- 1Experimental Haematology Unit, Division of Immunology Transplantation and Infectious Diseases, Vita-Salute San Raffaele University, Milan, Italy
- 2Translational and Clinical Research Institute, Faculty of Medical Sciences, Newcastle University, Newcastle upon Tyne, United Kingdom
- 3JLacerda Lab, Hematology and Transplantation Immunology, Instituto de Medicina Molecular João Lobo Antunes, Faculdade de Medicina da Universidade de Lisboa, Lisbon, Portugal
- 4Serviço de Hematologia e Transplantação de Medula, Hospital de Santa Maria, Centro Hospitalar Universitário de Lisboa Norte, Lisbon, Portugal
- 5Department of Hematology, Oncology, and Cancer Immunology, Charité Universitätsmedizin Berlin, Berlin, Germany
- 6Alcyomics Ltd, Newcastle upon Tyne, United Kingdom
- 7Department of Dermatology, Medical University of Vienna, Vienna, Austria
- 8Ludwig Boltzmann Institute for Rare and Undiagnosed Diseases, Vienna, Austria
- 9CeMM Research Center for Molecular Medicine of the Austrian Academy of Sciences, Vienna, Austria
- 10Systems Biology Ireland, School of Medicine, Conway Institute, University College Dublin, Dublin, Ireland
- 11Department of Pharmacology, University of Oslo and Oslo University Hospital, Oslo, Norway
Hematopoietic allogeneic stem cell transplantation (allo-SCT) is a curative option for patients with hematological malignancies. However, due to disparities in major and minor histocompatibility antigens between donor and recipient, severe inflammatory complications can occur, among which chronic graft-versus-host disease (cGVHD) can be life-threatening. A classical therapeutic approach to the prevention and treatment of cGVHD has been broad immunosuppression, but more recently adjuvant immunotherapies have been tested. This review summarizes and discusses immunomodulatory approaches with T cells, including chimeric antigen receptor (CAR) and regulatory T cells, with natural killer (NK) cells and innate lymphoid cells (ILCs), and finally with mesenchymal stromal cells (MSC) and extracellular vesicles thereof. Clinical studies and pre-clinical research results are presented likewise.
1 Introduction
Chronic graft-versus-host disease (cGVHD) is a multisystem inflammatory disease that results from a multifaceted response of allogeneic immune effector cells against a variety of tissues in patients surviving early phases of allogeneic hematopoietic stem cell transplantation (alloHSCT). Mild forms of cGVHD associate with better control of the malignant disease. In contrast, severe cGVHD is the main reason for morbidity and mortality in long-term survivors of alloHSCT. Standard therapies for severe cGVHD have limited efficacy and significant toxicity (1), and require suppression of the immune system that may result in severe and/or fatal infections (2). Furthermore, current pharmacological interventions are largely unsuccessful in reversing established fibrosis in cGVHD, without compromising immune function. Therefore, new approaches for the prevention and treatment of cGVHD are needed in order to improve the long-term outcomes and quality of life post alloHSCT (3). Here, we highlight novel strategies to prevent and treat cGVHD using cellular therapy, and discuss the latest developments in this field (Figure 1).
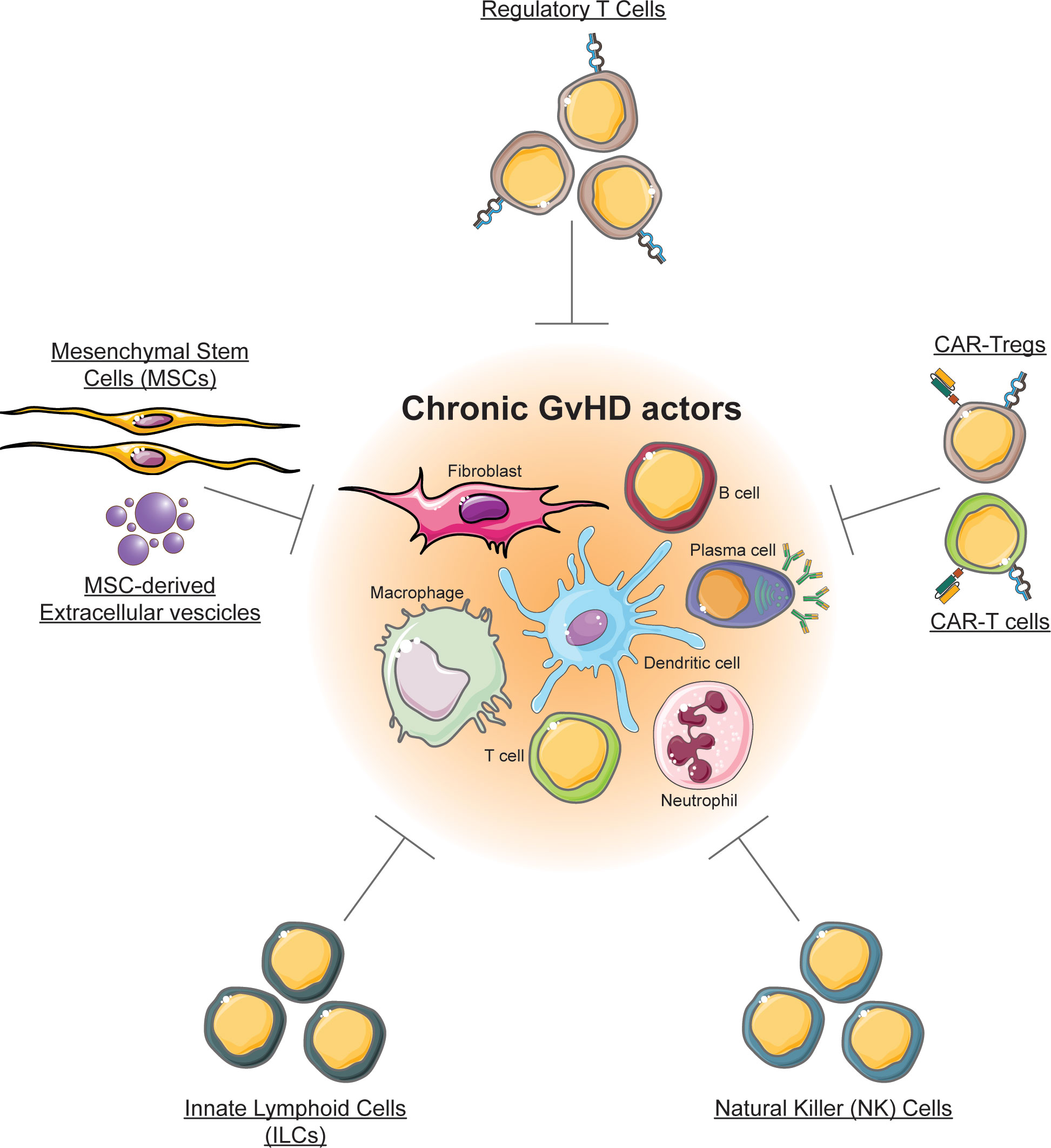
Figure 1 Cellular therapy for chronic GVHD. The figure illustrates cell types that are currently exploited or that can be exploited for chronic GVHD, and that are discussed in this review.
Regulatory T cells (Tregs) represent a subset of CD4+ T lymphocytes specialized in controlling immune responses and in maintaining immune tolerance. The efficacy of Tregs in GVHD treatment has been initially demonstrated in murine models (4), and further in human patients (5). An innovative possibility to increase the anti-GVHD efficacy of cellular therapy is represented by Chimeric Antigen Receptors (CARs). As an example, CAR-Tregs targeting allo-activated immune cells expressing CD38 were highly effective in reducing GVHD (6). Invariant NKT (iNKT) cells can dampen cGVHD through induction of Tregs. Also, NK cells may be exploited to target the alloreactive cells, while innate lymphoid cells (ILC) that have a crucial role in tissue regeneration of the intestinal tract, could represent an attractive treatment option for severe cases of intestinal GVHD (7). Another focus is treatment with cell types that are involved in tissue homeostasis and repair, as mesenchymal stromal cells (MSCs), and we also discuss the utility of extracellular vesicles derived from MSCs.
2 T cell-based therapies
T lymphocytes have a pivotal role in the immune system. Conventional CD4+ T cells (Tconvs) secrete a number of immunoregulatory cytokines, while cytotoxic CD8+ T cells are equipped to kill malignant or infected cells. T cells have been extensively exploited in the context of bone marrow transplantation, with the aim to recognize and kill tumor cells (Graft-versus-Leukemia effect, GvL), and control infections, especially opportunistic ones (8).
However, T cells are also involved in the development of GVHD due to mismatches of HLA class I molecules and minor histocompatibility antigens between the host and the donor, which elicit an inflammatory response (9, 10). For this reason, different strategies have been developed to dampen T cell activation, aiming at blocking this detrimental process (11). Several drugs have been approved so far for the treatment of GVHD, however, their activity is broad and unspecific, acting on different components of the inflammatory cascade with a generalized immunosuppressive action, blocking not only harmful T cells but also vital anti-viral and anti-tumor effects (11, 12).
For this reason, the development of more specific treatments that target directly key components of GVHD, is required. To this end, the same Tconvs could be employed to eliminate specific cell populations that sustain the inflammatory process (13, 14). However, this approach is limited by the low number of antigen-specific cells able to kill relevant pathologic cells (15). A possible solution to this problem is represented by CAR-T-cells.
2.1 CAR-T-cells
CARs are chimeric molecules composed of two major components (Figure 2). An extracellular part consisting of a single-chain variable fragment (scFv) conferring antigen specificity, is fused to an intracellular part providing the machinery for cell signaling transduction. The first generation of CARs was composed of an intracellular CD3 zeta chain, but this version showed only minimal functionality. In the subsequent generations, different stimulatory domains were fused together, obtaining much higher cellular activation. CARs greatly enhance cellular functions such as cytotoxicity, proliferation, survival and cytokine secretion. The constructs can be inserted in different effector cells, as T cells and NK cells. Their major field of application is in oncology, where CAR-T cells have displayed striking results, in particular against CD19+ acute lymphoblastic leukemia (ALL) and B cell-derived lymphomas (16).
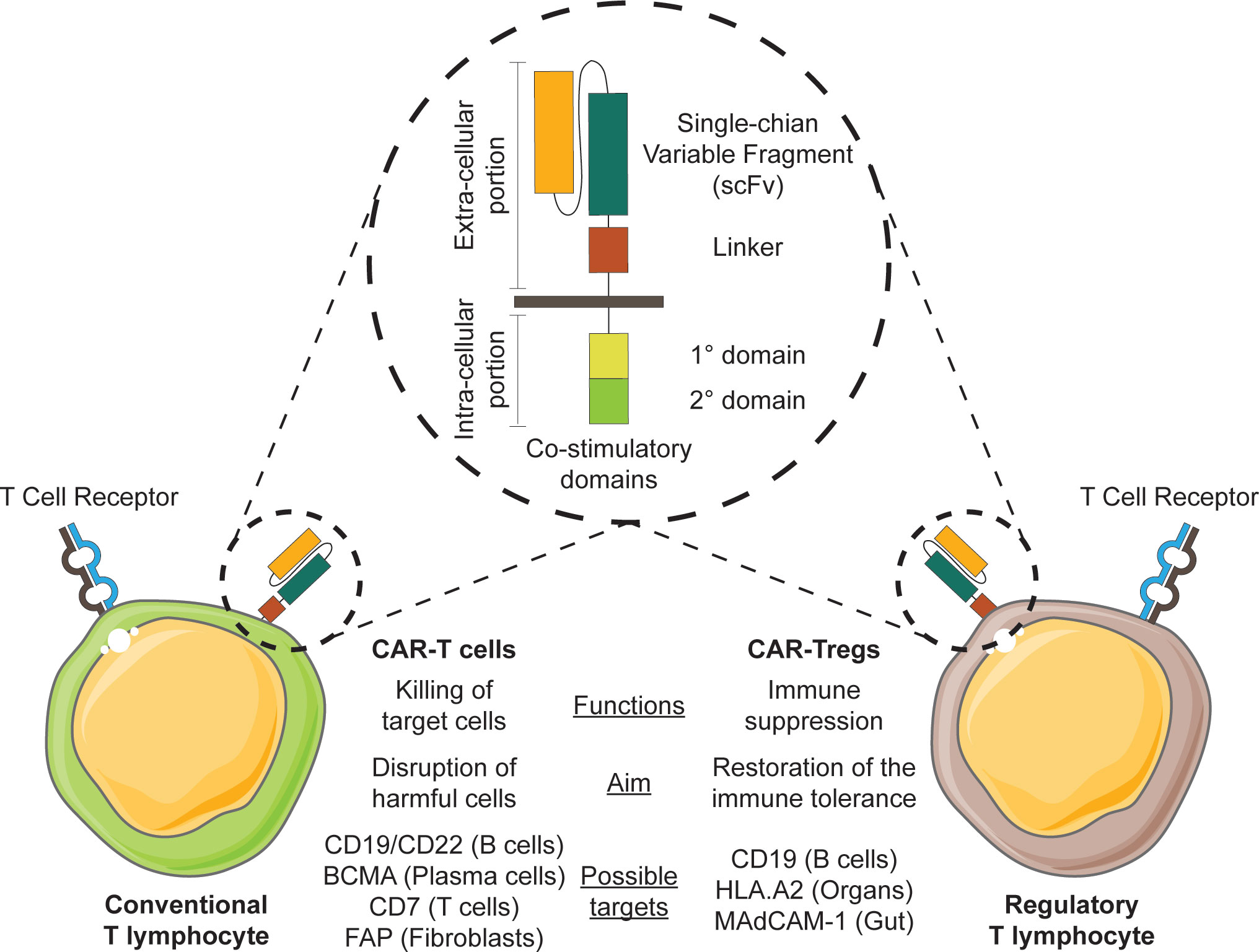
Figure 2 Exploiting CAR-T cell therapy for chronic GVHD. The engineering of CAR constructs (illustrated in the circle) in conventional T cells or T regulatory cells may be exploited in therapy for chronic GVHD.
While CAR constructs initially were developed for use in cancer therapy (17, 18), some CARs might also be employed to tackle cells that mediate the inflammatory processes occurring during cGVHD (19). Various immune cells contribute to the pathology of cGVHD, and among them B cells produce antibodies and release pro-inflammatory cytokines, and operate as antigen-presenting cells to promote T cell activation. The B cells appear to be hyper-responsive due to both intrinsic and extrinsic factors, favoring the selection of potential harmful cells reactive against both allo- and auto-antigens (20, 21).
Due to their pro-inflammatory properties, the selective depletion of B cells might represent a potential therapeutic option in patients with cGVHD. Several drugs have been approved for this purpose, with variable results. One of the major limitations of the drugs resides in the absence of a clear specificity, often targeting markers that are expressed on multiple cell populations (22). A possible solution is the employment of CAR-T cells, such as the anti-CD19 or the anti-CD22 CARs, which could eliminate B lymphocytes. In particular, a beneficial anti-inflammatory activity of anti-CD19 CAR-T cells have been shown in the context of autoimmune diseases, like systemic lupus erythematosus, where reduced levels of auto-antibodies and immune complexes were demonstrated in lupus-prone mice. However, clinical evidence for a role of anti-CD19 CAR-T cells in the treatment of cGVHD in humans is still lacking (23).
It has been demonstrated that cGVHD patients may develop auto-antibodies during the course of the disease, which might have a role in mediating tissue injuries (24, 25). Pre-clinical mouse models clearly showed that the depletion of antibody-secreting cells could attenuate clinical manifestations of cGVHD (26). Anti-BCMA CAR-T cells can efficiently kill plasma cells and they have been employed for the treatment of patients with multiple myeloma with encouraging results (27). Considering their potential role in the pathogenesis of cGVHD, anti-BCMA CAR-T cells might open new therapeutic scenarios due to the depletion of allo- and auto-reactive plasma cells.
Anti-CD19 and anti-BCMA CARs eliminate B cells and plasma cells, respectively, without a specific activity against the pathological clones. In 2016, Ellebrecht et al. developed a new chimeric construct to selectively target and deplete antigen-specific B cells, called chimeric auto-antibody receptor (CAAR) (28). The authors employed CAAR-T cells for the treatment of pemphigus vulgaris, an autoimmune disease caused by the production of auto-antibodies against the dermal basal membrane, by redirecting T lymphocytes against anti-desmoglein 3 self-reactive B cells, showing encouraging results in pre-clinical mouse models. With this approach, the authors managed to selectively deplete only self-reactive cells. CAARs might be employed in the context of cGVHD to selectively eliminate those cells that sustain the pathologic process, avoiding the generalized depletion of either B lymphocytes or plasma cells caused by anti-CD19 and anti-BCMA CAR-T cells (28).
Beside B lymphocytes, also T cells have an important role in the pathogenesis of GVHD, being able to recognize HLA mismatches and to stimulate pro-inflammatory responses. Several drugs target T cells, controlling the inflammatory response and showing encouraging results in cGVHD patients. Also, CAR-T cells might be employed to this extent: anti-CD7 CAR-T cells have been designed to specifically eliminate T cells in the context of T cell malignancies, due to the selective expression of CD7 on T lymphocytes and NK cells. To circumvent a potential fratricide effect, engineered cells were manipulated with CRISPR/Cas9 to disrupt the CD7 gene. This product showed promising result in cancer and might be potentially employed also in the context of cGVHD to control the inflammatory response mediated by T cells (29).
Apart from immune cells, other cells participate in the generation of tissue injuries in cGVHD. In particular, fibroblasts have a well-established role in mediating collagen deposition and fibrosis, especially in the skin and lungs. Lung fibrosis represents one of the most important causes of disability and mortality in cGVHD patients. Macrophages and T cells have an important role in inducing fibroblast proliferation and collagen deposition and their depletion ameliorates the occurrence of fibrosis in pre-clinical models. In particular, the polarization of macrophages toward the M2 phenotype is a key event in this process: these cells secrete high amounts of TGF-β and IL-4 that stimulate fibroblasts and boost their activity (30, 31). In 2019, Aghajanian and colleagues developed an anti-fibroblast activation protein (FAP) CAR construct to target and kill fibroblasts (32). Anti-FAP CAR-T cells were evaluated in a pre-clinical mouse model of cardiac fibrosis, proving effective in ameliorating the cardiac lesions. In particular, treated mice displayed a significant reduction of the number of fibroblasts and consequently of the fibrosis compared to the control group. The therapy was coupled to an improvement of cardiac functional parameters, like fractional shortening and mitral valve inflow velocity (32). Considering the important role of fibroblasts in cGVHD pathogenesis, anti-FAP CAR-T cells might represent a promising therapeutic tool for patients in order to limit fibroblast proliferation and subsequent fibrosis, especially in lungs.
2.2 Regulatory T cells (Tregs)
Tregs represent a subset of CD4+ T lymphocytes specialized in controlling immune responses and in maintaining immune tolerance (33). Tregs can be divided into two distinct subsets: thymic or centrally derived Tregs, and peripherally induced Tregs. The first group encompasses those cells that directly exit the thymus with already acquired immune suppressive capabilities, and are responsible for the maintenance of self-tolerance. The second group includes those lymphocytes generated in the periphery after the activation of CD4+ Tconvs and are thought to control local activation of the immune response (34, 35). A unique marker for the identification of Tregs is still lacking, and up to now a combination of markers is required for their identification. Tregs are characterized by high expression of the FoxP3 (Forkhead Box P3) transcription factor, which represents the master regulator for Treg development and function. High CD25 (the alpha subunit of the interleukin-2 (IL-2) receptor) expression level is another Treg feature, also denoting their great dependency of IL-2 (36).
Regardless of their origin, Tregs are endowed with suppressive capabilities that can be exerted either via the secretion of mediators or via cell-to-cell contact. Their action is fundamental for the correct homeostasis of the immune system. Indeed, patients diagnosed with the IPEX syndrome (congenital deficiency of FoxP3) lack functional Tregs and suffer from several autoimmune manifestations (37).
In the context of HSCT, the equilibrium between effector and suppressive T cells appears to play an important role in the development of cGVHD. Even though some studies did not find lower Treg function in these patients, a plethora of studies have now shown that altered Treg homeostasis and decreased Treg counts are associated with cGVHD (38–43).
Based on the recognition that Treg counts are decreased in patients with cGVHD and that the emergence of this syndrome is associated with poor immune tolerance post-transplant, Koreth et al. at the Dana-Farber Cancer Institute conducted Phase 1 and Phase 2 studies investigating whether daily low-dose IL-2 administered sub-cutaneously for 8 to 12 weeks would have an effect in patients with steroid refractory (SR) cGVHD, and if there was any correlation with Treg counts and function. In these trials, approximately 50-60% of the patients responded clinically, some quite significantly, allowing for steroid doses to be tapered, which was correlated with a significant increase in Treg counts (44, 45). These studies set the stage for trials of donor Treg infusions, with the rationale that the patients not responding to low-dose IL-2 were likely to have an exhausted Treg compartment and could therefore benefit from the infusion of fresh donor Tregs, with or without low-dose IL-2.
2.2.1 cGVHD prevention employing Tregs
Several preclinical and clinical studies have suggested that enriching donor grafts with donor Tregs could improve the outcome of transplant by facilitating immune reconstitution and reducing the risk of GVHD, without interfering with the anti-tumor activity (4, 46, 47).
A major advantage of Treg-therapy is the capacity to induce allo-specific suppression, avoiding the side effects of general immunosuppressive drugs. In humans, Treg-based cell therapy consists of isolation and purification of circulating Tregs from peripheral blood (PB) or umbilical cord-blood (UCB), expansion, and transfer to the patient. However, the purification and expansion of sufficient numbers of Tregs for effective immunotherapy is hampered by their low number in peripheral blood, the lack of specific phenotypic markers for their isolation, and the slow rate of in vitro expansion for clinical use (48). To bypass these issues, several protocols have been applied for de novo generation and expansion of functional Tregs (49, 50).
Expansion protocols usually start from peripheral blood mononuclear cells (PBMCs), either involving the use of stimulatory antibodies (e.g. anti-CD3, soluble CD28, anti-CD3/CD28 magnetic beads) or engineered APCs. In addition, several compounds have been employed to enrich for Tregs at the end of the cell culture, either by blocking Tconv activation, boosting Treg expansion or combining the two approaches (51). Tregs can be isolated from PBMCs using a CD4+CD25+CD127dim/− Treg cell isolation kit. However, activated Tconvs display high levels of CD25 and up-regulate FoxP3, thus limiting the sorting strategy. Furthermore, experimental evidence showed that cells with a Treg phenotype in pro-inflammatory environments can be reprogrammed toward Th17 pro-inflammatory cells with an amplification rather than an attenuation of tissue damage. Considering these factors, the risk of having a contaminant Tconv population after sorting for Tregs is high, in particular in in vitro expanded cells starting from PBMCs (52, 53).
In the last decade, the safety and feasibility of Treg-based cell therapy for GVHD prevention and treatment has been tested in several clinical trials, as mentioned in Table 1. The Perugia group was the first to provide proof of principle that purified natural polyclonal Tregs can prevent GVHD mediated by Tconvs in the setting of haploidentical transplantation for hematologic malignancies, with no pharmacologic GVHD prophylaxis. Patients received CliniMACS immunomagnetic purified donor Tregs (2×106/kg) at day -4, followed by a purified CD34+ hematopoietic stem cell graft and Tconv infusion at day 0. The upfront infusion of Tregs into conditioned patients permitted the in vivo expansion of Tregs, as previously described in mouse models (66). To establish the optimal Treg : Tconv ratio, different escalating doses of donor Tconv (0.5 - 2x106/kg) were administered. Di Ianni et al. reported the first results from 28 patients (24 in any complete remission and 4 in relapse) showing a rapid immune reconstitution with a wide repertoire, an increased reconstitution of pathogen-specific CD4+ and CD8+ T cells, and a low incidence of CMV reactivation. Two patients developed ≥ grade 2 acute GVHD (aGVHD) and no patients developed cGVHD (5). Martelli et al. extended the analysis to 43 patients with high-risk acute myeloid leukemia (AML) in any remission to investigate whether Treg-Tconv adoptive immunotherapy would prevent post-transplant leukemia relapse. In this setting, the incidence of ≥ grade 2 aGVHD was 15% and only one patient developed cGVHD (54). These results show that adoptive therapy with Tregs is a feasible option in HLA-haploidentical transplant, since it enables the control of alloreactive co-infused Tconvs, with low incidence of GVHD and persistence of a strong GvL effect.
More recently, the same Treg-Tconv adoptive immunotherapy protocol was tested in combination with a low-toxicity conditioning regimen including total marrow and lymphoid irradiation (TMLI). Pierini et al. designed this protocol for aged or unfit AML patients, unable to tolerate total body irradiation (TBI). Fourteen patients were included and the results showed that Tregs (2x106/kg, day-4) can prevent cGVHD with no need for post-transplant immunosuppression, and the co-administration of Tconvs (1x106/kg, day-1) could eradicate minimal residual disease and ensure relapse free survival (55). These results have been replicated in an updated trial conducted by the same group, which combined and age-adapted myeloablative conditioning regimen, using TBI or TMLI, with Treg-Tconv adoptive immunotherapy (60). At a median follow-up of 29 months, moderate/severe cGVHD occurred in only one patient and 2 patients relapsed.
Considering the low number of Tregs in the peripheral blood, several groups have explored ex-vivo Treg expansion for therapeutic application using different protocols while retaining Treg suppressive activity (67, 68). The first-in-human clinical trial demonstrating safety and preliminary effectiveness of ex-vivo expanded Tregs for GVHD prophylaxis in double UCB transplant was published in 2011 by Brunstein et al. (68). Cryopreserved UCB-derived Tregs were expanded in culture with anti-CD3/anti-CD28 antibody-coated beads and recombinant human IL-2. Twenty-three patients received a dose of 0.1-30 ×105 Treg/kg at day +1, followed by GVHD prophylaxis with cyclosporine A (CsA)/sirolimus and mycophenolate mofetil (MMF). After infusion, Tregs could be detected for 14 days, with the greatest proportion of circulating Tregs observed on day + 2. There were no transfusion-related adverse events and compared with identically treated 108 historical controls, there was a reduced incidence of grade II-IV aGVHD (43% vs 61%; p=0.05) with no increased risk of infection, relapse, or early mortality (56).
In 2016 an extended analysis was published, confirming the safety profile and maximal tolerated dose. Here, in order to maximize the infused Treg dose and increase Treg potency, selected CD25+ UCB cells were expanded in cultures with K562 cells modified to express the high-affinity Fc receptor CD64 and CD86 (CD28L), in the presence of IL-2. Eleven patients received a dose of 3-100×106 Treg/kg. There were no dose-limiting infusion adverse events. The incidence of grade II-IV aGVHD at 100 days was 9% vs 45% (p=0.05) comparing to a non-randomized Sirolimus/MMF control group. The incidence of cGVHD at one year was 0 vs 14% (57).
Another approach to expanded UCB-derived Tregs for GVHD prophylaxis was developed using fucosylation of the expanded human Tregs in order to improve their trafficking pattern. Tregs bind endothelial E- and P-selectins for trafficking to sites of inflammation, and the incubation of Tregs with fucosyltransferase-VI has shown to effectively increased their homing in severe immunodeficient mice (69). Based on these results, Kellner et al. designed a pilot study of adoptive therapy with fucosylated ex-vivo expanded UCB-derived Tregs in allo-HSCT patients. Five patients were included and a dose of 1 x106 Treg/kg was infused at day -1. Although all patients developed grade II-IV aGVHD, there was no evidence of cGVHD at a median follow up of 25 months (58).
Despite the different protocols, the purity of Treg products has been difficult to control, which can limit the efficacy of Treg infusion due to contamination with effector cells. In order to increase Treg purity, Meyer et al. developed a strategy of double Treg selection using immunomagnetic selection and high-speed flow cytometric sorting. This protocol was used in 12 patients with various hematologic malignancies after a myeloablative transplant (59). A purity of 91%-96% was obtained and Tregs were infused on day 0 (1-3x106/Kg) followed by Tconv infusion at 1:1 ratio on day +2. The first 5 patients received cryopreserved Tregs, with 2 patients developing grade II-IV aGVHD. Seven patients received fresh Tregs and single-agent GVHD prophylaxis, with no acute nor cGVHD development at a median of 501 days post HSCT (59). These results suggest that there is a reduced functionality of Tregs after cryopreservation, and that highly purified Tregs may avoid Treg expansion.
2.2.2 Tregs for cGVHD treatment
Although adoptive Treg therapy has been mainly tested in GVHD prevention, ongoing and completed clinical trials investigate the safety and efficacy of polyclonal Treg infusion in the treatment of SR cGVHD. The first-in-human clinical study suggesting Treg infusion as an adjuvant therapy in refractory GVHD was published in 2009 by Trzonkowski et al. (61). Donor-Tregs were incubated with anti-CD3/CD28 beads and expanded with high dose IL-2; the results show significant alleviation of symptoms, allowing reduction of immunosuppression.
In 2015, Theil et al. used adoptive therapy with donor-expanded Tregs to treat refractory cGVHD in patients with AML who underwent allo-HSCT (62). After incubation with anti-CD3/CD28 and expansion with high dose IL-2 and rapamycin for 2–3 weeks, the final products contained mean quantity of 2.4x106 Treg/kg with an average purity of 84.1%. The expanded cells showed suppressive function in vitro and were infused at a median time of 35 weeks post-transplant. Five patients were infused; 2/5 patients had an increase of circulating Tregs and partial response of cGVHD. Three patients were alive at the end of the follow-up period and 2 patients developed skin cancers after 4 and 11 months of Treg infusion (62).
Later on, Johnston et al. accessed the safety and tolerability of Treg therapy for SR cGVHD in matched related donor recipients, using highly purified donor-derived Tregs, through clinical scale high speed cell sorting. Tregs were administered in a single infusion, at three different doses. The maximum feasible dose attainable without ex vivo expansion was 1.5 x106 Treg/kg, resulting in encouraging preliminary clinical responses (63).
In 2015, the clinical consortium TREGeneration, coordinated by João Lacerda Lab in Lisbon, was created with the primary aim to explore the safety (phase 1) and preliminary efficacy (phase 2) of different donor-derived Treg products for the treatment of patients with SR cGVHD after allo-HSCT. This research is running in parallel in several institutions, using different protocols for Treg preparation and/or administration (Table 1). Results from the phase 1 study conducted at the Dana Farber Cancer Institute-Boston were recently published. This group showed that a single infusion of polyclonal Treg-enriched lymphocytes, from the original stem cell donor, followed by daily low-dose IL-2 was safe and well tolerated, with clinical benefit, including in those with inadequate responses to IL-2 alone (64). Furthermore, there was an increase in Treg repertoire diversity, with expansion and long-term persistence of infused Treg clonotypes.
Overall, the safety of Treg adoptive immunotherapy has been successfully validated. However, new questions were raised concerning the ideal source, isolation method, dose and timing of Treg infusion. Ongoing clinical trials investigating the efficacy of Treg infusion for GVHD prevention and treatment in larger populations will help clarify these questions and will give us important additional information about the clinical implementation of these cells in the near future.
2.2.3 CAR-Tregs
Finally, in this new era of gene and cell therapy we anticipate that the use of polyclonal Treg-based therapy will shift towards engineered antigen-specific Tregs, likely able to generate more potent responses, without the concerns of off-target effects (51). In this setting, the development of CAR-Tregs appears to be a promising strategy. In two pre-clinical models, CAR-Tregs demonstrated effectiveness in controlling GVHD manifestations. In 2016, MacDonald et al. generated alloantigen-specific anti-HLA-A2 CAR-Tregs. The authors demonstrated that CAR-Tregs performed better than untransduced Tregs in suppressing the in vitro proliferation of HLA-A2 PBMCs. Strikingly, only anti-HLA-A2 CAR-Tregs were able to efficiently prevent the onset of xenogenic GVHD in a mouse model of HSCT (70). Similar results were published in 2017 by Pierini et al. The authors generated customizable CAR-Tregs, specific against CXCL12 and MAdCAM-1 to localize the cells in the gut. Engineered Tregs managed to efficiently suppress the inflammatory response in a mouse model of intestinal acute GVHD, thus ameliorating the clinical picture (71). In addition, Imura et al. in 2020 proved the efficacy of anti-CD19 CAR-Tregs in controlling the clinical manifestations in a mouse model of xeno-GvHD (72).
It is well established that Tregs can preserve the immune tolerance by directly killing activated target cells, e.g. Tconvs and APCs. However, the exact mechanisms are not fully elucidated due to contradictory results, which have been extensively discussed elsewhere (73). A direct cytotoxic effect might represent a potential concern for the use of engineered Tregs, limiting the employment of CAR-Tregs redirected against self-antigens and healthy tissues. Several CAR-Treg studies reported a negligible cytotoxic effect of engineered cells both in vitro and in vivo. Some authors described a minimal cytotoxicity only in vitro culturing the cells at high effector-to-target ratios and only with specific CAR molecules (74–76). It is still not clear whether this might be dependent on the manufacturing protocol, Treg selection, CAR structure or the CAR target. Although a potential cytotoxicity might represent a limitation for the use of anti-self CAR-Tregs, this capacity might be useful in specific circumstances. Indeed, the direct killing of pathogenic cells and the suppression of by-stander ones might help to better control the inflammatory response in GvHD. This is supported by some publications that demonstrated how CAR-Tregs might control GvHD, while preserving the Graft-versus-Tumor (GvT) effect in mice (77). Further studies are required to better elucidate the suppression mechanisms of CAR-Tregs and their regulation.
3 NK cells
NK cells are the first lymphocytes to reconstitute after both autologous or allogeneic HSCT, as well as after UCB transplantation. NK cells have been well studied in terms of acute GVHD, where they appear to protect from disease by targeting alloreactive T cells. There are fewer studies on NK cells in the context of cGVHD, and still inconclusive whether they contribute to or protect from disease. As such, there are currently no NK cell-based therapies, but strategies targeting or utilizing NK cells should be explored.
An early study reported that patients with extensive cGVHD had low blood NK cell counts (78), and another study showed that there were fewer NK cells in skin lesions of cGVHD patients compared to aGVHD patients in a cohort of patients receiving allo-HSCT from HLA-matched siblings (79). These finding suggest that low blood NK cell counts may not reflect increased tissue re-localization and infiltration of NK cells into sites of cGVHD. This notion is supported by studies showing correlations of NK cell numbers and their functional capacities with cGVHD outcome. A study comparing UCB with peripheral blood HSCT reported a higher increase of NK cells in recipients of UCB, with concomitant lower rate of cGVHD (80), although the authors could not demonstrate any direct association of risk to NK cell parameters. Another study in pediatric UCB transplant patients, reported that early detection of NK cells with functional capacity was a contributing factor for low incidence of cGVHD (81).
As to any direct role in cGVHD pathology, an early study suggested that NK cells in collaboration with suppressive CD8+ T cells could contribute to suppression of autoantibody-producing B cells, and that this function may be compromised in autoimmune diseases (82). Other studies strongly point to involvement of the MICA-NKG2D axis that could be detrimental in terms of cGVHD. Elevated soluble MICA levels in plasma have been associated to cGVHD susceptibility, in particular the MICA-129Val polymorphism (83). The MICA-NKG2D axis is well characterized in the context of cancer, where engagement of MICA on tumor cells by NKG2D unleash NK cell cytotoxicity. Elevated soluble MICA levels in a pro-inflammatory milieu in cGVHD could drive pathogenic IFN-γ from NK cells contributing to exacerbating cGVHD, as suggested in a study by Boukouaci and colleagues (84). Production of INF-γ by donor effector cells associate to onset of cGVHD, and IFN-γ promotes secretion of BAFF from monocytes that promote B cell activation and autoantibody production (85). Thus, dampening IFN-γ production by NK cells through the MICA-NKG2D axis could be a therapeutic option.
A perhaps more feasible avenue is to explore CAR NK cells, in analogy to the use of CAR-T cells described above. Potentially, CAR NK cells targeting CD19 or CD20 could be exploited in cGVHD as a potentially safer alternative to CAR-T cells. The use of CAR NK cells against cancer has shown great promise, and CD19 or CD20 CAR NK-92 cells or allogeneic primary NK cells have successfully been used to eradicate B cell malignancies (86–88). There are currently no trials on CAR NK cells in context of cGVHD, but it is obvious that CAR NK cells could have utility in targeting and eradicating pathologic B cells in cGVHD patients.
4 Invariant NKT cells
Invariant NKT cells, also known as classical or type I NKT cells, is a group of T cells with innate properties. The cells express a semi-invariant TCR α-chain (V α 24Jα18 paiting with β11 in humans, or Vα14Jα18 in mice pairing with a restricted set of TCRβ chains (89), together with NK cell receptors. iNKT cells produce high amounts of IL-4 and IFN-γ, and their development is dependent on the transcription factor promyelocytic leukemia zink finger (PLZF). Their TCR recognize glycolipids presented by the non-polymorphic MHC-1-like molecule CD1d (90). Although they are normally in low numbers in peripheral blood, they represent an important population of immunoregulatory cells.
Studies on the relationship of doses of different immune cell populations in allografts have demonstrated that the dose of iNKT cells, as well as Tregs, impact both disease-free survival and overall survival (91). Early studies showed that expanding iNKT cells in murine models of GVHD protected against GVHD through polarization towards a Th2 response and donor Treg expansion, effects that were dependent on IL-4 produced by the iNKT cells (92). Recent studies have demonstrated that iNKT represent a promising candidate for cellular therapy of cGvHD. Adoptive transfer of donor iNKT cells to mice were shown to prevent cGVHD and even reverse lung cGVHD, and the authors suggested this was linked to increased frequencies of follicular Tregs via production of IL-4 from the iNKT cells (93). In terms of their future clinical use, several studies have shown the feasibility of expanding iNKT cells using IL-2 and the synthetic ligand α-GalCer (94).
5 Innate lymphoid cells
ILCs comprise a group of cells with lymphoid morphology and various functions, ranging from important roles in tissue homeostasis and autoimmunity to immune defense against invading pathogens (95, 96). Depending on the pattern of cytokine secretion and the expression of transcription factors, ILC1, ILC2 and ILC3 have been described with different functions for immunity and tissue integrity. While ILC1 are proinflammatory IFN-γ-producing cells (97), ILC2 play a role in atopic diseases and tissue repair (98), and ILC3 are important for tissue homeostasis and can inhibit pathological T cell responses (99, 100). Given their involvement in tissue repair and homeostasis, ILC are optimal cellular targets in GVHD, as tissue damage and impaired tissue homeostasis are the main factors in GVHD pathophysiology and drivers of the disease, in addition to autoreactive immune components (101).
Indeed, evidence from mouse models of GVHD suggests that ILC play a role in the pathogenesis of GVHD. Elegant mouse models showed that IL-23-responsive ILC3 produce IL-22, which protects intestinal cells from tissue damage and acts as critical regulator for GVHD (102). ILC-derived IL-22 reduced the severity of acute GVHD and GVHD-related mortality in this mouse model (102). A similar effect was shown in another mouse model with ILC2, which were severely affected by conditioning regimens (103). However, upon infusion of donor ILC2, gastrointestinal tract homeostasis was restored, Th1 and Th17 cells were reduced, while GVL was still preserved (103). Importantly, these effects were also observed upon adoptive transfer of ILC2 in mice with established GVHD, suggesting that ILC2 can be used not only to prevent GVHD, but also in a therapeutic setting (103).
In humans, correlations of ILC activation and incidence of GVHD in patients after allo-HSCT suggest that ILC recovery affects the development of GVHD (104). Furthermore, ILC3 are reduced in SR GVHD and can be restored by fecal microbiota transplantation correlating with the response to this experimental treatment (105). A subtle balance between proinflammatory Th17 and cytotoxic T cells on the one side and regulatory T cells and ILC3 on the other side seems to be important for the success of fecal microbiota transplantation (105). Besides their positive effect on tissue damage and restoring homeostasis, a subset of ILC3 expressing the ectoenzymes CD39 and CD73 can directly suppress T cell responses via the production of adenosine (106). This subset of ILC3 is depleted in patients with GVHD (106), which could be another factor contributing to the development of GVHD. As the presence of ILC3 in HCT grafts is associated with a reduced risk for GVHD (107), this cell population could be a candidate for adoptive transfer as GVHD-preventive or -therapeutic strategy.
Taken together, ILC2 and ILC3 positively affect GVHD and, together with other cellular targets, might represent a novel immune cell population to be utilized for therapeutic intervention.
6 Mesenchymal stromal cells
MSCs are fibroblast-like multipotent progenitor cells with immunosuppressive properties in vitro and in vivo (108). Although MSCs have extensively been used in clinical trials for GVHD therapy and have shown some efficacy, some studies did not reach statistical significance. Indeed, overall response rates of MSC for aGVHD range from 30-80% (109). This may be due to the fact that many trial patients had been heavily pre-treated, and due to different application procedures, doses, as well as production protocol (110). Furthermore, the source of MSCs may be critical for the therapeutic efficacy, as pre-clinical in vivo and in vitro studies have shown. Finally, the identification of adequate biomarkers could help to personalize and adapt effective cGVHD therapy or even prevention (111, 112).
6.1 Prophylactic use of MSCs in cGVHD
The prophylactic use of MSCs for cGVHD is summarized in a review by Morata-Tarifa et al. (113). Their meta-analysis confirms that the prophylactic administration of allogeneic bone marrow and umbilical cord MSCs can increase survival of pediatric and adult patients undergoing HSCT by reducing the incidence of cGVHD in 148 treated patients as compared to 236 controls. There was a significant heterogeneity in the overall survival across the individual studies indicating that there must be additional factors determining the survival rate (113). These could include the level of HLA-mismatch between donor and recipient (114), conditioning regimens (115), length and nature of immunosuppression (116, 117), as well as the number of repeated MSC doses (113). These diversifying factors given above may have caused a certain publication bias which can overcome by a standardization in the manufacturing process as well as adequately powered prospective studies. Both may help to confirm efficacy and safety of cGVHD treatment with MSC and thus improve the consistency of future clinical trials (118, 119).
6.2 Treatment of cGVHD with MSC
To our knowledge the first report on treatment of SR cGVHD with MSCs enclosed 19 patients (120). They received a median dose of bone marrow-derived MSCs from healthy donors with 0.6 x 106 cells/kg. 73.3% of the patients responded well with 4 complete and 10 partial remissions whereas 5 patients died from relapse (n=2) and cGVHD-related complications (n=3). No patients experienced adverse events during or immediately after MSC administration. Interestingly, the clinical improvement was accompanied by an increased ratio of CD5+/CD5- B cells and CD28-/CD28+ T cells, respectively, suggesting a shift to the more tolerant immunological phenotype. The authors conclude that MSC administration can be an effective salvage therapy for refractory cGVHD (120).
Recently, Li et al. reviewed and meta-analyzed 6 randomized and 13 non-randomized controlled trials comparing MSC co-transplantation in allo-HSCT with allo-HSCT alone (121). Generally, a co-infusion of MSCs improved engraftment and reduced the risk of cGVHD, notably less pronounced for aGVHD and non-relapse mortality. Specifically, the data obtained support the application of MSCs co-transplanted with HLA-nonidentical HSCT in children and young individuals (121). The data corroborates a previous study demonstrating that MSC therapy led to substantial improvements in terms of complete response and overall survival for cGVHD, with less influence, again, on aGVHD incidence, relapse or death (122).
MSCs may also work in patients with severe refractory cGVHD and still induce durable responses. In a recent study by Boberg et al. 11 patients received repeated infusions of allogeneic bone-marrow-derived MSCs over 6 to 12 months. 6 patients responded to MSC treatment following National Institutes of Health response criteria, accompanied by improvement in GVHD-related symptoms and quality of life. Of note, MSC treatment was associated with significant increases in naïve T cells, B cells, and Tregs 7 days after each infusion. Even prior to treatment responders had higher levels of naïve T and B cells. In addition, CXCL9 and CXCL10 chemokine levels were strongly elevated in responders as compared to non-responders, rendering them as potential new biomarkers of MSC therapy outcome (123).
An alternative mechanism of action how MSCs could benefit patients with cGVHD may be the induction of CD5+ regulatory B cells. In a prospective clinical study 23 refractory cGVHD patients were treated with 3rd party bone marrow MSCs. 20/23 patients had a complete or partial response over a 12-month follow-up period. Clinical improvement was accompanied by a significantly increased number of IL-10-producing CD5+ B cells. Importantly, CD5+ B cells from cGVHD patients showed increased IL-10 expression after MSC treatment, which was associated with reduced inflammatory cytokine production by T cells (124).
It is well established that the vascular endothelium plays a pivotal role in the establishment of cGVHD and that endothelial cells can be targets of cGVHD-mediated immune responses (125, 126). There is in vitro evidence that some of these immune responses are not just allo-, but strictly endothelial-specific and cannot be controlled by the usual mechanisms, such as Tregs (127, 128). Allogeneic MSCs from different sources (bone marrow, umbilical cord, amniotic epithelium) have turned out to be protective against CD8+ endothelial-specific cytotoxic T cells (129), which may be another mechanism of action on how MSCs act.
A great deal of work has been spent on priming of MSCs to ameliorate their immunomodulatory/protective function. For example, pre-clinical data suggest that priming of MSCs with interferon gamma and hypoxia (130) or even with aGVHD and cGVHD-derived plasma (131) can significantly enhance MSC performance. Furthermore, in a humanized mouse model, umbilical cord-derived MSC primed with hypoxia and calcium ions attenuated GVHD significantly better than their naïve counterparts (132). It remains to be elucidated if this approach will also work for clinical cGVHD.
In conclusion, MSCs generally qualify for prophylaxis and/or treatment of refractory cGVHD, but larger randomised controlled trials with repeated doses of cells accompanied by well-designed biomarker studies will be necessary to further advance this therapeutic option.
6.3 MSC-derived extracellular vesicles
Despite their beneficial properties as a cellular therapy for aGVHD, MSCs have demonstrated a lack of correlation between functional improvement and engraftment as well as differentiation at the site of tissue damage or injury. Many hypotheses now center around the belief that it is not the MSCs themselves, but rather their secretome, including extracellular vesicles (EVs) that are driving their therapeutic efficacy. Thus, known as the ‘paracrine hypothesis’. Indeed, co-culture of MSCs with Tconvs in a non-direct transwell system results in Treg induction (133). The potency attainable by MSC may also be inferior to MSC-EV. For instance, MSC suppressed T cell expansion to a lesser extent than MSC-EVs derived from the same number of MSC cells (134). Overall, MSC-EV have been shown to exert an inhibitory effect on T-cell activation and differentiation, as well as reducing T-cell proliferation and IFN-γ release (135). Although the full beneficial use of MSC-EV as a GVHD therapeutic is still being explored, several studies are indicating promising results.
MSCs have been shown to secrete the main subcategories of EV, including microvesicles (136), microparticles (137) and exosomes (138). MSC-derived EVs are enriched in bioactive molecules including lipids, proteins, mRNA, tRNA, lncRNA, microRNA and mitochondrial DNA (139) and have been proposed to interact with heterogeneous cell types as a communication vesicle to maintain a dynamic and homeostatic tissue environment (140) (Figure 3).
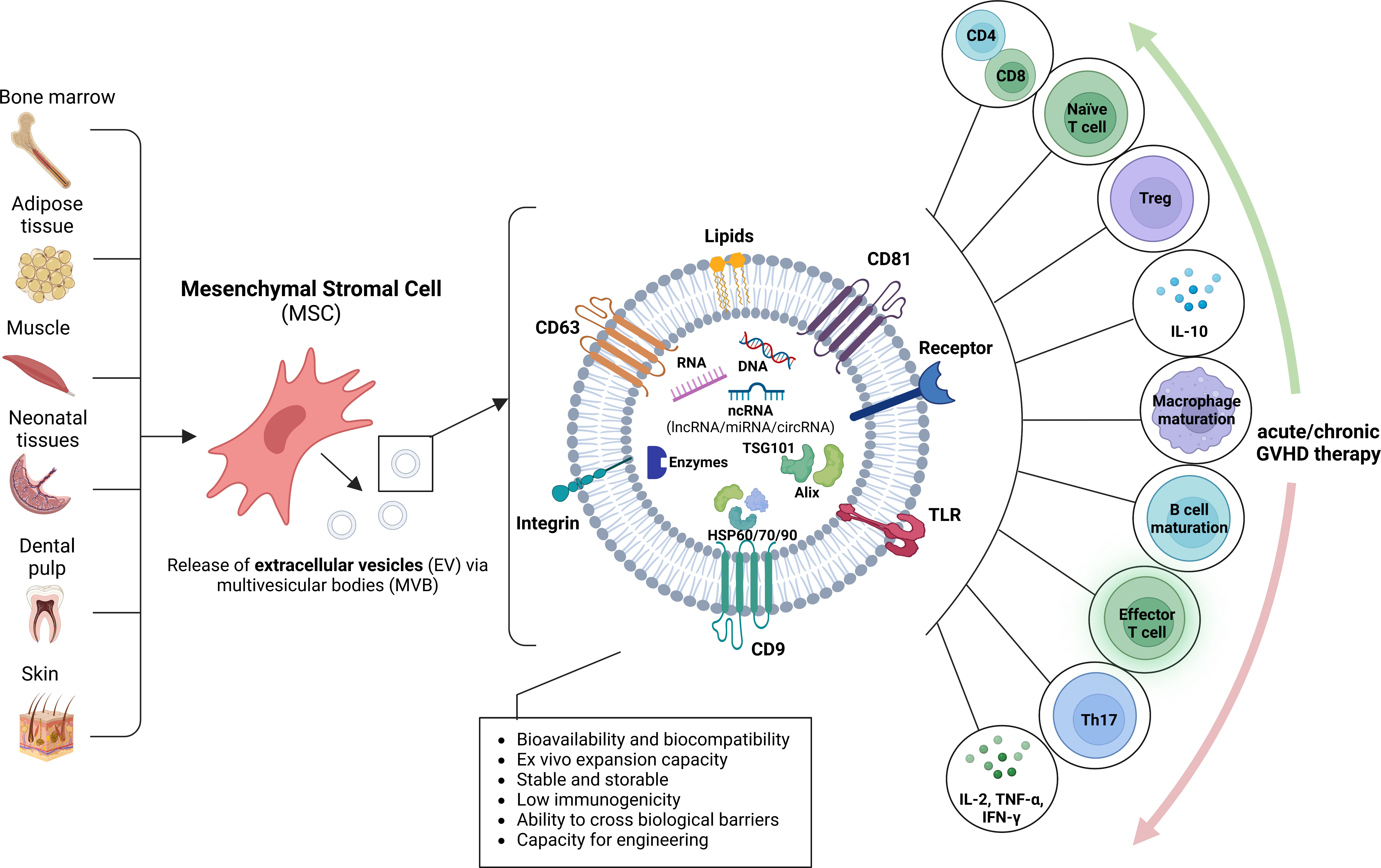
Figure 3 Origin of mesenchymal stromal cell extracellular vesicles and putative role for GVHD. Mesenchymal stromal cells (MSC) may be derived from a variety of sources, including bone marrow, adipose tissue, muscle, neonatal tissues, dental pulp and skin. The MSC release extracellular vesicles (EV) by inward budding of the plasma membrane and formation of intracellular multivesicular bodies (MVB), followed by exocytosis. MSC-derived EVs are enriched in various proteins with multiple functions, such as biogenesis-related proteins (e.g., TSG101, ALIX), common surface markers (e.g., CD9, CD81, CD29, CD44 and CD90), membrane transporter and fusion proteins (e.g., Rab GTPases and annexins), integrins, heat shock proteins (e.g., HSP60, HSP70 and HSP90) and MHC class I and II proteins, DNA, RNA and noncoding RNA (ncRNA), including miRNA, lncRNA, and circRNA. The MSC-EVs may modulate acute or chronic GVHD by acting on diverse immune cells.
MSC-EV demonstrate great potential as therapeutic agents, as they carry a number of advantages over their parental cells. From a practical perspective, MSC-EV can be easily isolated from healthy adult tissues and they have great ex vivo expansion capacity. Their small size and robust membrane makes them easier to handle than cells, and freezing, thawing and storage conditions are less critical (141). Furthermore, as they are produced from cell supernatants and not the cells themselves, large scale production is more feasible (141).
MSC-EV are less likely to trigger an adverse immune response compared to their parental cells, due to their lack of major histocompatibility complex class I/II molecules, which makes them attractive as a safer therapy (142). Indeed, they have proven to be safe in both human and animal models, with no observed side effects (143, 144). As MSC-EV are nano-sized in nature, they can migrate through most physiological barriers, allowing effective concentrations to accumulate in target tissues (145). As EVs are non-viable and non-replicating they may avoid the risk of unregulated cell growth, autoimmune disease and occlusion in the microvasculature (145, 146). Furthermore, the biological activity and functional properties of MSC-EV may be more precisely defined compared to their parental MSCs, as they lack complex metabolic activity and as such, the risk of reprogramming by the environment is reduced (141).
The nano-size of MSC-EV allows for sterilization by filtration. This should effectively minimize the risk of biological contamination, and accordingly, the regulatory requirements for clinical grade production of EVs may not be as restrictive as for cellular therapy (147). Indeed, since MSC-EV from conventional MSC do not contain a nucleus or transgenic product they do not fall into a currently defined advanced therapy medicinal product (ATMP) category. They are therefore exempt from regulations under the European Medicines Agency (EMA), and guidelines for their standardized production and quality assurance are not yet defined.
However, the use of MSC-EV comes with some caveats. To date, recommendations concerning the production and application of EV-based therapies have been advised by the International Society for Extracellular Vesicles (ISEV), but these are simply guidelines and not currently regulated (148). Although MSC-EVs possess potent immunomodulatory properties, their immunosuppressive capacity is not constitutive. In addition, there is no standardization surrounding the optimal protocols for isolation of MSC-EV and identifying or characterizing MSC-EV phenotypes (145). Much work is required between researchers, clinicians and the regulatory authorities in order to stand arise all aspects relating to production of EV-based therapeutics prior to routine clinical application, including but not limited to the source of the starting material, EV isolation and storage methods, quality control aspects and in vivo analyses/EV application, as summarized in an eloquent position paper by the International Society for Extracellular Vesicles (ISEV) (141).
6.4 MSC-EVs for treatment of cGvHD
MSC-EVs are immunologically active and induce elevated expression of anti-inflammatory IL10 and TGFβ1, and reduced levels of pro-inflammatory IL1β, IL6, TNFA and IL12P40. Furthermore, they can induce Tregs both in vitro and in vivo, and MSC-EV infusion has been shown to enhance the survival of allogeneic skin grafts in mice (149). Studies by Zhang et al. demonstrated that the immunosuppressive activities of MSC-EVs are mediated in part by activation of MYD88-dependant signaling in monocytes to induce an anti-inflammatory M2-like phenotype via a TLR-dependent signaling pathway (149). Activated monocytes then polarize activated Tconvs to Tregs, inducing Treg expansion and an attenuated activated immune system (149).
MSC-EVs have been shown to replicate the therapeutic effects of MSC cells in models of acute lung injury, skin wounds and myocardial ischemia (150, 151). Although studies relating to the therapeutic effect of MSC-EV in cGVHD are in their infancy, MSC-EV have been demonstrated as a promising therapeutic tool for treating pulmonary complications of cGVHD (145) and exhibit potent suppression of autoreactive T cell activation and migration, which is essential in the pathogenesis of cGVHD (145).
In their seminal 2014 study, Kordelas et al. administered MSC derived EVs to a SR GVHD patient, with the hypothesis that MSC-EVs may offer improved outcome compared to MSC therapy (143). The MSC-EV fractions from four unrelated bone marrow donors were tested for their effect on patient derived PBMC cytokine secretion. MSC-EV fraction 1 contained the highest content of anti-inflammatory molecules, and a mixed lymphocyte reaction (MLR) with patient PBMC’s resulted in immunosuppressive action by reducing IL-1β, TNF-α and IFN-ɣ expression (143). Following patient administration, all applications were tolerated well with no detected side effects. During the course of MSC-EV therapy, the patient’s pro-inflammatory cytokine response were reduced (IL-1β, TNF-α and IFN-ɣ) and the clinical symptoms of GVHD improved, including indications of cutaneous and mucosal GVHD, which was stable 4 months following completion of therapy (147).
In a follow up clinical case report, Norooznezhad et al. used human placental MSC (hPMSC)-derived EVs to treat a patient with cutaneous cGVHD that was unresponsive to ECP and high-dose corticosterioids (152). The patient received 4 treatments at a weekly interval, comprising of 1.9-2.6 x 1011 EV particles administered in saline, and treatment was well tolerated with no side-effects observed. 15 days-post treatment, skin hyperpigmentation was reduced and the frequency and severity of ulcers, wounds and keratotic lesions was decreased. Monocyte levels were also significantly reduced from 18% to 5%, and clinical changes were sustained for 5 months of follow up assessment (152). This study further highlights the potential of MSC-EV therapy for cGVHD and strengthens the need for follow up larger scale trials, as well as investigation of the impact of MSC source for EV harvesting.
In mouse model pre-clinical studies to assess mechanisms of MSC-EV action, Lai et al. employed a cGVHD mouse model and tail vein injected bone marrow derived MSC-EV on day 22 following BMT (145). The EV injections were administered once per week for 6 weeks and test mice were compared to control mice injected with human dermal fibroblast EVs, or blank control mice injected with equal volumes of PBS (145). They observed that MSC-EV treatment effectively prolonged the survival of cGVHD mice and diminished the clinical and pathological cGVHD scores (145). The activation and lung infiltration of CD4+ T cells was reduced, and skin, lung and liver fibrosis was ameliorated (145). The potent immunomodulatory effects observed were shown to be via inhibition of IL-17-expressing pathogenic T cells and induction of IL-10 regulatory cells (145). By 35 days post-BMT, the MSC-EV mice demonstrated few clinical GVHD features, low disease and skin scores of cGVHD pathology and significantly improved survival compared to control mice, which demonstrated characteristic clinical signs of cGVHD, suggesting MSC-EV to have an inhibitory effect on cGVHD. The activation and infiltration of CD4+ T cells in target organs was also inhibited, and CCR6 expression was reduced, which normally functions to recruit Th17 cells (145). Reduced Th17 frequency and upregulated IL-10 and FOXP3 was also observed in the splenocytes and lymph nodes of MSC-EV treated mice (145). Lai et al. also assessed the effect of MSC-EV on pro-inflammatory cytokine production and observed significantly lower levels of IL-17A, IL-22 and IL-21 and IL-2 in MSC-EV treated mice, while IL-10 levels were increased 2-fold (145). Importantly, the effect of MSC-EV on human PBMCs was tested in vitro. The authors showed that MSC-EV are taken up by CD3 cells and upregulate the percentage of CD25+Foxp3+CD4+ Treg in PBMCs from normal donors. When the same PBMCs were cultured under Th17 conditions, MSC-EV suppressed Th17 differentiation. In PBMCs from patients with active GVHD, IL-17 expressing CD4+ T cells were reduced and IL-10 production was increased upon MSC-EV treatment. Finally, MSC-EV suppressed expression of RORɣt and Stat3, while upregulating production of Foxp3 (145). These important findings indicate that MSC-EV’s therapeutic action in cGVHD may be attributed to expansion of Tregs while inhibiting pro-inflammatory Th17 cells, suppressing migration and infiltration of CD4+ T cells into target organs. The MSC-EV also exert immunosuppressive effects on cytokine production (145). However, despite these informative results, the group did not explore the molecular cargo of the MSC-EV in order to fully elucidate their immunomodulatory effects in the mouse model of cGVHD studied, or the Th17/Treg differentiation modulation observed.
In a further pre-clinical study, Fujii et al. also investigated the mechanisms by which MSC-EV may ameliorate GVHD-associated complications, based on an acute model of disease (134). They used MSC-EVs from the bone marrow of healthy volunteers and assessed the effect of EVs on functional T cell subsets in vitro. In the presence of MSC-EVs, analysis of peripheral blood T cell revealed suppression of CD8+ T cell expansion, a decreased frequency of effector T cells and increased frequency and number of naïve T cells, suggesting an overall suppression of functional differentiation of T cells from a naïve to effector phenotype (134). MSC-EV suppressed T cell expansion, while B cell, NK cell and mature myeloid cell populations were not affected. The MSC-EV treated mice demonstrated prolonged survival, and mitigated GVHD-associated pathology in the skin and large bowel. Interestingly, authors performed some limited assessment of the MSC-EV cargo and microarray analysis identified 336 microRNAs that were upregulated and 337 microRNAs that were downregulated compared to normal human dermal fibroblast EVs (NHDF-EV), including miR-125a-3p which was the most highly upregulated microRNA in MSC-EV (134). GO enrichment analysis revealed the most highly up and down-regulated microRNAs in MSC-EVs were predicted to target proliferation-related genes, while KEGG pathway analysis revealed genes with a role in cell cycle regulation, T cell receptor signalling and GVHD.
In a pre-clinical model to investigate prevention of GVHD, Zhang et al. showed that MSC-EVs can replicate the paracrine potency of MSCs in generating Tregs, in a capacity mediated by antigen presenting cells (146). This was corroborated in a chimeric hu-SCID mouse of GVHD, whereby GVHD symptoms and survival were improved by MSC-EV treatment, with surviving mice demonstrating higher Treg levels in both the blood and spleen (146). Wang et al. demonstrated that human umbilical cord-derived EVs (hUC-MSC-EVs) had the capacity to act prophylactically against aGVHD following intravenous administration to a mouse model of allo-HSCT on days 0 and 7 post-HSCT (153). Indeed, treated mice showed significantly lower frequencies and absolute numbers of CD8+ T cells, reduced serum IL-2, TNF-α and IFN-γ levels, a higher ratio of CD4+ and CD8+ T cells and higher serum IL-10 levels, strengthening the prophylactic use of MSC-EV in order to modulate immune responses. Overall, the severity of GVHD manifestations were reduced at day 28 post-HSCT, with reduced weight loss, improved GVHD score, prolonged survival and reduced histology scores for GVHD-associated changes (153).
Despite these numerous reports highlighting the beneficial potential of MSC-EV for GVHD prevention and treatment, as well as their role in informing on the pathophysiology of GVHD, use of MSC-EV prevents several caveats. Indeed, the field of EVs is still in its infancy, for which several Task Force groups within the International Society for Extracellular Vesicles are working to address fundamental issues supporting their use. Many of these issues are comprehensively described elsewhere (141, 148, 154, 155) and are beyond the scope of this review, but when considering MSC-EV for therapy, special attention should be given to factors such as MSC source (e.g bone marrow, adipose tissue, synovial membrane, umbilical cord), EV production (eg. culture system, medium composition, cell-adherence support, bioreactors, stimulation), EV isolation (e.g. centrifugation techniques, size-based fractionation, ultrafiltration), quality controls, EV dosage and storage, and stability. Thus, the heterogeneity of MSCs used for EV production as well as of the isolated EVs requires extensive further consideration and will be the focus of researchers, clinicians and regulatory authorities prior to any approved industrial or clinical use of MSC-EV.
7 Safety aspects associated with cellular therapy
Cell and gene therapy ATMPs are extensively regulated by a number of regulatory bodies world-wide. These include the US Food and Drug Administration (FDA) and EMA. The FDA and EMA both interact via the International Council for Harmonisation of Technical Requirements for Pharmaceuticals for Human Use (ICH) with the aim to ensure adequate guidelines and procedures for the development of advanced investigational medicinal products for clinical trial use. Apart from the manufacturing safety considerations, which include batch consistency, detection of impurities, specificity and purity of the product, specific safety aspects associated with each type of ATMP needs to be considered.
Within this review we have discussed T cells, NK cells, iNKT cells, ILCs, and mesenchymal cells. Although some of these cellular therapies have very good safety indications, such as Tregs, anti-viral T cell products, NK cells, and MSC-EV, other therapies such as CAR-T cells have been associated with bystander effects such as cytokine release syndrome, graft versus host disease (if an allogeneic product) and neurotoxicity. Consideration therefore needs to be given to the development of novel human based in vitro models to predict and understand some of these safety issues prior to clinical trial development.
The current guidelines from EMA, FDA and ICH detail safety requirements for clinical trials based on that ensuring patient safety and clear risk assessment strategies are well documented1. Although immunogenicity risks are clearly defined for pharmaceuticals, the types of in vitro testing requirements are less clear within the guidelines and not specified especially with regard to cellular therapies. There is a current consensus that in vitro testing using human cells or tissues, if validated and have clear indications of predicting clinical outcome, should be used in preference to animal model experimentation. This has been further advocated by the passing of the FDA Modernization Act of 2021 in June 2022 in the US House2, ending the outdated mandate that all drugs must be tested on animals for registration dossiers. More research is needed, for example to develop assays for the more complex toxicities, such as neurotoxicity which will need collaborations between both and commercial and academic groups in order to achieve safe and effective ATMP development in the future.
Author contributions
MD, RC, OP, AA, AD, GS, JL, GE, and MI planned contents and wrote the manuscript. All authors contributed to the article and approved the submitted version.
Funding
This work was supported by COST (European Cooperation in Science and Technology). www.cost.eu - COST Action 17138 EUROGRAFT.
Conflict of interest
AD was employed by Alcyomics Ltd.
The remaining authors declare that the research was conducted in the absence of any commercial or financial relationships that could be construed as a potential conflict of interest.
Publisher’s note
All claims expressed in this article are solely those of the authors and do not necessarily represent those of their affiliated organizations, or those of the publisher, the editors and the reviewers. Any product that may be evaluated in this article, or claim that may be made by its manufacturer, is not guaranteed or endorsed by the publisher.
Footnotes
- ^ https://www.ema.europa.eu/en/documents/scientific-guideline/guideline-strategies-identify-mitigate-risks-first-human-early-clinical-trials-investigational_en.pdf.
- ^ https://www.congress.gov/117/bills/hr2565/BILLS-117hr2565ih.xml.
References
1. Penack O, Marchetti M, Ruutu T, Aljurf M, Bacigalupo A, Bonifazi F, et al. Prophylaxis and management of graft versus host disease after stem-cell transplantation for haematological malignancies: updated consensus recommendations of the European society for blood and marrow transplantation. Lancet Haematology (2020) 7:e157–67. doi: 10.1016/S2352-3026(19)30256-X
2. Velickovic VM, McIlwaine E, Zhang R, Spelman T. Adverse events in second-and third-line treatments for acute and chronic graft-versus-host disease: Systematic review. Ther Adv Hematol (2020) 11:2040620720977039. doi: 10.1177/2040620720977039
3. Voermans C, Hazenberg MD. Cellular therapies for graft-versus-host disease: a tale of tissue repair and tolerance. Blood (2020) 136:410–7. doi: 10.1182/blood.2019000951
4. Edinger M, Hoffmann P, Ermann J, Drago K, Fathman CG, Strober S, et al. CD4+ CD25+ regulatory T cells preserve graft-versus-tumor activity while inhibiting graft-versus-host disease after bone marrow transplantation. Nat Med (2003) 9:1144–50. doi: 10.1038/nm915
5. Di Ianni M, Falzetti F, Carotti A, Terenzi A, Castellino F, Bonifacio E, et al. Tregs prevent GVHD and promote immune reconstitution in HLA-haploidentical transplantation. Blood J Am Soc Hematol (2011) 117:3921–8. doi: 10.1182/blood-2010-10-311894
6. Shrestha B, Walton K, Reff J, Sagatys EM, Tu N, Boucher J, et al. Human CD83-targeted chimeric antigen receptor T cells prevent and treat graft-versus-host disease. J Clin Invest (2020) 130:4652–62. doi: 10.1172/JCI135754
7. Omar SZ, Blom B, Hazenberg MD. Innate lymphoid cells in treatment-induced gastrointestinal pathogenesis. Curr Opin Supportive Palliative Care (2020) 14:135–41. doi: 10.1097/SPC.0000000000000499
8. Sweeney C, Vyas P. The graft-versus-leukemia effect in AML. Front Oncol (2019) 9:1217. doi: 10.3389/fonc.2019.01217
9. Divito SJ, Aasebø AT, Matos TR, Hsieh P-C, Collin M, Elco CP, et al. Peripheral host T cells survive hematopoietic stem cell transplantation and promote graft-versus-host disease. J Clin Invest (2020) 130:4624–36. doi: 10.1172/JCI129965
10. Ichiki Y, Bowlus CL, Shimoda S, Ishibashi H, Vierling JM, Gershwin ME. T Cell immunity and graft-versus-host disease (GVHD). Autoimmun Rev (2006) 5:1–9. doi: 10.1016/j.autrev.2005.02.006
11. Saidu NEB, Bonini C, Dickinson A, Grce M, Inngjerdingen M, Koehl U, et al. New approaches for the treatment of chronic graft-versus-host disease: current status and future directions. Front Immunol (2020) 11:578314. doi: 10.3389/fimmu.2020.578314
12. Pujanandez L. Tackling T cells in GVHD. Science (2017) 357:1250–2. doi: 10.1126/science.357.6357.1250-r
13. Smith M, Zakrzewski J, James S, Sadelain M. Posttransplant chimeric antigen receptor therapy. Blood J Am Soc Hematol (2018) 131:1045–52. doi: 10.1182/blood-2017-08-752121
14. Biernacki MA, Sheth VS, Bleakley M. T Cell optimization for graft-versus-leukemia responses. JCI Insight (2020) 5(9):e134939. doi: 10.1172/jci.insight.134939
15. Putnam A, Safinia N, Medvec A, Laszkowska M, Wray M, Mintz M, et al. Clinical grade manufacturing of human alloantigen-reactive regulatory T cells for use in transplantation. Am J Transplant (2013) 13:3010–20. doi: 10.1111/ajt.12433
16. Guedan S, Calderon H, Posey AD Jr., Maus MV. Engineering and design of chimeric antigen receptors. Mol Therapy-Methods Clin Dev (2019) 12:145–56. doi: 10.1016/j.omtm.2018.12.009
17. Feins S, Kong W, Williams EF, Milone MC, Fraietta JA. An introduction to chimeric antigen receptor (CAR) T-cell immunotherapy for human cancer. Am J Hematol (2019) 94:S3–9. doi: 10.1002/ajh.25418
18. Miliotou AN, Papadopoulou LC. CAR T-cell therapy: a new era in cancer immunotherapy. Curr Pharm Biotechnol (2018) 19:5–18. doi: 10.2174/1389201019666180418095526
19. Edinger M. Driving allotolerance: CAR-expressing tregs for tolerance induction in organ and stem cell transplantation. J Clin Invest (2016) 126:1248–50. doi: 10.1172/JCI86827
20. Rhoades R, Gaballa S. The role of b cell targeting in chronic graft-versus-host disease. Biomedicines (2017) 5:61. doi: 10.3390/biomedicines5040061
21. McManigle W, Youssef A, Sarantopoulos S. B cells in chronic graft-versus-host disease. Hum Immunol (2019) 80:393–9. doi: 10.1016/j.humimm.2019.03.003
22. Zeiser R, Sarantopoulos S, Blazar BR. B-cell targeting in chronic graft-versus-host disease. Blood J Am Soc Hematol (2018) 131:1399–405. doi: 10.1182/blood-2017-11-784017
23. Kansal R, Richardson N, Neeli I, Khawaja S, Chamberlain D, Ghani M, et al. Sustained B cell depletion by CD19-targeted CAR T cells is a highly effective treatment for murine lupus. Sci Trans Med (2019) 11:eaav1648. doi: 10.1126/scitranslmed.aav1648
24. Quaranta S, Shulman H, Ahmed A, Shoenfeld Y, Peter J, McDonald G, et al. Autoantibodies in human chronic graft-versus-host disease after hematopoietic cell transplantation. Clin Immunol (1999) 91:106–16. doi: 10.1006/clim.1998.4666
25. Patriarca F, Skert C, Sperotto A, Zaja F, Falleti E, Mestroni R, et al. The development of autoantibodies after allogeneic stem cell transplantation is related with chronic graft-vs-host disease and immune recovery. Exp Hematol (2006) 34:389–96. doi: 10.1016/j.exphem.2005.12.011
26. Srinivasan M, Flynn R, Price A, Ranger A, Browning JL, Taylor PA, et al. Donor b-cell alloantibody deposition and germinal center formation are required for the development of murine chronic GVHD and bronchiolitis obliterans. Blood J Am Soc Hematol (2012) 119:1570–80. doi: 10.1182/blood-2011-07-364414
27. Munshi NC, Anderson LD Jr., Shah N, Madduri D, Berdeja J, Lonial S, et al. Idecabtagene vicleucel in relapsed and refractory multiple myeloma. New Engl J Med (2021) 384:705–16. doi: 10.1056/NEJMoa2024850
28. Ellebrecht CT, Bhoj VG, Nace A, Choi EJ, Mao X, Cho MJ, et al. Reengineering chimeric antigen receptor T cells for targeted therapy of autoimmune disease. Science (2016) 353:179–84. doi: 10.1126/science.aaf6756
29. Gomes-Silva D, Srinivasan M, Sharma S, Lee CM, Wagner DL, Davis TH, et al. CD7-edited T cells expressing a CD7-specific CAR for the therapy of T-cell malignancies. Blood J Am Soc Hematol (2017) 130:285–96. doi: 10.1182/blood-2017-01-761320
30. Du J, Paz K, Flynn R, Vulic A, Robinson TM, Lineburg KE, et al. Pirfenidone ameliorates murine chronic GVHD through inhibition of macrophage infiltration and TGF-β production. Blood J Am Soc Hematol (2017) 129:2570–80. doi: 10.1182/blood-2017-01-758854
31. Ono R, Watanabe T, Kawakami E, Iwasaki M, Tomizawa-Murasawa M, Matsuda M, et al. Co-Activation of macrophages and T cells contribute to chronic GVHD in human IL-6 transgenic humanised mouse model. EBioMedicine (2019) 41:584–96. doi: 10.1016/j.ebiom.2019.02.001
32. Aghajanian H, Kimura T, Rurik JG, Hancock AS, Leibowitz MS, Li L, et al. Targeting cardiac fibrosis with engineered T cells. Nature (2019) 573:430–3. doi: 10.1038/s41586-019-1546-z
33. Sakaguchi S, Sakaguchi N, Asano M, Itoh M, Toda M. Immunologic self-tolerance maintained by activated T cells expressing IL-2 receptor alpha-chains (CD25). breakdown of a single mechanism of self-tolerance causes various autoimmune diseases. J Immunol (1995) 155:1151–64.
34. Owen DL, Mahmud SA, Sjaastad LE, Williams JB, Spanier JA, Simeonov DR, et al. Thymic regulatory T cells arise via two distinct developmental programs. Nat Immunol (2019) 20:195–205. doi: 10.1038/s41590-018-0289-6
35. Lathrop SK, Bloom SM, Rao SM, Nutsch K, Lio C-W, Santacruz N, et al. Peripheral education of the immune system by colonic commensal microbiota. Nature (2011) 478:250–4. doi: 10.1038/nature10434
36. Fraser H, Safinia N, Grageda N, Thirkell S, Lowe K, Fry LJ, et al. A rapamycin-based GMP-compatible process for the isolation and expansion of regulatory T cells for clinical trials. Mol Therapy-Methods Clin Dev (2018) 8:198–209. doi: 10.1016/j.omtm.2018.01.006
37. Barzaghi F, Passerini L, Bacchetta R. Immune dysregulation, polyendocrinopathy, enteropathy, x-linked syndrome: a paradigm of immunodeficiency with autoimmunity. Front Immunol (2012) 3:211. doi: 10.3389/fimmu.2012.00211
38. Cooke KR, Luznik L, Sarantopoulos S, Hakim FT, Jagasia M, Fowler DH, et al. The biology of chronic graft-versus-host disease: a task force report from the national institutes of health consensus development project on criteria for clinical trials in chronic graft-versus-Host disease. Biol Blood Marrow Transplant (2017) 23:211–34. doi: 10.1016/j.bbmt.2016.09.023
39. Zorn E, Kim HT, Lee SJ, Floyd BH, Litsa D, Arumugarajah S, et al. Reduced frequency of FOXP3+ CD4+ CD25+ regulatory T cells in patients with chronic graft-versus-host disease. Blood (2005) 106:2903–11. doi: 10.1182/blood-2005-03-1257
40. Matsuoka K-i, Kim HT, McDonough S, Bascug G, Warshauer B, Koreth J, et al. Altered regulatory T cell homeostasis in patients with CD4+ lymphopenia following allogeneic hematopoietic stem cell transplantation. J Clin Invest (2010) 120:1479–93. doi: 10.1172/JCI41072
41. Alho AC, Kim HT, Chammas MJ, Reynolds CG, Matos TR, Forcade E, et al. Unbalanced recovery of regulatory and effector T cells after allogeneic stem cell transplantation contributes to chronic GVHD. Blood J Am Soc Hematol (2016) 127:646–57. doi: 10.1182/blood-2015-10-672345
42. Soares MV, Azevedo RI, Ferreira IA, Bucar S, Ribeiro AC, Vieira A, et al. Naive and stem cell memory T cell subset recovery reveals opposing reconstitution patterns in CD4 and CD8 T cells in chronic graft vs. Host disease Front Immunol (2019) 10:334. doi: 10.3389/fimmu.2019.00334
43. Clark FJ, Gregg R, Piper K, Dunnion D, Freeman L, Griffiths M, et al. Chronic graft-versus-host disease is associated with increased numbers of peripheral blood CD4+ CD25high regulatory T cells. Blood (2004) 103:2410–6. doi: 10.1182/blood-2003-06-2073
44. Koreth J, Matsuoka K-i, Kim HT, McDonough SM, Bindra B, Alyea EP III, et al. Interleukin-2 and regulatory T cells in graft-versus-host disease. New Engl J Med (2011) 365:2055–66. doi: 10.1056/NEJMoa1108188
45. Koreth J, Kim HT, Jones KT, Lange PB, Reynolds CG, Chammas MJ, et al. Efficacy, durability, and response predictors of low-dose interleukin-2 therapy for chronic graft-versus-host disease. Blood J Am Soc Hematol (2016) 128:130–7. doi: 10.1182/blood-2016-02-702852
46. June CH, Blazar BR. Clinical application of expanded CD4+ 25+ cells. Semin Immunol (2006) 18(2):78–88. doi: 10.1016/j.smim.2006.01.006
47. Jones SC, Murphy GF, Korngold R. Post-hematopoietic cell transplantation control of graft-versus-host disease by donor CD4+ 25+ T cells to allow an effective graft-versus-leukemia response. Biol Blood Marrow Transplant (2003) 9:243–56. doi: 10.1053/bbmt.2003.50027
48. Roncarolo M-G, Battaglia M. Regulatory T-cell immunotherapy for tolerance to self antigens and alloantigens in humans. Nat Rev Immunol (2007) 7:585–98. doi: 10.1038/nri2138
49. Hippen KL, Merkel SC, Schirm DK, Sieben CM, Sumstad D, Kadidlo DM, et al. Massive ex vivo expansion of human natural regulatory T cells (Tregs) with minimal loss of in vivo functional activity. Sci Trans Med (2011) 3:83ra41–1. doi: 10.1126/scitranslmed.3001809
50. Alvarez-Salazar EK, Cortés-Hernández A, Arteaga-Cruz S, Alberú-Gómez J, Soldevila G. Large-Scale generation of human allospecific induced tregs with functional stability for use in immunotherapy in transplantation. Front Immunol (2020) 11:375. doi: 10.3389/fimmu.2020.00375
51. Raffin C, Vo LT, Bluestone JA. Treg cell-based therapies: challenges and perspectives. Nat Rev Immunol (2020) 20:158–72. doi: 10.1038/s41577-019-0232-6
52. Allan SE, Alstad AN, Merindol N, Crellin NK, Amendola M, Bacchetta R, et al. Generation of potent and stable human CD4+ T regulatory cells by activation-independent expression of FOXP3. Mol Ther (2008) 16:194–202. doi: 10.1038/sj.mt.6300341
53. Hoffmann P, Boeld TJ, Eder R, Huehn J, Floess S, Wieczorek G, et al. Loss of FOXP3 expression in natural human CD4+ CD25+ regulatory T cells upon repetitive in vitro stimulation. Eur J Immunol (2009) 39:1088–97. doi: 10.1002/eji.200838904
54. Martelli MF, Di Ianni M, Ruggeri L, Falzetti F, Carotti A, Terenzi A, et al. HLA-haploidentical transplantation with regulatory and conventional T-cell adoptive immunotherapy prevents acute leukemia relapse. Blood J Am Soc Hematol (2014) 124:638–44. doi: 10.1182/blood-2014-03-564401
55. Pierini A, Ruggeri L, Carotti A, Falzetti F, Piccinelli S, Saldi S, et al. The “ultimate” haploidentical transplantation for the elderly with high-risk acute myeloid leukemia. Bone Marrow Transplant (2019) 54:803–5. doi: 10.1038/s41409-019-0618-x
56. Brunstein CG, Miller JS, Cao Q, McKenna DH, Hippen KL, Curtsinger J, et al. Infusion of ex vivo expanded T regulatory cells in adults transplanted with umbilical cord blood: safety profile and detection kinetics. Blood J Am Soc Hematol (2011) 117:1061–70. doi: 10.1182/blood-2010-07-293795
57. Brunstein CG, Miller JS, McKenna DH, Hippen KL, DeFor TE, Sumstad D, et al. Umbilical cord blood–derived T regulatory cells to prevent GVHD: kinetics, toxicity profile, and clinical effect. Blood J Am Soc Hematol (2016) 127:1044–51. doi: 10.1182/blood-2015-06-653667
58. Kellner JN, Delemarre EM, Yvon E, Nierkens S, Boelens JJ, McNiece I, et al. Third party, umbilical cord blood derived regulatory T-cells for prevention of graft versus host disease in allogeneic hematopoietic stem cell transplantation: feasibility, safety and immune reconstitution. Oncotarget (2018) 9:35611. doi: 10.18632/oncotarget.26242
59. Meyer EH, Laport G, Xie BJ, MacDonald K, Heydari K, Sahaf B, et al. Transplantation of donor grafts with defined ratio of conventional and regulatory T cells in HLA-matched recipients. JCI Insight (2019) 4(10):e127244. doi: 10.1172/jci.insight.127244
60. Pierini A, Ruggeri L, Carotti A, Falzetti F, Saldi S, Terenzi A, et al. Haploidentical age-adapted myeloablative transplant and regulatory and effector T cells for acute myeloid leukemia. Blood Adv (2021) 5:1199–208. doi: 10.1182/bloodadvances.2020003739
61. Trzonkowski P, Bieniaszewska M, Juścińska J, Dobyszuk A, Krzystyniak A, Marek N, et al. First-in-man clinical results of the treatment of patients with graft versus host disease with human ex vivo expanded CD4+ CD25+ CD127– T regulatory cells. Clin Immunol (2009) 133:22–6. doi: 10.1016/j.clim.2009.06.001
62. Theil A, Tuve S, Oelschlägel U, Maiwald A, Döhler D, Oßmann D, et al. Adoptive transfer of allogeneic regulatory T cells into patients with chronic graft-versus-host disease. Cytotherapy (2015) 17:473–86. doi: 10.1016/j.jcyt.2014.11.005
63. Johnston L, Armstrong R, Baker J, Sahaf B, Otani J, Tate K, et al. A phase I study of donor regulatory T cells as treatment for steroid dependent/refractory chronic graft versus host disease. Blood (2016) 128:385. doi: 10.1182/blood.V128.22.385.385
64. Nikiforow S, Kim HT, Jones KT, Stewart J, Garrity H, Daley H, et al. Phase I trial of regulatory T-cell donor lymphocyte infusion plus daily low-dose interleukin-2 for steroid-refractory chronic graft-versus-host disease. Blood (2017) 130:511. doi: 10.1182/bloodadvances.2021006625
65. Ahmad I, Bijl J, Ivison S, Atenafu EG, Panzarella T, Broady R, et al. Continuous alloreactive T cell depletion and regulatory T cell expansion for the treatment of steroid-refractory or dependent chronic gvhd-a multicentre phase II clinical trial (CARE trial). TCT| Transplantation & Cellular Therapy Meetings of ASTCT and CIBMTR, Tandem Meetings (digital) (2021).
66. Nguyen VH, Zeiser R, DaSilva DL, Chang DS, Beilhack A, Contag CH, et al. In vivo dynamics of regulatory T-cell trafficking and survival predict effective strategies to control graft-versus-host disease following allogeneic transplantation. Blood (2007) 109:2649–56. doi: 10.1182/blood-2006-08-044529
67. MacDonald K, Piret J, Levings M. Methods to manufacture regulatory T cells for cell therapy. Clin Exp Immunol (2019) 197:52–63. doi: 10.1111/cei.13297
68. Hoffmann P, Eder R, Kunz-Schughart LA, Andreesen R, Edinger M. Large-Scale in vitro expansion of polyclonal human CD4+ CD25high regulatory T cells. Blood (2004) 104:895–903. doi: 10.1182/blood-2004-01-0086
69. Parmar S, Liu X, Najjar A, Shah N, Yang H, Yvon E, et al. Ex vivo fucosylation of third-party human regulatory T cells enhances anti–graft-versus-host disease potency in vivo. Blood J Am Soc Hematol (2015) 125:1502–6. doi: 10.1182/blood-2014-10-603449
70. MacDonald KG, Hoeppli RE, Huang Q, Gillies J, Luciani DS, Orban PC, et al. Alloantigen-specific regulatory T cells generated with a chimeric antigen receptor. J Clin Invest (2016) 126:1413–24. doi: 10.1172/JCI82771
71. Pierini A, Iliopoulou BP, Peiris H, Pérez-Cruz M, Baker J, Hsu K, et al. T Cells expressing chimeric antigen receptor promote immune tolerance. JCI Insight (2017) 2(20):e92865. doi: 10.1172/jci.insight.92865
72. Imura Y, Ando M, Kondo T, Ito M, Yoshimura A. CD19-targeted CAR regulatory T cells suppress b cell pathology without GvHD. JCI Insight (2020) 5(14):e136185. doi: 10.1172/jci.insight.136185
73. Bolivar-Wagers S, Larson JH, Jin S, Blazar BR. Cytolytic CD4+ and CD8+ regulatory T-cells and implications for developing immunotherapies to combat graft-versus-host disease. Front Immunol (2022) 13. doi: 10.3389/fimmu.2022.864748
74. Boroughs AC, Larson RC, Choi BD, Bouffard AA, Riley LS, Schiferle E, et al. Chimeric antigen receptor costimulation domains modulate human regulatory T cell function. JCI Insight (2019) 5(8):e126194. doi: 10.1172/jci.insight.126194
75. Koristka S, Kegler A, Bergmann R, Arndt C, Feldmann A, Albert S, et al. Engrafting human regulatory T cells with a flexible modular chimeric antigen receptor technology. J Autoimmun (2018) 90:116–31. doi: 10.1016/j.jaut.2018.02.006
76. Dawson NA, Rosado-Sánchez I, Novakovsky GE, Fung VC, Huang Q, McIver E, et al. Functional effects of chimeric antigen receptor co-receptor signaling domains in human regulatory T cells. Sci Trans Med (2020) 12:eaaz3866. doi: 10.1126/scitranslmed.aaz3866
77. Bolivar-Wagers S, Loschi ML, Jin S, Thangavelu G, Larson JH, McDonald-Hyman CS, et al. Murine CAR19 tregs suppress acute graft-versus-host disease and maintain graft-versus-tumor responses. JCI Insight (2022) 7(17):e160674. doi: 10.1172/jci.insight.160674
78. Abrahamsen IW, Somme S, Heldal D, Egeland T, Kvale D, Tjonnfjord G. Immune reconstitution after allogeneic stem cell transplantation: the impact of stem cell source and graft-versus-host disease. Haematologica (2005) 90:86–93.
79. Acevedo A, Aramburu J, López J, Fernández-Herrera J, Fern JM, López-Botet M. Identification of natural killer (NK) cells in lesions of human cutaneous graft-versus-host disease: expression of a novel NK-associated surface antigen (Kp43) in mononuclear infiltrates. J Invest Dermatol (1991) 97:659–66. doi: 10.1111/1523-1747.ep12483724
80. Bejanyan N, Brunstein CG, Cao Q, Lazaryan A, Luo X, Curtsinger J, et al. Delayed immune reconstitution after allogeneic transplantation increases the risks of mortality and chronic GVHD. Blood Adv (2018) 2:909–22. doi: 10.1182/bloodadvances.2017014464
81. Brahmi Z, Hommel-Berrey G, Smith F, Thomson B. NK cells recover early and mediate cytotoxicity via perforin/granzyme and Fas/FasL pathways in umbilical cord blood recipients. Hum Immunol (2001) 62:782–90. doi: 10.1016/S0198-8859(01)00275-0
82. Gray JD, Hirokawa M, Horwitz DA. The role of transforming growth factor beta in the generation of suppression: an interaction between CD8+ T and NK cells. J Exp Med (1994) 180:1937–42. doi: 10.1084/jem.180.5.1937
83. Boukouaci W, Busson M, Peffault de Latour R, Rocha V, Suberbielle C, Bengoufa D, et al. MICA-129 genotype, soluble MICA, and anti-MICA antibodies as biomarkers of chronic graft-versus-host disease. Blood J Am Soc Hematol (2009) 114:5216–24. doi: 10.1182/blood-2009-04-217430
84. Boukouaci W, Al-Daccak R, Dulphy N, Lauden L, Amokrane K, Fortier C, et al. Soluble MICA-NKG2D interaction upregulates IFN-γ production by activated CD3– CD56+ NK cells: Potential impact on chronic graft versus host disease. Hum Immunol (2013) 74:1536–41. doi: 10.1016/j.humimm.2013.08.281
85. Harigai M, Kawamoto M, Hara M, Kubota T, Kamatani N, Miyasaka N. Excessive production of IFN-γ in patients with systemic lupus erythematosus and its contribution to induction of b lymphocyte stimulator/B cell-activating factor/TNF ligand superfamily-13B. J Immunol (2008) 181:2211–9. doi: 10.4049/jimmunol.181.3.2211
86. Imai C, Iwamoto S, Campana D. Genetic modification of primary natural killer cells overcomes inhibitory signals and induces specific killing of leukemic cells. Blood (2005) 106:376–83. doi: 10.1182/blood-2004-12-4797
87. Chu Y, Hochberg J, Yahr A, Ayello J, van de Ven C, Barth M, et al. Targeting CD20+ aggressive b-cell non–Hodgkin lymphoma by anti-CD20 CAR mRNA-modified expanded natural killer cells in vitro and in NSG mice. Cancer Immunol Res (2015) 3:333–44. doi: 10.1158/2326-6066.CIR-14-0114
88. Müller T, Uherek C, Maki G, Chow KU, Schimpf A, Klingemann H-G, et al. Expression of a CD20-specific chimeric antigen receptor enhances cytotoxic activity of NK cells and overcomes NK-resistance of lymphoma and leukemia cells. Cancer Immunol Immunotherapy (2008) 57:411–23. doi: 10.1007/s00262-007-0383-3
89. Bendelac A, Savage PB, Teyton L. The biology of NKT cells. Annu Rev Immunol (2007) 25:297–336. doi: 10.1146/annurev.immunol.25.022106.141711
90. Beckman EM, Porcelli SA, Morita CT, Behar SM, Furlong ST, Brenner MB. Recognition of a lipid antigen by CD1-restricted αβ+ T cells. Nature (1994) 372:691–4. doi: 10.1038/372691a0
91. Vela-Ojeda J, Esparza G-R, Reyes-Maldonado E, Jimenez-Zamudio L, Garcia-Latorre E, Moreno-Lafont M, et al. Clinical relevance of NK, NKT, and dendritic cell dose in patients receiving G-CSF-mobilized peripheral blood allogeneic stem cell transplantation. Ann Hematol (2006) 85:113–20. doi: 10.1007/s00277-005-0037-5
92. Pillai AB, George TI, Dutt S, Strober S. Host natural killer T cells induce an interleukin-4–dependent expansion of donor CD4+ CD25+ Foxp3+ T regulatory cells that protects against graft-versus-host disease. Blood J Am Soc Hematol (2009) 113:4458–67. doi: 10.1182/blood-2008-06-165506
93. Du J, Paz K, Thangavelu G, Schneidawind D, Baker J, Flynn R, et al. Invariant natural killer T cells ameliorate murine chronic GVHD by expanding donor regulatory T cells. Blood J Am Soc Hematol (2017) 129:3121–5. doi: 10.1182/blood-2016-11-752444
94. Kuwatani M, Ikarashi Y, Iizuka A, Kawakami C, Quinn G, Heike Y, et al. Modulation of acute graft-versus-host disease and chimerism after adoptive transfer of in vitro-expanded invariant Vα14 natural killer T cells. Immunol Lett (2006) 106:82–90. doi: 10.1016/j.imlet.2006.05.001
95. Hazenberg MD, Spits H. Human innate lymphoid cells. Blood J Am Soc Hematol (2014) 124:700–9. doi: 10.1182/blood-2013-11-427781
96. Vivier E, Artis D, Colonna M, Diefenbach A, Di Santo JP, Eberl G, et al. Innate lymphoid cells: 10 years on. Cell (2018) 174:1054–66. doi: 10.1016/j.cell.2018.07.017
97. Fuchs A, Vermi W, Lee JS, Lonardi S, Gilfillan S, Newberry RD, et al. Intraepithelial type 1 innate lymphoid cells are a unique subset of IL-12-and IL-15-responsive IFN-γ-producing cells. Immunity (2013) 38:769–81. doi: 10.1016/j.immuni.2013.02.010
98. Monticelli LA, Sonnenberg GF, Abt MC, Alenghat T, Ziegler CG, Doering TA, et al. Innate lymphoid cells promote lung-tissue homeostasis after infection with influenza virus. Nat Immunol (2011) 12:1045–54. doi: 10.1038/ni.2131
99. Qiu J, Guo X, Zong-ming EC, He L, Sonnenberg GF, Artis D, et al. Group 3 innate lymphoid cells inhibit T-cell-mediated intestinal inflammation through aryl hydrocarbon receptor signaling and regulation of microflora. Immunity (2013) 39:386–99. doi: 10.1016/j.immuni.2013.08.002
101. Moreno DF, Cid J. Graft-versus-host disease. Medicina Clínica (English Edition) (2019) 152:22–8. doi: 10.1016/j.medcle.2018.11.008
102. Hanash AM, Dudakov JA, Hua G, O’Connor MH, Young LF, Singer NV, et al. Interleukin-22 protects intestinal stem cells from immune-mediated tissue damage and regulates sensitivity to graft versus host disease. Immunity (2012) 37:339–50. doi: 10.1016/j.immuni.2012.05.028
103. Bruce DW, Stefanski HE, Vincent BG, Dant TA, Reisdorf S, Bommiasamy H, et al. Type 2 innate lymphoid cells treat and prevent acute gastrointestinal graft-versus-host disease. J Clin Invest (2017) 127:1813–25. doi: 10.1172/JCI91816
104. Ilander MM, Olsson-Strömberg U, Lähteenmäki H, Tiina K, Koskenvesa P, Söderlund S, et al. Early disease relapse after tyrosine kinase inhibitor treatment discontinuation in CML is related both to low number and impaired function of NK-cells. Blood (2014) 124(21):812. doi: 10.1182/blood.V124.21.812.812
105. Spindelboeck W, Halwachs B, Bayer N, Huber-Krassnitzer B, Schulz E, Uhl B, et al. Antibiotic use and ileocolonic immune cells in patients receiving fecal microbiota transplantation for refractory intestinal GvHD: a prospective cohort study. Ther Adv Hematol (2021) 12:20406207211058333. doi: 10.1177/20406207211058333
106. Hazenberg MD, Haverkate NJ, van Lier YF, Spits H, Krabbendam L, Bemelman WA, et al. Human ectoenzyme-expressing ILC3: immunosuppressive innate cells that are depleted in graft-versus-host disease. Blood Adv (2019) 3:3650–60. doi: 10.1182/bloodadvances.2019000176
107. Kroeze A, van Hoeven V, Verheij MW, Turksma AW, Weterings N, van Gassen S, et al. Presence of innate lymphoid cells in allogeneic hematopoietic grafts correlates with reduced graft-versus-host disease. Cytotherapy (2022) 24:302–10. doi: 10.1016/j.jcyt.2021.10.011
108. Liu S, Liu F, Zhou Y, Jin B, Sun Q, Guo S. Immunosuppressive property of MSCs mediated by cell surface receptors. Front Immunol (2020) 11:1076. doi: 10.3389/fimmu.2020.01076
109. Kaipe H, Erkers T, Sadeghi B, Ringden O. Stromal cells–are they really useful for GVHD? Bone Marrow Transplant (2014) 49:737–43. doi: 10.1038/bmt.2013.237
110. Introna M, Golay J. Tolerance to bone marrow transplantation: do mesenchymal stromal cells still have a future for acute or chronic GvHD? Front Immunol (2020) 11:609063. doi: 10.3389/fimmu.2020.609063
111. Giaccone L, Faraci DG, Butera S, Lia G, Di Vito C, Gabrielli G, et al. Biomarkers for acute and chronic graft versus host disease: state of the art. Expert Rev Hematol (2021) 14:79–96. doi: 10.1080/17474086.2021.1860001
112. Lelas A, Greinix HT, Wolff D, Eissner G, Pavletic SZ, Pulanic D. Von Willebrand factor, factor VIII, and other acute phase reactants as biomarkers of inflammation and endothelial dysfunction in chronic graft-Versus-Host disease. Front Immunol (2021) 12:676756. doi: 10.3389/fimmu.2021.676756
113. Morata-Tarifa C, Macías-Sánchez M, Gutiérrez-Pizarraya A, Sanchez-Pernaute R. Mesenchymal stromal cells for the prophylaxis and treatment of graft-versus-host disease–a meta-analysis. Stem Cell Res Ther (2020) 11:1–12. doi: 10.1186/s13287-020-01592-z
114. Arcuri LJ, Aguiar MTM, Ribeiro AAF, Pacheco AGF. Haploidentical transplantation with post-transplant cyclophosphamide versus unrelated donor hematopoietic stem cell transplantation: a systematic review and meta-analysis. Biol Blood Marrow Transplant (2019) 25:2422–30. doi: 10.1016/j.bbmt.2019.07.028
115. Gyurkocza B, Sandmaier BM. Conditioning regimens for hematopoietic cell transplantation: one size does not fit all. Blood J Am Soc Hematol (2014) 124:344–53. doi: 10.1182/blood-2014-02-514778
116. Ram R, Gafter-Gvili A, Yeshurun M, Paul M, Raanani P, Shpilberg O. Prophylaxis regimens for GVHD: Systematic review and meta-analysis. Bone marrow Transplant (2009) 43:643–53. doi: 10.1038/bmt.2008.373
117. Ferrara JL, Levine JE, Reddy P, Holler E. Graft-versus-host disease. Lancet (2009) 373:1550–61. doi: 10.1016/S0140-6736(09)60237-3
118. Kelly K, Rasko JE. Mesenchymal stromal cells for the treatment of graft versus host disease. Front Immunol (2021) 12:4457. doi: 10.3389/fimmu.2021.761616
119. Li Y, Hao J, Hu Z, Yang Y-G, Zhou Q, Sun L, et al. Current status of clinical trials assessing mesenchymal stem cell therapy for graft versus host disease: a systematic review. Stem Cell Res Ther (2022) 13:1–22. doi: 10.1186/s13287-022-02751-0
120. Weng J, Du X, Geng S, Peng Y, Wang Z, Lu Z, et al. Mesenchymal stem cell as salvage treatment for refractory chronic GVHD. Bone marrow Transplant (2010) 45:1732–40. doi: 10.1038/bmt.2010.195
121. Li T, Luo C, Zhang J, Wei L, Sun W, Xie Q, et al. Efficacy and safety of mesenchymal stem cells co-infusion in allogeneic hematopoietic stem cell transplantation: a systematic review and meta-analysis. Stem Cell Res Ther (2021) 12:1–22. doi: 10.1186/s13287-021-02304-x
122. Zhao L, Chen S, Yang P, Cao H, Li L. The role of mesenchymal stem cells in hematopoietic stem cell transplantation: prevention and treatment of graft-versus-host disease. Stem Cell Res Ther (2019) 10:1–13. doi: 10.1186/s13287-019-1287-9
123. Boberg E, Bahr L, Afram G, Lindström C, Ljungman P, Heldring N, et al. Treatment of chronic GvHD with mesenchymal stromal cells induces durable responses: a phase II study. Stem Cells Trans Med (2020) 9:1190–202. doi: 10.1002/sctm.20-0099
124. Peng Y, Chen X, Liu Q, Zhang X, Huang K, Liu L, et al. Mesenchymal stromal cells infusions improve refractory chronic graft versus host disease through an increase of CD5+ regulatory b cells producing interleukin 10. Leukemia (2015) 29:636–46. doi: 10.1038/leu.2014.225
125. Biedermann BC, Sahner S, Gregor M, Tsakiris DA, Jeanneret C, Pober JS, et al. Endothelial injury mediated by cytotoxic T lymphocytes and loss of microvessels in chronic graft versus host disease. Lancet (2002) 359:2078–83. doi: 10.1016/S0140-6736(02)08907-9
126. Tichelli A, Gratwohl A. Vascular endothelium as ‘novel’target of graft-versus-host disease. Best Pract Res Clin haematology (2008) 21:139–48. doi: 10.1016/j.beha.2008.02.002
127. Biedermann BC, Pober JS. Human vascular endothelial cells favor clonal expansion of unusual alloreactive CTL. J Immunol (1999) 162:7022–30.
128. Eissner G, Hartmann I, Kesikli A, Haffner S, Sax T, Holler E, et al. Regulatory T cells enhance the allogeneic activity of endothelial-specific cytotoxic T lymphocytes–protective effect of defibrotide. Blood (2011) 118:4693. doi: 10.1182/blood.V118.21.4693.4693
129. Cahill EF, Sax T, Hartmann I, Haffner S, Holler E, Holler B, et al. Mesenchymal stromal cells protect endothelial cells from cytotoxic T lymphocyte-induced lysis. Scandinavian J Immunol (2016) 84:158–64. doi: 10.1111/sji.12459
130. Wobma HM, Kanai M, Ma SP, Shih Y, Li HW, Duran-Struuck R, et al. Dual IFN-γ/hypoxia priming enhances immunosuppression of mesenchymal stromal cells through regulatory proteins and metabolic mechanisms. J Immunol Regenerative Med (2018) 1:45–56. doi: 10.1016/j.regen.2018.01.001
131. Silva-Carvalho AÉ, Rodrigues LP, Schiavinato JL, Alborghetti MR, Bettarello G, Simões BP. GVHD-derived plasma as a priming strategy of mesenchymal stem cells. Stem Cell Res Ther (2020) 11:1–12. doi: 10.1186/s13287-020-01659-x
132. Kim Y, Jin HJ, Heo J, Ju H, Lee H-Y, Kim S, et al. Small hypoxia-primed mesenchymal stem cells attenuate graft-versus-host disease. Leukemia (2018) 32:2672–84. doi: 10.1038/s41375-018-0151-8
133. Tasso R, Ilengo C, Quarto R, Cancedda R, Caspi RR, Pennesi G. Mesenchymal stem cells induce functionally active T-regulatory lymphocytes in a paracrine fashion and ameliorate experimental autoimmune uveitis. Invest Ophthalmol Visual Sci (2012) 53:786–93. doi: 10.1167/iovs.11-8211
134. Fujii S, Miura Y, Fujishiro A, Shindo T, Shimazu Y, Hirai H, et al. Graft-versus-host disease amelioration by human bone marrow mesenchymal stromal/stem cell-derived extracellular vesicles is associated with peripheral preservation of naive T cell populations. Stem Cells (2018) 36:434–45. doi: 10.1002/stem.2759
135. Blazquez R, Sanchez-Margallo FM, de la Rosa O, Dalemans W, Álvarez V, Tarazona R, et al. Immunomodulatory potential of human adipose mesenchymal stem cells derived exosomes on in vitro stimulated T cells. Front Immunol (2014) 5:556. doi: 10.3389/fimmu.2014.00556
136. Bruno S, Grange C, Deregibus MC, Calogero RA, Saviozzi S, Collino F, et al. Mesenchymal stem cell-derived microvesicles protect against acute tubular injury. J Am Soc Nephrol (2009) 20:1053–67. doi: 10.1681/ASN.2008070798
137. Kim SJ, Moon GJ, Cho YH, Kang HY, Hyung NK, Kim D, et al. Circulating mesenchymal stem cells microparticles in patients with cerebrovascular disease. PloS One (2012) 7:e37036. doi: 10.1371/journal.pone.0037036
138. Lai RC, Arslan F, Tan SS, Tan B, Choo A, Lee MM, et al. Derivation and characterization of human fetal MSCs: an alternative cell source for large-scale production of cardioprotective microparticles. J Mol Cell Cardiol (2010) 48:1215–24. doi: 10.1016/j.yjmcc.2009.12.021
139. Keerthikumar S, Chisanga D, Ariyaratne D, Al Saffar H, Anand S, Zhao K, et al. ExoCarta: a web-based compendium of exosomal cargo. J Mol Biol (2016) 428:688–92. doi: 10.1016/j.jmb.2015.09.019
140. Lai RC, Yeo RWY, Lim SK. Mesenchymal stem cell exosomes. Semin Cell Dev Biol (2015) 40:82–8. Elsevier. doi: 10.1016/j.semcdb.2015.03.001
141. Lener T, Gimona M, Aigner L, Börger V, Buzas E, Camussi G, et al. Applying extracellular vesicles based therapeutics in clinical trials–an ISEV position paper. J Extracellular Vesicles (2015) 4:30087. doi: 10.3402/jev.v4.30087
142. You L, Mao L, Wei J, Jin S, Yang C, Liu H, et al. The crosstalk between autophagic and endo-/exosomal pathways in antigen processing for MHC presentation in anticancer T cell immune responses. J Hematol Oncol (2017) 10:1–9. doi: 10.1186/s13045-017-0534-8
143. Kordelas L, Rebmann V, Ludwig A, Radtke S, Ruesing J, Doeppner T, et al. MSC-derived exosomes: a novel tool to treat therapy-refractory graft-versus-host disease. Leukemia (2014) 28:970–3. doi: 10.1038/leu.2014.41
144. Börger V, Bremer M, Ferrer-Tur R, Gockeln L, Stambouli O, Becic A, et al. Mesenchymal stem/stromal cell-derived extracellular vesicles and their potential as novel immunomodulatory therapeutic agents. Int J Mol Sci (2017) 18:1450. doi: 10.3390/ijms18071450
145. Lai P, Chen X, Guo L, Wang Y, Liu X, Liu Y, et al. A potent immunomodulatory role of exosomes derived from mesenchymal stromal cells in preventing cGVHD. J Hematol Oncol (2018) 11:1–15. doi: 10.1186/s13045-018-0680-7
146. Zhang B, Yeo RWY, Lai RC, Sim EWK, Chin KC, Lim SK. Mesenchymal stromal cell exosome–enhanced regulatory T-cell production through an antigen-presenting cell–mediated pathway. Cytotherapy (2018) 20:687–96. doi: 10.1016/j.jcyt.2018.02.372
147. Giebel B, Kordelas L, Börger V. Clinical potential of mesenchymal stem/stromal cell-derived extracellular vesicles. Stem Cell Invest (2017) 4:84. doi: 10.21037/sci.2017.09.06
148. Théry C, Witwer KW, Aikawa E, Alcaraz MJ, Anderson JD, Andriantsitohaina R, et al. Minimal information for studies of extracellular vesicles 2018 (MISEV2018): A position statement of the international society for extracellular vesicles and update of the MISEV2014 guidelines. J Extracellular Vesicles (2018) 7:1535750. doi: 10.1080/20013078.2018.1535750
149. Zhang B, Yin Y, Lai RC, Tan SS, Choo ABH, Lim SK. Mesenchymal stem cells secrete immunologically active exosomes. Stem Cells Dev (2014) 23:1233–44. doi: 10.1089/scd.2013.0479
150. Lai RC, Chen TS, Lim SK. Mesenchymal stem cell exosome: a novel stem cell-based therapy for cardiovascular disease. Regenerative Med (2011) 6:481–92. doi: 10.2217/rme.11.35
151. Lai RC, Arslan F, Lee MM, Sze NSK, Choo A, Chen TS, et al. Exosome secreted by MSC reduces myocardial ischemia/reperfusion injury. Stem Cell Res (2010) 4:214–22. doi: 10.1016/j.scr.2009.12.003
152. Norooznezhad AH, Yarani R, Payandeh M, Hoseinkhani Z, Kiani S, Taghizadeh E, et al. Human placental mesenchymal stromal cell-derived exosome-enriched extracellular vesicles for chronic cutaneous graft-versus-host disease: A case report. J Cell Mol Med (2022) 26:588. doi: 10.1111/jcmm.17114
153. Wang L, Gu Z, Zhao X, Yang N, Wang F, Deng A, et al. Extracellular vesicles released from human umbilical cord-derived mesenchymal stromal cells prevent life-threatening acute graft-versus-host disease in a mouse model of allogeneic hematopoietic stem cell transplantation. Stem Cells Dev (2016) 25:1874–83. doi: 10.1089/scd.2016.0107
154. Reiner AT, Witwer KW, Van Balkom BW, De Beer J, Brodie C, Corteling RL, et al. Concise review: developing best-practice models for the therapeutic use of extracellular vesicles. Stem Cells Trans Med (2017) 6:1730–9. doi: 10.1002/sctm.17-0055
Keywords: chronic GVHD, Tregs, CAR, NK cells, ILCs, MSCs, extracellular vesicles
Citation: Doglio M, Crossland RE, Alho AC, Penack O, Dickinson AM, Stary G, Lacerda JF, Eissner G and Inngjerdingen M (2022) Cell-based therapy in prophylaxis and treatment of chronic graft-versus-host disease. Front. Immunol. 13:1045168. doi: 10.3389/fimmu.2022.1045168
Received: 15 September 2022; Accepted: 31 October 2022;
Published: 17 November 2022.
Edited by:
Tomomi Toubai, Yamagata University, JapanReviewed by:
Ivan Maillard, University of Pennsylvania, United StatesMauro Di Ianni, University of Studies G. d’Annunzio Chieti and Pescara, Italy
Daniel Peltier, Michigan Medicine, University of Michigan, United States
Copyright © 2022 Doglio, Crossland, Alho, Penack, Dickinson, Stary, Lacerda, Eissner and Inngjerdingen. This is an open-access article distributed under the terms of the Creative Commons Attribution License (CC BY). The use, distribution or reproduction in other forums is permitted, provided the original author(s) and the copyright owner(s) are credited and that the original publication in this journal is cited, in accordance with accepted academic practice. No use, distribution or reproduction is permitted which does not comply with these terms.
*Correspondence: Marit Inngjerdingen, bWFyaXRpQG1lZGlzaW4udWlvLm5v