- 1Department of Clinical Veterinary Medicine, College of Veterinary Medicine, China Agricultural University, Beijing, China
- 2Department of Production Animal Health, Faculty of Veterinary Medicine, University of Calgary, Calgary, AB, Canada
- 3College of Life Science, Ningxia University, Yinchuan, China
Prototheca bovis, a highly contagious pathogen, causes bovine mastitis, resulting in premature culling of affected cows and severe economic losses. Infection with P. bovis caused oxidative stress and apoptosis in bovine mammary epithelial cells (bMECs); however, mechanisms underlying P. bovis-induced autophagy remain unclear. Therefore, the autophagy flux induced by P. bovis in bMECs was analyzed by Western blot and laser scanning confocal microscopy. Expression levels of proteins in the HIF-1α and AMPKα/ULK1 pathway, including HIF-1α, AMPKα, p-AMPKα, ULK1, p-ULK1, mTOR, and p-mTOR, plus expression of autophagy-related genes including SQSTM1/p62, Atg5, Beclin1, and LC3II/LC3I, were quantified with Western blot. Infection with P. bovis induced autophagosomes and LC3 puncta in bMECs that were detected using transmission electron microscopy and laser scanning confocal microscopy, respectively. In addition, lysosome-associated proteins Rab7 and LAMP2a, and lysosomal activity were measured with Western blot and laser scanning confocal microscopy. Infection with P. bovis induced an unobstructed autophagic flux, increased protein expression of LC3II/LC3I, and decreased SQSTM1/p62 protein expression at 6 hpi. Furthermore, P. bovis upregulated protein expression in the HIF-1α and AMPKα/ULK1 pathway and increased the ratio of LC3II/LC3I, implying autophagy was activated in bMECs. However, deletion of AMPKα or ULK1 decreased LC3II/LC3I expression levels and LC3 puncta numbers, suggesting that autophagy was inhibited in bMECs. Additionally, deficiency of HIF-1α decreased protein expression of AMPKα and ULK1 as well as LC3 puncta numbers, and autophagy induced by P. bovis was also inhibited in bMECs. At 6 hpi, lysosome-associated protein Rab7 was decreased and LAMP2a was increased, indicating normal autophagy. In contrast, at 12 hpi, expression of Rab7 and LAMP2a proteins indicated that autophagy was inhibited in bMECs at that time. Therefore, we confirmed that P. bovis infection induced autophagy in bMECs via the HIF-1α and AMPKα/ULK1 pathway, with involvement of lysosome-associated protein Rab7 and LAMP2a.
Introduction
Bovine mastitis is inflammation of bovine mammary parenchyma, most commonly caused by an infection with various pathogens. Prototheca bovis (P. bovis), an obligate heterotroph lacking chlorophyll, is a unicellular achlorophyllous algae, 3-30 µm in diameter, that lacks a specific glucosamine cell wall, and is a highly contagious pathogen causing bovine mastitis (1). Prototheca mastitis increases somatic cell counts and decreases milk production and milk quality, causing substantial economic losses (2, 3). Infection of bovine mammary epithelial cells (bMECs) by P. bovis damages cellular and subcellular organelles (4). Autophagy is an integral part of resistance to various pathogenic infections, clearing pathogens and damaged organelles (5). Infection with P. bovis caused oxidative stress and apoptosis in bMECs (6, 7); however, mechanisms underlying P. bovis-induced autophagy remains unclear.
Autophagy is a highly conserved lysosome-mediated degradation mechanism in eukaryotic cells, mainly responsible for removal of misfolded proteins, damaged organelles, etc., with an important role in maintaining cellular homeostasis (8). Autophagy can be divided into macroautophagy, microautophagy, and molecular chaperone-mediated autophagy, based on targeted transport of substrates to lysosomes (9). Macroautophagy, the best-characterized form of autophagy, involves a specialized double-membrane vesicle known as the autophagosome (10); furthermore, it is a conserved degradative pathway that host cells use to remove invading pathogens (11). During autophagy, microtubule-associated protein 1A/1B-light chain 3 (LC3-I) is lipidated to form LC3-II, an indicator of autophagic activity and flux (12). The protein SQSTM1/p62 binds to ubiquitinated substrate cargo and targets them for degradation in the autophagy-lysosome system by interacting with LC3 (13).
The unc-51 like autophagy activating kinase 1 (ULK1), a signal that initiates autophagy, has a key role in recruitment of autophagy-related genes and proteins to phagocytic vesicle assembly sites (14). ULK1 is the key mediator of mTORC1 signaling to autophagy. In the presence of amino acids, mTORC1 is active and inhibits autophagy by phosphorylating ULK1 and ATG13. However, when nutrients are deficient, mTORC1 activity on the surface of lysosomes is inhibited, and ULK1 and ATG13 are rapidly dephosphorylated, leading to activation of ULK1 kinase and induction of autophagy (15). Additionally, AMP activated protein kinase (AMPK), a key energy sensor, can also activate ULK1 activity by phosphorylating ULK1 during nutrient energy deprivation, thereby further promoting autophagy (16). High mTOR activity prevents ULK1 activation by phosphorylating ULK1 and disrupting interactions between ULK1 and AMPK (17). Hypoxia-inducible factor-1α (HIF-1α) is upregulated by AMPK activation and mtROS and is required for expression of anti-inflammatory genes and induction of autophagy (18, 19). In addition to hypoxia, HIF-1α can also be activated in nonhypoxic conditions in many cell types (19). Activation of HIF-1α also involved in autophagy. Deficiency of HIF-1α enhances influenza A virus replication by promoting autophagy in alveolar type II epithelial cells (20). Additionally, lysosomal-associated membrane protein 2 (LAMP2) and ras associated protein 7 (Rab7, a member of the small GTPase family), have important roles in promoting integrity of the lysosome membrane and in regulating fusion of autophagy vesicles and lysosomes (21).
Autophagy is closely related to resistance to various pathogenic infections and clearance of pathogens and damaged intracellular organelles (5, 22); however, mechanisms underlying autophagy in bMECs caused by P. bovis infection remain unclear. Therefore, in this study, we established a model of autophagy induced by P. bovis infection of bMECs and demonstrated that P. bovis infection induced autophagy via the HIF-1α and AMPKα/ULK1 pathway, with involvement of lysosome-associated protein Rab7 and LAMP2a in bMECs.
Materials and methods
Prototheca bovis strains
Isolates of Prototheca bovis recovered from clinical mastitis milk samples on Chinese dairy farms were stored at 4°C in the College of Veterinary Medicine, China Agricultural University, Beijing, China (7). These isolates were multiplied by streaking on sabouraud dextrose agar (SDA) and incubating the plate at 37°C for 48 h. Then, a single colony was placed in sabouraud dextrose broth (SDB) and incubated for 72 h. Thereafter, organisms were diluted in DMEM to pre-defined concentrations.
Cell culture and infection
The MAC-T cells (Shanghai Jingma Biological Technology Co., Ltd. China) was cultured for use in various experiments. The bMECs were placed in DMEM medium supplemented with 10% fetal bovine serum, penicillin (100 U/mL) and streptomycin (100 U/mL), and grown in cell culture plates in 5% CO2 at 37°C. Cells from passages 2-8 were used for experiments. Before infection, cells were seeded in 6-well plates (1×105 cells per well) and cultured for 24 h. Then, we changed to a new medium (without penicillin or streptomycin), the bMECs were infected with P. bovis at a 5:1 multiplicity of infection (MOI; ratio of P. bovis to bMECs) and incubated in 5% CO2 at 37°C for 6 h. Thereafter, samples were collected and proteins extracted and used for Western blots. The experiment was repeated 3 times to ensure reproducibility.
Cell transfection and confocal microscope observation
The bMECs were seeded in 6-well plates and grown to 50% confluence. Ad-mCherry-GFP-LC3B (Beyotime, #C301) was used to transfect bMECs at a 20:1 MOI for 12 h in DMEM containing 10% FBS, following manufacturer’s instructions. Then, bMECs were infected with P. bovis at a 5:1 MOI for 0, 2, 4, 6, 8, 10, or 12 h. Additionally, rapamycin (20 µM; MCE, #AY-22989) and 3-Methyladenine (5 mM; MCE, #HY-19312) were used to treat cells for 6 h as an inducer and inhibitor of autophagy, respectively. Cell nuclei were stained with Hoechst 33342 (Beyotime, #C1025), washed 3 times with PBS, and observed with laser scanning confocal microscopy (Nikon, A1 LFOV) at laser wavelengths of 405, 488 and 561 nm. In addition, Ad-GFP-LC3B (Beyotime, #C3006) was also used to transfect bMECs at a 20:1 MOI for 12 h in DMEM containing 10% FBS. The bMECs were infected with P. bovis at a 5:1 MOI for 6 h. Cell nuclei were stained with Hoechst 33342, and after 3 PBS washes, cells were examined under a laser scanning confocal microscope (Nikon, A1 LFOV) at laser wavelengths of 405 and 488 nm.
Transmission electron microscopy
The bMECs (1×105 cells per well) were seeded in 6-well plates, grown to 80% confluence and infected with P. bovis at 5:1 MOI or treated with rapamycin (20 µM) and 3-Methyladenine (5 mM) for 6 h. For transmission electron microscopy, samples were prepared according to our previous method (23). Cells were washed 3 times with PBS, fixed with 2.5% glutaraldehyde for 4 h at room temperature, then post-fixed in 0.5% osmium tetroxide for 2 h. Samples were dehydrated in an ethanol gradient, followed by acetone (15 min in each solution). Thereafter, samples were embedded in resin and thin slices (100 nm) were cut with a glass knife. Sections were put on copper grids, stained with 2% uranyl acetate and lead citrate, and examined with a transmission electron microscope (H7650, Tokyo, Japan) at an accelerating voltage of 80 kV.
siRNA transfection
The bMECs were seeded into 6-well plates; when the cells had achieved 40-50% confluence, they were transiently transfected with siRNA by Lipo8000™ transfection reagent (Beyotime, #C0533), according to the manufacturer’s instructions. For cells in 1 well of the 6-well plate to be transfected, 100 pmol siRNA was added to 125 µL DMEM medium without antibiotics or serum, and mixed by pipetting gently; then 4 µL Lipo8000™ Transfection Reagent was added, mixed by gentle pipetting, and incubated at room temperature for 20 min. After transfection for 6 h, fresh medium was added into wells and transfected cells were cultured for another 24 h. The efficiency of siRNA transfection was determined with Western blot. The following siRNAs were used: HIF-1α siRNA, sense (5’-3’) GGAUAUGUCUGGAUAGAAATT, antisense (5’-3’) UUUCUAUCCAGACAUAUCCTT; ULK1 siRNA, sense (5’-3’) GCUUCAGCGCAACCACAAATT, antisense (5’-3’) UUUGUGGUUGCGCUGAAGCTT; AMPKα siRNA, sense (5’-3’) GCUGGUCCAGAGGUAGAUATT, antisense (5’-3’) UAUCUACCUCUGGACCAGCTT; NCsiRNA, sense (5’-3’) UUCUCCGAACGUGUCACGUTT, antisense (5’-3’) ACGUGACACGUUCGGAGAATT. All siRNAs used were synthesized by Sangon Biotech (Shanghai) Co., Ltd.
Western blot
The bMECs were treated as described above, lysed on ice for 5 min, and the cell lysate suspensions were collected and centrifuged at 12000×g for 15 min at 4°C. Total protein concentration in the supernatant was determined with a BCA protein assay kit, according to the manufacturer’s instructions (Beyotime, #P0012S). Protein samples were mixed with SDS-PAGE protein loading buffer (5×), denatured in boiling water for 10 min, then separated by SDS-PAGE and transferred onto polyvinylidene difluoride membranes. These membranes were blocked with 5% nonfat dry milk for 2 h at room temperature, then incubated overnight at 4°C with the following primary antibodies: β-actin (Abcam, #4970, 1:1000), HIF-1α (Abcam, #ab179483, 1:1000), ULK1 (Proteintech, #20986-1-AP, 1:1000), phospho-ULK1 (Ser 757: CST, #14202, 1:1000; Ser 556: Affinity Biosciences, #DF7587, 1:1000; Ser 317: Affinity Biosciences, #AF2301, 1:1000), AMPKα (CST, #5831, 1:1000), phospho-AMPKα (CST, #2535, 1:1000), mTOR (Proteintech, #28273-1-AP, 1:1000), phospho-mTOR (Proteintech, #67778-1-Ig, 1:1000), Atg5 (Abcam, #ab228668, 1:1000), LC3 (Proteintech, #14600-1-AP, 1:1000), Beclin1 (Abcam, #ab231341, 1:1000), SQSTM1/p62 (Proteintech, #118420-1-AP, 1:1000), LAMP2a (Proteintech, #66301-1-Ig, 1:1000), and Rab7 (Abcam, #ab229647, 1:1000). Thereafter, they were incubated with secondary antibody HRP-conjugated affinipure goat anti-rabbit IgG (H+L) (Proteintech, #SA00001-2, 1:5000) for 1 h at room temperature. After washing with Tris-buffered saline, the membrane was developed using ECL reagents and visualized with a chemiluminescence system. Results were normalized to β-actin, and band density assessed with Image J software (Version 1.8.0, National Institutes of Health, Bethesda, MD, USA).
Immunofluorescence
After infecting bMECs with P. bovis for 6 h in 6-well plates, bMECs were washed 3 times with PBS, fixed in 4% paraformaldehyde for 4 h, and subsequently permeabilized in 0.25% Triton X-100 (Beyotime, #ST797) for 15 min. Cells were incubated with 3% bovine serum for 30 min at room temperature and then incubated at 4°C overnight with primary antibody ULK1 (Proteintech, #20986-1-AP, 1:500). Next, cell samples were washed 3 times with PBS and incubated with Alexa Fluor 488-labeled goat anti-rabbit IgG (H+L) (CST, #8878, 1:500) for 1 h at room temperature, then washed 3 times with PBS and stained with DAPI (Beyotime, #C1002) for 5 min. After washing with PBS, slides were covered with glass cover slips and ULK1, examined with a laser scanning confocal microscope (Nikon, A1 LFOV) at laser wavelengths of 405 and 488 nm, and images captured were analyzed with Image J software (Version 1.8.0).
Lyso−tracker red staining
Lyso-Tracker Red, a lysosome red fluorescent probe that penetrates cell membranes, is used to detect acidification of lysosomes or mature autophagosomes in living cells. Ad-GFP-LC3B (Beyotime, #C3006) was used to transfect bMECs at a 20:1 MOI for 12 h in DMEM containing 10% FBS. After infecting bMECs with P. bovis for 6 h, cell culture medium was removed, cells were washed 3 times, and then incubated with Lyso-Tracker Red (50 nM) (Beyotime, #C1046) at 37°C for 30 min. The Lyso-Tracker Red staining solution was removed, and fresh cell culture solution was added, followed by examination with a laser scanning confocal microscope (Nikon, A1 LFOV) at laser wavelengths of 405, 488 and 561 nm.
Statistical analyses
All data were analyzed by Student’s t-test or one-way ANOVA with Bonferroni correction for multiple comparisons. For all analyses, P < 0.05 was considered significant in a 2-tailed statistical test. Data were reported as mean ± standard deviation (SD) of 3 independent experiments.
Results
Autophagy in bMECs induced by P. bovis infection
Quantification of changes in LC3-II and SQSTM1/p62 are widely used to monitor autophagy. To investigate the autophagy induced by P. bovis infection in bMECs, protein expression levels of ULK1, SQSTM1/p62 and LC3II/LC3I were assessed at various time points. Expression of ULK1 was increased after P. bovis infection in bMECs (Figure 1A). Infection with P. bovis decreased protein expression level of SQSTM1/p62 compared to level of protein in uninfected groups at 2, 4 and 6 hpi (Figure 1B). Protein expression level of SQSTM1/p62 had an upward trend at 8, 10, and 12 hpi, albeit without a significant difference (Figure 1B). Meanwhile, protein expression of LC3II/LC3I significantly increased compared to the uninfected group at 2, 4 and 6 hpi, suggesting autophagy had occurred during P. bovis infection in bMECs; however, it subsequently decreased at 8 and 10 hpi (Figure 1C, Figure 1SA), with no significant difference between expression level of protein in LC3II/LC3I and the uninfected group at 12 hpi (Figure 1C, Figure 1SA), implying that autophagy was inhibited or blocked. Adenovirus expressing mCherry-GFP-LC3B fusion protein is an adenovirus expressing mCherry-GFP-LC3B (Ad-mCherry-GFP-LC3B) fusion protein that can be used to detect autophagy after infecting cells. bMECs were continuously infected with P. bovis for 12 h to examine the dynamics of autophagy. Autophagolysosome fluorescence tended to increase within 6 h, along with duration of infection, whereas red mottled fluorescence increased, peaking at 12 h (Figure 1D), implying that autophagy was unobstructed for 6 hpi, but was subsequently inhibited or blocked in bMECs infected with P. bovis.
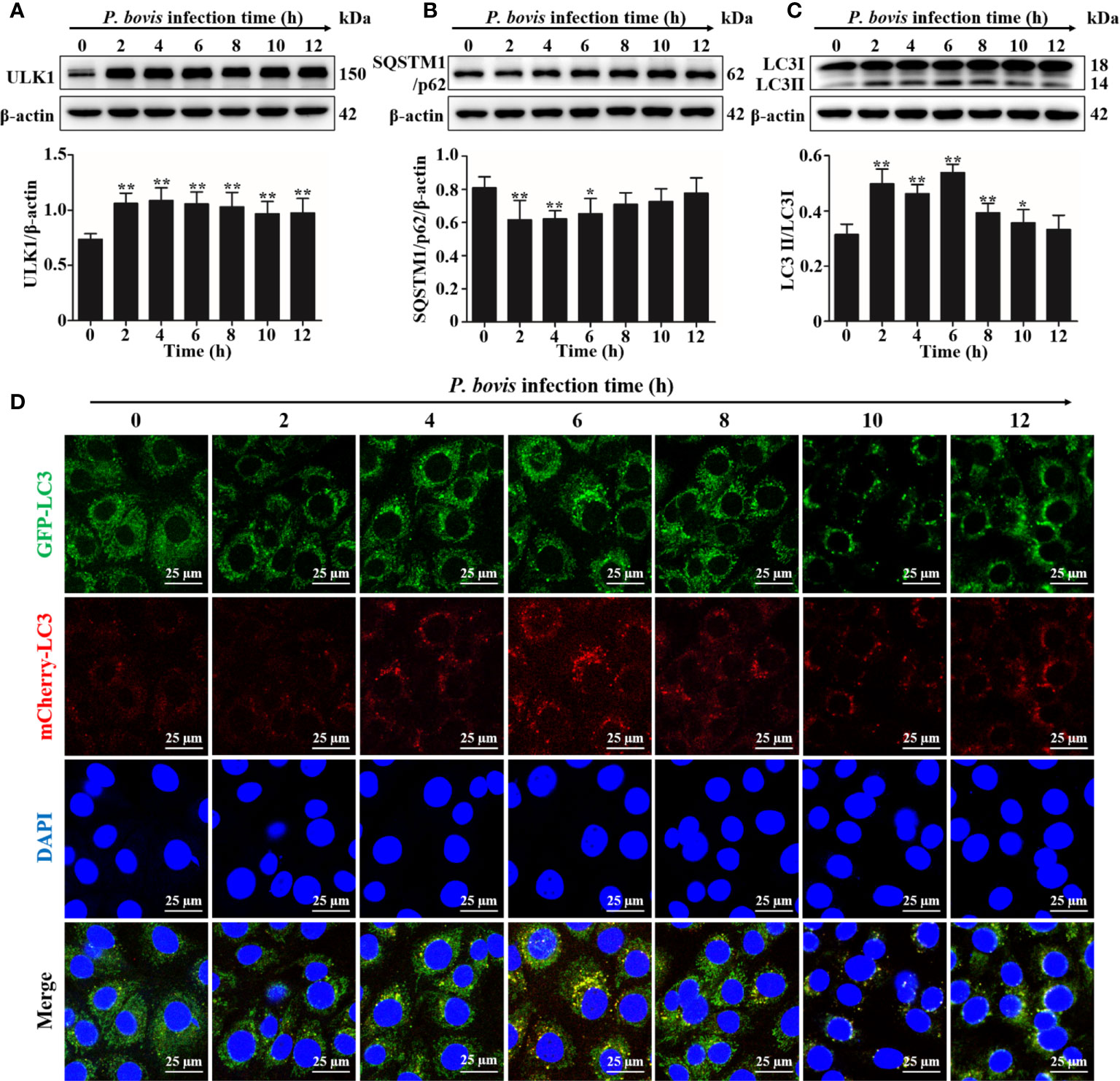
Figure 1 P. bovis infection induced autophagy in bMECs. (A) Protein expression of LC3II/LC3I and SQSTM1/p62 in bMECs continuously infected with P. bovis for 12 h (B) and (C) Quantification of LC3II/LC3I and SQSTM1/p62 proteins by ImageJ software. (D) Autophagic flux in bMECs. Cells were transfected with Ad-mCherry-GFP-LC3 (MOI=20:1) for 12 h, and then infected with P. bovis for various intervals (0, 2, 4, 6, 8, 10, and 12 h) to monitor the autophagic flux with laser scanning confocal microscopy. Data represent means ± SD of 3 independent experiments, compared to the control group (*P < 0.05 and **P < 0.01).
P. bovis infection activated autophagy by modulating the AMPKα/ULK1 pathway in bMECs
The AMPKα/ULK1 pathway is involved in regulation of autophagy. To measure activated autophagy in bMECs infected with P. bovis, protein expression levels in AMPKα/ULK1 were measured 6 h after P. bovis infection. Protein expression levels of HIF-1α, p-AMPKα, p-mTOR and p-ULK1(ser 757, 317 and 556) were increased after P. bovis infection (Figures 2A, B, Figure 2S), with no significant difference in protein expression between the mTOR and the control group. Elevated phosphorylation protein levels implied that the AMPKα/ULK1 pathway was activated by P. bovis. Furthermore, protein expression of autophagy-related genes was also examined, and protein expression level of SQSTM1/p62 was decreased, whereas expression of Atg5 and LC3II/LC3I was increased in bMECs after P. bovis infection (Figures 2A, B, Figure 1SB). Rapamycin increased protein expression of p-AMPKα, p-ULK1(ser 757, 317 and 556), Atg5, and LC3II/LC3I, but decreased p-mTOR and SQSTM1/p62 protein expression levels in bMECs compared to the control group (Figures 2A, B, Figure 1SB and Figure 2S). Pretreatment with 3MA decreased protein expression levels of AMPKα, p-AMPKα, p-mTOR, ULK1, p-ULK1(ser 757, 317 and 556) and LC3II/LC3I, but increased SQSTM1/p62 protein expression in bMECs compared to the P. bovis infection group (Figures 2A, B, Figure 1SB and Figure 2S). The activated AMPKα/ULK1 pathway was involved in P. bovis-induced autophagy in bMECs.
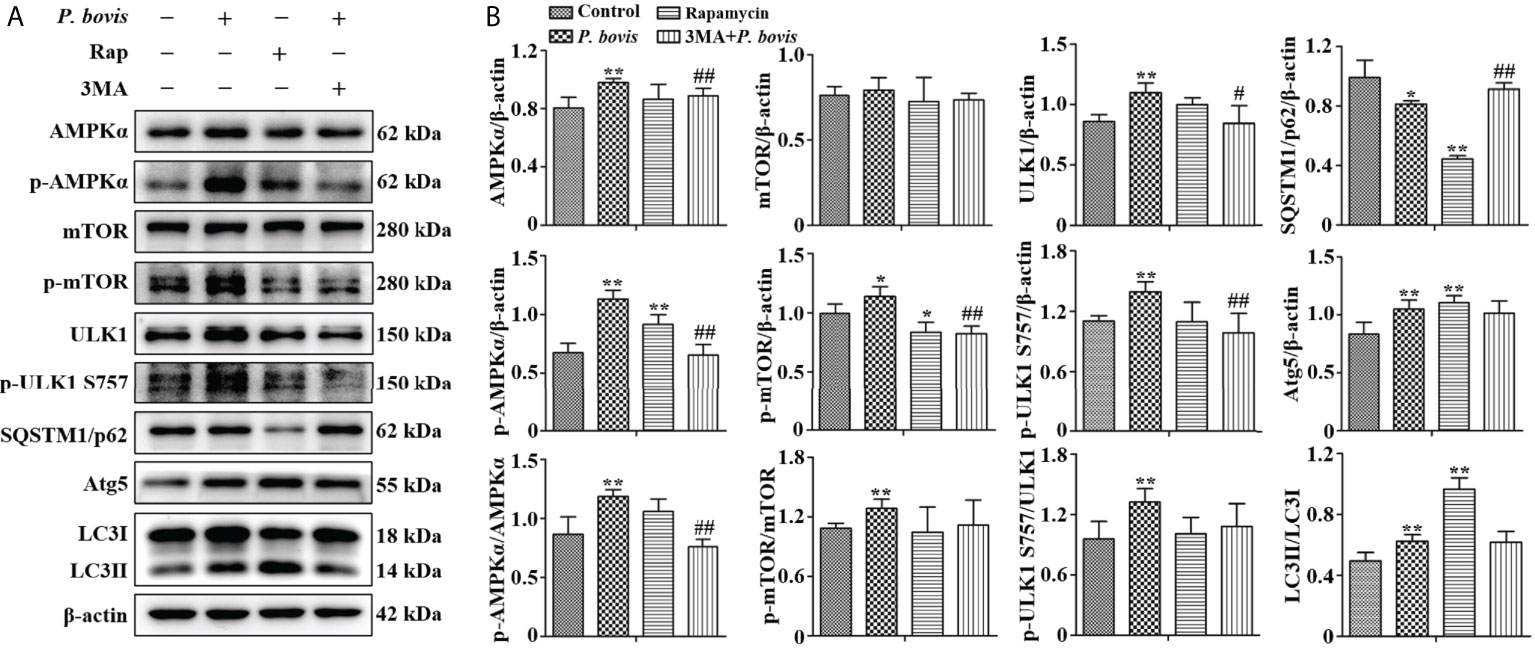
Figure 2 P. bovis infection induced autophagy via activated AMPKα/ULK1 signaling in bMECs. (A) and (B) Western blot analyses of AMPKα, p-AMPKα, p-mTOR, ULK1, p-ULK1, SQSTM1/p62, Atg5 and LC3II/LC3I in bMECs. The right panel is protein quantification with ImageJ software. The bMECs were infected with P. bovis and treated with rapamycin (20 µM) for 6 hAdditionally, bMECs were pretreated with 3MA (5 mM) for 2 h, and then infected with P. bovis for 6 h Data represent means ± SD of 3 independent experiments, compared to the control group, *P < 0.05 and **P < 0.01; compared to the P. bovis group, #P < 0.05 and ##P < 0.01.
P. bovis infection induced autophagosome formation in bMECs
Induction of autophagy is formation of an autophagosome. Based on cells transfected with Ad-mCherry-GFP-LC3, P. bovis infection induced autophagosome formation in bMECs, as indicated by green and yellow puncta in the P. bovis infection and rapamycin treatment group (Figure 3A). Meanwhile, autophagosomes were also observed in the P. bovis infection and rapamycin treatment group (Figure 3B). Pretreatment with 3MA inhibited autophagosome formation in P. bovis-infected bMECs, although some autophagosomes were observed in these bMECs (Figures 3A, B). Therefore, P. bovis infection induced autophagosome formation in bMECs.
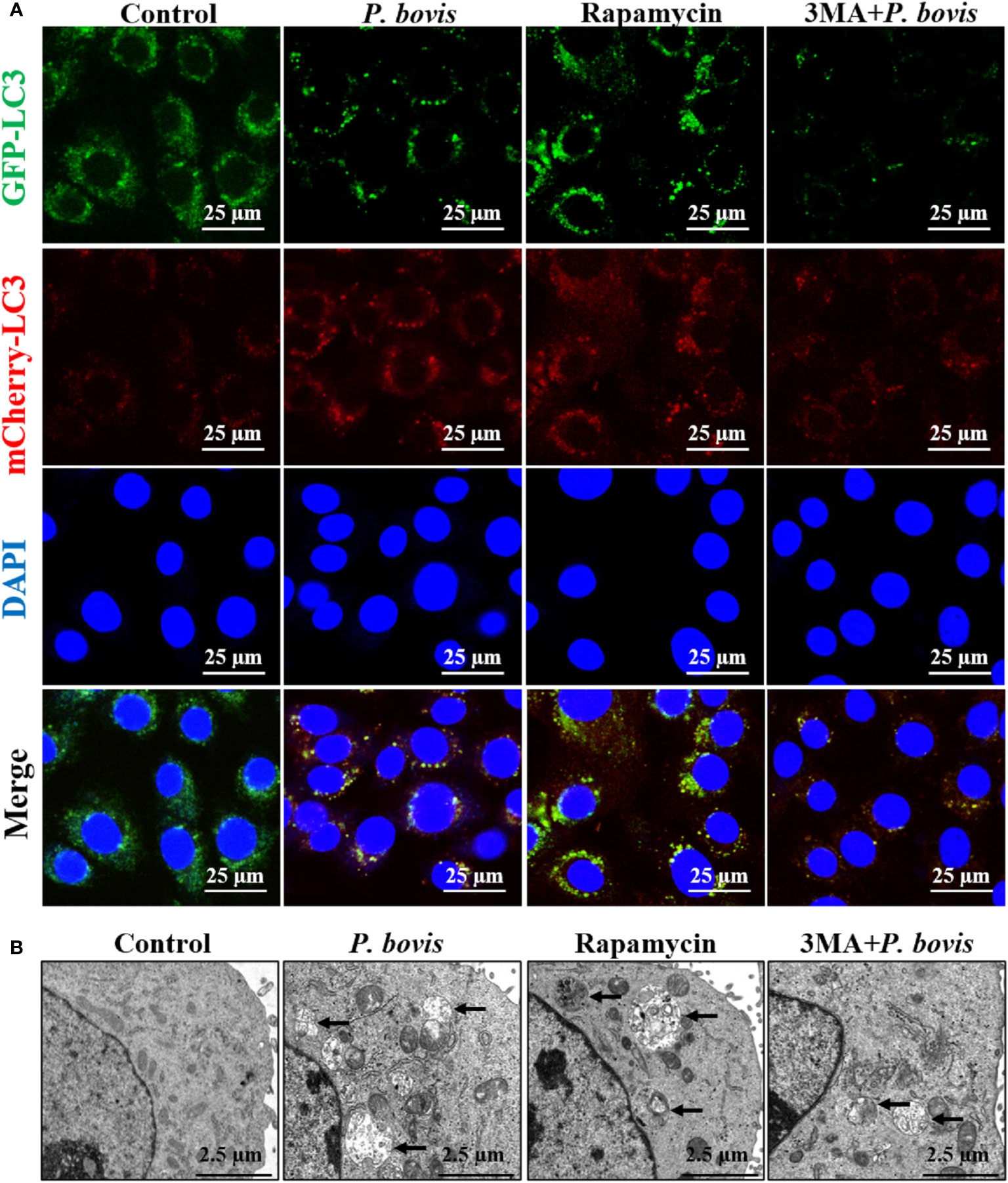
Figure 3 P. bovis infection induced autophagosome formation in bMECs. (A) Measurement of autophagosomes in bMECs. Cells were infected with P. bovis and treated with rapamycin (20 µM) or 3MA (5 mM) for 6 h (B) Ultrastructural observation after bMECs infection with P. bovis, treatment with rapamycin or 3MA for 6 h Black arrows indicated autophagosomes.
AMPKα deletion reduced ULK1 phosphorylation and also inhibited autophagy in bMECs infected with P. bovis
AMPKα has an important role in regulation of autophagy. To verify the role of AMPKα in P. bovis-induced autophagy in bMECs, we silenced AMPKα in cells. Infection with P. bovis increased protein expression levels of AMPKα, p-AMPKα, ULK1, p-ULK1 and LC3II/LC3I, but decreased SQSTM1/p62 protein expression in bMECs compared to the control group (Figures 4A, B, Figure 1SC), suggesting that autophagy induced by P. bovis was activated in bMECs. However, silencing AMPKα decreased p-AMPKα protein expression; furthermore, protein expression levels of HIF-1α, ULK1, p-ULK1 (ser 757, 317 and 556), SQSTM1/p62, and LC3II/LC3I were also decreased during P. bovis infection in bMECs (Figures 4A, B, Figure 1SC, Figure 3S). Therefore, we inferred that deletion of AMPKα inhibited autophagy induced by P. bovis. Additionally, deletion of AMPKα did not affect protein expression of HIF-1α during P. bovis infection (Figure 3S), and addition of non-target siRNA did not affect protein expression in P. bovis-infected bMECs (Figure 4S). Meanwhile, P. bovis infection increased the number of LC3 puncta (average of ~12.5 puncta per cell), deletion of AMPKα also reduced the number of LC3 puncta (average of ~4.5 puncta per cell) in P. bovis-infected bMECs (Figures 4C, D), and the fluorescence intensity of total GFP-LC3B was also decreased in bMECs (Figure 5SA). Deletion of AMPKα reduced ULK1 phosphorylation and inhibited P. bovis infection induced autophagy in bMECs, but did not affect protein expression of HIF-1α in bMECs.
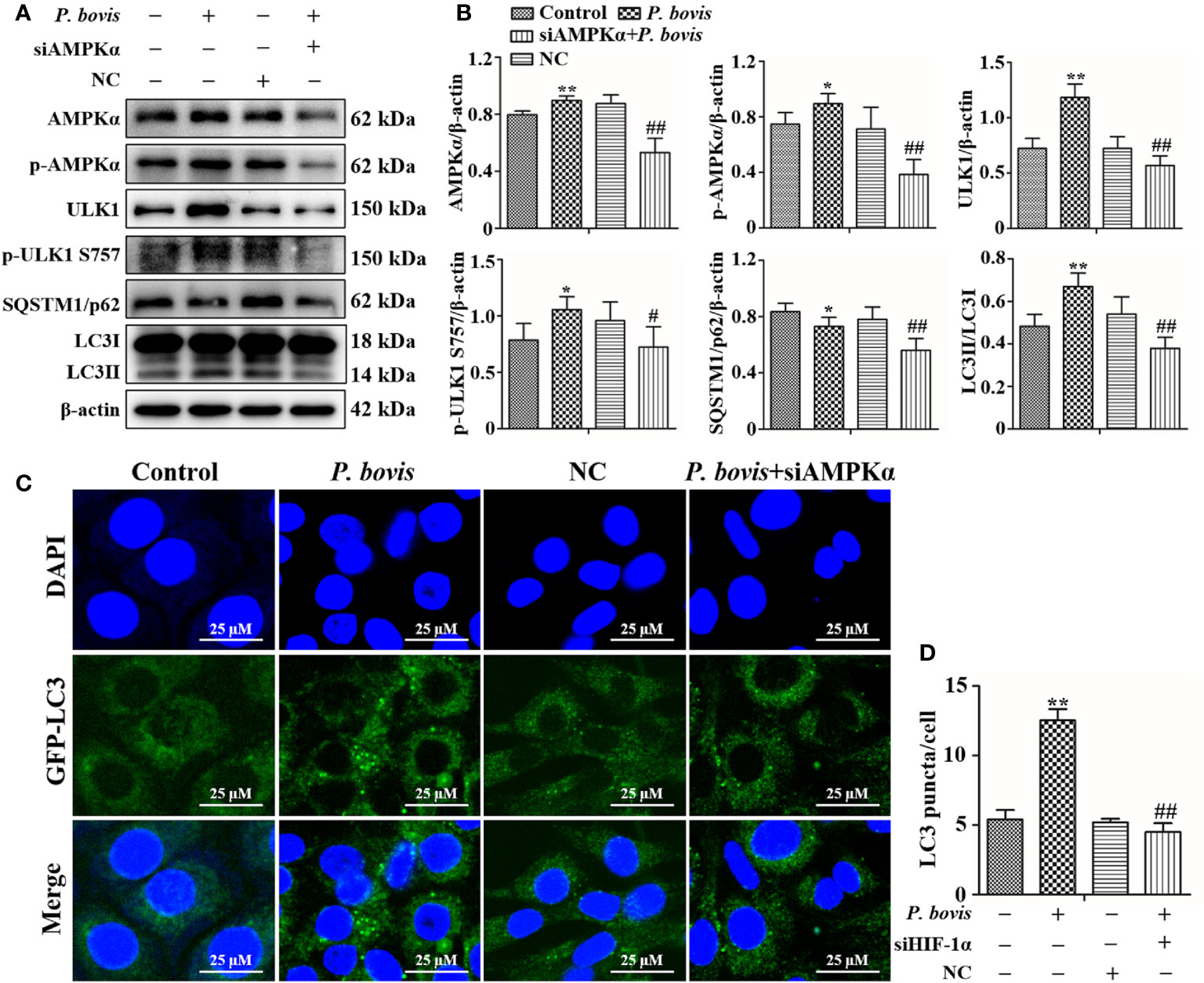
Figure 4 Silencing AMPKα reduced P. bovis-induced autophagy in bMECs. (A) Western blot analysis of AMPKα, p-AMPKα, ULK1, p-ULK1, SQSTM1/p62 and LC3II/LC3I in bMECs. The right panel indicates protein quantification (ImageJ software). (B) Autophagosome measurement in P. bovis- infected bMECs. First, Ad-GFP-LC3B was used to transfect bMECs at a 20:1 multiplicity of infection for 12 h in DMEM containing 10% FBS; thereafter, bMECs was infected with P. bovis for 6 h NC indicated non-target siRNA control. Data represent means ± SD of 3 independent experiments, compared to the control group, *P < 0.05 and **P < 0.01; compared to the P. bovis group, #P < 0.05 and ##P < 0.01.
Deletion of ULK1 reduced P. bovis infection induced autophagy in bMECs
ULK1 is a key gene for autophagy initiation; therefore, we examined effects of ULK1 deletion on the induction of autophagy in bMECs by P. bovis. Protein expression of ULK1 was significantly decreased in bMECs after silencing ULK1 (Figure 5A). Furthermore, the green fluorescence of intensity of ULK1 was also decreased compared to the control group (Figure 5B). These results suggested that the ULK1 gene was silenced. P. bovis infection increased protein expression levels of ULK1, p-ULK1(ser 757, 317 and 556), Atg5 and LC3II/LC3I in bMECs compared to the control group (Figures 5C, D, Figure 1SD and Figure 6S); although protein expression of Beclin1 was also increased, it was not significant. ULK1 deletion decreased protein expression levels of p-ULK1(ser 757, 317 and 556), Beclin1, Atg5, and LC3II/LC3I, whereas SQSTM1/p62 protein expression level was increased in bMECs infected with P. bovis (Figures 5C, D, Figure 1SD and Figure 6S). Therefore, protein expression levels were affected by deletion of ULK1 in P. bovis-infected cells. Meanwhile, P. bovis infection increased protein expression of HIF-1α and AMPKα, and deletion of ULK1 did not affect protein expression of HIF-1α and AMPKα in the P. bovis-infected group (Figure 6S). Furthermore, the addition of non-target siRNA did not affect protein expression in P. bovis-infected bMECs (Figure 4S). Additionally, P. bovis infection increased the number of LC3 puncta (average of ~10.1 puncta per cell), whereas silencing ULK1 reduced the numbers of LC3 puncta (average of ~5.3 puncta per cell) in bMECs infected with P. bovis (Figures 5E, F). In addition, the fluorescence intensity of total GFP-LC3B was also decreased in bMECs (Figure 5SB). These results suggested that ULK1 deletion reduced P. bovis infection-induced autophagy in bMECs.
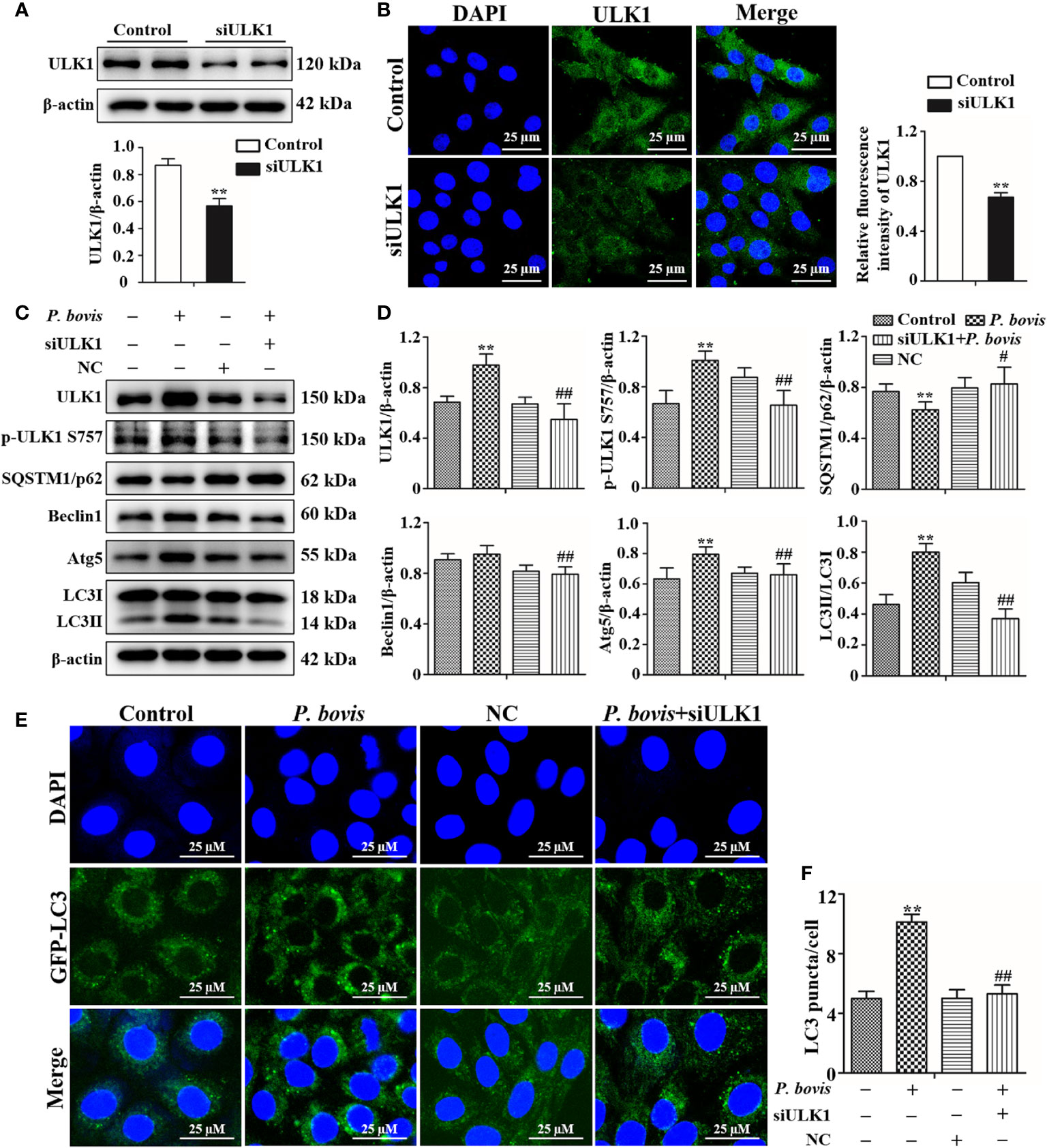
Figure 5 Silencing ULK1 reduced P. bovis induced autophagy in bMECs. (A) and (B) Western blot and immunofluorescence analyses of ULK1 after P. bovis infection for 6 h in bMECs. The right panel is protein quantification by ImageJ software. (C) and (D) Western blot analyses of ULK1, p-ULK1, SQSTM1/p62, Beclin1, Atg5, LC3I, and LC3II after P. bovis infection for 6 h in bMECs. The right panel indicates protein quantification by ImageJ software. (E) and (F) Autophagosome measurement in P. bovis infection bMECs. Ad-GFP-LC3B was used to transfect bMECs at a 20:1 multiplicity of infection for 12 h in DMEM containing 10% FBS; thereafter, bMECs were infected with P. bovis for 6 h NC indicated non-target siRNA control. Data represent means ± SD of 3 independent experiments, compared to the control group, **P < 0.01; compared to the P. bovis group, #P < 0.05 and ##P < 0.01.
HIF-1α involved in P. bovis induced autophagy in bMECs
To examine whether HIF-1α is involved in P. bovis-induced autophagy of bMECs, we silenced the HIF-1α gene and then measured effects on the AMPK/ULK1 pathway and autophagy. P. bovis infection increased protein expression levels of HIF-1α, AMPKα and ULK1, but decreased SQSTM1/p62 protein expression in bMECs (Figures 6A, B). However, silencing HIF-1α reduced AMPKα, ULK1, ULK1 and LC3II/LC3I expression, and it also increased SQSTM1/p62 protein expression (Figures 6A, B, Figure 1SE), which reduced autophagy induced by P. bovis. The protein expression levels of p-AMPKα and p-ULK1(ser 757, 317 and 556) were decreased after deletion of HIF-1α during P. bovis infection (Figure 7S). Furthermore, the addition of non-target siRNA did not affect protein expression in P. bovis-infected bMECs (Figure 4S). Meanwhile, P. bovis infection increased the number of LC3 puncta (average of ~10.5 puncta per cell), whereas silencing HIF-1α reduced the number of LC3 puncta (average of ~5.7 puncta per cell) compared to the P. bovis infection group (Figures 6C, D). Furthermore, fluorescence intensity of total GFP-LC3B was also decreased in bMECs (Figure 5SC). These results suggested that HIF-1α was involved in P. bovis-induced autophagy, and that deletion of HIF-1α inhibited autophagy in bMECs via the AMPKα and ULK1 pathway.
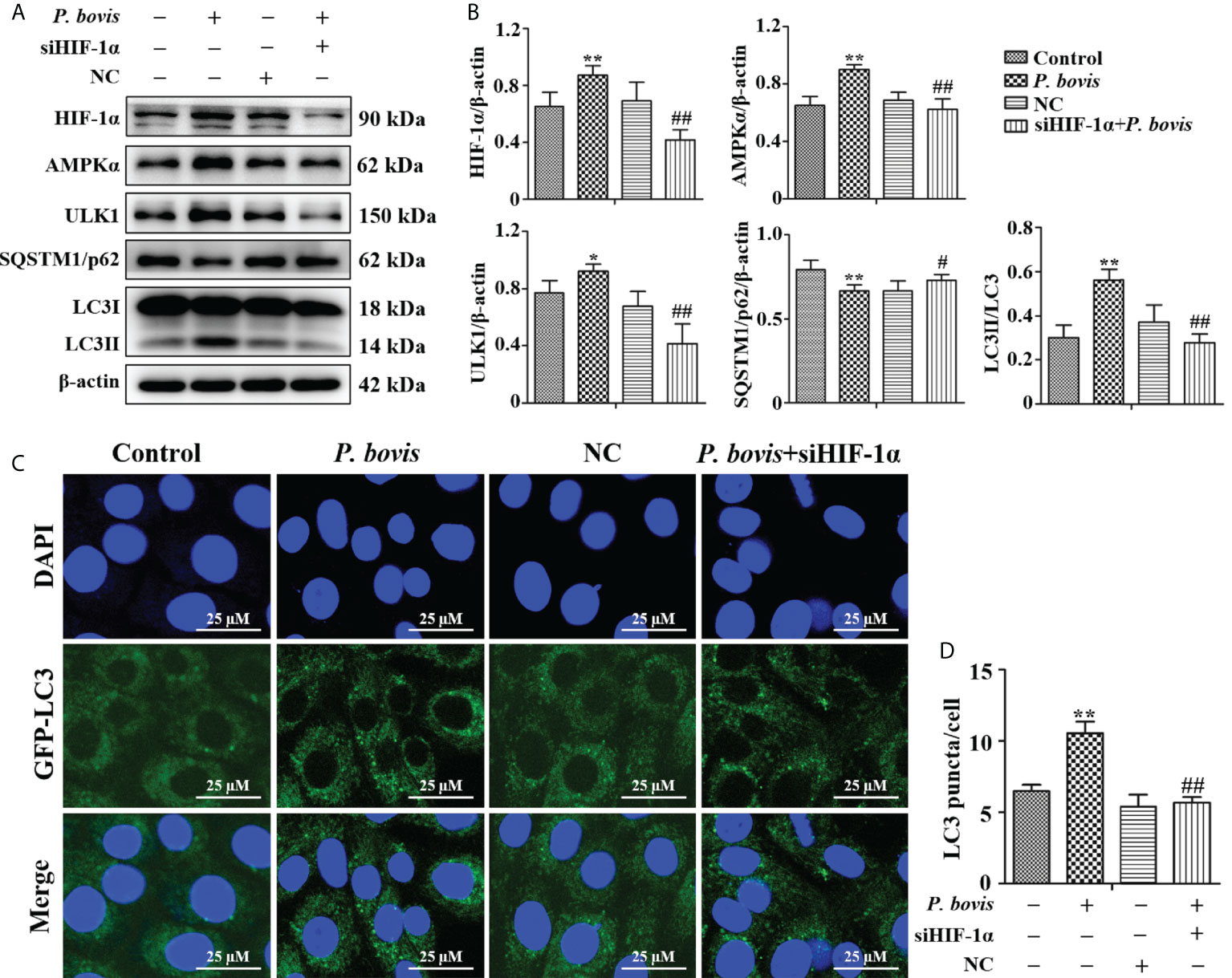
Figure 6 HIF-1α deletion reduced P. bovis induced autophagy in bMECs. (A) and (B) Western blot analyses of HIF-1α, AMPKα, ULK1, SQSTM1/p62, LC3I, and LC3II in bMECs after P. bovis infection for 6 h The right panel indicates protein quantification by ImageJ software. (C) Autophagosome measurement in P. bovis infection bMECs. Ad-GFP-LC3B was used to transfect bMECs at a 20:1 multiplicity of infection for 12 h in DMEM containing 10% FBS; then, bMECs were infected with P. bovis for 6 h NC indicated non-target siRNA control. Data represent means ± SD of 3 independent experiments, compared to the control group, *P < 0.05 and **P < 0.01; compared to the P. bovis group, #P < 0.05 and ##P < 0.01.
P. bovis infection destroyed lysosomes and inhibited autophagy in bMECs
In the late stage of autophagy, mature lysosomes fuse with autophagosomes to form autophagolysosomes to degrade damaged organelles or pathogens, with LAMP2a and Rab7 being involved in lysosome maturation. Protein expression of LAMP2a was decreased at 6 hpi, but increased at 12 hpi in bMECs infected with P. bovis, albeit not significantly, as compared to the control group (Figures 7A, B). Furthermore, rapamycin treatment increased protein expression of LAMP2a in bMECs at both 6 and 12 hpi (Figures 7A, B). Protein expression of Rab7 was increased at 6 hpi, but decreased at 12 hpi in bMECs, compared to the control group (Figures 7A, C). Furthermore, rapamycin treatment increased protein expression of Rab7 in bMECs at 6 and 12 hpi (Figures 7A, C). We inferred that the normal expression of LAMP2a and Rab7 was involved in maturation of lysosomes at 6 hpi, but lysosomes were destroyed at 12 hpi. In bMECs incubated with Lyso-Tracker Red and Ad-GFP-LC3B, red fluorescence was increased around intracellular LC3 puncta in the P. bovis infection and rapamycin treatment group at 6 h (Figure 7D). However, the red fluorescence was reduced around intracellular LC3 puncta at 12 h, although the red fluorescence in rapamycin treatment was stronger than in the P. bovis infection group at 12 h (Figure 7D). We inferred that P. bovis infection destroyed lysosomes, which inhibited autophagy in bMECs.
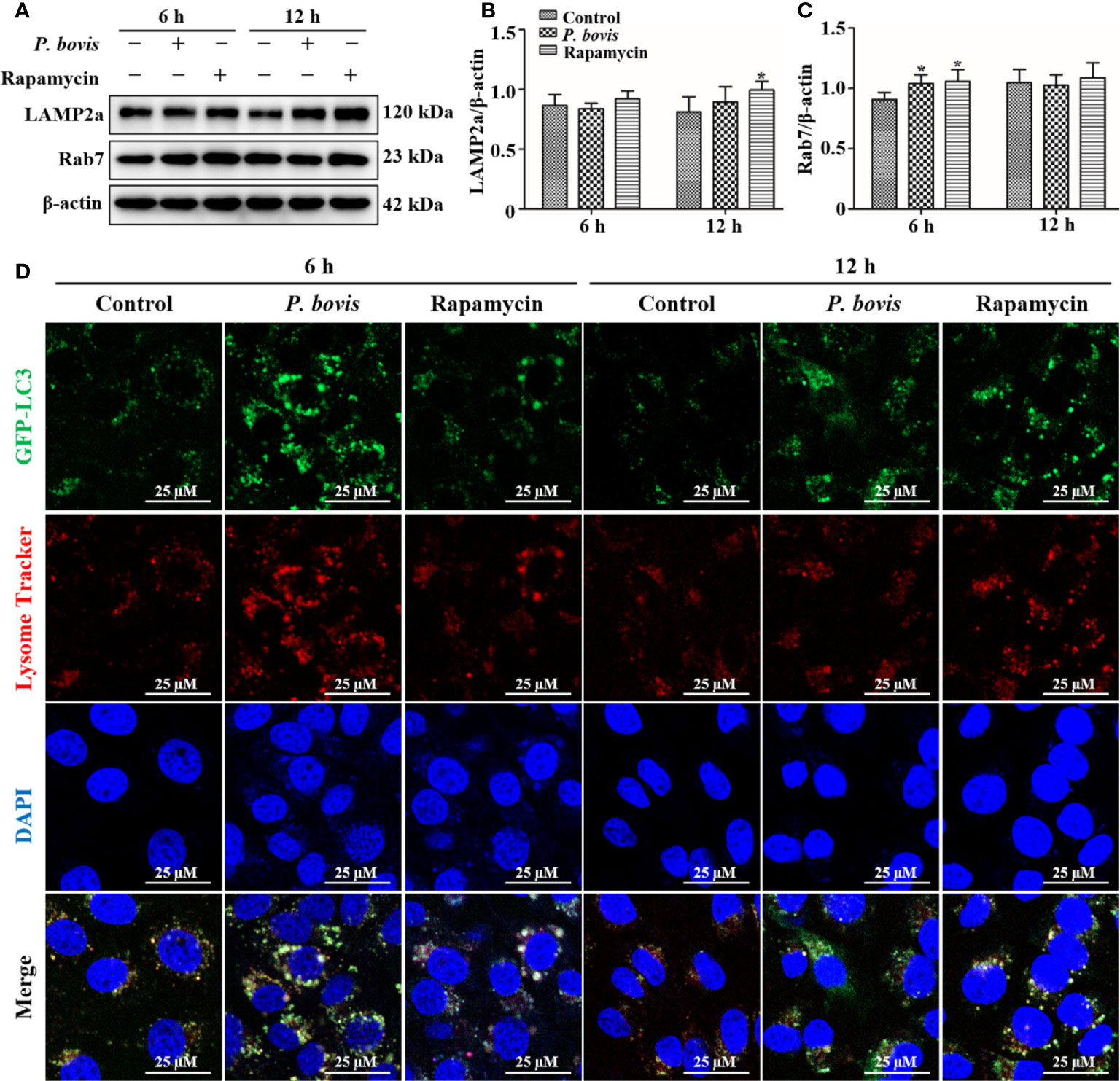
Figure 7 Autophagolysosome observations in bMECs. (A–C) Western blot analysis of LAMP2a and Rab7 in bMECs. The right panel indicates protein quantification by ImageJ software. The bMECs were infected with P. bovis and treated with rapamycin (20 µM) for 6 and 12 h, respectively. (D) Autophagolysosome measurement in P. bovis infection bMECs. Ad-GFP-LC3B was used to transfect bMECs at a 20:1 multiplicity of infection for 12 h in DMEM containing 10% FBS; then, bMECs were infected with P. bovis and treatment with rapamycin (20 µM) for 6 and 12 h, respectively, and incubated with Lyso-Tracker Red (50 nM) at 37°C for 30 min. Data represent means ± SD of 3 independent experiments, compared to the control group (*P < 0.05).
Discussion
Prototheca bovis infection induced autophagy flux, increased protein expression of LC3II/LC3I and decreased SQSTM1/p62 protein expression, which activated autophagy in bMECs. However, silencing AMPKα or ULK1 decreased LC3II/LC3I protein expression and inhibited autophagy in bMECs induced by P. bovis, whereas deletion of HIF-1α decreased protein expression levels of AMPKα and ULK1 and inhibited P. bovis-induced autophagy. Additionally, the decrease in LAMP2a and increase in Rab7 indicated that P. bovis induced autophagy in bMECs. Therefore, we concluded that P. bovis infection induced autophagy via the HIF-1α and AMPKα/ULK1 pathway in bMECs and that lysosome-associated protein Rab7 and LAMP2a were also involved.
Autophagy is a dynamic process; expression levels of LC3II/LC3I and SQSTM1/p62 indicate the magnitude of autophagic activity and flux (24). Increased expression of LC3II/LC3I and decreased expression of SQSTM1/p62 indicated a smooth autophagic flux; alternatively, the autophagic flux is inhibited (25). The LC3II/LC3I ratio had an upregulated trend during the first 6 hpi but was subsequently downregulated. Therefore, we inferred that autophagy was initially activated and the autophagic flux was smooth during the first 6 hpi, whereas the autophagic flux was subsequently inhibited at 8 to 12 hpi. During autophagosome formation, SQSTM1/p62 acts as a bridge linking LC3 and polyubiquitinated proteins, and expression levels of SQSTM1/p62 protein are negatively correlated with autophagy activity (26, 27). In this study, protein expression of SQSTM1/p62 was downregulated at 6 hpi, indicating that autophagy activity was normal. However, upregulation of SQSTM1/p62 protein at 8 to 12 hpi implied that autophagy was subsequently inhibited. Furthermore, the autophagic flux can be monitored by expression of mCherry-GFP-LC3B fusion protein from adenovirus (28). Using this approach in the present study, the number of green spots and red spots increased gradually, especially at the first 6 hpi, consistent with the autophagic flux being unobstructed. However, at 8 to 12 hpi, the autophagic flux was inhibited. Autophagosome formation is closely related to clearance of intracellular aggregated proteins, damaged organelles and invading pathogens. In cells infected with P. bovis and treated with rapamycin, autophagosomes were confirmed by transmission electron microscopy. However, we did not observe P. bovis within the autophagosome, perhaps due to the invading P. bovis having been cleared. Therefore, P. bovis infection induced autophagy in bMECs through upregulation of the LC3II/LC3I ratio and downregulation of SQSTM1/p62 protein expression.
Initiation of autophagy is regulated by a variety of intracellular signaling pathways. The AMPKα/mTOR signal pathway is the critical factor regulating autophagosome formation (29, 30). Furthermore, mTOR is a serine/threonine protein kinase in the mTORC1 complex and a major regulator of autophagy (31). Activation of AMPK may cause autophagy by negatively modulating mTOR (32). Prototheca bovis infection upregulated protein expression of p-mTOR, whereas rapamycin treatment decreased p-mTOR protein expression in bMECs. Furthermore, protein expression of autophagy-related genes, including Atg5, Beclin1 and LC3II/LC3I (but not SQSTM1/p62), were also upregulated in bMECs, indicating the occurrence of autophagy. High expression of p-mTOR protein may be related to AMPKα, as protein expression of AMPKα and p-AMPKα were upregulated in bMECs. Although activation of AMPKα did not negatively modulate mTOR, autophagy in bMECs may be linked to the ability of AMPK to directly phosphorylate ULK1 ser 317 and induce autophagy. Prototheca bovis infection upregulated protein expression of ULK1 and p-ULK1 (ser 317) in bMECs. Expression of ULK1 is also regulated by mTOR-dependent inhibitory phosphorylation under nutrient-rich conditions (33). Under nutrient sufficiency, high mTOR activity prevents ULK1 activation by phosphorylating ULK1 ser 757 and disrupting the interaction between ULK1 and AMPK. In contrast, under pathological conditions (infection or drug treatment), elevated phosphorylated ULK1 (ser 757) protein initiates autophagy, and high mTOR activity does not completely prevent ULK1 (ser 757) phosphorylation to inhibit autophagy in cells (33, 34). Prototheca bovis infection increased protein expression of ULK1 and p-ULK1 (ser 757) in bMECs, whereas mTOR did not completely inhibit autophagy by phosphorylating ULK1 ser 757. We inferred that activation and phosphorylation of ULK1 were not only related to activation of AMPKα, but also to elevation of mTOR, as there was no negative regulation between AMPKα and mTOR in bMECs. Therefore, activation of protein in the AMPKα/ULK1 pathway was involved in P. bovis infection-induced autophagy in bMECs.
Activation of ULK1 is considered a key regulator of autophagy, with AMPK and mTOR catalyzing activation of ULK1 (35, 36). In the current study, P. bovis infection contributed to AMPKα, mTOR, and ULK1 phosphorylation and induced autophagy in bMECs. However, deletion of AMPKα decreased protein expression of ULK1 and p-ULK1 in P. bovis infected bMECs, implicating AMPKα as having an important role in activation of ULK1 and promoting ULK1 phosphorylation. Meanwhile, protein expression of SQSTM1/p62 was downregulated in bMECs, indicating that AMPKα deletion did not affect SQSTM1/p62 expression. In addition, protein expression of LC3II/LC3I was downregulated and the number of LC3 puncta reduced in bMECs, indicating that P. bovis infection induced autophagy was inhibited in bMECs. AMPKα deletion reduced ULK1 phosphorylation, but protein expression of HIF-1α was not decreased compared to the P. bovis infection group, suggesting that AMPKα may be a downstream factor of HIF-1α. Silencing AMPKα reduced autophagy induced by P. bovis infection in bMECs. Therefore, AMPKα has an important role in autophagy activation by promoting ULK1 phosphorylation in bMECs infected with P. bovis. ULK1 acts as a bridge between upstream nutrient or energy sensors mTOR and AMPK and downstream autophagosome formation, and phosphorylated ULK1 is considered a key regulator of autophagy (37). In the current study, deletion of ULK1 upregulated SQSTM1/p62 protein expression and downregulated LC3II/LC3I protein expression in bMECs infected with P. bovis, which indicated that autophagy induced by P. bovis infection was inhibited. Furthermore, the reduced number of LC3 puncta also indicated that autophagy was inhibited due to ULK1 deletion in bMECs. Additionally, deletion of ULK1 did not decrease protein expression of AMPKα and HIF-1α compared to the P. bovis infection group, suggesting that ULK1 may be a downstream factor of AMPKα. Therefore, we concluded that P. bovis infection induced autophagy by modulating the AMPKα/ULK1 pathway in bMECs (Figure 8). As the regulatory subunit of hypoxia-inducible factor 1 (HIF-1), HIF-1α is the master regulator of oxygen homeostasis (38, 39). Furthermore, it is also involved in many cellular processes, including inflammatory response, apoptosis, and autophagy (40–42). Deficiency of HIF-1α enhances influenza A virus replication by promoting autophagy in alveolar type II epithelial cells (22). In the present study, P. bovis infection upregulated HIF-1α protein expression in bMECs. In addition, silencing HIF-1α decreased protein expression of AMPKα and ULK1 in bMECs during P. bovis infection, whereas SQSTM1/p62 protein expression was upregulated, suggesting that AMPKα and ULK1 may be a downstream factor of HIF-1α and HIF-1α may indirectly affect expression of ULK1 by regulating AMPKα in bMECs. Therefore, we inferred that HIF-1α deletion inhibited autophagy in bMECs induced by P. bovis. Furthermore, we concluded that HIF-1α has an important role in autophagy induced by P. bovis through regulated AMPKα and ULK1 protein expression in bMECs (Figure 8).
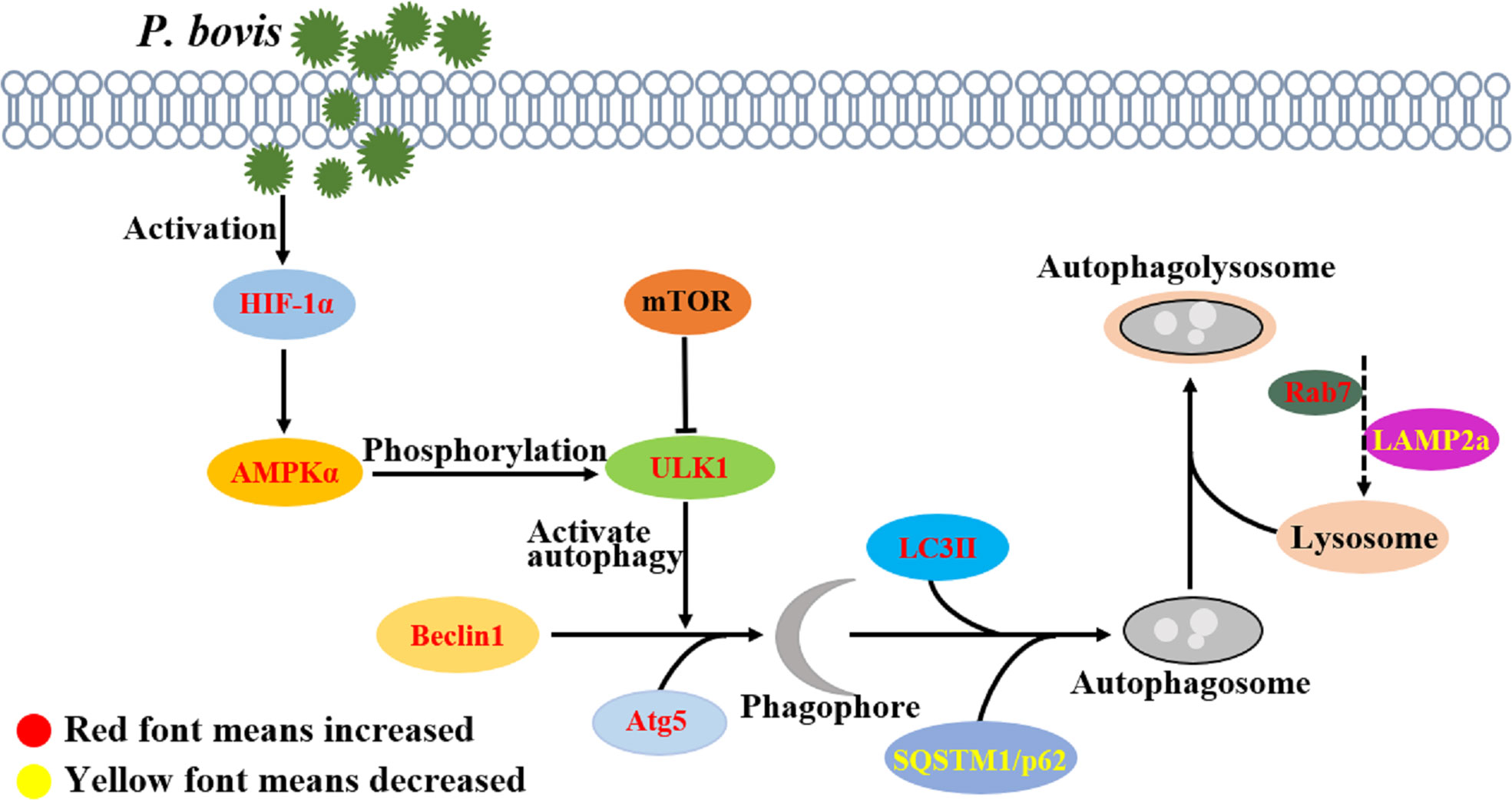
Figure 8 P. bovis infection induced autophagy in bMECs. P. bovis infection activates HIF-1α, and activation of HIF-1α has an important role in regulating AMPKα and ULK1, whereas activation of AMPKα promotes ULK1 phosphorylation. During P. bovis infection, ULK1 activates autophagy, and Beclin1 and Atg5 are involved in phagophore formation. Then, LC3II and SQSTM1/p62, as an indicator of autophagic activity, are involved in autophagosome formation. P. bovis infection induces autophagy via the HIF-1α and AMPKα/ULK1 pathway, and Rab7 and LAMP2a are involved in autophagolysosome formation induced by P. bovis in bMECs.
Lysosomes degrade cytoplasmic proteins and damaged organelles, as well as autophagolysosomes formed during autophagy (43). Furthermore, Rab7 is essential for autophagosome-lysosome fusion during in the last phases of autophagy, with downregulation of Rab7 causing an autophagic block (13, 44). Infection with P. bovis upregulated protein expression of Rab7 in bMECs at 6 hpi, but downregulated it at 12 hpi. We concluded that autophagy was normal at 6 hpi but blocked at 12 hpi, and furthermore, that changes in Rab7 causing autophagy were consistent with previous studies. Additionally, lysosome-associated membrane protein 2a (LAMP2a) is a component of the lysosomal membrane, with an important role in maintaining structural integrity of the lysosome (13, 45). Prototheca bovis infection downregulated protein expression of LAMP2a in bMECs at 6 hpi, but upregulated it at 12 hpi, albeit neither change was significant, suggesting that lysosomes were normal at 6 hpi but damaged at 12 hpi, and that this lysosomal damage prevented autophagy. Abnormal fusion of mature lysosomes and autophagosomes will severely affect the clearance of damaged organelles and pathogens, and autophagy (confirmed by lysosomal staining). Therefore, we concluded that lysosome-associated protein Rab7 and LAMP2a had important roles in P. bovis-induced autophagy in bMECs.
In conclusion, P. bovis infection is one of the main causes of mastitis in dairy cows, and autophagy is an effective way to defense against pathogenic infection. P. bovis infection activated HIF-1α and promoted expression of AMPKα and ULK1; knocking down either of them inhibited autophagy in bMECs. This was interpreted as evidence that P. bovis infection induces autophagy in bMECs via the HIF-1α and AMPKα/ULK1 pathway.
Data availability statement
The original contributions presented in the study are included in the article/Supplementary Material. Further inquiries can be directed to the corresponding authors.
Author contributions
BH and WZ conceived and designed the experiment. WZ, MX, XX and ZD performed the research and wrote the manuscript. HB, DW, YL, and SK assisted in the analyses and re-edited the manuscript. HB, JK and BH revised the manuscript. All authors read and approved the final manuscript.
Funding
Financial support for this study was received from the following: Beijing Municipal Natural Science Foundation (no. 6222031), Ningxia Key R&D Project (No. 2019BBF02027), the National Natural Science Foundation of China (No. 31572587, 31760751), the High-End Foreign Experts Recruitment Program (No. GDT20171100013) and the Natural Science Foundation of Ningxia (No. 2022AAC02022).
Conflict of interest
The authors declare that the research was conducted in the absence of any commercial or financial relationships that could be construed as a potential conflict of interest.
Publisher’s note
All claims expressed in this article are solely those of the authors and do not necessarily represent those of their affiliated organizations, or those of the publisher, the editors and the reviewers. Any product that may be evaluated in this article, or claim that may be made by its manufacturer, is not guaranteed or endorsed by the publisher.
Supplementary material
The Supplementary Material for this article can be found online at: https://www.frontiersin.org/articles/10.3389/fimmu.2022.934819/full#supplementary-material
References
1. Christopher DS, Linda M, Robin CM. Now for something completely different: Prototheca, pathogenic algae. PLoS Pathog (2021) 17(4):1009362. doi: 10.1371/journal.ppat.1009362
2. Jagielski T, Roeske K, Bakuła Z, Piech T, Wlazło Ł, Bochniarz M, et al. A survey on the incidence of Prototheca mastitis in dairy herds in Lublin Province, Poland. J Dairy Sci (2019) 102(1):619–28. doi: 10.3168/jds.2018-15495
3. Jagielski T, Krukowski H, Bochniarz M, Piech T, Roeske K, Bakula Z, et al. Prevalence of Prototheca spp. on dairy farms in Poland-a cross-country study. Microb Biotechnol (2019) 12(3):556–66. doi: 10.1111/1751-7915.13394
4. Shahid M, Cavalcante PA, Knight CG, Barkema HW, Han B, Gao J, et al. Murine and human cathelicidins contribute differently to hallmarks of mastitis induced by pathogenic Prototheca bovis algae. Front Cell Infect Microbiol (2020) 10:31. doi: 10.3389/fcimb.2020.00031
5. Kim JK, Park EJ, Jo EK. Itaconate, arginine, and gamma-aminobutyric acid: A host metabolite triad protective against mycobacterial infection. Front Immunol (2022) 13:832015. doi: 10.3389/fimmu.2022.832015
6. Zhao WP, He FM, Barkema HW, Xu SY, Gao J, Liu G. Prototheca spp. induce an inflammatory response via mtROS-mediated activation of NF-κB and NLRP3 inflammasome pathways in bovine mammary epithelial cell cultures. Vet Res (2021) 52(1):144. doi: 10.1186/s13567-021-01014-9
7. Shahid M, Cobo ER, Chen L, Cavalcante PA, Barkema HW, Gao J, et al. Prototheca zopfii genotype II induces mitochondrial apoptosis in models of bovine mastitis. Sci Rep (2020) 10(1):698. doi: 10.1038/s41598-020-57645-z
8. Fleming A, Bourdenx M, Fujimaki M, Karabiyik C, Krause GJ, Lopez A, et al. The different autophagy degradation pathways and neurodegeneration. Neuron (2022) 110(6):935–66. doi: 10.1016/j.neuron.2022.01.017
9. Siva SD, Hu Z, Dengjel J. The complex interplay between ULK1 and protein phosphatases in autophagy regulation. Autophagy (2022) 18(2):455–6. doi: 10.1080/15548627.2021.2002546
10. Losier TT, Russell RC. Bacterial outer membrane vesicles trigger pre-activation of a xenophagic response via AMPK. Autophagy (2019) 15(8):1489–91. doi: 10.1080/15548627.2019.1618640
11. Zhou L, Li Y, Gao S, Yuan H, Zuo L, Wu C, et al. Salmonella spvC gene inhibits autophagy of host cells and suppresses NLRP3 as well as NLRC4. Front Immunol (2021) 12:639019. doi: 10.3389/fimmu.2021.639019
12. Fang C, Woo JA, Liu T, Zhao X, Cazzaro S, Yan Y, et al. SSH1 impedes SQSTM1/p62 flux and MAPT/Tau clearance independent of CFL (cofilin) activation. Autophagy (2021) 17(9):2144–65. doi: 10.1080/15548627.2020.1816663
13. Eskelinen EL. Roles of LAMP-1 and LAMP-2 in lysosome biogenesis and autophagy. Mol Aspects Med (2006) 27:495–502. doi: 10.1016/j.mam.2006.08.005
14. King KE, Losier TT, Russell RC. Regulation of autophagy enzymes by nutrient signaling. Trends Biochem Sci (2021) 46(8):687–700. doi: 10.1016/j.tibs.2021.01.006
15. Karabiyik C, Rubinsztein DC. AMPK-activated ULK1 phosphorylates PIKFYVE to drive formation of PtdIns5P-containing autophagosomes during glucose starvation. Autophagy (2021) 17(11):3877–8. doi: 10.1080/15548627.2021.1961409
16. Joungmok K, Mondira K, Benoit V, Guan K. AMPK and mTOR regulate autophagy through direct phosphorylation of ULK1. Nat Cell Biol (2011) 13(2):132–41. doi: 10.1038/ncb2152
17. Liu R, Li X, Ma H, Yang Q, Shang Q, Song L, et al. Spermidine endows macrophages anti-inflammatory properties by inducing mitochondrial superoxide dependent AMPK activation, hif-1α upregulation and autophagy. Free Radic Biol Med (2020) 161:339–50. doi: 10.1016/j.freeradbiomed.2020.10.029
18. Afsane B, Stephen LA, Muhammed M, Amirhossein S. Effects of curcumin on hypoxia-inducible factor as a new therapeutic target. Pharmacol Res (2018) 137:159–69. doi: 10.1016/j.phrs.2018.10.009
19. Li X, Lee Y, Kang Y, Dai B, Perez MR, Pratt M, et al. Hypoxia-induced autophagy of stellate cells inhibits expression and secretion of lumican into microenvironment of pancreatic ductal adenocarcinoma. Cell Death Differ (2019) 26(2):382–93. doi: 10.1038/s41418-018-0207-3
20. Zhao C, Chen J, Cheng L, Xu K, Yang Y, Su X. Deficiency of HIF-1α enhances influenza a virus replication by promoting autophagy in alveolar type II epithelial cells. Emerg Microbes Infect (2020) 9(1):691–706. doi: 10.1080/22221751.2020.1742585
21. Hurley JH, Young LN. Mechanisms of autophagy initiation. Ann Rev Biochem (2017) 86:225–44. doi: 10.1146/annurev-biochem-061516-044820
22. Li T, Kong L, Li X, Wu S, Attri KS, Li Y, et al. Listeria monocytogenes upregulates mitochondrial calcium signalling to inhibit LC3-associated phagocytosis as a survival strategy. Nat Microbiol (2021) 6(3):366–79. doi: 10.1038/s41564-020-00843-2
23. Zhao W, Deng Z, Barkema HW, Xu M, Gao J, Liu G, et al. Nrf2 and NF-κB/NLRP3 inflammasome pathways are involved in Prototheca bovis infections of mouse mammary gland tissue and mammary epithelial cells. Free Radic Biol Med (2022) 184:148–57. doi: 10.1016/j.freeradbiomed.2022.04.005
24. Goode A, Butler K, Long J, Cavey J, Scott D, Shaw B, et al. Defective recognition of LC3B by mutant SQSTM1/p62 implicates impairment of autophagy as a pathogenic mechanism in ALS-FTLD. Autophagy (2016) 12(7):1094–104. doi: 10.1080/15548627.2016.1170257
25. Miyakawa K, Nishi M, Ogawa M, Matsunaga S, Sugiyama M, Nishitsuji H, et al. Galectin-9 restricts hepatitis b virus replication via p62/SQSTM1-mediated selective autophagy of viral core proteins. Nat Commun (2022) 13(1):531. doi: 10.1038/s41467-022-28171-5
26. Tanida I. Autophagosome formation and molecular mechanism of autophagy. Antioxid Redox Signal (2011) 14(11):2201–14. doi: 10.1089/ars.2010.3482
27. Geng N, Wang XZ, Yu XH, Wang R, Zhu YR, Zhang MH, et al. Staphylococcus aureus avoids autophagy clearance of bovine mammary epithelial cells by impairing lysosomal function. Front Immunol (2020) 11:746. doi: 10.3389/fimmu.2020.00746
28. Guo H, Ouyang Y, Yin H, Cui H, Deng H, Liu H, et al. Induction of autophagy via the ROS-dependent AMPK-mTOR pathway protects copper-induced spermatogenesis disorder. Redox Biol (2022) 49:102227. doi: 10.1016/j.redox.2021.102227
29. Luo Q, Song Y, Kang J, Wu Y, Wu F, Li Y, et al. mtROS-mediated Akt/AMPK/mTOR pathway was involved in copper-induced autophagy and it attenuates copper-induced apoptosis in RAW264.7 mouse monocytes. Redox Biol (2021) 41:101912. doi: 10.1016/j.redox.2021.101912
30. Shariq M, Quadir N, Alam A, Zarin S, Sheikh JA, Sharma N, et al. The exploitation of host autophagy and ubiquitin machinery by Mycobacterium tuberculosis in shaping immune responses and host defense during infection. Autophagy (2022). doi: 10.1080/15548627.2021.2021495
31. Tesseraud S, Avril P, Bonnet M, Bonnieu A, Cassar-Malek I, Chabi B, et al. Autophagy in farm animals: Current knowledge and future challenges. Autophagy (2021) 17(8):1809–27. doi: 10.1080/15548627.2020.1798064
32. Kim IJ, Lee J, Oh SJ, Yoon MS, Jang SS, Holland RL, et al. Helicobacter pylori infection modulates host cell metabolism through vaca-dependent inhibition of mTORC1. Cell Host Microbe (2018) 23(5):583–93. doi: 10.1016/j.chom.2018.04.006
33. Zhao X, Tang X, Guo N, An Y, Chen X, Shi C, et al. Biochanin a enhances the defense against Salmonella enterica infection through AMPK/ULK1/mTOR-mediated autophagy and extracellular traps and reversing SPI-1-dependent macrophage (MΦ) M2 polarization. Front Cell Infect Microbiol (2018) 8:318. doi: 10.3389/fcimb.2018.00318
34. Yu W, Zha W, Ren J. Exendin-4 and liraglutide attenuate glucose toxicity-induced cardiac injury through mTOR/ULK1-dependent autophagy. Oxid Med Cell Longev (2018) 2018:5396806. doi: 10.1155/2018/5396806
35. Mukhopadhyay R, Venkatadri R, Katsnelson J, Arav-Boger R. Digitoxin suppresses human cytomegalovirus replication via na+, K+/ATPase α1 subunit-dependent AMP-activated protein kinase and autophagy activation. J Virol (2018) 92(6):e01861–17. doi: 10.1128/JVI.01861-17
36. McGettrick AF, O'Neill LAJ. The role of HIF in immunity and inflammation. Cell Metab (2020) 32(4):524–36. doi: 10.1016/j.cmet.2020.08.002
37. Kim J, Kundu M, Viollet B, Guan KL. AMPK and mTOR regulate autophagy through direct phosphorylation of Ulk1. Nat Cell Biol (2011) 13(2):132–41. doi: 10.1038/ncb2152
38. Catrina SB, Zheng X. Hypoxia and hypoxia-inducible factors in diabetes and its complications. Diabetologia (2021) 64(4):709–16. doi: 10.1007/s00125-021-05380-z
39. He Q, Ma Y, Liu J, Zhang D, Ren J, Zhao R, et al. Biological functions and regulatory mechanisms of hypoxia-inducible factor-1α in ischemic stroke. Front Immunol (2021) 12:801985. doi: 10.3389/fimmu.2021.801985
40. Oliveira MF, Medeiros RCA, Mietto BS, Calvo TL, Mendonça APM, Rosa TLSA, et al. Reduction of host cell mitochondrial activity as Mycobacterium leprae’s strategy to evade host innate immunity. Immunol Rev (2021) 301(1):193–208. doi: 10.1111/imr.12962
41. Quäschling T, Friedrich D, Deepe GS Jr, Rupp J. Crosstalk between autophagy and hypoxia-inducible factor-1α in antifungal immunity. Cells (2020) 9(10):2150. doi: 10.3390/cells9102150
42. Li Q, Ni Y, Zhang L, Jiang R, Xu J, Yang H, et al. HIF-1α-induced expression of m6A reader YTHDF1 drives hypoxia-induced autophagy and malignancy of hepatocellular carcinoma by promoting ATG2A and ATG14 translation. Signal Transduct Target Ther (2021) 6(1):76. doi: 10.1038/s41392-020-00453-8
43. David L, Taieb F, Pénary M, Bordignon PJ, Planès R, Bagayoko S, et al. Outer membrane vesicles produced by pathogenic strains of Escherichia coli block autophagic flux and exacerbate inflammasome activation. Autophagy (2022). doi: 10.1080/15548627.2022.2054040
44. Krause K, Caution K, Badr A, Hamilton K, Saleh A, Patel K, et al. CASP4/caspase-11 promotes autophagosome formation in response to bacterial infection. Autophagy (2018) 14(11):1928–42. doi: 10.1080/15548627.2018.1491494
Keywords: mastitis, Prototheca bovis, autophagy, bovine mammary epithelial cells, HIF-1α, AMPKα/ULK1
Citation: Zhao W, Xu M, Barkema HW, Xie X, Lin Y, Khan S, Kastelic JP, Wang D, Deng Z and Han B (2022) Prototheca bovis induces autophagy in bovine mammary epithelial cells via the HIF-1α and AMPKα/ULK1 pathway. Front. Immunol. 13:934819. doi: 10.3389/fimmu.2022.934819
Received: 03 May 2022; Accepted: 10 August 2022;
Published: 02 September 2022.
Edited by:
Taylor Sitarik Cohen, AstraZeneca, United StatesReviewed by:
Hee Sam Na, Pusan National University, South KoreaLin Wang, Shandong Agricultural University, China
Lian Li, Nanjing Agricultural University, China
Jinfeng Miao, Nanjing Agricultural University, China
Copyright © 2022 Zhao, Xu, Barkema, Xie, Lin, Khan, Kastelic, Wang, Deng and Han. This is an open-access article distributed under the terms of the Creative Commons Attribution License (CC BY). The use, distribution or reproduction in other forums is permitted, provided the original author(s) and the copyright owner(s) are credited and that the original publication in this journal is cited, in accordance with accepted academic practice. No use, distribution or reproduction is permitted which does not comply with these terms.
*Correspondence: Zhaoju Deng, emhhb2p1MjAyMEBob3RtYWlsLmNvbQ==; Bo Han, aGFuYm9AY2F1LmVkdS5jbg==