- 1GSK, Siena, Italy
- 2Medical Microbiology, University Medical Center Utrecht, Utrecht University, Utrecht, Netherlands
The skin is an immunocompetent tissue that harbors several kinds of immune cells and a plethora of commensal microbes constituting the skin microbiome. Staphylococcus aureus is a prominent skin pathogen that colonizes a large proportion of the human population. We currently have an incomplete understanding of the correlates of protection against S. aureus infection, however genetic and experimental evidence has shown that CD4+ T cells play a key role in orchestrating a protective anti-S. aureus immune response. A high S. aureus-specific memory CD4+ T cell response has been reported in the blood of healthy subjects. Since T cells are more abundant in the skin than in blood, we hypothesized that S. aureus-specific CD4+ T cells could be present in the skin of healthy individuals. Indeed, we observed proliferation of tissue-resident memory CD4+ T cells and production of IL-17A, IL-22, IFN-γ and TNF-β by cells isolated from abdominal skin explants in response to heat-killed S. aureus. Remarkably, these cytokines were produced also during an ex vivo epicutaneous S. aureus infection of human skin explants. These findings highlight the importance of tissue-resident memory CD4+ T cells present at barrier sites such as the skin, a primary entry site for S. aureus. Further phenotypical and functional characterization of these cells will ultimately aid in the development of novel vaccine strategies against this elusive pathogen.
Introduction
The skin provides a physical and immunological barrier for invading pathogens, while also maintaining symbiotic interactions with skin commensals. There are numerous specialized immune cells present in the skin that maintain skin homeostasis and act as the first line of defense against pathogens. It has been estimated that human skin contains roughly twice as many memory T cells than blood (1). Different memory T cell subsets can be phenotypically identified in human skin based on the presence of surface markers and the capacity to emigrate and enter the circulation. Tissue resident memory T (Trm) cells are a subset of memory T cells phenotypically and functionally distinct from their circulating counterparts (1, 2). In particular, human skin-resident memory T (Tsrm) cells can be identified through the surface expression of the skin-homing marker cutaneous lymphocyte-associated antigen (CLA), the memory T cell marker CD45RO and the tissue-retention marker CD69. CLA binds selectively and avidly to the vascular lectin E-selectin while CD69 prevents sphingosine-1-phosphate receptor 1 mediated egress from tissues into the circulation (3). Skin-resident T cell memory has been observed in response to Candida albicans, Leishmania major, Herpes simplex virus as well as commensal bacteria. Most importantly, Tsrm cells contribute to localized protection against re-infection with cutaneous pathogens (4–9). In addition, Trm cell development has been tracked in mice following vaccination and was positively correlated with vaccination efficacy (10–13), making Trm cells a promising target for vaccination (14–19).
The Gram-positive bacterium Staphylococcus aureus is the leading cause of skin and soft tissue infections globally (20). In addition, the rapid emergence of antibiotic resistance has highlighted the need for alternative treatments such as vaccination to combat S. aureus infections. However, to design an efficacious vaccine, it is important to have a complete understanding of the correlates of protection against this pathogen, which is currently lacking (21, 22).
Based on data from mouse and human studies, there is a general consensus that CD4+ T cells, and in particular Th17 and Th1 subsets, contribute to protective immunity against S. aureus infection (23–26). Furthermore, healthy individuals have a considerable number of circulating memory CD4+ T cells specific for S. aureus, likely due to repeated encounters over time with this skin pathobiont (27, 28). However, to our knowledge, the existence of S. aureus-specific tissue resident memory CD4+ T cells in healthy human skin has not yet been addressed.
Using human skin explants, which represent a valuable model to study skin-resident immune responses of human skin to microbes (29), we here show that S. aureus-specific CD4+ Tsrm cells are commonly found in the skin of healthy individuals. This finding uncovers CD4+ Tsrm cells as previously neglected cellular players in the cutaneous human immune response to S. aureus and thus may aid in the development of novel vaccine strategies against S. aureus SSTIs.
Materials and Methods
Preparation of Single Cell Suspensions From Human Skin Explants
Fresh human skin explants (16 cm2) derived from abdominoplasty surgical waste of healthy women (age 40 ± 11, body mass index 25 ± 3) were purchased from Biopredic (France). Explants were shipped at 4°C and received within 48 h following the surgery. Upon arrival, samples were immediately processed as shown in Figure 1A. In short, adipose tissue was removed with dissection scissors followed by additional scraping with a disposable scalpel (Swann-Morton, Sheffield). Skin was cut in 1 cm2 pieces, washed repeatedly with PBS and incubated for 1 h at RT in RPMI 1640 (Invitrogen) containing 1 mg/ml collagenase type 1 (Life technologies). Next, skin pieces were extensively minced with disposable scalpels and incubated overnight at 37°C at 5% CO2 in a 6-well plate with 1 mg/ml collagenase type 1 (Life technologies) and 20 μg/ml DNAse (Sigma) in 5 ml c-RPMI [RPMI 1640 containing penicillin-streptomycin-glutamine, sodium pyruvate, minimum essential medium non-essential amino acids (all from Gibco), and 10% heat-inactivated FBS (Hyclone)]. The next day, the skin cell suspension was pipetted vigorously, pooled and filtered sequentially through a 100 μm and a 40 μm cell strainers (Corning). Skin debris was further removed by Ficoll-Paque Premium (GE Healthcare) gradient separation. The viability (83% ± 6.5) and the cell yield (0.63 ± 0.33 × 106 cells/cm2 skin) of the obtained single cell suspensions were assessed with a Vi-CELL XR cell counter (Beckman Coulter).
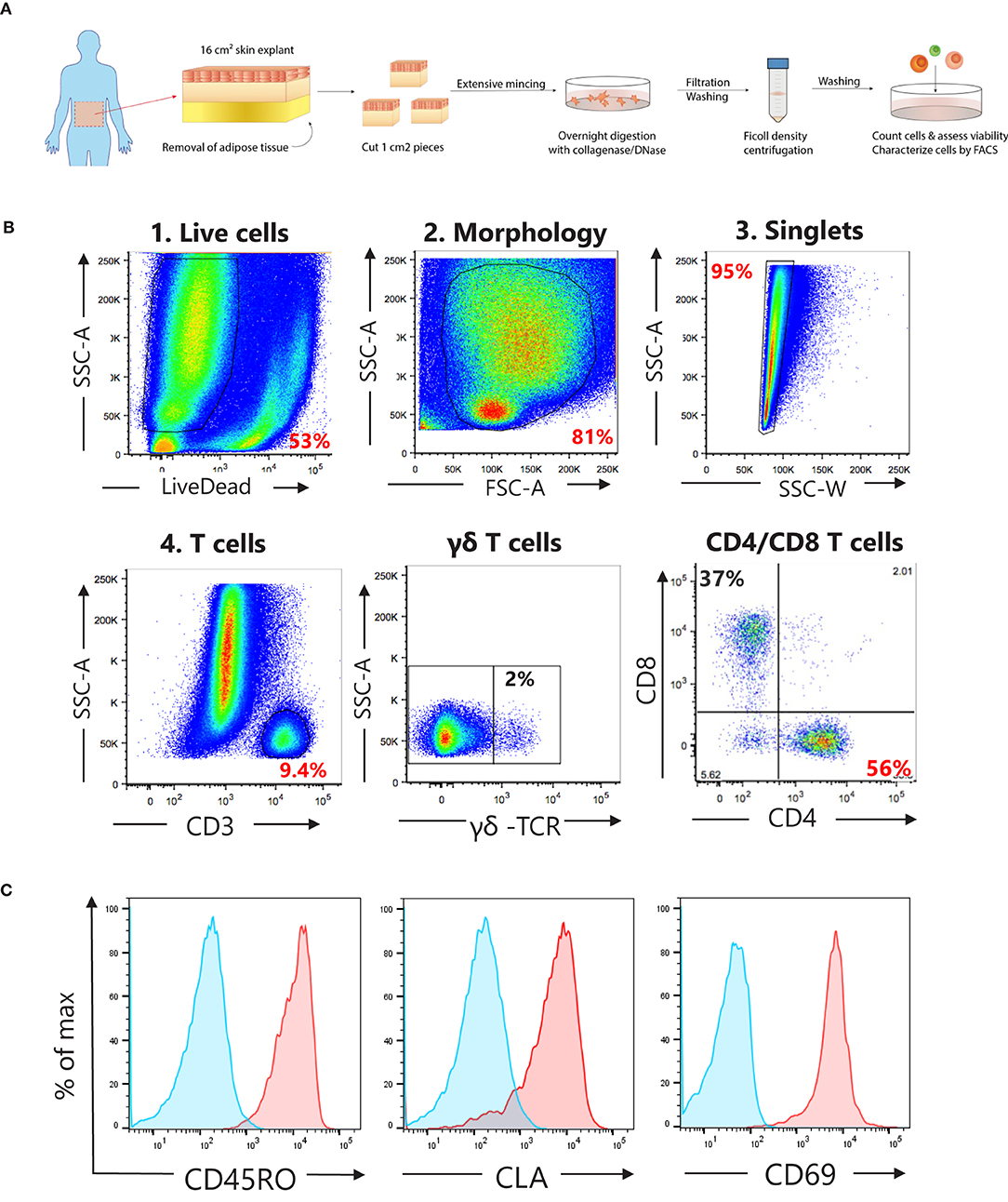
Figure 1. Characterization of T cell subsets in cell suspensions obtained from human skin explants. (A) Schematic overview of the protocol used to obtain single cell suspensions from human skin explants. (B) Gating strategy to analyze T cell subsets, γδ-, CD4+, and CD8+ T cells, in single cell suspensions. (C) Representative histograms (in red) showing the surface expression for skin resident memory T cells markers, CD45RO (memory), CLA (skin homing), and CD69 (tissue retention) on live CD4+ cells. Blue histograms represent unstained cells.
Heat-Killed (HK) Microbes
Methicillin resistant S. aureus USA300 LAC strain and the coagulase negative staphylococci S. epidermidis 1457 strain and S. lugdunensis SL13 strain were grown to mid-exponential phase (OD600 0.6) (30, 31). Next, bacteria were washed with PBS to remove secreted proteins, resuspended in sterile PBS, plated on Tryptic Soy Agar (TSA) for CFU counts and inactivated in a dry block heater at 90°C for 45 min. After inactivation bacteria were washed three times with PBS and protein content was measured using the Pierce™ BCA Protein Assay kit (Thermo Scientific). Samples concentrations were adjusted to 25 μg/ml, which corresponds to ~1 × 108 CFU/ml (25). Bacterial killing was verified by plating the HK bacteria for 5 days on TSA. Heat-killed (HK) bacteria were aliquoted and stored at −20°C. HK S. epidermidis (FDA strain PCI 1200), HK C. albicans (ATCC 10231), and HK E. coli (O111:B4) were purchased from Invivogen.
CD4+ T Cell Proliferation and Cytokine Production in Response to HK Microbes by Click-iT EdU/V-PLEX Assay
Single cell suspensions obtained from the skin explants were seeded at 500,000 live cells/well for all conditions but CD3/CD28 (for which half the number of cells were plated) in a final volume of 200 μl c-RPMI in round-bottom 96 wells plates (Corning). Cells were rested for at least 24 h at 37°C with 5% CO2 to restore surface marker expression and reduce cellular stress due to the isolation procedure. Cell culture medium was replaced with: (1) fresh medium alone (no stimulation, negative control) or containing: (2) 106 CFU HK microbes corresponding to a multiplicity of infection of 2; (3) Tetanus toxoid (5 μg/ml, Novartis); (4) anti-CD28 (2 μg/ml, clone CD28.2, BD Bioscience, cat # 555725) added to anti-CD3 (1 μg/ml, clone OKT3, BD Bioscience, cat # 566685) coated wells (CD3/CD28, polyclonal stimulation, positive control). After 3 days of culture the thymidine analog EdU (10 μM) was added to the cultures for the last 16 h. At day four, cell culture supernatants were collected and stored at −20°C for cytokine analysis while CD4+ T cell proliferation was assessed by Click-iT EdU assay (Click-iT Plus EdU Alexa Fluor 488 Flow cytometry assay kit, Invitrogen), as recently described (Clemente et al., manuscript in preparation). Cytokines were measured using the 27-V-PLEX human kit (MesoScale Discovery) following manufacturer's instructions. Plates were analyzed by a MESO Quickplex SQ 120 reader and cytokine concentrations were determined using MSD discovery workbench 4.0. Values below or above the detection limits were given the value of ½ LLOD (Lower Limit Of Detection) or 2x ULOD (Upper Limit Of Detection), respectively. Cytokines that were consistently above or below the detection limits, or showed no differences across all stimuli were excluded from further analysis.
Flow Cytometry
For the phenotypic characterization of T cell subsets in the single skin cell suspensions, cells were stained with Live/Dead Near-IR Dead cell stain kit (Invitrogen) for 20 min at room temperature (RT), washed and blocked with 2% rabbit serum in PBS on ice for 20 min. Next, cells were stained for CD4, CD8, γδ-TCR, CD45RO, CLA, and CD69 for 20 min at 4°C, washed with PBS, and fixed with Cytofix (BD Bioscience). Gating strategy is shown in Figure 1B.
For T cell proliferation experiments, after surface staining with CD4, CD8, CD45RO and CLA and fixation, cells were permeabilized with PBS 1% BSA, 0.5% saponin for 30 min at 4°C, washed with PBS 1% BSA, 0.5% saponin followed by the Click-iT reaction (Click-iT Plus EdU Alexa Fluor 488 Flow cytometry assay kit, Invitrogen). After 30 min at RT, cells were washed with PBS 1% BSA, 0.5% saponin and stained for CD3 for 15 min at RT. After two washes, the cells were analyzed on a BD LSR II flow cytometer, and data was analyzed using FlowJo 10 (TreeStars). All antibodies used in this study are shown in Supplementary Table 1.
Cytokine Production in Response to ex vivo Epicutaneous S. aureus Infection of Human Skin Explants
Infection of human skin explants was performed according to a previously described protocol (32). In short, after removal of adipose tissue, the skin sheet was pinned in a dissection board and stripped 30 times with a hypoallergenic tape (Transpore, 3M). Eight mm punch biopsies were collected using disposable biopsy punches (Kai Medical). The punches were washed with culture medium (Advanced DMEM; Gibco) once, followed by two washes with PBS to remove antibiotics. Next, the punches were placed in 12-well transwell plates with 0.4 μm pore size (Corning), containing 1 ml of culture medium. Finally, the punches were infected in duplicate with USA300 LAC strain (5 × 106 CFU in 1 μl PBS) and cultured at air-liquid interface for 2, 24, or 72 h at 37°C, 5% CO2. At each indicated time point, culture supernatants were collected, filtered and stored at −20°C for cytokine analysis that was performed using the 27-V-PLEX human kit (MesoScale Discovery).
Statistical Analysis
GraphPad Prism 8.0.1 was used to perform statistical analysis. Data were analyzed using one-way ANOVA with Dunnett's test, paired Wilcoxon test or paired t-test, as indicated. Significant differences (p < 0.05) are shown.
Results
Tissue-Resident Memory CD4+ T Cells Present in the Skin of Healthy Subjects Proliferate in Response to S. aureus
To investigate if healthy human skin contains CD4+ Tsrm cells specific for S. aureus, we stimulated single cell suspensions prepared from skin explants from eight healthy donors with heat-killed (HK) S. aureus. The vast majority (>90%) of the isolated CD4+ T cells had a Tsrm phenotype based on the surface expression of the memory marker CD45RO, the skin-tropic marker CLA and the tissue-retention marker CD69 (Figure 1C). After 4 day stimulation with HK microbes, we identified CD4+ T cells that have neo-synthesized DNA by the flow cytometry-based Click-iT EdU proliferation assay (CD4+EdU+ cells, Figures 2A,B). These cells were CD4+ Tsrm based on their expression of CD45RO and CLA (Supplementary Figure 1). As shown in Figure 2C, the analysis of 8 healthy subjects showed a statistically significant CD4+ Tsrm cell proliferation in response to HK S. aureus but not to HK S. epidermidis, which is a major component of the human skin microbiome (33). In agreement with previous studies, we observed specific CD4+ Tsrm cell proliferation in response to C. albicans (Figure 2C) (6), while no proliferation was observed in response to E. coli, which is not part of the skin microbiome (Figure 2C). Interestingly, a strong proliferation of CD4+ Tsrm cells was also induced by the recall antigen Tetanus toxoid (Figure 2C) that commonly induces a strong T cell response in human blood (27, 34). Polyclonal T cell stimulation with anti-CD3/CD28 antibodies, which was used as positive control, induced the strongest CD4+ Tsrm cell proliferation in all donors as expected (Figure 2C).
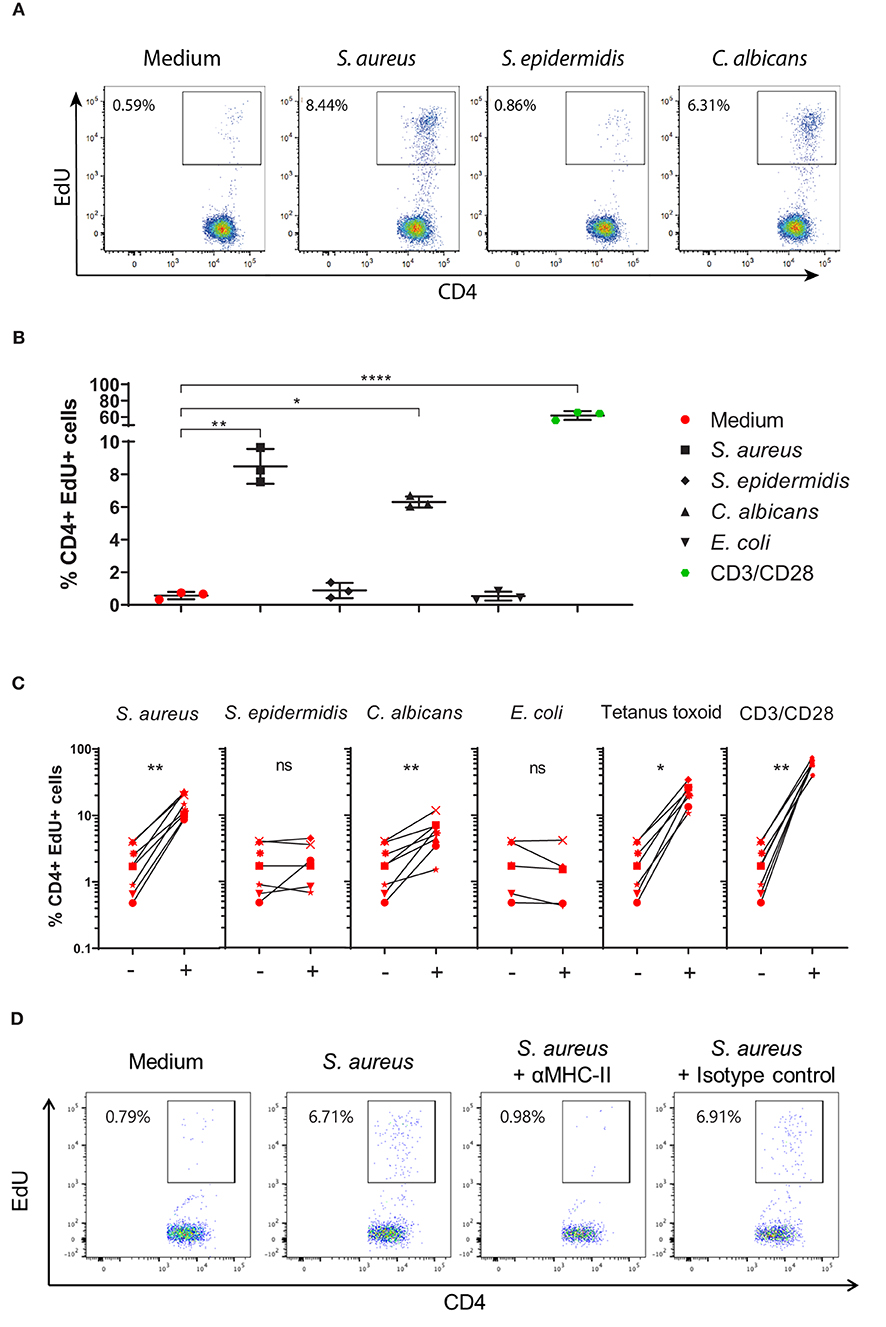
Figure 2. Tissue-resident memory CD4+ T cells present in the skin of healthy subjects proliferate in response to S. aureus. (A) Representative dot-plots showing CD4+EdU+ cells in cell cultures, obtained from a skin explant of an healthy subject, stimulated for 4 days with heat-killed (HK): S. aureus USA300 LAC strain, S. epidermidis PCI 1200 strain, C. albicans, or left unstimulated (medium). (B) Reproducibility of the Click-iT EdU assay. Representative results showing the percentages of CD4+EdU+ T cells obtained from triplicate skin cell cultures from the healthy subject shown in (A) in response to different stimuli. *p < 0.05, **p < 0.01, ****p < 0.0001 as assessed by one-way ANOVA. (C) Proliferation of CD4+ Tsrm cells from skin explants of 8 healthy subjects in response to different HK microbes, Tetanus toxoid, anti-CD3/anti-CD28 antibodies or medium alone. Average percentages of CD4+EdU+ cells of each of the 8 subjects analyzed, in triplicate, are shown by an identifying symbol. Per donor, each stimulated group, indicated by a +, was compared to the non-stimulated group (medium), indicated by a –, by paired Wilcoxon test, *p < 0.05, **p < 0.01. (D) Representative dot plots showing proliferating CD4+ Tsrm cells (CD4+EdU+) after 4-day culture in medium alone (negative control) or with HK S. aureus alone or in combination with MHC class-II blocking antibodies or isotype control antibodies.
Heat-inactivated intact bacteria have been described to be devoid of superantigens, which stimulate T cells in a non-specific manner (25, 35). To further prove that the observed CD4+ Tsrm cell proliferation was antigen-specific, we added MHC class-II blocking antibodies or the isotype control to the skin cell cultures. Indeed, in the presence of MHC-II blocking antibodies, CD4+ T cell proliferation in response to HK S. aureus was abolished while the isotype control had no effect (Figure 2D). In addition, no CD4+ T cell proliferation was detected by Click-iT EdU assay upon stimulation of peripheral blood mononuclear cells (PBMCs) of some healthy subjects with HK S. aureus, while a strong proliferation was observed in response to the staphylococcal T cell superantigen SEB, as expected (Supplementary Figure 2A).
To further assess the staphylococcal species-specificity on CD4+ Tsrm cell proliferation, we analyzed the proliferative response to the coagulase-negative S. lugdunensis, which is also a skin commensal (33). Analysis of cells obtained from explants from five healthy subjects showed no proliferation in response to either S. lugdunensis SL13 strain or S. epidermidis 1457 strain while proliferation to S. aureus USA300 LAC strain was confirmed (Supplementary Figure 2B). Taken together, these findings support the presence of S. aureus-specific CD4+ tissue-resident memory T cells in healthy human skin.
Cells Isolated From the Skin of Healthy Subjects Produce Pro-inflammatory Cytokines in Response to S. aureus
To further investigate the response of healthy human skin to S. aureus, we analyzed the cytokine profile in the supernatants of S. aureus-specific CD4+ Tsrm cells analyzed by Click-iT EdU assay (Figure 2C), collected after 4 days of stimulation, by 27-V-PLEX. As shown in Figure 3, significant increases in production of IL-17A (141.20 vs. 12.34 pg/ml), IL-22 (131.67 vs. 4.22 pg/ml), IFN-γ (176.61 vs. 78.82 pg/ml), GM-CSF (138.73 vs. 13.34 pg/ml), and TNF-β (18.48 vs. 1.84 pg/ml) were observed in response to HK S. aureus stimulation while HK S. epidermidis induced a significant increase in IL-17A production only (48.29 vs. 12.34 pg/ml). Interestingly, C. albicans, as well as Tetanus toxoid and the polyclonal stimulation with anti-CD3/CD28 antibodies induced the same pattern of cytokines as S. aureus while in response to E. coli none of the analyzed cytokines was induced. Remarkably, the production of IL-17A, IL-22, IFN-γ, GM-CSF, and TNF-β in response to HK S. aureus stimulation were substantially decreased by MHC class II blocking antibodies, indicating that CD4+ Tsrm cells were a major source of these cytokines (data not shown). In addition we obtained direct evidence of IL-17A and IL-22 production by CD4+EdU+ Tsrm cells by intracellular cytokine staining (data not shown).
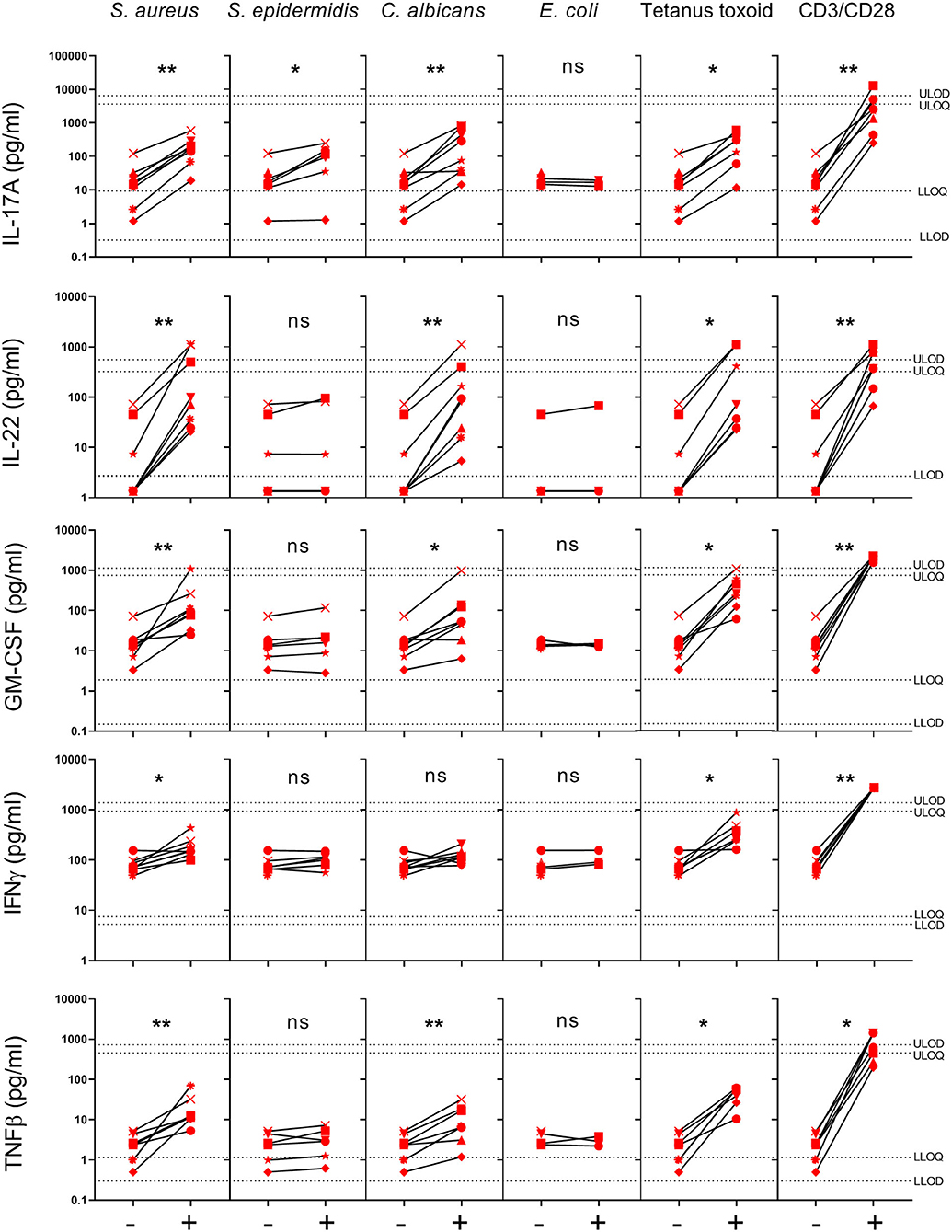
Figure 3. Cytokines secreted in the supernatants of skin cell cultures stimulated with different HK microbes. Supernatants were collected from samples used to determine CD4+ Tsrm cell proliferation by the Click-iT EdU assay. Cytokines were quantified by V-PLEX assays. Cytokine production in response to the different stimuli is shown for 8 donors (indicated by individual symbols), except for Tetanus toxoid (7), S. epidermidis (6) and E. coli (3). Per donor, each stimulated group, indicated by a +, was compared to the non-stimulated group (medium), indicated by a –, by paired Wilcoxon test, *p < 0.05, **p < 0.01. LLOD, Lower limit of detection; ULOD, upper limit of detection; LLOQ, lower limit of quantification; ULOQ, upper limit of quantification.
Ex vivo Epicutaneous S. aureus Infection of Healthy Human Skin Induces Pro-inflammatory Cytokines
To understand the local immune response to S. aureus within healthy human skin, we used an ex vivo epicutaneous infection model (32). Skin explants were tape-stripped to remove the stratum corneum, followed by topical application of 5 × 106 CFU S. aureus USA300 LAC strain. Cytokines were quantified in the skin explants culture media by 27-V-PLEX at different time-points post infection (p.i.). At 24 h p.i. only IL-10, IL-1α, and IL-2 were produced at significantly higher levels compared to the non-infected control while at 72 h p.i. also IL-17A, IL-22, IFN-γ, IL-1α, IL-1β, GM-CSF, IL-12p40, TNF-α, and TNF-β levels were increased (Figure 4). These data show that, while at an initial stage of S. aureus infection the cytokine response of the skin is limited, it becomes strongly proinflammatory at later stages of infection. Furthermore, the cytokines induced by stimulation of cells extracted from the skin with HK S. aureus, namely IL-17A, IL-22, GM-CSF, IFN-γ, and TNF-β, were induced also by epicutaneous infection of human skin with live S. aureus, strengthening the value of this in vitro model.
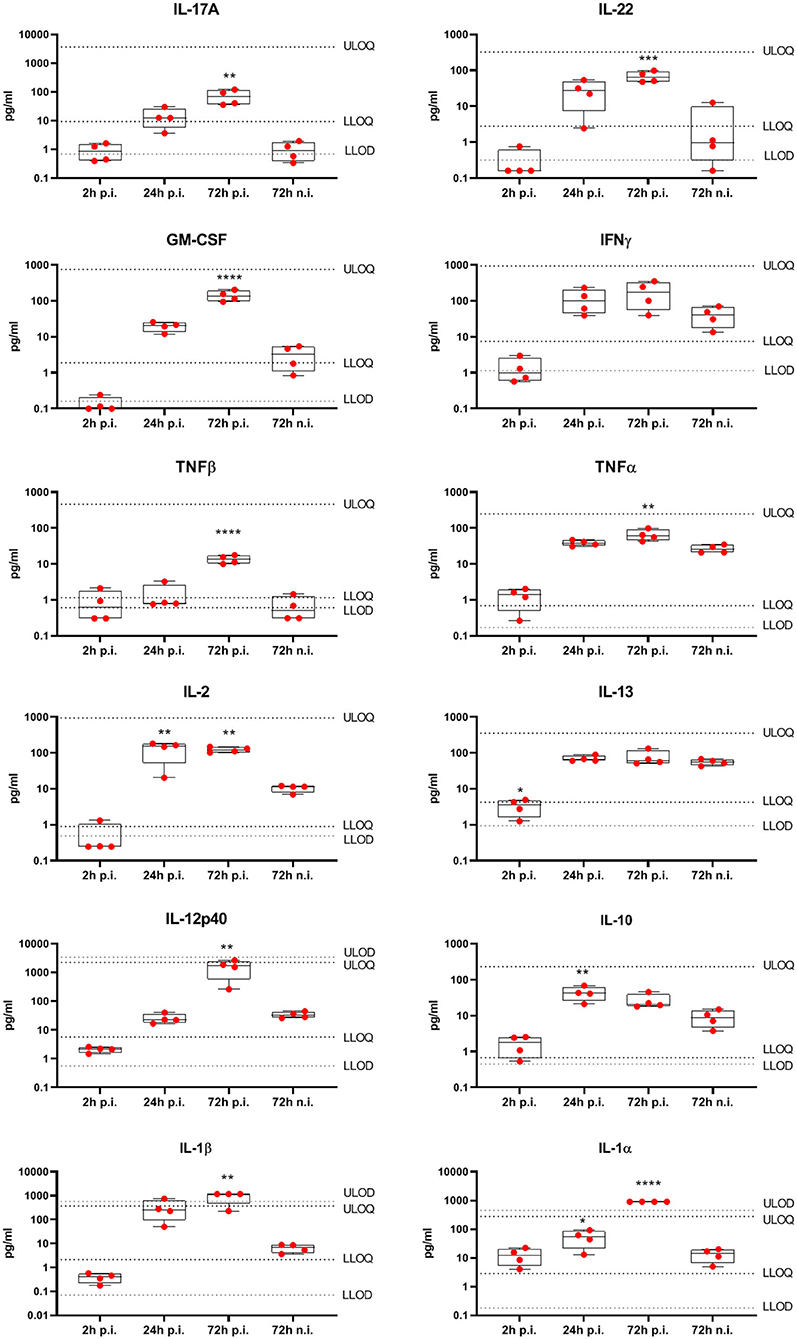
Figure 4. Cytokines produced by human skin from healthy subjects in response to ex vivo epicutaneous S. aureus USA300 LAC infection. Eight mm biopsy punches, prepared from tape stripped human skin explants from four donors, were put in transwells at air-liquid interface and infected epicutaneously with 5 × 106 CFU S. aureus USA300 LAC strain in duplicate, or were left non-infected (n.i.) for 2, 24, or 72 h when culture medium was collected. Cytokine concentrations were assessed by V-PLEX assay. Box-and-whiskers extend from the 25th to 75th percentiles and the line inside the box represents the median. LLOD, Lower limit of detection; ULOD, upper limit of detection; LLOQ, lower limit of quantification; ULOQ, upper limit of quantification. Differences between cytokine concentrations measured at 2, 24, 72 hpi were compared with non-infected control at 72 h using an one-way ANOVA, *p < 0.05, **p < 0.01, ***p < 0.001, ****p < 0.0001.
Discussion
Here, we show that S. aureus-specific CD4+ tissue-resident memory T cells are abundant in the skin of healthy subjects. In particular, by the use of a novel assay, Click-iT EdU/V-PLEX, which allows simultaneous detection of CD4+ T cell proliferation and cytokine quantification in cell culture supernatants, we revealed that stimulation of cells isolated from abdominal skin explants with HK S. aureus USA300 LAC strain induced: (1) The proliferation of CD4+ T cells that were identified as skin-resident memory T (Tsrm) cells based on the expression of the CLA skin-homing, CD45RO memory, and CD69 tissue-retention markers. (2) The secretion of proinflammatory cytokines, namely IL-17A, IL-22, GM-CSF, IFN-γ, and TNF-β. Remarkably, neither proliferation of CD4+ Tsrm cells nor secretion of proinflammatory cytokines except for IL-17A was observed in response to the common skin commensal S. epidermidis.
Mechanisms enabling the host to mount protective immune responses against pathogens while establishing a privileged relationship with commensal bacteria have been intensively studied but still remain largely unknown. One important feature of commensal-specific immunity is its uncoupling from inflammation and the maintenance of tissue homeostasis (8). Cytokines play a key role not only in the promotion of skin inflammation but also in skin homeostasis. Cytokine analysis of culture medium of both isolated skin cells stimulated with HK S. aureus and human skin explants infected ex vivo with S. aureus showed the production of cytokines involved in skin inflammation and tissue repair. Genetic evidence has highlighted a key role for IL-17-mediated immunity in protection against S. aureus skin infection, but not invasive staphylococcal disease, similarly to what has been observed for C. albicans infections (36, 37). IL-17 enhances the recruitment of neutrophils, which can kill S. aureus, to the site of infection, and stimulates the production of antimicrobial peptides (AMPs) that can be directly bactericidal (38–40). IL-22 promotion of skin inflammation is well-established (41), however a role of IL-22 in skin homeostasis has also emerged. In particular, IL-22 has been shown to induce the proliferation of keratinocytes and AMPs production (42, 43). In addition, IL-22 induces MHC class II expression on keratinocytes thereby promoting the selective accumulation of commensal-induced IFN-γ producing CD4+ T cells within murine skin (44). TNF-β induces angiogenesis, thereby contributing to wound repair (45, 46). Interestingly, cytokine analysis of human skin explants infected epicutaneously with S. aureus revealed also a significant production of IL-1α and IL-1β (Figure 4). These results are in agreement with previous studies showing that these cytokines were produced by murine keratinocytes after an epicutaneous S. aureus challenge (47, 48). The lack of IL-1 production by isolated human skin cells stimulated with HK S. aureus could be due to the lack of secreted bacterial proteins, including alpha-toxin that has a prominent role in the induction of IL-1 production by keratinocytes (49).
The Click-iT EdU/V-PLEX assay does not allow the identification of the cellular source of the detected cytokines. However, since we observed that the polyclonal T cell stimulation with anti-CD3/CD28 antibodies induced the same cytokine profile as HK S. aureus stimulation, and MHC class II blocking antibodies substantially decreased the production of these cytokines in response to HK S. aureus stimulation (data not shown) it seems likely that CD4+ Tsrm cells are a major source of the observed cytokines (50), although cytokine production by non-classical T cells cannot be ruled out (51–53). In addition we obtained direct evidence of IL-17A and IL-22 production by CD4+EdU+ Tsrm cells by intracellular cytokine staining (data not shown). It should be noted that studies, performed both in human and murine skin, suggest that Trm cells accumulate in the skin as a function of the number of infectious and inflammatory events over time. Indeed, laboratory mice, like newborn, but not adult, humans lack effector-differentiated and peripherally distributed memory T cells, including Tsrm cells (54, 55). In mice, γδ T cells have been identified as key IL-17 producers upon S. aureus infection (52). However, it has been shown that following infection of laboratory mice with C. albicans, while the initial IL-17-producing cells were γδ T cells, at later times the majority of C. albicans-reactive IL-17-producing T cells were CD4+ Tsrm cells. Importantly, IL-17-producing CD4+ Trm cells that responded to C. albicans were identified in normal human skin (4). Similarly, since humans, unlike laboratory mice, naturally encounter S. aureus repeatedly over time, we hypothesize that CD4+ Tsrm cells are the primary source of IL-17 produced in response to this bacterium in human skin. Indeed, comparable levels of IL-17A were produced in response to HK S. aureus or C. albicans in our experiments. On the other hand, S. epidermidis colonization of mouse skin has been shown to induce IL-17A-producing CD8+ T cells restricted to non-classical MHC class I molecules and characterized by immunoregulatory and tissue-repair signatures, which home to the epidermis (9). These cells could be the source of IL-17A produced in response to HK S. epidermidis in our experiments, although further research is needed to address this point.
A major difference between S. aureus and S. epidermidis is the secretion of numerous virulence factors including proteases and toxins such as alpha-toxin, that can damage the skin epithelial integrity (32, 56). Our results suggest that in order to halt S. aureus invasion, the cutaneous immunity deploys CD4+ Tsrm cells that secrete several cytokines with proven anti-S. aureus and tissue-repair activities. Induction of such a mild anti-bacterial immune response might be a strategy to limit local infection and prevent systemic spread, promoting a long-lasting equilibrium between this pathobiont and the host. Interestingly, this could be achieved through alpha-toxin that has been shown to modulate mouse CD4+ T cell differentiation limiting Th1 while promoting Th17 responses (57). However, once the skin is breached, the local immunity is dampened or the bacterial load is exceedingly high, this local response is no longer sufficient to control S. aureus (37). Indeed, the importance of antibodies and Th1 cells in controlling systemic S. aureus infections has been highlighted (25, 58).
Interestingly, a recent paper showed that neonatal mouse skin colonization with S. epidermidis facilitated immune tolerance to this bacterium via the induction of regulatory T (Treg) cells (49). This was not the case for S. aureus that, through alpha-toxin mediated IL-1β production by myeloid cells, limited the development of S. aureus-specific Treg cells thus enhancing skin inflammation upon later-life exposure to S. aureus (49). Similarly, we cannot rule out the presence of S. epidermidis-specific Treg cells, which are known to proliferate under homeostatic conditions, in our experiments (6).
The immune response of the skin to S. aureus has been intensively investigated in a number of elegant mouse studies (19). However, the anatomical and immunological differences between murine and human skin together with the different composition and exposure to skin microbiome limit the translational value of the results obtained in mice (29, 59). As a more biologically relevant model, we used human skin explants generated as surgical waste from cosmetic surgery performed on the abdomen. Of note, although Staphylococcus aureus colonization is most consistently identified in humans in the anterior nares, colonization has also been reported at other body sites including axilla, inguinal and rectal areas (60). In addition, S. aureus was cultured from 30% of abdominal skin swabs from healthy subjects (61). Since variability was reported among different skin sites, as a consequence differences in the relative abundance in CD4+ Trm cells specific for S. aureus at different locations can be envisaged. Nevertheless, studies have shown that following skin infection, Tsrm cells can migrate out of the skin and populate distant skin sites thus forming global skin immunity (16, 62). Interestingly, S. aureus-specific CD4+ Trm cells have been identified in gut tissue of healthy individuals. These cells showed increased IL-17A and reduced IFN-γ production as compared to cells with similar reactivity present in the circulation (63), similarly to what we report here for S. aureus-specific CD4+ Tsrm cells. Indeed, this seems to be a common characteristic of barrier-protective Trm cells. In addition, since some inborn errors of IL-17 immunity predispose not only to skin but also to lung S. aureus infection (37), the presence of S. aureus-specific CD4+ Tsrm cells in the lungs and their phenotype should be assessed.
While numerous efforts have been made toward designing a vaccine against S. aureus, unfortunately to this date none have been successful (64). Perhaps the most fundamental reason explaining the past failures of S. aureus vaccines is the lack of a complete understanding of protective immunity. Our results enforce the conclusion that since the contribution of local immune memory within tissues is becoming evident, it should be evaluated in vaccination efficacy studies (50). Since a sizable percentage of people experiencing S. aureus SSTI has recurrent infections (22, 65), it will be very informative to analyze the CD4+ Tsrm response to S. aureus in this population. In addition, it would be interesting to investigate the S. aureus-specific CD4+ Tsrm response in patients with atopic dermatitis, a chronic and relapsing inflammatory skin disorder associated with skin barrier impairment and the predominant S. aureus colonization (66).
In summary, we describe a skin-resident memory CD4+ T cell population within healthy human skin that is specific for the human skin pathogen S. aureus. While further research is needed to better characterize the phenotype, the antigen-specificity and the protective potential of these cells, this finding highlights that skin-resident memory CD4+ T cells could be a powerful and exploitable arm of adaptive immunity against this elusive pathobiont.
Data Availability Statement
The raw data supporting the conclusions of this article will be made available by the authors, without undue reservation.
Ethics Statement
The studies involving human participants were reviewed and approved by French Ministry of Higher Education, Research and Innovation. The patients/participants provided their written informed consent to participate in this study.
Author Contributions
AH, MEM, and ES were involved in designing the study and wrote the paper. AH, MEM, BC, and ARC performed experiments. ST and BC set up the Click-iT EdU assay. ST provided valuable technical support with flow cytometry. AH, MEM, BC, and ES analyzed the data. FB and ES supervised the project. All authors critically revised the manuscript and approved it before submission.
Funding
This work was supported by the European Union's Horizon 2020 research and innovation program under the Marie Skłodowska-Curie grant agreement no. 675106 coordinated by FB (GSK, Siena, Italy).
Conflict of Interest
AH, MEM, and ARC are Ph.D. fellows and are enrolled in the Infection and Immunity Ph.D. program, part of the graduate school of Life Sciences at Utrecht University and participated in a post graduate studentship program at GSK. BC was a PhD student from the University of Siena funded by GSK. ST, FB, and ES are employees of the GSK group of companies. FB hold shares in the GSK group of companies and holds pending and issued patents (WO/2019/158537, WO/2015/144691, WO/2015/144653, WO/2015/144655, WO/2014/033190, WO/2014/033191, WO/2014/033192, WO/2014/033193, WO/2013/030378, and WO/2010/119343) on S. aureus vaccine formulations.
Acknowledgments
We would like to thank Rachael Clark and Thomas Kupper for sharing their expertise and technical details on the skin digestion protocol, Nina van Sorge and Jonah Clegg for providing critical feedback on the manuscript, Federica Baffetta for statistical support, Michela Brazzoli and Oretta Finco for support and scientific advice.
Supplementary Material
The Supplementary Material for this article can be found online at: https://www.frontiersin.org/articles/10.3389/fimmu.2021.642711/full#supplementary-material
References
1. Clark RA, Chong B, Mirchandani N, Brinster NK, Yamanaka K, Dowgiert RK, et al. The vast majority of CLA+ T cells are resident in normal skin. J Immunol. (2006) 176:4431–9. doi: 10.4049/jimmunol.176.7.4431
2. Watanabe R, Gehad A, Yang C, Scott LL, Teague JE, Schlapbach C, et al. Human skin is protected by four functionally and phenotypically discrete populations of resident and recirculating memory T cells. Sci Transl Med. (2015) 7:279RA39. doi: 10.1126/scitranslmed.3010302
3. Kumar BV, Ma W, Miron M, Granot T, Guyer RS, Carpenter DJ, et al. Human tissue-resident memory T cells are defined by core transcriptional and functional signatures in lymphoid and mucosal sites. Cell Rep. (2017) 20:2921–34. doi: 10.1016/j.celrep.2017.08.078
4. Park CO, Fu X, Jiang X, Pan Y, Teague JE, Collins N, et al. Staged development of long-lived T-cell receptor αβ TH17 resident memory T-cell population to Candida albicans after skin infection. J Allergy Clin Immunol. (2018) 142:647–62. doi: 10.1016/j.jaci.2017.09.042
5. Glennie ND, Yeramilli VA, Beiting DP, Volk SW, Weaver CT, Scott P. Skin-resident memory CD4+ T cells enhance protection against Leishmania major infection. J Exp Med. (2015) 212:1405–14. doi: 10.1084/jem.20142101
6. Seneschal J, Clark RA, Gehad A, Baecher-Allan CM, Kupper TS. Human epidermal langerhans cells maintain immune homeostasis in skin by activating skin resident regulatory T cells. Immunity. (2012) 36:873–84. doi: 10.1016/j.immuni.2012.03.018
7. Sparber F, De Gregorio C, Steckholzer S, Ferreira FM, Dolowschiak T, Ruchti F, et al. The skin commensal yeast malassezia triggers a type 17 response that coordinates anti-fungal immunity and exacerbates skin inflammation. Cell Host Microbe. (2019) 25:389–403.e6. doi: 10.1016/j.chom.2019.02.002
8. Naik S, Bouladoux N, Linehan JL, Han SJ, Harrison OJ, Wilhelm C, et al. Commensal-dendritic-cell interaction specifies a unique protective skin immune signature. Nature. (2015) 520:104–8. doi: 10.1038/nature14052
9. Linehan JL, Harrison OJ, Han SJ, Byrd AL, Vujkovic-Cvijin I, Villarino AV, et al. Non-classical immunity controls microbiota impact on skin immunity and tissue repair. Cell. (2018) 172:784–96. doi: 10.1016/j.cell.2017.12.033
10. Wakim LM, Smith J, Caminschi I, Lahoud MH, Villadangos JA. Antibody-targeted vaccination to lung dendritic cells generates tissue-resident memory CD8 T cells that are highly protective against influenza virus infection. Mucosal Immunol. (2015) 8:1060–71. doi: 10.1038/mi.2014.133
11. Wilk MM, Mills KHG. CD4 TRM cells following infection and immunization: Implications for more effective vaccine design. Front Immunol. (2018) 9:1860. doi: 10.3389/fimmu.2018.01860
12. Kadoki M, Patil A, Thaiss CC, Brooks DJ, Pandey S, Deep D, et al. Organism-level analysis of vaccination reveals networks of protection across tissues. Cell. (2017) 171:398–413. doi: 10.1016/j.cell.2017.08.024
13. Louis L, Clark M, Wise MC, Glennie N, Wong A, Broderick K, et al. Intradermal synthetic DNA vaccination generates leishmania-specific T cells in the skin and protection against leishmania major. Infect Immun. (2019) 87:e00227–19. doi: 10.1128/IAI.00227-19
14. Seidel JA, Vukmanovic-Stejic M, Muller-Durovic B, Patel N, Fuentes-Duculan J, Henson SM, et al. Skin resident memory CD8+ T cells are phenotypically and functionally distinct from circulating populations and lack immediate cytotoxic function. Clin Exp Immunol. (2018) 194:79–92. doi: 10.1111/cei.13189
15. Pan Y, Tian T, Park CO, Lofftus SY, Mei S, Liu X, et al. Survival of tissue-resident memory T cells requires exogenous lipid uptake and metabolism. Nature. (2017) 543:252–6. doi: 10.1038/nature21379
16. Jiang X, Clark RA, Liu L, Wagers AJ, Fuhlbrigge RC, Kupper TS. Skin infection generates non-migratory memory CD8+ TRM cells providing global skin immunity. Nature. (2012) 483:227–31. doi: 10.1038/nature10851
17. Beura LK, Mitchell JS, Thompson EA, Schenkel JM, Mohammed J, Wijeyesinghe S, et al. Intravital mucosal imaging of CD8+ resident memory T cells shows tissue-autonomous recall responses that amplify secondary memory article. Nat Immunol. (2018) 19:173–82. doi: 10.1038/s41590-017-0029-3
18. Ariotti S, Hogenbirk MA, Dijkgraaf FE, Visser LL, Hoekstra ME, Song JY, et al. Skin-resident memory CD8+ T cells trigger a state of tissue-wide pathogen alert. Science. (2014) 346:101–5. doi: 10.1126/science.1254803
19. Clegg J, Soldaini E, Bagnoli F, McLoughlin RM. Targeting skin-resident memory T cells via vaccination to combat Staphylococcus aureus infections. Trends Immunol. (2020) 42:6–17. doi: 10.1016/j.it.2020.11.005
20. Ray GT, Suaya JA, Baxter R. Microbiology of skin and soft tissue infections in the age of community-acquired methicillin-resistant Staphylococcus aureus. Diagn Microbiol Infect Dis. (2013) 76:24–30. doi: 10.1016/j.diagmicrobio.2013.02.020
21. Montgomery CP, Daniels M, Zhao F, Alegre ML, Chong AS, Daum RS. Protective immunity against recurrent Staphylococcus aureus skin infection requires antibody and interleukin-17A. Infect Immun. (2014) 82:2125–34. doi: 10.1128/IAI.01491-14
22. Montgomery CP, David MZ, Daum RS. Host factors that contribute to recurrent staphylococcal skin infection. Curr Opin Infect Dis. (2015) 28:253–8. doi: 10.1097/QCO.0000000000000156
23. Minegishi Y, Saito M, Nagasawa M, Takada H, Hara T, Tsuchiya S, et al. Molecular explanation for the contradiction between systemic Th17 defect and localized bacterial infection in hyper-IgE syndrome. J Exp Med. (2009) 206:1291–301. doi: 10.1084/jem.20082767
24. Utay NS, Roque A, Timmer JK, Morcock DR, DeLeage C, Somasunderam A, et al. MRSA infections in HIV-infected people are associated with decreased MRSA-specific Th1 immunity. PLoS Pathog. (2016) 12:e1005580. doi: 10.1371/journal.ppat.1005580
25. Brown AF, Murphy AG, Lalor SJ, Leech JM, O'Keeffe KM, Mac Aogáin M, et al. Memory Th1 Cells Are Protective In Invasive Staphylococcus aureus infection. PLoS Pathog. (2015) 11:1–32. doi: 10.1371/journal.ppat.1005226
26. Lin L, Ibrahim AS, Xu X, Farber JM, Avanesian V, Baquir B, et al. Th1-Th17 cells mediate protective adaptive immunity against Staphylococcus aureus and Candida albicans infection in mice. PLoS Pathog. (2009) 5:e1000703. doi: 10.1371/journal.ppat.1000703
27. Kolata JB, Kühbandner I, Link C, Normann N, Vu CH, Steil L, et al. The fall of a Dogma? Unexpected high T-cell memory response to Staphylococcus aureus in humans. J Infect Dis. (2015) 212:830–8. doi: 10.1093/infdis/jiv128
28. Zielinski CE, Mele F, Aschenbrenner D, Jarrossay D, Ronchi F, Gattorno M, et al. Pathogen-induced human TH17 cells produce IFN-γ or IL-10 and are regulated by IL-1β. Nature. (2012) 484:514–8. doi: 10.1038/nature10957
29. Boero E, Mnich ME, Manetti AGO, Soldaini E, Grimaldi L, Bagnoli F. Human three-dimensional models for studying skin pathogens. In: Current Topics in Microbiology and Immunology. Berlin: Springer (2020).
30. Chassain B, Lemée L, Didi J, Thiberge JM, Brisse S, Pons JL, et al. Multilocus sequence typing analysis of Staphylococcus lugdunensis implies a clonal population structure. J Clin Microbiol. (2012) 50:3003–9. doi: 10.1128/JCM.00988-12
31. Mack D, Siemssen N, Laufs R. Parallel induction by glucose of adherence and a polysaccharide antigen specific for plastic-adherent Staphylococcus epidermidis: Evidence for functional relation to intercellular adhesion. Infect Immun. (1992) 60:2048–57. doi: 10.1128/IAI.60.5.2048-2057.1992
32. Olaniyi RO, Pancotto L, Grimaldi L, Bagnoli F. Deciphering the pathological role of staphylococcal α-Toxin and panton-valentine leukocidin using a novel ex vivo human skin model. Front Immunol. (2018) 9:951. doi: 10.3389/fimmu.2018.00951
33. Byrd AL, Belkaid Y, Segre JA. The human skin microbiome. Nat Rev Microbiol. (2018) 16:143–55. doi: 10.1038/nrmicro.2017.157
34. Da Silva Antunes R, Paul S, Sidney J, Weiskopf D, Dan JM, Phillips E, et al. Definition of human epitopes recognized in tetanus toxoid and development of an assay strategy to detect Ex vivo tetanus CD4+ T cell responses. PLoS ONE. (2017) 12:e0169086. doi: 10.1371/journal.pone.0169086
35. Choi Y, Kotzin B, Herron L, Callahan J, Marrack P, Kappler J. Interaction of Staphylococcus aureus toxin “superantigens” with human T cells. Proc Natl Acad Sci USA. (1989) 86:8951–45. doi: 10.1073/pnas.86.22.8941
36. Puel A, Cypowyj S, Maródi L, Abel L, Picard C, Casanova JL. Inborn errors of human IL-17 immunity underlie chronic mucocutaneous candidiasis. Curr Opin Allergy Clin Immunol. (2012) 12:616–22. doi: 10.1097/ACI.0b013e328358cc0b
37. Puel A. Human inborn errors of immunity underlying superficial or invasive candidiasis. Hum Genet. (2020) 139:1011–22. doi: 10.1007/s00439-020-02141-7
38. Peric M, Koglin S, Kim S-M, Morizane S, Besch R, Prinz JC, et al. IL-17A enhances vitamin D3 -induced expression of cathelicidin antimicrobial peptide in human keratinocytes. J Immunol. (2008) 181:8504–12. doi: 10.4049/jimmunol.181.12.8504
39. Archer NK, Adappa ND, Palmer JN, Cohen NA, Harro JM, Lee SK, et al. Interleukin-17A (IL-17A) and IL-17F are critical for antimicrobial peptide production and clearance of Staphylococcus aureus nasal colonization. Infect Immun. (2016) 84:3575–83. doi: 10.1128/IAI.00596-16
40. Ishigame H, Kakuta S, Nagai T, Kadoki M, Nambu A, Komiyama Y, et al. Differential roles of interleukin-17A and−17F in host defense against mucoepithelial bacterial infection and allergic responses. Immunity. (2009) 30:108–19. doi: 10.1016/j.immuni.2008.11.009
41. Dudakov JA, Hanash AM, Van Den Brink MRM. Interleukin-22: immunobiology and pathology. Annu Rev Immunol. (2015) 33:747–85. doi: 10.1146/annurev-immunol-032414-112123
42. Avitabile S, Odorisio T, Madonna S, Eyerich S, Guerra L, Eyerich K, et al. Interleukin-22 promotes wound repair in diabetes by improving keratinocyte pro-healing functions. J Invest Dermatol. (2015) 135:2862–70. doi: 10.1038/jid.2015.278
43. Mulcahy ME, Leech JM, Renauld JC, Mills KHG, McLoughlin RM. Interleukin-22 regulates antimicrobial peptide expression and keratinocyte differentiation to control Staphylococcus aureus colonization of the nasal mucosa. Mucosal Immunol. (2016) 9:1429–41. doi: 10.1038/mi.2016.24
44. Tamoutounour S, Han SJ, Deckers J, Constantinides MG, Hurabielle C, Harrison OJ, et al. Keratinocyte-intrinsic MHCII expression controls microbiota-induced Th1 cell responses. Proc Natl Acad Sci USA. (2019) 116:23643–52. doi: 10.1073/pnas.1912432116
45. Upadhyay V, Fu YX. Lymphotoxin signalling in immune homeostasis and the control of microorganisms. Nat Rev Immunol. (2013) 13:270–9. doi: 10.1038/nri3406
46. Mounzer RH, Svendsen OS, Baluk P, Bergman CM, Padera TP, Wiig H, et al. Lymphotoxin-alpha contributes to lymphangiogenesis. Blood. (2010) 116:2173–82. doi: 10.1182/blood-2009-12-256065
47. Nakagawa S, Matsumoto M, Katayama Y, Oguma R, Wakabayashi S, Nygaard T, et al. Staphylococcus aureus virulent PSMα peptides induce keratinocyte alarmin release to orchestrate IL-17-dependent skin inflammation. Cell Host Microbe. (2017) 22:667–77. doi: 10.1016/j.chom.2017.10.008
48. Liu H, Archer NK, Dillen CA, Wang Y, Ashbaugh AG, Ortines RV, et al. Staphylococcus aureus epicutaneous exposure drives skin inflammation via IL-36-mediated T cell responses. Cell Host Microbe. (2017) 22:653–66. doi: 10.1016/j.chom.2017.10.006
49. Leech JM, Dhariwala MO, Lowe MM, Chu K, Merana GR, Cornuot C, et al. Toxin-triggered interleukin-1 receptor signaling enables early-life discrimination of pathogenic versus commensal skin bacteria. Cell Host Microbe. (2019) 26:795–809.e5. doi: 10.1016/j.chom.2019.10.007
50. Amezcua Vesely MC, Pallis P, Bielecki P, Low JS, Zhao J, Harman CCD, et al. Effector TH17 cells give rise to long-lived TRM cells that are essential for an immediate response against bacterial infection. Cell. (2019) 178:1176–88.e15. doi: 10.1016/j.cell.2019.07.032
51. Constantinides MG, Link VM, Tamoutounour S, Wong AC, Perez-Chaparro PJ, Han SJ, et al. MAIT cells are imprinted by the microbiota in early life and promote tissue repair. Science. (2019) 366:eaax6624. doi: 10.1126/science.aax6624
52. Marchitto MC, Dillen CA, Liu H, Miller RJ, Archer NK, Ortines RV, et al. Clonal Vγ6+Vδ4+ T cells promote IL-17–mediated immunity against Staphylococcus aureus skin infection. Proc Natl Acad Sci USA. (2019) 116:10917–26. doi: 10.1073/pnas.1818256116
53. Visvabharathy L, Id SG, Id LC, He Y, Alonzo F, Id III, et al. Group 1 CD1-restricted T cells contribute to control of systemic Staphylococcus aureus infection. PLoS Pathog. (2020) 16:e1008443. doi: 10.1371/journal.ppat.1008443
54. Beura LK, Hamilton SE, Bi K, Schenkel JM, Odumade OA, Casey KA, et al. Normalizing the environment recapitulates adult human immune traits in laboratory mice. Nature. (2016) 532:512–6. doi: 10.1038/nature17655
55. Reese TA, Bi K, Kambal A, Filali-Mouhim A, Beura LK, Bürger MC, et al. Sequential infection with common pathogens promotes human-like immune gene expression and altered vaccine response. Cell Host Microbe. (2016) 19:713–9. doi: 10.1016/j.chom.2016.04.003
56. Williams MR, Cau L, Wang Y, Kaul D, Sanford JA, Zaramela LS, et al. Interplay of staphylococcal and host proteases promotes skin barrier disruption in netherton syndrome. Cell Rep. (2020) 30:2923–33.e7. doi: 10.1016/j.celrep.2020.02.021
57. Bonifacius A, Goldmann O, Floess S, Holtfreter S, Robert PA, Nordengrün M, et al. Staphylococcus aureus alpha-toxin limits type 1 while fostering type 3 immune responses. Front Immunol. (2020) 11:1579. doi: 10.3389/fimmu.2020.01579
58. Zhang F, Ledue O, Jun M, Goulart C, Malley R, Lu YJ. Protection against Staphylococcus aureus colonization and infection by B-and T-cell-mediated mechanisms. MBio. (2018) 9:1–13. doi: 10.1128/mBio.01949-18
59. Pasparakis M, Haase I, Nestle FO. Mechanisms regulating skin immunity and inflammation. Nat Rev Immunol. (2014) 14:289–301. doi: 10.1038/nri3646
60. Yang ES, Tan J, Eells S, Rieg G, Tagudar G, Miller LG. Body site colonization in patients with community-associated methicillin-resistant Staphylococcus aureus and other types of S. aureus skin infections. Clin Microbiol Infect. (2010) 16:425–31. doi: 10.1111/j.1469-0691.2009.02836.x
61. Williams RE. Healthy carriage of Staphylococcus aureus: its prevalence and importance. Bacteriol Rev. (1963) 27:56–71. doi: 10.1128/BR.27.1.56-71.1963
62. Klicznik MM, Morawski PA, Höllbacher B, Varkhande SR, Motley SJ, Kuri-Cervantes L, et al. Human CD4+CD103+ cutaneous resident memory T cells are found in the circulation of healthy individuals. Sci Immunol. (2019) 4:eaav8995. doi: 10.1126/sciimmunol.aav8995
63. Hegazy AN, West NR, Stubbington MJT, Wendt E, Suijker KIM, Datsi A, et al. Circulating and tissue-resident CD4+ T cells with reactivity to intestinal microbiota are abundant in healthy individuals and function is altered during inflammation. Gastroenterology. (2017) 153:1320–37.e16. doi: 10.1053/j.gastro.2017.07.047
64. Bagnoli F, Bertholet S, Grandi G. Inferring reasons for the failure of Staphylococcus aureus vaccines in clinical trials. Front Cell Infect Microbiol. (2012) 2:16. doi: 10.3389/fcimb.2012.00016
65. Vella V, Galgani I, Polito L, Arora AK, Creech CB, David MZ, et al. Staphylococcus aureus skin and soft tissue infection recurrence rates in outpatients: a retrospective database study at three US medical centers. Clin Infect Dis. (2020). doi: 10.1093/cid/ciaa1717
Keywords: Staphylococcus aureus, infection, immunity, human skin, tissue-resident memory T cells, CD4+ T cells
Citation: Hendriks A, Mnich ME, Clemente B, Cruz AR, Tavarini S, Bagnoli F and Soldaini E (2021) Staphylococcus aureus-Specific Tissue-Resident Memory CD4+ T Cells Are Abundant in Healthy Human Skin. Front. Immunol. 12:642711. doi: 10.3389/fimmu.2021.642711
Received: 16 December 2020; Accepted: 18 February 2021;
Published: 16 March 2021.
Edited by:
Fabienne Tacchini-Cottier, University of Lausanne, SwitzerlandReviewed by:
Mirian Nacagami Sotto, University of São Paulo, BrazilOliver Harrison, Benaroya Research Institute, United States
Copyright © 2021 Hendriks, Mnich, Clemente, Cruz, Tavarini, Bagnoli and Soldaini. This is an open-access article distributed under the terms of the Creative Commons Attribution License (CC BY). The use, distribution or reproduction in other forums is permitted, provided the original author(s) and the copyright owner(s) are credited and that the original publication in this journal is cited, in accordance with accepted academic practice. No use, distribution or reproduction is permitted which does not comply with these terms.
*Correspondence: Elisabetta Soldaini, ZWxpc2FiZXR0YS54LnNvbGRhaW5pQGdzay5jb20=
†These authors have contributed equally to this work and share first authorship