- 1Department of Nephrology, University Medical Center Groningen, Groningen, Netherlands
- 2Department of Immunology, University Medical Center Groningen, Groningen, Netherlands
- 3Department of Immunopathology, Sanquin Research, Amsterdam, Netherlands
Background: Kidney transplant recipients (KTRs) have an impaired immune response after vaccination against severe acute respiratory syndrome coronavirus-2 (SARS-CoV-2). Iron deficiency (ID) may adversely affect immunity and vaccine efficacy. We aimed to investigate whether ferric carboxymaltose (FCM) treatment improves humoral and cellular responses after SARS-CoV-2 vaccination in iron-deficient KTRs.
Methods: We randomly assigned 48 iron-deficient KTRs to intravenous FCM (1-4 doses of 500mg with six-week intervals) or placebo. Co-primary endpoints were SARS-CoV-2-specific anti-Receptor Binding Domain (RBD) Immunoglobulin G (IgG) titers and T-lymphocyte reactivity against SARS-CoV-2 at four weeks after the second vaccination with mRNA-1273 or mRNA-BNT162b2.
Results: At four weeks after the second vaccination, patients receiving FCM had higher plasma ferritin and transferrin saturation (P<0.001 vs. placebo) and iron (P=0.02). However, SARS-CoV-2-specific anti-RBD IgG titers (FCM: 66.51 [12.02-517.59] BAU/mL; placebo: 115.97 [68.86-974.67] BAU/mL, P=0.07) and SARS-CoV-2-specific T-lymphocyte activation (FCM: 93.3 [0.85-342.5] IFN-ɣ spots per 106 peripheral blood mononuclear cells (PBMCs), placebo: 138.3 [0.0-391.7] IFN-ɣ spots per 106 PBMCs, P=0.83) were not significantly different among both arms. After the third vaccination, SARS-CoV-2-specific anti-RBD IgG titers remained similar between treatment groups (P=0.99).
Conclusions: Intravenous iron supplementation efficiently restored iron status but did not improve the humoral or cellular immune response against SARS-CoV-2 after three vaccinations.
Introduction
Coronavirus Disease 2019 (COVID-19) has affected more than 500 million people worldwide since the beginning of the pandemic early 2020, leading to more than six million deaths (1). Kidney transplant recipients (KTRs) who are infected with severe acute respiratory syndrome coronavirus-2 (SARS-CoV-2) have an increased risk of adverse outcome, with a 17-23% mortality rate (2–5). Immunosuppressive medication in KTRs impedes powerful humoral (6–11) and cellular (10, 12) immune responses against SARS-CoV-2 after vaccination. Previous studies identified higher age, pre-transplantation dialysis, deceased donor type, worse graft function, recent use of high-dose corticosteroids and use of mycophenolic acid as risk factors for a poor antibody response after SARS-CoV-2 vaccination in KTRs (8, 10, 13). Still, identification of new modifiable risk factors for an impaired immune response in KTRs is urgently needed (6, 8, 10).
Iron deficiency (ID) is highly prevalent after kidney transplantation (14), and has recently been proposed as potential treatment target to improve vaccine efficacy (15). Iron is involved in nucleotide synthesis for replication of deoxyribonucleic acid and in mitochondrial energy metabolism (16). Therefore, rapidly proliferating cells such as lymphocytes are prone to be affected by ID. Recent studies demonstrated impaired B-cell proliferation, plasma cell differentiation and immunoglobulin production in iron-deficient mice (17). In humans, ID is associated with reduced antibody production in response to various vaccinations (17), while pre-vaccination iron supplements improved vaccination-induced immune responses (18). Given these observations, the European Hematology Association recently published an expert opinion advising to correct ID before vaccination against SARS-CoV-2 (19). Whether correcting ID improves SARS-CoV-2 vaccine efficacy in KTRs is unknown. Therefore, we aimed to address the hypothesis that in iron-deficient KTRs, ferric carboxymaltose (FCM) improves the humoral and cellular response after vaccination against SARS-CoV-2.
Materials and methods
Patient population and study design
COVAC-EFFECT is a secondary analysis performed in a subpopulation of the ongoing EFFECT-KTx study (NCT03769441, also covering COVAC-EFFECT), a randomized, placebo-controlled, parallel-arm clinical trial aiming to address the effects of FCM versus placebo on exercise tolerance, cardiac function and other clinical outcomes in iron-deficient KTRs. In the mother trial, 158 subjects receive up to four doses of FCM (containing 500 mg Fe3+ per dose) or placebo (0.9% sodium chloride solution) intravenously, with six-week intervals. In case of severe hypophosphatemia (plasma phosphate ≤1.55 mg/dL) or active systemic infection on the day of the study visit, one treatment is withheld. In case of imminent iron overload, defined as a plasma ferritin level of ≥800 ug/L or 500-799 ug/L in combination with a transferrin saturation (TSAT) of ≥45% on the day of the study visit, patients in the FCM arm receive a dose of placebo instead of FCM. The study protocol of the EFFECT-KTx study and COVAC-EFFECT was approved by the medical ethical committee of the University Medical Center Groningen (METc 2018/482), conducted in accordance with the principles of the Declaration of Helsinki and consistent with the Good Clinical Practice guidelines provided by the International Council for Harmonization of Technical Requirements for Pharmaceuticals for Human Use. All participants had given their informed consent prior to enrollment in the EFFECT-KTx study as well as to enrollment in COVAC.
The current study could be performed since the ongoing EFFECT-KTx trial coincided with the Dutch national SARS-CoV2 vaccination program. Given this setting, a formal a priori sample size calculation was not performed; instead, all patients enrolled in the mother trial (EFFECT-KTx) who met the inclusion criteria for the current substudy were invited to participate. Participants who had received at least one treatment with study medication were eligible. The unblinded researchers who analyzed the data for the current study worked completely independent of the study team working on the mother trial, to ensure that these investigators remained blinded. An overview of the study design is provided in Figure 1. For the current study, we enrolled 48 iron-deficient EFFECT-KTx participants with a functional graft for more than six months post-transplantation who had not reported COVID-19 and who agreed to vaccination against SARS-CoV-2. Two patients who tested positive for SARS-CoV-2 within four weeks after the first or the second vaccination were excluded.
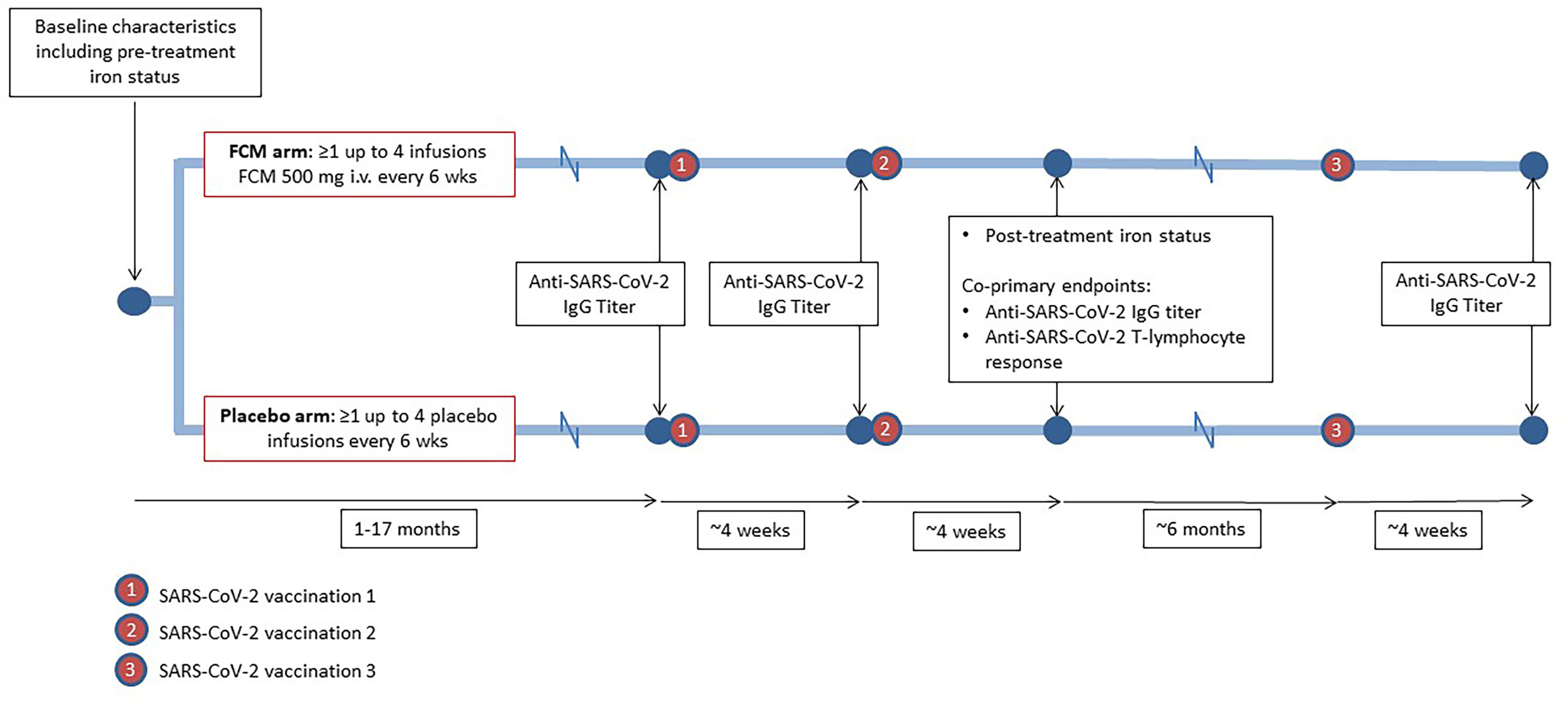
Figure 1 Study design. During participation in the EFFECT-KTx study, iron-deficient kidney transplant recipients are being randomized to receive four doses of ferric carboxymaltose (FCM) or placebo with six-week intervals after baseline measurements, including iron status assessment, have been performed. During or after participation in the EFFECT-KTx study, patients received three vaccinations against SARS-CoV-2. Before the first vaccination and four weeks after the successive vaccinations, anti-SARS-CoV-2 IgG titer was measured. Four weeks after the second vaccination, anti-SARS-CoV-2 T-lymphocyte response was assessed.
Vaccination
Participants were vaccinated against SARS-Cov-2 as part of the Dutch national vaccination campaign. Patients who were eligible for early vaccination, because of high age or occupation in healthcare, received two mRNA-BNT162b2 vaccinations (COMIRNATY, ®Pfizer-BioNTech) with an interval of 35 days. All other participants received two mRNA-1273 vaccinations (®Moderna Biotech Spain, S.L.) with an interval of 28 days according to the manufacturer’s instructions. Six months after the second vaccination dose, participants received a third vaccination dose.
Data collection and definitions
Co-primary outcomes of this study were the humoral and cellular immune responses at four weeks after the second vaccination. At this timepoint, SARS-CoV-2-specific anti-Receptor Binding Domain (RBD) immunoglobulin G (IgG) levels, expressed in international Binding Antibody Units (BAU)/mL, were measured in venous blood. Furthermore, capillary blood was collected with a self-collection finger prick set prior to the first and the second vaccination and four weeks after the third vaccination for additional SARS-CoV-2-specific anti-RBD IgG titer measurements. To assess cellular immunogenicity, peripheral blood mononuclear cells (PBMCs) were isolated from the blood sample that was taken four weeks after the second vaccination.
Baseline characteristics were registered and measurements were performed before treatment with FCM or placebo. Body Mass Index (BMI) was calculated as weight in kilograms divided by height in meters squared. Plasma creatinine was measured in venous blood with an enzymatic photometry assay (Roche Diagnostics, Mannheim, Germany). The estimated glomerular filtration rate (eGFR) was calculated using the Chronic Kidney Disease Epidemiology Collaboration (CKD‐EPI) equation, omitting the black race coefficient (20). Plasma iron was measured with a colorimetric assay, ferritin with an immunoassay and transferrin with a turbidimetric assay (Roche Diagnostics), all in venous blood samples. TSAT was calculated as 100 x (plasma iron (μg/dL) ÷ (total iron binding capacity (μg/dL)). Hemoglobin was measured in venous blood with spectrophotometry. ID was defined as a plasma ferritin concentration below 100 μg/L or a ferritin concentration between 100 and 299 μg/L in combination with a TSAT below 20% (21). Severe ID was defined as a ferritin concentration below 30 μg/L (22). IgG deficiency was defined as a total IgG concentration below 7.0 g/L (23).
Anti-SARS-CoV-2 antibody response
Serum was isolated from capillary blood samples (fingerpick sampled at home) taken before the first and the second vaccination and four weeks after the third vaccination, or from venous blood sample drawn four weeks after the second vaccination. SARS-CoV-2-specific anti-RBD IgG antibodies were measured using an in-house (Sanquin, Amsterdam, the Netherlands) enzyme-linked immunosorbent assay (ELISA) assay, as described by Steenhuis et al. (24) IgG titers were compared with the World Health Organization reference sample (NIBSC code: 20/136) and expressed as BAU/ml. Cut-off for seroconversion was defined based on the 98th percentile of signals of 240 pre‐outbreak plasma samples, corresponding to an anti-RBD IgG titer of ≥50 BAU/mL (24, 25). In addition, to detect previous exposure to SARS-CoV-2, a highly sensitive and specific total antibody SARS-CoV-2 RBD bridging assessment was performed on all samples, using a double antigen sandwich ELISA based assay developed by Sanquin, as described previously (24, 26). Outcome of this assay was compared to a Sanquin in-house calibrator of pooled convalescent plasma and expressed as normalized optical density units (nOD). An nOD >0.1 was considered as seropositive for total SARS-CoV-2- specific anti-RBD antibodies (24, 26). Furthermore, SARS-CoV-2-specific anti-nucleocapsid protein IgG antibodies were measured in samples from patients with a nOD >0.1 or an anti-RBD IgG titer of ≥50 BAU/mL at baseline, using an ELISA assay (26). Since these antibodies do not react to vaccination and are highly specific for previous exposure to the virus itself, they can be used to assess whether antibodies are induced by SARS-CoV-2 vaccination or infection.
T-lymphocyte reactivity against Spike protein
In all participants, the SARS-CoV-2-specific T-lymphocyte response was measured after stimulation of PBMCs isolated from heparinized venous blood obtained four weeks after the second vaccination, using SepMate tubes (STEMCELL). The number of Interferon-gamma (IFN-ɣ)-producing T-lymphocytes after stimulation with SARS-CoV-2 Spike overlapping peptide pools was assessed using an IFN-ɣ enzyme-linked immune adsorbent spot (ELISpot) assay. SARS-CoV-2 S1 and S2 peptide pools (JPT Peptide Technologies), consisting of 15-mer peptides overlapping 11 amino acids that cover the entire sequence of the viral proteins were used for overnight stimulation of the PBMCs in a concentration of 0.5 µg/mL. 0.4% dimethyl sulfoxide (DMSO, Sigma) was used as negative control and Phytohaemagglutinin (Remel Europe Ltd; 4 µg/mL) as a positive control. Spot forming cells (SFC) were quantified with the AID ELISpot/Fluorospot reader and calculated to SFCs/106 PBMCs. The average of the DMSO negative control was subtracted per stimulation. To define the total Spike-specific SFC, the SFC of the separate S1 and S2 peptide pools were summed. Results are expressed in number of IFN-ɣ spots per 106 PBMCs. KTRs who had more than 50 IFN-ɣ spots per 106 PBMCs were considered to be responders (27).
Statistical analyses
We used IBM SPSS Statistics version 23.0 (SPSS Inc., Chicago, USA) and Prism 8.0 (GraphPad Software Inc., San Diego, USA) to analyze the data. Normally distributed data are presented as mean ± standard deviation (SD). Data with a skewed distribution are presented as median (interquartile range [IQR]). Categorical data are expressed as number (percentage).
To assess changes between baseline and post-vaccination measurements, we used the paired T-test for normally distributed data or the Wilcoxon-signed rank test for data with a skewed distribution. To assess differences between the two study arms at four weeks after the successive vaccinations, the independent samples T-test and Mann-Whitney Test were used. To adjust for differences in baseline lymphocyte count or eGFR, analysis of covariance was performed. Correlations were analyzed using the Spearman’s rank test.
The primary analyses were performed according to the intention-to-treat principle. We additionally performed ten sensitivity analyses. First, a per-protocol analysis was performed, excluding KTRs who were still iron-deficient after treatment with FCM, as well as KTRs who were not iron-deficient despite placebo treatment. In a second sensitivity analysis, we excluded four patients who were seropositive for total SARS-CoV-2-specific anti-RBD antibodies (n=3) or anti-SARS-CoV-2 IgG antibodies (n=1) at baseline. As a third sensitivity analysis, we excluded KTRs who were IgG-deficient at baseline. Fourth, we repeated the analyses in a subpopulation restricted to patients who had received the mRNA-1273 vaccine. In a fifth sensitivity analysis, only patients with severe ID (ferritin <30 μg/L) were included. Sixth, we analyzed patients on dual or triple immunosuppressive therapy separately. Seventh, patients who had received treatment with alemtuzumab, methylprednisolone or anti-thymocyte globulin <2 years prior to vaccination were excluded. In an eighth sensitivity analysis, the subgroup of patients who used mycophenolic acid was studied. Moreover, the subgroup of patients using mycophenolic acid with a dosage of 500mg twice daily was analyzed separately. Finally, we analyzed men and women separately. In all analyses, a P value of ≤0.05 was considered significant.
Results
Baseline characteristics and vaccines
Forty-six KTRs (age 53 [43-65] years, 61% male) were included. Baseline characteristics are shown in Table 1. Patient characteristics in both treatment arms were generally well balanced, except for small but significant differences in lymphocyte count, eGFR and prevalence of hypertension. Forty-one patients received the mRNA-1273 vaccine, and five KTRs received the mRNA-BNT162b2 vaccine. Four patients had a nOD of >0.1 or an anti-RBD IgG titer of ≥50 BAU/mL at baseline; three of them also had anti-nucleocapsid protein IgG antibodies at baseline. Time between enrollment in the EFFECT-KTx study and first vaccination was 33 ± 24 weeks.
Iron status
Iron status was assessed at baseline before treatment with FCM or placebo and four weeks after the second vaccination, which was on average 42 ± 24 weeks after baseline (Table 2). Patients in the FCM arm showed an increase in plasma ferritin levels from 49 [26–79] μg/L to 464 [272–621] μg/L (P<0.001 vs baseline), while in the placebo group, ferritin did not significantly change (34 [24–62] μg/L to 42 [23–69] μg/L, P=0.39, Figure 2A). Ferritin levels at four weeks after the second vaccination were significantly higher in the FCM arm than in the placebo arm (P<0.001). TSAT also increased significantly in the FCM arm (from 21 ± 8% to 34 ± 12%, P<0.001), but not in the placebo arm (21 ± 8% vs 21 ± 10%, P=0.84 vs placebo baseline, P<0.001 vs FCM, Figure 2B). Plasma iron levels increased significantly in the FCM arm (from 75.4 ± 25.7 µg/dL to 98.8 ± 29.0 µg/dL, P=0.004), but not in the placebo arm (78.2 ± 22.9 µg/dL to 79.3 ± 34.1 µg/dL, P=0.89 vs placebo baseline, P=0.02 vs FCM, Figure 2C). Two months after the second vaccination, only two patients in the FCM arm were still iron-deficient. One patient was not iron-deficient anymore despite placebo treatment. Finally, there was a small but significant effect of FCM on hemoglobin levels (from 13.2 ± 1.1 g/dL to 14.0 ± 1.1 g/dL, P<0.001) which was not observed in the placebo arm (13.5 ± 1.0 g/dL vs 13.5 ± 1.1 g/dL, P=0.95).
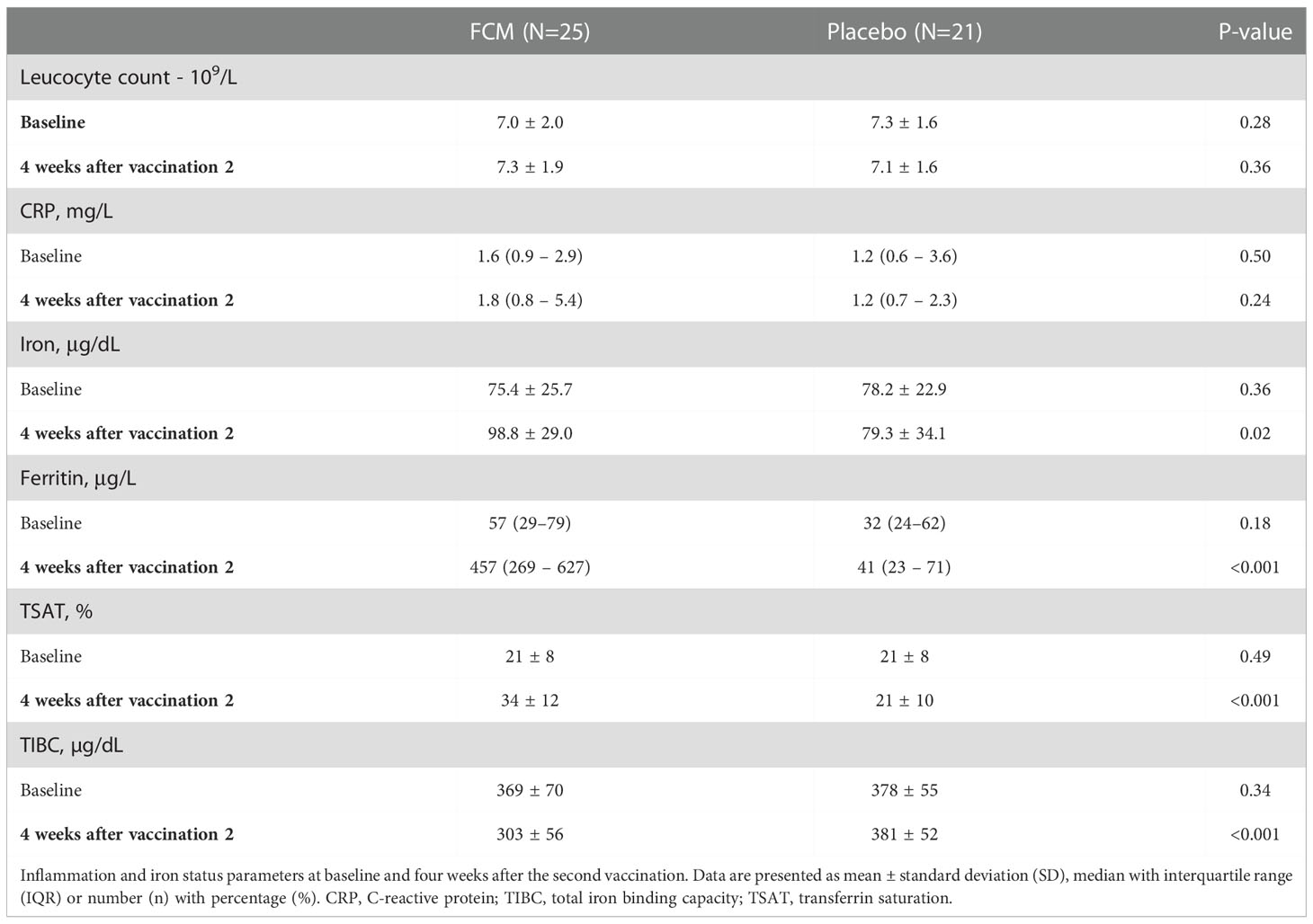
Table 2 Inflammation and iron status parameters at baseline and four weeks after the second vaccination.
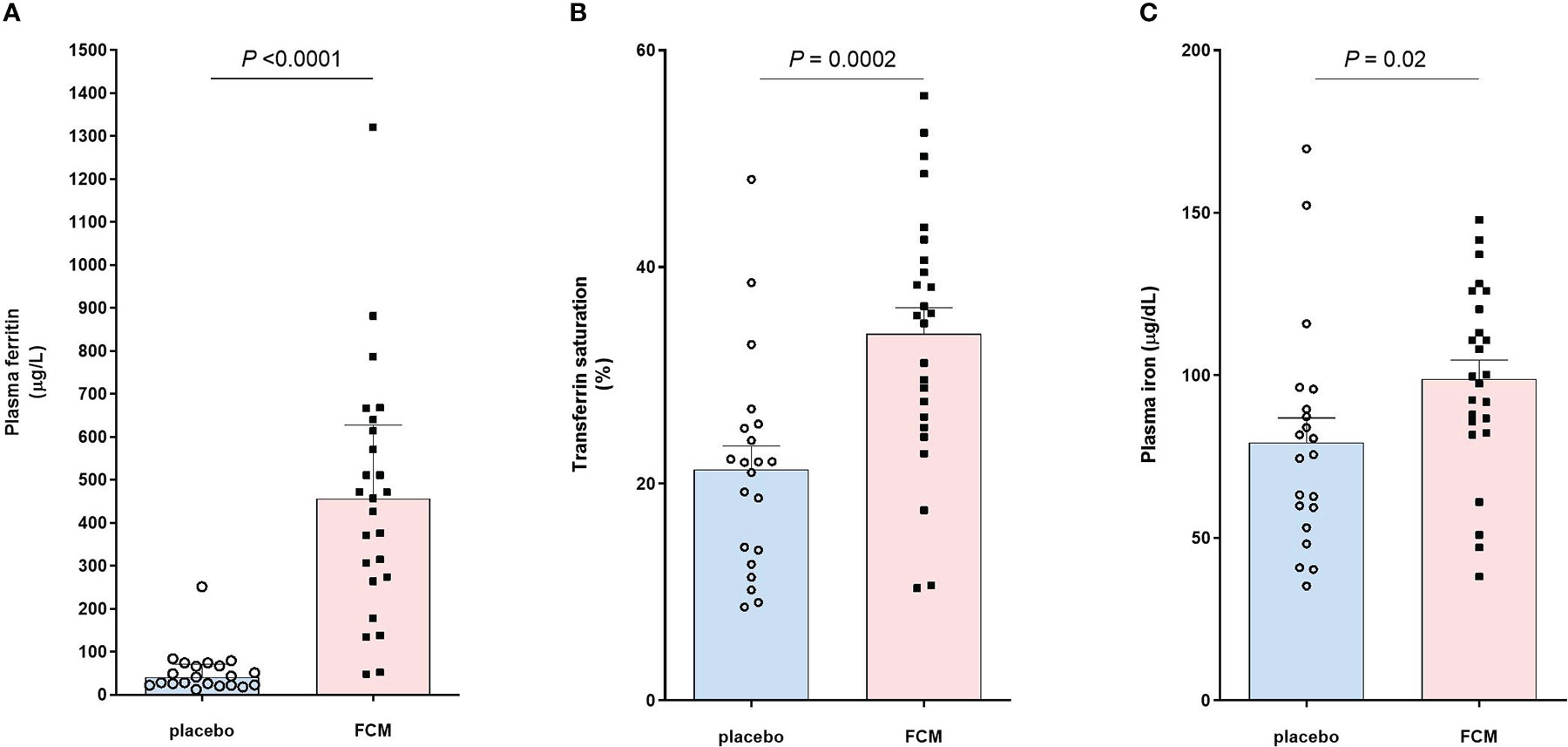
Figure 2 Effect of FCM or placebo on (A) plasma ferritin levels, (B) transferrin saturation and (C) plasma iron levels in iron-deficient KTRs.
Anti-SARS-CoV-2 antibody response
There was no significant difference between the treatment groups in SARS-CoV-2-specific anti-RBD IgG concentration at four weeks after the second vaccination dose (P=0.07), which was one of the two co-primary outcomes of this study. Also after the first (P=0.12), or the third vaccination (P=0.99) there was no difference in SARS-CoV-2-specific anti-RBD IgG concentration between the study groups (Figure 3A). During the four weeks after the first vaccination, there was no significant increase in SARS-CoV-2-specific anti-RBD IgG concentration in patients in the FCM arm (2.31 [1.18–9.53] BAU/mL to 1.18 [1.18-20.63] BAU/mL, P=0.14). At four weeks after the second vaccination, SARS-CoV-2-specific anti-RBD IgG concentration significantly increased to 66.51 [12.02–517.59] BAU/mL in the FCM group (P<0.001 vs before first vaccination). Finally, four weeks after the third vaccination, SARS-CoV-2-specific anti-RBD IgG concentration further increased to 464.71 [52.13 – 1255.30] in the FCM group (P<0.001 vs before first vaccination). In the placebo arm, SARS-CoV-2-specific anti-RBD IgG concentration increased from 1.18 [1.18–10.33] BAU/mL before the first vaccination to 13.75 [1.18–46.18] BAU/mL four weeks later (P=0.03), to 115.97 [68.86–974.67] BAU/mL at four weeks after the second vaccination (P<0.001 vs before the first vaccination) and to 476.46 [45.00 – 1286.60] after the third vaccination (P=0.004 vs before first vaccination). An a posteriori power calculation indicated that, based on measured antibody levels, this study had 80% power to detect a 30% increase in (natural log-transformed) SARS-CoV-2-specific anti-RBD IgG concentrations at four weeks after the third vaccination.
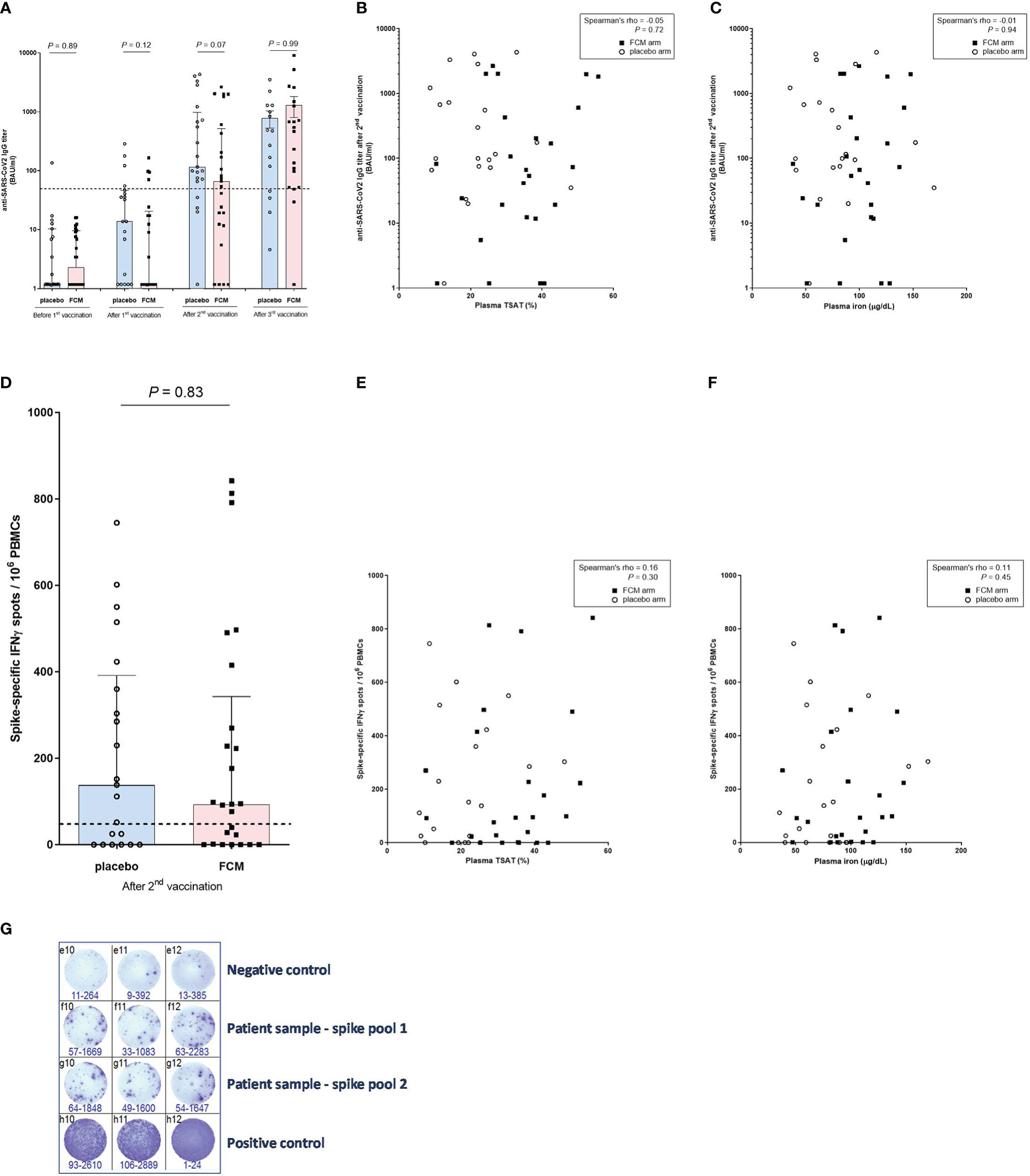
Figure 3 Anti-SARS-CoV-2 antibody and T-lymphocyte response. (A) Anti-SARS-CoV-2 IgG titers before vaccination and after the successive vaccinations in iron-deficient KTRs who had been treated with ferric carboxymaltose or placebo. The dashed horizontal line represents the threshold for IgG seropositivity. (B) Anti-SARS-CoV-2 IgG titers and transferrin saturation (in all participants). (C) Anti-SARS-CoV-2 IgG titers and plasma iron (in all participants). (D) SARS-CoV-specific T-lymphocyte response in iron-deficient KTRs who had been treated with ferric carboxymaltose or placebo. The dashed horizontal line represents the threshold for a positive T-lymphocyte response. (E) SARS-CoV-specific T-lymphocyte response and transferrin saturation (in all participants). (F) SARS-CoV-specific T-lymphocyte response and plasma iron (in all participants). (G) Example of representative results of an ELISpot assay, with which the SARS-CoV-2-specific T-lymphocyte response was measured in a patient (Spike pool 1 and 2). Interferon-gamma-producing T-lymphcytes after stimulation with SARS-CoV-2 Spike overlapping peptide pools are colored purple. All stimulations are performed in triplicate per peptide pool and the average of the six measurements is calculated. To correct for background activation, the average signal of the negative control is subtracted from the spike response.
After adjusting for total lymphocyte count (P=0.61) or eGFR (P=0.19) at baseline, there was still no significant difference in SARS-CoV-2-specific anti-RBD IgG concentration between the treatment groups at four weeks after the second vaccination. We found no correlation between TSAT (Spearman’s rho -0.05, P=0.72) or plasma ferritin (Spearman’s rho -0.15, P=0.33) or plasma iron (Spearman’s rho -0.01, P=0.94) and SARS-CoV-2-specific anti-RBD IgG concentration at four weeks after the second vaccination (Figures 3B, C). There was also no significant correlation between SARS-CoV-2-specific anti-RBD IgG concentration measured at four weeks after the second vaccination, and the total lymphocyte count at baseline (Spearman’s rho 0.26, P=0.09).
Seroconversion increased from 19% in the FCM group and 17% in the placebo group (P=0.85 between groups) at four weeks after the first vaccination to 56% in the FCM group and 80% in the placebo group (P=0.09 between groups) after the second vaccination and to 84% in the FCM group and 79% in the placebo group (P= 0.68 between groups) after the third vaccination. In all sensitivity analyses, results were highly comparable to those of the main analysis (Supplementary Tables 1–24).
SARS-CoV-2-specific T-lymphocyte response
We subsequently evaluated the effect of FCM on the SARS-CoV2-specific cellular vaccination response, expressed in number of IFN-ɣ-producing T-lymphocytes after stimulation with SARS-CoV-2 Spike, at four weeks after the second vaccination. This was the second co-primary outcome of this study. KTRs in the FCM arm had a median of 93.3 [0.85–342.5] IFN-ɣ spots per 106 PBMCs, compared to 138.3 [0.0–391.7] IFN-ɣ spots per 106 PBMCs in the KTRs in the placebo arm (P=0.83, Figure 3D). Also after adjusting for total lymphocyte count (P=0.93) or eGFR (P=0.96) at baseline, there was no difference in SARS-CoV2-specific cellular response between the treatment groups after the second vaccination. The number of IFN-ɣ spots significantly correlated with the SARS-CoV-2-specific anti-RBD IgG concentration (Spearman’s rho 0.44, P=0.002), but not with TSAT (Spearman’s rho 0.16, P=0.30, [Figure 3E]), plasma ferritin (Spearman’s rho 0.00, P=0.98), plasma iron (Spearman’s rho 0.11, P=0.45, Figure 3F) or total lymphocyte count at baseline (Spearman’s rho 0.10, P=0.50). 60% of KTRs in the FCM group and 62% of KTRs in the placebo group were T-lymphocyte responders (P=0.90). The between-group differences were non-significant in all sensitivity analyses (Supplementary Tables 25–36). An example of the results of an ELISPOT assay is depicted in Figure 3G.
Discussion
The main finding of this study is that correction of ID with FCM does not improve the humoral or cellular post-vaccination immune response against SARS-CoV-2 in KTRs. Although iron-deficient KTRs who were treated with FCM showed a significant increase in plasma ferritin and TSAT compared to placebo, there was no difference in SARS-CoV-2-specific anti-RBD IgG concentration, seroconversion rate or number of IFN-ɣ-producing T-lymphocytes after vaccination. These results are in contrast with studies in other populations, reporting improved vaccination efficacy after iron supplementation (15). A prior study showed that the antibody response after vaccination against measles virus was significantly stronger in iron-sufficient, compared to iron-deficient Chinese individuals (17). In Kenyan infants, higher TSAT predicted a stronger antibody response after vaccination against Corynebacterium diphtheriae and Streptococcus pneumonia while a lower transferrin receptor level was associated with a stronger antibody response after vaccination against poliovirus (18). In a randomized trial among the same population, oral iron supplementation before vaccination improved antibody response against measles virus (18). Iron is essential for activation, proliferation and function of T- and B-lymphocytes (17, 28), which might explain impaired vaccine efficacy associated with ID. Based on these findings we hypothesized that correction of ID would improve the immune response against SARS-CoV-2 after vaccination in KTRs. There are several potential explanations for the negative outcome of our study. First, other transplantation-related factors affecting the immune system, most importantly the use of immunosuppressive medication, may have overruled the potential effect of iron supplementation. The majority (83%) of participants was on triple immunosuppressive therapy and used mycophenolic acid; both are factors strongly associated with impaired vaccine response (10). The detrimental effect of mycophenolic acid on vaccine efficacy in KTRs was highlighted by a german study (29) reporting a beneficial effect of temporarily withholding antimetabolite treatment around the time of vaccination, although a recently published trial could not confirm these results (30). Second, we cannot exclude the possibility that iron supplementation has unfavorable effects on the immune system that might have counterbalanced potential beneficial effects. In a recently published meta-analysis, intravenous iron supplementation was associated with a higher infection risk (31), although this was not found in the PIVOTAL trial (32). While specific strains of pathogens need iron to thrive, intravenous iron supplementation may also indirectly increase the risk of infection by inducing oxidative stress, which is toxic to macrophages, neutrophils (33, 34), and lymphocytes (33, 35, 36). Third, the pathophysiological basis of ID in Dutch KTRs likely differs from ID in the previously reported populations, in which nutritional deficits and parasite infections may have played a major role (37). In our KTR population, ID is more likely induced by pro-inflammatory cytokines through upregulation of hepcidin, which prevents iron absorption from the gut and promotes iron entrapment in monocytes (38). In the context of inflammation, systemic ID may result from disordered iron distribution rather than an absolute deficit (39). It could be that only absolute ID affects vaccination response. However, in animal studies, it has been shown that not only absolute ID but also inflammation-associated ID affects T-lymphocyte response to vaccination against adenovirus (40). Furthermore, in humans with genetic hepcidin overexpression, antibody titers against various pathogens after vaccination are decreased (40). Finally, the definition of ID used in the current study may be too liberal. Since ferritin is an acute-phase protein, it can be increased by pro-inflammatory cytokines despite a depletion of iron (38). Therefore, we used a much higher cut-off value for plasma ferritin levels than what would be appropriate as a reference in the general population, although it is commonly used in chronic heart failure patients (41). In a sensitivity analysis including only KTRs with severe ID, although with limited statistical power, there was also no difference in SARS-CoV-2-specific vaccine efficacy between the two treatment arms (Supplementary Tables 5, S16, S27).
Notably, although there were no significant differences in antibody response between the treatment groups after any of the three vaccination doses, a small advance of the placebo-treated group seemed to decrease after each vaccination dose, until after the third dose, the median SARS-CoV-2-specific anti-RBD IgG concentration was slightly higher in the FCM-treated group. Another study (13), focusing on the lower antibody response to vaccination in individuals of higher age, showed that the difference between age groups decreased with each dose, thereby highlighting the efficacy of booster vaccination doses, which has also been observed in kidney transplant recipients (30).
In the current study, only 68% of patients had a positive SARS-CoV-2-specific anti-RBD IgG response and 61% had SARS-CoV-2-specific T-lymphocyte activation after the second vaccination. These numbers are similar to results of other studies among KTRs (6–10, 12). In accordance with prior studies (10, 12), there was a significant correlation between SARS-CoV-2-specific anti-RBD IgG titer and the number of IFN-ɣ spots per 106 PBMCs. However, it should be emphasized that in some KTRs who remain seronegative after vaccination against SARS-CoV-2, a cellular antibody response can be detected (42). One patient in our study had a SARS-CoV-2-specific anti-RBD IgG concentration above the threshold for a positive response before the first vaccination. This patient was seronegative for total SARS-CoV-2-specific anti-RBD antibodies, but positive for antinucleocapsid antibodies. Therefore, it is unclear whether this patient had previous exposure to SARS-CoV-2 before or the positive IgG titer was based on cross-reactivity with antibodies against other coronaviruses or antigens. Previous studies among KTRs show an incidence of a positive pre-vaccination SARS-CoV-2-specific anti-RBD IgG response of 10% (12). Three patients had a total SARS-CoV-2- specific anti-RBD antibody OD above the threshold at baseline. Two of them also had anti-nucleocapsid antibodies, suggesting previous exposure to SARS-CoV-2. In a sensitivity analysis, all four patients were excluded, and this did not affect the results (Supplementary Table 2, S13, S24).
Our study has several strengths as well as limitations. We had the unique chance to assess vaccine efficacy within the scope of a running clinical trial assessing the impact of treatment with FCM versus placebo in iron-deficient KTRs. Another strength is the simultaneous availability of SARS-CoV-2-specific anti-RBD IgG concentration and data on SARS-CoV-2-specific T-lymphocyte activation. Limitations include the relatively small number of participants; nevertheless, since we did not find any trend towards a positive effect, a larger sample size would be unlikely to lead to a different outcome. The current study involved patients participating in an ongoing clinical trial who had not received all study treatments at the time of the Dutch national COVID-19 vaccination campaign, as well as patients who had finished their participation in the trial up to a year before vaccination. Therefore, there was considerable heterogenicity in the number of treatments received at the time of vaccination and the time between the last treatment and vaccination. Nevertheless, there was a clear difference in iron status between the two arms at the time of measurements four weeks after the second vaccination. At the time of the first vaccination, only a small amount of serum was collected from a finger capillary blood sample (collected with a fingerpick sampled at home). Unfortunately, these samples did not allow us to measure iron status parameters at that time. Furthermore, most patients received the mRNA-1273 vaccine whereas some received the mRNA-BNT162b2 vaccine. However, the results were robust in a sensitivity analysis excluding patients who were vaccinated with mRNA-BNT162b2 (Supplementary Tables 4, S15, S26). We did not perform a pre-vaccination measurement of the SARS-CoV-2-specific T-lymphocyte activation. It can therefore not be excluded that some participants had baseline cellular reactivity, for example resulting from cross-reactivity against antigens of other coronaviruses, which is found in 11% of KTRs (12). Moreover, the results may be biased by other differences between the treatment arms. Patients in both groups were generally well balanced at baseline except for a slightly but significantly lower lymphocyte count and eGFR in the FCM arm. Although lymphocytopenia might restrain an adequate immune response after vaccination (10), thereby masking an effect of FCM, we did not observe an effect of FCM on vaccine efficacy after adjusting for these potential confounders. Of note, there was no correlation between lymphocyte count and number of IFN-ɣ-producing T-lymphocytes or antibody titer. Furthermore, we have not measured neutralizing antibody responses, which would have been an interesting secondary outcome. Finally, the results of our study may be specific for KTRs and cannot be extrapolated to other immunocompromised populations.
In conclusion, in KTRs with ID, intravenous iron supplementation efficiently restored iron status but did not improve the humoral or cellular immune response against SARS-CoV-2 after three vaccinations.
Data availability statement
The raw data supporting the conclusions of this article will be made available by the authors, without undue reservation.
Ethics statement
The studies involving human participants were reviewed and approved by Medical ethical committee of the University Medical Center Groningen (METc 2018/482). The patients/participants provided their written informed consent to participate in this study.
Author contributions
MD and JV developed the study design. JV coordinated the trial. MHdB supervised the trial. DB, MS, TR and J-SS contributed to the study design. DA, RJ, TN, MvdH.and MS performed analyses. All authors contributed to the article and approved the submitted version.
Funding
This study was financially supported by the Dutch Kidney Foundation (grant 17OKG18), Vifor Pharma, and the Tekke Huizenga Foundation (grant STHF-2021.01.02).
Acknowledgments
We thank R. Karsten for organisational support, and W. Dam for technical support.
Conflict of interest
JV received consulting fees from Vifor Pharma (to employer). MHdB has consultancy agreements with Amgen, Astellas, Astra Zeneca, Bayer, Kyowa Kirin, Vifor Pharma, and Sanofi Genzyme, and received grant support from Sanofi Genzyme and Vifor Pharma (all to employer). ME received consultant fees and speakers bureaus from Vifor Pharma and serves on the Advisory Board for Cablon Medical. The results presented in this paper have not been published previously in whole or part, except in abstract format.
The remaining authors declare that the research was conducted in the absence of any commercial or financial relationships that could be construed as a potential conflict of interest.
Publisher’s note
All claims expressed in this article are solely those of the authors and do not necessarily represent those of their affiliated organizations, or those of the publisher, the editors and the reviewers. Any product that may be evaluated in this article, or claim that may be made by its manufacturer, is not guaranteed or endorsed by the publisher.
Supplementary material
The Supplementary Material for this article can be found online at: https://www.frontiersin.org/articles/10.3389/fimmu.2022.1017178/full#supplementary-material
Abbreviations
- BAU, international Binding Antibody Units; BMI, body mass index; CKD-EPI, Chronic Kidney Disease Epidemiology Collaboration; COVID-19, coronavirus disease 2019; DMSO, dimethyl sulfoxide; eGFR, estimated glomerular filtration rate; ELISA, enzyme-linked immunosorbent assay; FCM, ferric carboxymaltose; ID, iron deficiency; IFN-ɣ, interferon-gamma; IgG, immunoglobulin G; IQR, interquartile range; KTR, kidney transplant recipient; METc, medical ethical committee; nOD, normalized optical density unit; PBMC, peripheral blood mononuclear cell; RBD, receptor binding domain; SARS-CoV-2, severe acute respiratory virus 2; SD, standard deviation; SFC, spot forming cells; TSAT, transferrin saturation.
References
1. Available at: https://covid19.who.int/.
2. Hilbrands LB, Duivenvoorden R, Vart P, Franssen CFM, Hemmelder MH, Jager KJ, et al. COVID-19-related mortality in kidney transplant and dialysis patients: results of the ERACODA collaboration. Nephrol Dial Transplant (2020) 35(11):1973–83. doi: 10.1093/ndt/gfaa261
3. Kremer D, Pieters TT, Verhaar MC, Berger SP, Bakker SJL, van Zuilen AD, et al. A systematic review and meta-analysis of COVID-19 in kidney transplant recipients: Lessons to be learned. Am J Transplant (2021) 21(12):3936–45. doi: 10.1111/ajt.16742
4. Caillard S, Chavarot N, Francois H, Matignon M, Greze C, Kamar N, et al. Is COVID-19 infection more severe in kidney transplant recipients? Am J Transplant (2021) 21(3):1295–303. doi: 10.1111/ajt.16424
5. Goffin E, Candellier A, Vart P, Noordzij M, Arnol M, Covic A, et al. COVID-19-related mortality in kidney transplant and haemodialysis patients: A comparative, prospective registry-based study. Nephrol Dial Transplant (2021) 36(11):2094–105. doi: 10.1093/ndt/gfab200
6. Grupper A, Rabinowich L, Schwartz D, Schwartz IF, Ben-Yehoyada M, Shashar M, et al. Reduced humoral response to mRNA SARS-CoV-2 BNT162b2 vaccine in kidney transplant recipients without prior exposure to the virus. Am J Transplant (2021) 21(8):2719–26. doi: 10.1111/ajt.16615
7. Husain SA, Tsapepas D, Paget KF, Chang JH, Crew RJ, Dube GK, et al. Postvaccine anti-SARS-CoV-2 spike protein antibody development in kidney transplant recipients. Kidney Int Rep (2021) 6(6):1699–700. doi: 10.1016/j.ekir.2021.04.017
8. Rozen-Zvi B, Yahav D, Agur T, Zingerman B, Ben-Zvi H, Atamna A, et al. Antibody response to SARS-CoV-2 mRNA vaccine among kidney transplant recipients: A prospective cohort study. Clin Microbiol Infect (2021) 27(8):1173. doi: 10.1016/j.cmi.2021.04.028
9. Danthu C, Hantz S, Dahlem A, Duval M, Ba B, Guibbert M, et al. Humoral response after SARS-CoV-2 mRNA vaccination in a cohort of hemodialysis patients and kidney transplant recipients. J Am Soc Nephrol (2021) 32(9):2153–8. doi: 10.1681/ASN.2021040490
10. Sanders JF, Bemelman FJ, Messchendorp AL, Baan CC, van Baarle D, van Binnendijk R, et al. The RECOVAC immune-response study: The immunogenicity, tolerability, and safety of COVID-19 vaccination in patients with chronic kidney disease, on dialysis, or living with a kidney transplant. Transplantation (2022) 106(4):821–34. doi: 10.1097/TP.0000000000003983
11. Korth J, Jahn M, Dorsch O, Anastasiou OE, Sorge-Hädicke B, Eisenberger U, et al. Impaired humoral response in renal transplant recipients to SARS-CoV-2 vaccination with BNT162b2 (Pfizer-BioNTech). Viruses (2021) 13(5):756. doi: 10.3390/v13050756
12. Cucchiari D, Egri N, Bodro M, Herrera S, Del Risco-Zevallos J, Casals-Urquiza J, et al. Cellular and humoral response after MRNA-1273 SARS-CoV-2 vaccine in kidney transplant recipients. Am J Transplant (2021) 21(8):2727–39. doi: 10.1111/ajt.16701
13. van den Hoogen LL, Boer M, Postema A, de Rond L, de Zeeuw-Brouwer ML, Pronk I. Reduced antibody acquisition with increasing age following vaccination with BNT162b2: Results from two longitudinal cohort studies in the Netherlands. Vaccines (Basel) (2022) 10(9):1480. doi: 10.3390/vaccines10091480
14. Eisenga MF, Minović I, Berger SP, Kootstra-Ros JE, van den Berg E, Riphagen IJ. Iron deficiency, anemia, and mortality in renal transplant recipients. Transpl Int (2016) 29(11):1176–83. doi: 10.1111/tri.12821
15. Drakesmith H, Pasricha SR, Cabantchik I, Hershko C, Weiss G, Girelli D. Vaccine efficacy and iron deficiency: an intertwined pair? Lancet Haematol (2021) 8(9):e666–9. doi: 10.1016/S2352-3026(21)00201-5
17. Jiang Y, Li C, Wu Q, An P, Huang L, Wang J, et al. Iron-dependent histone 3 lysine 9 demethylation controls b cell proliferation and humoral immune responses. Nat Commun (2019) 10(1):2935. doi: 10.1038/s41467-019-11002-5
18. Stoffel NU, Uyoga MA, Mutuku FM, Frost JN, Mwasi E, Paganini D, et al. Iron deficiency anemia at time of vaccination predicts decreased vaccine response and iron supplementation at time of vaccination increases humoral vaccine response: A birth cohort study and a randomized trial follow-up study in Kenyan infants. Front Immunol (2020) 11:1313. doi: 10.3389/fimmu.2020.01313
19. Dufour C, Papadaki H, Warren A. Available at: https://ehaweb.org/covid-19/eha-statement-on-covid-19-vaccines/recommendations-for-covid-19-vaccination-in-patients-with-non-malignant-hematologic-diseases/.
20. Levey AS, Stevens LA, Schmid CH, Zhang Y, Castro AF, Feldman HI, et al. A new equation to estimate glomerular filtration rate. Ann Intern Med (2009) 150(9):604–12. doi: 10.7326/0003-4819-150-9-200905050-00006
21. Okonko DO, Grzeslo A, Witkowski T, Mandal AKJ, Slater RM, Roughton M, et al. Effect of intravenous iron sucrose on exercise tolerance in anemic and nonanemic patients with symptomatic chronic heart failure and iron deficiency FERRIC-HF: a randomized, controlled, observer-blinded trial. J Am Coll Cardiol (2008) 51(2):103–12. doi: 10.1016/j.jacc.2007.09.036
22. Camaschella C. Iron-deficiency anemia. N Engl J Med (2015) 372(19):1832–43. doi: 10.1056/NEJMra1401038
23. Jolliff CR, Cost KM, Stivrins PC, Grossman PP, Nolte CR, Franco SM, et al. Reference intervals for serum IgG, IgA, IgM, C3, and C4 as determined by rate nephelometry. Clin Chem (1982) 28(1):126–8. doi: 10.1093/clinchem/28.1.126
24. Steenhuis M, van Mierlo G, Derksen NI, Ooijevaar-de Heer P, Kruithof S, Loeff FL, et al. Dynamics of antibodies to SARS-CoV-2 in convalescent plasma donors. Clin Transl Immunol (2021) 16(10)(5):e1285. doi: 10.1002/cti2.1285
25. Ducloux D, Colladant M, Chabannes M, Bamoulid J, Courivaud C. Factors associated with humoral response after BNT162b2 mRNA COVID-19 vaccination in kidney transplant patients. Clin Kidney J (2021) 14(10):2270–2. doi: 10.1093/ckj/sfab125
26. Vogelzang EH, Loeff FC, Derksen NIL, Kruithof S, Ooijevaar-de Heer P, van Mierlo G, et al. Development of a SARS-CoV-2 total antibody assay and the dynamics of antibody response over time in hospitalized and nonhospitalized patients with COVID-19. J Immunol (2020) 205(12):3491–9. doi: 10.4049/jimmunol.2000767
27. van der Veldt AAM, Oosting SF, Dingemans AC, Fehrmann RSN, GeurtsvanKessel C, Jalving M, et al. COVID-19 vaccination: the VOICE for patients with cancer. Nat Med (2021) 27(4):568–9. doi: 10.1038/s41591-021-01240-w
28. Schaefer B, Effenberger M, Zoller H. Iron metabolism in transplantation. Transpl Int (2014) 27(11):1109–17. doi: 10.1111/tri.12374
29. Schrezenmeier E, Rincon-Arevalo H, Jens A, Jens A, Stefanski AL, Hammett C, Osmanodja B, et al. Temporary antimetabolite treatment hold boosts SARS-CoV-2 vaccination-specific humoral and cellular immunity in kidney transplant recipients. JCI Insight (2022) 7(9):e157836. doi: 10.1172/jci.insight.157836
30. Kho MML, Lianne Messchendorp A, Frölke SC, Imhof C, Koomen VJCH, Malahe SRK, et al. Alternative strategies to increase the immunogenicity of COVID-19 vaccines in kidney transplant recipients not responding to two or three doses of an mRNA vaccine (RECOVAC): A randomised clinical trial. Lancet Infect Dis (2022). doi: 10.2139/ssrn.4176376
31. Shah AA, Donovan K, Seeley C, Dickson EA, Palmer AJR, Doree C, et al. Risk of infection associated with administration of intravenous iron: A systematic review and meta-analysis. JAMA Netw Open (2021) 4(11):e2133935. doi: 10.1001/jamanetworkopen.2021.33935
32. Macdougall IC, Bhandari S, White C, Anker SD, Farrington K, Kalra PA, et al. Intravenous iron dosing and infection risk in patients on hemodialysis: A prespecified secondary analysis of the PIVOTAL trial. J Am Soc Nephrol (2020) 31(5):1118–27. doi: 10.1681/ASN.2019090972
33. Ichii H, Masuda Y, Hassanzadeh T, Saffarian M, Gollapudi S, Vaziri ND. Iron sucrose impairs phagocytic function and promotes apoptosis in polymorphonuclear leukocytes. Am J Nephrol (2012) 36(1):50–7. doi: 10.1159/000339285
34. Rosen GM, Pou S, Ramos CL, Cohen MS, Britigan BE. Free radicals and phagocytic cells. FASEB J (1995) 9(2):200–9. doi: 10.1096/fasebj.9.2.7540156
35. Weiss G. Iron and immunity: a double-edged sword. Eur J Clin Invest (2002) 32 Suppl 1:70–8. doi: 10.1046/j.1365-2362.2002.0320s1070.x
36. de Sousa M, Reimão R, Porto G, Grady RW, Hilgartner MW, Giardina P. Iron and lymphocytes: reciprocal regulatory interactions. Curr Stud Hematol Blood Transfus (1991) 58):171–7. doi: 10.1159/000419357
37. Pasricha SR, Atkinson SH, Armitage AE, Khandwala S, Veenemans J, Cox SE, et al. Expression of the iron hormone hepcidin distinguishes different types of anemia in African children. Sci Transl Med (2014) 6(235):235re3. doi: 10.1126/scitranslmed.3008249
38. Camaschella C, Girelli D. The changing landscape of iron deficiency. Mol Aspects Med (2020) 75:100861. doi: 10.1016/j.mam.2020.100861
39. Malyszko J, Malyszko JS, Mysliwiec M. A possible role of hepcidin in the pathogenesis of anemia among kidney allograft recipients. Transplant Proc (2009) 41(8):3056–9. doi: 10.1016/j.transproceed.2009.08.003
40. Frost JN, Tan TK, Abbas M, Wideman SK, Bonadonna M, Stoffel NU, et al. Hepcidin-mediated hypoferremia disrupts immune responses to vaccination and infection. Med (N Y) (2021) 12 (2)(2):164–79. doi: 10.1016/j.medj.2020.10.004
41. Anker SD, Comin Colet J, Filippatos G, Willenheimer R, Dickstein K, Drexler H, et al. Ferric carboxymaltose in patients with heart failure and iron deficiency. N Engl J Med (2009) 361(25):2436–48. doi: 10.1056/NEJMoa0908355
Keywords: iron deficiency, SARS-CoV-2, kidney transplantation, vaccination, randomized controlled (clinical) trial
Citation: Vinke JSJ, Altulea DHA, Eisenga MF, Jagersma RL, Niekolaas TM, van Baarle D, Heiden MvD, Steenhuis M, Rispens T, Abdulahad WH, Sanders J-SF and De Borst MH (2023) Ferric carboxymaltose and SARS-CoV-2 vaccination-induced immunogenicity in kidney transplant recipients with iron deficiency: The COVAC-EFFECT randomized controlled trial. Front. Immunol. 13:1017178. doi: 10.3389/fimmu.2022.1017178
Received: 11 August 2022; Accepted: 05 December 2022;
Published: 04 January 2023.
Edited by:
Ziwei Zhang, Northeast Agricultural University, ChinaReviewed by:
Nicole Ursula Stoffel, ETH Zürich, SwitzerlandSebastian Dolff, University Duisburg-Essen, Germany
Copyright © 2023 Vinke, Altulea, Eisenga, Jagersma, Niekolaas, van Baarle, Heiden, Steenhuis, Rispens, Abdulahad, Sanders and De Borst. This is an open-access article distributed under the terms of the Creative Commons Attribution License (CC BY). The use, distribution or reproduction in other forums is permitted, provided the original author(s) and the copyright owner(s) are credited and that the original publication in this journal is cited, in accordance with accepted academic practice. No use, distribution or reproduction is permitted which does not comply with these terms.
*Correspondence: Martin H. De Borst, bS5oLmRlLmJvcnN0QHVtY2cubmw=