- Infection and Innate Immunity Laboratory, Department of Biological Sciences, The George Washington University, Washington, DC, United States
Zika is a member of the Flaviviridae virus family that poses some of the most significant global health risks, causing neurologic complications that range from sensory neuropathy and seizures to congenital Zika syndrome (microcephaly) in infants born to mothers infected during pregnancy. The recent outbreak of Zika virus (ZIKV) and its serious health threats calls for the characterization and understanding of Zika pathogenesis, as well as host antiviral immune functions. Although ZIKV has been associated with activating the RNA interference (RNAi) immune pathway and altering host metabolism, in-depth studies are still required to uncover the specifics of the complex host-virus interactions and provide additional insights into the molecular components that determine the outcome of this disease. Previous research establishes the fruit fly Drosophila melanogaster as a reliable model for studying viral pathogens, as it shares significant similarities with that of vertebrate animal systems. Here, we have developed an in vivo Drosophila model to investigate ZIKV-mediated perturbed metabolism in correlation to the RNAi central mediator Dicer-2. We report that ZIKV infection reprograms glucose and glycogen metabolism in Dicer-2 mutants to maintain efficient replication and successful propagation. Flies that exhibit these metabolic effects also show reduced food intake, which highlights the complicated neurological defects associated with ZIKV. We show that ZIKV infection significantly reduces insulin gene expression in Dicer-2 mutants, suggesting an insulin antiviral role against ZIKV and a direct connection to RNAi immunity. Moreover, we find that the insulin receptor substrate chico is crucial to the survival of ZIKV-infected flies. These observations are remarkably more severe in adult female flies compared to males, indicating possible sex differences in the rates of infection and susceptibility to the development of disease. Such findings not only demonstrate that metabolic alterations can be potentially exploited for developing immune therapeutic strategies but also that preventive measures for disease development may require sex-specific approaches. Therefore, further studies are urgently needed to explore the molecular factors that could be considered as targets to inhibit ZIKV manipulation of host cell metabolism in females and males.
Introduction
With World Health Organization (WHO) scientists confirming recent outbreaks of Zika virus (ZIKV) in India, investigating and understanding the molecular mechanisms underlying immune responses against ZIKV have become ever more urgent (1, 2). Zika, a single-stranded positive-sense RNA virus and a member of the Flaviviridae family, is transmitted to humans primarily by the infected Aedes mosquito species (Ae. aegypti and Ae. albopictus) and associated with abnormal functions of neuronal cells leading to neurological disorders such as microcephaly in newborns and Guillain-Barreí syndrome in adults (3, 4). Since 2015, ZIKV spread rapidly and created an enormous global alarm, with more than 1,000,000 estimated cases occurring in the Americas (5). There are currently no licensed treatments or vaccines effective against ZIKV infection. Hence, the development of an in vivo model to uncover the complex host-ZIKV interactions and identify the molecular components involved in immune signaling are crucial to the advancement of innovative concepts and means for the efficient control of ZIKV disease and clarifying anti-ZIKV immune mechanisms in humans.
The fruit fly Drosophila melanogaster has proven to be a significant model organism for researching cellular interactions of various human pathogens including arboviruses (arthropod-borne viruses) such as ZIKV and investigating the genetic basis of antiviral resistance (6–10). Particularly, the large conservation between Drosophila and mosquitoes has already paved the way for the recent, novel insights into Zika pathogenesis and host immune function using Drosophila models (11–14). With the availability of well-established techniques for developmental and genetic manipulations, Drosophila is considered a potent model for studying host-pathogen interactions and innate immunity, which shares significant similarities with that of vertebrate animal systems (15, 16). As in vertebrates, numerous Drosophila immune responses are triggered by diverse pathogen infections, leading to the transcriptional induction of downstream NF-κB-dependent effectors including antimicrobial peptides (AMPs) that can attenuate or clear infection (17, 18). These antibacterial and antifungal signaling mechanisms, regulated by the inflammatory NF-κB pathways Toll and immune deficiency (IMD), are well-characterized and documented (19, 20). However, their roles in antiviral defense remain poorly understood. Little is also known about the roles of other major humoral and cellular immune pathways such as JAK/STAT, autophagy, and melanization (21, 22).
The central antiviral immune response in the fly involves the canonical RNA interference (RNAi) pathway, a highly conserved nucleic acid–based immune defense that is induced by double stranded RNA (dsRNA) to mediate sequence-specific gene silencing (23–25). In Drosophila, RNAi involves the RNase Dicer-2 that recognizes and cleaves dsRNA to generate viral small interfering RNAs (siRNAs). These siRNAs are loaded onto Argonaute-2 (Ago-2) protein to guide the RNA-induced silencing complex (RISC) to complementary RNAs in the cell, leading to their sequence-specific degradation. Recent findings show that upon infection with ZIKV, flies display a substantial increase in viral replication that does not compromise their survival (11, 26). Most importantly, Dicer-2 mutants display enhanced ZIKV load and increased susceptibility to ZIKV infection, suggesting that RNAi confers resistance to ZIKV infection in Drosophila (11). ZIKV is also confirmed to exhibit tissue tropism by infecting the fat body, crop, and gut of the adult fly, which results in local pathologies marked by changes in the host homeostasis and metabolism (11). These alterations indicate a significant role of metabolism in the defense against ZIKV, thereby stressing the need for a more comprehensive look into the intricacies of host–ZIKV dynamics to understand the molecular and physiological processes that determine the outcome of this disease.
In this study, we used a Drosophila model system to study the interplay between host immunometabolism and ZIKV infection. We show that not only does ZIKV reduce feeding in infected flies but also trigger internal changes such as carbohydrate metabolism in Dicer-2 mutant flies. These fluctuations during infection confirm perturbed host metabolism and homeostasis, which pathogens manipulate to acquire their specific metabolic needs (27). We also find that insulin signaling, one of the main metabolic pathways involved in host-pathogen interactions, is targeted by ZIKV in Dicer-2 mutants, therefore reporting an antiviral role for insulin during ZIKV infection and a direct correlation to the RNAi pathway. In addition, we show for the first time that the insulin receptor substrate chico is required for the survival of ZIKV-infected flies. Interestingly, these observations were sex-dependent, which highlights the importance of using both male and female animals for in vivo efficacy studies. Overall, this work identifies host carbohydrate metabolism and insulin signaling as components of Drosophila immunity against ZIKV infection and demonstrates that insulin works cooperatively with the RNAi antiviral immune pathway for host protection. These are important findings that give more insight into the arms race between hosts and pathogens in general and more specifically the mechanisms Drosophila and ZIKV use to regulate metabolic pathways to their own advantage.
Materials and Methods
Fly and Nematode Stocks
Dicer-2L811fsX and chico14337 were compared to a YW background control. dFOXOC01841 was also used in comparison to w1118 background control. All flies were reared on Bloomington Drosophila Stock Center cornmeal food (LabExpress) supplemented with yeast (Carolina Biological Supply), and maintained at 25°C with a 12:12-h light:dark photoperiodic cycle.
Fly Infection
ZIKV strain MR766 was prepared as previously described (11). Injections were performed by anesthetizing flies with carbon dioxide on a gas pad. For each experiment, 2–5-day-old adult males and female flies were sorted into separate vials and injected with ZIKV suspensions in PBS (pH 7.5) using a nanoinjector (Nanoject III; Drummond Scientific). Heat-inactivated or live ZIKV solution (11,000 PFU/fly) (100 nl) was injected into the thorax of flies, and an injection of the same volume of PBS acted as negative control. Injected flies were then maintained at 25°C and transferred to fresh vials every third day throughout the experiment. They were collected at 4 days post injection and directly used for other experiments in this study. Statistical analyses of the differences in gene expression between fly strains and experimental conditions were conducted with duplicate replicates from three independent experiments.
Fly Survival
For each fly strain, three groups of 20 male and female flies were injected with ZIKV and control groups were injected with PBS. Following injection, flies were maintained at a constant temperature of 25°C with a 12-hour light/dark cycle, and mortality was recorded daily. Fly deaths occurring within 1 day of injection were attributed to injury and were not included in the results.
Gene Expression Analysis
Total RNA was extracted from 10 male or female flies, using TRIzol according to manufacturer’s protocol. Total RNA (500 ng–1 mg) was used to synthesize cDNA using the High-Capacity cDNA Reverse Transcription Kit (Applied Biosystems). Quantitative RT-PCR (qRT-PCR) experiments were performed with two technical replicates and gene-specific primers (Table 1) using a CFX96 Real-Time PCR detection system (Bio-Rad Laboratories). Cycle conditions were as follows: 95°C for 2 min, 40 repetitions of 95°C for 15 s followed by 61°C for 30 s, and then one round of 95°C for 15 s, 65°C for 5 s, and finally 95°C for 5 s. Quantification was performed from three biological replicates for both test and control treatments. Fold changes were calculated with the
method using Ribosomal protein L32 (RpL32) as a housekeeping gene (28, 29).
Feeding Assays
Food intake measurements were performed as described in prevalent feeding protocols using dye-labeled food (30). For each experimental condition, 20 male and female flies were maintained separately on the previously mentioned food for 4 days post infection. Flies were then transferred to vials with identical media containing 1% (w/v) FD&C Blue #1 (Spectrum Chemical). After 1 hour, feeding was interrupted by freezing the vials at −80°C. Frozen flies were transferred to 1.7 mL Eppendorf tubes and homogenized with a pestle on ice in 50 μl of 1x PBS + 1% Triton X-100. After centrifugation for 15 min at 9000 × g and 4°C to clear the debris, the absorbance of the supernatant was measured at 630 nm (A630) on a NanoDrop 2000 Spectrophotometer (Thermo Fisher Scientific). Flies fed non-labeled food were used as controls and their A630 values were subtracted from experimental readings. Serial dilutions of an initial 10 μl aliquot of the non-solidified dye-labeled food added to 0.99 ml of 1x PBS + 1% Triton X-100 were used to generate a standard curve. After determining the equivalent dye concentration of each homogenate using the linear fit of the standard curve, consumption was calculated by multiplying with the homogenate volume (50 μl) and dividing by the number of flies per sample.
Glucose, Glycogen, and Trehalose Levels
Previously established enzymatic-based methods were used to quantify basic metabolites in ZIKV-infected flies (31). At 4 days post infection, 5 flies were collected for each experimental condition and rinsed several times with 1 ml of 1x PBS. Flies were then homogenized with a pellet pestle on ice in 100 μl of 1x PBS to determine glucose and glycogen levels, or in 100 μl of Trehalase buffer (TB; 5 mM Tris pH 6.6, 137 mM NaCl, 2.7 mM KCl) to measure trehalose levels.
Glucose and glycogen levels were measured by initially diluting the samples 1:3 in PBS and then further diluting them 1:1 in either amyloglucosidase stock solution (1.5 ml of amyloglucosidase in 1 ml of PBS, Sigma-Aldrich) or PBS. Diluted samples (30 μl) were loaded in duplicate rows onto a clear 96-well plate and incubated at 37°C for 60 min. Hexokinase reagent (100 μl; Glucose Assay Reagent, Sigma-Aldrich; G3293, St. Louis, MO, USA) was added to each well and samples were incubated at room temperature for an additional 15 min. Absorbance was measured at 340 nm. Glucose standard curve was used to determine glucose levels, whereas glycogen levels were calculated by subtracting the absorbance of glucose from the absorbance of samples diluted with amyloglucosidase stock.
To determine trehalose content, samples were diluted 1:3 in TB, followed by 1:1 dilution in either TB or Trehalase Stock (TS; 3 μl of porcine trehalase in 1 ml of TB). Samples (30 μl) were incubated in duplicate rows in a clear 96-well plate at 37°C for 24 hours, after which hexokinase (100 μl) was added to each well and the absorbance was measured at 340 nm. The measured absorbance for free glucose in the untreated samples (containing TB) was first subtracted from the absorbance of the samples that were digested with trehalase (TS). Trehalose levels were then calculated based on a trehalose standard curve.
Glucose, glycogen, and trehalose measurements were normalized to the amount of proteins in each sample. Protein quantification was determined by Pierce™ BCA Protein Assay Kit (Thermo Fisher Scientific; 23227, Waltham, MA, USA). Each experiment was run in technical duplicates and repeated three times.
Cholesterol Quantification
Cholesterol levels were determined using Amplex Red Cholesterol Assay Kit (Invitrogen; A12216, Carlsbad, CA, USA). At 4 days post infection, 10 adult male and female flies were collected separately and homogenized with a pestle on ice in 100 μl of 1x reaction buffer provided by assay kit. In a black 96-well plate (Fisher Scientific; 509051574), 50 μl of each sample were mixed with 90 μl of the reaction mix provided by the kit and incubated for 30 min at 37°C. Fluorescence was measured with excitation at 530 nm and emission at 590 nm. A standard curve was used to determine cholesterol levels, and the assay was repeated three times. All data were calculated relative to the proteins in each sample. Protein quantification was determined by PierceTM BCA Protein Assay Kit (Thermo Fisher Scientific; 23227, Waltham, MA, USA).
Statistical Analysis
All statistical analyses were performed using GraphPad Prism 9 software. Gene expression and metabolic analyses were compared using a one-way ANOVA and Bonferroni multiple comparisons test to determine differences between specific treatments. Survival curves were assessed using a Log-Rank (Mantel-Cox) test within the GraphPad Prism program. All analyses were performed on data accumulated through three independent experiments.
Results
ZIKV Infection Reduces Feeding in Drosophila Hosts
Behavioral plasticity during infections has been closely linked to host fitness and pathogen spread, thus making the integration of behavioral variation into our understanding of pathogenic infections imperative (32–34). To investigate the interplay between ZIKV infection and host behavior, we injected 11,000 PFU/fly of the strain MR766 into the thorax of Dicer-2 mutants and their YW controls. We estimated food consumption, a fundamental behavioral parameter, by performing a quantitative assay that involves labeling food with FD&C Blue, a soluble, non-absorbable dye (30). Food intake measurements were taken at 4 days post injection for infected female and male adult flies. We found significantly lower consumption rates in ZIKV-infected, Dicer-2 female mutants compared to PBS control–treated flies whereas males showed no differences in feeding trends (Figures 1A, B). To exclude possible effects due to PBS injections, we confirmed similar feedings rates in untreated Dicer-2 and YW flies (Figures S1A, B). We also measured feeding rates in Dicer-2 and YW following 1 hour starvation to reduce possible irregular feeding patterns, which showed similar results to non-starved flies (Figures S2A, B). Collectively, these results show that ZIKV infection alters Drosophila feeding rates in a sex-dependent manner, suggesting severe motor and metabolic dysfunction as disease outcomes.
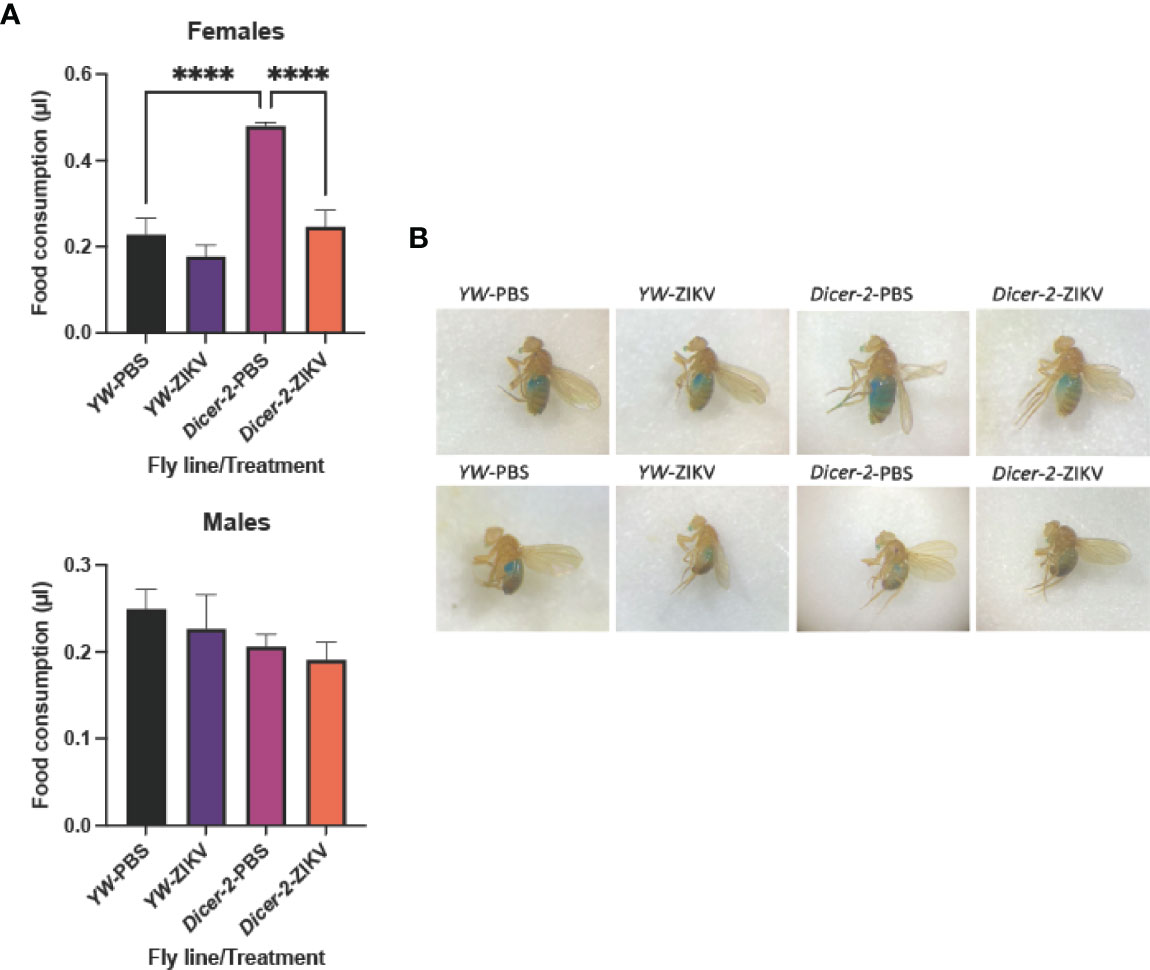
Figure 1 Feeding rate of Drosophila melanogaster upon infection with Zika virus. (A) Drosophila female and male adult Dicer-2 mutants and their background controls (YW) were injected with ZIKV (African strain MR766; 110 million PFUs/ml), and feeding was estimated at 4 days post infection. Injections with PBS served as negative controls. (B) Dye-stained food in the abdomen of ZIKV-injected or PBS-injected Dicer-2 mutants and YW individuals. Data represent three biological replicates of at least 20 flies for each experimental condition. (One-way ANOVA, ****p < 0.0001).
ZIKV-Infected Flies Show Perturbed Carbohydrate Metabolism
To further characterize the ZIKV-induced pathology, we next examined the effect of ZIKV infection on host metabolism, which is essential for cellular energy maintenance and survival during infection (27). Drosophila regulates glucose and trehalose (a disaccharide of glucose) as circulating carbohydrates, and stores excesses in the form of glycogen and lipids in the fat body (35). We first assessed all three types of Drosophila carbohydrates in Dicer-2 mutants and YW adult flies at 4 days following injection with the MR766 strain. Both glucose and trehalose levels were strongly reduced in ZIKV-infected females compared to their respective PBS-treated controls (Figures 2A, C). More specifically, ZIKV-infected, Dicer-2 females had lower levels of glucose in comparison to ZIKV-infected YW flies, indicating a positive correlation between the RNAi pathway and metabolism (Figure 2A). We found no significant differences in glucose levels in male flies, regardless of their experimental conditions (Figure 2B). However, trehalose levels in infected Dicer-2 and YW flies were lower in comparison to their PBS-treated controls (Figure 2D).
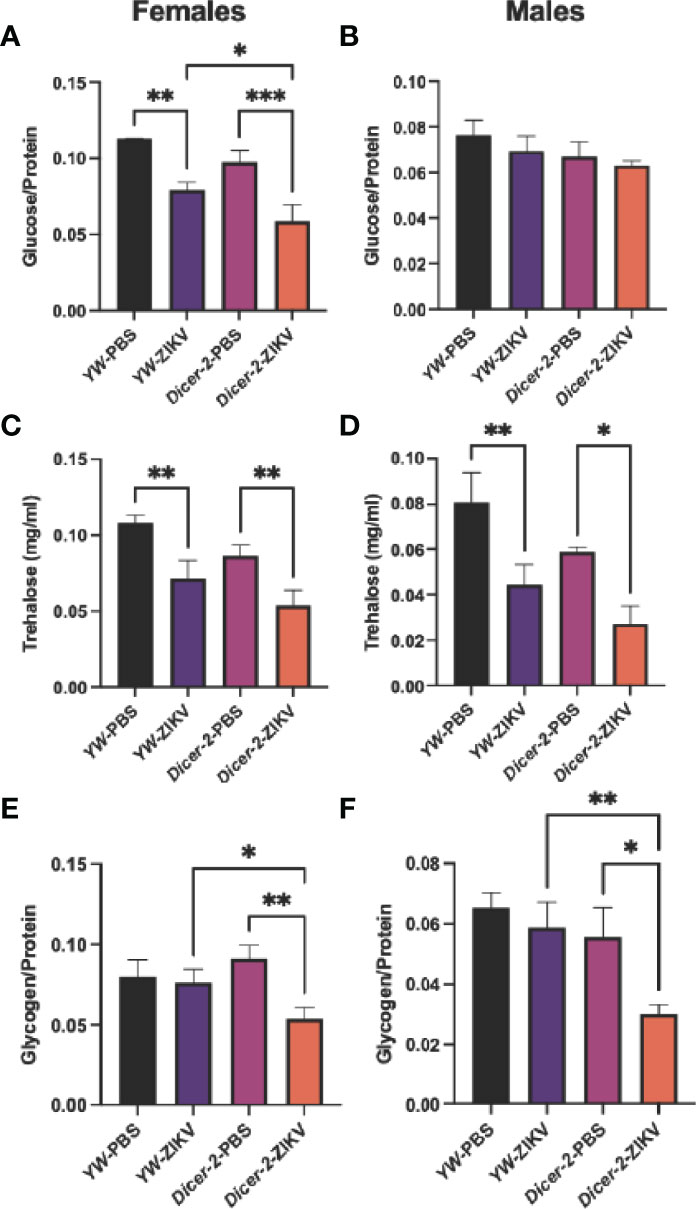
Figure 2 Carbohydrate metabolism in Drosophila melanogaster 4 days after infection with Zika virus (ZIKV). (A, B) Glucose levels in infected female and male Dicer-2 mutant adults compared to YW (background line) and PBS (no infection treatment) controls. (C, D) Trehalose levels in ZIKV-infected and PBS-injected Dicer-2 mutants and YW flies. (E, F) Glycogen levels in ZIKV-infected and PBS-injected Dicer-2 mutants and their YW background controls. All experiments were performed in duplicates and repeated three times. (One-way ANOVA, *p < 0.01, **p < 0.001, ***p < 0.0001).
Glycogen levels in Dicer-2 female mutants infected with ZIKV also decreased compared to their infected YW backgrounds and PBS treatment controls (Figure 2E). Interestingly, despite the lack of change in glucose levels in Dicer-2 male mutants and their controls, glycogen from the same treatment groups showed similar results to female Dicer-2 mutants, in which glycogen levels in infected Dicer-2 mutants were lower compared to their infected YW backgrounds and PBS treatment controls (Figure 2F). Together, these results assert the sex-dependent ZIKV pathology while suggesting a key role for carbohydrate metabolism during ZIKV infection.
Because lipids form the primary source of energy reserve, we also explored whether ZIKV infection triggers cholesterol changes in Drosophila hosts. Unlike the observed changes in carbohydrate metabolism, we found no differences among female and male groups (Figures 3A, B).
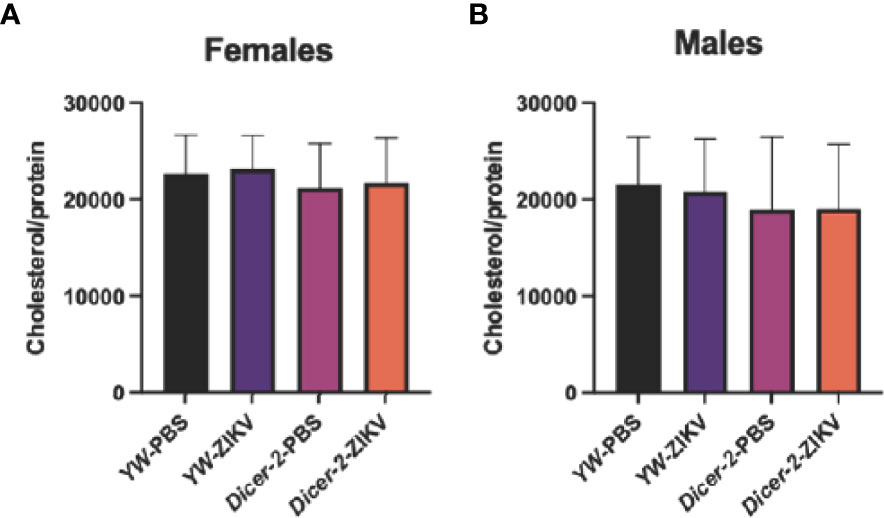
Figure 3 Cholesterol levels in Drosophila melanogaster Dicer-2 mutant adult flies and their YW background controls infected with Zika virus (ZIKV) or injected with PBS (uninfected controls). Quantification experiments were performed in biological duplicates and repeated three times using female (A) and male (B) individuals. (One-way ANOVA).
ZIKV Infection Suppresses the Insulin Pathway in Dicer-2 Drosophila Mutants
The canonical RNAi pathway is closely associated with the insulin signaling pathway, as both have been established as key determinants in vector competence and disease outcome (7, 36). Studies using Drosophila demonstrate that insulin signaling presumes an antiviral role by promoting the expression of the RNAi components Dicer-2 and Ago-2 (36, 37). Thus, we asked whether ZIKV infection affects insulin gene expression in Dicer-2 mutants and YW adult flies. We found a strong downregulation of insulin-regulated genes foxo, chico, dilp2, InR, spargel, and Thor in infected Dicer-2 female mutants compared to their infected YW background controls (Figure 4A). Similar to these findings, Dicer-2 male mutants showed low expression levels in all insulin genes except for chico (Figure 4B). Collectively, this indicates a significant correlation between the RNAi and insulin pathways in Drosophila in the context of ZIKV infection.
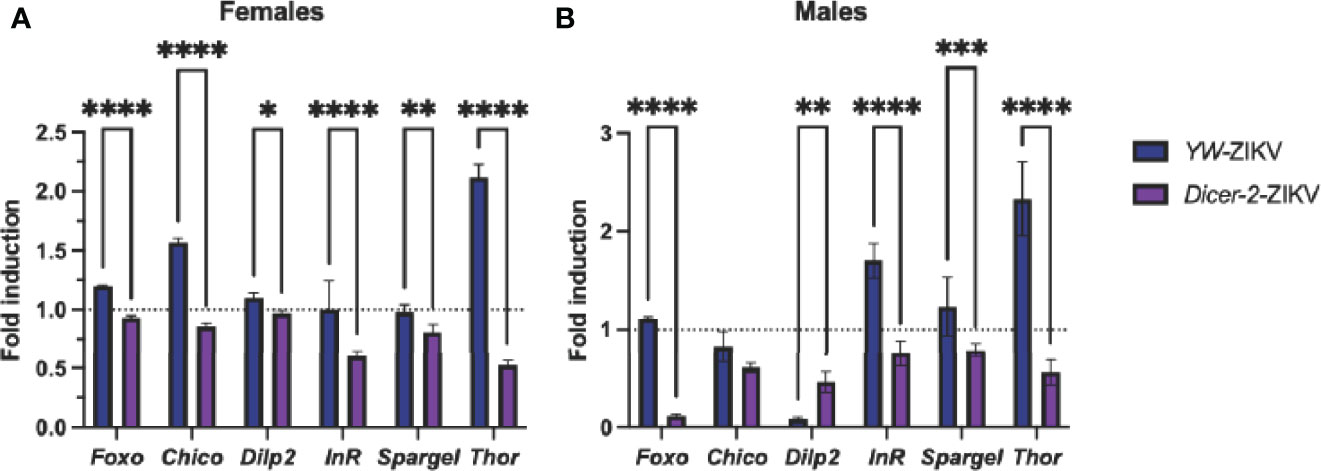
Figure 4 Analysis of insulin-regulated gene expression through quantitative RT-PCR in Zika virus (ZIKV)-infected Drosophila melanogaster Dicer-2 mutants and YW background control flies compared to uninfected (PBS-injected) background controls. Levels of mRNA were normalized against RpL32 (housekeeping gene), and three independent experiments were performed using adult females (A) and males (B). (Two-way ANOVA, *p = 0.0252, **p = 0.0018, ***p = 0.0009, ****p < 0.0001).
Insulin Signaling Promotes Drosophila Resistance Against ZIKV Infection
To understand whether insulin signaling modulates survival to ZIKV infection in Drosophila, we injected flies carrying loss-of-function mutations in foxo and chico with MR766 and estimated survival rates over time. We found that infection with this ZIKV strain failed to reduce fly survival in both female and male foxo mutants, which were similar to the survival of PBS-injected controls and background flies (Figures 5A, B). In contrast, female and male chico mutants succumbed to ZIKV infection at a much faster rate compared to their background controls, indicating an essential role for chico in host immunity against ZIKV (Figures 5C, D). We then estimated ZIKV copy numbers in the infected flies compared to PBS control-treated flies at 4 days post injection using primer sequences against NS5, the largest and most critical nonstructural protein in the ZIKV replication complex (38–40). In corroboration with the previous findings, we found strongly elevated levels of ZIKV copies in both female and male chico mutants compared to their respective controls (Figures 6B, D). foxo mutants on the other hand showed no difference in fold induction compared to their controls (Figures 6A, C), which further emphasizes that chico plays an antiviral role in Drosophila during ZIKV infection.
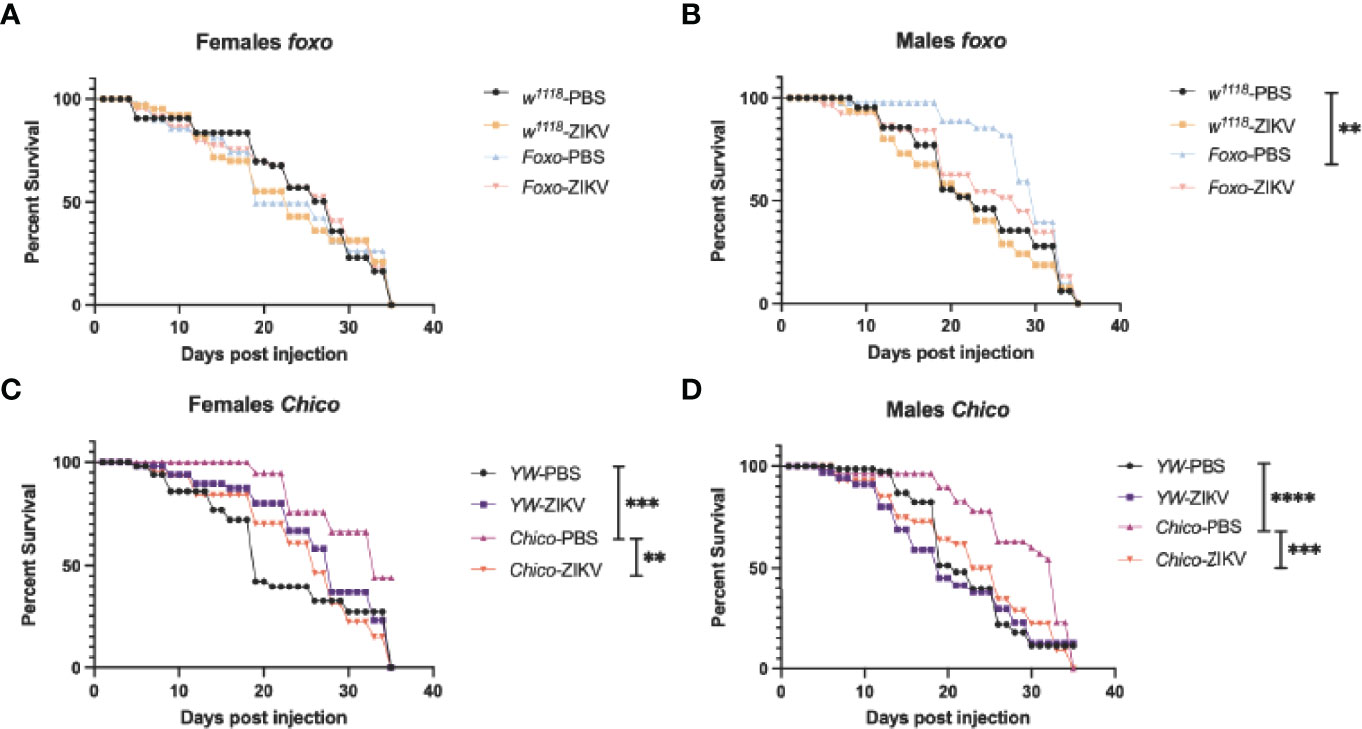
Figure 5 Survival of Drosophila melanogaster adult flies after intrathoracic injection with Zika virus (ZIKV) was monitored at 24-h intervals for 35 days. Injections with PBS served as negative controls. (A, B) Survival of FOXO female and male mutants compared to their background (w1118) controls. (C, D) Survival of Chico female and male mutants compared to YW background controls. Data represent three biological replicates of at least 20 adult flies. Log-rank (Mantel–Cox) was used for statistical analysis, **p = 0.0033, ***p = 0.0001, ****p < 0.0001.
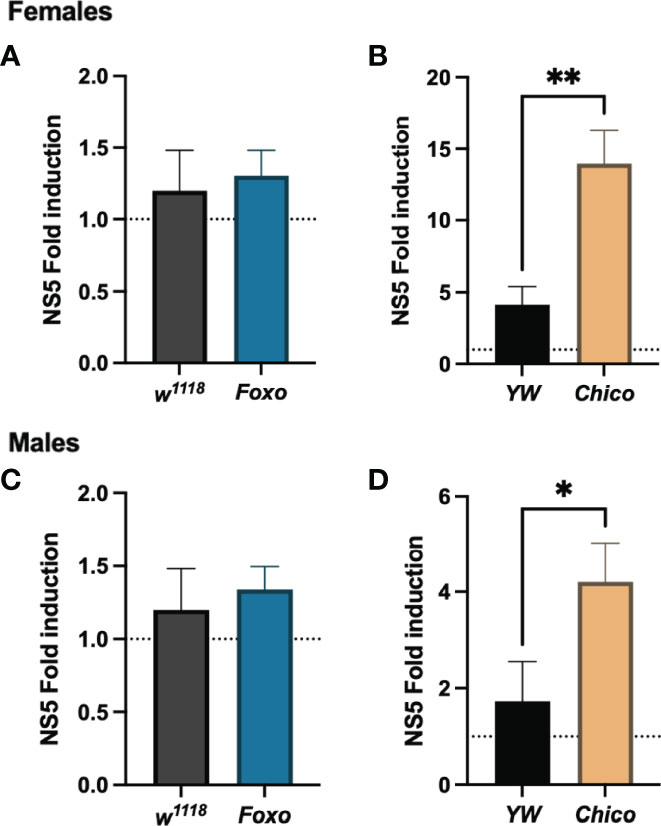
Figure 6 Zika virus (ZIKV) load using qRT-PCR analysis and NS5 gene-specific primers in Drosophila melanogaster Foxo and Chico mutants compared to their background controls YW and w1118, respectively. Data, collected from female (A, B) and male (C, D) adult flies, were normalized to the housekeeping gene RpL32 shown relative to control flies injected with PBS (injury control). Three independent experiments were carried out with 10 flies per sample in technical duplicates (One-way ANOVA, *p < 0.01, **p < 0.001).
Insulin Promotes RNAi Signaling to Fight ZIKV Infection in Drosophila
To test whether insulin signaling confers RNAi resistance to ZIKV infection in Drosophila hosts, we estimated the transcript levels of Dicer-2 and Ago-2 in foxo and chico null mutants. Dicer-2 was significantly reduced in infected foxo and chico female mutants (Figures 7A, B), whereas Ago-2 decreased only in infected chico female mutants (Figure 7A). On the other hand, Dicer-2 expression levels were strongly reduced in chico male mutants only, while Ago-2 levels were lower in both in foxo and chico males (Figures 7C, D). This indicates that insulin potentiates RNAi signaling in Drosophila and renders resistance to ZIKV infection differently in both sexes.
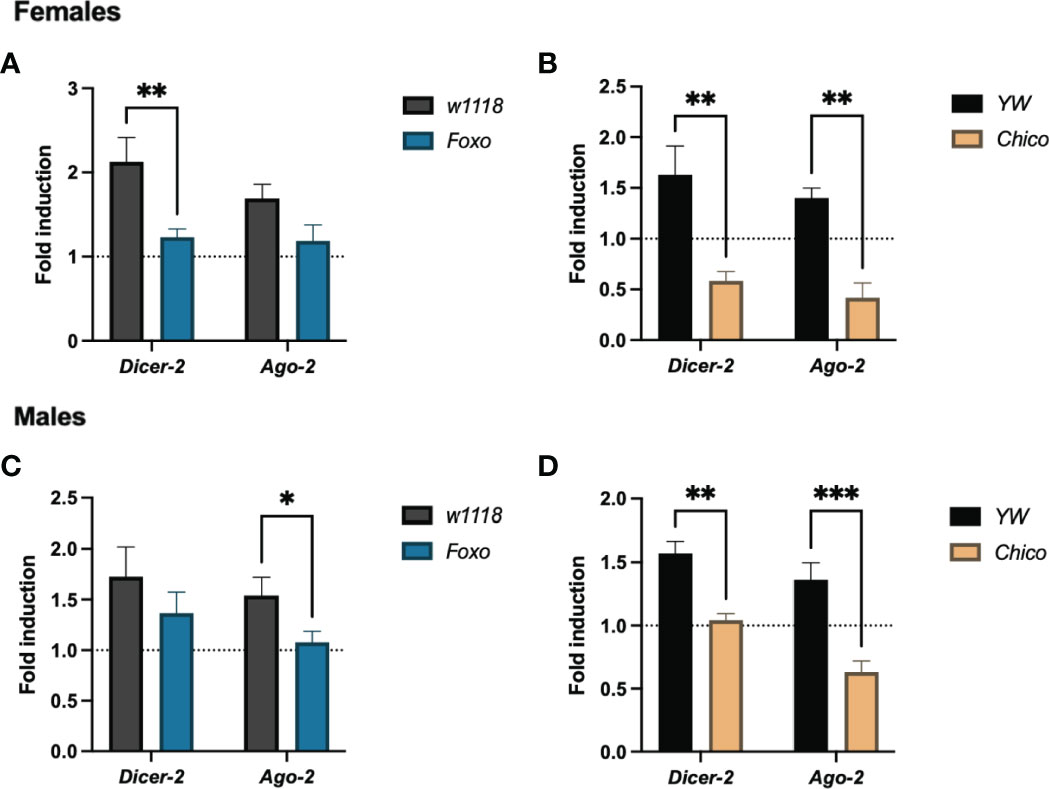
Figure 7 RNAi signaling activity in Zika virus (ZIKV)-infected Drosophila melanogaster insulin mutants. Mutant adult flies for Foxo and Chico were processed for RNA analysis and gene expression levels were determined by qRT-PCR at 4 days post infection. Transcript levels of the RNAi genes Dicer-2 and Ago-2 in females (A, B) and males (C, D) are shown (Two-way ANOVA, *p = 0.0272, **p < 0.001, ***p = 0.0005).
Discussion
Drosophila possesses distinct antiviral immune mechanisms that are found in both mosquitos and vertebrate animals, thus providing a suitable model for studying host-arbovirus interactions (6–10, 41). Our previous work showed that Drosophila flies can sustain the replication of the ZIKV African strain MR766 following intrathoracic injection of the virus (11). This work also highlighted the RNAi component Dicer-2 as a key regulator of fly immunity against ZIKV, which is closely associated with host homeostasis and metabolic processes. Using Dicer-2 mutant flies, here we show that ZIKV infection results in behavioral and metabolic shifts that promote a successful replication of the virus and progression of the disease, therefore uncovering viral mechanisms aimed to manipulate host immune and metabolic systems. Our findings show that ZIKV infected Dicer-2 female mutants display strikingly reduced food consumption rates compared to YW background and uninfected controls, which signifies neurobehavioral modifications triggered by the virus. Interestingly, infected Dicer-2 male mutants do not exhibit the same behavior, indicating sex-dependent deficits caused by ZIKV infection. Similar differences have been reported in mouse models, in which sex-dependent, long-term behavioral abnormalities after ZIKV exposure were observed (42). These findings establish a foundation for identifying susceptibility factors that lead to the development of ZIKV pathologies and secondary behavioral changes in infected individuals.
Although many viruses enhance their replication by reprogramming host metabolism, whether ZIKV drives similar modifications, and the functional consequences of Zika-induced metabolic changes, remain largely unclear. Our findings show that ZIKV infection alters carbohydrate metabolism in Dicer-2 female mutants by targeting glucose, trehalose, and glycogen levels, which are critical regulators of the glycolysis pathway in Drosophila (43, 44). Infected Dicer-2 male flies also show a significant decrease in glycogen levels despite the lack of change in the other carbohydrates. Noticeably, Dengue virus (DENV), which is closely related to ZIKV, depends on the glycolysis pathway for its replication (45), and high glucose levels have been shown to restrict ZIKV infection in human kidney cells (46). In line with our findings, recent studies in human cells also report dramatic changes in carbohydrate concentrations after ZIKV infection (47). These alterations target proteins including enzymes, transcription regulators, transporters, and kinases associated with energy generation and immune cell activation. Thus, the downregulation of glucose, trehalose, and glycogen suggests that ZIKV hijacks host carbohydrate metabolism to secure the energy required for replication and virion production (48). Consequently, the glycolysis pathway could be a potential target against ZIKV and needs further investigation in the future.
It was previously also shown that ZIKV modulates host cell lipid metabolism, resulting in reduced fat body lipid droplets in Dicer-2 female mutants (11). More precisely, the reduced size of lipid droplets was linked to the decrease of triacylglycerides stores, causing lipodystrophy, a severe effect of ZIKV infection on fat body homeostasis (49). Our results show no cholesterol level differences among the various Drosophila experimental groups, suggesting that cholesterol is not involved in fly resistance against ZIKV and confirming triacylglycerols as the primary candidates for additional studies on lipid metabolism and energy homeostasis in the context of ZIKV infection.
The reduced metabolite levels can be also attributed to insulin signaling, which regulates both carbohydrate and lipid metabolism to maintain nutritional homeostasis and energy reserves during infection (27, 43, 49). In fact, Drosophila insulin signaling has been implicated in host antiviral defense by activating several other canonical immune pathways including RNAi, JAK/STAT, and autophagy, all of which are key determinants in vector competency and disease outcome (7, 11, 26, 36). Our results present evidence of severe reduction in insulin gene expression in both Dicer-2 female and male mutants, therefore revealing insulin signaling as a target involved in the regulation of ZIKV infection. To determine the correlation between the RNAi and insulin pathways during ZIKV infection, we used gene-specific primers to estimate Dicer-2 and Ago-2 expression in insulin mutants. It should be of note that gene expression was estimated between fly lines of the same background in this study to ensure similar basal levels of Dicer-2 and Ago-2 before viral infection (Figures S3A–D). Interestingly, infected female foxo and chico mutants exhibited a downregulation of Dicer-2, but only chico loss-of-function female mutants had a strong decrease in Ago-2. In contrast, infected male foxo and chico mutants presented reduced expression of Ago-2, but only chico male mutants had a reduced Dicer-2 expression. These sex-specific differences, parallel to the behavioral changes observed earlier, call for future exploration and explicit reporting of mechanisms underpinning immunity in both sexes. Overall, these results indicate a strong link between RNAi and insulin signaling, through which Drosophila hosts can combat ZIKV infection.
To further characterize the antiviral role of insulin signaling during ZIKV infection, we show for the first time that chico, which encodes an insulin receptor substrate that functions in the Drosophila insulin/insulin-like growth factor (IGF) signaling pathway, is required for an insulin-mediated antiviral response. Chico regulates the Drosophila insulin receptor (InR), which controls a diverse array of biological processes including cellular metabolism (50). Its essential role in cell growth regulation is confirmed by the small size of chico mutants, which are half the size of normal flies (51). Most importantly, the similarities of growth defects caused by chico mutations in Drosophila and insulin/IGF1 signaling pathway in vertebrates suggest that this pathway, chico in particular, plays a conserved role in antiviral immunity and metabolism during ZIKV infection (52, 53).
Remarkably, loss of function in the transcription factor foxo does not compromise fly survival ability in either female or male experimental groups upon ZIKV infection. Foxo is known for its association with nutritional signaling and induction of RNAi components during arboviral infection (54). However, the complex nature and interplay between immune pathways complicates endeavors to characterize the distinct roles of insulin signaling components, making imperative to further investigate foxo gene expression and the specific mechanism that insulin contributes to antiviral immunity. Higher viral copy numbers in the host also corresponded to the survival trends observed in this study. Infected chico mutants, both females and males, showed significantly increased levels of NS5 compared to background and PBS controls-treated flies. Together, these results confirm chico as indispensable for fly survival and resistance against ZIKV infection.
Taken together, our results show that Drosophila provides an ideal model system for identifying key regulators of host metabolism and revealing their vital functions in homeostasis and immunity during viral infection. Our findings reveal that ZIKV dramatically modifies host metabolism to ensure an optimal environment for its replication and spread. A better understanding of these metabolic alterations required for arboviruses, such as Zika, could lead to novel therapeutic approaches through targeted inhibition of specific cellular metabolic pathways.
Data Availability Statement
The raw data supporting the conclusions of this article will be made available by the authors, without undue reservation.
Author Contributions
GT-E designed and conducted the experiments, analyzed the data, constructed the figures, interpreted the results, and wrote drafts of the manuscript. AK conducted the experiments, analyzed the data, and constructed the figures. IE designed the experiments, interpreted the results, and revised the manuscript. All authors contributed to the article and approved the submitted version.
Funding
This work was supported by a supplement to grant R01AI110675 to IE from the National Institute of Allergy and Infectious Diseases.
Conflict of Interest
The authors declare that the research was conducted in the absence of any commercial or financial relationships that could be construed as a potential conflict of interest.
Publisher’s Note
All claims expressed in this article are solely those of the authors and do not necessarily represent those of their affiliated organizations, or those of the publisher, the editors and the reviewers. Any product that may be evaluated in this article, or claim that may be made by its manufacturer, is not guaranteed or endorsed by the publisher.
Acknowledgments
We thank members of the Eleftherianos lab for maintaining and amplifying the laboratory fly lines, and members of the Department of Biological Sciences at George Washington University for providing feedback to the project.
Supplementary Material
The Supplementary Material for this article can be found online at: https://www.frontiersin.org/articles/10.3389/fimmu.2022.903860/full#supplementary-material
References
1. Sasi MS, Rajendran R, Meenakshy V, Suresh T, Heera Pillai R, Dilip Kumar T, et al. Study on Vector Dynamics of Zika Virus Outbreak in Thiruvananthapuram, Kerala, India. Int J Curr Microbiol App Sci (2021) 10:54–71. doi: 10.20546/ijcmas.2021.1012.008
2. Zhang X, Li G, Chen G, Zhu N, Wu D, Wu Y, et al. Recent Progresses and Remaining Challenges for the Detection of Zika Virus. Med Res Rev (2021) 41:2039–108. doi: 10.1002/med.21786
3. Martins MM, Alves da Cunha AJL, Robaina JR, Raymundo CE, Barbosa AP, Medronho RA. Fetal, Neonatal, and Infant Outcomes Associated With Maternal Zika Virus Infection During Pregnancy: A Systematic Review and Meta-Analysis. PLoS One (2021) 16:e0246643. doi: 10.1371/journal.pone.0246643
4. Martins MM, Medronho RA, Cunha AJLAD. Zika Virus in Brazil and Worldwide: A Narrative Review. Paediatr Int Child Health (2021) 41:28–35. doi: 10.1080/20469047.2020.1776044
5. Espinal MA, Andrus JK, Jauregui B, Waterman SH, Morens DM, Santos JI, et al. Emerging and Reemerging Aedes-Transmitted Arbovirus Infections in the Region of the Americas: Implications for Health Policy. Am J Public Health (2019) 109:387–92. doi: 10.2105/AJPH.2018.304849
6. Mussabekova A, Daeffler L, Imler JL. Innate and Intrinsic Antiviral Immunity in Drosophila. Cell Mol Life Sci (2017) 74:2039–54. doi: 10.1007/s00018-017-2453-9
7. Trammell CE, Goodman AG. Emerging Mechanisms of Insulin-Mediated Antiviral Immunity in Drosophila Melanogaster. Front Immunol (2019) 20:2973. doi: 10.3389/fimmu.2019.02973
8. Hughes TT, Allen AL, Bardin JE, Christian MN, Daimon K, Dozier KD, et al. Drosophila as a Genetic Model for Studying Pathogenic Human Viruses. Virol (2012) 423:1–5. doi: 10.1016/j.virol.2011.11.016
9. Tafesh-Edwards G, Eleftherianos I. Drosophila Immunity Against Natural and Nonnatural Viral Pathogens. Virol (2020) 15:165–71. doi: 10.1016/j.virol.2019.12.001
10. Palmer WH, Dittmar M, Gordesky-Gold B, Hofmann J, Cherry S. Drosophila Melanogaster as a Model for Arbovirus Infection of Adult Salivary Glands. Virol (2020) 543:1–6. doi: 10.1016/j.virol.2020.01.010
11. Harsh S, Ozakman Y, Kitchen SM, Paquin-Proulx D, Nixon DF, Eleftherianos I. Dicer-2 Regulates Resistance and Maintains Homeostasis Against Zika Virus Infection in. Drosophila. J Immunol (2018) 201:3058–72. doi: 10.4049/jimmunol.1800597
12. Harsh S, Fu Y, Kenney E, Han Z, Eleftherianos I. Zika Virus Non-Structural Protein NS4A Restricts Eye Growth in Drosophila Through Regulation of JAK/STAT Signaling. Dis Model Mech (2020) 13:dmm040816. doi: 10.1242/dmm.040816
13. Liu Y, Cherry S. Zika Virus Infection Activates Sting-Dependent Antiviral Autophagy in the Drosophila Brain. Autophagy (2019) 15:174–5. doi: 10.1080/15548627.2018.1528813
14. Link N, Chung H, Jolly A, Withers M, Tepe B, Arenkiel BR, et al. Mutations in ANKLE2, a ZIKA Virus Target, Disrupt an Asymmetric Cell Division Pathway in Drosophila Neuroblasts to Cause Microcephaly. Dev Cell (2019) 51:713–29.e6. doi: 10.1016/j.devcel.2019.10.009
16. Skalsky RL, Cullen BR. Viruses, MicroRNAs, and Host Interactions. Annu Rev Microbiol (2010) 64:123–41. doi: 10.1146/annurev.micro.112408.134243
17. Buchon N, Silverman N, Cherry S. Immunity in Drosophila Melanogaster — From Microbial Recognition to Whole-Organism Physiology. Nat Rev Immunol (2014) 14:796–810. doi: 10.1038/nri3763
18. Hoffmann JA, Reichhart JM. Drosophila Innate Immunity: An Evolutionary Perspective. Nat Immunol (2002) 3:121–6. doi: 10.1038/ni0202-121
19. Hanson MA, Lemaitre B. New Insights on Drosophila Antimicrobial Peptide Function in Host Defense and Beyond. Curr Opin Immunol (2020) 62:22–30. doi: 10.1016/j.coi.2019.11.008
20. Ferrandon D, Imler JL, Hetru C, Hoffmann JA. The Drosophila Systemic Immune Response: Sensing and Signalling During Bacterial and Fungal Infections. Nat Rev Immunol (2007) 7:862–74. doi: 10.1038/nri2194
21. Schneider J, Imler JL. Sensing and Signalling Viral Infection in Drosophila. Dev Comp Immunol (2021) 117:103985. doi: 10.1016/j.dci.2020.103985
22. Swevers L, Liu J, Smagghe G. Defense Mechanisms Against Viral Infection in Drosophila: RNAi and Non-RNAi. Viruses (2018) 10:230. doi: 10.3390/v10050230
23. Wang PH, He JG. Nucleic Acid Sensing in Invertebrate Antiviral Immunity. Int Rev Cell Mol Biol (2019) 345:287–360. doi: 10.1016/bs.ircmb.2018.11.002
24. Wang XH, Aliyari R, Li WX, Li HW, Kim K, Carthew R, et al. RNA Interference Directs Innate Immunity Against Viruses in Adult Drosophila. Science (2006) 312:452–4. doi: 10.1126/science.1125694
25. Lyu B, Wang C, Bie Y, Kong J, Wang A, Jin L, et al. Enoxacin Shows Broad-Spectrum Antiviral Activity Against Diverse Viruses by Enhancing Antiviral RNA Interference in Insects. Virol (2022) 96(4):e0177821. doi: 10.1128/JVI.01778-21
26. Liu Y, Gordesky-Gold B, Leney-Greene M, Weinbren NL, Tudor M, Cherry S. Inflammation-Induced, STING-Dependent Autophagy Restricts Zika Virus Infection in the Drosophila Brain. Cell Host Microbe (2018) 24:57–68.e3. doi: 10.1016/j.chom.2018.05.022
27. Mattila J, Hietakangas V. Regulation of Carbohydrate Energy Metabolism in Drosophila Melanogaster. Genetics (2017) 207:1231–53. doi: 10.1534/genetics.117.199885
28. Livak KJ, Schmittgen TD. Analysis of Relative Gene Expression Data Using Realtime Quantitative PCR, and the 2–11CT Method. Methods (2001) 25:402–8. doi: 10.1006/meth.2001.1262
29. Schmittgen TD, Livak KJ. Analyzing Real-Time PCR Data by the Comparative CT Method. Nat Protoc (2008) 3:1101–8. doi: 10.1038/nprot.2008.73
30. Deshpande SA, Carvalho GB, Amador A, Phillips AM, Hoxha S, Lizotte KJ, et al. Quantifying Drosophila Food Intake: Comparative Analysis of Current Methodology. Nat Methods (2014) 11:535–40. doi: 10.1038/nmeth.2899
31. Tennessen JM, Barry WE, Cox J, Thummel CS. Methods for Studying Metabolism in Drosophila. Methods (2014) 68:105–15. doi: 10.1016/j.ymeth.2014.02.034
32. Vale PF, Jardine MD. Infection Avoidance Behavior: Viral Exposure Reduces the Motivation to Forage in Female Drosophila Melanogaster. Fly (Austin) (2017) 11:3–9. doi: 10.1080/19336934.2016.1207029
33. Vale PF, Jardine MD. Sex-Specific Behavioural Symptoms of Viral Gut Infection and Wolbachia in Drosophila Melanogaster. J Insect Physiol (2015) 82:28–32. doi: 10.1016/j.jinsphys.2015.08.005
34. Barron DG, Gervasi SS, Pruitt JN, Martin LB. Behavioral Competence: How Host Behaviors Can Interact to Influence Parasite Transmission Risk. Curr Opin Behav Sci (2015) 6:35–40. doi: 10.1016/j.cobeha.2015.08.002
35. Teleman AA. Molecular Mechanisms of Metabolic Regulation by Insulin in Drosophila. Biochem J (2009) 425:13–26. doi: 10.1042/BJ20091181
36. Ahlers LRH, Trammell CE, Carrell GF, Mackinnon S, Torrevillas BK, Chow CY, et al. Insulin Potentiates JAK/STAT Signaling to Broadly Inhibit Flavivirus Replication in Insect Vectors. Cell Rep (2019) 29(7):1946–60.e5. doi: 10.1016/j.celrep.2019.10.029
37. Deddouche S, Matt N, Budd A, Mueller S, Kemp C, Galiana-Arnoux D, et al. The DExD/H-Box Helicase Dicer-2 Mediates the Induction of Antiviral Activity in Drosophila. Nat Immunol (2008) 9:1425–32. doi: 10.1038/ni.1664
38. Lim SP, Noble CG, Shi PY. The Dengue Virus NS5 Protein as a Target for Drug Discovery. Antiviral Res (2015) 119:57–67. doi: 10.1016/j.antiviral.2015.04.010
39. Wang B, Tan XF, Thurmond S, Zhang ZM, Lin A, Hai R, et al. The Structure of Zika Virus NS5 Reveals a Conserved Domain Conformation. Nat Commun (2017) 8:14763. doi: 10.1038/ncomms14763
40. Saw WG, Chan KW, Vasudevan SG, Grüber G. Zika Virus Nonstructural Protein 5 Residue R681 Is Critical for Dimer Formation and Enzymatic Activity. FEBS Lett (2019) 593:1272–91. doi: 10.1002/1873-3468.13437
41. Hillyer JF. Insect Immunology and Hematopoiesis. Dev Comp Immunol (2016) 58:102–18. doi: 10.1016/j.dci.2015.12.006
42. Snyder-Keller A, Kramer LD, Zink S, Bolivar VJ. Mouse Strain and Sex-Dependent Differences in Long-Term Behavioral Abnormalities and Neuropathologies After Developmental Zika Infection. J Neurosci (2019) 39:5393–403. doi: 10.1523/JNEUROSCI.2666-18.2019
43. Chatterjee N, Perrimon N. What Fuels the Fly: Energy Metabolism in Drosophila and its Application to the Study of Obesity and Diabetes. Sci Adv (2021) 7:eabg4336. doi: 10.1126/sciadv.abg4336
44. Owusu-Ansah E, Perrimon N. Modeling Metabolic Homeostasis and Nutrient Sensing in Drosophila: Implications for Aging and Metabolic Diseases. Dis Model Mech (2014) 7(3):343–50. doi: 10.1242/dmm.012989
45. Fontaine KA, Sanchez EL, Camarda R, Lagunoff M. Dengue Virus Induces and Requires Glycolysis for Optimal Replication. J Virol (2015) 89:2358–66. doi: 10.1128/JVI.02309–14
46. Reslan A, Haddad JG, Moukendza Koundi L, Desprès P, Bascands JL, Gadea G. Zika Virus Growth in Human Kidney Cells Is Restricted by an Elevated Glucose Level. Int J Mol Sci (2021) 22:2495. doi: 10.3390/ijms22052495
47. Rashid MU, Lao Y, Spicer V, Coombs KM. Zika Virus Infection of Sertoli Cells Alters Protein Expression Involved in Activated Immune and Antiviral Response Pathways, Carbohydrate Metabolism and Cardiovascular Disease. Viruses (2022) 14:377. doi: 10.3390/v14020377
48. Sanchez EL, Lagunoff M. Viral Activation of Cellular Metabolism. Virol (2015) 479–480:609–18. doi: 10.1016/j.virol.2015.02.038
49. Liu Z, Huang X. Lipid Metabolism in Drosophila: Development and Disease. Acta Biochim Biophys Sin (Shanghai) (2013) 45:44–50. doi: 10.1093/abbs/gms105
50. Li CR, Guo D, Pick L. Independent Signaling by Drosophila Insulin Receptor for Axon Guidance and Growth. Front Physiol (2014) 4:385. doi: 10.3389/fphys.2013.00385
51. Böhni R, Riesgo-Escovar J, Oldham S, Brogiolo W, Stocker H, Andruss BF, et al. Autonomous Control of Cell and Organ Size by CHICO, a Drosophila Homolog of Vertebrate IRS1-4. Cell (1999) 97:865–75. doi: 10.1016/s0092-8674(00)80799-0
52. Clancy DJ, Gems D, Harshman LG, Oldham S, Stocker H, Hafen E, et al. Extension of Life-Span by Loss of CHICO, a Drosophila Insulin Receptor Substrate Protein. Science (2001) 292:104–6. doi: 10.1126/science.1057991
Keywords: Drosophila, Zika virus, innate immunity, RNA interference, metabolism
Citation: Tafesh-Edwards G, Kalukin A and Eleftherianos I (2022) Zika Virus Induces Sex-Dependent Metabolic Changes in Drosophila melanogaster to Promote Viral Replication. Front. Immunol. 13:903860. doi: 10.3389/fimmu.2022.903860
Received: 24 March 2022; Accepted: 06 June 2022;
Published: 30 June 2022.
Edited by:
Akira Goto, Institut National de la Santé et de la Recherche Médicale (INSERM), FranceReviewed by:
Erjun Ling, Shanghai Institutes for Biological Sciences (CAS), ChinaIlias Kounatidis, The Open University, United Kingdom
Copyright © 2022 Tafesh-Edwards, Kalukin and Eleftherianos. This is an open-access article distributed under the terms of the Creative Commons Attribution License (CC BY). The use, distribution or reproduction in other forums is permitted, provided the original author(s) and the copyright owner(s) are credited and that the original publication in this journal is cited, in accordance with accepted academic practice. No use, distribution or reproduction is permitted which does not comply with these terms.
*Correspondence: Ioannis Eleftherianos, aW9hbm5pc2VAZ3d1LmVkdQ==