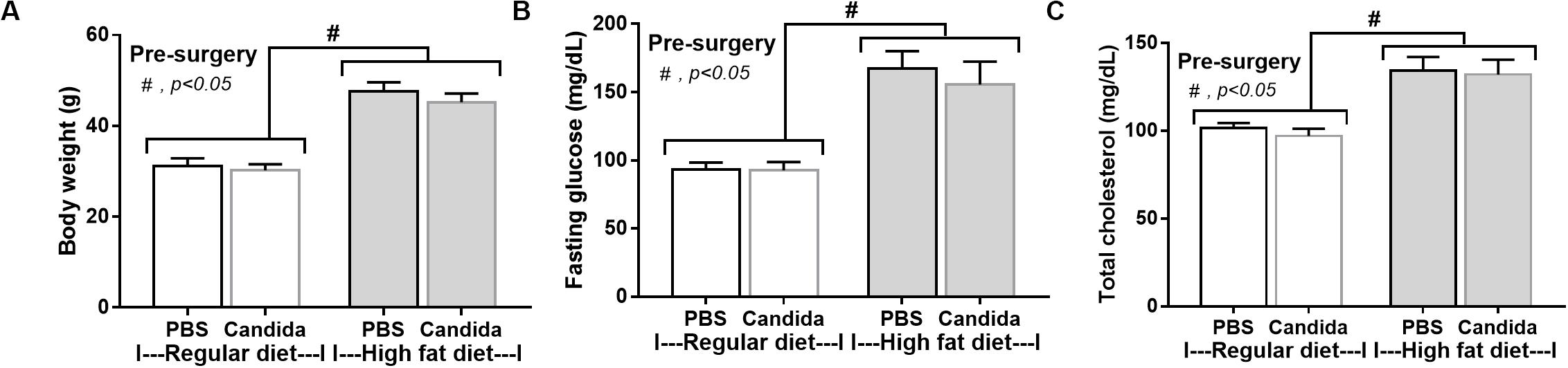
Figure 5. Characteristics of mice fed with a regular diet or a high-fat diet (HFD) treated with phosphate buffer solution (PBS) or Candida at 5th month of the experiments as determined by body weight (A), fasting glucose and total cholesterol in blood (B,C; n = 6–8/group) are demonstrated. #p < 0.05.
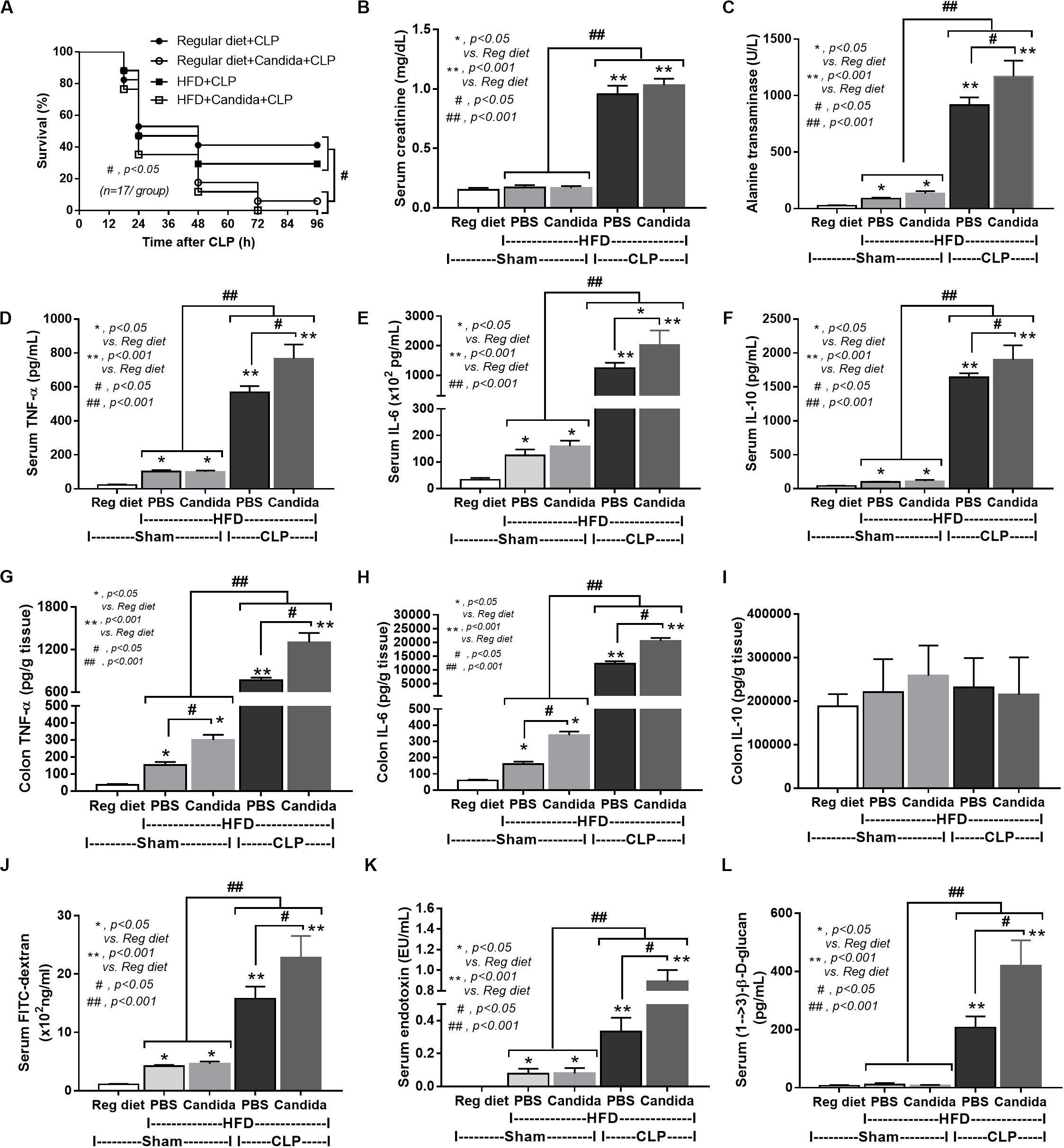
Figure 6. Characteristics of mice fed a regular diet that subjected to sham surgery and mice fed a high-fat diet (HFD) treated with phosphate buffer solution (PBS) or Candida that were subjected to sham or cecal ligation and puncture (CLP) as determined by survival analysis (A), kidney and liver injury (B,C), serum cytokines (D–F), colon cytokine (G–I), gut leakage by FITC–dextran (J), endotoxemia (K), and serum (1→3)-β-D-glucan (L; n = 6–8/group) are demonstrated. *p < 0.05; **p < 0.001; #p < 0.05; ##p < 0.001.
In addition, mice in the HFD-CLP group treated with Candida exhibited more severe sepsis as determined by increased mortality, liver injury, serum cytokines, colon inflammation, gut leakage, endotoxemia, and glucanemia, but not serum creatinine when compared with mice in the HFD-CLP group that were not treated with Candida (Figures 6A–L). In mice fed a HFD but not subjected to CLP, Candida did not worsen obesity-induced liver injury, gut leakage, and serum cytokines, but it did activate local inflammation (colon TNF-α and IL-6; Figures 6C–H) without diarrhea when compared with mice in the HFD group that were not treated with Candida. This implies healthy mucosal barriers in non-CLP mice. Without sepsis, there were non-different fecal total Gram-negative bacteria (increased Bacteroides but decreased Halomonas spp.; Figure 7) and gut leakage (Figure 6I) when comparing between HFD mice with versus without Candida.
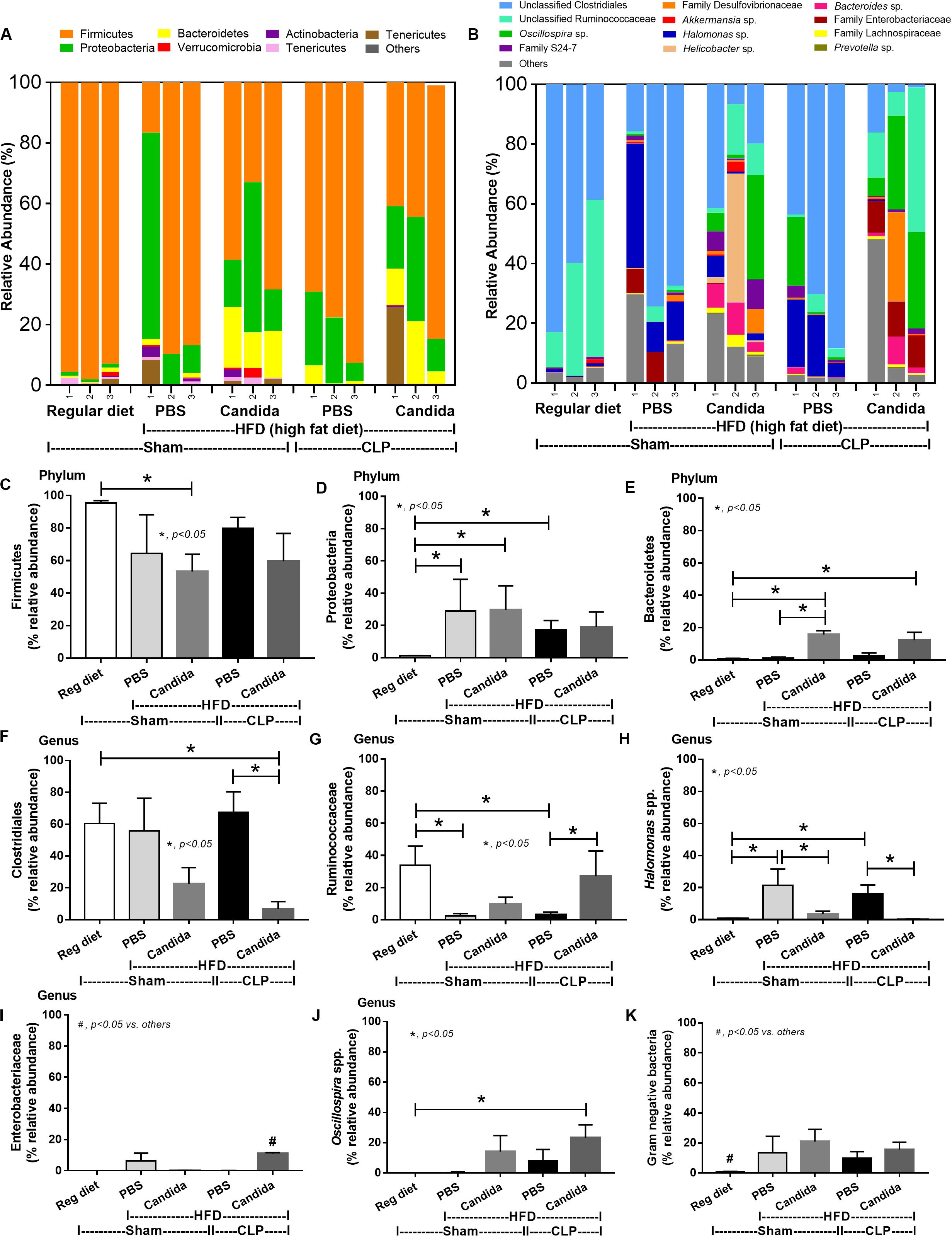
Figure 7. Gut microbiota analysis from feces of mice fed a regular diet that were subjected to sham surgery and mice fed a high-fat diet (HFD) treated with phosphate buffer solution (PBS) or Candida that were subjected to sham or cecal ligation and puncture (CLP) by relative abundance of bacterial diversity at phylum (A) and at genus (B) with the better visualization (C–J) and abundance of total Gram-negative bacteria determined from phylum (K) are demonstrated. *p < 0.05; #p < 0.05.
Of note, mice fed a HFD but not subjected to CLP-induced dysbiosis were demonstrated by increased total fecal Gram-negative bacteria (Proteobacteria and Halomonas spp.) with reduced beneficial Gram-positive anaerobes (Ruminococcaceae) when compared with mice in the regular diet group that were not treated with Candida (Figure 7). Mice in the HFD-CLP group treated with Candida demonstrated no change in total Gram-negative bacteria with increased Enterobacteriaceae, pathogenic Gram-negative aerobes (51, 52) in comparison with mice in the HFD-CLP group that were not treated with Candida (Figure 7). Only slight alterations in bacterial diversity were demonstrated between HFD versus regular diet with CLP (non-Candida) and between HFD with versus without Candida (Supplementary Figures 1A–F).
Additive Effect Between Endotoxin and (1→3)-β-D-Glucan Toward Hepatocytes and Macrophages
To provide mechanistic data for the previously described phenomena, we studied the interactions of LPS, BG, and PA on inflammation (53, 54) and mitochondrial function in hepatocytes (HepG2) and macrophages (RAW264.7 cells) (31). As such, PA, a representative saturated fatty acid, induced lipid accumulation, mild cytokine production, and amplified cytokine responses in HepG2 cells after stimulation with LPS plus BG (LPS + BG; Figures 8A–E). Supernatant cytokines of LPS + BG activated hepatocytes were suppressed by Dectin-1 inhibitor (Figures 8F–H) implying Dectin-1-dependent signaling. Although BG activation with or without PA induced only mild cytokine responses, BG was an effective adjuvant for LPS stimulation as LPS + BG induced higher cytokine production compared with LPS alone (Figures 8C–E). In addition, the separated activation by LPS, BG, or PA in HepG2 cells showed a tendency of reduced mitochondrial respiration compared with media control, but it did not reach a significant level (Figures 8I,J). Meanwhile, LPS + BG significantly reduced glycolysis capacity (glycolysis activity during mitochondrial cessation) and respiratory capacity (mitochondria activity during glycolysis blocking) compared with media control (Figures 8I,J). However, an addition of PA into LPS + BG could not alter hepatocyte energy metabolism when compared with LPS + BG (Figures 8I,J). In macrophages, PA enhanced lipid accumulation and increased supernatant cytokines of LPS or LPS + BG activation when compared with the conditions without PA (Figures 9A–D). The activation by PA + LPS + BG in macrophages demonstrated the most prominent cytokine responses (Figures 9A–D). Furthermore, Dectin-1 inhibitor reduced macrophage responses against LPS + BG (Figures 9E–G). These data support the possible systemic inflammatory effect of LPS and BG from gut translocation against both hepatocytes and macrophages.
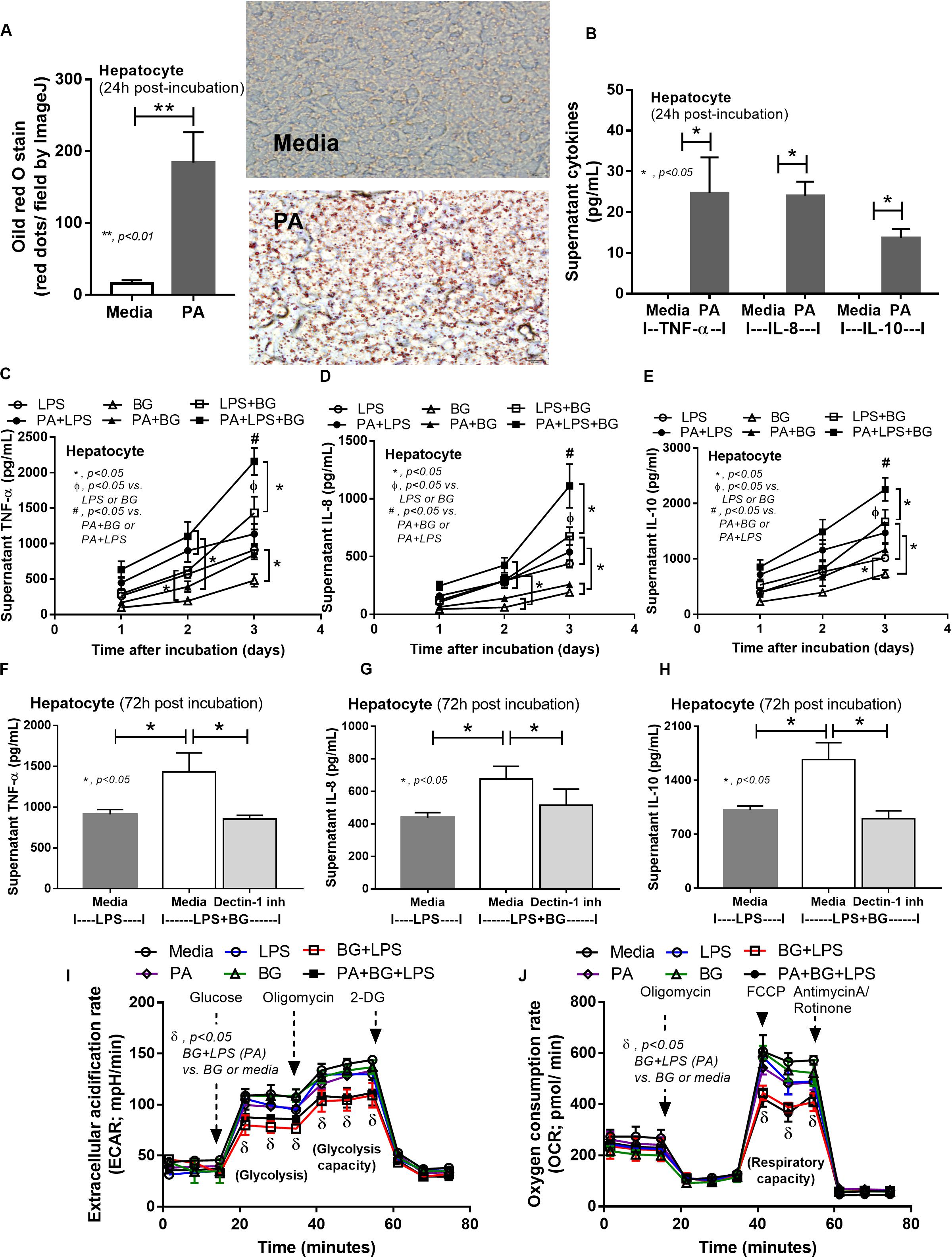
Figure 8. Intracellular lipid accumulation by Oil Red O staining with representative pictures and supernatant cytokines in HepG2 cells (hepatocytes) after activation by palmitic acid (PA), a representative saturated fatty acid, or media control (A,B), supernatant cytokines from PA-activated hepatocytes with endotoxin (LPS), (1→3)-β-D-glucan (BG), or LPS plus BG (LPS + BG; C–E), supernatant cytokines with or without Dectin-1 inhibitor (F–H), and extracellular flux analysis pattern at 48 h of several activations (I,J) are demonstrated. 2-DG, 2-Deoxy-D-glucose; FCCP, carbonyl cyanide-4-(trifluoromethoxy) phenylhydrazone (independent triplicate experiments were performed). *p < 0.05; **p < 0.01; #p < 0.05.
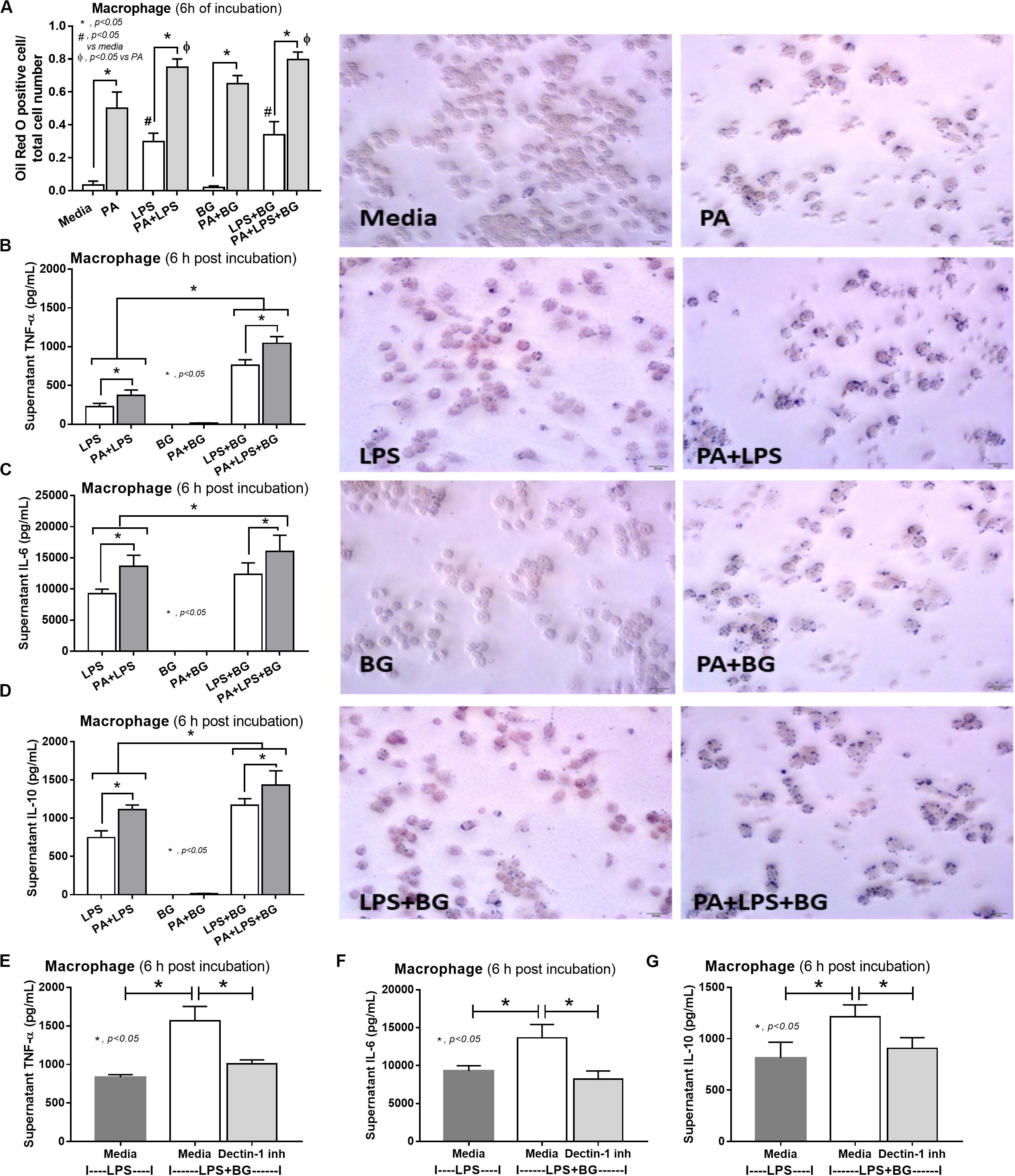
Figure 9. Characteristics of RAW264.7 cells (macrophages) after 6-h incubation of palmitic acid (PA), a representative saturated fatty acid, or media control together with endotoxin (LPS), (1→3)-β-D-glucan (BG), or LPS plus BG (LPS + BG) as determined by intracellular lipid accumulation by Oil Red O staining with representative pictures (A), supernatant cytokines (B–D), and supernatant cytokines with or without Dectin-1 inhibitor (E–G) are demonstrated (independent triplicate experiments were performed). *p < 0.05; #p < 0.05.
Probiotic Attenuates Sepsis Severity in Obese Mice, Regardless of Candida Administration
Although L34 neither induced diarrhea (data not shown) nor improved obesity complications (Figure 10), L34 attenuated CLP severity in HFD mice regardless of Candida administration as determined by survival, organ injury, serum cytokines, colon inflammation, gut leakage, and fecal fungal burdens (Figures 11A–L) partly through amelioration of gut dysbiosis. Accordingly, in mice fed a HFD-Candida but not subjected to CLP, L34 reduced fecal fungal burdens (Figure 11M) and increased Ruminococcaceae bacteria, a beneficial short-chain fatty acid–producing bacterial group (55, 56), without an effect on total fecal Gram-negative bacteria (Figure 12). In the HFD-CLP group that were not treated with Candida, L34 reduced total Gram-negative bacteria in feces, especially Proteobacteria in Halomonas spp. (Figure 13). On the other hand, L34 reduced fecal fungi and Enterobacteriaceae bacteria, pathogenic Gram-negative aerobe, without an effect on total fecal Gram-negative bacteria in the HFD-CLP group treated with Candida (Figure 13). However, L34 did not alter bacterial diversity index (Supplementary Figures 1G–L). Of note, the rarefaction curves are demonstrated in the microbiome analysis data (Supplementary Figure 1M).
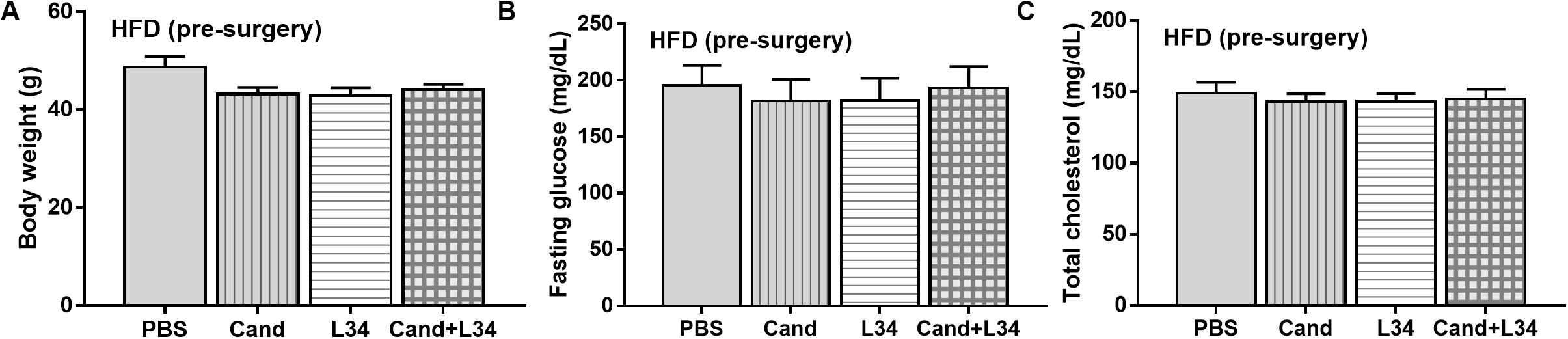
Figure 10. Characteristics of mice fed a high-fat diet (HFD) treated with phosphate buffer solution (PBS) or Candida with or without Lactobacillus rhamnosus L34 (L34) at 5th month of the experiments (before cecal ligation and puncture operation) as determined by body weight (A), fasting glucose and total cholesterol in blood (B,C; n = 6–8/group) are demonstrated.
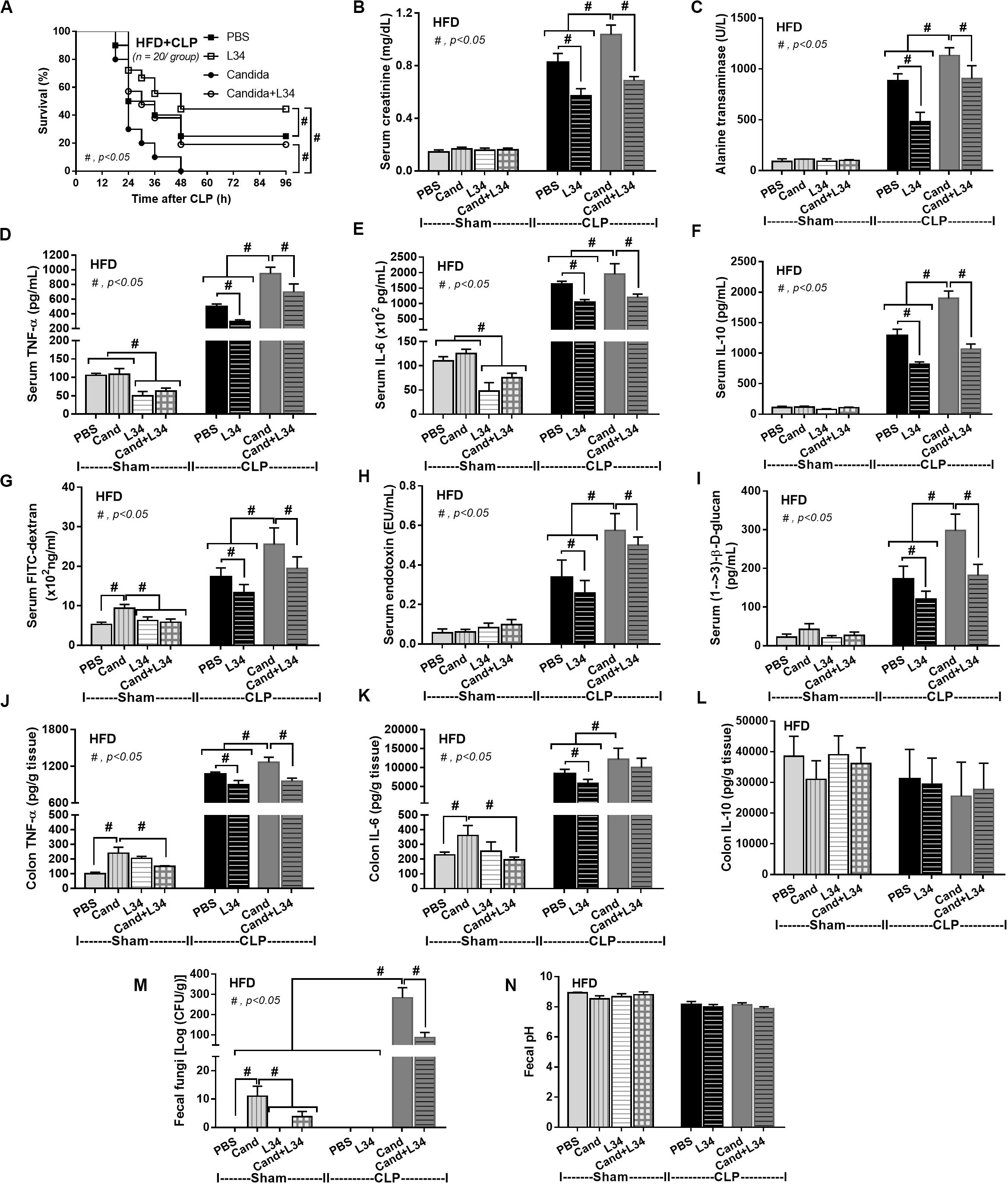
Figure 11. Characteristics of mice fed a high-fat diet (HFD) treated with phosphate buffer solution (PBS) or Candida with or without Lactobacillus rhamnosus L34 (L34) that were subjected to sham or cecal ligation and puncture (CLP) surgery as determined by survival analysis (A), kidney and liver injury (B,C), serum cytokines (D–F), gut leakage by FITC–dextran (G), endotoxemia (H), serum (1→3)-β-D-glucan (I), cytokines from ascending colon (J–L), fecal fungal burdens and fecal pH (M, N; n = 6–8/group for B–N) are demonstrated. #p < 0.05.
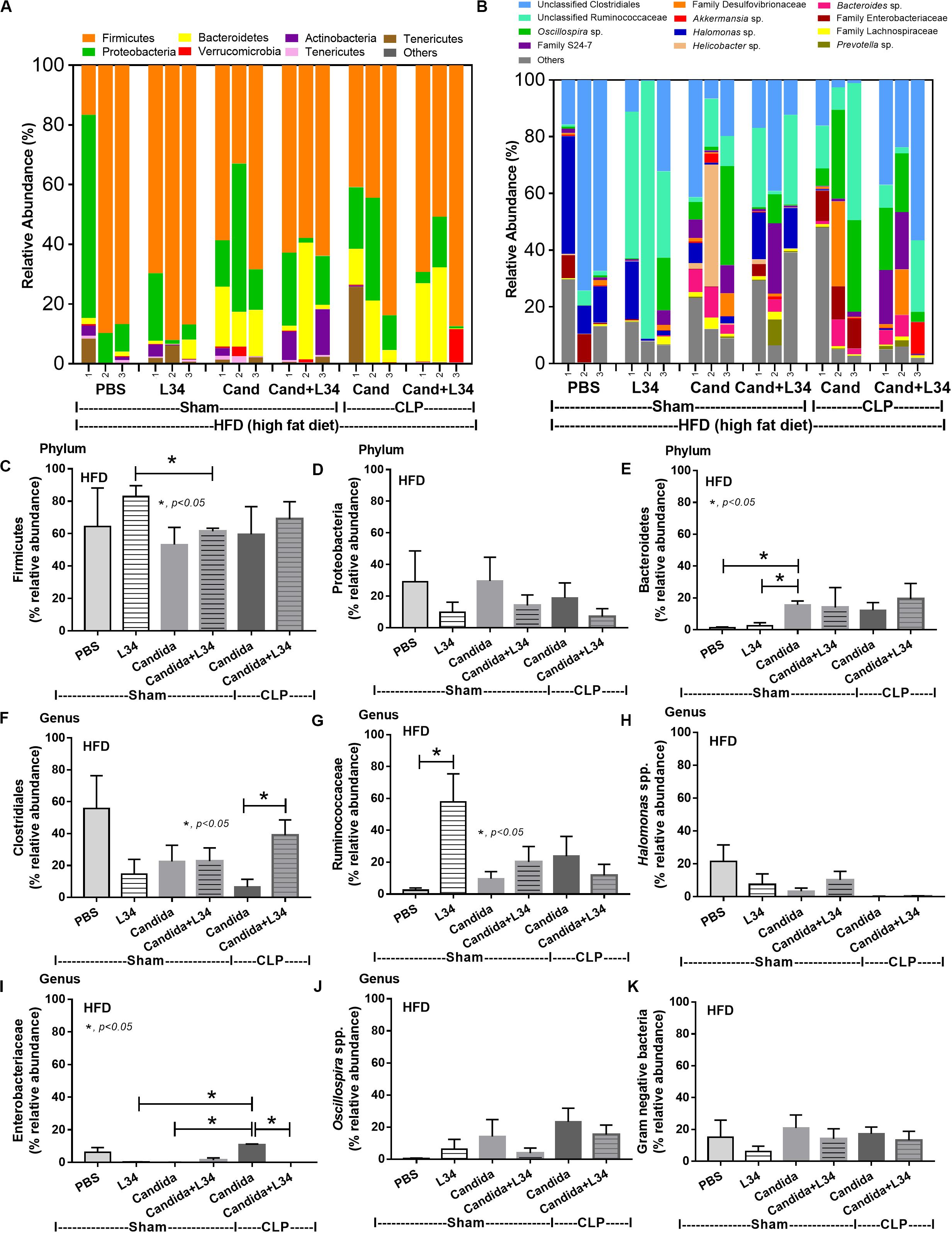
Figure 12. Gut microbiota analysis from feces of mice fed a high-fat diet (HFD) treated with phosphate buffer solution (PBS) or Candida with or without Lactobacillus rhamnosus L34 (L34) that were subjected to sham or cecal ligation and puncture (CLP) surgery by relative abundance of bacterial diversity at phylum (A) and at genus (B) with better visualization (C–J) and abundance of total Gram-negative bacteria determined from phylum (K) are demonstrated. *p < 0.05.
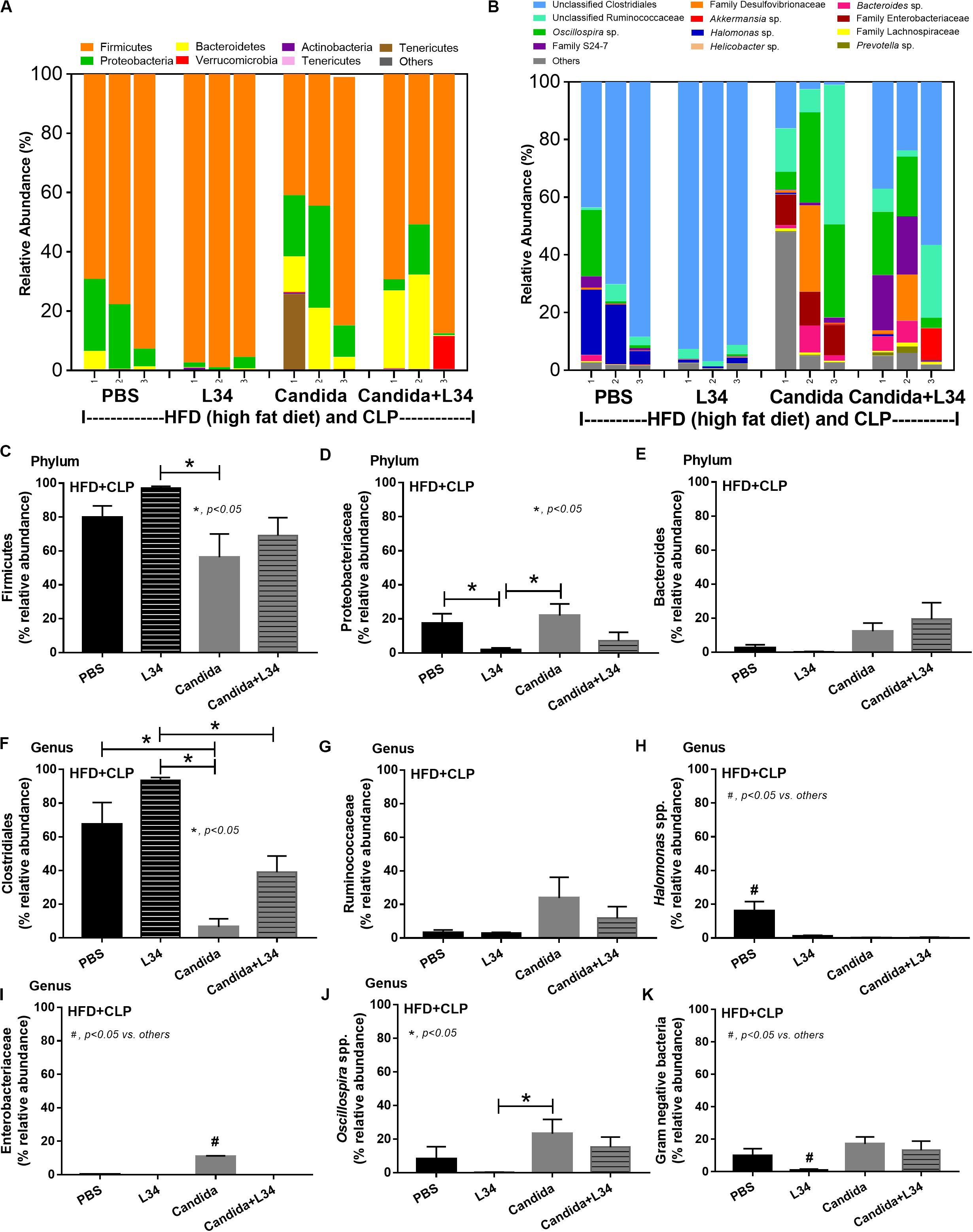
Figure 13. Gut microbiota analysis from feces of mice fed a high-fat diet (HFD) treated with phosphate buffer solution (PBS) or Candida with or without Lactobacillus rhamnosus L34 (L34) that were subjected to cecal ligation and puncture (CLP) surgery by relative abundance of bacterial diversity at phylum (A) and at genus (B) with the better visualization (C–J) and abundance of total Gram-negative bacteria determined from phylum (K) are demonstrated. *p < 0.05; #p < 0.05.
Discussion
Because C. albicans in mouse feces are detectable only by PCR (10), but not by culture (25) and differs from the human condition (26), the influence of C. albicans is evaluated through C. albicans administration. Here, Candida pretreatment in obese mice worsened sepsis through enhanced systemic inflammation induced by LPS and BG from gut translocation which implies the importance of gut fungi toward sepsis in obesity.
Impact on Gut Leakage and Gut Dysbiosis of Candida in Sepsis-Obese Mice
Endotoxemia (57) in obesity (without sepsis) as a result of HFD increased fecal Gram-negative bacteria (58, 59) that is enhanced by sepsis-induced gut leakage has been previously mentioned (60–62). Here, several patterns of bacterial dysbiosis in obese mice in comparison with regular-diet mice were demonstrated including (1) increased total Gram-negative bacteria in mice fed a HFD without Candida and not subjected to CLP (Figure 4K), (2) increased Bacteroides, Gram-negative anaerobes in several pathogenic conditions (63), in mice fed a HFD-Candida but not subjected to CLP (Figure 7E), (3) increased pathogenic bacteria (Clostridiales) in HFD-CLP mice that were not treated with Candida (Figure 4F), and (4) increased mucosal-invasive pathogenic bacteria, Enterobacteriaceae (49, 52, 64), in HFD-CLP mice treated with Candida. In addition, CLP also increased Candida burdens in feces compared with CLP non-Candida and supported the impact of mucosal-immunity defect in sepsis (17, 65–67). Although Candida gavage in healthy mice did not increase fecal fungi, gut Candida induced local gut inflammation without gut leakage. Hence, intestinal Candida could enhance gut leakage in obese-sepsis mice from both direct Candida mucosal damage and indirect injury through Candida-induced bacterial gut dysbiosis.
Enhanced Inflammatory Responses of Candida in Sepsis-Obese Mice and Role of Saturated Fatty Acid
During gut leakage, intestinal Candida increases BG in gut contents that could be delivered to the liver and lymphatic system (31). In hepatocytes, an additive inflammatory effect of LPS was amplified by BG through the activation on Dectin-1, a receptor for BG, as the amplification was neutralized by a Dectin-1 inhibitor. In addition, LPS + BG, but not in separation, reduced the capacity of glycolysis and mitochondria function in hepatocytes which might be associated with a significant hepatocyte injury (68, 69). It is interesting to note that the property of LPS and BG from different organisms might be different. This includes the quantity of lipid A, an LPS conserved lipid region with the pro-inflammatory property (70, 71) and BG molecular size (72). Here, LPS and BG from E. coli and Pachyman, respectively, were used as proof-of-concept experiments which might be different from other representative molecules. There has been a previous report that LPS E. coli K12 significantly reduce mitochondrial function in HEPG2 cells (73). Meanwhile, LPS E. coli 026:B6 in our experiments showed only a tendency of reduction. Despite this limitation, LPS + BG altered cytokine responses and cell energy metabolism in hepatocytes enhanced by saturated fatty acid. This supports HFD-induced metabolic pro-inflammation (53, 54, 74). Saturated fatty acid alone did not alter cell energy metabolism of hepatocytes. Moreover, additive effects of BG on LPS that are enhanced by saturated fatty acids have been also observed in macrophages in the current study and in other publications (22–24, 75). However, extracellular flux analysis in macrophages was not performed here due to well-known LPS-enhanced glycolysis (76). Our data support that saturated fatty acids, which are absorbed through portal vein (77), enhances LPS activity in hepatocytes and macrophages (53, 54) and induced cytokine production (78). This suggests the inflammatory aggravating property of dietary saturated fatty acids on LPS + BG in sepsis with obesity.
Probiotic Treatment in Sepsis, the Attenuation of Gut Dysbiosis, and Gut Leakage
Administration of L34 attenuated sepsis severity in obese mice with and without Candida, at least in part, through the reduced severity of gut leakage and gut dysbiosis. Here, several patterns of the attenuation of gut dysbiosis by L34 were demonstrated including (1) increased Ruminococcaceae which is a beneficial butyrate (short-chain fatty acid)–producing bacterial group (55, 56), in mice fed a HFD without Candida but not subjected to CLP; (2) reduced fecal Gram-negative bacteria which are a source of LPS in gut contents in HFD-CLP mice that were not treated with Candida; and (3) reduced pathogenic Enterobacteriaceae (79) and fungi in HFD-CLP mice treated with Candida. In translation, manipulation of gut leakage and/or fungal burdens by probiotics should be one interesting strategy against sepsis in obesity. However, several limitations on the similarity to patient obesity should be mentioned: (1) ingestion of 60% saturated fat diet is higher than most of the regular diets in human (80), (2) oral gavage also induced stress that might different from obesity in patients (81, 82), and (3) the dose of probiotics that is equivalent to human weight is 2 × 1012 CFU/dose that possibly induces some adverse effects (83). More studies in patients are warranted.
In conclusion, obesity and Candida administration enhanced sepsis severity through gut dysbiosis–induced gut leakage and saturated fatty acid–amplified pathogen-associated molecules induced inflammation which could be attenuated by probiotics (Figure 14).
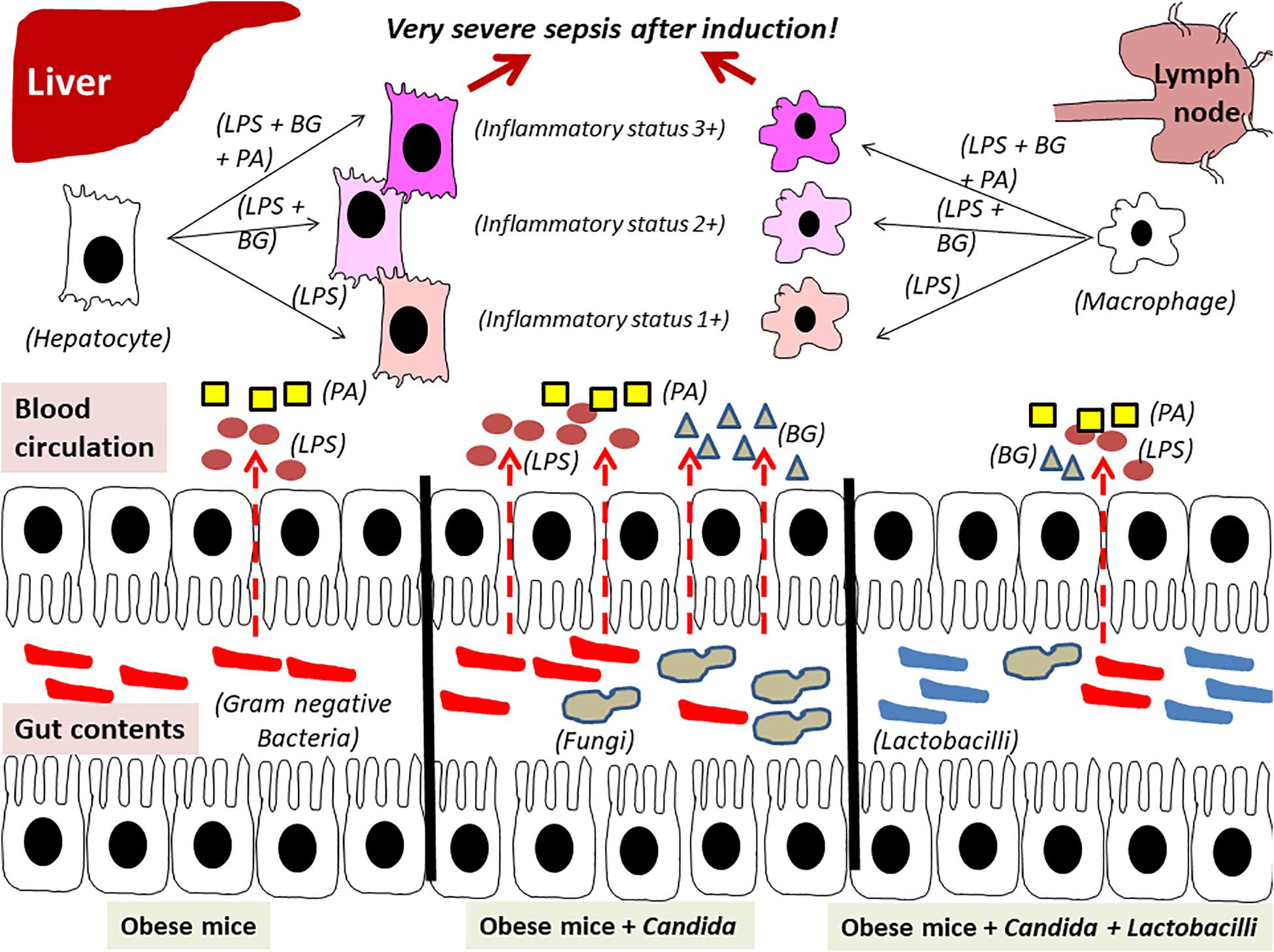
Figure 14. The proposed hypothesis demonstrates gut leakage in mice fed a high-fat diet (HFD) treated with Candida is more severe than mice fed a HFD that were not treated with Candida due to prominent gut translocation of lipopolysaccharide (LPS) and (1→3)-β-D-glucan (BG), a major cell wall component of Gram-negative bacteria and Candida, respectively, that are delivered to liver and systemic circulation (31). Additive effect of LPS with BG (LPS + BG) on hepatocytes and macrophages is amplified by palmitic acid (PA), a pro-inflammatory saturated fatty acid, resulting in higher inflammatory status that enhances sepsis severity. Meanwhile, Lactobacilli spp. attenuate gut dysbiosis, gut leakage, systemic inflammation, and sepsis severity (dotted line is gut translocation of LPS and BG).
Data Availability Statement
The raw data supporting the conclusions of this article will be made available by the authors, without undue reservation, to any qualified researcher.
Ethics Statement
The animal study was reviewed and approved by The Institutional Animal Care and Use Committee of the Faculty of Medicine, Chulalongkorn University, Bangkok, Thailand (SST 04/2561).
Author Contributions
WP designed and coordinated all the experiments, performed in vitro and in vivo experiments, and wrote the manuscript and approved. VS performed microbiome analysis and approved the manuscript. PC performed microbiome analysis and approved the manuscript. TO performed in vitro experiments and approved the manuscript. CD performed in vitro experiments and approved the manuscript. SP supervised microbiome analysis and approved the manuscript. ST supervised the in vitro experiment and also provided the probiotic in this study. AL designed and coordinated all the experiments, analyzed all of these experiment, and wrote the manuscript and approved. All authors contributed to the article and approved the submitted version.
Funding
This study was supported by Thailand Government Fund (RSA-6080023), Thailand Research Fund (RES_61_202_30_022), and Ratchadaphiseksomphot Endowment Fund 2017 (76001-HR). WP was supported by Rachadapisek Sompote Fund for Postdoctoral Fellowship, Chulalongkorn University.
Conflict of Interest
The authors declare that the research was conducted in the absence of any commercial or financial relationships that could be construed as a potential conflict of interest.
Supplementary Material
The Supplementary Material for this article can be found online at: https://www.frontiersin.org/articles/10.3389/fimmu.2020.561652/full#supplementary-material
Supplementary Figure 1 | Alpha diversity by Chao 1 richness estimation and Shannon evenness, analysis with β-diversity plot of mice in different groups, including regular diet or high-fat diet (HFD) after sham or cecal ligation and puncture (CLP; A–C), sham regular diet versus sham or CLP of HFD with Candida or phosphate buffer solution (PBS; D–F), sham or CLP in HFD with PBS, Lactobacillus rhamnosus L34 (L34), Candida with or without L34 (G–I), CLP and HFD (HFD + CLP) with PBS or Candida with or without L34 (J–L), and rarefaction curves of microbiome analysis (M) are demonstrated. OTUs, operational taxonomic units; PC, principal component.
References
1. Levy MM, Fink MP, Marshall JC, Abraham E, Angus D, Cook D, et al. 2001 SCCM/ESICM/ACCP/ATS/SIS International sepsis definitions conference. Intensive Care Med. (2003) 29:530–8. doi: 10.1007/s00134-003-1662-x
2. Singer M, Deutschman CS, Seymour CW, Shankar-Hari M, Annane D, Bauer M, et al. The third international consensus definitions for sepsis and septic shock (Sepsis-3). JAMA. (2016) 315:801–10. doi: 10.1001/jama.2016.0287
3. Mayr FB, Yende S, Angus DC. Epidemiology of severe sepsis. Virulence. (2014) 5:4–11. doi: 10.4161/viru.27372
4. Collaborators GBDO, Afshin A, Forouzanfar MH, Reitsma MB, Sur P, Estep K, et al. Health effects of overweight and obesity in 195 countries over 25 years. N Engl J Med. (2017) 377:13–27. doi: 10.1056/NEJMoa1614362
5. Allison DB, Fontaine KR, Manson JE, Stevens J, VanItallie TB. Annual deaths attributable to obesity in the United States. JAMA. (1999) 282:1530–8. doi: 10.1001/jama.282.16.1530
6. Bercault N, Boulain T, Kuteifan K, Wolf M, Runge I, Fleury JC. Obesity-related excess mortality rate in an adult intensive care unit: a risk-adjusted matched cohort study. Crit Care Med. (2004) 32:998–1003. doi: 10.1097/01.ccm.0000119422.93413.08
7. Ross PA, Newth CJ, Leung D, Wetzel RC, Khemani RG. Obesity and mortality risk in critically Ill children. Pediatrics. (2016) 137:e20152035. doi: 10.1542/peds.2015-2035
8. Kolyva AS, Zolota V, Mpatsoulis D, Skroubis G, Solomou EE, Habeos IG, et al. The role of obesity in the immune response during sepsis. Nutr Diabetes. (2014) 4:e137. doi: 10.1038/nutd.2014.34
9. Singer G, Stokes KY, Terao S, Granger DN. Sepsis-induced intestinal microvascular and inflammatory responses in obese mice. Shock. (2009) 31:275–9. doi: 10.1097/shk.0b013e3181834ab3
10. Heisel T, Montassier E, Johnson A, Al-Ghalith G, Lin YW, Wei LN, et al. High-fat diet changes fungal microbiomes and interkingdom relationships in the murine gut. mSphere. (2017) 2:e00351-17. doi: 10.1128/mSphere.00351-17
11. Murphy EA, Velazquez KT, Herbert KM. Influence of high-fat diet on gut microbiota: a driving force for chronic disease risk. Curr Opin Clin Nutr Metab Care. (2015) 18:515–20. doi: 10.1097/MCO.0000000000000209
12. Ni YN, Luo J, Yu H, Wang YW, Hu YH, Liu D, et al. Can body mass index predict clinical outcomes for patients with acute lung injury/acute respiratory distress syndrome? A meta-analysis. Crit Care. (2017) 21:36. doi: 10.1186/s13054-017-1615-3
13. Sakr Y, Alhussami I, Nanchal R, Wunderink RG, Pellis T, Wittebole X, et al. Being overweight is associated with greater survival in ICU patients: results from the intensive care over nations audit. Crit Care Med. (2015) 43:2623–32. doi: 10.1097/CCM.0000000000001310
14. Leelahavanichkul A, Worasilchai N, Wannalerdsakun S, Jutivorakool K, Somparn P, Issara-Amphorn J, et al. Gastrointestinal leakage detected by serum (1–>3)-beta-D-glucan in mouse models and a pilot study in patients with sepsis. Shock. (2016) 46:506–18. doi: 10.1097/SHK.0000000000000645
15. Samonis G, Gikas A, Toloudis P, Maraki S, Vrentzos G, Tselentis Y, et al. Prospective study of the impact of broad-spectrum antibiotics on the yeast flora of the human gut. Eur J Clin Microbiol Infect Dis. (1994) 13:665–7. doi: 10.1007/BF01973996
16. Yang AM, Inamine T, Hochrath K, Chen P, Wang L, Llorente C, et al. Intestinal fungi contribute to development of alcoholic liver disease. J Clin Invest. (2017) 127:2829–41. doi: 10.1172/JCI90562
17. Lang S, Duan Y, Liu J, Torralba MG, Kuelbs C, Ventura-Cots M, et al. Intestinal fungal dysbiosis and systemic immune response to fungi in patients with alcoholic hepatitis. Hepatology. (2020) 71:522–38. doi: 10.1002/hep.30832
18. Saltzman ET, Palacios T, Thomsen M, Vitetta L. Intestinal microbiome shifts, dysbiosis, inflammation, and non-alcoholic fatty liver disease. Front Microbiol. (2018) 9:61. doi: 10.3389/fmicb.2018.00061
19. Blasi E, Pitzurra L, Puliti M, Mazzolla R, Barluzzi R, Saleppico S, et al. Different events involved in the induction of macrophage tumor necrosis factor by Candida albicans and lipopolysaccharide. Cell Immunol. (1994) 157:501–9. doi: 10.1006/cimm.1994.1245
20. Panpetch W, Somboonna N, Bulan DE, Issara-Amphorn J, Finkelman M, Worasilchai N, et al. Oral administration of live- or heat-killed Candida albicans worsened cecal ligation and puncture sepsis in a murine model possibly due to an increased serum (1–>3)-beta-D-glucan. PLoS One. (2017) 12:e0181439. doi: 10.1371/journal.pone.0181439
21. Panpetch W, Somboonna N, Bulan DE, Issara-Amphorn J, Worasilchai N, Finkelman M, et al. Gastrointestinal colonization of Candida albicans increases serum (1–>3)-beta-D-glucan, without candidemia, and worsens cecal ligation and puncture sepsis in murine model. Shock. (2018) 49:62–70. doi: 10.1097/SHK.0000000000000896
22. Ferwerda G, Meyer-Wentrup F, Kullberg BJ, Netea MG, Adema GJ. Dectin-1 synergizes with TLR2 and TLR4 for cytokine production in human primary monocytes and macrophages. Cell Microbiol. (2008) 10:2058–66. doi: 10.1111/j.1462-5822.2008.01188.x
23. Kikkert R, Bulder I, de Groot ER, Aarden LA, Finkelman MA. Potentiation of Toll-like receptor-induced cytokine production by (1–>3)-beta-D-glucans: implications for the monocyte activation test. J Endotoxin Res. (2007) 13:140–9. doi: 10.1177/0968051907080024
24. Engstad CS, Engstad RE, Olsen JO, Osterud B. The effect of soluble beta-1,3-glucan and lipopolysaccharide on cytokine production and coagulation activation in whole blood. Int Immunopharmacol. (2002) 2:1585–97. doi: 10.1016/s1567-5769(02)00134-0
25. Koh AY. Murine models of Candida gastrointestinal colonization and dissemination. Eukaryot Cell. (2013) 12:1416–22. doi: 10.1128/EC.00196-13
26. Borges FM, de Paula TO, Sarmiento MRA, de Oliveira MG, Pereira MLM, Toledo IV, et al. Fungal diversity of human gut microbiota among eutrophic, overweight, and obese individuals based on aerobic culture- dependent approach. Curr Microbiol. (2018) 75:726–35. doi: 10.1007/s00284-018-1438-8
27. Panpetch W, Hiengrach P, Nilgate S, Tumwasorn S, Somboonna N, Wilantho A, et al. Additional Candida albicans administration enhances the severity of dextran sulfate solution induced colitis mouse model through leaky gut-enhanced systemic inflammation and gut-dysbiosis but attenuated by Lactobacillus rhamnosus L34. Gut Microbes. (2019) 11:465–80. doi: 10.1080/19490976.2019.1662712
28. Hiengrach P, Panpetch W, Worasilchai N, Chindamporn A, Tumwasorn S, Jaroonwitchawan T, et al. Administration of Candida albicans to dextran sulfate solution treated mice causes intestinal dysbiosis, emergence and dissemination of intestinal Pseudomonas aeruginosa and lethal sepsis. Shock. (2020) 53:189–98. doi: 10.1097/SHK.0000000000001339
29. Lobo LA, Benjamim CF, Oliveira AC. The interplay between microbiota and inflammation: lessons from peritonitis and sepsis. Clin Transl Immunol. (2016) 5:e90. doi: 10.1038/cti.2016.32
30. Dickson RP. The microbiome and critical illness. Lancet Respir Med. (2016) 4:59–72. doi: 10.1016/S2213-2600(15)00427-0
31. Amornphimoltham P, Yuen PST, Star RA, Leelahavanichkul A. Gut leakage of fungal-derived inflammatory mediators: part of a gut-liver-kidney axis in bacterial sepsis. Dig Dis Sci. (2019) 64:2416–28. doi: 10.1007/s10620-019-05581-y
32. Scaldaferri F, Gerardi V, Lopetuso LR, Del Zompo F, Mangiola F, Boskoski I, et al. Gut microbial flora, prebiotics, and probiotics in IBD: their current usage and utility. Biomed Res Int. (2013) 2013:435268. doi: 10.1155/2013/435268
33. Hager CL, Ghannoum MA. The mycobiome: role in health and disease, and as a potential probiotic target in gastrointestinal disease. Dig Liver Dis. (2017) 49:1171–6. doi: 10.1016/j.dld.2017.08.025
34. Zuo T, Ng SC. The gut microbiota in the pathogenesis and therapeutics of inflammatory bowel disease. Front Microbiol. (2018) 9:2247. doi: 10.3389/fmicb.2018.02247
35. Yadav H, Lee JH, Lloyd J, Walter P, Rane SG. Beneficial metabolic effects of a probiotic via butyrate-induced GLP-1 hormone secretion. J Biol Chem. (2013) 288:25088–97. doi: 10.1074/jbc.M113.452516
36. Panpetch W, Chancharoenthana W, Bootdee K, Nilgate S, Finkelman M, Tumwasorn S, et al. Lactobacillus rhamnosus L34 attenuates gut translocation-induced bacterial sepsis in murine models of leaky gut. Infect Immun. (2018) 86:IAI.00700-17. doi: 10.1128/IAI.00700-17
37. Huffnagle GB, Noverr MC. The emerging world of the fungal microbiome. Trends Microbiol. (2013) 21:334–41. doi: 10.1016/j.tim.2013.04.002
38. Boonma P, Spinler JK, Venable SF, Versalovic J, Tumwasorn S. Lactobacillus rhamnosus L34 and Lactobacillus casei L39 suppress Clostridium difficile-induced IL-8 production by colonic epithelial cells. BMC Microbiol. (2014) 14:177. doi: 10.1186/1471-2180-14-177
39. Pratchayasakul W, Kerdphoo S, Petsophonsakul P, Pongchaidecha A, Chattipakorn N, Chattipakorn SC. Effects of high-fat diet on insulin receptor function in rat hippocampus and the level of neuronal corticosterone. Life Sci. (2011) 88:619–27. doi: 10.1016/j.lfs.2011.02.003
40. Leelahavanichkul A, Panpetch W, Worasilchai N, Somparn P, Chancharoenthana W, Nilgate S, et al. Evaluation of gastrointestinal leakage using serum (1–>3)-beta-D-glucan in a Clostridium difficile murine model. FEMS Microbiol Lett. (2016) 363:fnw204. doi: 10.1093/femsle/fnw204
41. Savari F, Mard SA, Badavi M, Rezaie A, Gharib-Naseri MK. A new method to induce nonalcoholic steatohepatitis (NASH) in mice. BMC Gastroenterol. (2019) 19:125. doi: 10.1186/s12876-019-1041-x
42. Wu L, Wen C, Qin Y, Yin H, Tu Q, Van Nostrand JD, et al. Phasing amplicon sequencing on Illumina Miseq for robust environmental microbial community analysis. BMC Microbiol. (2015) 15:125. doi: 10.1186/s12866-015-0450-4
43. Bolyen E, Rideout JR, Dillon MR, Bokulich NA, Abnet CC, Al-Ghalith GA, et al. Reproducible, interactive, scalable and extensible microbiome data science using QIIME 2. Nat Biotechnol. (2019) 37:852–7. doi: 10.1038/s41587-019-0209-9
44. Rognes T, Flouri T, Nichols B, Quince C, Mahé F. VSEARCH: a versatile open source tool for metagenomics. PeerJ. (2016) 4:e2584. doi: 10.7717/peerj.2584
45. Edgar RC, Haas BJ, Clemente JC, Quince C, Knight R. UCHIME improves sensitivity and speed of chimera detection. Bioinformatics. (2011) 27:2194–200. doi: 10.1093/bioinformatics/btr381
46. DeSantis TZ, Hugenholtz P, Larsen N, Rojas M, Brodie EL, Keller K, et al. Greengenes, a chimera-checked 16S rRNA gene database and workbench compatible with ARB. Appl Environ Microbiol. (2006) 72:5069–72. doi: 10.1128/AEM.03006-05
47. Jaroonwitchawan T, Visitchanakun P, Dang PC, Ritprajak P, Palaga T, Leelahavanichkul A. Dysregulation of lipid metabolism in macrophages is responsible for severe endotoxin tolerance in FcgRIIB-deficient lupus mice. Front Immunol. (2020) 11:959. doi: 10.3389/fimmu.2020.00959
48. Ondee T, Gillen J, Visitchanakun P, Somparn P, Issara-Amphorn J, Dang Phi C, et al. Lipocalin-2 (Lcn-2) attenuates polymicrobial sepsis with LPS preconditioning (LPS tolerance) in FcGRIIb deficient lupus mice. Cells. (2019) 8:1064. doi: 10.3390/cells8091064
49. Liguori G, Lamas B, Richard ML, Brandi G, da Costa G, Hoffmann TW, et al. Fungal dysbiosis in mucosa-associated microbiota of Crohn’s disease patients. J Crohns Colitis. (2016) 10:296–305. doi: 10.1093/ecco-jcc/jjv209
50. Bien J, Palagani V, Bozko P. The intestinal microbiota dysbiosis and Clostridium difficile infection: is there a relationship with inflammatory bowel disease? Therap Adv Gastroenterol. (2013) 6:53–68. doi: 10.1177/1756283X12454590
51. Shin NR, Whon TW, Bae JW. Proteobacteria: microbial signature of dysbiosis in gut microbiota. Trends Biotechnol. (2015) 33:496–503. doi: 10.1016/j.tibtech.2015.06.011
52. Garrett WS, Gallini CA, Yatsunenko T, Michaud M, DuBois A, Delaney ML, et al. Enterobacteriaceae act in concert with the gut microbiota to induce spontaneous and maternally transmitted colitis. Cell Host Microbe. (2010) 8:292–300. doi: 10.1016/j.chom.2010.08.004
53. Korbecki J, Bajdak-Rusinek K. The effect of palmitic acid on inflammatory response in macrophages: an overview of molecular mechanisms. Inflamm Res. (2019) 68:915–32. doi: 10.1007/s00011-019-01273-5
54. Li Y, Lu Z, Ru JH, Lopes-Virella MF, Lyons TJ, Huang Y. Saturated fatty acid combined with lipopolysaccharide stimulates a strong inflammatory response in hepatocytes in vivo and in vitro. Am J Physiol Endocrinol Metab. (2018) 315:E745–57. doi: 10.1152/ajpendo.00015.2018
55. Julliand V, de Vaux A, Millet L, Fonty G. Identification of Ruminococcus flavefaciens as the predominant cellulolytic bacterial species of the equine cecum. Appl Environ Microbiol. (1999) 65:3738–41. doi: 10.1128/AEM.65.8.3738-3741.1999
56. Tomova A, Bukovsky I, Rembert E, Yonas W, Alwarith J, Barnard ND, et al. The effects of vegetarian and vegan diets on gut microbiota. Front Nutr. (2019) 6:47. doi: 10.3389/fnut.2019.00047
57. Boutagy NE, McMillan RP, Frisard MI, Hulver MW. Metabolic endotoxemia with obesity: is it real and is it relevant? Biochimie. (2016) 124:11–20. doi: 10.1016/j.biochi.2015.06.020
58. Zhang C, Zhang M, Pang X, Zhao Y, Wang L, Zhao L. Structural resilience of the gut microbiota in adult mice under high-fat dietary perturbations. ISME J. (2012) 6:1848–57. doi: 10.1038/ismej.2012.27
59. Hildebrandt MA, Hoffmann C, Sherrill-Mix SA, Keilbaugh SA, Hamady M, Chen YY, et al. High-fat diet determines the composition of the murine gut microbiome independently of obesity. Gastroenterology. (2009) 137:1716–24.e1–2. doi: 10.1053/j.gastro.2009.08.042
60. Fay KT, Klingensmith NJ, Chen CW, Zhang W, Sun Y, Morrow KN, et al. The gut microbiome alters immunophenotype and survival from sepsis. FASEB J. (2019) 33:11258–69. doi: 10.1096/fj.201802188R
61. Sertaridou E, Papaioannou V, Kolios G, Pneumatikos I. Gut failure in critical care: old school versus new school. Ann Gastroenterol. (2015) 28:309–22.
62. Hiltebrand LB, Krejci V, tenHoevel ME, Banic A. Sigurdsson GH. Redistribution of microcirculatory blood flow within the intestinal wall during sepsis and general anesthesia. Anesthesiology. (2003) 98:658–69. doi: 10.1097/00000542-200303000-00014
63. Bloom SM, Bijanki VN, Nava GM, Sun L, Malvin NP, Donermeyer DL, et al. Commensal Bacteroides species induce colitis in host-genotype-specific fashion in a mouse model of inflammatory bowel disease. Cell Host Microbe. (2011) 9:390–403. doi: 10.1016/j.chom.2011.04.009
64. Zeng MY, Inohara N, Nunez G. Mechanisms of inflammation-driven bacterial dysbiosis in the gut. Mucosal Immunol. (2017) 10:18–26. doi: 10.1038/mi.2016.75
65. Lam S, Zuo T, Ho M, Chan FKL, Chan PKS, Ng SC. Review article: fungal alterations in inflammatory bowel diseases. Aliment Pharmacol Ther. (2019) 50:1159–71. doi: 10.1111/apt.15523
66. Bishehsari F, Magno E, Swanson G, Desai V, Voigt RM, Forsyth CB, et al. Alcohol and gut-derived inflammation. Alcohol Res. (2017) 38:163–71.
67. Haussner F, Chakraborty S, Halbgebauer R, Huber-Lang M. Challenge to the intestinal mucosa during sepsis. Front Immunol. (2019) 10:891. doi: 10.3389/fimmu.2019.00891
68. Decleer M, Jovanovic J, Vakula A, Udovicki B, Agoua REK, Madder A, et al. Oxygen consumption rate analysis of mitochondrial dysfunction caused by Bacillus cereus cereulide in Caco-2 and HepG2 Cells. Toxins. (2018) 10:266. doi: 10.3390/toxins10070266
69. Nishikawa T, Bellance N, Damm A, Bing H, Zhu Z, Handa K, et al. A switch in the source of ATP production and a loss in capacity to perform glycolysis are hallmarks of hepatocyte failure in advance liver disease. J Hepatol. (2014) 60:1203–11. doi: 10.1016/j.jhep.2014.02.014
70. Lukiw WJ. Bacteroides fragilis Lipopolysaccharide and inflammatory signaling in Alzheimer’s disease. Front Microbiol. (2016) 7:1544. doi: 10.3389/fmicb.2016.01544
71. Ogawa T, Asai Y, Makimura Y, Tamai R. Chemical structure and immunobiological activity of Porphyromonas gingivalis lipid A. Front Biosci. (2007) 12:3795–812. doi: 10.2741/2353
72. Ruiz-Herrera J, Ortiz-Castellanos L. Cell wall glucans of fungi. A review. Cell Surf. (2019) 5:100022. doi: 10.1016/j.tcsw.2019.100022
73. Jeger V, Brandt S, Porta F, Jakob SM, Takala J, Djafarzadeh S. Dose response of endotoxin on hepatocyte and muscle mitochondrial respiration in vitro. Biomed Res Int. (2015) 2015:353074. doi: 10.1155/2015/353074
74. Duan Y, Zeng L, Zheng C, Song B, Li F, Kong X, et al. Inflammatory links between high fat diets and diseases. Front Immunol. (2018) 9:2649. doi: 10.3389/fimmu.2018.02649
75. Seong SK, Kim HW. Potentiation of innate immunity by beta-glucans. Mycobiology. (2010) 38:144–8. doi: 10.4489/MYCO.2010.38.2.144
76. Thapa B, Lee K. Metabolic influence on macrophage polarization and pathogenesis. BMB Rep. (2019) 52:360–72. doi: 10.5483/BMBRep.2019.52.6.140
77. Cabre E, Hernandez-Perez JM, Fluvia L, Pastor C, Corominas A, Gassull MA. Absorption and transport of dietary long-chain fatty acids in cirrhosis: a stable-isotope-tracing study. Am J Clin Nutr. (2005) 81:692–701. doi: 10.1093/ajcn/81.3.692
78. Joshi-Barve S, Barve SS, Amancherla K, Gobejishvili L, Hill D, Cave M, et al. Palmitic acid induces production of proinflammatory cytokine interleukin-8 from hepatocytes. Hepatology. (2007) 46:823–30. doi: 10.1002/hep.21752
79. Chen L, Li H, Li J, Chen Y, Yang Y. Lactobacillus rhamnosus GG treatment improves intestinal permeability and modulates microbiota dysbiosis in an experimental model of sepsis. Int J Mol Med. (2019) 43:1139–48. doi: 10.3892/ijmm.2019.4050
80. German JB, Dillard CJ. Saturated fats: what dietary intake? Am J Clin Nutr. (2004) 80:550–9. doi: 10.1093/ajcn/80.3.550
81. Balcombe JP, Barnard ND, Sandusky C. Laboratory routines cause animal stress. Contemp Top Lab Anim Sci. (2004) 43:42–51.
82. Brown AP, Dinger N, Levine BS. Stress produced by gavage administration in the rat. Contemp Top Lab Anim Sci. (2000) 39:17–21.
Keywords: intestinal Candida, obesity, high-fat diet, probiotics, cecal ligation and puncture, dysbiosis, gut leakage
Citation: Panpetch W, Sawaswong V, Chanchaem P, Ondee T, Dang CP, Payungporn S, Tumwasorn S and Leelahavanichkul A (2020) Candida Administration Worsens Cecal Ligation and Puncture-Induced Sepsis in Obese Mice Through Gut Dysbiosis Enhanced Systemic Inflammation, Impact of Pathogen-Associated Molecules From Gut Translocation and Saturated Fatty Acid. Front. Immunol. 11:561652. doi: 10.3389/fimmu.2020.561652
Received: 13 May 2020; Accepted: 19 August 2020;
Published: 25 September 2020.
Edited by:
Alessio Fasano, Massachusetts General Hospital and Harvard Medical School, United StatesReviewed by:
Harry D. Dawson, Agricultural Research Service, United States Department of Agriculture, United StatesParameth Thiennimitr, Chiang Mai University, Thailand
Copyright © 2020 Panpetch, Sawaswong, Chanchaem, Ondee, Dang, Payungporn, Tumwasorn and Leelahavanichkul. This is an open-access article distributed under the terms of the Creative Commons Attribution License (CC BY). The use, distribution or reproduction in other forums is permitted, provided the original author(s) and the copyright owner(s) are credited and that the original publication in this journal is cited, in accordance with accepted academic practice. No use, distribution or reproduction is permitted which does not comply with these terms.
*Correspondence: Asada Leelahavanichkul, YWxlZWxhaGF2YW5pdEBnbWFpbC5jb20=; YV9sZWVsYWhhdmFuaXRAeWFob28uY29t