- 1School of Pharmacy and Biomedical Sciences, University of Central Lancashire, Preston, United Kingdom
- 2Laboratory on Thymus Research, Oswaldo Cruz Foundation, Oswaldo Cruz Institute, Rio de Janeiro, Brazil
- 3National Institute of Science and Technology on Neuroimmunomodulation (INCT-NIM), Oswaldo Cruz Institute, Oswaldo Cruz Foundation, Rio de Janeiro, Brazil
- 4Rio de Janeiro Research Network on Neuroinflammation (RENEURIN), Oswaldo Cruz Institute, Oswaldo Cruz Foundation, Rio de Janeiro, Brazil
- 5Laboratory of Molecular Psychiatry, Centro de Pesquisa Experimental (CPE) and Centro de Pesquisa Clínica (CPC), Hospital de Clínicas de Porto Alegre (HCPA), Porto Alegre (RS), Brazil
- 6Division of Biomedical and Life Sciences, Faculty of Health and Medicine, Lancaster University, Lancaster, United Kingdom
Autism spectrum disorder (ASD) is a heterogeneous neurodevelopmental disorder characterised by stereotyped behaviours, specific interests, and impaired communication skills. Elevated levels of pro-inflammatory cytokines, such as interleukin-17A (IL-17A or IL-17), have been implicated as part of immune alterations that may contribute to this outcome. In this context, rodent models have helped elucidate the role of T-cell activation and IL-17 secretion in the pathogenesis of ASD. Regarding the preclinical findings, the data available is contradictory in offspring but not in the pregnant dams, pointing to IL-17 as one of the main drivers of altered behaviour in some models ASD, whilst there are no alterations described in IL-17 levels in others. To address this gap in the literature, a systematic review of altered IL-17 levels in rodent models of ASD was conducted. In total, 28 studies that explored IL-17 levels were included and observed that this cytokine was generally increased among the different models of ASD. The data compiled in this review can help the choice of animal models to study the role of cytokines in the development of ASD, seeking a parallel with immune alterations observed in individuals with this condition.
Systematic Review Registration: PROSPERO, identifier CRD42022306558.
Introduction
Autism spectrum disorder (ASD) is a complex, heterogeneous neurodevelopmental disorder characterised by restricted patterns of repetitive behaviour, activities, interests and impairments in social skills and communication (1). In Europe, the prevalence was 0.38% in children aged 4 years and 1.55% in children aged 8 years, while in the USA, the prevalence ranged between 1.70% and 1.85%, respectively (2). ASD is also etiologically heterogeneous, and its precise mechanism remains undefined. However, studies have suggested that it results from a combination of immunological, environmental, and genetic factors which can affect brain development and synaptic plasticity (3).
There are currently no biomarkers identified for ASD as its diagnosis is mainly based on clinical assessments (4). However, increasing evidence suggests that an aberrant immune phenotype may be linked to the development of ASD (5). Elevated levels of inflammatory cytokines, such as IL-6, TNF-α, IFN-γ, IL-1β, and IL-12, have been detected in several tissues of individuals with ASD, such as serum, plasma, and brain (6, 7). Elevations in these cytokine levels have been associated with increased stereotypical behaviours, increased aberrant behavioural scores, increased impaired neurodevelopment, and a regressive form of ASD (8, 9). Further studies have also observed increased concentrations of pro-inflammatory cytokines in the amniotic fluid of mothers whose children developed ASD, supporting the role of increased immune activity in ASD development (10, 11).
Another pro-inflammatory cytokine implicated in ASD is IL-17 (12). IL-17 comprises a family of six structurally related cytokines that includes IL-17A (commonly referred to as IL-17), IL-17B, IL-17C, IL-17D, IL-17E, and IL-17F (12). Although IL-17 is well known for its role in pathogenic responses, physiological effects of IL-17 include maintenance of mucosal integrity (13).
Elevated levels of IL-17 have been described in the serum of children with ASD (14), and enrichment in IL-17 genes was reported in individuals with ASD, indicating a possible role for this cytokine in the pathophysiology of this condition (15). Data suggesting the involvement of IL-17 in the development of ASD is also supported by findings in preclinical models of maternal immune activation (MIA) that linked the upregulation of IL-17 during pregnancy to the development of ASD-like behaviours (16).
Animal models have provided ground-breaking data in investigating the relationship between features related to ASD and altered IL-17 levels. It has been demonstrated that inducible models such as MIA by injection of polyinosinic:polycytidylic acid (poly(I:C)) or lipopolysaccharide (LPS), displayed elevated levels of IL-17 in maternal serum followed by upregulation of IL17Ra in foetal brains (16, 17). However, IL-17 levels in foetal, postnatal, and adult offspring of inducible or genetic models of ASD display conflicting data (18–20).
Therefore, a systematic review of data reporting altered IL-17 levels in rodent models of ASD was conducted. We aimed to answer the following questions (1): Are there IL-17 aberrations across rodent models of ASD? (2); If there are IL-17 aberrations across models, what is the magnitude of the difference? (3); Do these IL-17 aberrations differ across the age of rodent models?
Methods
Study Selection
Seven electronic databases were utilised for this systematic review: Web of Science, PubMed, BMC, Scopus, SciELO, Proquest Dissertations and Theses — Global, and British Library EthOS. SciELO (South America) was included to address English language bias. The search terms used and the number of records found in each electronic database can be seen in the Supplementary Material (see Supplementary Table 1).
We included records containing mouse or rat models of ASD that fitted our inclusion criteria, presenting ASD-like phenotypes and IL-17 analysis. We included all records regardless of the sex or age of the animals. Furthermore, we added all records published between January 1st, 2000, and January 1st, 2022.
We excluded studies that did not analyse IL-17 levels and were not original research studies. Additionally, review articles, posters, and abstracts from conferences, case studies, and retracted articles were excluded.
Data Extraction, Synthesis, and Quality Assessment
Three reviewers (AJT, LPV, and FDRP) independently screened abstracts, assessed full-text articles, extracted data, and evaluated the risk of bias. Disagreements and inconsistencies were resolved by the consensus of all review group members. The study search strategy is outlined by the Preferred Reporting Items for Systematic Reviews and Meta-Analyses (PRISMA) guidelines. The study is registered on PROSPERO (CRD42022306558).
Data extracted included the type of mouse or rat model, IL-17 alterations, intervention characteristics (i.e. dosing regimen for MIA models), the age, and sex of the animals. Studies were assessed using the CAMARADES’ study quality checklist criteria adapted as follows (21): peer-reviewed publication; random allocation to treatment or control; appropriate animal model (MIA, chemically induced, inbred strain); sex-matched animals; age-matched animals; sample size calculation; compliance with animal welfare regulations; and statement of potential conflict of interests. Each study was given a quality score out of eight points. These criteria were chosen to identify the overall methodological quality of those records.
Furthermore, by amending the quality checklist to include age and sex match animals, this review seeks to ensure that appropriate comparisons between control and treatment groups were made. It is possible that cytokine aberrations in ASD models significantly differ across age and sex and ensuring that animals were age and sex-matched may reduce this bias (Table 1).
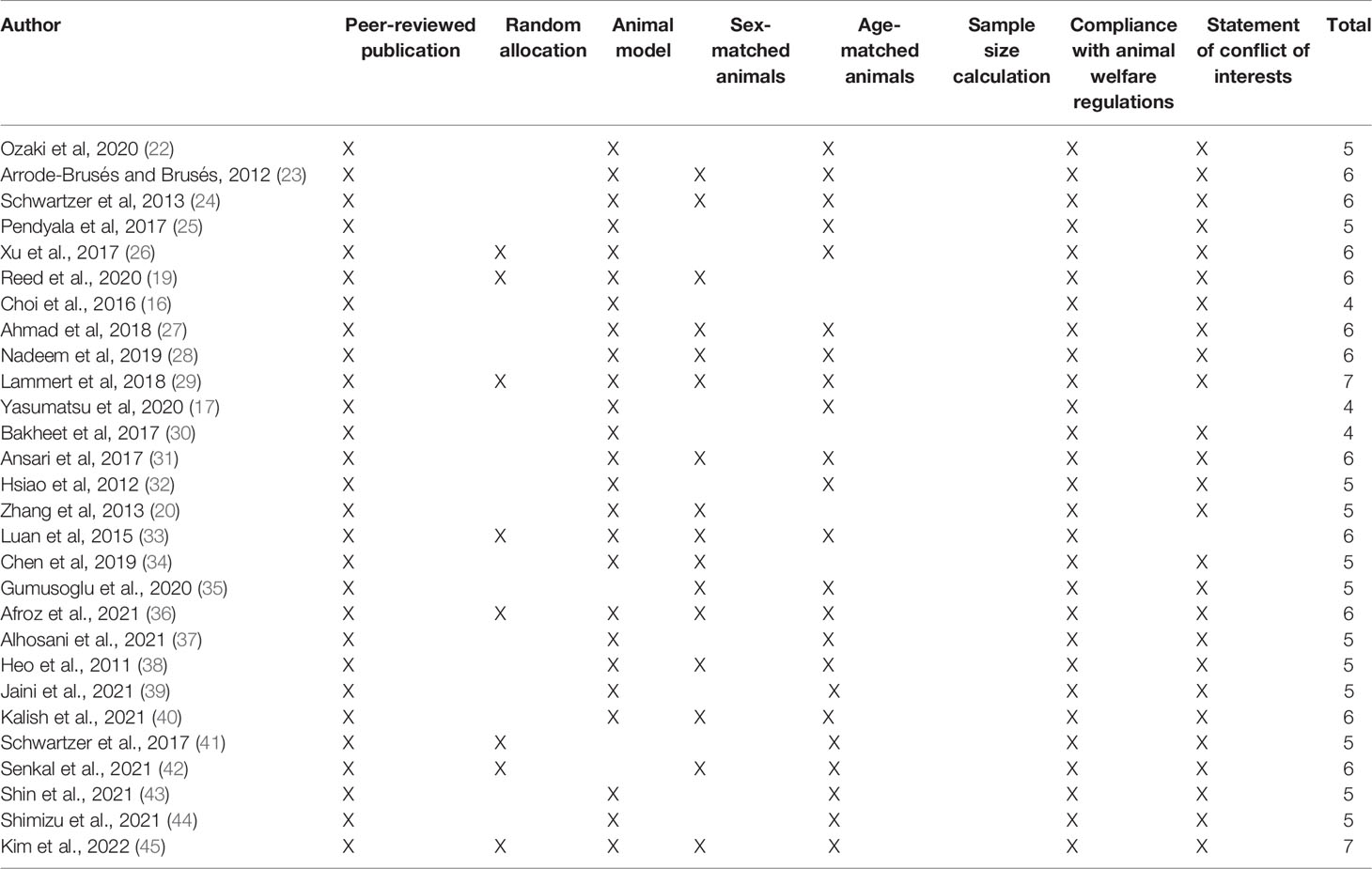
Table 1 Bias assessment and study quality using animal data from experimental studies (CAMARADES) checklist items.
One reviewer (AJT) assessed the risk of bias and included records using the Systematic Review Centre for Laboratory Animal Experimentation (SYRCLE) (46). This risk of bias tool assessed methodological quality using animal study-specific criteria and was adapted (46) from the Cochrane Risk of Bias tool. For each included study, the parameters for each type of bias were marked as Yes (Y), No (N), or Unclear (U). A final score out of 10 was given to each study (Table 2).
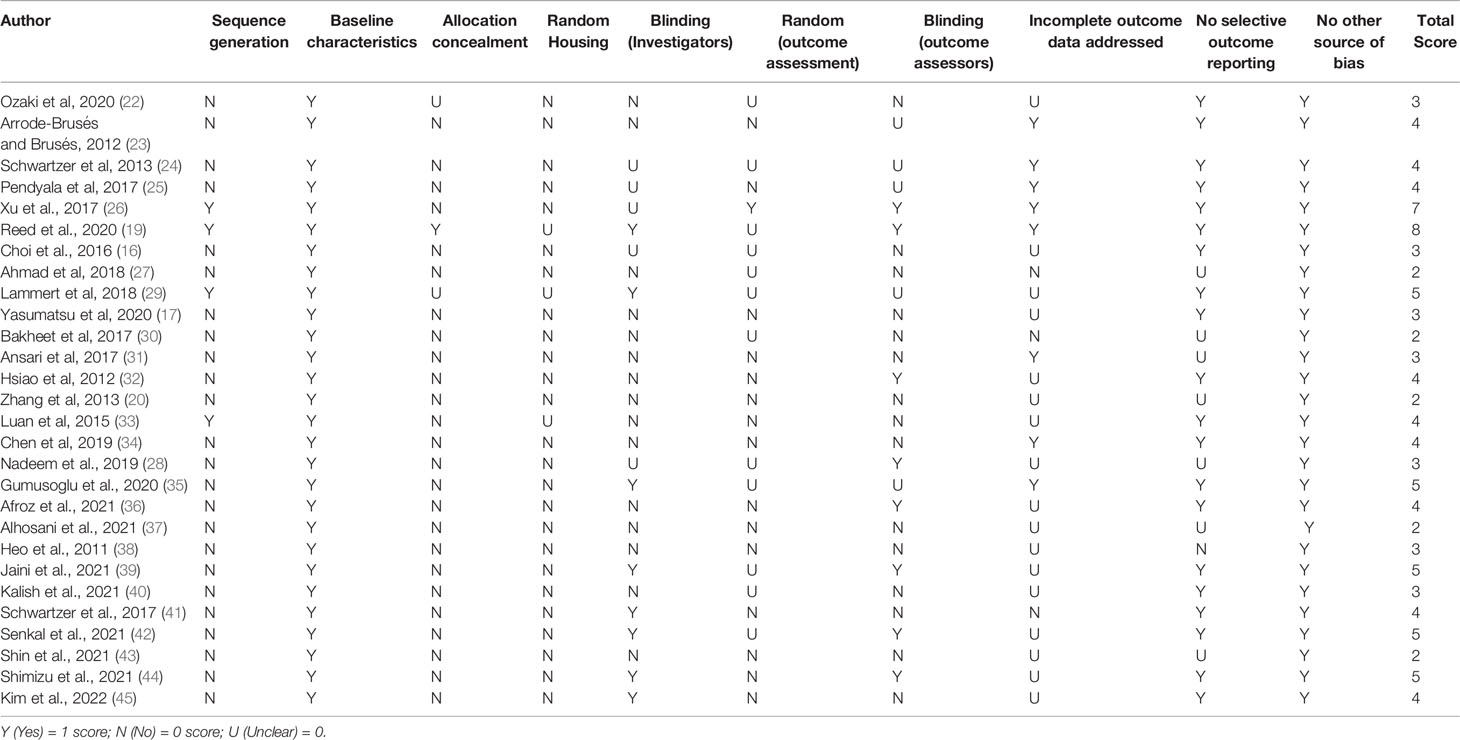
Table 2 Systematic Review Centre for Laboratory animal Experimentation (SYRCLE) scale for risk of bias.
Results
Study Selection
A total of 643 records were obtained from the database search (see Supplementary Table 1). Following duplicate removal, we screened 630 records, and 28 studies met inclusion criteria (Figure 1). All these articles were considered appropriate and were included in this review. Among them, two articles - Chen et al., 2019 and Ozaki et al. (22, 34) - were added using a snowballing technique as they fitted the inclusion criteria but did not show up in the total searches and one article - Kim et al. (45) - was included as it met the inclusion criteria and was published in January, 11 2022. A total of 28 papers were analysed in this review.
Only two studies using rat models matched the criteria for this review (26, 42). Many of the studies generated by the database searches focused on mouse models of ASD. This indicates a data gap and raises the question of if mouse models have better mechanical viability when modelling immune aberrations of ASD compared to rat models; however, this may also be due to the broader availability of mouse cytokine evaluation kits and the prevalence of mouse models in immunology.
Quality Assessment
According to CAMARADES’ quality scale, the 28 records included articles had a mean score of 5.42 ± 0.77 (mean ± standard deviation) out of a possible 8 (Table 1). All papers were published in peer-reviewed journals, and most used models of ASD. Most records used age and sex-matched animals. However, some articles were unclear on whether female rodents were included. This may affect the results obtained since sex-biased alterations of IL-17 in rodent models of ASD is unknown. Furthermore, none of the articles showed the sample size calculations, further affecting the results. Also, the allotment of treatment groups, the use of different litters, and the number of pups per litter were not mentioned. Nevertheless, all articles complied with animal welfare regulations, and many articles included disclosure of potential conflicts of interest.
The assessment of the risk of bias can be seen in Table 2. The mean SYRCLE score among all included records was 3.82 ± 1.41. Of the 28 studies included, no papers randomly housed the animals within the animal unit. Most of the records did not blind investigators, and similarly, only 9 papers utilised blinded outcome assessors. In addition, many of the studies did not appropriately generate and apply a sequence generation to the animals used. One study completed a random outcome assessment (26). Similarly, only 8 studies addressed incomplete data.
Furthermore, only 1 of the studies included in this review, Reed et al. (19), concealed the allocation of control and treatment groups (19). All papers had no other apparent sources of bias when reviewed with the SYRCLE guidelines. All studies also established the baseline characteristic of control IL-17 levels compared with ASD rodent models.
Qualitative Synthesis
Altered IL-17 in MIA Models
A total of 16 studies identified from the database search utilised MIA models of ASD, and all of them described alterations in IL-17 levels (16, 17, 19, 22–26, 29, 32, 33, 35, 41, 42, 44) (Table 3). Most of the papers used one of two different protocols of inducing MIA, either utilising an intraperitoneal (i.p.) injection of 20 mg/kg of poly(I:C), or an injection of 0.05 mg/kg of LPS, at embryonic day (E) 12.5. One study used a subcutaneous osmotic pump releasing IL-17 at a rate of 0.025 mg/kg per hour to simulate chronic inflammation (35). A further study induced the MIA model through sensitisation with 10µg of ovalbumin to mimic chronic maternal allergic asthma in C57BL/6 and FVB mice, which develop mild and a more severe asthma respectively (41). Out of the 15 studies, 13 reported increases in IL-17 levels, with one article reporting no alteration in IL-17 production compared to BTBR mice (a well-established model of ASD) (24) and a second report noting a significant decrease in IL-17 at postnatal day 30 (25). A further study found that IL-17 levels decreased at 48 hours post injection (44). IL-17 was assessed at different ages throughout the studies, from foetal to adult (15 weeks old) and in both offspring and dams. When comparing results for each age point, much of the data obtained for each age was similar. There was also a variety of tissues used, the most frequent being offspring serum, brain, kidney, spleen, maternal serum, placenta, and uterus. One study examined IL-17 secretion in lymphocytes from the lamina propria derived from colon tissue (45). Many of the papers that used the same tissue types for the analysis reported similar IL-17 alterations. The studies utilised a variety of techniques, including ELISA, flow cytometry, cytometric bead array, and real-time PCR to assess and infer IL-17 levels.
Altered IL-17 in Chemically Induced Models
One study investigated if exposure to teratogens during pregnancy could induce similar alterations to the well-established model of maternal activation poly(I:C) (34). In this case, pregnant C57BL/6 mice were exposed to the polybrominated diphenyl ether (PBDE) 209 and lead (Pb), common pollutants that co-exist in the environment. The study suggested that the perinatal exposure to the mixture of PBDE209 and Pb elicited restricted, repetitive patterns of behaviour and affected learning in male offspring, and IL-17 levels were increased in the serum of mice at postnatal day 30 (34). However, social behaviour was not impaired after chemical exposure, differently from the observed in the poly(I:C) model (34). This suggests that although not all behavioural alterations typically associated with animal models of ASD were induced by these teratogens, neurobehavioral alterations were still present and therefore presenting relevant validities as a model. A further study found that neonatal and P1 pups from dams treated with valproic acid to induce chronic immune response had significantly higher IL-17A in both the skin and brain. These IL-17A increases were also correlated to ASD-like features, as examined through the Morris Water Maze (43).
Altered IL-17 in Inbred Models
A total of 8 studies utilised inbred rodent models of ASD, and 6 of them found alterations in IL-17 concentrations (20, 24, 27, 28, 30, 31). All papers used the BTBR mice, with five of the eight papers only using males. However, some studies used a smaller age range of mice than the papers that used the MIA models, with a range of 6-13 weeks. Out of the eight studies that observed IL-17 aberrations, all found a significant increase in IL-17 intracellular levels and mRNA expression (Table 3). One paper found a significant increase in IL-17A intracellular staining in T CD4+ cells from the spleen, as well as increase in IL-17A mRNA expression and protein expression in brain tissue when compared to C57BL/6 mice (37).
A 2011 study by Heo et al. found that 3 days following infection with Keyhole limpet haemocyanin (KLH) and Listeria monocytogenes, BTBR mice had significantly increased levels of IL-17 in the liver when compared to C57BL/6 mice. However, the data regarding IL-17 alterations found in this study is not shown, making it difficult to fully understand and evaluate the immune alterations related to IL-17 (38).
Interestingly, BTBR mice, which present higher basal levels of IL-17 when compared to controls, were described to increase these levels even further when exposed to perinatal poly(I:C), suggesting that BTBR mice may have a dysregulated cytokine response that could impact the ASD-like behavioural alterations (24).
All 8 studies utilised similar tissues including the spleen and brain, mostly focusing on investigating intracellular levels of IL-17 in splenocytes from BTBR mice, as well as IL-17 expression in brain tissue. Techniques varied from ELISA assays, flow cytometry, Western blotting, and real-time PCR to assess IL-17 concentrations.
Altered IL-17 in Genetic Models
A total of 2 studies utilised genetically modified rodent model of ASD (19, 39). Reed et al. (19) utilised mice models in which the animals harbour mutations in genes related to an increased chance of ASD, including contactin-associated protein-like 2 (Cntnap2), fragile X mental retardation-1 (Fmr1), and multiple ankyrin repeat domains 3 (Shank3) genes, for comparison with environmental ASD model such as MIA. This paper showed that inflammatory response caused by LPS injection on offspring born to mothers injected with poly(I:C) at embryonic day (E)12.5 (MIA-offspring) rescued social behaviour deficits, and that this LPS-induced rescue is dependent on IL-17A. However, this same result was not observed in the monogenic models of ASD, suggesting that differences in cytokine production across models are instrumental to the behavioural rescue observed. This hypothesis was further supported since after LPS injection both (a) the monogenic mice did not display an increase in plasmatic IL-17A and (b) IL-17A blockage in the MIA-offspring mice prevented the sociability rescue (19).
Jaini et al. (39) utilised PtenWT/m3m4 mouse dams to breed 2 offspring genotypes, PtenWT/m3m4 and PtenWT/WT, which were then bred to produce offspring. PTEN mutations are associated with ASD, accounting for 2% of all ASD and 17% of macrocephalic-ASD cases (39). Inflammatory markers, cellular phenotypes and gene expression were examined in the dams and offspring via multiplex ELISA, multiplex bead-based cytokine assays and gene expression panel analysis on E17.5. This paper found no IL-17A transcripts in the spleen from either mouse genotype used in this study and no differences in IL-17A in the serum were found (39).
Altered IL-17 in High Salt Diet Model
A total of 2 papers used a model in which animals were fed diets that included parental high salt consumption in an attempt to induce the development of ASD-like characteristics in the offspring. Afroz et al. (2021) used 8% NaCl in chow and an additional 1% NaCl in drinking water for male and female C57BL/6 mice for 8 weeks, after this period of time animals were used for breeding (36). IL-17A was detected on maternal serum without any alterations as compared to controls using flow cytometry-based assay (36). Another study exposed pregnant Sprague-Dawley rats to three different hypertonic solutions, including 3% NaCl, mineral water (3% NaHCO3+magnesium+calcium content) and Ayran (0.8% NaCl content) (42). Pregnant rats were exposed to hypertonic solutions throughout the pregnancy (21 days) via oral gavage at a dose of 5 mL/kg with dosing repeated up to 3 times within 24 h period (42). IL-17A increase was detected in the brain homogenate from male offspring of hypertonic solution fed-rats via ELISA kit (42).
Discussion
To our knowledge, this is the first systematic review assessing IL-17 levels in rodent models of ASD. Our findings show that IL-17 levels are increased in different tissues and in the serum of ASD rodent models, as reported by 23 out of 28 studies included in this review. Also, increased IL-17 levels could be observed in pregnant mice, embryos, and offspring, highlighting the possible role of this cytokine during neurodevelopment, pathogenesis and pathophysiology of ASD, as well as in behavioural alterations.
This review has also highlighted the potential role of IL-17 in the establishment of ASD-like behaviours across rodent models with a specific focus on offspring and maternal IL-17 levels. The studies examined in this review have demonstrated the role of the maternal immune state and the increase in IL-17 production in the development of ASD-like features. Several papers stressed the importance of the maternal immune state and how it relates to microbiota (29, 45), genetics (39), and nutrition (36).
Several papers have suggested the important role of homeostatic maternal immune state is and the development of ASD (11, 16, 22). Whereas other studies found increased pro-inflammatory cytokine levels in the amniotic fluid of mothers whose children develop ASD, including IL-6, a cytokine necessary for the differentiation of Th17 cells (10, 47).
As previously discussed in this review, much of the increased IL-17 production appeared to be derived from Th17 cells (27, 28, 30, 31). However, another possible producer of IL-17 in ASD models seems to be γδ T cells. Increased concentrations of IL-17 producing γδ T cells were observed in the uterus of pregnant mice after MIA (17). Previous data have shown that residential IL-17 producing γδ T cells in the meninges of the brain can control anxiety-like behaviour in mice via IL-17 receptor A signalling (18, 48). Other studies found that increased meningeal γδ T cells reduce synaptic density via IL-17 pathways (48). Taken together, these findings suggest that whilst the increase of uterine γδ T cells may induce a systemic increase of IL-17 in pregnant mice, the increase may cause a localised inflammatory response in the brain of their offspring, which may affect synaptic density (48). However, uterine γδ T cells may not be the only source of upregulated IL-17. Kim et al. (49) have demonstrated that T cells isolated from gut connective tissue of poly(I:C) treated mice expressed increased levels of IL-17A when compared to tissue from PBS treated mice (49). This study offers insightful data relating to the role of the gut in ASD mouse models. This study, however, did not match the inclusion criteria and was not included in the present systematic review.
In the gut, another factor potentially playing a role in the development of ASD-like features is maternal microbiota. Lammert et al. (29) found that C57BL/6 mice from Taconic Biosciences (Tac) displayed higher levels of IL-17A after poly(I:C) injections when compared to C57BL/6 Jackson Laboratory’s (JAX) mice. They also presented a high relative abundance of segmented filamentous bacteria (SFB), known to promote IL-17A inflammatory response (29). Offspring from poly(I:C) treated Tac mice displayed ASD-like behavioural alterations. However, those alterations were not observed in saline controls or in the offspring of JAX mice. Additionally, experiments of co-housing were able to induce behavioural alterations in JAX mice offspring and led to an increase in IL-17A in maternal serum after poly(I:C) injection, further relating the role of the gut microbiota in the production and regulation of IL-17 and Th17 cells (49). Those findings are also supported by Choi et al. (16), who demonstrated that upregulation of IL-17A after poly(I:C) led to abnormal cortical development in the offspring as well as ASD-like altered behaviour (16). Those alterations were reversed after pre-treatment with IL-17A blocking antibodies, reinforcing the hypothesis that altered maternal IL-17A response plays an important role in the development of ASD in the offspring (16). Furthermore, data from Kim et al. (2022) (45) have demonstrated that MIA immune alterations observed in the offspring may happen postnatally, as crossfostering pups from PBS-treated mothers with MIA mothers led to an increase in the percentage of colonic IL-17A and IFN- γ -producing Th17 cells in the pups after infection (37). This immune priming seems to be related to the maternal microbiota as experiments with stool transfer have demonstrated that offspring from animals that received stool transfer from MIA-mothers displayed pro-inflammatory immune phenotype after immune challenge (45).
Clinical evidence also supports that microbiota plays a role in the pathogenesis and development of ASD. It has been demonstrated that children with ASD displayed a decrease in anti-inflammatory Faecalibacterium bacteria and an increase in the pathogenic inflammatory Enterobacteriaceae and Sutterellaceae bacteria in the faecal microbiome (50). A further study found that an oral administration of the endogenous human micro-organism, B. fragilis, improved the behavioural ASD-like features in mouse models as well as metabolite modulation in the gut (51).
In line with alterations induced by maternal microbiota, data resulting from altered diet and the development of ASD-like features in the offspring has also been debated (36, 42). Previous reports have demonstrated that excess dietary salt leads to cognitive impairment, increased Th17 differentiation in the intestine and IL-17 plasmatic levels in mice (52). This impairment is dependent on lymphocytes, as it was not observed in mice lacking IL-17 or Rag1−/− mice (52). However, Afroz et al. (2021) have demonstrated that a high salt diet (HSD) did not induce any behavioural or immune alteration in HSD-fed mice. Furthermore, behaviour alterations were present in the offspring of HSD-fed mice, including altered social behaviour and increased repetitive behaviours (36, 52). In another paper analysed in this review, Senkal et al. (2021) (42) have demonstrated that male offspring rats from animals exposed to hypertonic solutions during pregnancy displayed a pro-inflammatory immune profile in brain tissue with increased IL-17 as well as ASD-like behaviours, reinforcing the hypothesis that maternal state in regards to diet and microbiota may play a role in the development of ASD-like characteristics in the offspring (42).
The kinetics of induction of MIA may play a pivotal role in the development and severity of ASD-like features in rodent models, with alteration of IL-17 levels being associated with specific points of synaptogenesis (25). Synaptic genes were also enriched in the brains of the offspring (35), suggesting a link between the MIA induction time point, maternal and foetal IL-17 levels, and synaptic development, which may induce autistic-like behaviour and affect the immune phenotype (53, 54). However, further studies are necessary to confirm the association between the aberrant IL-17 levels and unregulated synapses seen in ASD.
ASD has a strong genetic component, as demonstrated by high heritability amongst siblings (55). Another evidence for the genetic impact of ASD comes from single gene alterations linked with developmental disorders such as mutations in FMR1 (fragile X syndrome), MECP2 (Rett syndrome), TSC1/TSC2 (tuberous sclerosis complex), and CACNA1C (Timothy syndrome), as well as mutations in CNTNAP2 (cortical dysplasia–focal epilepsy syndrome) (55, 56). Animal models with single gene alterations allow for the understatement of how those mutations play a role in the development of the core characteristics of ASD, such as behavioural alterations (56). However, as it was described by Reed et al. (19), animal models for Cntnap2, Fmr1, and Shank3 did not display any IL-17 alteration after immune challenge with LPS as compared to the MIA-offspring model, indicating that immune alterations may be related to environmental factors or alteration in several genes (19). In this regard, Jaini et al. 2021 (39) hypothesised that maternal genetics may be an important modulator of neuro-immune alterations in the offspring and using the mice model for mutation in PTEN (PtenWT/m3m4), which has also been associated with ASD, set to investigate the influence of maternal genetics on ASD development in offspring (39). However, no IL-17A transcription was detected on the spleen of pregnant mice, nor any difference in the cytokine was detected in serum (39). Nevertheless, the authors demonstrated that low IL-10 during pregnancy was directly correlated with decreased complement expression in the foetal liver and offspring from mutant mice also displayed altered social and repetitive behaviours without external immune insult (39).
Several limitations should be highlighted when describing our analyses. We were not able to perform a meta-analysis due to the high methodological heterogeneity among studies, including diverse outcomes, distinct species, experimental designs, and types of animal models. Most of the studies analysed in this systematic review used male mice or did not explicitly examine the differences between the sexes. Although this is understandable due to the increased prevalence of ASD in male individuals, excluding one sex can hinder the translational validity of the results (57, 58). The exclusion of female-specific results could potentially lead to a data gap regarding immune aberrations in females, which could affect research and potential future treatments.
Another aspect that could affect IL-17 levels is receptor desensitisation due to persistent stimulation (59). Further studies are necessary to examine how IL-17 receptors could be altered in rodent models of ASD and how their altered signalling can affect pathways dependent on IL-17, such as mRNA stabilisation via the ACT1 dependent pathway (60).
IL-17 production and the effects of IL-17 have been noted to change with age within the papers examined in this review. The study by Pendyala et al. showed that although IL-17 was altered at all stages of development, the aberration was only significant at postnatal day 30 when IL-17 was decreased (25). Equivalent results were found with IL-17 concentrations significantly up-regulated by MIA after poly(I:C) exposure at gestational day 17 followed by lower levels at postnatal day 5 in the study by Arrode-Brusés and Brusés (2012) (23). These results suggest that MIA primes offspring’s immune system for a pro-inflammatory response following secondary exposure to poly(I:C), and IL-17 levels may be altered with age. However, this requires further exploration in both clinical and preclinical settings.
Additionally, the technique used to measure cytokine levels, which can changed between the studies, may play a role in the observed outcome. While some methods used in the papers, i.e. ELISA and flow cytometry, measure IL-17 protein levels, qRT-PCR measures IL-17 mRNA levels. Many of the studies that utilised qRT-PCR also included a technique to measure the IL-17 protein concentration, such as in a study where ELISA assays and flow cytometry were used to measure protein IL-17 and IL-17 producing cells alongside RT-PCR (16). One study confirmed the presence of elevated levels of IL-17 in BTBR mice by intracellular staining. However, they did not provide the data demonstrating such increase, mainly pointing out that even though there were elevated levels of IL-17, there was no differentiation into a Th17 profile (20). It is difficult to understand the full scope of IL-17 alterations in the model used in this work, as some data was not shown in the article; this absence of data also makes it difficult to incorporate the findings of this article in a meta-analyses. In despite of that, the study was included in this systematic review as it met all inclusion criteria.
In summary, this systematic review identified common alterations in IL-17 across different models of ASD and almost the totality of the paper reported increases in the level of IL-17 or in IL-17 producing cells, across different detection techniques. The selection of the papers and parameters analysed may help the choice of animal models to study the role of IL-17 and other cytokines in the development of ASD and/or behavioural alterations. It is important to notice that, although the results were consistent, there is a need for further characterisation of the meaning of IL-17 increase, and its importance to the pathophysiology of ASD.
Data Availability Statement
The original contributions presented in the study are included in the article/Supplementary Material. Further inquiries can be directed to the corresponding authors.
Author Contributions
Conception and design of the work: AT, DM-d-C, VB-J, IR. Acquisition, analysis, and interpretation of data for the work: AT, LV, and FR-d-P. Drafting of the work: AT, LV, and FR-d-P. Revision: FR-d-P, DM-d-C, IR, VB-J. Final approval of the version to be published: DM-d-C, IR, VB-J. All authors contributed to the article and approved the submitted version.
Funding
This study was supported by the University of Central Lancashire, UK; the Oswaldo Cruz Foundation, the National Institute of Science and Technology on Neuroimmunomodulation (INCT-NIM, CNPq), the Rio de Janeiro Research Network on Neuroinflammation (RENEURIN - Faperj), Brazil; the MercoSur Fund for Structural Convergence (FOCEM); and the ERASMUS+ programme.
Conflict of Interest
The authors declare that the research was conducted in the absence of any commercial or financial relationships that could be construed as a potential conflict of interest.
Publisher’s Note
All claims expressed in this article are solely those of the authors and do not necessarily represent those of their affiliated organizations, or those of the publisher, the editors and the reviewers. Any product that may be evaluated in this article, or claim that may be made by its manufacturer, is not guaranteed or endorsed by the publisher.
Acknowledgments
The authors thank their institutions and funding agencies/programs, for providing the support needed for this work.
Supplementary Material
The Supplementary Material for this article can be found online at: https://www.frontiersin.org/articles/10.3389/fimmu.2022.874064/full#supplementary-material
References
1. American Psychiatric Association. Diagnostic and Statistical Manual of Mental Disorders (DSM-5®). 5th ed. Arlington, VA, American Psychiatric Pub (2013). 991 p.
2. Bougeard C, Picarel-Blanchot F, Schmid R, Campbell R, Buitelaar J. Prevalence of Autism Spectrum Disorder and Co-Morbidities in Children and Adolescents: A Systematic Literature Review. Front Psychiatry (2021) 12:744709. doi: 10.3389/fpsyt.2021.744709
3. Lyall K, Croen L, Daniels J, Fallin MD, Ladd-Acosta C, Lee BK, et al. The Changing Epidemiology of Autism Spectrum Disorders. Annu Rev Public Health (2017) 38:81–102. doi: 10.1146/annurev-publhealth-031816-044318
4. Masi A, Glozier N, Dale R, Guastella AJ. The Immune System, Cytokines, and Biomarkers in Autism Spectrum Disorder. Neurosci Bull (2017) 33:194–204. doi: 10.1007/s12264-017-0103-8
5. Wei H, Alberts I, Li X. Brain IL-6 and Autism. Neuroscience (2013) 252:320–5. doi: 10.1016/j.neuroscience.2013.08.025
6. Siniscalco D, Schultz S, Brigida AL, Antonucci N. Inflammation and Neuro-Immune Dysregulations in Autism Spectrum Disorders. Pharmaceuticals (Basel) (2018) 11(2):56. doi: 10.3390/ph11020056
7. Depino AM. Peripheral and Central Inflammation in Autism Spectrum Disorders. Mol Cell Neurosci (2013) 53:69–76. doi: 10.1016/j.mcn.2012.10.003
8. Ashwood P, Nguyen DV, Hessl D, Hagerman RJ, Tassone F. Plasma Cytokine Profiles in Fragile X Subjects: Is There a Role for Cytokines in the Pathogenesis? Brain Behav Immun (2010) 24:898–902. doi: 10.1016/j.bbi.2010.01.008
9. Estes ML, McAllister AK. Immune Mediators in the Brain and Peripheral Tissues in Autism Spectrum Disorder. Nat Rev Neurosci (2015) 16:469–86. doi: 10.1038/nrn3978
10. Goines PE, Croen LA, Braunschweig D, Yoshida CK, Grether J, Hansen R, et al. Increased Midgestational IFN-γ, IL-4 and IL-5 in Women Bearing a Child With Autism: A Case-Control Study. Mol Autism (2011) 2:13. doi: 10.1186/2040-2392-2-13
11. Abdallah MW, Larsen N, Mortensen EL, Atladóttir HÓ, Nørgaard-Pedersen B, Bonefeld-Jørgensen EC, et al. Neonatal Levels of Cytokines and Risk of Autism Spectrum Disorders: An Exploratory Register-Based Historic Birth Cohort Study Utilising the Danish Newborn Screening Biobank. J Neuroimmunol (2012) 252:75–82. doi: 10.1016/j.jneuroim.2012.07.013
12. Amatya N, Garg AV, Gaffen SL. IL-17 Signaling: The Yin and the Yang. Trends Immunol (2017) 38:310–22. doi: 10.1016/j.it.2017.01.006
13. Li X, Bechara R, Zhao J, McGeachy MJ, Gaffen SL. IL-17 Receptor–Based Signaling and Implications for Disease. Nat Immunol (2019) 20:1594–602. doi: 10.1038/s41590-019-0514-y
14. Suzuki K, Matsuzaki H, Iwata K, Kameno Y, Shimmura C, Kawai S, et al. Plasma Cytokine Profiles in Subjects With High-Functioning Autism Spectrum Disorders. PloS One (2011) 6:e20470. doi: 10.1371/journal.pone.0020470
15. van der Zwaag B, Franke L, Poot M, Hochstenbach R, Spierenburg HA, Vorstman JAS, et al. Gene-Network Analysis Identifies Susceptibility Genes Related to Glycobiology in Autism. PloS One (2009) 4:e5324. doi: 10.1371/journal.pone.0005324
16. Choi GB, Yim YS, Wong H, Kim S, Kim H, Kim SV, et al. The Maternal Interleukin-17a Pathway in Mice Promotes Autism-Like Phenotypes in Offspring. Science (2016) 351:933–9. doi: 10.1126/science.aad0314
17. Yasumatsu K, Nagao J-I, Arita-Morioka K-I, Narita Y, Tasaki S, Toyoda K, et al. Bacterial-Induced Maternal Interleukin-17A Pathway Promotes Autistic-Like Behaviors in Mouse Offspring. Exp Anim (2020) 69:250–60. doi: 10.1538/expanim.19-0156
18. Ribeiro M, Brigas HC, Temido-Ferreira M, Pousinha PA, Regen T, Santa C, et al. Meningeal γδ T Cell–Derived IL-17 Controls Synaptic Plasticity and Short-Term Memory. Sci Immunol (2019) 4(40):eaay5199. doi: 10.1126/sciimmunol.aay5199
19. Reed MD, Yim YS, Wimmer RD, Kim H, Ryu C, Welch GM, et al. IL-17a Promotes Sociability in Mouse Models of Neurodevelopmental Disorders. Nature (2020) 577:249–53. doi: 10.1038/s41586-019-1843-6
20. Zhang Y, Gao D, Kluetzman K, Mendoza A, Bolivar VJ, Reilly A, et al. The Maternal Autoimmune Environment Affects the Social Behavior of Offspring. J Neuroimmunol (2013) 258:51–60. doi: 10.1016/j.jneuroim.2013.02.019
21. Macleod MR, O’Collins T, Howells DW, Donnan GA. Pooling of Animal Experimental Data Reveals Influence of Study Design and Publication Bias. Stroke (2004) 35:1203–8. doi: 10.1161/01.STR.0000125719.25853.20
22. Ozaki K, Kato D, Ikegami A, Hashimoto A, Sugio S, Guo Z, et al. Maternal Immune Activation Induces Sustained Changes in Fetal Microglia Motility. Sci Rep (2020) 10:21378. doi: 10.1038/s41598-020-78294-2
23. Arrode-Brusés G, Brusés JL. Maternal Immune Activation by Poly(I:C) Induces Expression of Cytokines IL-1β and IL-13, Chemokine MCP-1 and Colony Stimulating Factor VEGF in Fetal Mouse Brain. J Neuroinflamm (2012) 9:83. doi: 10.1186/1742-2094-9-83
24. Schwartzer JJ, Careaga M, Onore CE, Rushakoff JA, Berman RF, Ashwood P. Maternal Immune Activation and Strain Specific Interactions in the Development of Autism-Like Behaviors in Mice. Transl Psychiatry (2013) 3:e240. doi: 10.1038/tp.2013.16
25. Pendyala G, Chou S, Jung Y, Coiro P, Spartz E, Padmashri R, et al. Maternal Immune Activation Causes Behavioral Impairments and Altered Cerebellar Cytokine and Synaptic Protein Expression. Neuropsychopharmacology (2017) 42:1435–46. doi: 10.1038/npp.2017.7
26. Xu X, Wu D, Hou S, Zhu J, Li J, Tang J. Prenatal Exposure to TAK242 Affects the Childhood Autism in Offspring in Animal Models of Autism Spectrum Disorder. Iran J Basic Med Sci (2017) 20:1016–20. doi: 10.22038/IJBMS.2017.9270
27. Ahmad SF, Ansari MA, Nadeem A, Bakheet SA, Alshammari MA, Attia SM. Protection by Tyrosine Kinase Inhibitor, Tyrphostin AG126, Through the Suppression of IL-17a, Rorγt, and T-Bet Signaling, in the BTBR Mouse Model of Autism. Brain Res Bull (2018) 142:328–37. doi: 10.1016/j.brainresbull.2018.08.020
28. Nadeem A, Ahmad SF, Al-Harbi NO, Attia SM, Bakheet SA, Ibrahim KE, et al. Nrf2 Activator, Sulforaphane Ameliorates Autism-Like Symptoms Through Suppression of Th17 Related Signaling and Rectification of Oxidant-Antioxidant Imbalance in Periphery and Brain of BTBR T+tf/J Mice. Behav Brain Res (2019) 364:213–24. doi: 10.1016/j.bbr.2019.02.031
29. Lammert CR, Frost EL, Bolte AC, Paysour MJ, Shaw ME, Bellinger CE, et al. Cutting Edge: Critical Roles for Microbiota-Mediated Regulation of the Immune System in a Prenatal Immune Activation Model of Autism. J Immunol (2018) 201:845–50. doi: 10.4049/jimmunol.1701755
30. Bakheet SA, Alzahrani MZ, Ansari MA, Nadeem A, Zoheir KMA, Attia SM, et al. Resveratrol Ameliorates Dysregulation of Th1, Th2, Th17, and T Regulatory Cell-Related Transcription Factor Signaling in a BTBR T + Tf/J Mouse Model of Autism. Mol Neurobiol (2017) 54:5201–12. doi: 10.1007/s12035-016-0066-1
31. Ansari MA, Nadeem A, Attia SM, Bakheet SA, Raish M, Ahmad SF. Adenosine A2A Receptor Modulates Neuroimmune Function Through Th17/retinoid-Related Orphan Receptor Gamma T (Rorγt) Signaling in a BTBR T Itpr3/J Mouse Model of Autism. Cell Signal (2017) 36:14–24. doi: 10.1016/j.cellsig.2017.04.014
32. Hsiao EY, McBride SW, Chow J, Mazmanian SK, Patterson PH. Modeling an Autism Risk Factor in Mice Leads to Permanent Immune Dysregulation. Proc Natl Acad Sci U S A (2012) 109:12776–81. doi: 10.1073/pnas.1202556109
33. Luan R, Cheng H, Li L, Zhao Q, Liu H, Wu Z, et al. Maternal Lipopolysaccharide Exposure Promotes Immunological Functional Changes in Adult Offspring CD4+ T Cells. Am J Reprod Immunol (2015) 73:522–35. doi: 10.1111/aji.12364
34. Chen Y, Liu S, Xu H, Zheng H, Bai C, Pan W, et al. Maternal Exposure to Low Dose BDE209 and Pb Mixture Induced Neurobehavioral Anomalies in C57BL/6 Male Offspring. Toxicology (2019) 418:70–80. doi: 10.1016/j.tox.2019.02.016
35. Gumusoglu SB, Hing BWQ, Chilukuri ASS, Dewitt JJ, Scroggins SM, Stevens HE. Chronic Maternal Interleukin-17 and Autism-Related Cortical Gene Expression, Neurobiology, and Behavior. Neuropsychopharmacology (2020) 45:1008–17. doi: 10.1038/s41386-020-0640-0
36. Afroz KF, Reyes N, Young K, Parikh K, Misra V, Alviña K. Altered Gut Microbiome and Autism Like Behavior are Associated With Parental High Salt Diet in Male Mice. Sci Rep (2021) 11(1):8364. doi: 10.1038/s41598-021-87678-x. Erratum in: Sci Rep (2022) 12(1):5686.
37. Alhosaini K, Ansari MA, Nadeem A, Bakheet SA, Attia SM, Alhazzani K, et al. 5-Aminoisoquinolinone, a PARP-1 Inhibitor, Ameliorates Immune Abnormalities Through Upregulation of Anti-Inflammatory and Downregulation of Inflammatory Parameters in T Cells of BTBR Mouse Model of Autism. Brain Sci (2021) 11:249. doi: 10.3390/brainsci11020249
38. Heo Y, Zhang Y, Gao D, Miller VM, Lawrence DA. Aberrant Immune Responses in a Mouse With Behavioral Disorders. PloS One (2011) 6:e20912. doi: 10.1371/journal.pone.0020912
39. Jaini R, Wolf MR, Yu Q, King AT, Frazier TW Jr, Eng C. Maternal Genetics Influences Fetal Neurodevelopment and Postnatal Autism Spectrum Disorder-Like Phenotype by Modulating in-Utero Immunosuppression. Transl Psychiatry (2021) 11:348. doi: 10.1038/s41398-021-01472-x
40. Kalish BT, Kim E, Finander B, Duffy EE, Kim H, Gilman CK, Yim YS, et al. Maternal Immune Activation in Mice Disrupts Proteostasis in the Fetal Brain. Nat Neurosci (2021) 24(2):204–13. doi: 10.1038/s41593-020-00762-9
41. Schwartzer JJ, Careaga M, Coburn MA, Rose DR, Hughes HK, Ashwood P. Behavioral Impact of Maternal Allergic-Asthma in Two Genetically Distinct Mouse Strains. Brain Behav Immun (2017) 63:99–107. doi: 10.1016/j.bbi.2016.09.007
42. Senkal E, Bagcioglu E, Eryigit U, Erbas O, Solmaz V. Exposure to Hypertonic Solutions During Pregnancy Induces Autism-Like Behaviors via the NFAT-5 Pathway in Offspring in a Rat Model. Physiol Behav (2021) 240:113545. doi: 10.1016/j.physbeh.2021.113545
43. Shin K-O, Crumrine DA, Kim S, Lee Y, Kim B, Abuabara K, et al. Phenotypic Overlap Between Atopic Dermatitis and Autism. BMC Neurosci (2021) 22:43. doi: 10.1186/s12868-021-00645-0
44. Shimizu Y, Tsukada T, Sakata-Haga H, Sakai D, Shoji H, Saikawa Y, et al. Exposure to Maternal Immune Activation Causes Congenital Unfolded Protein Response Defects and Increases the Susceptibility to Postnatal Inflammatory Stimulation in Offspring. J Inflamm Res (2021) 14:355–65. doi: 10.2147/JIR.S294238
45. Kim E, Paik D, Ramirez RN, Biggs DG, Park Y, Kwon H-K, et al. Maternal Gut Bacteria Drive Intestinal Inflammation in Offspring With Neurodevelopmental Disorders by Altering the Chromatin Landscape of CD4 T Cells. Immunity (2022) 55:145–158.e7. doi: 10.1016/j.immuni.2021.11.005
46. Hooijmans CR, Rovers MM, de Vries RBM, Leenaars M, Ritskes-Hoitinga M, Langendam MW. Syrcle’s Risk of Bias Tool for Animal Studies. BMC Med Res Method (2014) 14:43. doi: 10.1186/1471-2288-14-43
47. Abdallah MW, Larsen N, Grove J, Nørgaard-Pedersen B, Thorsen P, Mortensen EL, et al. Amniotic Fluid Inflammatory Cytokines: Potential Markers of Immunologic Dysfunction in Autism Spectrum Disorders. World J Biol Psychiatry (2013) 14:528–38. doi: 10.3109/15622975.2011.639803
48. Alves de Lima K, Rustenhoven J, Da Mesquita S, Wall M, Salvador AF, Smirnov I, et al. Meningeal γδ T Cells Regulate Anxiety-Like Behavior via IL-17a Signaling in Neurons. Nat Immunol (2020) 21:1421–9. doi: 10.1038/s41590-020-0776-4
49. Kim S, Kim H, Yim YS, Ha S, Atarashi K, Tan TG, et al. Maternal Gut Bacteria Promote Neurodevelopmental Abnormalities in Mouse Offspring. Nature (2017) 549:528–32. doi: 10.1038/nature23910
50. De Angelis M, Piccolo M, Vannini L, Siragusa S, De Giacomo A, Serrazzanetti DI, et al. Fecal Microbiota and Metabolome of Children With Autism and Pervasive Developmental Disorder Not Otherwise Specified. PloS One (2013) 8:e76993. doi: 10.1371/journal.pone.0076993
51. Hsiao EY, McBride SW, Hsien S, Sharon G, Hyde ER, McCue T, et al. Microbiota Modulate Behavioral and Physiological Abnormalities Associated With Neurodevelopmental Disorders. Cell (2013) 155:1451–63. doi: 10.1016/j.cell.2013.11.024
52. Faraco G, Brea D, Garcia-Bonilla L, Wang G, Racchumi G, Chang H, et al. Dietary Salt Promotes Neurovascular and Cognitive Dysfunction Through a Gut-Initiated TH17 Response. Nat Neurosci (2018) 21:240–9. doi: 10.1038/s41593-017-0059-z
53. Thomas MSC, Davis R, Karmiloff-Smith A, Knowland VCP, Charman T. The Over-Pruning Hypothesis of Autism. Dev Sci (2016) 19:284–305. doi: 10.1111/desc.12303
54. Sacai H, Sakoori K, Konno K, Nagahama K, Suzuki H, Watanabe T, et al. Autism Spectrum Disorder-Like Behavior Caused by Reduced Excitatory Synaptic Transmission in Pyramidal Neurons of Mouse Prefrontal Cortex. Nat Commun (2020) 11:5140. doi: 10.1038/s41467-020-18861-3
55. Gaugler T, Klei L, Sanders SJ, Bodea CA, Goldberg AP, Lee AB, et al. Most Genetic Risk for Autism Resides With Common Variation. Nat Genet (2014) 46:881–5. doi: 10.1038/ng.3039
56. Chen JA, Peñagarikano O, Belgard TG, Swarup V, Geschwind DH. The Emerging Picture of Autism Spectrum Disorder: Genetics and Pathology. Annu Rev Pathol (2015) 10:111–44. doi: 10.1146/annurev-pathol-012414-040405
57. Werling DM, Geschwind DH. Sex Differences in Autism Spectrum Disorders. Curr Opin Neurol (2013) 26:146–53. doi: 10.1097/WCO.0b013e32835ee548
58. Karp NA, Reavey N. Sex Bias in Preclinical Research and an Exploration of How to Change the Status Quo. Br J Pharmacol (2019) 176:4107–18. doi: 10.1111/bph.14539
59. Shi P, Zhu S, Lin Y, Liu Y, Liu Y, Chen Z, et al. Persistent Stimulation With Interleukin-17 Desensitises Cells Through Scfβ-TrCP-Mediated Degradation of Act1. Sci Signal (2011) 4:ra73. doi: 10.1126/scisignal.2001653
Keywords: IL-17, autism spectrum disorder, animal model, systematic review, inflammation
Citation: Thawley AJ, Veneziani LP, Rabelo-da-Ponte FD, Riederer I, Mendes-da-Cruz DA and Bambini-Junior V (2022) Aberrant IL-17 Levels in Rodent Models of Autism Spectrum Disorder: A Systematic Review. Front. Immunol. 13:874064. doi: 10.3389/fimmu.2022.874064
Received: 11 February 2022; Accepted: 04 May 2022;
Published: 10 June 2022.
Edited by:
Juehua Yu, The First Affiliated Hospital of Kunming Medical University, ChinaReviewed by:
Hongjin Wu, Harbin Institute of Technology, ChinaMarcela Davoli-Ferreira, University of Calgary, Canada
Copyright © 2022 Thawley, Veneziani, Rabelo-da-Ponte, Riederer, Mendes-da-Cruz and Bambini-Junior. This is an open-access article distributed under the terms of the Creative Commons Attribution License (CC BY). The use, distribution or reproduction in other forums is permitted, provided the original author(s) and the copyright owner(s) are credited and that the original publication in this journal is cited, in accordance with accepted academic practice. No use, distribution or reproduction is permitted which does not comply with these terms.
*Correspondence: Daniella Areas Mendes-da-Cruz, ZGFuaWVsbGFAaW9jLmZpb2NydXouYnI=; Victorio Bambini-Junior, di5iYW1iaW5pQGxhbmNhc3Rlci5hYy51aw==