- 1Leeds Institute of Medical Research at St James’s, University of Leeds, Wellcome Trust Brenner Building, St James’s University Hospital, Leeds, United Kingdom
- 2Ludwig Institute for Cancer Research, University of Lausanne, Epalinges, Switzerland
T cell activation is dependent upon the integration of antigenic, co-stimulatory and cytokine-derived signals and the availability and acquisition of nutrients from the environment. Furthermore, T cell activation is accompanied by reprogramming of cellular metabolism to provide the energy and building blocks for proliferation, differentiation and effector function. Transforming growth factor β (TGFβ) has pleiotropic effects on T cell populations, having both an essential role in the maintenance of immune tolerance but also context-dependent pro-inflammatory functions. We set out to define the mechanisms underpinning the suppressive effects of TGFβ on mouse CD8+ T cell activation. RNA-sequencing analysis of TCR-stimulated T cells determined that Myc-regulated genes were highly enriched within gene sets downregulated by TGFβ. Functional analysis demonstrated that TGFβ impeded TCR-induced upregulation of amino acid transporter expression, amino acid uptake and protein synthesis. Furthermore, TCR-induced upregulation of Myc-dependent glycolytic metabolism was substantially inhibited by TGFβ treatment with minimal effects on mitochondrial respiration. Thus, our data suggest that inhibition of Myc-dependent metabolic reprogramming represents a major mechanism underpinning the suppressive effects of TGFβ on CD8+ T cell activation.
Introduction
CD8+ T cell function is determined by the sum of inputs received from the environment, including antigenic and co-stimulatory signals, cytokines, or nutrient availability. In recent years, research focused on describing the metabolic regulation of immunity has defined cell-intrinsic modulatory networks that tightly regulate T cell responses through the integration of these environmental cues. For example, CD8+ T cells respond to TCR-stimulation by inducing metabolic reprogramming in part mediated by the activation of the transcription factor Myc (1). Myc activity boosts aerobic glycolysis and the upregulation of amino acid transporters such as SLC7A5, which are crucial to sustain the energetic and biosynthetic demands required for clonal expansion and differentiation (1–3). On the other hand, signals such as CD28 co-stimulation (4), or the cytokines IL-7 and IL-15 (5, 6), have been shown to promote mitochondrial metabolism, key to determine the engagement of memory formation. Moreover, the manipulation of metabolic pathways has proved to be a powerful tool to shape T cell activity and develop therapeutic strategies (7). Thus, studying how diverse signals influence T cell metabolism provides valuable knowledge to further understand regulatory mechanisms of T cell function (or dysfunction) and find therapeutic approaches.
Transforming growth factor β (TGFβ) is a pleiotropic cytokine that acts on both immune and non-immune cells, regulating many biological processes including cell migration, wound healing and angiogenesis. In T lymphocytes, TGFβ is involved in the development and suppressive activity of regulatory T cells as well as in the restriction of effector T cell activation, therefore playing an important function in preventing autoimmunity. Furthermore, TGFβ is a key immunosuppressive cytokine secreted by pro-tumour cells and cancer cells to mediate immune evasion and promote metastasis. Previous work determined that TGFβ inhibits mitochondrial respiration and limits IFNγ secretion of human CD4+ T cells through direct interaction of SMAD proteins with the mitochondria (8). Further reports demonstrated that TGFβ restricts mitochondrial metabolism and glycolysis in NK cells by decreasing mechanistic target of rapamycin (mTOR) activity (9, 10). It has been more recently described that TGFβ can also limit mTOR activity in CD8+ T cells which, paradoxically, promotes mitochondrial metabolism and protects T cells from entering a terminally exhausted phenotype in the context of LCMV-induced chronic stimulation (11). These discrepancies indicate that the roles and mechanisms of TGFβ in the immune response are highly variable depending on cell type and context.
In the current work, we describe how TGFβ modulates early stages of CD8+ T cell activation. Transcriptomic analysis identified the transcription factor Myc as a key target of TGFβ signalling. In this regard, TGFβ treatment restricted TCR-induced Myc-dependent metabolic reprogramming. Specifically, TGFβ-treated CD8+ T cells display impaired expression of genes involved in glycolytic and amino acid (AA) metabolism which subsequently limited nutrient uptake and appropriate engagement of glycolysis or protein synthesis upon T cell activation. This study sheds light into a mechanism by which TGFβ regulates early CD8+ T cell activation and metabolism through inhibition of Myc expression.
Materials and methods
Mice
All experiments were performed using OT-I Rag1-/- or OT-I mice. OT-I Rag1-/- mice were maintained at the University of Leeds St. James’s Biomedical Services (SBS). OT-I mice were maintained at the animal facility of University of Lausanne. All experiments were performed using age- and sex-matched mice (7-12 weeks).
Cell lines
ID8 ovarian carcinoma cells expressing ovalbumin variants (ID8-OVA-N4 and ID8-OVA-T4) were originally a gift from Prof. Dietmar Zehn (Technical University of Munich, Germany). ID8-OVA-N4 and ID8-OVA-T4 cells were maintained in IMDM supplemented with 5% FBS, 50μM β-mercaptoethanol, penicillin-streptomycin and ciprofloxacin (0.5%).
Cell culture
OT-I T cells were obtained lymph from nodes or spleens of OT-I or OT-I Rag1-/- transgenic mice. Briefly, lymphoid organs were dissociated using a 70 μM filter and single cell suspensions prepared. Purity of CD8 T cells prepared from OT-I Rag1-/- lymph nodes was typically >90%. OT-I T lymphocytes were cultured in Iscove’s Modified Dulbecco’s Medium (IMDM, Gibco) supplemented with 5% FBS (Gibco), penicillin-streptomycin (Gibco) and β-mercaptoethanol (Gibco, 50μM). OT-I T cells were activated with 10-8 SIINFEKL or SIITFEKL (Cambridge Peptides) in the presence or absence of recombinant human TGFβ (Peprotech, 5ng/ml). Where indicated, OT-I T cells were maintained unactivated in vitro (‘No TCR’ condition) with recombinant mouse IL-7 (Peprotech, 10ng/ml). For IL-2 replenishment experiments, recombinant human IL-2 (Peprotech, 1ng/ml) was added to the wells at timepoint 0h. For IL-2 secretion experiments, OT-I T cells were cultured in the presence of blocking CD25 mAb (Biolegend). After 24h of stimulation, supernatants were collected and IL-2 concentration was determined using the Mouse DuoSet IL-2 ELISA (R&D Systems), following manufacturer’s instructions. For ID8-OT-I co-culture experiments, either ID8-OVA-N4 or ID8-OVA-T4 cells were seeded in 48-well plates at 2x104 cells/well. After 4h of incubation to allow adhesion, freshly isolated OT-I T cells were added into the wells (2x105 cells/well) and cultured for 48h. Brefeldin A (2.5mg/ml) was added during the last 6h of culture for optimal detection of cytokine expression by flow cytometry.
Flow cytometry
The following conjugated antibodies were used: CD8β-phycoerythrin cynanine 7 (PE-Cy7) (clone YTS156.7.7, 1:400, BioLegend), CD25–PE (clone 3C7, 1:200, BioLegend), CD44–allophycocyanin (APC)-Cy7 (clone IM7, 1:200, BioLegend), CD71–fluorescein isothiocyanate (FITC) (clone RI7217, 1:200, BioLegend), CD98–peridinin chlorophyll protein (PerCP) Cy5.5 (clone RL388, 1:200, BioLegend), IFNγ–alexafluor (AF) 488 (clone XMG1.2, 1:100, BioLegend), Granzyme B– brilliant violet (BV) 421 (clone GB11, 1:100, BioLegend), c-Myc–APC (clone D84C12, 1:50, Cell Signalling Technologies), GLUT1–AF488 (clone EPR3915, 1:400, Abcam) phospho-mTOR Ser2448-PE (clone MRRBY, 5μL/sample, eBioscience). ASCT2 (clone V501, 1:50, Cell Signalling Technologies) and phosphoS6 Ser240/244 (clone D68F8, 1:200, Cell Signalling Technologies) were stained with unconjugated antibodies. An additional staining was performed with secondary goat Anti-Rabbit IgG Pacific Blue (1:1000, Life Technologies). For assessment of intracellular markers, cells were fixed and permeabilised using FoxP3 fix/perm buffer (eBioscience). For live/dead discrimination, LD Aqua or Zombie NIR were used (LifeTechnologies and BioLegend, respectively). For assessment of protein synthesis, cells were treated with O-propargyl-puromycin (OPP, 20μM, Jena Bioscience) for 30 minutes. OPP was detected using Click-iT Plus AF488 picolyl azide kit (Life Technologies). Cycloheximide (CHX, 100μg/ml, SigmaAldrich) was added as a negative control 15’ prior to OPP treatment. For amino acid uptake assays, T cells were treated with kynurenine (200μM in HBSS buffer, SigmaAldrich) ± 2-Aminobicyclo- (2,2,1)-heptane-2-carboxylic acid (BCH, 10mM in HBSS buffer, Cayman Chemicals) for 4 min at 37°C (12). After incubation, cells were fixed with 1% paraformaldehyde for 30 min at RT.
Seahorse metabolic analysis
For ECAR and OCR measurements, OT-I T cells were plated in a Cell-Tak coated (22.4μg/ml) Seahorse XFe96 Microplate (105 cells/well). For MitoStress tests, Seahorse assay media was supplemented with glucose (10mM), pyruvate (1mM) and glutamine (2mM). OCR values were acquired with Seahorse XFe96 Analyser at basal levels and upon sequential injection of oligomycin (1μM), carbonyl cyanide-p-trifluoromethoxyphenylhradone (FCCP, 1.5μM) and rotenone/Antimycin A (0.5μM), following standard Seahorse XFe96 protocol. For Glycolysis Stress Test, Seahorse media was supplemented with pyruvate (1mM) and glutamine (2mM), and ECAR values were acquired at basal levels and after sequential injection of glucose (10mM), oligomycin (1μM) and 2-deoxyglucose (50mM), following standard Seahorse XFe96 protocol.
Lactate assay
To measure lactate secretion, 106 OT-I T cells were stimulated in vitro with OVA-T4 ± TGFβ in 48-well plates. After 24h, levels of lactate in deproteinised culture supernatants were assessed following manufacturer’s instructions (Kit 700510, Cayman Chemicals). Four biological replicates per condition were included.
RNA extraction and RNA-seq
OT-I T cells (5x106 cells/sample) were stimulated with OVA-T4 ± TGFβ, as described above. After 24h, cells were lysed with TRI Reagent® (Zymo Research, R2050-1-50) and RNA was extracted using Direct-zol™ RNA Miniprep Plus (Zymo Research, R2070), following manufacturers’ instructions. RNA purity was checked using Nano Drop™ spectrophotometer (A260/A280 and A260/A230 > 1.8). RNA-seq and data analysis was performed by Novogene Bioinformatic Technology Co. (Hong Kong). For Kyoto Encyclopaedia of Genes and Genomes (KEGG) pathway analysis and ChIP Enrichment Analysis (ChEA), RNA-Seq data was processed using Enrichr. All RNA-Seq data have been deposited at NCBI and are publicly accessible under GEO accession numbers: GSE205654, GSM6216059, GSM6216060, GSM6216061, GSM6216062, GSM6216063, GSM6216064, GSM6216065, GSM6216066.
Statistics
Statistical significance (p-value < 0.05) was determined performing Student’s t-test, Mann-Whitney test, and one- or two- way ANOVA with Tukey’s multiple comparison tests using GraphPad Prism, as described in figure legends. Dots in graphs represent replicate samples and error bars represent SDs, unless otherwise stated. For RNA-Seq data, statistical significance was determined using DESeq2 package and p-values were adjusted with the Benjamini-Hochberg procedure.
Study approval
Mouse breeding and experiments performed at University of Leeds (UoL) were reviewed by the UoL Animal Welfare and Ethical Review Committee (AWERC) and were approved by and subject to the conditions of a UK Home Office Project Licence (PDAD2D507) held by RJS.
Results
TGFβ preferentially inhibits CD8+ T cell responses to low affinity antigen
To define the inhibitory capacity of TGFβ on CD8+ T cell activation, OT-I TCR transgenic T cells were activated with high affinity ovalbumin peptide SIINFEKL (OVA-N4) or low affinity SIITFEKL (OVA-T4) ± TGFβ for 48 hours. Assessment of forward scatter-side scatter parameters by flow cytometry demonstrated that TGFβ treatment limited TCR-induced OT-I T cell growth with a more prominent effect on low affinity OVA-T4-induced responses (Figure 1A). TGFβ inhibited OVA-N4-induced granzyme B expression but had little effect on surface expression levels of either CD25 or CD71 (Figure 1B). By contrast, TGFβ treatment strongly inhibited low affinity OVA-T4-induced CD25, CD71 and granzyme B expression (Figure 1C). As previously reported (13), CD44 expression was elevated following peptide + TGFβ treatment as compared to peptide alone, irrespective of antigen affinity (Figures 1B, C).
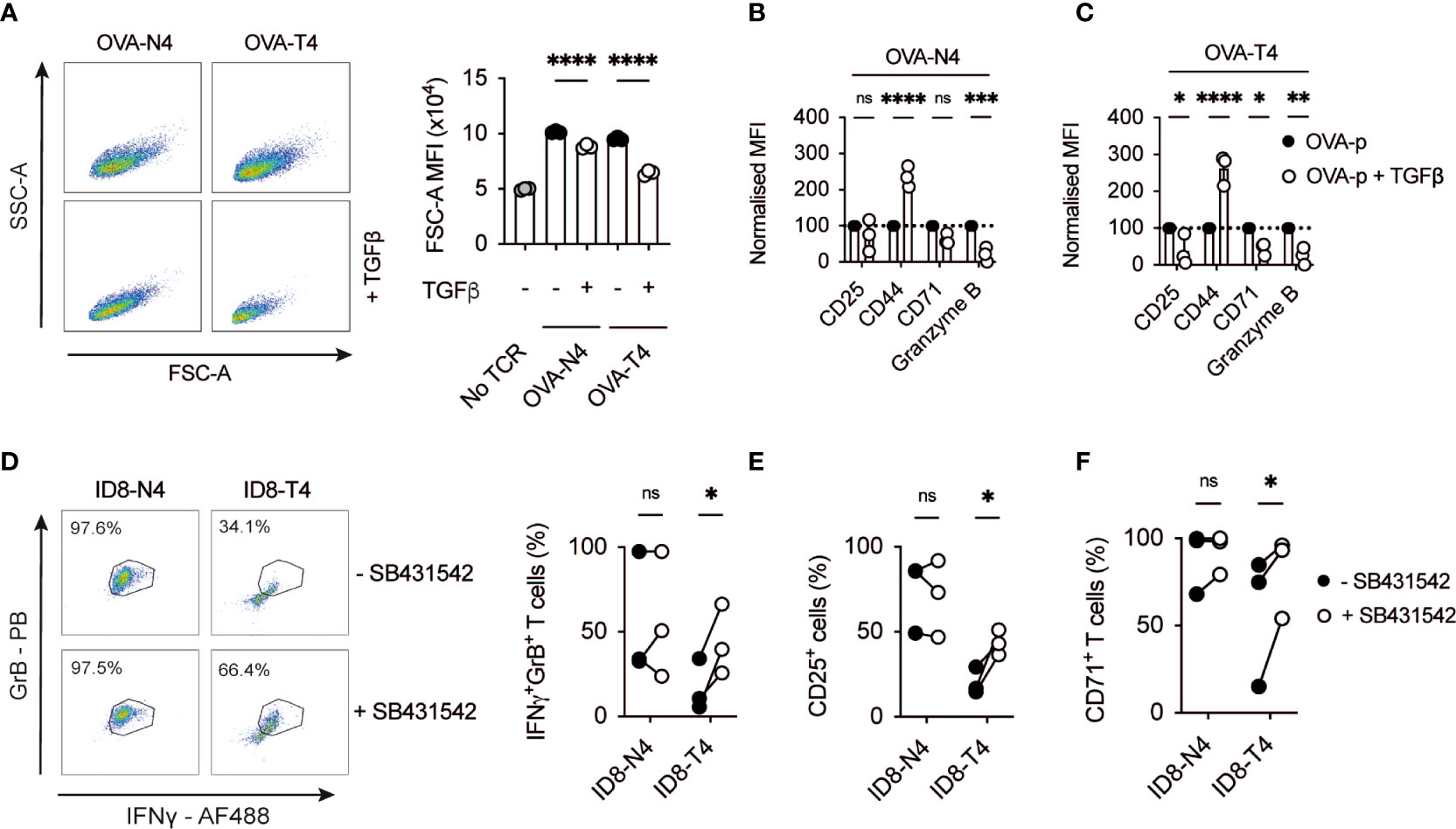
Figure 1 TGFβ inhibits CD8+ T cell activation in response to low affinity antigen (A-C) OT-I T cell receptor (TCR) transgenic T cells were stimulated with cognate SIINFEKL (OVA-N4) or low affinity SIITFEKL (OVA-T4) peptides in the presence or absence of TGFβ for 48 hours in vitro. (A) T cell growth was assessed by analysis of forward and side scatter area (FSC-A/SSC-A) by FACS. (B, C) Cell surface expression of activation markers (CD25, CD44, CD71) or intracellular granzyme B were determined by flow cytometry. Data shown represent mean fluorescence intensity (MFI) values for markers, normalised to control (TCR-stimulated) levels. (D–F) OT-I T cells were co-cultured in the presence of ID8-OVA-N4 or ID8-OVA-T4 ovarian carcinoma cells in the presence or absence of TGFβRII inhibitor SB431542 for 48 hours in vitro. Values in dotplots represent % Granzyme B-IFNγ double-positive cells. (D) Data show representative FACS dotplots of intracellular IFNγ and granzyme B expression. Cell surface expression of CD25 (E) and CD71 (F) were determined by flow cytometry. Data points joined by lines represented paired SB431542-treated and untreated samples (E, F). Data are from 1 of 3 experiments (A–C) or are pooled data from 3 experiments (D–F). Individual data points represent technical replicates (A–C) or biological replicates (D-F) (n=3). NS – not significant, * p<0.05, ** p<0.01, *** p<0.001, **** p<0.0001 as assessed by 1-way ANOVA, with Tukey’s multiple comparisons test.
Many cancer cell types, including ovarian carcinomas, secrete TGFβ to suppress T cell responses (14–16). We took advantage of mouse ID8 ovarian carcinoma cell lines expressing OVA-peptides to assess the impact of tumour cell-derived TGFβ on OT-I T cell activation. FACS analysis demonstrated that a higher proportion of OT-I T cells co-expressed granzyme B and IFNγ following co-culture for 48h in the presence of ID8 cells expressing OVA-N4 as compared to OVA-T4 (Figure 1D). Addition of the TGFβRII inhibitor SB431542 to OT-I:ID8-OVA-T4 co-cultures doubled the proportions of IFNγ+granzyme B+ OT-I cells, but did not impact upon OT-I T cell responses to ID8-OVA-N4 tumour cells. Similarly, OT-I expression of CD25 and CD71 following co-culture with ID8-OVA-T4, but not ID8-OVA-N4 cells, were elevated upon TGFβRII blockade (Figures 1E, F). Together, these data indicate that both recombinant and tumour cell-derived TGFβ limit TCR-induced T cell activation with a substantially greater effect on responses to lower affinity antigens.
TGF β inhibits the TCR-induced Myc-dependent transcriptome
To define the global impact of TGFβ on the TCR-induced transcriptional programme, OT-I CD8+ T cells were stimulated for 24h with OVA-T4 ± TGFβ and RNA-Sequencing performed. A total of 5800 transcripts were found to be differentially expressed (adjusted p value < 0.05, no fold-change cut-off) between TGFβ−treated and -untreated groups, with 3248 upregulated and 2552 downregulated (Figure 2A). The well-characterized TGFβ target genes Gzmb and Eomes were amongst the most-downregulated, whilst chemokine receptor-encoding Ccr8 was amongst the most-upregulated (Figure 2B). Kyoto Encyclopedia of Genes and Genomes (KEGG) pathway analysis of the TGFβ-upregulated gene list (fold-change > 1.5, n=2141) identified “Th17 cell differentiation”, “Leishmaniasis” and “Inflammatory bowel disease” to be among the top upregulated pathways (Supplementary Table 1), consistent with previously described roles for TGFβ in Th17/Tc17/Treg differentiation and function (16, 17).
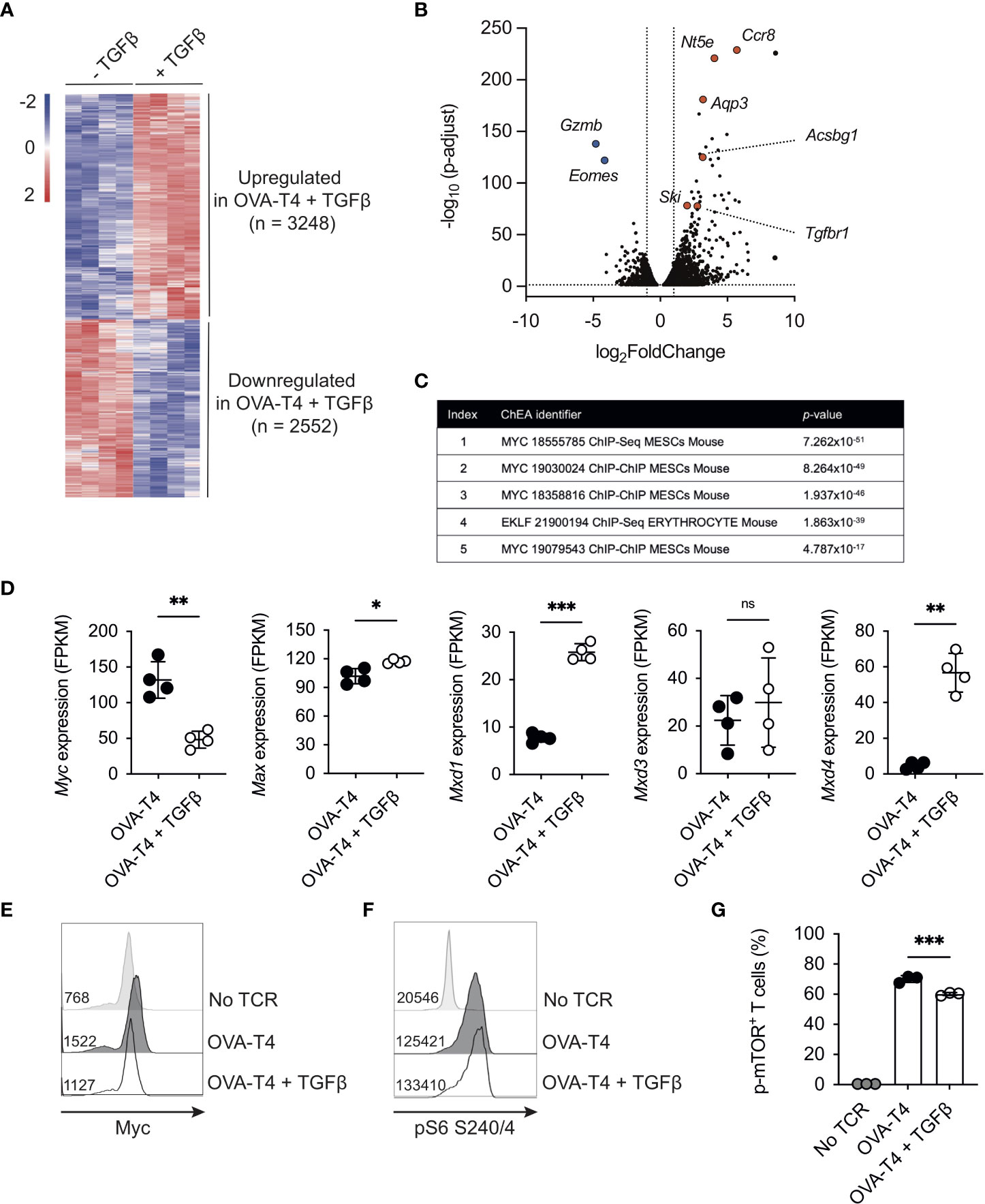
Figure 2 TGFβ impedes the Myc-dependent transcriptome OT-I TCR transgenic T cells were stimulated with SIITFEKL (OVA-T4) peptide in the presence or absence of TGFβ for 24 (A–D, F, G) or 48 (E) hours. RNA-Sequencing (RNA-Seq) analysis was performed (A) The heatmap shows log2 fold changes of differentially expressed genes (p adjust <0.05). (B) Volcano plot of RNA-Seq data identifies genes substantially downregulated (Gzmb, Eomes) or upregulated (Nt5e, Ccr8, Aqp3, Ski, Tgfbr1, Acsbg1) in TGFβ -treated OT-I T cells. (C) ChIP-X enrichment analysis (ChEA) within the Enrichr platform identified Myc-regulated genes as highly enriched within the TGFβ-downregulated gene set. (D) Levels of gene expression of Myc and Myc-associated genes. Data shown represent fragments per kilobase million (FPKM) values taken from RNA-Seq analysis. Histograms show levels of Myc expression (E) and phosphorylated ribosomal protein S6 (pS6) (F) whilst barcharts show proportions of total OT-I cells expressing phospho-Ser2448 mTOR (G) as determined by intracellular staining and flow cytometry. In histograms, figures represent MFI values for each condition. Individual data points from RNA-Seq data (A–D) represent biological replicates (n=4), whilst FACS plots represent data from 1 of 3 repeated experiments (E, F) or technical replicates (n=3) from 1 of 2 repeated experiments (G). For RNA-Seq data, NS – not significant, * p<0.05, ** p<0.01, *** p<0.001 as determined using DESeq2 package with p-values adjusted using Benjamini-Hochberg procedure. For (G), *** p<0.001 as determined by one way ANOVA, with Tukey's multiple comparisons test.
We focused on analysis of the transcripts downregulated following OVA-T4 + TGFβ treatment (“TGFβ-down”, n = 1139, fold-change > 1.5). Use of the ChIP-X Enrichment Analysis (ChEA) tool (18) within the Enrichr platform (19) identified Myc target genes as highly enriched within the TGFβ-down gene list (Figure 2C). MYC was previously identified as a TGFβ-regulated, SMAD-repressed gene in human keratinocytes (20), and to be transcriptionally downregulated in TGFβ treated mouse effector CD8+ T cells (21). Consistent with these previous reports, TCR-induced Myc mRNA (Figure 2D) and protein levels (Figure 2E, Supplementary Figure 1) were substantially impaired by TGFβ in OT-I T cells. By contrast, levels of Max mRNA, that encodes the Myc binding partner Myc-associated factor X (MAX) were marginally increased by TGFβ whereas levels of Mxd1 and Mxd4, encoding transcriptional repressor Max dimer (MAD) proteins, were substantially elevated (Figure 2D).
In T cells, Myc expression is predominantly regulated by a combination of TCR- and IL-2-dependent signals (22). Consistent with previous publications (13, 21) TGFβ reduced OVA-N4 and OVA-T4-induced OT-I T cell IL-2 production (Supplementary Figure 1A). We sought to determine whether a paucity of autocrine IL-2 production accounted for, or contributed to, reduced Myc expression in TGFβ-treated OT-I T cells. Il2 transcription peaks within the first 6-12h, then drops substantially, following T cell activation (23, 24). We reasoned that delaying the addition of TGFβ for 24h would uncouple indirect effects of reduced Il2 levels on Myc expression. When added after 24h of TCR-stimulation, TGFβ suppressed Myc expression, as assessed at 48h, albeit to a lesser extent than when added from timepoint 0h (Supplementary Figure 1B). To determine whether exogenous IL-2 could rescue Myc expression, OT-I T cells were stimulated with either OVA-N4 or OVA-T4 ± TGFβ ± IL-2. Data indicated that IL-2 replenishment had no effect on the levels of Myc expression in T cells stimulated with OVA-N4 and TGFβ, whereas it was slightly but not significantly increased in OVA-T4 stimulated T cells (Supplementary Figure 1C).
Previous studies have linked TGFβ-mediated suppression of NK cell function to inhibition of mTOR (25). Levels of TCR-induced phosphorylation of the mTORC1 downstream target ribosomal protein S6 were not impeded by TGFβ in OT-I T cells (Figure 2F). As TCR-induced rpS6 phosphorylation can be mediated via both mTORC1 and RSK MAPK pathways (26), we further assessed the impact of TGFβ on mTOR phosphorylation. In this regard, phosphorylation of mTOR at Ser2448 was only mildly reduced by TGFβ (Figure 2G). Together, these analyses indicate that TGFβ-mediated suppression of CD8+ T cell activation is linked to repression of Myc activity and expression, independent of effects on IL-2 production and with little impact on mTOR.
TGF β impairs amino acid uptake and protein synthesis
Myc transcriptional activity is required for TCR-induced T cell metabolic reprogramming. In particular, Myc-dependent T cell activation is strongly associated with the regulation of amino acid (AA) transporter expression and AA uptake (1, 3). Similarly, our RNA-Seq data indicated that TGFβ treatment resulted in decreased gene expression of several amino acid transporters including Slc1a5 (encoding Alanine/Serine/Cysteine-preferring transporter 2 [ASCT2]), Slc3a2 and Slc7a5 (encoding CD98/Large neutral amino acid transporter 1 [LAT1]) (Figure 3A). Furthermore, FACS analysis confirmed that TCR-induced upregulation of cell surface ASCT2 (Figure 3B) and CD98 (Figure 3C) was impeded by TGFβ. Kynurenine, a metabolite of tryptophan, can be detected by virtue of its spectral fluorescent properties to assess amino acid transport via SLC7A5/CD98 in T cells by flow cytometry (12). Consistent with decreased transcript and protein levels of CD98, uptake of kynurenine was reduced in TGFβ-treated OT-I T cells (Figure 3D), suggesting that TCR-induced upregulation of AA uptake was impaired. Importantly, incubation with BCH, an inhibitor of L-type amino acid transporters, blocked both basal and TCR-induced Kyn uptake of OT-I T cells, demonstrating the dependence for SLC7A5 in this assay (Figure 3D). A fundamental role for amino acids in cell biology is to act as building blocks for protein synthesis. Levels of nascent protein synthesis in activated T cells were assessed using incorporation of O-propargyl-puromycin (OPP) and flow cytometry. Importantly, TGFβ treatment substantially impaired TCR stimulated upregulation of OPP incorporation by OT-I T cells (Figures 3E).
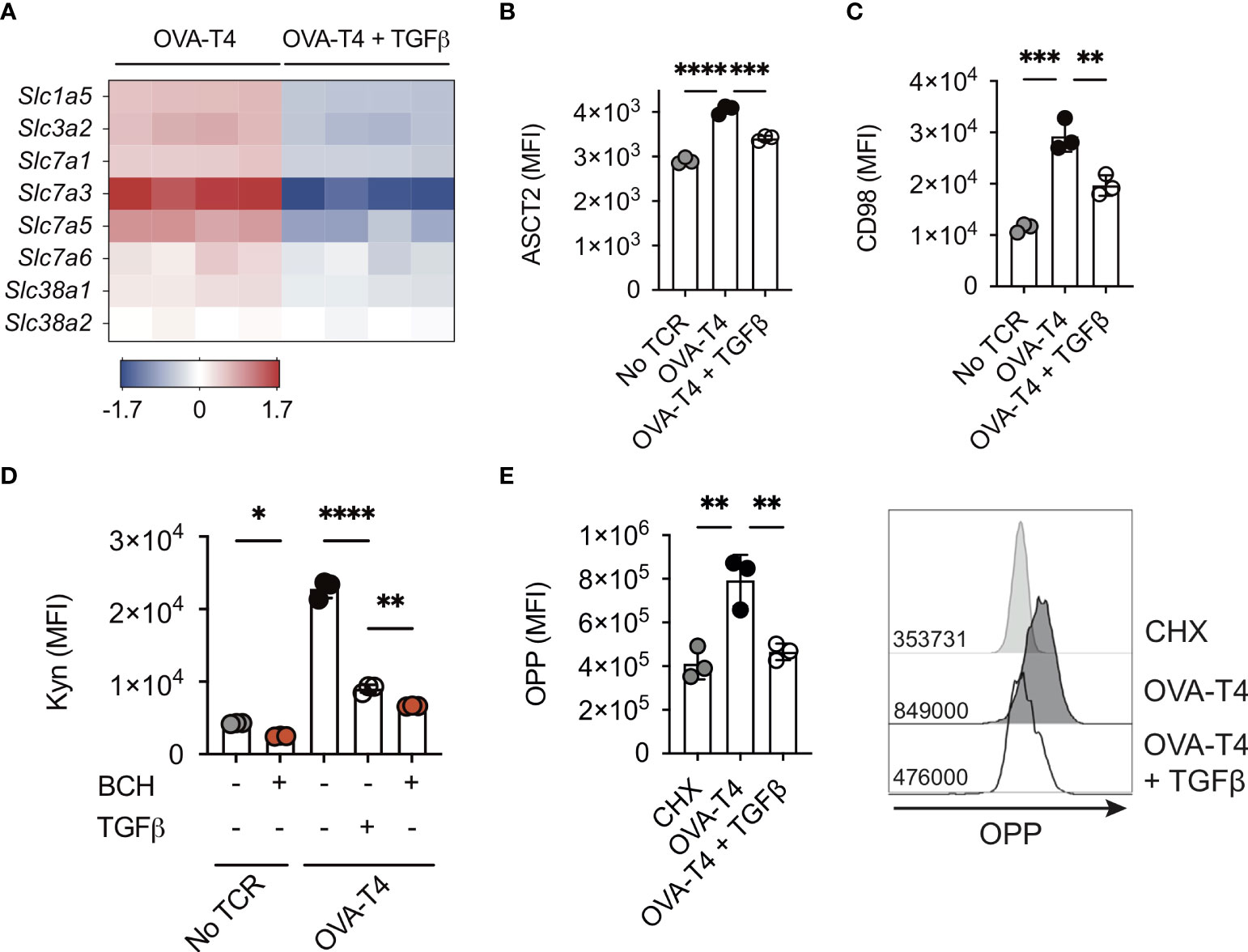
Figure 3 TGFβ impairs amino acid uptake and protein synthesis OT-I TCR transgenic T cells were stimulated with SIITFEKL (OVA-T4) peptide in the presence or absence of TGFβ for 24 (A) or 48 (B–E) hours. (A) The heatmap shows log2 fold changes in expression of selected amino acid transporter-encoding genes as determined by RNA-sequencing. Levels of surface expression of ASCT2 (B) and CD98 (C) and uptake of kynurenine (D) were determined by flow cytometry. To assess requirement for L-type amino acid transporters in Kyn uptake, cells were additionally treated with or without BCH (D). Incorporation of O-propargyl-puromycin (OPP) was detected by Click chemistry staining and flow cytometry (E). For flow cytometry analyses data shown represent mean fluorescence intensity (MFI) values. Individual data points represent biological replicates (A) or technical replicates (B–E) from 1 of 3 repeated experiments. * p<0.05, ** p<0.01, *** p<0.001, **** p<0.0001 as assessed by 1-way ANOVA, with Tukey’s multiple comparisons test.
TGF β inhibits TCR-induced upregulation of glycolytic metabolism
Myc expression also mediates the upregulation of glycolytic metabolism following TCR stimulation (1). Analysis of RNA-Seq data indicated that TGFβ modestly impaired mRNA expression of the majority of glycolytic enzymes, with the exception of Hk1, Aldoc and Ldh2b (Figure 4A). Furthermore, levels of Slc2a1 and Slc2a3 (encoding glucose transporters 1 and 3 (GLUT1/3), respectively) were significantly lower in OT-I T cells activated with OVA-T4 + TGFβ as compared to OVA-T4 alone. Consistent with RNA-Seq data, FACS analysis demonstrated that TCR-induced surface expression of GLUT1 protein was impaired in TGFβ-treated cells (Figure 4B). Furthermore, analysis of lactate secretion (Figure 4C) and extracellular acidification rate (ECAR) (Figures 4D–F) determined that TGFβ substantially impairs TCR-induced upregulation of glycolytic metabolism.
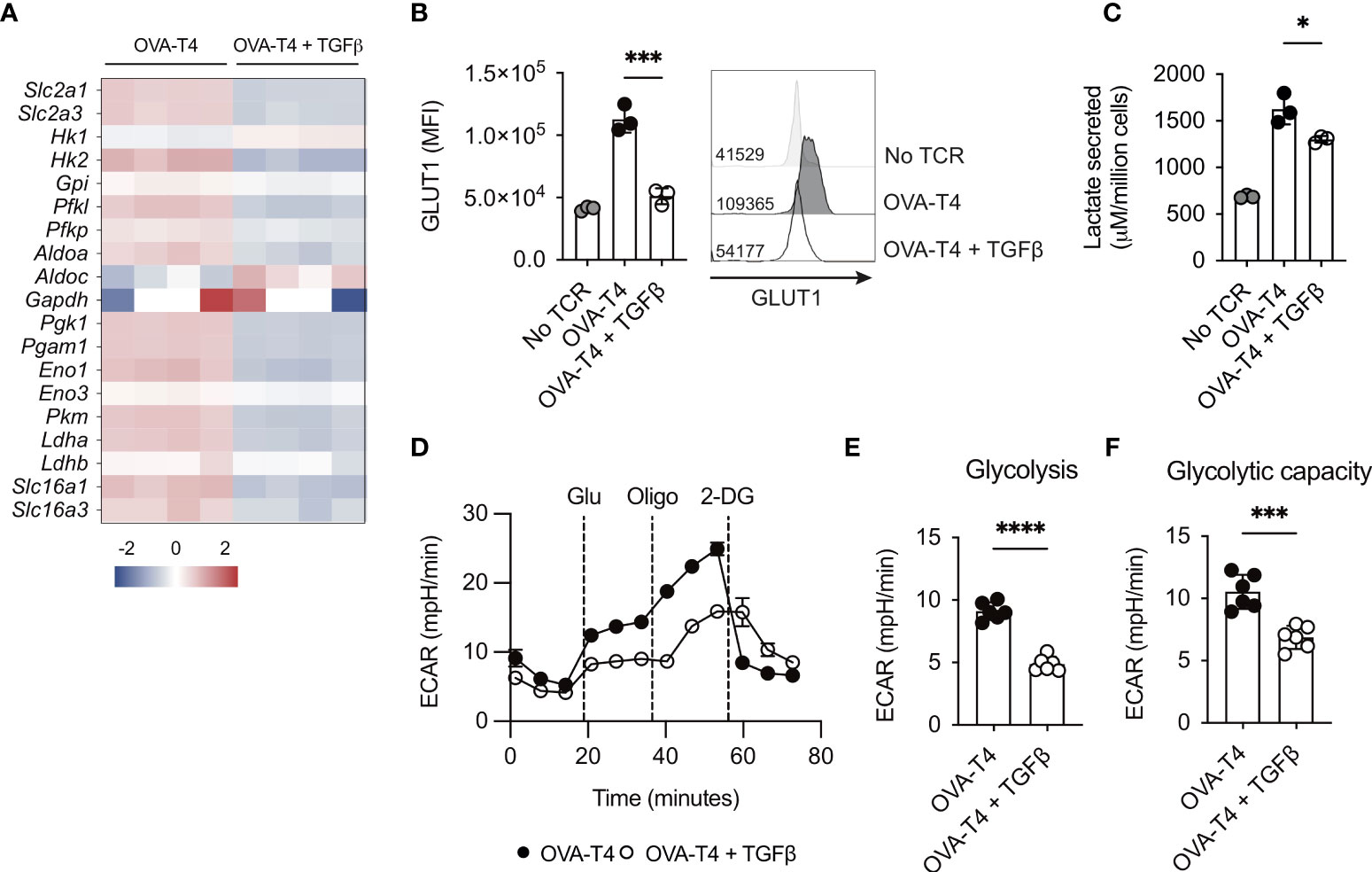
Figure 4 TGFβ impairs CD8+ T cell glycolytic metabolism OT-I TCR transgenic T cells were stimulated with SIITFEKL (OVA-T4) peptide in the presence or absence of TGFβ for 24 (A, C) or 48 (B, D-F) hours. (A) The heatmap shows log2 fold changes in expression of glycolytic enzyme-encoding genes as determined by RNA-sequencing. Levels of surface expression of glucose transporter (GLUT)1 (B) were determined by flow cytometry. For flow cytometry analyses data shown represent mean fluorescence intensity (MFI) values. Individual data points represent technical replicates (n=3) from 1 of 3 repeated experiments. (C) Lactate secretion was determined by analysis of deproteinated culture supernatants using a luminescent assay. Individual data points represent biological replicates (n=3) from 1 of 4 repeated experiments. (D) Seahorse metabolic analysis determined extracellular acidification rates (ECAR) of OT-I T cells. Dotted vertical lines represent injection times for glucose (Glu), oligomycin (Oligo) and 2-deoxyglucose (2-DG) in the Glycolytic Stress Test™. Levels of Glycolysis (E) were calculated by subtracting background ECAR values from those determined following glucose injection, whilst glycolytic capacity (F) represent ECAR values following oligomycin injection. For Seahorse metabolic analyses, error bars represent SD and individual data points represent technical replicates (n=6) from 1 of 4 repeated experiments. NS – not significant, * p<0.05, *** p<0.001, **** p<0.0001 as assessed by 1-way ANOVA, with Tukey’s multiple comparisons test (B, C) or Student’s t-test (E, F).
Finally, we assessed the impact of TGFβ on T cell mitochondrial metabolism by performing a Mitostress Test using the Seahorse analyser. Analysis of oxygen consumption rates determined (Figure 5A) that both basal respiration (Figure 5B) and spare respiratory capacity (Figure 5C) were comparable in TCR as compared to TCR+TGFβ treated OT-I T cells. Furthermore, flow cytometry analysis of tetramethylrhodamine, methyl ester (TMRM) (Figure 5D) and MitoTracker Green (Figure 5E) fluorescence in OT-I T cells indicated that TGFβ did not impinge upon T cell mitochondrial membrane potential or mitochondrial mass, respectively.
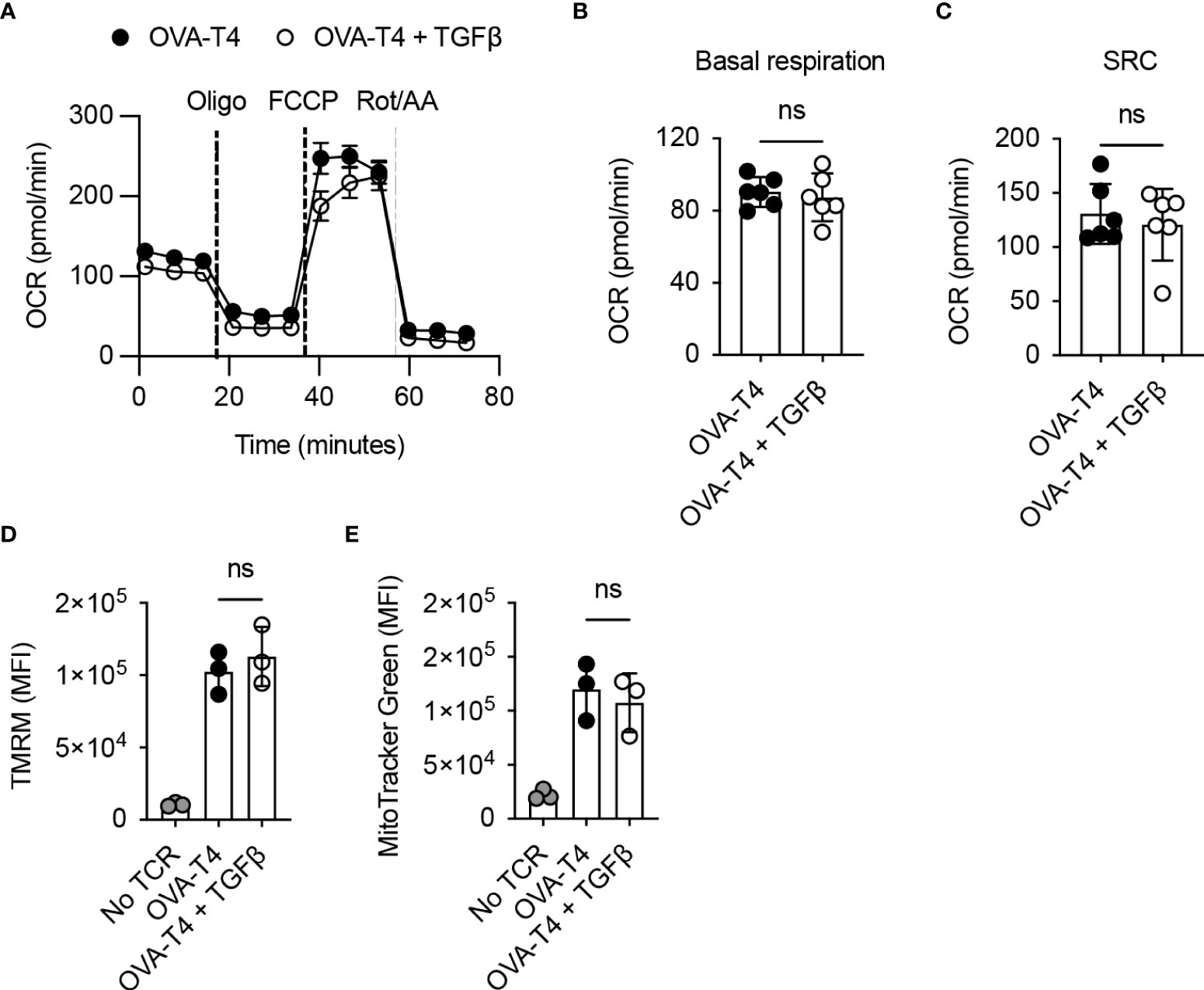
Figure 5 TGFβ inhibits CD8+ T cell activation independently of effects on mitochondrial metabolism OT-I TCR transgenic T cells were stimulated with SIITFEKL (OVA-T4) peptide in the presence or absence of TGFβ for 48 hours. (A) Seahorse metabolic analysis determined oxygen consumption rates (OCR) of OT-I T cells. Dotted vertical lines represent injection times for oligomycin (Oligo), carbonyl cyanide-p-trifluoromethoxyphenylhydrazone (FCCP) and rotenone/antimycin A (Rot/AA) in the MitoStress Test™. Basal respiration (B) and spare respiratory capacity (SRC) (C) were determined as baseline OCR values and OCR values following FCCP injection, respectively. Mitochondrial membrane potential (D) and mitochondrial mass (E) were assessed by flow cytometry analysis of tetramethylrhodamine methyl ester (TMRM) and mitotracker green fluorescence, respectively. For flow cytometry analyses, data shown represent mean fluorescence intensity (MFI) values. Individual data points represent technical replicates (n=3-6) from 1 of 3 repeated experiments. NS – not significant as assessed by Student’s t-test (A–C) or 1-way ANOVA, with Tukey’s multiple comparisons test (D, E).
Discussion
CD8+ T cell activation depends on transcriptional and metabolic reprogramming mediated by Myc (1). Here we have shown that an important mechanism by which TGFβ impedes early T cell activation is through repression of Myc expression upon TCR-priming. Consequently, T cells activated in the presence of TGFβ display deficient engagement of Myc downstream signalling that is reflected in reduced upregulation of glycolytic and AA-dependent metabolism. These metabolic defects are likely to contribute towards the disruption of TCR-induced CD8+ T cell activation, proliferation and effector function.
TGFβ is a master regulator of effector and regulatory T cell activity during autoimmune reactions. Loss of TGFβ signalling in genetically modified murine models leads to aberrant hyperactivation of T cells against self-peptides (27–29). In accordance with our data, these previous studies indicate that TGFβ has a particularly important role in limiting T cell responses upon weak activation of TCR signalling (13, 30). Beyond its implications in autoimmunity here we have shown, in experiments using ID8 ovarian carcinoma cells, that cancer-cell derived TGFβ also restricts T cell activity more efficiently in response to low affinity as compared to strong agonist peptides. In the context of anti-cancer immunity, these results add to a body of work indicating that TGFβ neutralisation could boost responses against tumour associated antigens leading to an increased tumour immunogenicity. A limitation of immune checkpoint blockade therapy is that weakly immunogenic (or “cold”) tumours respond poorly to treatment. Anti-TGFβ therapies might therefore be a promising strategy to convert “cold” tumours into “hot” tumours and improve immune checkpoint blockade when administered in combination.
TGFβ suppresses cytotoxic functions of CD8+ T cells through well-defined direct interactions of SMAD proteins with Gzmb, Ifng and other inflammatory gene loci, which has been described as a mechanism of immune escape during anti-tumour responses and has also been observed in this study (21). In particular, our transcriptomic data shows that the SMAD-target Gzmb is the most downregulated gene in the presence of TGFβ highlighting its powerful inhibition of effector functions. It is important to mention that the regulation of multiple transcription factors and signalling pathways likely contributes to the overall impact of TGFβ on the activated T cell transcriptome. In the current manuscript we have focussed on the suppression of Myc as a key inhibitory mechanism mediated by TGFβ. However, TGFβ also inhibits TCR-induced activation of NFAT signalling (13), modulates gene expression via SMAD activity and regulates the expression of other key transcription factors. For example, we also observed substantial repression of Eomes gene expression. Eomesodermin is involved in transcriptional regulation of CD8+ T cell differentiation, particularly through its cooperation with Tbet to promote memory formation. Whether TGFβ exposure during TCR-priming primarily results in deficient CD8+ T cell activation or whether it shapes T cell fate and differentiation is likely to be highly context-dependent. Indeed, in the context of an inflammatory cytokine milieu, TGFβ has a well-established role in driving the differentiation of inflammatory Tc17 cells, whilst KEGG pathway analysis of our RNA-Seq data confirmed an upregulation of Tc17/Th17-associated genes in TGFβ-treated cells.
Our RNA-seq data has allowed us to gain a broader understanding of how TGFβ shapes the T cell transcriptome and to dissect possible underlying mechanisms by which it suppresses early T cell activation. After performing KEGG and ChEA analysis we found that Myc-regulated genes were strongly enriched in the TGFβ-downregulated gene set. Myc regulates metabolic reprogramming induced upon TCR-priming of naïve cells to promote the exit from quiescence. Specifically, Myc orchestrates the upregulation of genes involved in glucose and AA transport, mainly Slc2a1 and Slc7a5, in addition to metabolic enzymes related to glycolysis and glutaminolysis. Loss of Myc expression in CD8+ T cells prevents TCR-induced blastogenesis, proliferation and differentiation, indicating the essential role of Myc in the initiation of T cell responses (1, 3, 22). Our results confirmed that the marked Myc repression observed in the RNA-Seq data results in lower Myc protein levels as well as an important suppression of its main downstream metabolic pathways. Interestingly, while we observe an impairment of glycolysis, protein synthesis and nutrient transport, no significant differences were shown when mitochondrial metabolism was assessed. These results suggest that the effects of TGFβ on T cell metabolism during early stages of activation are a specific consequence of impaired Myc expression rather than being an indirect effect of an overall reduced T cell activation state. In contrast to the current work suggesting minimal effects of TGFβ on mouse CD8+ T cell mitochondrial metabolism, previous studies interrogating human CD4+ T cells indicated that TGFβ signalling influences respiration via direct effects of SMAD proteins within mitochondria (8). Further work is required to determine if these divergent effects of TGFβ signalling on T cell metabolism are explained by species differences, T cell activation status or intrinsic differences between CD4+ and CD8+ T cells.
An association between TGFβ signalling and Myc has been previously described in keratinocytes, oligodendrocytes, NK and T cells. Indeed, some studies determined that SMAD proteins are able to directly interact with Myc promoter in transformed cells (31). An investigation by Gu et al. showed that Smad4 can also act independently of TGFβ signalling inducing Myc-dependent proliferation in T cells (32). Consequently, Smad4-deficient T cells fail to upregulate Myc expression leading to a deficient expansion. Whether the activation of TGFβ signalling recruits Smad4 and prevents the Smad4-dependent stimulation of Myc expression is still unknown. Myc expression is regulated at both the transcriptional and post-transcriptional level following T cell activation. TCR-induced activation and IL-2 signalling contribute to transcriptional regulation of Myc whilst post-translational modifications impact upon protein stability and turnover. Our data indicate that Myc transcript levels were reduced by TGFβ, however it is possible that reduced uptake of glucose and amino acids as a consequence of reduced nutrient transporter expression may further limit Myc protein levels. In particular, glucose and glutamine-dependent O-GlcNAcylation are important for maintenance of Myc expression in activated T cells (33). Further investigation is required to elucidate the relative contribution of transcriptional and post-transcriptional impacts of TGFβ signalling in the control of Myc expression in CD8+ T cells, which may identify potential mechanisms to prevent the TGFβ-mediated suppression of T cell activation.
A surprising finding in our study has been the mild effect of TGFβ on rpS6 and mTOR phosphorylation considering the important role of AA levels in mTOR regulation (34–36). It is worthy of note that TCR-stimulated naïve T cells are fully competent to induce mTOR activation prior to upregulation of AA transporters. Furthermore, the inhibitory effects of TGFβ on Kyn uptake were less pronounced than that mediated by BCH, suggesting that SLC7A5 activity was not entirely compromised. Our data suggest that whilst TGFβ may limit AA uptake, this is insufficient to compromise rpS6 and mTOR phosphorylation over a short time-span. Previous studies performed in NK cells and in both CD4+ and CD8+ T cells have suggested that, in some circumstances, mTOR activity decreases upon TGFβ treatment (8–11). Interestingly, Zaiatz-Bittencourt and colleagues described that mTOR activity in human NK cells was not affected with short TGFβ treatments and that inhibitory effects could only be observed when treatment was sustained for longer periods (5 days) (10). These results suggest that, in specific contexts, the impact of TGFβ signalling on mTOR activity might not be direct and that the effects may only be detected upon the accumulation of other events that could subsequently impact mTOR function.
In summary, we have described here that TGFβ signalling significantly interferes with the Myc-induced transcriptional signature that occurs during early stages of CD8+ T cell activation, leading to deficient metabolic reprogramming. Therefore, TGFβ signals during CD8+ T cell activation mediate direct inhibition of cytotoxic gene expression by SMAD proteins and metabolic disruption following disruption of Myc expression, both of which contribute to defective CD8+ T cell activation. These inhibitory effects are likely important for the maintenance of self-tolerance in vivo but may also contribute to T cell immunosuppression during anti-cancer immune responses.
Data availability statement
The datasets presented in this study can be found in online repositories. The names of the repository/repositories and accession number(s) can be found below: NCBI GEO, accession numbers: GSE205654, GSM6216059, GSM6216060, GSM6216061, GSM6216062, GSM6216063, GSM6216064, GSM6216065 and GSM6216066.
Ethics statement
The animal study was reviewed and approved by University of Leeds Animal Welfare and Ethical Review Committee.
Author contributions
HH and RS designed the study. HH performed the majority of experiments. HH, GP, PG and RS acquired and analysed the data. NV and GPC supervised parts of the project. HH and RS wrote the manuscript. All authors read and discussed manuscript drafts. All authors contributed to the article and approved the submitted version.
Funding
This work was supported by grant 23269 from Cancer Research UK (to RS) and a University of Leeds PhD scholarship (to HH). NV and HH are supported by grant KFS-4993-02-2020-R from the Swiss Cancer League.
Conflict of interest
The authors declare that the research was conducted in the absence of any commercial or financial relationships that could be construed as a potential conflict of interest.
Publisher’s note
All claims expressed in this article are solely those of the authors and do not necessarily represent those of their affiliated organizations, or those of the publisher, the editors and the reviewers. Any product that may be evaluated in this article, or claim that may be made by its manufacturer, is not guaranteed or endorsed by the publisher.
Supplementary material
The Supplementary Material for this article can be found online at: https://www.frontiersin.org/articles/10.3389/fimmu.2022.913184/full#supplementary-material.
References
1. Wang R, Dillon Christopher P, Shi Lewis Z, Milasta S, Carter R, Finkelstein D, et al. The transcription factor myc controls metabolic reprogramming upon T lymphocyte activation. Immunity (2011) 35(6):871–82. doi: 10.1016/j.immuni.2011.09.021
2. Sinclair LV, Rolf J, Emslie E, Shi Y-B, Taylor PM, Cantrell DA. Control of amino-acid transport by antigen receptors coordinates the metabolic reprogramming essential for T cell differentiation. Nat Immunol (2013) 14(5):500–8. doi: 10.1038/ni.2556
3. Marchingo JM, Sinclair LV, Howden AJ, Cantrell DA. Quantitative analysis of how myc controls T cell proteomes and metabolic pathways during T cell activation. eLife (2020) 9:e53725. doi: 10.7554/eLife.53725
4. Klein Geltink RI, O'Sullivan D, Corrado M, Bremser A, Buck MD, Buescher JM, et al. Mitochondrial priming by Cd28. Cell (2017) 171(2):385–97.e11. doi: 10.1016/j.cell.2017.08.018
5. Cui G, Staron MM, Gray SM, Ho PC, Amezquita RA, Wu J, et al. Il-7-Induced glycerol transport and tag synthesis promotes memory Cd8+ T cell longevity. Cell (2015) 161(4):750–61. doi: 10.1016/j.cell.2015.03.021
6. van der Windt GJW, Everts B, Chang C-H, Curtis JD, Freitas TC, Amiel E, et al. Mitochondrial respiratory capacity is a critical regulator of Cd8+ T cell memory development. Immunity (2012) 36(1):68–78. doi: 10.1016/j.immuni.2011.12.007
7. Hope HC, Salmond RJ. Targeting the tumor microenvironment and T cell metabolism for effective cancer immunotherapy. Eur J Immunol (2019) 49(8):1147–52. doi: 10.1002/eji.201848058
8. Dimeloe S, Gubser P, Loeliger J, Frick C, Develioglu L, Fischer M, et al. Tumor-derived tgf-Βinhibits mitochondrial respiration to suppress ifn-Γ production by human Cd4<Sup>+</Sup> T cells. Sci Signaling (2019) 12(599):eaav3334. doi: 10.1126/scisignal.aav3334
9. Viel S, Marçais A, Guimaraes FS-F, Loftus R, Rabilloud J, Grau M, et al. Tgf-Β inhibits the activation and functions of nk cells by repressing the mtor pathway. Sci Signaling (2016) 9(415):ra19–ra. doi: 10.1126/scisignal.aad1884
10. Zaiatz-Bittencourt V, Finlay DK, Gardiner CM. Canonical tgf-Β signaling pathway represses human nk cell metabolism. J Immunol (2018) 200(12):3934–41. doi: 10.4049/jimmunol.1701461
11. Gabriel SS, Tsui C, Chisanga D, Weber F, Llano-León M, Gubser PM, et al. Transforming growth factor-Β-Regulated mtor activity preserves cellular metabolism to maintain long-term T cell responses in chronic infection. Immunity (2021) 54(8):1698–714.e5. doi: 10.1016/j.immuni.2021.06.007
12. Sinclair LV, Neyens D, Ramsay G, Taylor PM, Cantrell DA. Single cell analysis of kynurenine and system l amino acid transport in T cells. Nat Commun (2018) 9(1):1981. doi: 10.1038/s41467-018-04366-7
13. Brownlie RJ, Garcia C, Ravasz M, Zehn D, Salmond RJ, Zamoyska R. Resistance to tgfbeta suppression and improved anti-tumor responses in Cd8(+) T cells lacking Ptpn22. Nat Commun (2017) 8(1):1343. doi: 10.1038/s41467-017-01427-1
14. Gordinier ME, Zhang HZ, Patenia R, Levy LB, Atkinson EN, Nash MA, et al. Quantitative analysis of transforming growth factor beta 1 and 2 in ovarian carcinoma. Clin Cancer Res (1999) 5(9):2498–505.
15. Wei H, Liu P, Swisher E, Yip YY, Tse JH, Agnew K, et al. Silencing of the tgf-Beta1 gene increases the immunogenicity of cells from human ovarian carcinoma. J Immunother (2012) 35(3):267–75. doi: 10.1097/CJI.0b013e31824d72ee
16. Chen W, Ten Dijke P. Immunoregulation by members of the tgfbeta superfamily. Nat Rev Immunol (2016) 16(12):723–40. doi: 10.1038/nri.2016.112
17. Flavell RA, Sanjabi S, Wrzesinski SH, Licona-Limon P. The polarization of immune cells in the tumour environment by tgfbeta. Nat Rev Immunol (2010) 10(8):554–67. doi: 10.1038/nri2808
18. Lachmann A, Xu H, Krishnan J, Berger SI, Mazloom AR, Ma'ayan A. Chea: Transcription factor regulation inferred from integrating genome-wide chip-X experiments. Bioinformatics (2010) 26(19):2438–44. doi: 10.1093/bioinformatics/btq466
19. Kuleshov MV, Jones MR, Rouillard AD, Fernandez NF, Duan Q, Wang Z, et al. Enrichr: A comprehensive gene set enrichment analysis web server 2016 update. Nucleic Acids Res (2016) 44(W1):W90–7. doi: 10.1093/nar/gkw377
20. Frederick JP, Liberati NT, Waddell DS, Shi Y, Wang XF. Transforming growth factor beta-mediated transcriptional repression of c-myc is dependent on direct binding of Smad3 to a novel repressive smad binding element. Mol Cell Biol (2004) 24(6):2546–59. doi: 10.1128/mcb.24.6.2546-2559.2004
21. Thomas DA, Massague J. Tgf-beta directly targets cytotoxic T cell functions during tumor evasion of immune surveillance. Cancer Cell (2005) 8(5):369–80. doi: 10.1016/j.ccr.2005.10.012
22. Preston GC, Sinclair LV, Kaskar A, Hukelmann JL, Navarro MN, Ferrero I, et al. Single cell tuning of myc expression by antigen receptor signal strength and interleukin-2 in T lymphocytes. EMBO J (2015) 34(15):2008–24. doi: 10.15252/embj.201490252
23. Bose D, Neumann A, Timmermann B, Meinke S, Heyd F. Differential interleukin-2 transcription kinetics render mouse but not human T cells vulnerable to splicing inhibition early after activation. Mol Cell Biol (2019) 39(16). doi: 10.1128/MCB.00035-19
24. Hope HC, Brownlie RJ, Fife CM, Steele L, Lorger M, Salmond RJ. Coordination of asparagine uptake and asparagine synthetase expression modulates Cd8+ T cell activation. JCI Insight (2021) 6(9). doi: 10.1172/jci.insight.137761
25. Viel S, Marcais A, Guimaraes FS, Loftus R, Rabilloud J, Grau M, et al. Tgf-beta inhibits the activation and functions of nk cells by repressing the mtor pathway. Sci Signal (2016) 9(415):ra19. doi: 10.1126/scisignal.aad1884
26. Salmond RJ, Emery J, Okkenhaug K, Zamoyska R. Mapk, phosphatidylinositol 3-kinase, and mammalian target of rapamycin pathways converge at the level of ribosomal protein S6 phosphorylation to control metabolic signaling in Cd8 T cells. J Immunol (2009) 183(11):7388. doi: 10.4049/jimmunol.0902294
27. Gorelik L, Flavell RA. Abrogation of tgfbeta signaling in T cells leads to spontaneous T cell differentiation and autoimmune disease. Immunity (2000) 12(2):171–81. doi: 10.1016/s1074-7613(00)80170-3
28. Lucas PJ, Kim SJ, Melby SJ, Gress RE. Disruption of T cell homeostasis in mice expressing a T cell-specific dominant negative transforming growth factor beta ii receptor. J Exp Med (2000) 191(7):1187–96. doi: 10.1084/jem.191.7.1187
29. Marie JC, Liggitt D, Rudensky AY. Cellular mechanisms of fatal early-onset autoimmunity in mice with the T cell-specific targeting of transforming growth factor-beta receptor. Immunity (2006) 25(3):441–54. doi: 10.1016/j.immuni.2006.07.012
30. Arumugam V, Bluemn T, Wesley E, Schmidt AM, Kambayashi T, Malarkannan S, et al. Tcr signaling intensity controls Cd8+ T cell responsiveness to tgf-Β. J Leukoc Biol (2015) 98(5):703–12. doi: 10.1189/jlb.2HIMA1214-578R
31. Chen CR, Kang Y, Siegel PM, Massagué J. E2f4/5 and P107 as smad cofactors linking the tgfbeta receptor to c-myc repression. Cell (2002) 110(1):19–32. doi: 10.1016/s0092-8674(02)00801-2
32. Gu AD, Zhang S, Wang Y, Xiong H, Curtis TA, Wan YY. A critical role for transcription factor Smad4 in T cell function that is independent of transforming growth factor Β receptor signaling. Immunity (2015) 42(1):68–79. doi: 10.1016/j.immuni.2014.12.019
33. Swamy M, Pathak S, Grzes KM, Damerow S, Sinclair LV, van Aalten DMF, et al. Glucose and glutamine fuel protein O-glcnacylation to control T cell self-renewal and malignancy. Nat Immunol (2016) 17(6):712–20. doi: 10.1038/ni.3439
34. Chapman NM, Chi H. Mtor links environmental signals to T cell fate decisions. Front Immunol (2015) 5:686. doi: 10.3389/fimmu.2014.00686
35. Park Y, Reyna-Neyra A, Philippe L, Thoreen CC. Mtorc1 balances cellular amino acid supply with demand for protein synthesis through post-transcriptional control of Atf4. Cell Rep (2017) 19(6):1083–90. doi: 10.1016/j.celrep.2017.04.042
Keywords: T cells, T cell receptor, metabolism, cytokines, signalling, TGFβ (transforming growth factor-beta)
Citation: Hope HC, Pickersgill G, Ginefra P, Vannini N, Cook GP and Salmond RJ (2022) TGFβ limits Myc-dependent TCR-induced metabolic reprogramming in CD8+ T cells. Front. Immunol. 13:913184. doi: 10.3389/fimmu.2022.913184
Received: 05 April 2022; Accepted: 06 July 2022;
Published: 26 July 2022.
Edited by:
Klaus Okkenhaug, University of Cambridge, United KingdomReviewed by:
David K. Finlay, Trinity College Dublin, IrelandAntoine Marçais, UMR5308 Centre International de Recherche en Infectiologie (CIRI), France
Copyright © 2022 Hope, Pickersgill, Ginefra, Vannini, Cook and Salmond. This is an open-access article distributed under the terms of the Creative Commons Attribution License (CC BY). The use, distribution or reproduction in other forums is permitted, provided the original author(s) and the copyright owner(s) are credited and that the original publication in this journal is cited, in accordance with accepted academic practice. No use, distribution or reproduction is permitted which does not comply with these terms.
*Correspondence: Helen Carrasco Hope, aGVsZW4uY2FycmFzY29ob3BlQHVuaWwuY2g=; Robert J. Salmond, ci5qLnNhbG1vbmRAbGVlZHMuYWMudWs=