- 1Department of Radiology, The Third Xiangya Hospital of Central South University, Changsha, China
- 2Molecular Imaging Research Center, Central South University, Changsha, China
The complex tumor microenvironment (TME) plays a vital role in cancer development and dramatically determines the efficacy of immunotherapy. Tertiary lymphoid structures (TLSs) within the TME are well recognized and consist of T cell-rich areas containing dendritic cells (DCs) and B cell-rich areas containing germinal centers (GCs). Accumulating research has indicated that there is a close association between tumor-associated TLSs and favorable clinical outcomes in most types of cancers, though a minority of studies have reported an association between TLSs and a poor prognosis. Overall, the double-edged sword role of TLSs in the TME and potential mechanisms need to be further investigated, which will provide novel therapeutic perspectives for antitumor immunoregulation. In this review, we focus on discussing the main functions of TLSs in the TME and recent advances in the therapeutic manipulation of TLSs through multiple strategies to enhance local antitumor immunity.
Introduction
Tumors originate and develop in a complicated and dynamic microenvironment, and there are endothelial cells, immune cells, and stromal cells existing around or within the tumor microenvironment (TME) and interacting with tumor cells (1, 2). Effective antitumor immunity is recognized to require the existence and activation of a variety of immune cells, including B cells, CD8+ T cells, and CD4+ T cells, etc. This concept is confirmed by the presence of intratumoral tertiary lymphoid structures (TLSs), which are well-organized tumor-infiltrating lymphocyte (TIL) clusters and may generate an advanced immune response (3). As is known to us, immunotherapy can utilize positive feedback to activate the immune system and boost the infiltration of endogenous T cells into tumors and subsequent destruction of tumor cells (4–6). However, only 5%-30% of patients with malignancies exhibit activated intratumoral T cell immunity after anti-programmed cell death protein-1 (PD-1)/programmed death-ligand 1 (PD-L1) immunotherapy (7, 8). This failure is mainly due to the extensive immunosuppressive mechanisms in the TME that lead to the decreased number and dysfunction of infiltrating T cells (9–11).
TLSs are ectopic lymphoid organs that can develop at sites of chronic inflammation, such as those associated with infection and autoimmunity, but also form within the TME (12, 13). TLSs share similar structural and functional characteristics with secondary lymphoid organs (SLOs) (14). However, TLSs lack a capsule and can form in various nonlymphoid tissues, such as stroma and epithelium (15). The prognostic impact of TLSs has been widely explored and most reports have indicated that TLSs are associated with positive immunoreactivity and favorable clinical outcomes in most types of cancers (12, 16–20). For example, TLSs are shown to be associated with relapse-free survival in patients with oral carcinoma or early-stage hepatocellular carcinoma (21, 22). Moreover, germinal centers (GCs) in TLSs may determine the prognostic value of TLSs (23, 24). Even though, TLSs show an association with poor prognosis in a minority of studies (25–27). It is urgent to comprehensively illustrate the function of TLSs in the TME.
SLOs, such as lymph nodes (LNs), provide three-dimensional structures for immune cells to optimize cell-cell interactions and produce an effective immune response (28, 29). Effector T (Teff) cells are activated after being instructed by DCs, and migrate from external draining LNs into the tumor to exert their function (30, 31). Increasing studies have shown that the antitumor immune response originates not only in LNs but also directly in TLSs (32). In general, the cells and molecules that regulate the signaling underlying TLS formation and promote immune responses within TLS remain to be further studied. In this review, we briefly summarize the development of TLSs and focus on discussing the function of TLSs and multiple approaches that had been developed to induce TLS formation.
Development and Formation of TLSs
SLO development is a highly organized process that is initiated and continued during embryogenesis, which has similarities to TLS formation and provides a classical model for understanding TLS development (33). However, there are some differences between the canonical SLOs and TLSs. A chronic inflammatory state is sufficient to induce TLS formation even in the absence of lymphoid tissue inducer (LTi) cells (34), indicating that chronic inflammation may be an important factor that favors lymphoid neogenesis and promotes TLS formation (35). Some studies have revealed that DCs (36), T helper 17 (Th17) cells (37, 38), B cells (39), M1 macrophages (40), and T follicular helper (TFH) cells (41) can initiate TLS neogenesis in various pathological conditions. In addition, group 3 innate lymphoid cells (ILC3s) are associated with ectopic lymphoid aggregates (42). Tumor-infiltrating ILC3s may interact with fibroblasts and lung tumor cells to facilitate cytokine release, contributing to protective TLS formation (43). Lymphotoxin (LT) signaling plays a vital role in TLS formation (44, 45). The LTα1β2-LTβ receptor (LTβR) interaction initiates signaling that leads to the production of various chemokines and adhesion molecules, such as CCL19, CCL21, CXCL13, and CXCL12 (46). CCR7-expressing T cells are recruited by their homologous ligands CCL21 and CCL19, and this recruited population forms a T cell zone. In addition, B cells can express CXCR5 and CXCR4 on their surface to transmigrate to the follicle through the activity of the CXCL12-CXCR4 and CXCL13-CXCR5 axis (47, 48). These findings show that the CCL19/CCL21-CCR7 and CXCL13-CXCR5 axes are vital for regulating TLS development (49). Although the knowledge of TLS formation mechanisms is widely researched, the possible mechanisms and factors need to be further explored in future studies. The main molecular and cellular mechanisms of TLS formation are shown in Figure 1.
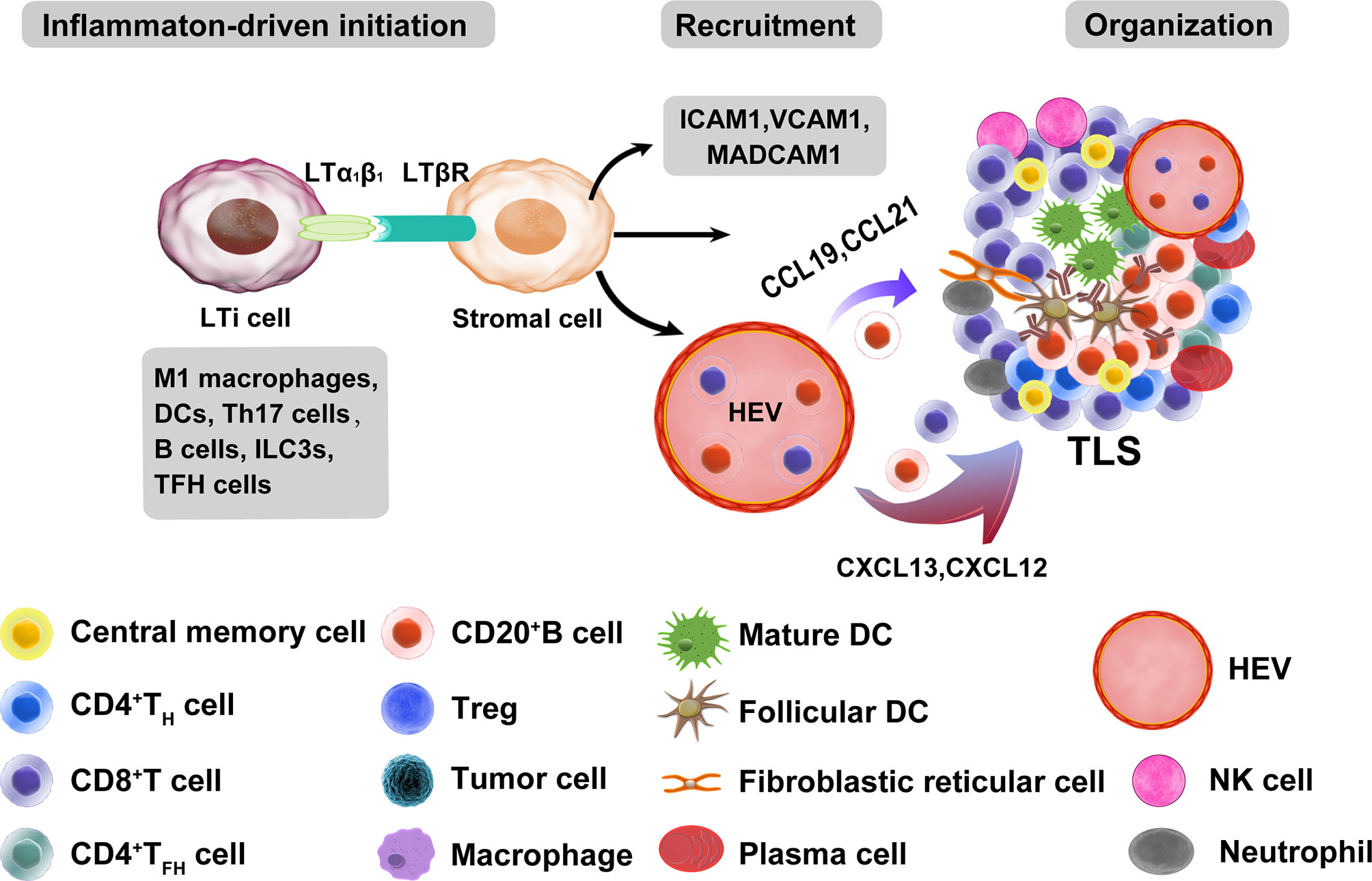
Figure 1 Main molecular and cellular mechanisms of TLS formation in tumors. The development of TLSs is similar to that of SLOs. A chronic inflammatory state is sufficient to induce TLS formation in the absence of lymphoid tissue inducer (LTi) cells. Many immune cells can be used as LTi cells, such as B cells, DCs, M1 macrophages, Th17 cells, ILC3s, and TFH cells. Immune and stromal cell cross-talk mediates TLS formation mainly through the binding of lymphotoxin (LT) αβ and LTβR, which can further release many chemokines (CXCL13, CXCL12, CCL21, and CCL19) and adhesion molecules (VCAM1, ICAM1, and MADCAM1). These chemokines recruit lymphocytes from HEVs and form T and B cell zones. ILC3s, group 3 innate lymphoid cells; VCAM1, vascular cell adhesion molecule 1; ICAM1, intercellular adhesion molecule 1; MADCAM1, mucosal addressable cell adhesion molecule 1.
The Function of TLSs in the TME
Favorable Impact of TLSs on Antitumor Properties
Mature TLSs are similar to SLOs, which contain T cell-rich areas with CD3+ T cells and dendritic cell (DC)-lysosomal associated membrane protein+ (DC-LAMP) mature DCs and follicular CD20+ B cell-rich zones (12). The B cell follicles in TLSs comprise follicular dendritic cells (FDCs), B cells, plasmablasts, and TFH cells required for GC formation and B cell differentiation (50). Macrophages, neutrophils, and regulatory T (Treg) cells have been discovered in the TLSs of lung cancer, pancreatic cancer, and ovarian cancer (51–54). TLSs are divided into classical and nonclassical structures. Classical structures are mature and contain T cells, DCs, B cells, and FDC compartments and comprise more active components than nonclassical structures, mainly B cells. Nonclassical TLSs usually contain B cells that are less activated than those in classical structures (14). TLSs are distributed intratumorally and peritumorally and are more abundant in the invasive margin than in the tumor core (12). Intratumoral TLSs may have greater prognostic significance, but this has not been widely established. In some studies, intratumoral TLSs are a favorable prognosticator in pancreatic cancer and hepatocellular carcinoma (20, 21). One study proposed a hypothesis to explain the better prognosis of intratumoral TLSs. Tumors with, rather than without, intratumoral TLSs are less invasive, especially regarding blood vessel invasion, and have a role related to the immune response. These tumors retain a relatively complete vascular network to transport immune cells and other molecules into the tumor and initiate a more effective antitumor immune response (17).
Increasing evidence shows that TLSs play an important role in controlling tumor invasion. Mature TLSs exhibit evidence for the formation of GCs (24) and GC B cells in TLSs are characterized by FDCs and Ki67+ proliferating B cells (51). Oligoclonal B cell responses have been identified in melanoma, which suggests an active humoral antitumor response within TLSs that is driven by B cells (55). High PC counts are associated with higher numbers of TLSs and B cells in breast cancer and neck carcinomas (56, 57). PCs surrounded by TLSs are associated with the highest levels of TILs and cytotoxicity-related gene products in ovarian cancer. This study showed that CD8+ TILs can predict prognosis only in combination with PCs, CD20+ TILs, and CD4+ TILs, suggesting that these four lymphocyte subsets work in concert to promote antitumor immunity, which indicates that TLSs may facilitate coordinated antitumor responses involving the combined actions of cytolytic T cells and PCs (58). B cells in TLSs are organized and highly differentiated and can produce tumor-specific antibodies in adenocarcinomas and ovarian cancer (59). In omental metastases from ovarian cancer, memory B lymphocytes essentially located within TLSs had higher clonality and somatic hypermutation rates, and they produced chemokines attracting DCs, T cells, and natural killer (NK) cells. The density of B cells also correlated with that of mature DCs in the stroma of tumors (53). Recent studies have shown the important role of TLSs and B cells in immunotherapy. The frequencies of memory B cells, PCs, and GC-like B cells in the tumors of responders treated with immune checkpoint blockade (ICB) therapy are significantly higher than those in nonresponders. Increased B cell proliferation indicating GC activity and formation within TLSs has been observed (60). High expression of B-lineage markers is associated with improved prognosis and TLS formation in sarcoma (61). B cells within TLSs can predict favorable prognosis in melanoma patients receiving ICB therapy. In addition, B cell-rich tumors are associated with elevated levels of initial and memory T cells. T cells in tumors without TLSs possess a dysfunctional molecular phenotype, which indicates that TLSs have a key role in the melanoma TME by conferring distinct T cell phenotypes (62). In summary, these studies demonstrate a major role of TLS-associated B cells in TLS function. B cells probably act together with key immune constituents of TLSs by altering T cell activation and function. Memory B cells may act as antigen-presenting cells (APCs) to drive the expansion of both memory and naive T cell responses. B cells can also activate and recruit other immune effector cells by secreting an array of cytokines (60). The potential functions of TLSs in the TME are shown in Figure 2.
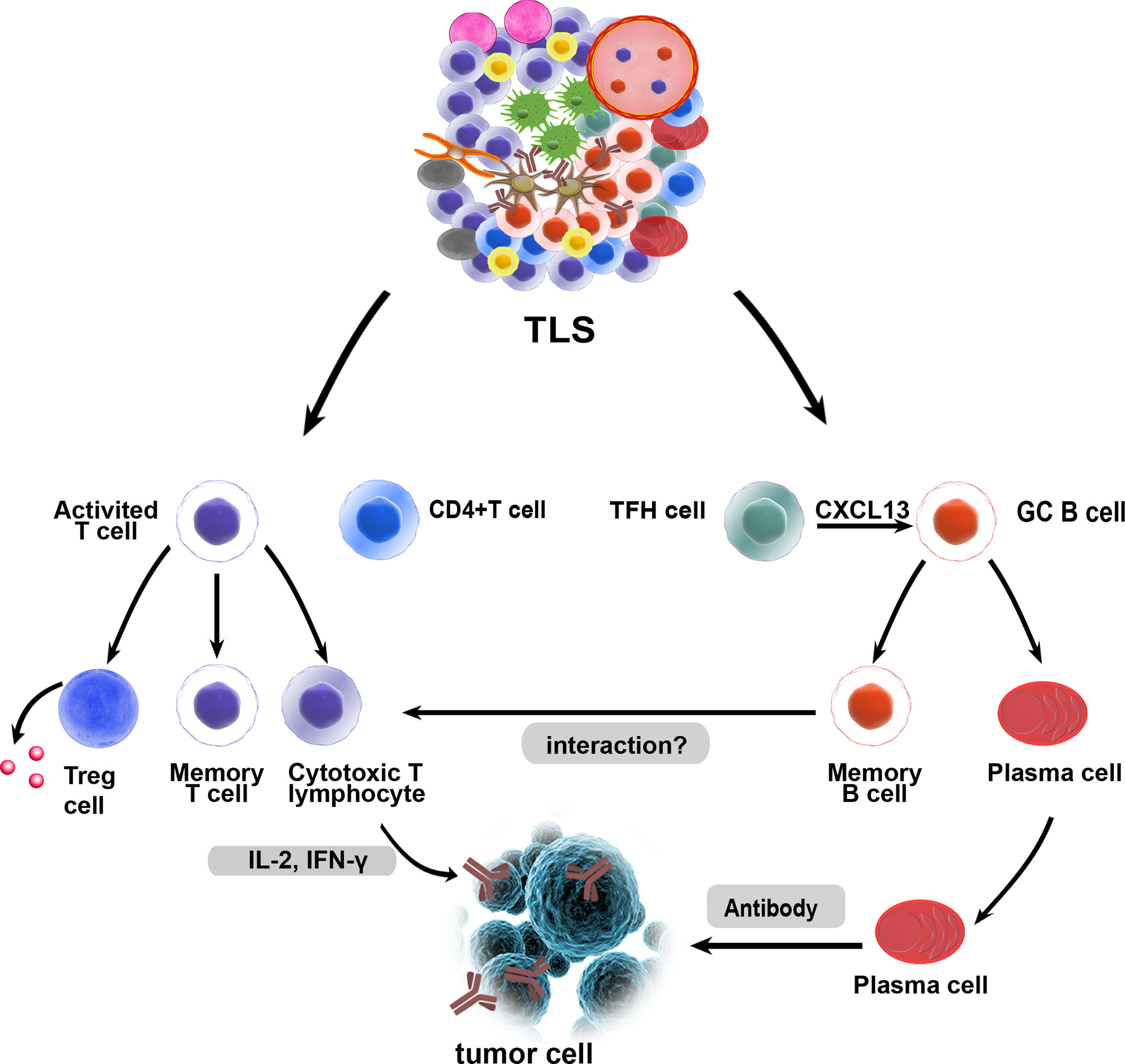
Figure 2 Potential functions of tertiary lymphoid structures in the TME. As in canonical SLOs, TLSs may constitute a critical site where specific T and B cells can undergo terminal differentiation into effector cells. GC B cells can differentiate into memory B cells and plasma cells in TLSs, and fully differentiated B cells and exert their antitumor effects. T cells can differentiate and expand, and they are activated as effector cells that exert cytotoxic effects. B cells and T cells in TLSs may interact with each other and play a synergistic role, which needs to be confirmed by more studies. Treg cells within TLSs could exert a negative influence on the capacity of TLSs to generate effector and memory lymphocytes.
The existence of TLSs at metastatic tumor sites is the key factor in the level of TILs, which directly determines the antitumor effect (19). Moreover, the presence of TLSs led to increased infiltration and activation of T cells and other immune cells and was associated with a good prognosis in liver cancer and pancreatic carcinoma (21, 63, 64). There is evidence that TLSs can activate effector T cells in tumors (65). In MC38 tumors, T cells from TLSs exhibited a largely enhanced baseline level of IFN-γ (interferon-gamma) release. This finding revealed successful antitumor T cell priming activity within induced TLSs, and TLSs may act as immune factories where T cells activate effector cells to mediate synergistic antitumor effects (66). Studies of lung and ovarian cancers showed that TLS-associated DCs establish unique immune states characterized by a strong T helper (Th) 1 orientation and facilitate a good prognosis, indicating that antigen presentation allows local T cells to initiate responses to tumor-associated antigens in TLSs (67, 68). Whether TLS-associated DCs present tumor antigens directly to CD8+ T cells or whether CD4+ Th cells participate in the production of CD8+ cytotoxic T cell responses in TLSs remains to be further studied. TFH cells produce CXCL13, potentially resulting in the formation of TLSs to trigger the GC B cell response (69). FDCs can also produce chemokines and cytokines involved in B cell proliferation and migration in LN, such as interleukin (IL) -6 and CXCL13 (70). B cells produce LTα1β2, which has a crucial function in the differentiation of FDCs within TLSs (71).
HEVs in TLSs are associated with T and B cell infiltration and indicate favorable outcomes in oral carcinoma and breast cancer (72, 73). The emergence of HEVs also contributes to the formation of TLSs (74). There is ample evidence that the function of HEVs in TLSs is similar to that in LNs, providing a channel for immune cells to accumulate in the tumor. HEVs in TLSs express molecules similar to those expressed in LNs, such as CCL21 and peripheral node addressing (PNAd), and cells expressing CCR7 and L-selectin ligands for receptors on HEVs are found in TLSs (75). PNAd expression indicates that HEVs are essential for recruiting lymphocytes to lymphoid organs (76), an event that orchestrates the extravasation of Lselectin+ and CCR7+ immune cells into TLSs (77, 78). LTαβ plays a key role in PNAd expression in HEV (79). Single-cell analysis revealed the heterogeneity of HEVs in LN. LTβR signaling and inflammation also have crucial effects on HEV transcriptomes (80). However, the study showed that HEV neogenesis is dependent on tumor necrosis factor receptor (TNFR) rather than LTβR signaling in Treg-depleted tumors, suggesting another mechanism for HEV formation. The expression of PNAd is not dependent on the LTβR signal but is stimulated by activation of TNFR mediated by LTα3 derived from CD8+ T cells (81). HEV formation is associated with increased T and B lymphocyte infiltration and activation in murine pancreatic cancer and breast cancer (82, 83). In mouse models of melanoma and lung cancer, the LN-like vasculature in tumors, characterized by the expression of PNAd and chemokine CCL21, induced by effector lymphocytes allows naive T cells to enter tumors and enhance antitumor immunity. Vasculogenesis is regulated by a mechanism involving CD8+ T cells that secrete IFN-γ and LTα3 (84). In summary, T cells may contribute to the formation of the peripheral vasculature and HEV.
The Adverse Impact of TLSs on Tumors
Nevertheless, a few studies have indicated that TLSs have a negative impact on prognosis in colorectal cancer and breast cancer (25–27). The studies showed that TLSs that develop in the inflamed liver during hepatitis can function as a niche for tumor progenitor cells in hepatocellular carcinoma and are associated with an increased risk of late recurrence and decreased survival. It can be postulated that TLSs, which persist in the liver and are associated with a viral infection, play a different role than TLSs induced by tumors (25). Lymphoid aggregates are associated with more advanced diseases and indicate an adverse prognosis in colorectal cancers. These structures form in association with more advanced tumors, suggesting that they are a reaction to progressive tumor invasion, and their prognostic significance varies with disease progression and according to the inherent immunogenicity of the tumor (26). TLSs are associated with adverse prognosis in renal carcinoma with lung metastasis. TLSs are rarely found in lung metastasis of renal carcinoma, and studies speculated that the presence of T cells may not be educated in peritumoral TLSs but may reflect a chronic inflammatory response, which is known to be harmful to the host. At the same time, the high expression of vascular endothelial growth factor (VEGF) and IL-6 genes in renal carcinoma may also inhibit the differentiation of DCs, resulting in an impaired T cell response and poor prognosis (85). TLS Treg cells are detected in breast and colorectal cancers (86, 87). The decrease in the number of TLS Treg cells is associated with tumor regression in metastatic prostate cancer (88). Treg cells are present in TLSs in tumor-bearing lungs and exhibit activated phenotypes. Costimulatory ligand expression by DCs and T cell proliferation rates increased in TLSs after Treg cell depletion, enhancing the antitumor immune response. The reason may be that Treg cells in TLSs regulate DC function by reducing costimulatory levels, the immunosuppression of Treg cells to DCs is relieved after Treg cell depletion, and the TLS microenvironment may become more immunostimulatory to promote antitumor responses by T cells (54). The recruitment of Treg cells and myeloid-derived suppressor cells (MDSCs) to lymphoid aggregates in mouse B16 melanomas expressing CCL21 was found to correlate with the promotion of tumor growth (89). Therefore, TLS-associated Treg cells and MDSC presence may exert a negative influence on the capacity of TLSs to generate effector and memory lymphocytes.
Development of Multiple Approaches to Induce the TLS Formation
A variety of LN modifications to improve the efficacy of tumor immunotherapy have been widely discussed and researched. Targeting LN can affect the efficacy of cancer vaccines, ICB therapy, and adoptive cell transfer (ACT) at the cellular level. Macroscopic biomaterials mimicking LN characteristics can be used as immune niches for cell reprogramming and in vivo transmission and can be used for preclinical testing of drugs and vaccines in vitro at the tissue level (90). TLSs may be the first line of T cell differentiation and expansion and are the key to inducing intratumoral immune sensitization in situ. Therefore, similar principles can be used for developing strategies to induce TLS formation, and a new antitumor immune strategy can be constructed. Although biomaterials for transporting or recruiting APCs can mimic the cellular characteristics of SLOs, other strategies aim to induce TLS formation specifically, as observed in situ. These strategies aim to mimic the chemokine and inflammatory signals of the main molecular and cellular mechanisms of TLS formation. In the next section, we discuss strategies that induce TLS formation through the delivery of chemokine-expressing cells or chemokines, implantation of biomaterial scaffolds containing these inflammatory factors and agents, and multiple therapeutic approaches.
Chemokines and Cytokines
A chemokine delivery strategy for TLSs provides a convenient way to generate ectopic lymphoid tissue in tumors. Recent electronic screening techniques involving the identification of TLS-related chemokine genes that induce lymphocyte chemotaxis have offered a framework for a more effective design of TLSs (91, 92). A 12-chemokine gene signature also provided a promising starting point for the potential construction of designed TLSs (93–96). In early studies, chemokines produced by lymphoid structures were expressed in various ways, which led to the formation of lymphoid tissue structures. For example, transgenic mice expressed B lymphocyte chemokines in pancreatic islets, and the expression of B lymphocyte chemokines resulted in the formation of LN-like structures that included HEVs, interstitial cells, and B and T cell zones and illustrated that the maintenance of B lymphocyte chemokine-induced lymphoid structures depends on LTβR signaling (97). CCL21 exhibits a stronger capacity than CCL19 to induce more organized infiltrates in the islets of transgenic mice (98). Intratumoral injection of CCL21 facilitated lymphocyte infiltration into pancreatic tumors (99), and targeting lymphotoxin-α can induce lymphocyte infiltration and lymphoid-like tissue formation in B16 melanoma (100). LN-like lymphocyte infiltration was also found in transgenic mice expressing CCL21 driven by the thyroglobulin promoter in the thyroid gland and transgenic pancreas (101, 102). Type I interferon can also drive B cell recruitment by CXCR5–CXCL13 signaling and initiate ectopic GC formation within TLSs in pulmonary virus infection (103). In the salivary glands of adult mice, IL-7 regulates lymphatic vessel expansion and promotes the neogenesis of TLSs in the first phase, and LTβR signaling regulates TLS neogenesis in the second phase (104). Th17 cytokines can regulate TLS development and function. For instance, IL-22 modulates CXCL12, CXCL13, and IL-23 production, contributing to the formation of TLSs (105–107). In conclusion, these studies show that many chemokines and cytokines involved in lymphoid structure formation can be used as novel and feasible inducers in combination with other stimulants and multiple methods to induce the formation of TLSs.
LIGHT, the 14th member of the tumor necrosis factor superfamily (TNFSF14), is a protein primarily expressed on activated T cells and immature DCs (108). LIGHT can function as both a soluble and cell surface-bound type II membrane protein and interact with its two primary functional receptors: Herpes Virus Entry Mediator and LTβR (109). LIGHT can interact with Herpes Virus Entry Mediator and deliver co-stimulatory signals to T cells (44). LTβR is found on the surface of epithelial, stromal, and myeloid cells (110). LIGHT-LTβR signaling plays an important role in immune responses, functioning to repair tumor vasculature and to support effector cells cell trafficking to and infiltration into tumors (111). Recently, LTβR signal transduction induced by LIGHT has become a focus of the investigation. When combined with an anti-VEGF antibody, LIGHT can activate LTβR signaling and mediate chemokine production to recruit T cells (112). In pancreatic cancer, targeting LIGHT for homing to tumor vessels via a vascular targeting peptide (VTP), LIGHT-VTP showed a dual ability to induce TLS formation and regulate the angiogenic vasculature (83). LIGHT targeting to tumor vessels induces vessel normalization, and HEVs and TLS formation may occur through a self-amplifying loop in pancreatic cancer. The mechanism may involve the LIGHT-triggered expression of inflammatory cytokines in macrophages, such as IL-1β, IL-6, CXCL13, TNF, and CCL21, These chemokines further recruit T cells. Macrophages and T cells have been deemed essential for HEV and TLS formation (83, 113) (Figure 3A). LIGHT-VTP in combination with ICB therapy can produce intratumoral memory T cells and Teff cells and improve prognosis (114). In addition, LIGHT-VTP combined with anti-VEGF and ICB therapy can increase the frequency of HEVs and normalize tumor vessels and the accumulation of T cells in glioblastoma and lung metastases (115, 116). The LT-LIGHT axis provides key differentiation signals guiding the differentiation of the reticular network and vascular system, maintaining the mesenchymal differentiation pathway of the specialized network, and remodeling reactive LNs (117). The LTβR signaling pathway plays a critical role in HEV differentiation and function in LN (44). Because of the similarity between SLOs and TLSs, it is speculated that LTβR signaling is also involved in HEV differentiation and function in TLSs. Further studies are required to understand the precise mechanisms by which HEV formation in TLSs is induced and the effects of HEVs on different types of cancer. This knowledge may guide the therapeutic objectives of cancer interventions. Other means can also be used to deliver LIGHT to tumor sites, and the oncolytic activity of attenuated Salmonella Typhimurium was enhanced by the stable insertion of the gene encoding LIGHT. Attenuated S. Typhimurium expressing LIGHT inhibited the growth of primary tumors and the spread of lung metastasis (118). The findings suggest that avirulent bacteria can be used as targeted carriers for the local production of therapeutic proteins in tumors. In recent years, the potential use of exosomes in the treatment and control of many diseases has expanded because of their inherent characteristics in regulating complex intracellular pathways. The characteristics of exosomes can also be exploited to induce TLSs. Exosomes are extracellular vesicles derived from endosomes and have a diameter of approximately 40-160 nm. They can carry a variety of substances, such as proteins and DNA, to allow these substances to be absorbed by other cells (119, 120). Therefore, we hypothesize that exosomes can be used as carriers to load many chemokines and cytokines to induce the formation of TLSs.
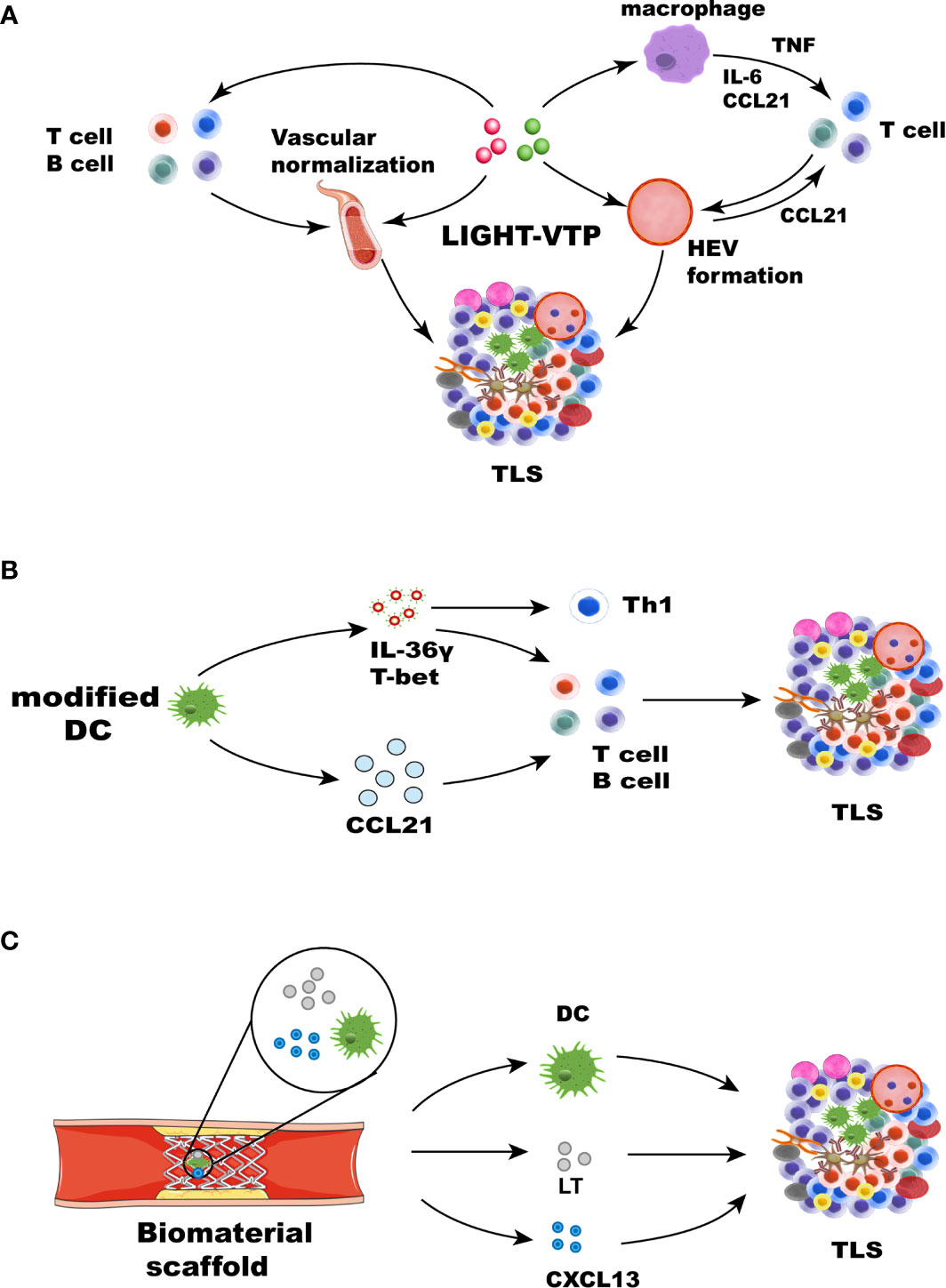
Figure 3 Strategies for therapeutic induction of TLS formation. TLS inducers, such as chemokines, cytokines, DCs, and therapeutic approaches, can induce TLS formation in different ways. (A) Cytokines and chemokines involved in lymphogenesis, including LIGHT, CCL21, CXCL13, LT, and IFN-γ, can lead to the formation of TLSs. LIGHT-VTP targeting tumor vessels can induce vessel normalization, and HEV and TLS formation may occur through a self-amplifying loop. The mechanism may be related to the LIGHT-triggered expression of the inflammatory cytokines IL-6, TNF, and CCL21 in macrophages. These chemokines can recruit T cells. Macrophages and T cells play an important role in the formation of HEVs and TLSs (83). HEV formation and vascular normalization can also recruit more immune cells. (B) Some immune cells, such as modified DCs and stromal cells, leading to the formation of TLSs. DCs engineered to secrete IL-36γ also initiate therapeutic TLS formation, which can upregulate the expression of T-bet. T-bet and IL-36γ cooperate to reinforce their expression and recruit immune cells, leading to TLS formation. DCs modified with the CCL21 gene can significantly increase T cell infiltration. Activated DCs can also upregulate many factors associated with TLS formation. (C) Biomaterials can provide 3D scaffolds in situ and deliver cells and chemokines. Collagen scaffolds containing LT, CCL21, CXCL13, and activated DCs were transplanted into mice. The recruited lymphocytes can form artificial lymph node structures.
Toll-like receptors (TLRs) have also been researched concerning TLS formation. Myofibroblasts were stimulated with TLR agonists and cytokines in giant cell arteritis, which upregulated B cell-activating factor and CXCL13 and resulted in the formation of TLSs (121). Inhalation of TLR9 agonists can generate profound remodeling of tumor-bearing lungs and lead to TLS formation in adjacent tumors (122). In addition, both the anti-HBV response to the TLR7 agonist GS-9620 and TLR4 agonists in mouse models of myasthenia gravis can induce TLS generation (123, 124). Transforming growth factor-β (TGF-β) plays a noncanonical role in coordinating immune responses against ovarian cancer. CD8+ T cells in the presence of TGF-β upregulate the secretion of CD103 and CXCL13, and CD8+ TILs play a role in mediating B cell recruitment and TLS formation (125).
Cells
An alternative approach to produce TLSs is to deliver cells that express chemokines or to engineer chemokines that are associated with lymphomagenesis. DC-based therapeutic strategies can be used therapeutically to promote the extranodal priming of antitumor immunity (126). DCs expressing T cell chemokines were injected into melanoma tumors, which yielded rapid T cell infiltration and initiation of intratumor responses (127). Additionally, intraperitoneal injection of murine DCs promoted the acute infiltration of immature T cells and NK cells into the TME, an effect related to upregulated expression of NK and T cell recruitment chemokines by murine DCs (128). DCs engineered to overexpress T-bet suppressed the growth of sarcomas in vivo after intratumoral injection and prolonged the overall survival of mice (126, 128). DCs promote LT signaling through LTβR for HEV differentiation and function in LN (129). DCs, which coordinate adaptive immune responses, have historically been a promising target. DCs are a source of LT, and homeostatic chemokines (CXCL12, CXCL13, CCL19, and CCL21) are known to contribute to TLS formation in the lungs of influenza virus-infected mice. Similar to the depletion of DCs, blockade of LTβR signaling after virus clearance leads to the disintegration of TLSs and GC reactions. It is suggested that the DC-mediated LTBR pathway contributes to the formation of TLSs (36). Other methods have focused on modifying and editing DCs to express the transcription factor T-bet and secrete IL-36γ to play a vital role. IL-36 cytokines are an IL-1 subfamily consisting of three agonists that signal through the common heterodimeric receptor IL-36R (130), which is expressed on endothelial cells and many immune cells, including T cells and DCs (131). IL-36γ is involved in polarizing type-1 immune responses. It is a downstream target of the type-1 transactivator T-bet and can induce T-bet expression in target cells (132). Research has shown that IL-36γ predominantly expressed by M1 macrophages and vasculature cells, including smooth muscle cells and HEVs, mediates polarization toward a type 1 immune response. This pattern of IL-36γ expression increased CD4+ central memory T cell infiltrate and the density of B cells and led to TLS formation in human colorectal cancer (133). The injection of tumors with DCs engineered to secrete a bioactive form of mIL-36γ also initiated therapeutic TLS formation and slowed tumor progression in a mouse model of colorectal carcinoma. Furthermore, DC.IL-36γ cells show strongly upregulated expression of T-bet, suggesting that T-bet and IL-36γ cooperate to reinforce each other’s expression in DCs, rendering them competent to promote TLS formation in the therapeutic TME (134) (Figure 3B). In lung cancer, autologous DCs transduced with an adenoviral vector modified with the CCL21 gene significantly reduced the tumor load and T cell infiltration (135), accompanied by enhanced expression of IFN-γ, IL-12, and CXCL10, as well as molecules related to reduced immunosuppression in the TME (136). Mice vaccinated with DCs charged with apoptotic/necrotic B16 cells are protected against B16 challenge, and TLSs form at the vaccination site (137). In conclusion, DCs play an important role in inducing TLS formation. These results provide a framework for the usage of DCs. Promoting the expression of multiple chemokines by targeting DCs is a valuable strategy to induce TLS formation.
LTi-like cells from newborn mouse LNs were injected intradermally into adult mice and formed TLSs in the skin, and the results indicated that hyperactivated lymphocytes can fulfill the role of LTi cells during inflammatory responses (138). Subcutaneous injection of the LN-derived stromal cell line resulted in the formation of TLSs that promote infiltration of immune cell subsets and inhibit tumor growth by improving the antitumor activity of TILs (66). Lymphoid tissue-like organoids were constructed by transplantation of stromal cells embedded in biocompatible scaffolds into the renal subcapsular space in mice. The structure is similar to that of SLOs and contains clusters of B and T cells and HEVs, DCs, and FDC networks (139). Other cells, such as immune fibroblasts, bone marrow mesenchymal stem cells (MSCs), adipocytes, and macrophages, also play their roles. Research on autoimmune conditions demonstrated that external triggers at mucosal sites can induce gradual differentiation of stromal cell populations into immune fibroblast networks, which supports the establishment of TLSs at an early stage. This process is mediated mainly by paracrine and autocrine signals regulated by IL-13. Once lymphocytes are recruited, the initial fibroblast network is expanded by local production of IL-22 and lymphotoxin. This finding demonstrates the role of immune fibroblasts in maintaining TLS and supporting their formation and identifies new therapeutic targets (140). Human MSCs stimulated with TNF-α and IL-1β significantly increased the expression of CCL19, VCAM1, ICAM1. Stimulated MSCs can induce CD4+ T cell proliferation. MSCs could play a key role as LTo cells in promoting the early inflammatory and initiating the formation of kidney-specific TLSs (141). Mucosa-resident CXCL13+CX3CR1hi macrophages are responsible for recruiting B cells and CD4+ T cells to sites of Salmonella invasion and subsequently activating them, resulting in TLS formation and a local pathogen-specific IgA response (142). Recently, the combination of TNF-α and lipopolysaccharide was shown to directly induce adipocytes to produce TLS-related chemokines, thereby coordinating the formation of functional TLSs in the mesentery affected by Crohn’s disease (143). In summary, these studies have further proved the various initiating factors and mechanisms of the formation of TLSs and provided more references and insights for inducing the TLS formation.
Biomaterials
Biomaterials can support the formation of TLSs by locally and controllably releasing chemokines and providing cellular support. Scaffolds are usually three-dimensional microporous structures designed to achieve cell encapsulation in vitro or cell penetration in vivo while providing mechanical support, cell adhesion capability, and a continuous supply of biological cues to promote cell migration and interaction (144). Biomaterial scaffolds can boost the efficacy of immunotherapies, such as cancer vaccines and ACT (145–147). For instance, biomaterials loaded with signaling molecules and engineered T cells have been evaluated in vitro. These biomaterials were surgically implanted near the tumor or under a resected tumor bed, where they maintained continuous proliferation and release of specific T cells (148).
In early cases, collagen scaffolds containing both thymus-derived stromal cells expressing LTα and activated DCs were transplanted into the renal subcapsular space of mice. The recruited lymphocytes formed artificial lymph nodes (ALN) structures, which contained FDC, T cell, and B cell regions and HEV-like structures. ALN induced a potent immune response in vivo and the accumulation of memory and effector T and B cells. The engineered structures elicited a humoral response after vaccination and could be transplanted into immunodeficient mice to secrete antibodies after secondary immunization (139, 149, 150) (Figure 3C). Based on this strategy, cell-free biomaterials have been explored. Hydrogels can provide a controlled cellular microenvironment for immune cells so that they can be recruited, expanded, and activated in vitro and in vivo (151). Hydrogels can be used to deliver antigens, chemokines, and other factors to DCs and induce T and B cell responses, and they can effectively encapsulate immunomodulators and immune cells. DCs can be activated in vitro in hydrogels before implantation and can be recruited and activated in gels by immobilized stimulators, as in a bioreactor (152, 153). In another study, collagen sponge scaffolds embedded with sustained-release gel beads containing LTα1β2, and many chemokines were transplanted into the subcapsular space of mice to establish ALN-like TLSs, recruiting memory T cells and B cells and induced a strong antigen-specific immune response (154). A synthetic immune priming center consisting of an in situ cross-linking hydrogel delivering chemokines and particles loaded with DNA and siRNA can attract numerous DCs and can both generate a strong transition to a T helper 1 response and increase the cytotoxic T lymphocyte (CTL) response. The multimode injectable system can simultaneously deliver chemokines, DNA, and siRNA antigens to DCs. This system constitutes a novel strategy to regulate immunotherapy in situ and could provide an effective vaccine strategy to prevent cancer (155).
Other biomaterials include polylactide-coglycolide (PLG), nano-sapper, and nanoparticles. A macroporous PLG matrix was used to deliver granulocyte-macrophage colony-stimulating factor (GM-CSF), tumor antigens, and danger signals in vivo. GM-CSF recruited DCs and significantly enhanced their homing to LNs, and danger signals and cancer antigens further activated the recruited DCs. These materials elicited protective antitumor immunity and showed prospects as cancer vaccines (156). A study demonstrated improved immune function by targeting DCs with adjuvant vector cells engineered from MKT cell ligands loaded with tumor antigen mRNAs. This method also enhanced the local immune response via TLS formation (157). Importantly, these polymers may be designed to program the transport of various types of cells in vivo. Nano-sapper was co-loaded with an antifibrotic protein and a plasmid expressing LIGHT. By normalizing the tumor vasculature, reducing collagen deposition, and stimulating the expression of lymphocyte-recruiting chemokines, Nano-sapper induces TLS formation to promote CTL infiltration and remodel the TME (158). Recognition of ectopic HEVs in human pancreatic ductal adenocarcinoma by engineered MECA79-coated nanoparticles can increase the transport of Taxol to the tumor and distinctly reduce tumor growth (159). Nanomaterials are promising for inducing TLS formation. Local delivery of engineered biomaterials can play a role by establishing synthetic immune niches to enhance antitumor immunity. Immunotherapy based on biomaterials will facilitate the development of the next generation of tumor therapies.
Other Therapeutic Approaches
Multiple cancer therapeutic strategies, such as cancer vaccines, ICB therapy, antiangiogenic therapy, radiotherapy, and chemotherapy, contribute to TLS formation. After therapeutic vaccination against human papillomavirus serotype 16 with E6/E7 antigens, significant immune changes in the TME were observed in subjects with CIN2/3, and TLSs formed in the immune-infiltrated cervical tissues. At the molecular level, these histological changes in the matrix were characterized by increased gene expression and associated with immune activation (CXCR3) and effector function (T-bet and IFN-β) (160). A prominent study of patients with resected pancreatic cancer showed that 33 of the 39 patients treated with the GM-CSF vaccine exhibited TLS formation after 2 weeks. Further analysis showed that these structures could regulate adaptive immunity. Inhibition of the Treg signaling pathway and enhancement of the Th17 signaling pathway in TLS aggregates were associated with increased survival and intratumoral Teff: Treg ratios and upregulation of the mechanism of immunosuppression (161). The findings help to guide the production of the next generation of effective cancer vaccines and facilitate better responses to ICB therapy. TLSs containing lymphocytes and APCs appeared in all 11 patients who received cisplatin neoadjuvant chemotherapy in a study on hepatoblastoma, indicating that cisplatin can induce TLS infiltration and synergistically induce the death of immunogenic cells and trigger an antitumor immune response. This may involve so-called immunogenic cell death, a controlled cell death process that produces damage-associated molecular patterns that can be used as adjuvants to initiate an immune response through the recruitment and activation of DCs (162). Administration of preoperative chemoradiotherapy (neoadjuvant chemotherapy, NAC) was associated with increased TLS formation and may affect the immunological composition of the TME and confer a favorable prognosis in patients with pancreatic ductal adenocarcinoma (163). However, corticosteroid therapy during chemotherapy impaired GC formation and reduced TLS prognostic value in patients with lung cancer (164). After radiotherapy, apoptosis in tumors with TLSs increased significantly. The TLSs also showed an acute increase in apoptosis and size reduction. Although their size tended to normalize after 2 weeks, the apoptotic rate remained high, suggesting active and continuous proliferation in residual irradiated cells and providing them with a window to optimize their unique function (165). Low-dose stimulator of interferon genes (STING) agonist treatment can upregulate the expression of various cytokines and increase the infiltration of T cells and DCs to establish a proinflammatory TME, which can also lead to normalization of the tumor vasculature, ultimately inducing the formation of TLSs and controlling tumor growth. Stimulating DC maturation and local production of vascular normalization-promoting and TLS-promoting factors, such as CCL19, CCL21, LTα, LTβ, and LIGHT (166). A study showed that Treg elimination can activate CD8+ T cells and promote the development of HEVs in tumors. The study proposed a model in which a positive feedback loop of T cell activation by Treg cell depletion can promote HEV development, T cell infiltration, and tumor destruction (81). A prostate cancer study showed that Treg cells and cyclooxygenase 2 are attractive therapeutic targets that can be used to strengthen TLS-driven tumor immunity. In particular, the existence of HEVs and lymphatic vessels suggests that TLSs can also be used as a platform for cell-based or cyclooxygenase 2 blockade therapy to control tumor growth (88).
PD-L1+, PD-L2+, LAG3+, and TIM3+ cells were detected in some breast cancer-related TLSs, and PD-1 was used as a marker of T cell activity in both the T and B cell areas in TLSs. The expression levels of immune checkpoint molecules were associated with the level of TILs and TLS formation (167). In a group of patients with renal carcinoma, the low expression of immune checkpoints and the localization of mature DCs in TLSs are associated with a better prognosis (168) (NCT03387761). Recently, some prominent studies have shown that B cell and TLS formation promote the immunotherapy response in patients with melanoma and sarcoma after ICB therapy (60–62). In a study of locoregionally advanced urothelial carcinoma, the formation of TLSs was observed in responding patients after treatment with combined CTLA-4 and PD-1 blockade therapy, which could be an effective preoperative treatment strategy (169). Another study compared the metabolic, transcriptional, and functional characteristics of intratumoral CD8+ T cell subtypes with high, moderate, and no PD-1 expression from patients with non-small cell lung carcinoma. PD-1+ high T lymphocytes produce CXCL13, which mediates the recruitment of immune cells to TLSs and has the potential to be predicted after treatment with PD-1 blockade therapy (170). Combination therapy with anti-VEGFR2 and anti-PD-L1 antibodies can induce HEV formation in pancreatic and breast cancer. LTβR signaling plays an important role in the generation and activation of tumor HEVs. HEV formation can increase the activity of CTLs, which makes tumors sensitive to ICB therapy (82). An anti-mouse LTβR agonistic antibody increased TIL infiltration in a mouse model of colon cancer. Agonistic monoclonal antibodies targeting LTβR are a novel method for treating colorectal cancer and potentially other types of cancer (171). Considering that the formation of TLSs is strongly related to the LTBR signaling pathway, targeting LTβR can also be used as an approach to induce TLS formation and enhance antitumor immunity.
TLSs formation induced by multiple therapeutic strategies may involve a complex network of mechanisms, such as various types of cells, chemokines, and molecular mechanisms. We speculate that the main reason for TLS formation may be that the immune suppression in the TME is relieved after multiple therapeutic strategies, and the function of many immune cells can be restored. These cells interact with each other to activate LTβR signaling and other pathways and induce the production of various chemokines and cytokines, which can ultimately lead to the formation of TLSs. TLSs formation further enhances antitumor immunity, which may explain why the existence of TLSs is related to a more favorable prognosis after therapy.
Conclusion and Future Perspectives
In summary, current research has revealed the significance of TLSs in tumor immunotherapy. TLSs may constitute a privileged niche for educating T cells and B cells, which can activate and enhance immune responses. Although the major cellular and molecular mechanisms of TLSs have been elucidated, how to utilize them as an important part of the immune-related cancer control strategy is still being developed. Targeting the molecular pathways of TLSs development to induce formation is a promising immunotherapeutic strategy, which may directly enhance the antitumor response in situ. HEV induction therapy deserves more research in the design of new immunotherapies, and a more in-depth understanding of the mechanisms in terms of the types of cytokines and chemokines leading to the formation of HEVs in different types of cancer is needed. In the future, we need to focus on the combination of methods inducing HEV and TLSs formation with new therapeutic strategies that can alleviate immunosuppression, such as chemotherapies, radiotherapies, and ICB therapies. These strategies may promote the formation of TLSs as well to synergistically enhance adaptive immunity and provide insight into ultimately effective immune-mediated tumor control.
Author Contributions
Conceptualization: WK and ZH. Writing original draft: WK. Supervision: ZF and PR. All authors contributed to the article and approved the submitted version.
Funding
This work was supported by funding from the National Natural Science Foundation of China [grant numbers: 82071986, 81771827] and the Province Natural Science Foundation of Hunan [grant numbers: 2020JJ4855, 2020JJ4841].
Conflict of Interest
The authors declare that the research was conducted in the absence of any commercial or financial relationships that could be construed as a potential conflict of interest.
Publisher’s Note
All claims expressed in this article are solely those of the authors and do not necessarily represent those of their affiliated organizations, or those of the publisher, the editors and the reviewers. Any product that may be evaluated in this article, or claim that may be made by its manufacturer, is not guaranteed or endorsed by the publisher.
Abbreviations
TLSs, tertiary lymphoid structures; TILs, tumor-infiltrating lymphocytes; TME, tumor microenvironment; PD-1, programmed cell death protein-1; PD-L1, programmed death-ligand 1; SLOs, secondary lymphoid organs; GCs, germinal centers; HEVs, high endothelial venules; LN, lymph node; DCs, dendritic cells; Teff, effector T cell; FDCs, follicular DCs; PCs, plasma cells; PNAd, peripheral node addressing; TFH cells, T follicular helper cells; Treg cells, regulatory T cells; LTi, lymphoid tissue inducer; LT, lymphotoxin; LTβR, lymphotoxin-β receptor; IL, interleukin; ICB, immune checkpoint blockade; APCs, antigen-presenting cells; TGF-β, transforming growth factor-β; TNFR, tumor necrosis factor receptor; TNF-α, tumor necrosis factor-α; ACT, adoptive cell transfer; VEGF, vascular endothelial growth factor; VTP, vascular targeting peptide; TLRs, Toll-like receptors; IFN-γ, interferon-gamma; ILC3s, group 3 innate lymphoid cells; CTL, cytotoxic T lymphocyte; PLG, poly (lactide-coglycolide); TRAIL, TNF-related apoptosis-inducing ligand; MDSCs, myeloid-derived suppressor cells; GM-CSF, granulocyte-macrophage colony-stimulating factor; ICAM-1, intercellular cell adhesion molecule 1; VCAM1, vascular cell adhesion molecule 1; MADCAM1, mucosal addressable cell adhesion molecule 1.
References
1. Sautès-Fridman C, Cherfils-Vicini J, Damotte D, Fisson S, Fridman WH, Cremer I, et al. Tumor Microenvironment is Multifaceted. Cancer Metastasis Rev (2011) 30(1):13–25. doi: 10.1007/s10555-011-9279-y
2. Maman S. Witz IP. A History of Exploring Cancer in Context. Nat Rev Cancer (2018) 18(6):359–76. doi: 10.1038/s41568-018-0006-7
3. Paijens ST, Vledder A, de Bruyn M, Nijman HW. Tumor-Infiltrating Lymphocytes in the Immunotherapy Era. Cell Mol Immunol (2020) 18(4):842–59. doi: 10.1038/s41423-020-00565-9
4. Schumacher TN, Schreiber RD. Neoantigens in Cancer Immunotherapy. Sci (New York NY) (2015) 348(6230):69–74. doi: 10.1126/science.aaa4971
5. Topalian SL, Weiner GJ, Pardoll DM. Cancer Immunotherapy Comes of Age. J Clin Oncol (2011) 29(36):4828–36. doi: 10.1200/JCO.2011.38.0899
6. Wei SC, Levine JH, Cogdill AP, Zhao Y, Anang N-AAS, Andrews MC, et al. Distinct Cellular Mechanisms Underlie Anti-CTLA-4 and Anti-PD-1 Checkpoint Blockade. Cell (2017) 170(6):1120–33.e17. doi: 10.1016/j.cell.2017.07.024
7. Christofi T, Baritaki S, Falzone L, Libra M, Zaravinos A. Current Perspectives in Cancer Immunotherapy. Cancers (2019) 11(10):1472. doi: 10.3390/cancers11101472
8. Feng Z, Rong P, Wang W. Meta-Analysis of the Efficacy and Safety of PD-1/PD-L1 Inhibitors Administered Alone or in Combination With Anti-VEGF Agents in Advanced Hepatocellular Carcinoma. Gut (2020) 69(10):1904–6. doi: 10.1136/gutjnl-2019-320116
9. Devalaraja S, To TKJ, Folkert IW, Natesan R, Alam MZ, Li M, et al. Tumor-Derived Retinoic Acid Regulates Intratumoral Monocyte Differentiation to Promote Immune Suppression. Cell (2020) 180(6):1098–114.e16. doi: 10.1016/j.cell.2020.02.042
10. Thommen DS. Schumacher TN. T Cell Dysfunction in Cancer. Cancer Cell (2018) 33(4):547–62. doi: 10.1016/j.ccell.2018.03.012
11. Togashi Y, Shitara K, Nishikawa H. Regulatory T Cells in Cancer Immunosuppression - Implications for Anticancer Therapy. Nat Rev Clin Oncol (2019) 16(6):356–71. doi: 10.1038/s41571-019-0175-7
12. Sautès-Fridman C, Petitprez F, Calderaro J, Fridman WH. Tertiary Lymphoid Structures in the Era of Cancer Immunotherapy. Nat Rev Cancer (2019) 19(6):307–25. doi: 10.1038/s41568-019-0144-6
13. Di Caro G, Castino GF, Bergomas F, Cortese N, Chiriva-Internati M, Grizzi F, et al. Tertiary Lymphoid Tissue in the Tumor Microenvironment: From its Occurrence to Immunotherapeutic Implications. Int Rev Immunol (2015) 34(2):123–33. doi: 10.3109/08830185.2015.1018416
14. Rodriguez AB, Engelhard VH. Insights Into Tumor-Associated Tertiary Lymphoid Structures: Novel Targets for Antitumor Immunity and Cancer Immunotherapy. Cancer Immunol Res (2020) 8(11):1338–45. doi: 10.1158/2326-6066.CIR-20-0432
15. Pimenta EM, Barnes BJ. Role of Tertiary Lymphoid Structures (TLS) in Anti-Tumor Immunity: Potential Tumor-Induced Cytokines/Chemokines That Regulate TLS Formation in Epithelial-Derived Cancers. Cancers (2014) 6(2):969–97. doi: 10.3390/cancers6020969
16. Munoz-Erazo L, Rhodes JL, Marion VC, Kemp RA. Tertiary Lymphoid Structures in Cancer - Considerations for Patient Prognosis. Cell Mol Immunol (2020) 17(6):570–5. doi: 10.1038/s41423-020-0457-0
17. Hiraoka N, Ino Y, Yamazaki-Itoh R. Tertiary Lymphoid Organs in Cancer Tissues. Front Immunol (2016) 7:244. doi: 10.3389/fimmu.2016.00244
18. Li K, Guo Q, Zhang X, Dong X, Liu W, Zhang A, et al. Oral Cancer-Associated Tertiary Lymphoid Structures: Gene Expression Profile and Prognostic Value. Clin Exp Immunol (2020) 199(2):172–81. doi: 10.1111/cei.13389
19. Lee M, Heo S-H, Song IH, Rajayi H, Park HS, Park IA, et al. Presence of Tertiary Lymphoid Structures Determines the Level of Tumor-Infiltrating Lymphocytes in Primary Breast Cancer and Metastasis. Mod Pathol (2019) 32(1):70–80. doi: 10.1038/s41379-018-0113-8
20. Hiraoka N, Ino Y, Yamazaki-Itoh R, Kanai Y, Kosuge T, Shimada K. Intratumoral Tertiary Lymphoid Organ Is a Favourable Prognosticator in Patients With Pancreatic Cancer. Br J Cancer (2015) 112(11):1782–90. doi: 10.1038/bjc.2015.145
21. Li H, Wang J, Liu H, Lan T, Xu L, Wang G, et al. Existence of Intratumoral Tertiary Lymphoid Structures Is Associated With Immune Cells Infiltration and Predicts Better Prognosis in Early-Stage Hepatocellular Carcinoma. Aging (Albany NY) (2020) 12(4):3451–72. doi: 10.18632/aging.102821
22. Li Q, Liu X, Wang D, Wang Y, Lu H, Wen S, et al. Prognostic Value of Tertiary Lymphoid Structure and Tumour Infiltrating Lymphocytes in Oral Squamous Cell Carcinoma. Int J Oral Sci (2020) 12(1):24. doi: 10.1038/s41368-020-00092-3
23. Posch F, Silina K, Leibl S, Mündlein A, Moch H, Siebenhüner A, et al. Maturation of Tertiary Lymphoid Structures and Recurrence of Stage II and III Colorectal Cancer. Oncoimmunology (2018) 7(2):e1378844. doi: 10.1080/2162402X.2017.1378844
24. Siliņa K, Soltermann A, Attar FM, Casanova R, Uckeley ZM, Thut H, et al. Germinal Centers Determine the Prognostic Relevance of Tertiary Lymphoid Structures and Are Impaired by Corticosteroids in Lung Squamous Cell Carcinoma. Cancer Res (2018) 78(5):1308–20. doi: 10.1158/0008-5472.CAN-17-1987
25. Finkin S, Yuan D, Stein I, Taniguchi K, Weber A, Unger K, et al. Ectopic Lymphoid Structures Function as Microniches for Tumor Progenitor Cells in Hepatocellular Carcinoma. Nat Immunol (2015) 16(12):1235–44. doi: 10.1038/ni.3290
26. Bento DC, Jones E, Junaid S, Tull J, Williams GT, Godkin A, et al. High Endothelial Venules are Rare in Colorectal Cancers But Accumulate in Extra-Tumoral Areas With Disease Progression. Oncoimmunology (2015) 4(3):e974374. doi: 10.4161/2162402X.2014.974374
27. Figenschau SL, Fismen S, Fenton KA, Fenton C, Mortensen ES. Tertiary Lymphoid Structures Are Associated With Higher Tumor Grade in Primary Operable Breast Cancer Patients. BMC Cancer (2015) 15:101. doi: 10.1186/s12885-015-1116-1
28. Willard-Mack CL. Normal Structure, Function, and Histology of Lymph Nodes. Toxicol Pathol (2006) 34(5):409–24. doi: 10.1080/01926230600867727
29. Rosenberg SA. Cancer Immunotherapy Comes of Age. Nat Clin Pract Oncol (2005) 2(3):115. doi: 10.1038/ncponc0101
30. Menares E, Gálvez-Cancino F, Cáceres-Morgado P, Ghorani E, López E, Díaz X, et al. Tissue-Resident Memory CD8 T Cells Amplify Anti-Tumor Immunity by Triggering Antigen Spreading Through Dendritic Cells. Nat Commun (2019) 10(1):4401. doi: 10.1038/s41467-019-12319-x
31. Garris CS, Arlauckas SP, Kohler RH, Trefny MP, Garren S, Piot C, et al. Successful Anti-PD-1 Cancer Immunotherapy Requires T Cell-Dendritic Cell Crosstalk Involving the Cytokines IFN-γ and IL-12. Immunity (2018) 49(6):1148–61.e7. doi: 10.1016/j.immuni.2018.09.024
32. Drayton DL, Liao S, Mounzer RH, Ruddle NH. Lymphoid Organ Development: From Ontogeny to Neogenesis. Nat Immunol (2006) 7(4):344–53. doi: 10.1038/ni1330
33. Jones GW, Hill DG, Jones SA. Understanding Immune Cells in Tertiary Lymphoid Organ Development: It Is All Starting to Come Together. Front Immunol (2016) 7:401. doi: 10.3389/fimmu.2016.00401
34. Furtado GC, Pacer ME, Bongers G, Bénézech C, He Z, Chen L, et al. Tnfα-Dependent Development of Lymphoid Tissue in the Absence of Rorγt⁺ Lymphoid Tissue Inducer Cells. Mucosal Immunol (2014) 7(3):602–14. doi: 10.1038/mi.2013.79
35. Luo S, Zhu R, Yu T, Fan H, Hu Y, Mohanta SK, et al. Chronic Inflammation: A Common Promoter in Tertiary Lymphoid Organ Neogenesis. Front Immunol (2019) 10:2938. doi: 10.3389/fimmu.2019.02938
36. GeurtsvanKessel CH, Willart MA, Bergen IM, van Rijt LS, Muskens F, Elewaut D, et al. Dendritic Cells Are Crucial for Maintenance of Tertiary Lymphoid Structures in the Lung of Influenza Virus-Infected Mice. J Exp Med (2009) 206(11):2339–49. doi: 10.1084/jem.20090410
37. Peters A, Pitcher LA, Sullivan JM, Mitsdoerffer M, Acton SE, Franz B, et al. Th17 Cells Induce Ectopic Lymphoid Follicles in Central Nervous System Tissue Inflammation. Immunity (2011) 35(6):986–96. doi: 10.1016/j.immuni.2011.10.015
38. Grogan JL, Ouyang W. A Role for Th17 Cells in the Regulation of Tertiary Lymphoid Follicles. Eur J Immunol (2012) 42(9):2255–62. doi: 10.1002/eji.201242656
39. Lochner M, Ohnmacht C, Presley L, Bruhns P, Si-Tahar M, Sawa S, et al. Microbiota-Induced Tertiary Lymphoid Tissues Aggravate Inflammatory Disease in the Absence of RORgamma T and LTi Cells. J Exp Med (2011) 208(1):125–34. doi: 10.1084/jem.20100052
40. Guedj K, Khallou-Laschet J, Clement M, Morvan M, Gaston A-T, Fornasa G, et al. M1 Macrophages Act as Ltβr-Independent Lymphoid Tissue Inducer Cells During Atherosclerosis-Related Lymphoid Neogenesis. Cardiovasc Res (2014) 101(3):434–43. doi: 10.1093/cvr/cvt263
41. Jones GW, Jones SA. Ectopic Lymphoid Follicles: Inducible Centres for Generating Antigen-Specific Immune Responses Within Tissues. Immunology (2016) 147(2):141–51. doi: 10.1111/imm.12554
42. Shikhagaie MM, Björklund ÅK, Mjösberg J, Erjefält JS, Cornelissen AS, Ros XR, et al. Neuropilin-1 Is Expressed on Lymphoid Tissue Residing LTi-Like Group 3 Innate Lymphoid Cells and Associated With Ectopic Lymphoid Aggregates. Cell Rep (2017) 18(7):1761–73. doi: 10.1016/j.celrep.2017.01.063
43. Carrega P, Loiacono F, Di Carlo E, Scaramuccia A, Mora M, Conte R, et al. NCR(+)ILC3 Concentrate in Human Lung Cancer and Associate With Intratumoral Lymphoid Structures. Nat Commun (2015) 6:8280. doi: 10.1038/ncomms9280
44. Tang H, Zhu M, Qiao J, Fu Y-X. Lymphotoxin Signalling in Tertiary Lymphoid Structures and Immunotherapy. Cell Mol Immunol (2017) 14(10):809–18. doi: 10.1038/cmi.2017.13
45. Wolf MJ, Seleznik GM, Zeller N, Heikenwalder M. The Unexpected Role of Lymphotoxin Beta Receptor Signaling in Carcinogenesis: From Lymphoid Tissue Formation to Liver and Prostate Cancer Development. Oncogene (2010) 29(36):5006–18. doi: 10.1038/onc.2010.260
46. Colbeck EJ, Ager A, Gallimore A, Jones GW. Tertiary Lymphoid Structures in Cancer: Drivers of Antitumor Immunity, Immunosuppression, or Bystander Sentinels in Disease? Front Immunol (2017) 8:1830. doi: 10.3389/fimmu.2017.01830
47. Nerviani A, Pitzalis C. Role of Chemokines in Ectopic Lymphoid Structures Formation in Autoimmunity and Cancer. J Leukoc Biol (2018) 104(2):333–41. doi: 10.1002/JLB.3MR0218-062R
48. Tokunaga R, Naseem M, Lo JH, Battaglin F, Soni S, Puccini A, et al. B Cell and B Cell-Related Pathways for Novel Cancer Treatments. Cancer Treat Rev (2019) 73:10–9. doi: 10.1016/j.ctrv.2018.12.001
49. Schulz O, Hammerschmidt SI, Moschovakis GL, Förster R. Chemokines and Chemokine Receptors in Lymphoid Tissue Dynamics. Annu Rev Immunol (2016) 34:203–42. doi: 10.1146/annurev-immunol-041015-055649
50. Teillaud J-L, Dieu-Nosjean M-C. Tertiary Lymphoid Structures: An Anti-Tumor School for Adaptive Immune Cells and an Antibody Factory to Fight Cancer? Front Immunol (2017) 8:830. doi: 10.3389/fimmu.2017.00830
51. Germain C, Gnjatic S, Tamzalit F, Knockaert S, Remark R, Goc J, et al. Presence of B Cells in Tertiary Lymphoid Structures is Associated With a Protective Immunity in Patients With Lung Cancer. Am J Respir Crit Care Med (2014) 189(7):832–44. doi: 10.1164/rccm.201309-1611OC
52. Stromnes IM, Hulbert A, Pierce RH, Greenberg PD. Hingorani SR. T-Cell Localization, Activation, and Clonal Expansion in Human Pancreatic Ductal Adenocarcinoma. Cancer Immunol Res (2017) 5(11):978–91. doi: 10.1158/2326-6066.CIR-16-0322
53. Montfort A, Pearce O, Maniati E, Vincent BG, Bixby L, Böhm S, et al. A Strong B-Cell Response Is Part of the Immune Landscape in Human High-Grade Serous Ovarian Metastases. Clin Cancer Res (2017) 23(1):250–62. doi: 10.1158/1078-0432.CCR-16-0081
54. Joshi NS, Akama-Garren EH, Lu Y, Lee D-Y, Chang GP, Li A, et al. Regulatory T Cells in Tumor-Associated Tertiary Lymphoid Structures Suppress Anti-Tumor T Cell Responses. Immunity (2015) 43(3):579–90. doi: 10.1016/j.immuni.2015.08.006
55. Selitsky SR, Mose LE, Smith CC, Chai S, Hoadley KA, Dittmer DP, et al. Prognostic Value of B Cells in Cutaneous Melanoma. Genome Med (2019) 11(1):36. doi: 10.1186/s13073-019-0647-5
56. Seow DYB, Yeong JPS, Lim JX, Chia N, Lim JCT, Ong CCH, et al. Tertiary Lymphoid Structures and Associated Plasma Cells Play an Important Role in the Biology of Triple-Negative Breast Cancers. Breast Cancer Res Treat (2020) 180(2):369–77. doi: 10.1007/s10549-020-05548-y
57. Lechner A, Schlößer HA, Thelen M, Wennhold K, Rothschild SI, Gilles R, et al. Tumor-Associated B Cells and Humoral Immune Response in Head and Neck Squamous Cell Carcinoma. Oncoimmunology (2019) 8(3):1535293. doi: 10.1080/2162402X.2018.1535293
58. Kroeger DR, Milne K, Nelson BH. Tumor-Infiltrating Plasma Cells Are Associated With Tertiary Lymphoid Structures, Cytolytic T-Cell Responses, and Superior Prognosis in Ovarian Cancer. Clin Cancer Res (2016) 22(12):3005–15. doi: 10.1158/1078-0432.CCR-15-2762
59. Schlößer HA, Thelen M, Lechner A, Wennhold K, Garcia-Marquez MA, Rothschild SI, et al. B Cells in Esophago-Gastric Adenocarcinoma Are Highly Differentiated, Organize in Tertiary Lymphoid Structures and Produce Tumor-Specific Antibodies. Oncoimmunology (2019) 8(1):e1512458. doi: 10.1080/2162402X.2018.1512458
60. Helmink BA, Reddy SM, Gao J, Zhang S, Basar R, Thakur R, et al. B Cells and Tertiary Lymphoid Structures Promote Immunotherapy Response. Nature (2020) 577(7791):549–55. doi: 10.1038/s41586-019-1922-8
61. Petitprez F, de Reyniès A, Keung EZ, Chen TW-W, Sun C-M, Calderaro J, et al. B Cells Are Associated With Survival and Immunotherapy Response in Sarcoma. Nature (2020) 577(7791):556–60. doi: 10.1038/s41586-019-1906-8
62. Cabrita R, Lauss M, Sanna A, Donia M, Skaarup Larsen M, Mitra S, et al. Tertiary Lymphoid Structures Improve Immunotherapy and Survival in Melanoma. Nature (2020) 577(7791):561–5. doi: 10.1038/s41586-019-1914-8
63. Zhang W-H, Wang W-Q, Han X, Gao H-L, Xu S-S, Li S, et al. Infiltrating Pattern and Prognostic Value of Tertiary Lymphoid Structures in Resected Non-Functional Pancreatic Neuroendocrine Tumors. J Immunother Cancer (2020) 8(2):e001188. doi: 10.1136/jitc-2020-001188
64. Poschke I, Faryna M, Bergmann F, Flossdorf M, Lauenstein C, Hermes J, et al. Identification of a Tumor-Reactive T-Cell Repertoire in the Immune Infiltrate of Patients With Resectable Pancreatic Ductal Adenocarcinoma. Oncoimmunology (2016) 5(12):e1240859. doi: 10.1080/2162402X.2016.1240859
65. Dieu-Nosjean M-C, Giraldo NA, Kaplon H, Germain C, Fridman WH, Sautès-Fridman C. Tertiary Lymphoid Structures, Drivers of the Anti-Tumor Responses in Human Cancers. Immunol Rev (2016) 271(1):260–75. doi: 10.1111/imr.12405
66. Zhu G, Nemoto S, Mailloux AW, Perez-Villarroel P, Nakagawa R, Falahat R, et al. Induction of Tertiary Lymphoid Structures With Antitumor Function by a Lymph Node-Derived Stromal Cell Line. Front Immunol (2018) 9:1609. doi: 10.3389/fimmu.2018.01609
67. Goc J, Germain C, Vo-Bourgais TKD, Lupo A, Klein C, Knockaert S, et al. Dendritic Cells in Tumor-Associated Tertiary Lymphoid Structures Signal a Th1 Cytotoxic Immune Contexture and License the Positive Prognostic Value of Infiltrating CD8+ T Cells. Cancer Res (2014) 74(3):705–15. doi: 10.1158/0008-5472.CAN-13-1342
68. Truxova I, Kasikova L, Hensler M, Skapa P, Laco J, Pecen L, et al. Mature Dendritic Cells Correlate With Favorable Immune Infiltrate and Improved Prognosis in Ovarian Carcinoma Patients. J Immunother Cancer (2018) 6(1):139. doi: 10.1186/s40425-018-0446-3
69. Gu-Trantien C, Migliori E, Buisseret L, de Wind A, Brohée S, Garaud S, et al. CXCL13-Producing TFH Cells Link Immune Suppression and Adaptive Memory in Human Breast Cancer. JCI Insight (2017) 2(11):e91487. doi: 10.1172/jci.insight.91487
70. Stranford S, Ruddle NH. Follicular Dendritic Cells, Conduits, Lymphatic Vessels, and High Endothelial Venules in Tertiary Lymphoid Organs: Parallels With Lymph Node Stroma. Front Immunol (2012) 3:350. doi: 10.3389/fimmu.2012.00350
71. Le Pottier L, Devauchelle V, Fautrel A, Daridon C, Saraux A, Youinou P, et al. Ectopic Germinal Centers Are Rare in Sjogren’s Syndrome Salivary Glands and Do Not Exclude Autoreactive B Cells. J Immunol (2009) 182(6):3540–7. doi: 10.4049/jimmunol.0803588
72. Wirsing AM, Ervik IK, Seppola M, Uhlin-Hansen L, Steigen SE, Hadler-Olsen E. Presence of High-Endothelial Venules Correlates With a Favorable Immune Microenvironment in Oral Squamous Cell Carcinoma. Mod Pathol (2018) 31(6):910–22. doi: 10.1038/s41379-018-0019-5
73. Martinet L, Garrido I, Filleron T, Le Guellec S, Bellard E, Fournie J-J, et al. Human Solid Tumors Contain High Endothelial Venules: Association With T- and B-Lymphocyte Infiltration and Favorable Prognosis in Breast Cancer. Cancer Res (2011) 71(17):5678–87. doi: 10.1158/0008-5472.CAN-11-0431
74. Daum S, Hagen H, Naismith E, Wolf D, Pircher A. The Role of Anti-Angiogenesis in the Treatment Landscape of Non-Small Cell Lung Cancer - New Combinational Approaches and Strategies of Neovessel Inhibition. Front Cell Dev Biol (2020) 8:610903. doi: 10.3389/fcell.2020.610903
75. Ruddle NH. High Endothelial Venules and Lymphatic Vessels in Tertiary Lymphoid Organs: Characteristics, Functions, and Regulation. Front Immunol (2016) 7:491. doi: 10.3389/fimmu.2016.00491
76. Ager A. High Endothelial Venules and Other Blood Vessels: Critical Regulators of Lymphoid Organ Development and Function. Front Immunol (2017) 8:45. doi: 10.3389/fimmu.2017.00045
77. Buckley CD, Barone F, Nayar S, Bénézech C, Caamaño J. Stromal Cells in Chronic Inflammation and Tertiary Lymphoid Organ Formation. Annu Rev Immunol (2015) 33:715–45. doi: 10.1146/annurev-immunol-032713-120252
78. Weinstein AM, Storkus WJ. Biosynthesis and Functional Significance of Peripheral Node Addressin in Cancer-Associated TLO. Front Immunol (2016) 7:301. doi: 10.3389/fimmu.2016.00301
79. Drayton DL, Ying X, Lee J, Lesslauer W, Ruddle NH. Ectopic LT Alpha Beta Directs Lymphoid Organ Neogenesis With Concomitant Expression of Peripheral Node Addressin and a HEV-Restricted Sulfotransferase. J Exp Med (2003) 197(9):1153–63. doi: 10.1084/jem.20021761
80. Veerman K, Tardiveau C, Martins F, Coudert J, Girard J-P. Single-Cell Analysis Reveals Heterogeneity of High Endothelial Venules and Different Regulation of Genes Controlling Lymphocyte Entry to Lymph Nodes. Cell Rep (2019) 26(11):3116–31.e5. doi: 10.1016/j.celrep.2019.02.042
81. Colbeck EJ, Jones E, Hindley JP, Smart K, Schulz R, Browne M, et al. Treg Depletion Licenses T Cell-Driven HEV Neogenesis and Promotes Tumor Destruction. Cancer Immunol Res (2017) 5(11):1005–15. doi: 10.1158/2326-6066.CIR-17-0131
82. Allen E, Jabouille A, Rivera LB, Lodewijckx I, Missiaen R, Steri V, et al. Combined Antiangiogenic and Anti-PD-L1 Therapy Stimulates Tumor Immunity Through HEV Formation. Sci Transl Med (2017) 9(385):eaak9679. doi: 10.1126/scitranslmed.aak9679
83. Johansson-Percival A, He B, Li Z-J, Kjellén A, Russell K, Li J, et al. De Novo Induction of Intratumoral Lymphoid Structures and Vessel Normalization Enhances Immunotherapy in Resistant Tumors. Nat Immunol (2017) 18(11):1207–17. doi: 10.1038/ni.3836
84. Peske JD, Thompson ED, Gemta L, Baylis RA, Fu Y-X, Engelhard VH. Effector Lymphocyte-Induced Lymph Node-Like Vasculature Enables Naive T-Cell Entry Into Tumours and Enhanced Anti-Tumour Immunity. Nat Commun (2015) 6:7114. doi: 10.1038/ncomms8114
85. Remark R, Alifano M, Cremer I, Lupo A, Dieu-Nosjean M-C, Riquet M, et al. Characteristics and Clinical Impacts of the Immune Environments in Colorectal and Renal Cell Carcinoma Lung Metastases: Influence of Tumor Origin. Clin Cancer Res (2013) 19(15):4079–91. doi: 10.1158/1078-0432.CCR-12-3847
86. Gobert M, Treilleux I, Bendriss-Vermare N, Bachelot T, Goddard-Leon S, Arfi V, et al. Regulatory T Cells Recruited Through CCL22/CCR4 Are Selectively Activated in Lymphoid Infiltrates Surrounding Primary Breast Tumors and Lead to an Adverse Clinical Outcome. Cancer Res (2009) 69(5):2000–9. doi: 10.1158/0008-5472.CAN-08-2360
87. Schweiger T, Berghoff AS, Glogner C, Glueck O, Rajky O, Traxler D, et al. Tumor-Infiltrating Lymphocyte Subsets and Tertiary Lymphoid Structures in Pulmonary Metastases From Colorectal Cancer. Clin Exp Metastasis (2016) 33(7):727–39. doi: 10.1007/s10585-016-9813-y
88. García-Hernández M, Uribe-Uribe NO, Espinosa-González R, Kast WM, Khader SA, Rangel-Moreno J. A Unique Cellular and Molecular Microenvironment Is Present in Tertiary Lymphoid Organs of Patients With Spontaneous Prostate Cancer Regression. Front Immunol (2017) 8:563. doi: 10.3389/fimmu.2017.00563
89. Shields JD, Kourtis IC, Tomei AA, Roberts JM, Swartz MA. Induction of Lymphoidlike Stroma and Immune Escape by Tumors That Express the Chemokine CCL21. Sci (New York NY) (2010) 328(5979):749–52. doi: 10.1126/science.1185837
90. Najibi AJ, Mooney DJ. Cell and Tissue Engineering in Lymph Nodes for Cancer Immunotherapy. Adv Drug Deliv Rev (2020) 161–162:42–62. doi: 10.1016/j.addr.2020.07.023
91. Yagawa Y, Robertson-Tessi M, Zhou SL, Anderson ARA, Mulé JJ, Mailloux AW. Systematic Screening of Chemokines to Identify Candidates to Model and Create Ectopic Lymph Node Structures for Cancer Immunotherapy. Sci Rep (2017) 7(1):15996. doi: 10.1038/s41598-017-15924-2
92. Zhu G, Falahat R, Wang K, Mailloux A, Artzi N, Mulé JJ. Tumor-Associated Tertiary Lymphoid Structures: Gene-Expression Profiling and Their Bioengineering. Front Immunol (2017) 8:767. doi: 10.3389/fimmu.2017.00767
93. Yagawa Y, Robertson-Tessi M, Zhou SL, Anderson ARA, Mulé JJ, Mailloux AW. Systematic Screening of Chemokines to Identify Candidates to Model and Create Ectopic Lymph Node Structures for Cancer Immunotherapy. Sci Rep (2017) 7(1):15996. doi: 10.1038/s41598-017-15924-2
94. Coppola D, Nebozhyn M, Khalil F, Dai H, Yeatman T, Loboda A, et al. Unique Ectopic Lymph Node-Like Structures Present in Human Primary Colorectal Carcinoma are Identified by Immune Gene Array Profiling. Am J Pathol (2011) 179(1):37–45. doi: 10.1016/j.ajpath.2011.03.007
95. Prabhakaran S, Rizk VT, Ma Z, Cheng C-H, Berglund AE, Coppola D, et al. Evaluation of Invasive Breast Cancer Samples Using a 12-Chemokine Gene Expression Score: Correlation With Clinical Outcomes. Breast Cancer Res: BCR (2017) 19(1):71. doi: 10.1186/s13058-017-0864-z
96. Tokunaga R, Nakagawa S, Sakamoto Y, Nakamura K, Naseem M, Izumi D, et al. 12-Chemokine Signature, a Predictor of Tumor Recurrence in Colorectal Cancer. Int J Cancer (2020) 147(2):532–41. doi: 10.1002/ijc.32982
97. Luther SA, Lopez T, Bai W, Hanahan D, Cyster JG. BLC Expression in Pancreatic Islets Causes B Cell Recruitment and Lymphotoxin-Dependent Lymphoid Neogenesis. Immunity (2000) 12(5):471–81. doi: 10.1016/S1074-7613(00)80199-5
98. Luther SA, Bidgol A, Hargreaves DC, Schmidt A, Xu Y, Paniyadi J, et al. Differing Activities of Homeostatic Chemokines CCL19, CCL21, and CXCL12 in Lymphocyte and Dendritic Cell Recruitment and Lymphoid Neogenesis. J Immunol (2002) 169(1):424–33. doi: 10.4049/jimmunol.169.1.424
99. Turnquist HR, Lin X, Ashour AE, Hollingsworth MA, Singh RK, Talmadge JE, et al. CCL21 Induces Extensive Intratumoral Immune Cell Infiltration and Specific Anti-Tumor Cellular Immunity. Int J Oncol (2007) 30(3):631–9. doi: 10.3892/ijo.30.3.631
100. Schrama D, thor Straten P, Fischer WH, McLellan AD, Bröcker EB, Reisfeld RA, et al. Targeting of Lymphotoxin-Alpha to the Tumor Elicits an Efficient Immune Response Associated With Induction of Peripheral Lymphoid-Like Tissue. Immunity (2001) 14(2):111–21. doi: 10.1016/S1074-7613(01)00094-2
101. Martin AP, Coronel EC, G-i S, Chen S-C, Vassileva G, Canasto-Chibuque C, et al. A Novel Model for Lymphocytic Infiltration of the Thyroid Gland Generated by Transgenic Expression of the CC Chemokine CCL21. J Immunol (2004) 173(8):4791–8. doi: 10.4049/jimmunol.173.8.4791
102. Chen S-C, Vassileva G, Kinsley D, Holzmann S, Manfra D, Wiekowski MT, et al. Ectopic Expression of the Murine Chemokines CCL21a and CCL21b Induces the Formation of Lymph Node-Like Structures in Pancreas, But Not Skin, of Transgenic Mice. J Immunol (2002) 168(3):1001–8. doi: 10.4049/jimmunol.168.3.1001
103. Denton AE, Innocentin S, Carr EJ, Bradford BM, Lafouresse F, Mabbott NA, et al. Type I Interferon Induces CXCL13 to Support Ectopic Germinal Center Formation. J Exp Med (2019) 216(3):621–37. doi: 10.1084/jem.20181216
104. Nayar S, Campos J, Chung MM, Navarro-Núñez L, Chachlani M, Steinthal N, et al. Bimodal Expansion of the Lymphatic Vessels Is Regulated by the Sequential Expression of IL-7 and Lymphotoxin α1β2 in Newly Formed Tertiary Lymphoid Structures. J Immunol (2016) 197(5):1957–67. doi: 10.4049/jimmunol.1500686
105. Barone F, Nayar S, Campos J, Cloake T, Withers DR, Toellner K-M, et al. IL-22 Regulates Lymphoid Chemokine Production and Assembly of Tertiary Lymphoid Organs. Proc Natl Acad Sci USA (2015) 112(35):11024–9. doi: 10.1073/pnas.1503315112
106. Grogan JL, Ouyang W. A Role for Th17 Cells in the Regulation of Tertiary Lymphoid Follicles. Eur J Immunol (2012) 42(9):2255–62. doi: 10.1002/eji.201242656
107. Savage AK, Liang HE, Locksley RM. The Development of Steady-State Activation Hubs Between Adult LTi ILC3s and Primed Macrophages in Small Intestine. J Immunol (2017) 199(5):1912–22. doi: 10.4049/jimmunol.1700155
108. Wang J, Lo JC, Foster A, Yu P, Chen HM, Wang Y, et al. The Regulation of T Cell Homeostasis and Autoimmunity by T Cell-Derived LIGHT. J Clin Invest (2001) 108(12):1771–80. doi: 10.1172/JCI200113827
109. Rooney IA, Butrovich KD, Glass AA, Borboroglu S, Benedict CA, Whitbeck JC, et al. The Lymphotoxin-Beta Receptor is Necessary and Sufficient for LIGHT-Mediated Apoptosis of Tumor Cells. J Biol Chem (2000) 275(19):14307–15. doi: 10.1074/jbc.275.19.14307
110. Ware CF. Targeting Lymphocyte Activation Through the Lymphotoxin and LIGHT Pathways. Immunol Rev (2008) 223:186–201. doi: 10.1111/j.1600-065X.2008.00629.x
111. Skeate JG, Otsmaa ME, Prins R, Fernandez DJ, Da Silva DM, Kast WM. TNFSF14: LIGHTing the Way for Effective Cancer Immunotherapy. Front Immunol (2020) 11:922. doi: 10.3389/fimmu.2020.00922
112. Tang H, Wang Y, Chlewicki LK, Zhang Y, Guo J, Liang W, et al. Facilitating T Cell Infiltration in Tumor Microenvironment Overcomes Resistance to PD-L1 Blockade. Cancer Cell (2016) 29(3):285–96. doi: 10.1016/j.ccell.2016.02.004
113. Johansson-Percival A, He B, Ganss R. Immunomodulation of Tumor Vessels: It Takes Two to Tango. Trends Immunol (2018) 39(10):801–14. doi: 10.1016/j.it.2018.08.001
114. Treps L. EnLIGHTenment of Tumor Vessel Normalization and Immunotherapy in Glioblastoma. J Pathol (2018) 246(1):3–6. doi: 10.1002/path.5103
115. He B, Jabouille A, Steri V, Johansson-Percival A, Michael IP, Kotamraju VR, et al. Vascular Targeting of LIGHT Normalizes Blood Vessels in Primary Brain Cancer and Induces Intratumoural High Endothelial Venules. J Pathol (2018) 245(2):209–21. doi: 10.1002/path.5080
116. He B, Johansson-Percival A, Backhouse J, Li J, Lee GYF, Hamzah J, et al. Remodeling of Metastatic Vasculature Reduces Lung Colonization and Sensitizes Overt Metastases to Immunotherapy. Cell Rep (2020) 30(3):714–24.e5. doi: 10.1016/j.celrep.2019.12.013
117. Lu TT, Browning JL. Role of the Lymphotoxin/LIGHT System in the Development and Maintenance of Reticular Networks and Vasculature in Lymphoid Tissues. Front Immunol (2014) 5:47. doi: 10.3389/fimmu.2014.00047
118. Loeffler M, Le’Negrate G, Krajewska M, Reed JC. Attenuated Salmonella Engineered to Produce Human Cytokine LIGHT Inhibit Tumor Growth. Proc Natl Acad Sci USA (2007) 104(31):12879–83. doi: 10.1073/pnas.0701959104
119. O’Brien K, Breyne K, Ughetto S, Laurent LC, Breakefield XO. RNA Delivery by Extracellular Vesicles in Mammalian Cells and Its Applications. Nat Rev Mol Cell Biol (2020) 21(10):585–606. doi: 10.1038/s41580-020-0251-y
120. Kalluri R, LeBleu VS. The Biology Function and Biomedical Applications of Exosomes. Sci (New York NY) (2020) 367(6478):eaau6977. doi: 10.1126/science.aau6977
121. Ciccia F, Rizzo A, Maugeri R, Alessandro R, Croci S, Guggino G, et al. Ectopic Expression of CXCL13, BAFF, APRIL and LT-β Is Associated With Artery Tertiary Lymphoid Organs in Giant Cell Arteritis. Ann Rheum Dis (2017) 76(1):235–43. doi: 10.1136/annrheumdis-2016-209217
122. Gallotta M, Assi H, Degagné É, Kannan SK, Coffman RL, Guiducci C. Inhaled TLR9 Agonist Renders Lung Tumors Permissive to PD-1 Blockade by Promoting Optimal CD4 and CD8 T-Cell Interplay. Cancer Res (2018) 78(17):4943–56. doi: 10.1158/0008-5472.CAN-18-0729
123. Li L, Barry V, Daffis S, Niu C, Huntzicker E, French DM, et al. Anti-HBV Response to Toll-Like Receptor 7 Agonist GS-9620 Is Associated With Intrahepatic Aggregates of T Cells and B Cells. J Hepatol (2018) 68(5):912–21. doi: 10.1016/j.jhep.2017.12.008
124. Robinet M, Villeret B, Maillard S, Cron MA, Berrih-Aknin S, Le Panse R. Use of Toll-Like Receptor Agonists to Induce Ectopic Lymphoid Structures in Myasthenia Gravis Mouse Models. Front Immunol (2017) 8:1029. doi: 10.3389/fimmu.2017.01029
125. Workel HH, Lubbers JM, Arnold R, Prins TM, van der Vlies P, de Lange K, et al. A Transcriptionally Distinct CXCL13(+)CD103(+)CD8(+) T-Cell Population Is Associated With B-Cell Recruitment and Neoantigen Load in Human Cancer. Cancer Immunol Res (2019) 7(5):784–96. doi: 10.1158/2326-6066.Cir-18-0517
126. Chen L, Fabian KL, Taylor JL, Storkus WJ. Therapeutic Use of Dendritic Cells to Promote the Extranodal Priming of Anti-Tumor Immunity. Front Immunol (2013) 4:388. doi: 10.3389/fimmu.2013.00388
127. Kirk CJ, Hartigan-O’Connor D, Mulé JJ. The Dynamics of the T-Cell Antitumor Response: Chemokine-Secreting Dendritic Cells Can Prime Tumor-Reactive T Cells Extranodally. Cancer Res (2001) 61(24):8794–802.
128. Chen L, Taylor JL, Sabins NC, Lowe DB, Qu Y, You Z, et al. Extranodal Induction of Therapeutic Immunity in the Tumor Microenvironment After Intratumoral Delivery of Tbet Gene-Modified Dendritic Cells. Cancer Gene Ther (2013) 20(8):469–77. doi: 10.1038/cgt.2013.42
129. Moussion C, Girard J-P. Dendritic Cells Control Lymphocyte Entry to Lymph Nodes Through High Endothelial Venules. Nature (2011) 479(7374):542–6. doi: 10.1038/nature10540
130. Debets R, Timans JC, Homey B, Zurawski S, Sana TR, Lo S, et al. Two Novel IL-1 Family Members, IL-1 Delta and IL-1 Epsilon, Function as an Antagonist and Agonist of NF-Kappa B Activation Through the Orphan IL-1 Receptor-Related Protein 2. J Immunol (2001) 167(3):1440–6. doi: 10.4049/jimmunol.167.3.1440
131. Bridgewood C, Stacey M, Alase A, Lagos D, Graham A, Wittmann M. IL-36γ Has Proinflammatory Effects on Human Endothelial Cells. Exp Dermatol (2017) 26(5):402–8. doi: 10.1111/exd.13228
132. Bachmann M, Scheiermann P, Härdle L, Pfeilschifter J, Mühl H. IL-36γ/IL-1F9, an Innate T-Bet Target in Myeloid Cells. J Biol Chem (2012) 287(50):41684–96. doi: 10.1074/jbc.M112.385443
133. Weinstein AM, Giraldo NA, Petitprez F, Julie C, Lacroix L, Peschaud F, et al. Association of IL-36γ With Tertiary Lymphoid Structures and Inflammatory Immune Infiltrates in Human Colorectal Cancer. Cancer Immunol Immunother (2019) 68(1):109–20. doi: 10.1007/s00262-018-2259-0
134. Weinstein AM, Chen L, Brzana EA, Patil PR, Taylor JL, Fabian KL, et al. Tbet and IL-36γ Cooperate in Therapeutic DC-Mediated Promotion of Ectopic Lymphoid Organogenesis in the Tumor Microenvironment. Oncoimmunology (2017) 6(6):e1322238. doi: 10.1080/2162402X.2017.1322238
135. Lee JM, Lee MH, Garon E, Goldman JW, Salehi-Rad R, Baratelli FE, et al. Phase I Trial of Intratumoral Injection of CCL21 Gene-Modified Dendritic Cells in Lung Cancer Elicits Tumor-Specific Immune Responses and CD8(+) T-Cell Infiltration. Clin Cancer Res (2017) 23(16):4556–68. doi: 10.1158/1078-0432.Ccr-16-2821
136. Yang SC, Batra RK, Hillinger S, Reckamp KL, Strieter RM, Dubinett SM, et al. Intrapulmonary Administration of CCL21 Gene-Modified Dendritic Cells Reduces Tumor Burden in Spontaneous Murine Bronchoalveolar Cell Carcinoma. Cancer Res (2006) 66(6):3205–13. doi: 10.1158/0008-5472.Can-05-3619
137. Mac Keon S, Gazzaniga S, Mallerman J, Bravo AI, Mordoh J, Wainstok R. Vaccination With Dendritic Cells Charged With Apoptotic/Necrotic B16 Melanoma Induces the Formation of Subcutaneous Lymphoid Tissue. Vaccine (2010) 28(51):8162–8. doi: 10.1016/j.vaccine.2010.09.095
138. Cupedo T, Jansen W, Kraal G, Mebius RE. Induction of Secondary and Tertiary Lymphoid Structures in the Skin. Immunity (2004) 21(5):655–67. doi: 10.1016/j.immuni.2004.09.006
139. Suematsu S, Watanabe T. Generation of a Synthetic Lymphoid Tissue-Like Organoid in Mice. Nat Biotechnol (2004) 22(12):1539–45. doi: 10.1038/nbt1039
140. Nayar S, Campos J, Smith CG, Iannizzotto V, Gardner DH, Mourcin F, et al. Immunofibroblasts are Pivotal Drivers of Tertiary Lymphoid Structure Formation and Local Pathology. Proc Natl Acad Sci USA (2019) 116(27):13490–7. doi: 10.1073/pnas.1905301116
141. Dorraji SE, Hovd A-MK, Kanapathippillai P, Bakland G, Eilertsen GØ, Figenschau SL, et al. Mesenchymal Stem Cells and T Cells in the Formation of Tertiary Lymphoid Structures in Lupus Nephritis. Sci Rep (2018) 8(1):7861. doi: 10.1038/s41598-018-26265-z
142. Koscsó B, Kurapati S, Rodrigues RR, Nedjic J, Gowda K, Shin C, et al. Gut-Resident CX3CR1 Macrophages Induce Tertiary Lymphoid Structures and IgA Response In Situ. Sci Immunol (2020) 5(46):eaax0062. doi: 10.1126/sciimmunol.aax0062
143. Guedj K, Abitbol Y, Cazals-Hatem D, Morvan M, Maggiori L, Panis Y, et al. Adipocytes Orchestrate the Formation of Tertiary Lymphoid Organs in the Creeping Fat of Crohn’s Disease Affected Mesentery. J Autoimmun (2019) 103:102281. doi: 10.1016/j.jaut.2019.05.009
144. Malafaya PB, Silva GA, Reis RL. Natural-Origin Polymers as Carriers and Scaffolds for Biomolecules and Cell Delivery in Tissue Engineering Applications. Adv Drug Deliv Rev (2007) 59(4-5):207–33. doi: 10.1016/j.addr.2007.03.012
145. Weber JS, Mulé JJ. Cancer Immunotherapy Meets Biomaterials. Nat Biotechnol (2015) 33(1):44–5. doi: 10.1038/nbt.3119
146. Weiden J, Tel J, Figdor CG. Synthetic Immune Niches for Cancer Immunotherapy. Nat Rev Immunol (2018) 18(3):212–9. doi: 10.1038/nri.2017.89
147. Kim J, Li WA, Choi Y, Lewin SA, Verbeke CS, Dranoff G, et al. Injectable, Spontaneously Assembling, Inorganic Scaffolds Modulate Immune Cells In Vivo and Increase Vaccine Efficacy. Nat Biotechnol (2015) 33(1):64–72. doi: 10.1038/nbt.3071
148. Stephan SB, Taber AM, Jileaeva I, Pegues EP, Sentman CL, Stephan MT. Biopolymer Implants Enhance the Efficacy of Adoptive T-Cell Therapy. Nat Biotechnol (2015) 33(1):97–101. doi: 10.1038/nbt.3104
149. Okamoto N, Chihara R, Shimizu C, Nishimoto S, Watanabe T. Artificial Lymph Nodes Induce Potent Secondary Immune Responses in Naive and Immunodeficient Mice. J Clin Invest (2007) 117(4):997–1007. doi: 10.1172/JCI30379
150. Kobayashi Y, Watanabe T. Synthesis of Artificial Lymphoid Tissue With Immunological Function. Trends Immunol (2010) 31(11):422–8. doi: 10.1016/j.it.2010.09.002
151. Singh A, Peppas NA. Hydrogels and Scaffolds for Immunomodulation. Adv Mater (2014) 26(38):6530–41. doi: 10.1002/adma.201402105
152. Gilam A, Conde J, Weissglas-Volkov D, Oliva N, Friedman E, Artzi N, et al. Local microRNA Delivery Targets Palladin and Prevents Metastatic Breast Cancer. Nat Commun (2016) 7:12868. doi: 10.1038/ncomms12868
153. Conde J, Oliva N, Zhang Y, Artzi N. Local Triple-Combination Therapy Results in Tumour Regression and Prevents Recurrence in a Colon Cancer Model. Nat Mater (2016) 15(10):1128–38. doi: 10.1038/nmat4707
154. Kobayashi Y, Watanabe T. Gel-Trapped Lymphorganogenic Chemokines Trigger Artificial Tertiary Lymphoid Organs and Mount Adaptive Immune Responses In Vivo. Front Immunol (2016) 7:316. doi: 10.3389/fimmu.2016.00316
155. Singh A, Qin H, Fernandez I, Wei J, Lin J, Kwak LW, et al. An Injectable Synthetic Immune-Priming Center Mediates Efficient T-Cell Class Switching and T-Helper 1 Response Against B Cell Lymphoma. J Control Rel (2011) 155(2):184–92. doi: 10.1016/j.jconrel.2011.06.008
156. Ali OA, Huebsch N, Cao L, Dranoff G, Mooney DJ. Infection-Mimicking Materials to Program Dendritic Cells in Situ. Nat Mater (2009) 8(2):151–8. doi: 10.1038/nmat2357
157. Shimizu K, Yamasaki S, Shinga J, Sato Y, Watanabe T, Ohara O, et al. Systemic DC Activation Modulates the Tumor Microenvironment and Shapes the Long-Lived Tumor-Specific Memory Mediated by CD8+ T Cells. Cancer Res (2016) 76(13):3756–66. doi: 10.1158/0008-5472.CAN-15-3219
158. Huang Y, Chen Y, Zhou S, Chen L, Wang J, Pei Y, et al. Dual-Mechanism Based CTLs Infiltration Enhancement Initiated by Nano-Sapper Potentiates Immunotherapy Against Immune-Excluded Tumors. Nat Commun (2020) 11(1):622. doi: 10.1038/s41467-020-14425-7
159. Bahmani B, Uehara M, Ordikhani F, Li X, Jiang L, Banouni N, et al. Ectopic High Endothelial Venules in Pancreatic Ductal Adenocarcinoma: A Unique Site for Targeted Delivery. EBioMedicine (2018) 38:79–88. doi: 10.1016/j.ebiom.2018.11.030
160. Maldonado L, Teague JE, Morrow MP, Jotova I, Wu TC, Wang C, et al. Intramuscular Therapeutic Vaccination Targeting HPV16 Induces T Cell Responses That Localize in Mucosal Lesions. Sci Transl Med (2014) 6(221):221ra13. doi: 10.1126/scitranslmed.3007323
161. Lutz ER, Wu AA, Bigelow E, Sharma R, Mo G, Soares K, et al. Immunotherapy Converts Nonimmunogenic Pancreatic Tumors Into Immunogenic Foci of Immune Regulation. Cancer Immunol Res (2014) 2(7):616–31. doi: 10.1158/2326-6066.CIR-14-0027
162. Morcrette G, Hirsch TZ, Badour E, Pilet J, Caruso S, Calderaro J, et al. Germline Hepatoblastomas Demonstrate Cisplatin-Induced Intratumor Tertiary Lymphoid Structures. Oncoimmunology (2019) 8(6):e1583547. doi: 10.1080/2162402X.2019.1583547
163. Kuwabara S, Tsuchikawa T, Nakamura T, Hatanaka Y, Hatanaka KC, Sasaki K, et al. Prognostic Relevance of Tertiary Lymphoid Organs Following Neoadjuvant Chemoradiotherapy in Pancreatic Ductal Adenocarcinoma. Cancer Sci (2019) 110(6):1853–62. doi: 10.1111/cas.14023
164. Siliņa K, Soltermann A, Attar FM, Casanova R, Uckeley ZM, Thut H, et al. Germinal Centers Determine the Prognostic Relevance of Tertiary Lymphoid Structures and Are Impaired by Corticosteroids in Lung Squamous Cell Carcinoma. Cancer Res (2018) 78(5):1308–20. doi: 10.1158/0008-5472.Can-17-1987
165. Boivin G, Kalambaden P, Faget J, Rusakiewicz S, Montay-Gruel P, Meylan E, et al. Cellular Composition and Contribution of Tertiary Lymphoid Structures to Tumor Immune Infiltration and Modulation by Radiation Therapy. Front Oncol (2018) 8:256. doi: 10.3389/fonc.2018.00256
166. Chelvanambi M, Fecek RJ, Taylor JL, Storkus WJ. STING Agonist-Based Treatment Promotes Vascular Normalization and Tertiary Lymphoid Structure Formation in the Therapeutic Melanoma Microenvironment. J Immunother Cancer (2021) 9(2):e001906. doi: 10.1136/jitc-2020-001906
167. Solinas C, Garaud S, De Silva P, Boisson A, Van den Eynden G, de Wind A, et al. Immune Checkpoint Molecules on Tumor-Infiltrating Lymphocytes and Their Association With Tertiary Lymphoid Structures in Human Breast Cancer. Front Immunol (2017) 8:1412. doi: 10.3389/fimmu.2017.01412
168. Giraldo NA, Becht E, Pagès F, Skliris G, Verkarre V, Vano Y, et al. Orchestration and Prognostic Significance of Immune Checkpoints in the Microenvironment of Primary and Metastatic Renal Cell Cancer. Clin Cancer Res (2015) 21(13):3031–40. doi: 10.1158/1078-0432.CCR-14-2926
169. van Dijk N, Gil-Jimenez A, Silina K, Hendricksen K, Smit LA, de Feijter JM, et al. Preoperative Ipilimumab Plus Nivolumab in Locoregionally Advanced Urothelial Cancer: The NABUCCO Trial. Nat Med (2020) 26(12):1839–44. doi: 10.1038/s41591-020-1085-z
170. Thommen DS, Koelzer VH, Herzig P, Roller A, Trefny M, Dimeloe S, et al. A Transcriptionally and Functionally Distinct PD-1 CD8 T Cell Pool With Predictive Potential in Non-Small-Cell Lung Cancer Treated With PD-1 Blockade. Nat Med (2018) 24(7):994–1004. doi: 10.1038/s41591-018-0057-z
Keywords: tertiary lymphoid structures, tumor immunity, lymphoid neogenesis, bioengineering, immunotherapy, LIGHT
Citation: Kang W, Feng Z, Luo J, He Z, Liu J, Wu J and Rong P (2021) Tertiary Lymphoid Structures in Cancer: The Double-Edged Sword Role in Antitumor Immunity and Potential Therapeutic Induction Strategies. Front. Immunol. 12:689270. doi: 10.3389/fimmu.2021.689270
Received: 31 March 2021; Accepted: 05 July 2021;
Published: 29 July 2021.
Edited by:
Catherine Sautes-Fridman, U1138 Centre de Recherche des Cordeliers (CRC) (INSERM), FranceReviewed by:
Marie-Cecile Michallet, University of Lyon, FranceYona Keisari, Tel Aviv University, Israel
Copyright © 2021 Kang, Feng, Luo, He, Liu, Wu and Rong. This is an open-access article distributed under the terms of the Creative Commons Attribution License (CC BY). The use, distribution or reproduction in other forums is permitted, provided the original author(s) and the copyright owner(s) are credited and that the original publication in this journal is cited, in accordance with accepted academic practice. No use, distribution or reproduction is permitted which does not comply with these terms.
*Correspondence: Pengfei Rong, cm9uZ3BlbmdmZWk2NkAxNjMuY29t