- 1Division of Pharmaceutics, Utrecht Institute for Pharmaceutical Sciences, Utrecht University, Utrecht, Netherlands
- 2Division of Pharmacology, Utrecht Institute for Pharmaceutical Sciences, Utrecht University, Utrecht, Netherlands
- 3Department of Immunology, Nutricia Research B.V., Utrecht, Netherlands
Cow’s milk allergy is a common food allergy among infants. Improved hygiene conditions and loss of microbial diversity are associated with increased risk of allergy development. The intestinal immune system is essential for oral tolerance induction. In this respect, bacterial CpG DNA is known to drive Th1 and regulatory T-cell (Treg) development via Toll-Like-Receptor 9 (TLR-9) signaling, skewing away from the allergic Th2 phenotype. We aimed to induce allergen specific tolerance via oral delivery of poly (lactic-co-glycolic acid) nanoparticles (NP) co-encapsulated with a selected β-lactoglobulin derived peptide (BLG-Pep) and TLR-9 ligand CpG oligodeoxynucleotide (CpG). In vivo, 3-4-week-old female C3H/HeOuJ mice housed in individually ventilated cages received 6-consecutive-daily gavages of either PBS, whey, BLG-Pep/NP, CpG/NP, a mixture of BLG-Pep/NP plus CpG/NP or co-encapsulated BLG-Pep+CpG/NP, before 5-weekly oral sensitizations with whey plus cholera toxin (CT) or only CT (sham) and were challenged with whey 5 days after the last sensitization. The co-encapsulated BLG-Pep+CpG/NP pretreatment, but not BLG-Pep/NP, CpG/NP or the mixture of BLG-Pep/NP plus CpG/NP, prevented the whey-induced allergic skin reactivity and prevented rise in serum BLG-specific IgE compared to whey-sensitized mice. Importantly, co-encapsulated BLG-Pep+CpG/NP pretreatment reduced dendritic cell (DC) activation and lowered the frequencies of PD-L1+ DC in the mesenteric lymph nodes compared to whey-sensitized mice. By contrast, co-encapsulated BLG-Pep+CpG/NP pretreatment increased the frequency of splenic PD-L1+ DC compared to the BLG-Pep/NP plus CpG/NP recipients, in association with lower Th2 development and increased Treg/Th2 and Th1/Th2 ratios in the spleen. Oral administration of PLGA NP co-encapsulated with BLG-Pep and CpG prevented rise in serum BLG-specific IgE and symptom development while lowering splenic Th2 cell frequency in these mice which were kept under strict hygienic conditions.
Introduction
Cow’s milk allergy (CMA) is one of the most common food allergies occurring early in life, with an overall incidence of 0.54% in Europe (1). Despite most infants can outgrow CMA, affected children need to avoid ingestion of cow’s milk proteins, which may restrict nutrient intake and decrease their quality of life. Early introduction of peanuts, between 4 and 11 months after birth, was found effective in prevention of peanut allergy development among high-risk infants (2) in the Learning Early About Peanut Allergy (LEAP) study. Similarly, early introduction of egg proteins between 4 and 6 months of age protected against the development of egg allergy (3). However, early introduction of cow’s milk protein was not found to reduce the risk on cow’s milk allergy among the general population in the Enquiring About Tolerance (EAT) observational study (4). Nevertheless, an earlier timing for cow’s milk protein exposure among a general population, in addition to breast feeding, within 14 days after birth (5) and during the first 3 months of life was found to be associated with a decreased risk of CMA (6).When breastfeeding is not possible, cow’s milk formula or hydrolyzed formula milk might be given to the atopic children at risk of CMA development as breastmilk substitute (7). However, neither cow’s milk formula nor hydrolyzed formula milk was proven effective in CMA prevention (7). Furthermore, early introduction of cow’s milk proteins (i.e. whole whey protein) might induce adverse reactions among infants that have been sensitized via environmental exposure via skin (8). Therefore, when breastfeeding is not possible for the high-risk infants, it is of importance to implement a safe and effective approach for CMA prevention. Previously 18-AA long synthetic peptides were identified within the β-lactoglobulin (BLG) sequence and shown to contribute to oral tolerance induction (9). In pre-clinical studies, BLG derived peptides showed reduced sensitizing capacity as compared to BLG protein (10), and were found capable of inducing oral tolerance to whole whey protein (9, 11). These peptides are too small to provoke allergic symptoms and therefore safe to provide early in life in children at risk to enrich formula milk. Our previous study showed a protective effect of BLG derived peptides encapsulated poly(lactic-co-glycolic acid) (PLGA) nanoparticles (NP) against CMA development when mice were housed in open cages (12). The gut-associated lymphoid tissue (GALT) is prone to induce peripheral immune tolerance to harmless food proteins (13) in the presence of the proper environmental stimuli as co-factors (14). Allergen loaded PLGA nanoparticles have been extensively exploited via subcutaneous (15) or oral route (16–18) for allergen immunotherapy in mice. In early life, microbial triggers are suggested to play a pivotal role in immune maturation and development of regulatory T-cells contributing to oral tolerance induction (19). Noteworthy, bacterial CpG DNA can activate the TLR-9 that is expressed in the endolysosomes of plasmacytoid DC and B-cells (20, 21) and drive regulatory immune maturation (22), skewing away from the allergic type 2 phenotype (23, 24). However, modern lifestyle and environmental changes, including improved hygiene conditions and use of antibiotics affect intestinal microbial diversity and commensal microbiota composition contributing to an immune imbalance and a rise in allergic diseases including food allergy (25, 26).
Housing mice in individual ventilated cages (IVC) simulates a strict hygienic environment with reduced exposure to bacterial ligands that are essential for immune function and/or contribute to immune maturation (27). We hypothesized that co-encapsulating TLR-9 ligand CpG together with a selected model synthetic peptide of 18-AA derived from BLG sequence (BLG-peptide, abbreviated as BLG-Pep) (12) in PLGA nanoparticles would improve the allergy preventive capacity of the BLG-Pep as CpG acts as a Th1 and Treg adjuvant (28–30). Noteworthy, this BLG-Pep was shown previously to be recognized by a cow’s milk specific human T-cell line in vitro and to reduce allergic skin reactivity to whole whey protein in mice when used in a relatively high dose (31) and at a lower dose when encapsulated in PLGA nanoparticles to optimize oral delivery (32). To further enhance the efficacy of this approach a strategy was developed to co-encapsulate BLG-Pep, to instruct allergen specificity, together with CpG, as an immunomodulatory adjuvant, into PLGA nanoparticles.
Previously, co-administration of antigen and class B CpG-oligonucleotides (CpG), as a Th1-skewing immune adjuvant, has been harnessed in food allergy (24) and allergic asthma immunotherapy (33) in mice. Current reported strategies for antigen and CpG co-delivery using PLGA carriers either incorporates cationic lipid dioleoyl-3-trimethylammonium propane (DOTAP) (34) or protamine (35). However, DOTAP functions as a Toll-like receptor 4 (TLR-4) agonist and interferes with the tolerance induction outcome. To preclude the influences from DOTAP and additional protein protamine, we have prepared well-defined CpG and BLG-Pep co-encapsulated PLGA nanoparticles.
In this study, we investigated the effect of orally administered nanoparticles co-encapsulated with CpG and BLG-Pep as a model peptide to prevent whey induced cow’s milk allergy in mice kept in IVC housing to mimic increased hygienic conditions.
Materials and methods
Peptide and CpG
A 18-AA-long sequential synthetic peptide that are derived from the chain B of bovine β-lactoglobulin, BLG-Pep (AASDISLLDAQSAPLRVY), previously indicated as Peptide 3 (12), was purchased from JPT Peptide Technologies (Berlin, Germany). Murine TLR-9 ligand CpG oligonucleotides 1826 (5’-tccatgacgttcctgacgtt-3’, further abbreviated as CpG) was purchased from In vivoGen (San Diego, USA).
Preparation and characterization of BLG-Pep and/or CpG encapsulated PLGA nanoparticles and release studies
Nanoparticles were prepared with poly (lactic-co-glycolic acid) (PLGA: lactide/glycolide molar ratio 50:50, 0.32 - 0.48 dL/g; PURASORB PDLG 5004A, Corbion, the Netherlands) using a double emulsion solvent evaporation method, as described in detail in the supplemental information. BLG-Pep (previously indicated as Peptide 3) were encapsulated in PLGA nanoparticles for the animal study as previously published (12, 32). The in vitro release method of BLG-Pep and CpG from the PLGA NP is described in detail in the supplemental method.
Animal study
Sixty 3-4-week-old pathogen free female C3H/HeOuJ mice were ordered from Charles River Laboratories (Sulzfeld, Germany). The mice were randomly allocated into 7 treatment groups and housed in individually ventilated cages in the animal facility of Utrecht University. The mice were fed cow’s milk protein free control purified diet AIN-93G (contains soy protein and supplemented methionine to replace casein as protein source, Ssniff diet obtained via Bio-services, Uden, the Netherlands) ad libitum in the study. Animal care and use in this study follows the guidelines of the Animal Ethics committee and the Center Commission for Animal use (CCD) of Utrecht University, with approval numbers of AVD108002015262-2.
Oral tolerance induction, sensitization and challenge
The CMA prevention murine model was developed and described by Schouten et al. (36) and Kostadinova et al. (37). As indicated in Figure 1, mice were randomly allocated into 7 groups and received 6-consecutive-daily oral pretreatments (Table 1) with the selected model BLG peptide (BLG-Pep) and/or CpG encapsulated in PLGA NP in a sterile biosafety cabinet starting 2 days after their arrival. Briefly, the mice received either PBS (Dulbecco’s phosphate-buffered saline, Sigma-Aldrich, Zwijdrecht, the Netherlands), 50 mg whey, BLG-Pep/NP (160 µg encapsulated BLG-Pep), CpG/NP (3 µg encapsulated CpG), a mixture of BLG-Pep/NP plus CpG/NP (160 µg BLG-Pep and 3 µg CpG in separate encapsulation form), or co-encapsulated BLG-Pep+CpG/NP (160 µg BLG-Pep and 3 µg CpG in co-encapsulation form) in 0.5 mL PBS via oral gavages. Two days after the tolerance induction phase mice received 5-consecutive-weekly sensitizations with 20 mg whey plus 10 µg cholera toxin (CT) in 0.5 mL PBS for all groups except sham (only CT) to break oral tolerance for whey from day 7 to day 35. Five days after the last whey-sensitization, mice received intradermal challenge with 10 µg whole whey protein in the ears (day 40) and at t=0 h and t=1 h the ear swelling response was measured (acute allergic skin response). Three hours after the intradermal whey challenge, the mice were orally challenged by means of gavage with 50 mg whey in 0.5 mL PBS. Eighteen hours after the oral whey challenge, mice were anesthetized with isoflurane and euthanized after blood sampling.
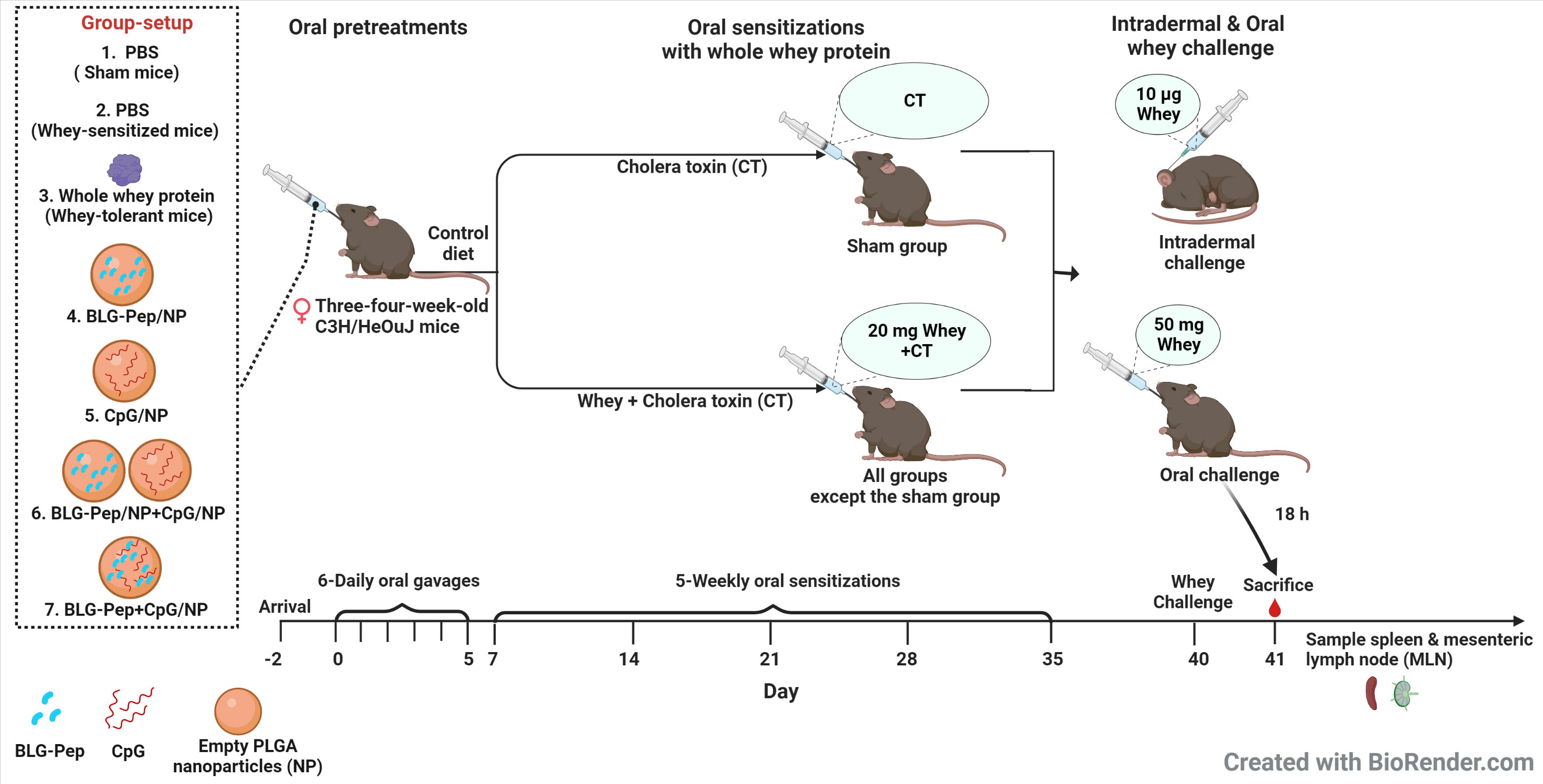
Figure 1 Experimental protocol for pre-treatment, sensitizations and challenge in murine model of cow’s milk allergy for the animal study. Three-four-week-old pathogen free female C3H/HeOuJ mice were given 6 daily oral pretreatments starting 2 days after arrival of the mice. Two days after the tolerance induction phase mice received 5-consecutive-weekly sensitizations with 20 mg whey plus 10 µg cholera toxin in 0.5 mL PBS for all groups but sham (only cholera toxin) from day 7 to day 35 to break whey tolerance. Five days after the last whey-sensitization, mice received intradermal challenge with 10 µg whole whey protein in the ears and at t=0 h and t=1 h the acute allergic skin response (ear swelling) was determined. Three hours after the intradermal whey challenge, the mice were orally challenged by means of gavage with 50 mg whey in 0.5 mL PBS. Eighteen hours after the oral whey challenge, mice were anesthetized with isoflurane and euthanized after blood sampling. Subsequently, spleen and mesenteric lymph nodes were collected immediately for further analysis. CT, Cholera toxin; NP, nanoparticles.
Assessment of acute allergic skin response
Five days after the last oral sensitization, the acute allergic skin response was recorded with the cage code concealed. Under anaesthesia by inhalation of isoflurane, the ear pinnae thickness of the mice was measured twice using a digital micrometer (Mitutoyo, Veenendaal, the Netherlands), before and 1 h after intradermal ear challenge with 10 µg whey protein in 20 µL PBS. Difference in ear pinnae thickness (ear swelling) was calculated by subtracting the basal ear pinnae thickness before challenge according to Schouten et al. (36). Meanwhile, body temperature and anaphylaxis symptoms score were recorded before and at 30 min and 1 h after intradermal challenge with whole whey protein according to Schouten et al. (36).
Serum mucosal mast cell protease-1 and allergen specific immunoglobulins
mMCP-1 was measured in serum that was collected 18 h after the oral whey challenge, using the mouse mucosal mast cell protease-1 (MCPT-1) Elisa kit (eBioscience, ThermoFisher, Massachusetts, USA) according to the manufacturer’s protocol. Serum whey- and BLG-specific immunoglobulins were measured a previously reported method (31).
Flow cytometry analysis of T cell subsets from spleen and DC from mesenteric lymph nodes and spleen
Eighteen hours after oral challenge of the mice with whole whey protein, isolation of cells from spleen (11) and mesenteric lymph nodes (MLN) (38) was performed as previously reported.
Approximate 8×105 isolated MLN or spleen cells were resuspended in 135 µL PBS (Sigma-Aldrich) and cultured in 96-wells falcon plates. Cell viability was determined by staining with 100 µL fixable viability dye (FVD)-eFluor™780 (ThermoFisher) at 2000 times dilution in PBS at 4˚C. Nonspecific binding sites were blocked by incubation with 25 µL anti-mouse CD16/CD32 (Mouse BD Fc block; BD Pharmingen, San Jose, USA) at 100 times dilution in 2% Fetal Bovine Serum (FBS)-1% Bovine Serum Albumin (BSA, Sigma-Aldrich)-PBS buffer for 5 min at 4˚C.
For extracellular staining of Th1/Th2 and Treg (Antibodies concentrations are shown in the Supplemental Tables 1, 2), splenocytes were incubated with CD4-BV510 (BioLegend, San Diego, USA), T1/ST2-FITC (MD Biosciences, Oakdale, USA), CXCR3-PE (ThermoFisher) and CD25-PerCP/eFluor710 (ThermoFisher) in 1% BSA-PBS buffer overnight at 4˚C. For intracellular Treg staining, cells were first fixed and permeabilized with FoxP3/Transcription Factor Staining Buffer Set (ThermoFisher) and then stained with FoxP3-FITC (ThermoFisher).
For extracellular staining of DC (antibodies concentrations are shown in the Supplemental Table 3), MLN and spleen cell suspension were incubated with MHCII-PE (ThermoFisher), CD11c-FITC (ThermoFisher), CD11b-PE/Cy7 (ThermoFisher), CD80-BV421 (BioLegend), CD86-BV510 (BioLegend) and programmed cell death ligand 1 (PD-L1)-PerCP/Cy5.5 (ThermoFisher) 1% BSA-PBS buffer overnight at 4˚C. Cells were analyzed with BD FACSCantoII flow cytometer (Becton Dickinson, Franklin Lakes, USA). Data analysis was conducted with FlowLogic software (Inivai Technologies, Mentone, Australia).
Ex vivo allergen re-stimulated cytokine release by splenocytes
Splenocytes (6×105 cells) were stimulated with either 200 μL 500 µg/mL whey protein (DMV International, Veghel, the Netherlands), 290 µg/mL β-Lactoglobulin B variant (from bovine milk, Sigma-Aldrich), or only blank RPMI 1640 medium supplemented with 10% FBS, 100 U/mL penicillin and 100 μg/mL streptomycin as control and cultured in a round-bottom culture plate at 37°C, 5% CO2. After 5-days, supernatants were collected and determined using a Procartaplex kit for mouse IL-13, IL-5, IL-10 and TNF-α and mouse IFN-γ, IL-17A and TGF-β ELISA kits (both from ThermoFisher) according to the manufacturer’s protocols and using a GloMax® Discover plate reader (Promega, Wisconsin, USA).
Statistical analysis
The obtained in vivo data were analyzed with GraphPad Prism 9.0.0 software (GraphPad Software, San Diego, USA). Normal distribution of the data was assessed by the Shapiro-Wilk test and Kolmogorov-Smirnov test. If required data were log transformed to obtain normal distribution or alternatively a non-parametric test was used. One-way ANOVA followed by Bonferroni’s post hoc test, or the non-parametric Kruskal-Wallis test followed by Dunn’s post hoc test was applied for selected pairs (as indicated in the figure legends). In the post hoc analyses for selected pairs the whey-sensitized group was compared to all the other groups and the BLG-Pep+CpG/NP group was compared to all groups except the sham group (non-sensitized group).
Results
Characteristics of PLGA nanoparticles
Empty and encapsulated PLGA nanoparticles had average hydrodynamic particle sizes in the range of 240-290 nm. As a parameter that described the size range of nanocarriers (39), low polydispersity indices (PDI) below 0.14 (Table 2) of these nanoparticles demonstrated their narrow size distributions. The particles had close to neutral zeta-potentials (around -1 mV), as a key parameter reflects the electrostatic interactions and stability of the nanoparticles dispersions (40), in 10 mM HEPES buffer (pH7.4). BLG-Pep was encapsulated without CpG (in BLG-Pep/NP) or with CpG (in BLG-Pep+CpG/NP) with encapsulation efficiencies (EE) between 41-65% and loading capacities (LC) between 0.7-0.8%. CpG was encapsulated in CpG/NP (i.e., without peptide) and in BLG-Pep+CpG/NP with EEs between 38-47% and LCs between 0.02-0.06%. Quantification of the encapsulated BLG-Pep and CpG cargos in the PLGA nanoparticles enabled further in vitro release study and dosing for the pretreatments in the animal study.
The in vitro release profiles of BLG-Pep/NP were similar to our previous study (12), except for a burst release of 18% BLG-Pep from the BLG-Pep/NP and no burst release of the peptide cargo from the BLG-Pep+CpG/NP used in the animal study (Supplemental Figure 1A). Thereafter, a slow-release phase and accelerated release phase of peptide cargo were observed during 49-58 days.
For the release of CpG, a burst release of 20% CpG from CpG/NP and 10% CpG from BLG-Pep+CpG/NP were observed, followed by a sustained release of CpG from day 7 onwards (Supplemental Figure 1B).
Co-encapsulated BLG-Pep+CpG/NP prevented the acute allergic skin response and serum BLG-specific IgE levels
The animal study was performed in IVC housing as indicated in Table 1 and Figure 1. Whey-sensitized (sham-pretreated mice) mice had significantly increased ear thickness one hour after intradermal whey challenge, as compared to the sham (PBS-pretreated mice) and whey-tolerant group (whey-pretreated mice), indicating successful establishment of a prophylatic CMA murine model in this study (Figure 2A and Supplemental Figure 2A). Pretreatments by nanoparticles encapsulated with only BLG-Pep (BLG-Pep/NP) did not prevent the acute allergic skin response (Figure 2A). In the animal study, only co-encapsulated BLG-Pep+CpG/NP, but not pretreatments with, PBS (sham group), BLG-Pep/NP, CpG/NP or BLG-Pep/NP+CpG/NP, effectively prevented the whey induced acute allergic skin response as compared to the whey-sensitized (sham-pretreated) mice. Moreover, co-encapsulated BLG-Pep+CpG/NP prevented the whey induced acute allergic skin response as compared to separately delivered BLG-Pep/NP+CpG/NP in mice (Figure 2A and Supplemental Figure 2A). No statistic difference was observed in body temperature or anaphylactic shock score of the mice during 1 h after intradermal whey challenge (Supplemental Figure 3). Despite lack of statistical significance, mMCP-1 measured in the serum showed a similar pattern as the acute allergic skin response result (Supplemental Figure 2B).
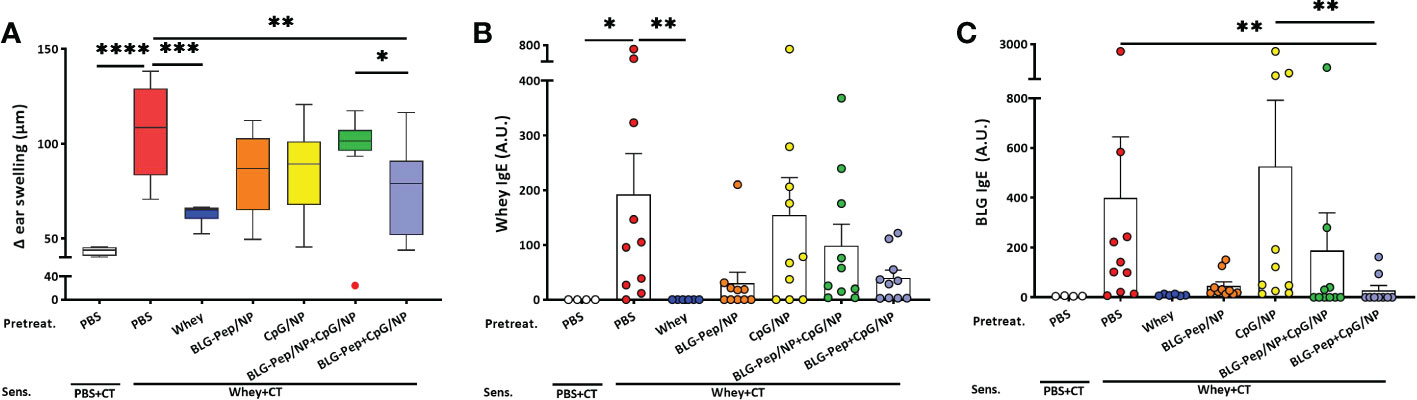
Figure 2 Acute allergic skin response and BLG- and whey-specific serum immunoglobulin E. Five days after last sensitization, mice were intradermally challenged in the ear pinnae with 10 µg whey followed by oral challenge. The acute allergic skin response was measured 60 min afterwards in the in vivo study (A). BLG- (B) and whey-specific (C) IgE levels are measured in serum, which were collected 18 h after last oral challenge with whey in mice from all groups of the animal study. Data are presented as box-and-whisker Tukey plots for (A), and mean ± SEM for (B, C) n=9-10 per group except for the sham group, n=4 and whey-tolerant group, n=6. (A–C) are presented with Y axis formatted in two segments to properly show the relevant part for the window of effect (A) or to be able to appreciate the full data set (B, C). (A) is analyzed by one-way ANOVA, followed by Bonferroni’s post hoc test for selected pairs. The outlier (in red) in the BLG-Pep/NP+CpG/NP group is excluded in (A) from statistics; (B, C) are analyzed with the Kruskal-Wallis non-parametric test, followed by Dunn’s post hoc test for selected pairs; *p<0.05, **p<0.01, ***p<0.001, ****p<0.0001; BLG, β-lactoglobulin; CT, Cholera toxin.
Consistent with the prevented allergic skin reactivity, mean BLG-specific IgE serum levels 18 h after oral whey challenge were significantly lower in the BLG-Pep+CpG/NP recipients compared to both whey-sensitized (sham-pretreated mice) and CpG/NP groups (Figure 2B). A similar overall pattern was found for whey-specific IgE, albeit this did not reach statistical significance (Figure 2C). In the whey-tolerant group (whey-pretreated mice), BLG- and whey-specific IgG1 and IgG2a remained low (Supplemental Figure 4A–D), but the other pretreatments did not reduce these immunoglobulins levels.
In mesenteric lymph nodes co-encapsulated BLG-Pep+CpG/NP decreased percentages of CD80/CD86+CD11b+ DC
No differences in the mean percentages of CD11c+MHCII+ DC in MLN were found among all pretreatment groups (Figure 3A). The co-encapsulated BLG-Pep+CpG/NP recipients showed a lower percentage CD11b+ DC and higher percentage of CD11b- DC subsets compared to both the CpG/NP group and BLG-Pep/NP+CpG/NP group (Figures 3D, G). The co-encapsulated BLG-Pep+CpG/NP pretreatment reduced the expression of costimulatory molecules CD80 and CD86 on DC and CD11b+ DC compared to BLG-Pep/NP+CpG/NP pretreatment, but not on CD11b- DC in MLN (Figures 3B, C, E, F, H, I). The gating strategy of the flow cytometry analysis for DC from MLN is shown in Supplemental Figure 8.
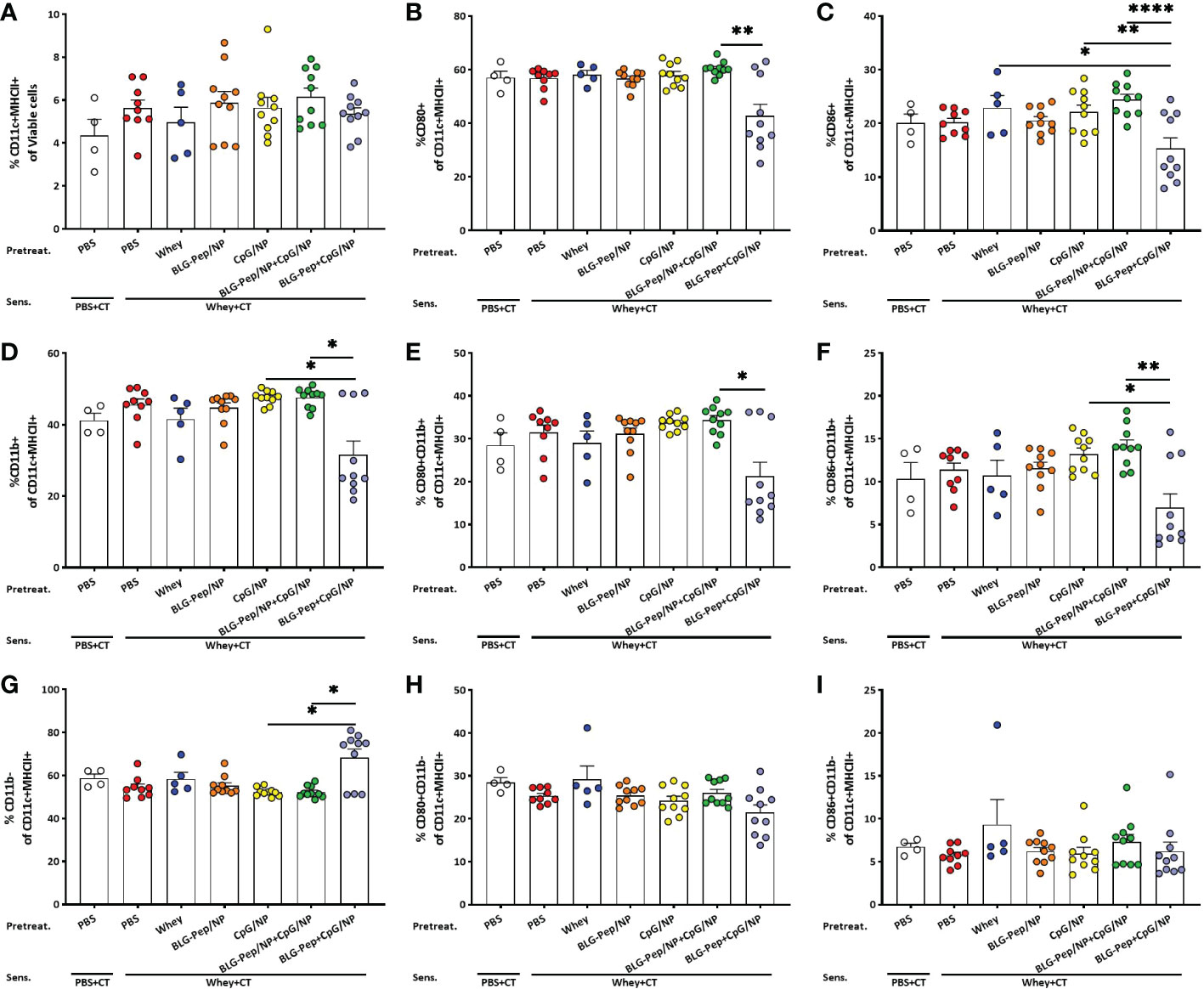
Figure 3 Surface activation markers expression on dendritic cells in mesenteric lymph nodes (MLN). Eighteen hours after oral whey-sensitization, MLN cells were isolated and analyzed using flow cytometry to determine the percentage of CD11c+MHCII+ DC (A) and CD11b+ (D) and CD11b- (G) DC subsets. Surface costimulatory molecules expression of CD80+ (B, E, H) and CD86+ (C, F, I) on CD11c+MHCII+ DC, CD11b+ and CD11b- DC subsets were determined respectively. Data are presented as mean ± SEM for n=9-10 per group except for the sham group, n=4 and whey-tolerant group, n=5. (A) is analyzed with one-way ANOVA for selected pairs after log transformation, followed by Bonferroni’s post hoc test; (B) and (D–I) are analyzed with the Kruskal-Wallis non-parametric test, followed by Dunn’s post hoc test for selected pairs; (C) is analyzed with one-way ANOVA for selected pairs after log transformation, followed by Bonferroni’s post hoc test; *p<0.05, **p<0.01; CT, Cholera toxin. ****p<0.0001.
Co-encapsulated BLG-Pep+CpG/NP reduced percentages of PD-L1+ DC subsets in mesenteric lymph nodes but increased PD-L1+CD11b+ DC frequency in spleen
In MLN, co-encapsulated BLG-Pep+CpG/NP, but not the other pretreatments, significantly decreased surface expression of PD-L1+ on CD11c+MHCII+ DC (Figure 4A) as compared to the whey-sensitized group in CD11b- DC (Figure 4C), and only showed a decreasing tendency in CD11b+ DC subset (p=0.0655, Figure 4B). In spleen, however, co-encapsulated BLG-Pep+CpG/NP pretreatment upregulated expression of PD-L1 on CD11b+ DC subset (Figure 4E) and tended to enhance PD-L1 expression on CD11c+MHCII+ DC (p=0.0617, Figure 4D), but not on its CD11b- DC subset (Figure 4F), as compared to the BLG-Pep/NP plus CpG/NP group.
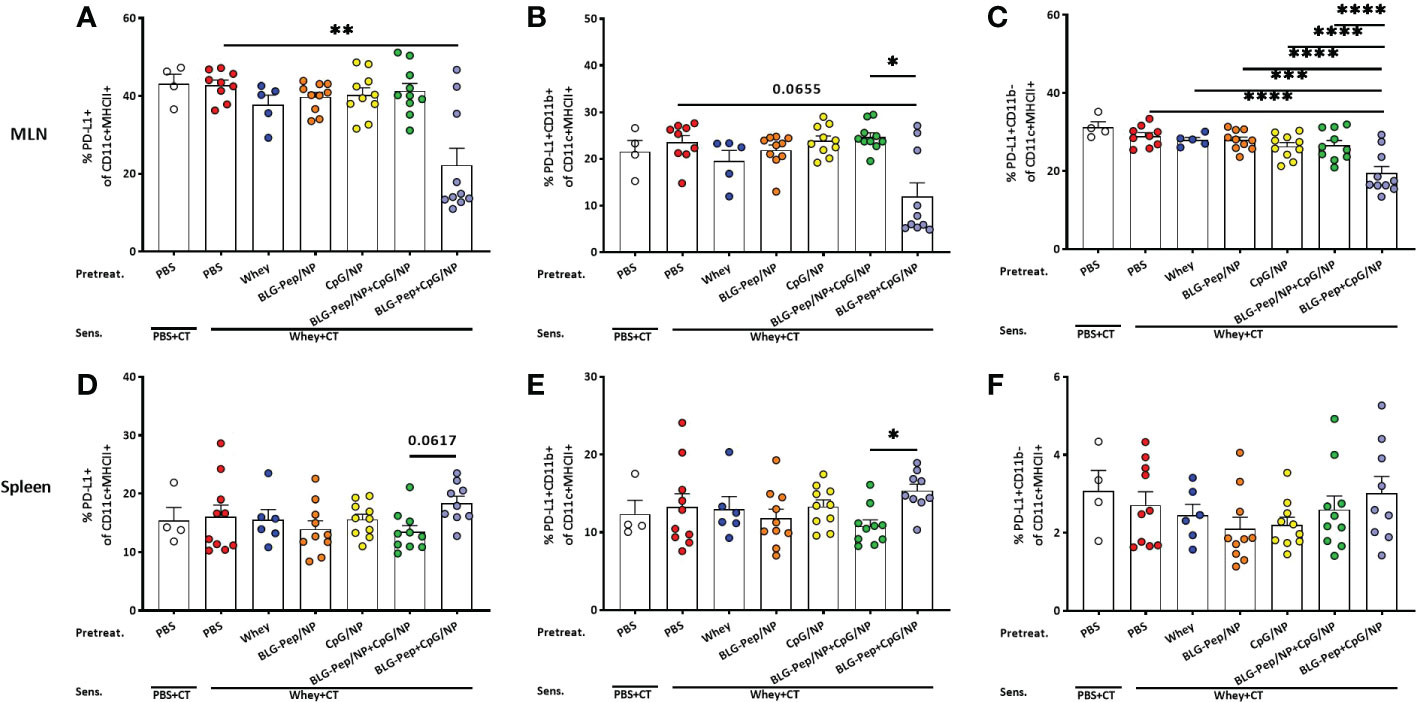
Figure 4 Expression of PD-L1 on dendritic cells from mesenteric lymph nodes (MLN) and spleen. Eighteen hours after the last oral challenge with whey, cells isolated from MLN and spleen were analyzed by flow cytometry for the frequencies of PD-L1+ on CD11c+MHCII+ DC (A, D), PD-L1+CD11b+ (B, E) and PD-L1+CD11b- (C, F) DC subsets in MLN and spleen respectively. Data are presented as mean ± SEM for n=8-10 per group except for the sham group, n=4 and whey-tolerant group, n=6. (A, B) and (D–F) are analyzed with the Kruskal-Wallis non-parametric test, followed by Dunn’s post hoc test for selected pairs; (C) is analyzed with one-way ANOVA for selected pairs after log transformation, followed by Bonferroni’s post hoc test; *p<0.05, **p<0.01; CT, Cholera toxin. ***p<0.001, ****p<0.0001.
In splenocytes co-encapsulated BLG-Pep+CpG/NP lowered the percentage of Th2 cell while increasing ratios Treg/Th2 and Th1/Th2
The co-encapsulated BLG-Pep+CpG/NP pretreatment resulted in an increasing trend in CD25+FoxP3+ Treg percentage as compared to the BLG-Pep/NP+CpG/NP pretreated mice (p=0.0518, Figure 5A).The co-encapsulated BLG-Pep+CpG/NP pretreatment reduced the percentage of T1/ST2+ Th2 cells (Figure 5B) compared to the whey-sensitized group, but no difference was found on the frequencies of CXCR3+ Th1 (Figure 5C) and CD196+ Th17 subsets (Data not shown for Th17) in all pretreatment groups. Co-encapsulated BLG-Pep+CpG/NP increased ratios of Treg/Th2 and Th1/Th2 as compared to the whey-sensitized mice (Figures 5D, F), and tended to increase the Treg/Th1 ratio in BLG-Pep+CpG/NP recipients as compared to whey-tolerant mice (p=0.0647, Figure 5E). The gating strategy of the flow cytometry analysis for Th1, Th2 and Treg subsets from splenocytes is shown in Supplemental Figure 9.
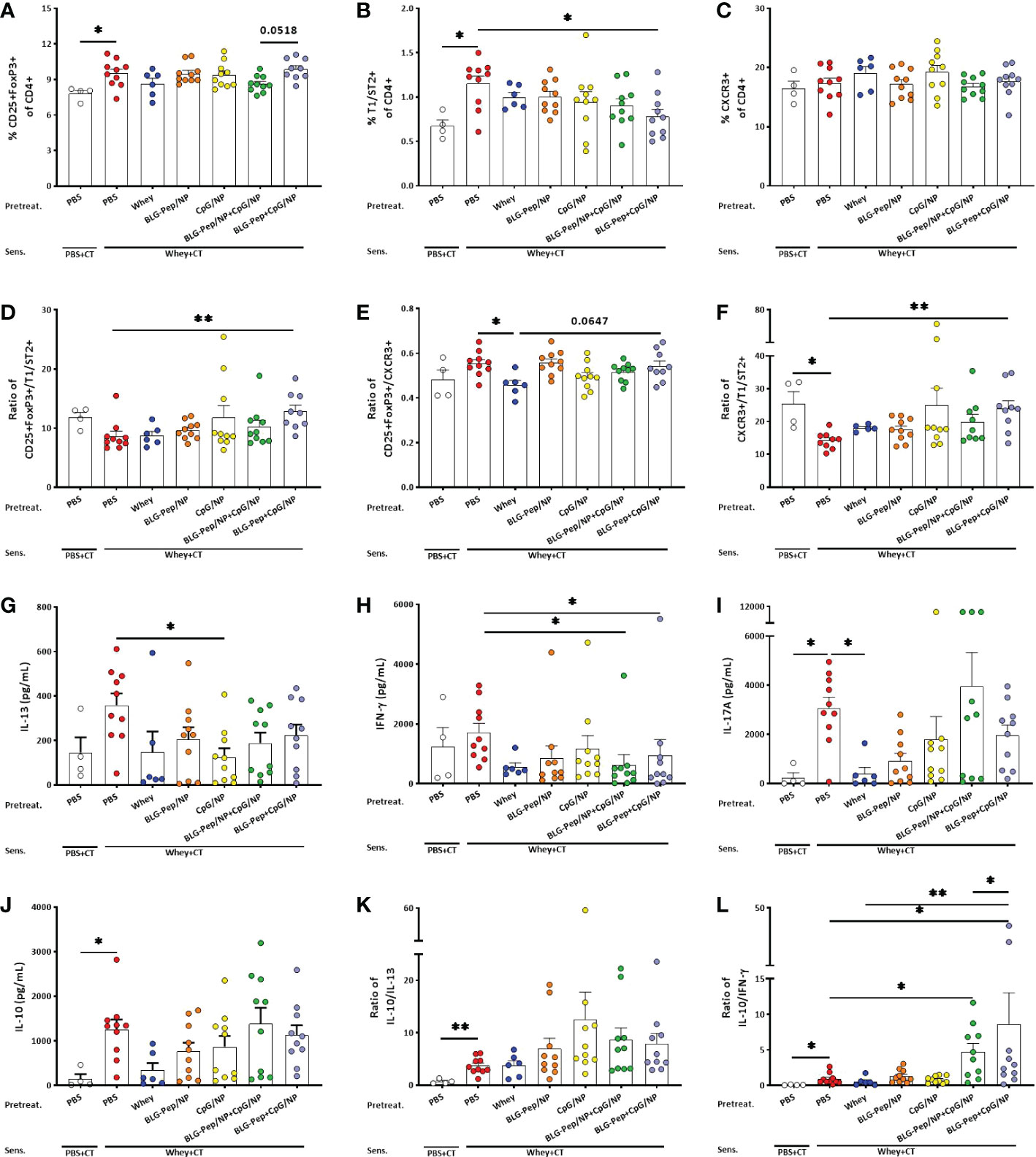
Figure 5 Splenic T-cell subsets and cytokines production of splenocytes upon ex vivo stimulation with whey for 5 days. Eighteen hours after the last oral challenge with whey, splenocytes were isolated and analyzed by flow cytometry for the percentages of T-cell subsets including Treg (A), Th2 (B), Th1 (C), ratio of Treg/Th2 (D), ratio of Treg/Th1 (E) and ratio of Th1/Th2 (F) respectively. Splenocytes were restimulated with whole whey protein for 5 days, Th2- (G), Th1- (H), Th17- (I), and Treg- (J) associated cytokines were measured in supernatants. Ratios of Treg-/Th2- associated (K) and Treg-/Th1-associated (L) cytokines were calculated. (F, I, K, L) are presented with Y axis formatted in two segments to be able to appreciate the full data set. Data are presented as mean ± SEM for n=9-10 per group except for the sham group, n=4 and whey-tolerant group, n=6. (A–C) and (E) are analyzed with one-way ANOVA for selected pairs, followed by Bonferroni’s post hoc test; (D) and (F–K) are analyzed with the Kruskal-Wallis non-parametric test, followed by Dunn’s post hoc test for selected pairs; (L) is analyzed with one-way ANOVA for selected pairs after log transformation, followed by Bonferroni’s post hoc test; *p<0.05, **p<0.01; CT, Cholera toxin.
Co-encapsulated BLG-Pep+CpG/NP decreased whey-restimulated IFN-γ and enhanced IL-10/IFN-γ ratio in splenocyte supernatants
CpG/NP pretreatment reduced whey-restimulated splenocytes release of IL-13 (Figure 5G), while BLG-Pep/NP+CpG/NP and co-encapsulated BLG-Pep+CpG/NP pretreatments lowered whey-restimulated splenocytes release of IFN-γ compared with the whey-sensitized mice (Figure 5H). Whey-sensitized mice showed higher whey-stimulated IL-17A and IL-10 production by splenocytes compared to sham mice (Figures 5I, J), which was prevented in the whey-tolerant group for IL-17A secretion (Figure 5I). Despite no statistical differences were found in IL-10 concentrations or in the ratio of IL-10/IL-13 (Figure 5K), BLG-Pep/NP+CpG/NP and co-encapsulated BLG-Pep+CpG/NP pretreatments significantly increased the ratio of IL-10/IFN-γ compared to whey-sensitized mice (Figure 5L). BLG-Pep+CpG/NP pretreatment also significantly increased the ratio of IL-10/IFN-γ compared to the BLG-Pep/NP+CpG/NP group and whey-tolerant group (Figure 5L). Supplemental Figure 5–7 shown medium versus whey-stimulated cytokine responses and additional whey or BLG induced re-stimulation responses.
Discussion
We hypothesized that CpG as an adjuvant can enforce the allergy preventive effect of a model selected BLG peptide co-encapsulated in PLGA (BLG-Pep/NP) under strict hygienic conditions. Previously, this selected BLG peptide (BLG-Pep) was shown to be recognized by a cow’s milk specific human T-cell line in vitro and to inhibit acute allergic skin response to whey protein in mice pretreated with a relatively high dose (31) and at a lower dose when encapsulated in PLGA nanoparticles to optimize oral delivery (32). In this study, 3-4-week-old female mice were chosen to investigate the oral tolerance induction in early life using pretreatment of BLG-peptide and CpG co-encapsulated PLGA NP before oral sensitization with whole whey protein. In the 3-4-week-old mice, microfold (M) cells have already reached adult levels, since this is achieved before 2-3 week of age. This will allow access of the PLGA nanoparticles to the Peyer’s patch and gut draining mesenteric lymph nodes where nanoparticle uptake and peptide presentation by the DC can instruct T-cell development (41, 42). Recruitment of T-cells to the neonatal mucosa (43, 44) and microbiome maturation (45) are pivotal for induction of Treg and thus establishment of oral tolerance (46). To further enhance the allergy preventive effect, a strategy was developed to co-encapsulate BLG peptide (to instruct allergen specificity) together with CpG (as an immunomodulatory adjuvant) into PLGA nanoparticles.
BLG-Pep and CpG were separately dissolved in the water phase (PBS), and both were emulsified with PLGA solubilized in oil phase (dichloromethane) to form two water-in-oil emulsions. This was followed by combining these two water-in-oil emulsions and next via preparing the water-in-oil-in-water emulsion (47) in order to co-encapsulate BLG-Pep and CpG into the PLGA nanoparticles. BLG-Pep and CpG were co-encapsulated in ~250 nm size PLGA nanoparticles with encapsulation efficiencies for both compounds of about 40%. In vitro, the BLG-Pep+CpG/NP showed a burst release of about 20% of the CpG, and no burst release of BLG-Pep from the NP matrix, which might be attributed to the insufficient washing and/or diffusion from the microporous channels that were resulted from the freeze drying process (48, 49). The sustained release of BLG-Pep+CpG/NP from PLGA NP demonstrates that a suitable formulation for in vivo evaluation was developed. Similar to many other drugs, there is a therapeutic dose window for CpG as an adjuvant, and toxicity was shown in mice that received a repetitive daily injection of CpG in a dose above 2.4 mg/kg (50). In this study, a relative low dose of 3 μg encapsulated CpG per daily oral gavage was given to the mice for a period of 6 days, which is a safe dosing scheme in terms of toxicity as reviewed (51). PLGA is biodegradable and biocompatible polymer as approved by the US Food and Drug Administration (FDA) for vaccine and drug delivery (52, 53). PLGA nanopaticles provide protection for the encapsulated BLG-peptide and CpG cargos (54) and facilitate cellular uptake by antigen-presenting cells (54).
As shown by previous studies, intestinal uptake and lymphatic transport of oral delivered nanoparticles are dependent on surface charge, particle size and dose of the administered nanoparticles (55–57). The similar size and surface charge of the different PLGA NP suggest similar mucus penetration and cellular uptake of these nanoparticles after oral administration. Despite that, only the co-encapsulation gave the allergy preventive effect (i.e., prevented acute allergic skin reactivity and rise in BLG-specific IgE) even compared to the cocktail of BLG-Pep/NP plus CpG/NP. Antigen-encapsulated PLGA nanoparticles can be internalized by DC into phagosomes via endocytosis (58), followed by endosomal degradation for the MHCII pathway (59). On the other hand, TLR-9 and MHCII are also present in the endolysosomes of plasmacytoid DC and B-cells (21, 30). Thus, simultaneous internalization and intracellular release of CpG and BLG-Pep from BLG-Pep+CpG/NP in the endolysosomes of DC may facilitate synergistic activation of TLR-9 and antigen-presentation via MHCII pathway in the same DC (see graphical abstract Figure 6).
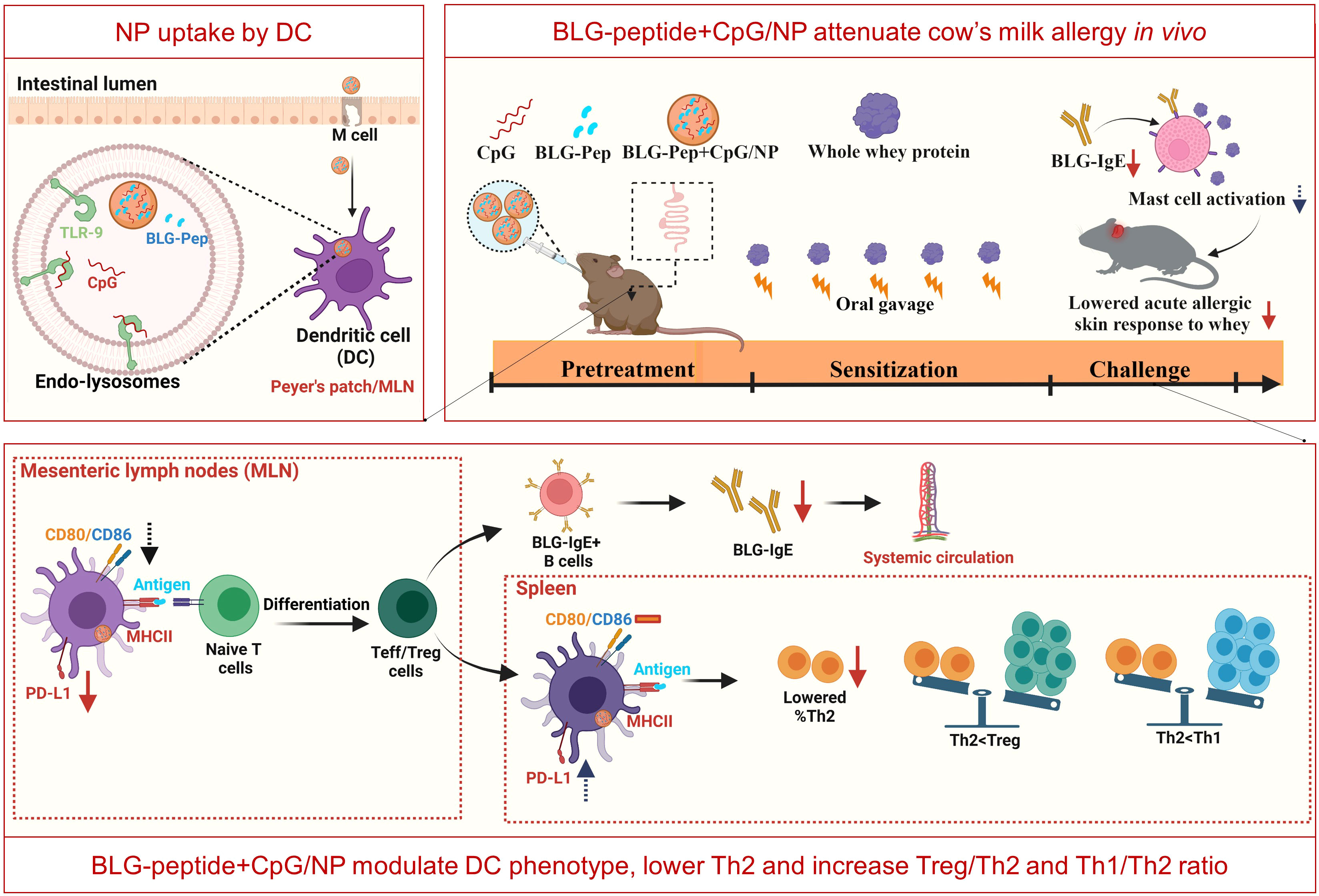
Figure 6 Graphical Abstract (Created with BioRender.com).
Oral pretreatment with a daily dosage of both antigen and adjuvant (160 μg BLG-Pep plus 3 μg CpG) for a short period (6 days) prevented skin reactivity and rise in serum BLG-specific IgE. Srivastava et al. (24) reported oral therapy using whole peanut extract encapsulated PLGA nanoparticles with surface conjugation of CpG, inhibited peanut allergic symptoms and facilitated peanut allergy outgrowth (24). Our study differs from this study substantially in that we investigated the cow’s milk allergy prevention effect using BLG-Pep (18-AA) and CpG co-encapsulated PLGA nanoparticles in a cow’s milk allergy murine model kept in IVC housing. In this study, BLG-Pep+CpG/NP pretreatment significantly prevented rise in BLG-specific IgE levels, but did not lower whey- or BLG-specific IgG1 and IgG2a levels. Despite the role of allergen-specific IgG1 in allergic diseases remains elusive (60), it is likely that full inhibition of allergen-specific IgE and IgG for both BLG and whey requires additional whey epitopes. Here we used only one small BLG model peptide of 18-AA while BLG protein itself consist of 162-AA (18.4 kDa) and multiple epitopes (61). Moreover, whole whey protein consists not only of BLG as allergen but also other allergenic proteins such as alpha-lactoglobulin (61). Thus, in future studies, an additional BLG-peptide from another region in the BLG protein sequence and/or peptides of other whey-derived allergens may be supplemented to the current regime and co-encapsulated with CpG to generate full tolerance for whole whey protein.
DC as antigen presenting cells, capture and recognize microorganisms via pathogen-associated-molecular-patterns (PAMPs) by Toll-Like Receptors (TLRs) (62), mediating tolerance (63) or activation of T-cell responses (64) to various antigens. Co-encapsulated BLG-Pep+CpG/NP recipients showed a lower frequency of the Th2/Th17 immune polarizing CD11b+ DC subset in the MLN (65), and a reduced expression of costimulatory molecules CD80/CD86 on CD11c+MHCII+ DC compared to the BLG-Pep/NP plus CpG/NP group, suggesting a more tolerogenic DC phenotype and consequent constraint of Th2 immunity (66). Programmed cell death ligand 1 (PD-L1) is expressed on non-lymphoid and lymphoid cells, including resting B-cells, T-cells, macrophages and DC, and regulates immune responses in secondary lymphoid and target organs (67). In particular, expression of PD-L1 on DC was reported essential for inducing IL-10 secreting T-cells in vitro (68), and required for generation of adaptive Treg subsets both in vitro and in vivo (69). Despite the diminished frequency of PD-L1+ DC in the MLN of the BLG-Pep+CpG/NP recipients, the simultaneous decreased surface expression of co-stimulatory molecules CD80/CD86 was previously shown to enforce a tolerogenic phenotype with a reduced maturation status (70).
Given the short lifespan of DC for less than 9 days (71), we speculate that the general DC phenotype may have undergone epigenetic changes and form immune memory responses via a process known as trained immunity resulting in a more tolerogenic DC phenotype (72, 73). Even though BLG-Pep+CpG/NP pretreatment reduced PD-L1 expression in the MLN compared to the whey-sensitized mice and the BLG-Pep/NP plus CpG/NP recipients, it enhanced the PD-L1 expression on splenic CD11b+ DC as compared to BLG-Pep/NP plus CpG/NP recipients. The latter was associated with an increase in the percentage of CD25+FoxP3+ Treg cells compared to the BLG-Pep/NP plus CpG/NP group. We hypothesize BLG-Pep+CpG/NP pretreatment to have instructed development of Treg that reside in the spleen, upon reactivation these cells may modify the phenotype of local DC resulting in enhanced PD-L1 expression actively contributing to systemic Treg function and a tolerogenic micromilieu (74–77). Alternatively, the BLG-Pep+CpG/NP pretreatment may have resulted in upregulation of PD-L1 on intestinal DC upon whey challenge, which than may have migrated via the MLN to the spleen for antigen presentation to naive CD4+ T-cells (78, 79), inducing development of regulatory T-cell and memory T-cells (74–77).
Indeed, a high dose CpG (50 μg) was reported to enhance expression of indoleamine 2,3-dioxygenase (IDO) in the plasmacytoid DC (80) after ligation to the Toll-like Receptor-9 in the endolysosomes, and hence activate a suppressor phenotype of Treg cells for tolerance induction (81). Therefore, we hypothesize the PLGA nanoparticles co-encapsulating only 3 µg CpG and 160 µg BLG-Pep might generate a tolerogenic micromilieu preventing allergy development for whole whey protein via supporting regulatory responses. The provided dose of CpG was relatively low and already effective. The dose-dependency of the BLG-Pep and CpG co-encapsulated PLGA NP for allergy prevention and antigen-specific tolerance induction via oral delivery and possible uptake by plasmacytoid DC warrants further investigation.
As reported, CD11b+ DCs initiate and maintain Th2 immunity towards allergens (82). However, BLG-Pep+CpG/NP pretreatment upregulated PD-L1 expression on these conventional CD11b+ splenic DC compared to BLG-Pep/NP plus CpG/NP recipients, which might also have promoted Treg development and reduced Th2 immunity upon re-exposure to whey allergens during sensitization and challenge stages (83, 84). Indeed, BLG-Pep+CpG/NP pretreatment induced a significant diminished frequency of T1/ST2+ Th2 cells, and higher splenic Treg/Th2, Th1/Th2 and IL-10/IFN-γ ratios compared to the whey-sensitized mice, which may favor a tolerogenic outcome (see graphical abstract Figure 6). The observed constraint of Th2 cells development might be attributed to the immunomodulatory effect from the co-encapsulated Type B CpG. As reported previously (85), systemic administered CpG reduces Th2 associated cytokines production without affecting Th1 cytokines in a murine asthma model. However, in the current study despite the decreased Th2 frequency in the CpG and peptide co-encapsulated group, upon BLG restimulation of the splenocytes the cytokine release was not silenced which was the case in the whey-tolerant group (Supplemental Figure 7). Hence, pretreatment with a single 18-AA BLG peptide may not be sufficient in overruling the immunogenic properties of the full immunogenic T-cell epitope repertoire of the BLG fraction in whey protein.
Collectively, these observations provide evidence that BLG-Pep+CpG/NP pretreatment changes the DC phenotype, reduces Th2 development and drives towards a Th1 and Treg microenvironment, which might contribute to reducing allergen-specific CMA development.
Data availability statement
The original contributions presented in the study are included in the article/Supplementary Material. Further inquiries can be directed to the corresponding author.
Ethics statement
The animal study was reviewed and approved by Animal Ethics committee of Utrecht University and the Center Commission for Animal use (CCD).
Author contributions
LW, CN, and ML designed the experiments; ML and ST performed the experimental procedures. ML performed data collection and analyses and drafted the manuscript. LW, CN, WH, and JG contributed to data interpretation and critically revised the manuscript. All authors contributed to the article and approved the submitted version.
Funding
ML is financially supported by China Scholarship Council (CSC), grant number 201707720004, and additional bench fee funding is supplied by Nutricia Research B.V., Utrecht, the Netherlands. The funder was not involved in the study design, analysis, interpretation of data, the writing of this article, or the decision to submit it for publication.
Conflict of interest
JG is employed by Nutricia Research B.V.
The remaining authors declare that the research was conducted in the absence of any commercial or financial relationships that could be construed as a potential conflict of interest.
Publisher’s note
All claims expressed in this article are solely those of the authors and do not necessarily represent those of their affiliated organizations, or those of the publisher, the editors and the reviewers. Any product that may be evaluated in this article, or claim that may be made by its manufacturer, is not guaranteed or endorsed by the publisher.
Supplementary material
The Supplementary Material for this article can be found online at: https://www.frontiersin.org/articles/10.3389/fimmu.2022.1053107/full#supplementary-material
References
1. Schoemaker AA, Sprikkelman AB, Grimshaw KE, Roberts G, Grabenhenrich L, Rosenfeld L, et al. Incidence and natural history of challenge-proven cow's milk allergy in European children–europrevall birth cohort. Allergy (2015) 70(8):963–72. doi: 10.1111/all.12630
2. Du Toit G, Roberts G, Sayre PH, Bahnson HT, Radulovic S, Santos AF, et al. Randomized trial of peanut consumption in infants at risk for peanut allergy. N Engl J Med (2015) 372(9):803–13. doi: 10.1056/NEJMoa1414850
3. Koplin JJ, Osborne NJ, Wake M, Martin PE, Gurrin LC, Robinson MN, et al. Can early introduction of egg prevent egg allergy in infants? a population-based study. J Allergy Clin Immunol (2010) 126(4):807–13. doi: 10.1016/j.jaci.2010.07.028
4. Perkin MR, Logan K, Marrs T, Radulovic S, Craven J, Flohr C, et al. Enquiring about tolerance (Eat) study: Feasibility of an early allergenic food introduction regimen. J Allergy Clin Immunol (2016) 137(5):1477–86.e8. doi: 10.1016/j.jaci.2015.12.1322
5. Katz Y, Rajuan N, Goldberg MR, Eisenberg E, Heyman E, Cohen A, et al. Early exposure to cow's milk protein is protective against ige-mediated cow's milk protein allergy. J Allergy Clin Immunol (2010) 126(1):77–82.e1. doi: 10.1016/j.jaci.2010.04.020
6. Peters RL, Koplin JJ, Dharmage SC, Tang MLK, McWilliam VL, Gurrin LC, et al. Early exposure to cow's milk protein is associated with a reduced risk of cow's milk allergic outcomes. J Allergy Clin Immunol Pract (2019) 7(2):462–70.e1. doi: 10.1016/j.jaip.2018.08.038
7. Halken S, Muraro A, de Silva D, Khaleva E, Angier E, Arasi S, et al. Eaaci guideline: Preventing the development of food allergy in infants and young children (2020 update). Pediatr Allergy Immunol (2021) 32(5):843–58. doi: 10.1111/pai.13496
8. Lack G. Epidemiologic risks for food allergy. J Allergy Clin Immunol (2008) 121(6):1331–6. doi: 10.1016/j.jaci.2008.04.032
9. Meulenbroek LA, van Esch BC, Hofman GA, den Hartog Jager CF, Nauta AJ, Willemsen LE, et al. Oral treatment with beta-lactoglobulin peptides prevents clinical symptoms in a mouse model for cow's milk allergy. Pediatr Allergy Immunol (2013) 24(7):656–64. doi: 10.1111/pai.12120
10. Bogh KL, Barkholt V, Madsen CB. The sensitising capacity of intact beta-lactoglobulin is reduced by Co-administration with digested beta-lactoglobulin. Int Arch Allergy Immunol (2013) 161(1):21–36. doi: 10.1159/000343042
11. Kostadinova AI, Pablos-Tanarro A, Diks MAP, van Esch B, Garssen J, Knippels LMJ, et al. Dietary intervention with beta-Lactoglobulin-Derived peptides and a specific mixture of fructo-oligosaccharides and bifidobacterium breve m-16v facilitates the prevention of whey-induced allergy in mice by supporting a tolerance-prone immune environment. Front Immunol (2017) 8:1303. doi: 10.3389/fimmu.2017.01303
12. Liu M, Thijssen S, van Nostrum CF, Hennink WE, Garssen J, Willemsen LEM. Inhibition of cow's milk allergy development in mice by oral delivery of beta-Lactoglobulin-Derived peptides loaded plga nanoparticles is associated with systemic whey-specific immune silencing. Clin Exp Allergy (2022) 52(1):137–48. doi: 10.1111/cea.13967
13. Mowat AM. To respond or not to respond - a personal perspective of intestinal tolerance. Nat Rev Immunol (2018) 18(6):405–15. doi: 10.1038/s41577-018-0002-x
14. Mowat AM. Anatomical basis of tolerance and immunity to intestinal antigens. Nat Rev Immunol (2003) 3(4):331–41. doi: 10.1038/nri1057
15. Scholl I, Weissenbock A, Forster-Waldl E, Untersmayr E, Walter F, Willheim M, et al. Allergen-loaded biodegradable Poly(D,L-Lactic-Co-Glycolic) acid nanoparticles down-regulate an ongoing Th2 response in the Balb/C mouse model. Clin Exp Allergy (2004) 34(2):315–21. doi: 10.1111/j.1365-2222.2004.01884.x
16. Walter F, Scholl I, Untersmayr E, Ellinger A, Boltz-Nitulescu G, Scheiner O, et al. Functionalisation of allergen-loaded microspheres with wheat germ agglutinin for targeting enterocytes. Biochem Biophys Res Commun (2004) 315(2):281–7. doi: 10.1016/j.bbrc.2004.01.057
17. Roth-Walter F, Scholl I, Untersmayr E, Ellinger A, Boltz-Nitulescu G, Scheiner O, et al. Mucosal targeting of allergen-loaded microspheres by aleuria aurantia lectin. Vaccine (2005) 23(21):2703–10. doi: 10.1016/j.vaccine.2004.11.052
18. Roth-Walter F, Scholl I, Untersmayr E, Fuchs R, Boltz-Nitulescu G, Weissenbock A, et al. M cell targeting with aleuria aurantia lectin as a novel approach for oral allergen immunotherapy. J Allergy Clin Immunol (2004) 114(6):1362–8. doi: 10.1016/j.jaci.2004.08.010
19. Gensollen T, Blumberg RS. Correlation between early-life regulation of the immune system by microbiota and allergy development. J Allergy Clin Immunol (2017) 139(4):1084–91. doi: 10.1016/j.jaci.2017.02.011
20. Round JL, Mazmanian SK. The gut microbiota shapes intestinal immune responses during health and disease. Nat Rev Immunol (2009) 9(5):313–23. doi: 10.1038/nri2515
21. Krieg AM. Cpg motifs in bacterial DNA and their immune effects. Annu Rev Immunol (2002) 20:709–60. doi: 10.1146/annurev.immunol.20.100301.064842
22. Atarashi K, Tanoue T, Oshima K, Suda W, Nagano Y, Nishikawa H, et al. Treg induction by a rationally selected mixture of clostridia strains from the human microbiota. Nature (2013) 500(7461):232–6. doi: 10.1038/nature12331
23. de Kivit S, van Hoffen E, Korthagen N, Garssen J, Willemsen LE. Apical tlr ligation of intestinal epithelial cells drives a Th1-polarized regulatory or inflammatory type effector response in vitro. Immunobiology (2011) 216(4):518–27. doi: 10.1016/j.imbio.2010.08.005
24. Srivastava KD, Siefert A, Fahmy TM, Caplan MJ, Li XM, Sampson HA. Investigation of peanut oral immunotherapy with Cpg/Peanut nanoparticles in a murine model of peanut allergy. J Allergy Clin Immunol (2016) 138(2):536–43.e4. doi: 10.1016/j.jaci.2016.01.047
25. Hanski I, von Hertzen L, Fyhrquist N, Koskinen K, Torppa K, Laatikainen T, et al. Environmental biodiversity, human microbiota, and allergy are interrelated. Proc Natl Acad Sci U.S.A. (2012) 109(21):8334–9. doi: 10.1073/pnas.1205624109
26. Stefka AT, Feehley T, Tripathi P, Qiu J, McCoy K, Mazmanian SK, et al. Commensal bacteria protect against food allergen sensitization. Proc Natl Acad Sci U.S.A. (2014) 111(36):13145–50. doi: 10.1073/pnas.1412008111
27. Lundberg R, Bahl MI, Licht TR, Toft MF, Hansen AK. Microbiota composition of simultaneously colonized mice housed under either a gnotobiotic isolator or individually ventilated cage regime. Sci Rep (2017) 7:42245. doi: 10.1038/srep42245
28. Kang SH, Hong SJ, Lee YK, Cho S. Oral vaccine delivery for intestinal immunity-biological basis, barriers, delivery system, and m cell targeting. Polymers (Basel) (2018) 10(9):948. doi: 10.3390/polym10090948
29. Jazayeri SD, Lim HX, Shameli K, Yeap SK, Poh CL. Nano and microparticles as potential oral vaccine carriers and adjuvants against infectious diseases. Front Pharmacol (2021) 12:682286. doi: 10.3389/fphar.2021.682286
30. Krishnamachari Y, Salem AK. Innovative strategies for Co-delivering antigens and cpg oligonucleotides. Adv Drug Delivery Rev (2009) 61(3):205–17. doi: 10.1016/j.addr.2008.12.013
31. van Esch BC, Schouten B, de Kivit S, Hofman GA, Knippels LM, Willemsen LE, et al. Oral tolerance induction by partially hydrolyzed whey protein in mice is associated with enhanced numbers of Foxp3+ regulatory T-cells in the mesenteric lymph nodes. Pediatr Allergy Immunol (2011) 22(8):820–6. doi: 10.1111/j.1399-3038.2011.01205.x
32. Kostadinova AI, Middelburg J, Ciulla M, Garssen J, Hennink WE, Knippels LMJ, et al. Plga nanoparticles loaded with beta-Lactoglobulin-Derived peptides modulate mucosal immunity and may facilitate cow's milk allergy prevention. Eur J Pharmacol (2018) 818:211–20. doi: 10.1016/j.ejphar.2017.10.051
33. Campbell JD, Kell SA, Kozy HM, Lum JA, Sweetwood R, Chu M, et al. A limited cpg-containing oligodeoxynucleotide therapy regimen induces sustained suppression of allergic airway inflammation in mice. Thorax (2014) 69(6):565–73. doi: 10.1136/thoraxjnl-2013-204605
34. Xu Z, Ramishetti S, Tseng YC, Guo S, Wang Y, Huang L. Multifunctional nanoparticles Co-delivering Trp2 peptide and cpg adjuvant induce potent cytotoxic T-lymphocyte response against melanoma and its lung metastasis. J Control Release (2013) 172(1):259–65. doi: 10.1016/j.jconrel.2013.08.021
35. Pali-Scholl I, Szollosi H, Starkl P, Scheicher B, Stremnitzer C, Hofmeister A, et al. Protamine nanoparticles with cpg-oligodeoxynucleotide prevent an allergen-induced Th2-response in Balb/C mice. Eur J Pharm Biopharm (2013) 85(3 Pt A):656–64. doi: 10.1016/j.ejpb.2013.03.003
36. Schouten B, van Esch BC, Hofman GA, van den Elsen LW, Willemsen LE, Garssen J. Acute allergic skin reactions and intestinal contractility changes in mice orally sensitized against casein or whey. Int Arch Allergy Immunol (2008) 147(2):125–34. doi: 10.1159/000135699
37. Kostadinova AI, Meulenbroek LA, van Esch BC, Hofman GA, Garssen J, Willemsen LE, et al. A specific mixture of fructo-oligosaccharides and bifidobacterium breve m-16v facilitates partial non-responsiveness to whey protein in mice orally exposed to beta-Lactoglobulin-Derived peptides. Front Immunol (2016) 7:673. doi: 10.3389/fimmu.2016.00673
38. van den Elsen LW, van Esch BC, Hofman GA, Kant J, van de Heijning BJ, Garssen J, et al. Dietary long chain n-3 polyunsaturated fatty acids prevent allergic sensitization to cow's milk protein in mice. Clin Exp Allergy (2013) 43(7):798–810. doi: 10.1111/cea.12111
39. Danaei M, Dehghankhold M, Ataei S, Hasanzadeh Davarani F, Javanmard R, Dokhani A, et al. Impact of particle size and polydispersity index on the clinical applications of lipidic nanocarrier systems. Pharmaceutics (2018) 10(2):57. doi: 10.3390/pharmaceutics10020057
40. Kaszuba M, Corbett J, Watson FM, Jones A. High-concentration zeta potential measurements using light-scattering techniques. Philos Trans A Math Phys Eng Sci (2010) 368(1927):4439–51. doi: 10.1098/rsta.2010.0175
41. Sanidad KZ, Zeng MY. Neonatal gut microbiome and immunity. Curr Opin Microbiol (2020) 56:30–7. doi: 10.1016/j.mib.2020.05.011
42. Zhang K, Dupont A, Torow N, Gohde F, Leschner S, Lienenklaus S, et al. Age-dependent enterocyte invasion and microcolony formation by salmonella. PloS Pathog (2014) 10(9):e1004385. doi: 10.1371/journal.ppat.1004385
43. D'Cruz LM, Klein L. Development and function of agonist-induced Cd25+Foxp3+ regulatory T cells in the absence of interleukin 2 signaling. Nat Immunol (2005) 6(11):1152–9. doi: 10.1038/ni1264
44. Fontenot JD, Rasmussen JP, Gavin MA, Rudensky AY. A function for interleukin 2 in Foxp3-expressing regulatory T cells. Nat Immunol (2005) 6(11):1142–51. doi: 10.1038/ni1263
45. Al Nabhani Z, Dulauroy S, Marques R, Cousu C, Al Bounny S, Dejardin F, et al. A weaning reaction to microbiota is required for resistance to immunopathologies in the adult. Immunity (2019) 50(5):1276–88.e5. doi: 10.1016/j.immuni.2019.02.014
46. Weström B, Arévalo Sureda E, Pierzynowska K, Pierzynowski SG, Pérez-Cano F-J. The immature gut barrier and its importance in establishing immunity in newborn mammals. Front Immunol (2020) 11:1153. doi: 10.3389/fimmu.2020.01153
47. Dordelmann G, Kozlova D, Karczewski S, Lizio R, Knauer S, Epple M. Calcium phosphate increases the encapsulation efficiency of hydrophilic drugs (Proteins, nucleic acids) into Poly(D,L-Lactide-Co-Glycolide acid) nanoparticles for intracellular delivery. J Mater Chem B (2014) 2(41):7250–9. doi: 10.1039/c4tb00922c
48. Samadi N, van Nostrum CF, Vermonden T, Amidi M, Hennink WE. Mechanistic studies on the degradation and protein release characteristics of Poly(Lactic-Co-Glycolic-Co-Hydroxymethylglycolic acid) nanospheres. Biomacromolecules (2013) 14(4):1044–53. doi: 10.1021/bm301900t
49. Park H, Ha DH, Ha ES, Kim JS, Kim MS, Hwang SJ. Effect of stabilizers on encapsulation efficiency and release behavior of exenatide-loaded plga microsphere prepared by the W/O/W solvent evaporation method. Pharmaceutics (2019) 11(12):627. doi: 10.3390/pharmaceutics11120627
50. Heikenwalder M, Polymenidou M, Junt T, Sigurdson C, Wagner H, Akira S, et al. Lymphoid follicle destruction and immunosuppression after repeated cpg oligodeoxynucleotide administration. Nat Med (2004) 10(2):187–92. doi: 10.1038/nm987
51. Montamat G, Leonard C, Poli A, Klimek L, Ollert M. Cpg adjuvant in allergen-specific immunotherapy: Finding the sweet spot for the induction of immune tolerance. Front Immunol (2021) 12:590054. doi: 10.3389/fimmu.2021.590054
52. Wang Y, Qin B, Xia G, Choi SH. Fda's poly (Lactic-Co-Glycolic acid) research program and regulatory outcomes. AAPS J (2021) 23(4):92. doi: 10.1208/s12248-021-00611-y
53. Vasir JK, Labhasetwar V. Biodegradable nanoparticles for cytosolic delivery of therapeutics. Adv Drug Delivery Rev (2007) 59(8):718–28. doi: 10.1016/j.addr.2007.06.003
54. Koerner J, Horvath D, Groettrup M. Harnessing dendritic cells for poly (D,L-Lactide-Co-Glycolide) microspheres (Plga ms)-mediated anti-tumor therapy. Front Immunol (2019) 10:707. doi: 10.3389/fimmu.2019.00707
55. Kim KS, Suzuki K, Cho H, Youn YS, Bae YH. Oral nanoparticles exhibit specific high-efficiency intestinal uptake and lymphatic transport. ACS Nano (2018) 12(9):8893–900. doi: 10.1021/acsnano.8b04315
56. Abdulkarim M, Agullo N, Cattoz B, Griffiths P, Bernkop-Schnurch A, Borros SG, et al. Nanoparticle diffusion within intestinal mucus: Three-dimensional response analysis dissecting the impact of particle surface charge, size and heterogeneity across polyelectrolyte, pegylated and viral particles. Eur J Pharm Biopharm (2015) 97(Pt A):230–8. doi: 10.1016/j.ejpb.2015.01.023
57. Pereira de Sousa I, Moser T, Steiner C, Fichtl B, Bernkop-Schnurch A. Insulin loaded mucus permeating nanoparticles: Addressing the surface characteristics as feature to improve mucus permeation. Int J Pharm (2016) 500(1-2):236–44. doi: 10.1016/j.ijpharm.2016.01.022
58. Donahue ND, Acar H, Wilhelm S. Concepts of nanoparticle cellular uptake, intracellular trafficking, and kinetics in nanomedicine. Adv Drug Delivery Rev (2019) 143:68–96. doi: 10.1016/j.addr.2019.04.008
59. Allahyari M, Mohit E. Peptide/Protein vaccine delivery system based on plga particles. Hum Vaccin Immunother (2016) 12(3):806–28. doi: 10.1080/21645515.2015.1102804
60. Kanagaratham C, El Ansari YS, Lewis OL, Oettgen HC. Ige and igg antibodies as regulators of mast cell and basophil functions in food allergy. Front Immunol (2020) 11:603050. doi: 10.3389/fimmu.2020.603050
61. Madureira AR, Pereira CI, Gomes AMP, Pintado ME, Xavier Malcata F. Bovine whey proteins – overview on their main biological properties. Food Res Int (2007) 40(10):1197–211. doi: 10.1016/j.foodres.2007.07.005
62. Siefert AL, Caplan MJ, Fahmy TM. Artificial bacterial biomimetic nanoparticles synergize pathogen-associated molecular patterns for vaccine efficacy. Biomaterials (2016) 97:85–96. doi: 10.1016/j.biomaterials.2016.03.039
63. Castenmiller C, Keumatio-Doungtsop BC, van Ree R, de Jong EC, van Kooyk Y. Tolerogenic immunotherapy: Targeting dc surface receptors to induce antigen-specific tolerance. Front Immunol (2021) 12:643240. doi: 10.3389/fimmu.2021.643240
64. Guery L, Hugues S. Tolerogenic and activatory plasmacytoid dendritic cells in autoimmunity. Front Immunol (2013) 4:59. doi: 10.3389/fimmu.2013.00059
65. Mayer JU, Demiri M, Agace WW, MacDonald AS, Svensson-Frej M, Milling SW. Different populations of Cd11b(+) dendritic cells drive Th2 responses in the small intestine and colon. Nat Commun (2017) 8:15820. doi: 10.1038/ncomms15820
66. Li JG, Du YM, Yan ZD, Yan J, Zhuansun YX, Chen R, et al. Cd80 and Cd86 knockdown in dendritic cells regulates Th1/Th2 cytokine production in asthmatic mice. Exp Ther Med (2016) 11(3):878–84. doi: 10.3892/etm.2016.2989
67. Galvan Morales MA, Montero-Vargas JM, Vizuet-de-Rueda JC, Teran LM. New insights into the role of pd-1 and its ligands in allergic disease. Int J Mol Sci (2021) 22(21):11898. doi: 10.3390/ijms222111898
68. Unger WW, Laban S, Kleijwegt FS, van der Slik AR, Roep BO. Induction of treg by monocyte-derived dc modulated by vitamin D3 or dexamethasone: Differential role for pd-L1. Eur J Immunol (2009) 39(11):3147–59. doi: 10.1002/eji.200839103
69. Li Wang KP-L, de Vries VC, Guleria I, . Sayegh MH, . Noelle RJ. Programmed death 1 ligand signaling regulates the generation of adaptive Foxp3+cd4+ regulatory T cells. PNAS (2008) 105:9331–6.
70. Roberts A, Bentley L, Tang T, Stewart F, Pallini C, Juvvanapudi J, et al. Ex vivo modelling of pd-1/Pd-L1 immune checkpoint blockade under acute, chronic, and exhaustion-like conditions of T-cell stimulation. Sci Rep (2021) 11(1):4030. doi: 10.1038/s41598-021-83612-3
71. . Kamath AT, Henri S, Battye F, . Tough DF, Shortman. K. Developmental kinetics and lifespan ofdendritic cells in mouse lymphoid organs. Blood (2002) 100:1734–41.
72. Michael H, Li Y, Wang Y, McCusker CT. Trained immunity induced by in vivo peptide-based Stat6 inhibition prevents ragweed allergy in mice. Allergy Asthma Clin Immunol (2021) 17(1):42. doi: 10.1186/s13223-021-00542-5
73. Boraschi D, Italiani P, Palomba R, Decuzzi P, Duschl A, Fadeel B, et al. Nanoparticles and innate immunity: New perspectives on host defence. Semin Immunol (2017) 34:33–51. doi: 10.1016/j.smim.2017.08.013
74. Alvarez D, Vollmann EH, von Andrian UH. Mechanisms and consequences of dendritic cell migration. Immunity (2008) 29(3):325–42. doi: 10.1016/j.immuni.2008.08.006
75. Bertolini TB, Biswas M, Terhorst C, Daniell H, Herzog RW, Pineros AR. Role of orally induced regulatory T cells in immunotherapy and tolerance. Cell Immunol (2021) 359:104251. doi: 10.1016/j.cellimm.2020.104251
76. Tekguc M, Wing JB, Osaki M, Long J, Sakaguchi S. Treg-expressed ctla-4 depletes Cd80/Cd86 by trogocytosis, releasing free pd-L1 on antigen-presenting cells. Proc Natl Acad Sci U.S.A. (2021) 118(30). doi: 10.1073/pnas.2023739118
77. Wakim LM, Waithman J, van Rooijen N, Heath WR, Carbone FR. Dendritic cell-induced memory T cell activation in nonlymphoid tissues. Science (2008) 319(5860):198–202. doi: 10.1126/science.1151869
78. Gatto D, Wood K, Caminschi I, Murphy-Durland D, Schofield P, Christ D, et al. The chemotactic receptor Ebi2 regulates the homeostasis, localization and immunological function of splenic dendritic cells. Nat Immunol (2013) 14(5):446–53. doi: 10.1038/ni.2555
79. Umemoto E, Otani K, Ikeno T, Verjan Garcia N, Hayasaka H, Bai Z, et al. Constitutive plasmacytoid dendritic cell migration to the splenic white pulp is cooperatively regulated by Ccr7- and Cxcr4-mediated signaling. J Immunol (2012) 189(1):191–9. doi: 10.4049/jimmunol.1200802
80. Wingender G, Garbi N, Schumak B, Jungerkes F, Endl E, von Bubnoff D, et al. Systemic application of cpg-rich DNA suppresses adaptive T cell immunity Via induction of ido. Eur J Immunol (2006) 36(1):12–20. doi: 10.1002/eji.200535602
81. Baban B, Chandler PR, Sharma MD, Pihkala J, Koni PA, Munn DH, et al. Ido activates regulatory T cells and blocks their conversion into Th17-like T cells. J Immunol (2009) 183(4):2475–83. doi: 10.4049/jimmunol.0900986
82. Plantinga M, Guilliams M, Vanheerswynghels M, Deswarte K, Branco-Madeira F, Toussaint W, et al. Conventional and monocyte-derived Cd11b(+) dendritic cells initiate and maintain T helper 2 cell-mediated immunity to house dust mite allergen. Immunity (2013) 38(2):322–35. doi: 10.1016/j.immuni.2012.10.016
83. Park MJ, Park KS, Park HS, Cho ML, Hwang SY, Min SY, et al. A distinct tolerogenic subset of splenic Ido(+)Cd11b(+) dendritic cells from orally tolerized mice is responsible for induction of systemic immune tolerance and suppression of collagen-induced arthritis. Cell Immunol (2012) 278(1-2):45–54. doi: 10.1016/j.cellimm.2012.06.009
84. Schulke S. Induction of interleukin-10 producing dendritic cells as a tool to suppress allergen-specific T helper 2 responses. Front Immunol (2018) 9:455. doi: 10.3389/fimmu.2018.00455
Keywords: β-lactoglobulin, cow’s milk allergy, CpG oligodeoxynucleotides, individual ventilated cages, poly (lactic-co-glycolic acid) nanoparticles, toll-like-receptor 9, whey protein, oral pretreatment
Citation: Liu M, Thijssen S, Hennink WE, Garssen J, van Nostrum CF and Willemsen L EM (2023) Oral pretreatment with β-lactoglobulin derived peptide and CpG co-encapsulated in PLGA nanoparticles prior to sensitizations attenuates cow’s milk allergy development in mice. Front. Immunol. 13:1053107. doi: 10.3389/fimmu.2022.1053107
Received: 25 September 2022; Accepted: 19 December 2022;
Published: 06 January 2023.
Edited by:
Caterina Vizzardelli, University of Vienna, AustriaReviewed by:
Isabella Pali-Schöll, University of Veterinary Medicine Vienna, AustriaNicholas A. Smith, Alkahest, United States
Copyright © 2023 Liu, Thijssen, Hennink, Garssen, van Nostrum and Willemsen. This is an open-access article distributed under the terms of the Creative Commons Attribution License (CC BY). The use, distribution or reproduction in other forums is permitted, provided the original author(s) and the copyright owner(s) are credited and that the original publication in this journal is cited, in accordance with accepted academic practice. No use, distribution or reproduction is permitted which does not comply with these terms.
*Correspondence: Linette E. M. Willemsen, bC5lLm0ud2lsbGVtc2VuQHV1Lm5s