- Department of Systems Immunology, Weizmann Institute of Science, Rehovot, Israel
The clinical use of anti-CD40 agonist monoclonal antibodies (mAbs) is aimed at recruiting the immune system to fight the tumor cells. This approach has been demonstrated to be effective in various preclinical models. However, human CD40 Abs displayed only modest antitumor activity in cancer patients, characterized by low efficacy and dose-limiting toxicity. While recent studies highlight the importance of engineering the Fc region of human CD40 mAbs to optimize their agonistic potency, toxicity remains the main limiting factor, restricting clinical application to suboptimal doses. Here, we discuss the current challenges in realizing the full potential of CD40 mAbs in clinical practice, and describe novel approaches designed to circumvent the systemic toxicity associated with CD40 agonism.
Introduction
The field of immuno-oncology has progressed steadily over the last decade. Immunotherapy has joined the ranks of surgery, chemotherapy, radiation, and targeted therapy in the arsenal of cancer treatments (1, 2). An increasing number of immune checkpoint-targeted monoclonal antibodies (mAbs) have been developed with the aim of harnessing the immune system to eradicate tumor cells (3, 4). These efforts have resulted in successful clinical application of blocking mAbs against CTLA-4 and PD-1/PD-L1 checkpoints on T lymphocytes (T cells) to induce effective tumor-eliminating immunity. However, a remaining unmet clinical challenge is to stimulate immunity against “cold” tumors, which lack significant immune infiltration at treatment onset. Agonist mAbs targeting the cluster of differentiation 40 (CD40) immune receptor emerge as a potential approach to increase the number and quality of tumor-infiltrating T cells (TILs) and, thereby, response effectiveness, either as a monotherapy or to reverse resistance to checkpoint-blocking antibodies (5–9).
CD40 is a tumor necrosis factor receptor (TNFR) superfamily member. It is expressed on antigen-presenting cells (APCs) including dendritic cells (DCs), B cells, macrophages, classical and non-classical monocytes (10–12), on a variety of non-immune cells including platelets and endothelial cells (13, 14), and on several types of tumor cells (15). CD40 plays a central role in stimulating immune synapses, including during T cell priming by APCs, when its interaction with the CD40 ligand (CD40L) licenses DCs to activate antigen-specific T cells (5, 16). This is accomplished through the upregulation of major histocompatibility complex (MHC) molecules, increased expression of the costimulatory molecules CD86/CD80, and upregulation of TNF superfamily ligands on the DC surface, as well as by secretion of interleukin-12 (IL-12), which fuels CD8+ T cell activation. Likewise, the CD40/CD40L axis plays a central role in the B-T cell immune synapse, promoting B cell activation and proliferation as well as antigen presentation (5, 6, 11, 16).
Agonistic anti-CD40 Abs are designed to mimic CD40L by crosslinking CD40 and, thereby, promote the maturation of DCs and improve their antigen presentation capabilities. This results in expansion of tumor antigen-specific cytotoxic T cells, which can lead to the eradication of tumors (5, 17, 18). Motivated by promising results in a variety of cancer animal models, several human CD40 mAbs have been developed and evaluated in clinical trials over the last two decades (6, 19–22). However, the preclinical potency has not yet been recapitulated in clinical setting and none of these mAbs has advanced beyond early trial phases. Among the challenges that were encountered during these evaluations are low detected levels of immune activation and high toxicity levels associated with the treatment. The toxicity limited the use of CD40 mAbs to suboptimal doses, resulting in insufficient immune activation and antitumor efficacy (21, 23–26). Here, we highlight key factors and cellular pathways associated with effective agonism and the observed clinical toxicity. Furthermore, we describe recent antibody-engineering approaches and treatment regimens that we find the most advanced and promising in the quest to overcome the challenges preventing the clinical use of CD40 agonistic mAbs.
Harnessing FcγRs to Potentiate the Activity of CD40 mAbs
Fc-gamma receptors (FcγR) are central players in the in vivo agonistic activity of CD40 mAbs (25, 27–29). This Fc-mediated mechanism involves higher order crosslinking of the CD40 mAbs by FcγRIIB expressed in trans by cells neighboring the CD40-expressing cells. This results in enhanced clustering of CD40 on the target cell and, consequently, increased CD40 signaling. The relatively low clinical response elicited by different anti-human CD40 mAbs (15, 19) can be attribute to the structure of their IgG scaffold, which is not optimized for FcγRIIB binding. It was demonstrated that the in vivo activity of human CD40 mAbs is dependent on their affinity to FcγRIIB and, notably, this activity was significantly improved by Fc engineering (25) (Figure 1). Following this preclinical observation, a second generation of Fc-engineered anti-human CD40 mAbs with enhanced FcγRIIB binding are now being tested in clinical trials (25, 30–32).
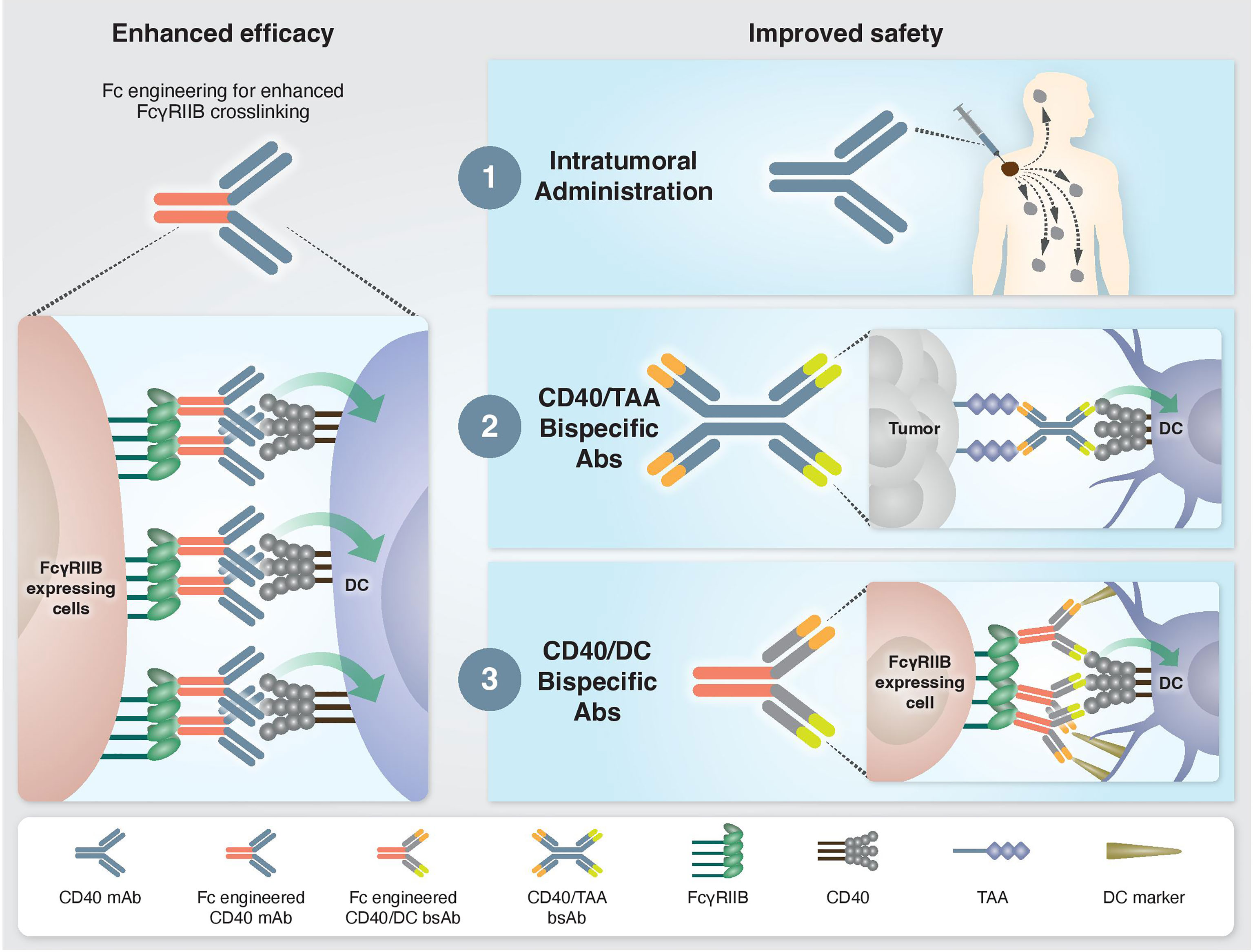
Figure 1 Approaches to enhance the efficacy and safety of CD40 agonistic mAbs. Left: Enhanced CD40 agonism by Fc-engineered mAbs designed to increase FcγRIIB-mediated crosslinking. Right: Approaches to bypass treatment associated toxicities. 1) Intratumoral administration. Injection of low mAb dose directly into the tumor enables local antitumor immune activation without systemic side effects. 2) Tumor-targeted bispecific CD40 antibodies direct the agonistic antibody to the TME by targeting tumor-associated antigens, which are overexpressed and/or selectively expressed at the tumor site. 3) Dendritic cell-targeted bispecific CD40 antibodies direct the agonistic antibody to the cell types that drive treatment-associated antitumor activity but not toxicity.
One such antibody is 2141-V11. Based on selicrelumab, the original IgG2 isotype was converted into IgG1 and the affinity to FcγRIIB was selectively enhance by Fc engineering. The Fc-engineered version of this mAb displayed a significantly enhanced in vivo antitumor response compared to the parental IgG2 variant in multiple tumor models, includes melanoma, colon adenocarcinoma and bladder cancer (25, 30, 31). APX005M (sotigalimab) is another CD40 mAb that was Fc-engineered to increase the interaction with FcγRIIB, now evaluated in several early-phase studies (32, 33). Different Fc mutations were introduced to the IgG1-Fc scaffold of 2141-V11 and APX005M. While the binding of 2141-V11 is enhanced selectively to FcγRIIB and not to other FcγRs, APX005M engages both the inhibitory FcγRIIB and the activating FcγRIIA131R. Preclinical studies showed increased in vivo agonistic activity for both Fc-engineered mAbs over their parental non-mutated IgG1 variant (25). However, due to the opposite effect of decreased mAb potency upon engagement with FcγRIIA, the FcγRIIB-selective enhanced Fc variant displayed superior agonistic activity.
Because crosslinking of CD40 on the membrane surface is key for the activity of CD40 agonists, various strategies to enhance CD40 receptor trimerization have been developed. These Fc-independent approaches include hinge engineering to the unique structural configuration of IgG2 subclass, which was reported to enhance CD40 agonistic activity. Mutations of specific cysteines in CD40 agonistic mAbs are used to prevent shuffling of disulfide bonds between the IgG2 hinge and CH1 regions, thus locking the hinge conformation that contributes to enhanced CD40 clustering (34, 35). Other approaches to promote CD40 receptor multimerization include the use of recombinant CD40L-based instead of antibody-based molecules (36), or utilizing Fc-docking scaffolds to multimerize anti-CD40 mAbs (37). A notable difference between these Fc-dependent and Fc-independent engineering approaches is the requirement for FcγRs engagement in addition to CD40 engagement in the former but not the latter, which may results in different biodistributions of these molecules. The consequences of these distinct properties for the mechanism and therapeutic index of these reagents should be clarified in future studies. A combination of different approaches to enhance agonism was also suggested in a study demonstrating synergistic agonist potency of a combined hinge and Fc-engineering strategy (38).
While these Fc and protein engineering strategies can improve the antitumor efficacy of CD40 agonists, the stronger potency of these next-generation agonists is accompanied by an increase in side effects and toxicity that characterize this type of immunotherapy (25, 30). Consequently, the systemic administration of these agonists is limited to suboptimal doses and their full potential could not be exploited.
Side Effects and Toxicities of CD40 mAbs
As mentioned, human CD40 agonistic mAbs were reported to trigger severe adverse effects and toxicities. These include hepatotoxicity, cytokine release syndrome (CRS) (19, 20, 39), thrombocytopenia (19, 24, 25, 30), general hyperimmune stimulation (40), and tumor angiogenesis in response to endothelial cell activation (41). The broad expression of CD40 by various immune and non-immune cells types in the tumor and in other organs is likely to contribute to the occurrence of these side effects.
Recent studies highlighted the role of macrophages, Kupffer cells, platelets and neutrophils in mediating liver toxicity. Using a single-cell RNA sequencing approach, Siwicki et al. described a mechanistic interplay involving IFN-γ-secreting lymphocytes and IL-12-producing tissue-resident Kupffer cells, resulting in liver toxicity (42). This anti-CD40 mediated hepatotoxicity is associated with an IL-12-dependent accumulation of MHC II+, CD14+ and CD11b+ macrophages in the liver (43). It was further shown that IL-12 and IFN-γ were not toxic by themselves and that neutrophils respond to these two cytokines by upregulation and secretion of TNF, the levels of which determine the severity of liver toxicity. Another player in the network that mediates the toxic effect of CD40 mAbs on the liver are platelets. In a recent study, we have demonstrated the causal role of macrophages and platelets in liver toxicity after CD40 treatment (44). Systemic cell depletion of macrophages or platelets completely abrogated the elevation in liver transaminases (ALT/AST) that was observed after anti-human CD40 treatment. While these findings highlight the involvement of macrophages, Kupffer cells, platelets and neutrophils, the full mechanistic interplay between these players driving liver toxicity following anti-CD40 treatment still needs to be elucidated.
In the clinic, CRS was evident within minutes to hours after CD40 mAb infusion and was associated in these patients with elevation in serum IL-6 (19). In vivo upregulation of intracellular IL-6 was detected by classical CD11c- monocytes in the blood, lymph nodes and spleen, after immunization with anti-human CD40 mAb in humanized CD40 mouse strain (44). This suggests monocytes as the major cell population driving IL-6 secretion.
DCs and, in particular, the conventional type-1 dendritic cells (cDC1s) are essential for CD40-targeted immunotherapy due to their key role in CD8+ T cell priming and early CD4+ T cell activation, which induce a strong and durable antitumor immunity (45). Unlike macrophages, monocytes and platelets that mediate hepatotoxicity, CRS and thrombocytopenia, respectively, cDC1 activation by CD40 agonist do not contribute to any of these dose-limiting toxicities.
Collectively, these findings suggest that different cellular pathways and locations are engaged by CD40 agonists, which determines the balance between antitumor immunity and side effects. Macrophages and, specifically, liver-resident Kupffer cells are the key population that is engaged by CD40 agonist to mediate hepatotoxicity, in which neutrophils and platelets have also been implicated. Other evidence suggests that IL-6 secretion by monocytes underlies CRS induced by CD40 mAbs.
Approaches to Increase the Therapeutic Window of CD40 mAbs
Intratumoral Administration
Understanding the mechanisms driving the antitumor immunity of CD40-targeted immunotherapy, as well as those causing adverse effects, provides a rationale on how to improve the efficacy and safety profile of existing treatments. For example, the finding that the location of immune activation is associated with distinct outcomes, i.e., antitumor activity vs. systemic toxicity, advanced approaches aiming to direct CD40 agonism selectively to the tumor microenvironment (TME) to avoid toxicity. One such strategy is an intratumoral route of mAb administration (30, 46) (Figure 1). Indeed, preclinical studies demonstrated a safe profile and lack of hepatotoxicity and thrombocytopenia when anti-human CD40 mAb was administered intratumorally. Treatment resulted in T cell activation and was shown to induce abscopal effects characterized by systemic antitumor T cell activity and long-term memory response (30, 31, 46, 47). Comparison of biodistribution profiles after local or systemic anti-CD40 mAb administration in bladder cancer model revealed that local injection led to CD40 mAb accumulation in the draining lymph node and spleen, presumably because of the high density of CD40+ immune cells, whereas systemic injection led to higher Ab concentration in the liver and blood circulation (47). In an early clinical study, intratumoral administration of anti-human CD40 mAb (ADC-1013) into superficial lesions was well tolerated and was accompanied by pharmacodynamic responses (48). Another advantage of local CD40 mAb administration is the avoidance of Ab sink effect by cells with high CD40 expression, mainly circulating B cells.
Tumor-Associated Antigen-Targeted Bispecific Antibodies
While intratumoral administration is a promising approach for some patients, it is not suitable for all tumors and may be limited to patients with primary or metastatic tumors near the skin, intravesical treatment of bladder cancers, and tumors that are accessible to radiographically directed therapy. This highlights the need to reduce the toxicity of CD40-targeted immunotherapy through systemic administration. One proposed solution is a bispecific antibody (bsAb) that contains a binding arm to tumor-associated antigens (TAA). The rationale behind this approach is that the anti-TAA arm will direct the antibody to the TME and activates APCs locally, thereby avoiding systemic immune stimulation and reducing toxicity (Figure 1). The first developed CD40 bsAb is ABBV-428, which is constructed from a CD40 arm and a mesothelin TAA (49). This molecule was designed to engage the TME due to the overexpression of mesothelin by several types of tumor cells (50). Indeed, preclinical studies with ABBV-428 suggested less systemic toxicity with similar antitumor immunity compared to the parental monospecific CD40 mAb (49). In a phase 1 clinical trial, ABBV-428 showed a safe profile and the maximum tolerated dose was not reached. However, efficacy was very limited, with no signs of substantial response in patients (51). This outcome could be explained by low expression of mesothelin on tumor cells, that would limit bsAb accessibility to the tumor and thus its ability to crosslink the CD40 receptor, which is required for CD40 signaling (50). Indeed, it was shown in animal models that the expression levels of mesothelin on tumor cell lines dictates the antitumor activity of ABBV-428 (49).
The results of the ABBV-428 trial highlight the need for a bsAb targeting a more highly expressed TAA. 4224 is a CD40/EpCAM bsAb that displayed improved in vivo antitumor efficacy compared to the corresponding monospecific CD40 mAb (52). EpCAM is highly expressed on certain tumors and on tumor exosomes, which may induce cross-presentation of tumor-derived neoantigen (i.e., in exosomes or debris), resulting in better priming of tumor neoantigen-specific T cells (52). The toxic profile of 4224 compared to the parental monospecific CD40 mAb has not been reported to date.
A drawback of the CD40/TAA approach is its dependence on sufficient expression levels and density of a specific TAA, which may not be uniformly expressed across different tumor lesions and patients. This may result in variable clinical efficacy, potentially limiting the use of the compound to selective tumor types. In addition, TAA targeted by bsAbs may be required for CD40 crosslinking, similar to FcγRIIB role in neighboring cells. Therefore, their density, membrane fluidity and the binding affinity of the targeting bsAb can substantially affect the potency of these reagents. Because of these limitations, only a handful of tumor surface antigens have thus far been identified as suitable targets for CD40 bispecific antibodies.
Dendritic Cells-Targeted Bispecific Antibodies
An alternative approach that bypasses the dose-limiting toxicity is to induce CD40 agonism in a cell-specific rather than organ- or tissue-specific manner, by delivering the agonist to the cell population driving treatment efficacy but not toxicity (Figure 1). As mentioned, cDC1s mediate the antitumor immunity of CD40 mAb without the toxic side effects. Harnessing this mechanistic understanding, our group recently developed Fc-engineered CD40/DC bsAbs, e.g. CD40/CD11c and CD40/CLEC9A, which exhibit preferred binding and selective activation towards cDC1 populations. This approach improved the therapeutic window of CD40-targeted immunotherapy significantly by increasing antitumor immunity and reducing systemic toxicity in vivo in an isogenic mouse model fully humanized for CD40 and FcγRs (44, 53). Importantly, these CD40/DC bsAbs displayed reduced binding and activation of B cells, macrophages and monocytes, the cell types that contribute to sink effect, liver toxicity and CRS. Comparing the mode of action in the TME of CD40/DC bsAb vs its parental monospecific CD40 mAb reveled similar activation of effector CD4+ and CD8+ T cell response, presumably the result of similar DC engagement by these two types of agonists, leading to DC maturation, expansion and subsequent T cell priming and activation (44). While the monospecific CD40 mAb induces remodeling of the cell state of non-DC CD40+ myeloid and B cells in the TME, the DC-targeted bsAb lacks this activity. Despite the more restricted engagement of myeloid cell types in the tumor, the CD40/DC bsAb retains antitumor potency, further supporting the importance of activating the DC-T cell axis for the antitumor activity of CD40 agonists. While this effect was observed in multiple tests in transplantable syngeneic tumor models, further validation in additional tumor types and, eventually, in clinical settings is essential to evaluate the generalization and translational potential of this approach.
The monovalent nature of the CD40 arm in the CD40/DC bispecific format required special considerations in their design. First, these bsAbs exhibit increased sensitivity to FcγR-mediated crosslinking as compared to bivalent IgG formats and Fc engineering was necessary to CD40 clustering and subsequent activation. Second, the monovalent CD40 targeting arm apparently reduces CD40 binding and agonism as compared to a bivalent parental CD40 mAb. However, fine-tuning the affinities of the Fab domains to optimize the DC selectivity of CD40 agonism allows to dose-up these bsAbs without compromising their safety profile, unlike with traditional CD40 mAbs. Thus, this new tri-functional antibody format requires efficient binding to FcγRIIB, CD40, and a DC marker to result in better safety profile and superior antitumor response compared to the parental monospecific CD40 mAb (Figure 2).
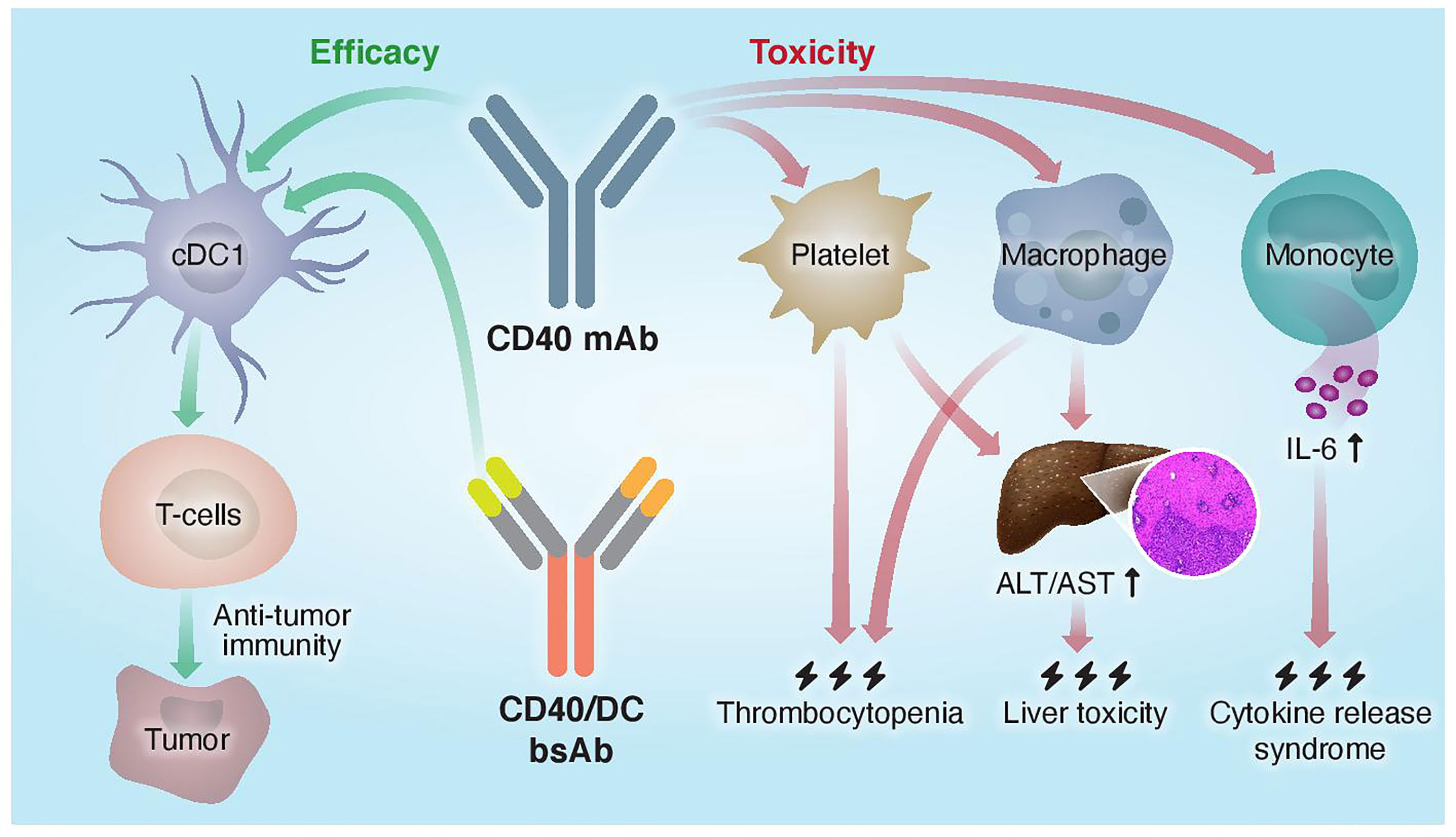
Figure 2 Proposed mechanisms of mono- and bispecific CD40 agonistic antibody activity. Traditional monoclonal anti-CD40 Abs do not distinguish between different CD40+ cells and activate both the efficacy arm, driven by cDC1s, and the toxicity arm, driven by macrophages, platelets, and monocytes. CD40/DC bsAbs, which display preferred binding to and selective activation of DC populations, improved the therapeutic window of CD40-targeted immunotherapy by increasing antitumor immunity and reducing systemic toxicity.
Discussion
Driven by recent mechanistic insights into the cellular pathways mediating efficacy and toxicity, as well as the latest developments in antibody and protein engineering, the next generation of Fc-engineered and multi-specific CD40 agonistic mAbs are being developed to bypass toxicity and optimize their potency. Multiple ongoing clinical trials evaluating the safety and potency of these new reagents, either as monotherapies or part of combination therapies, will soon reveal the potential of CD40 agonists as the next wave of immunotherapies. Besides CD40 agonists, the clinical translation of other types of agonistic mAbs has been restricted due to side effects associated with the broad expression profile of their targets on hematological and non-hematological cells. Lesson from pre-clinical and clinical studies of next-generation CD40 antibodies, together with mechanistic knowledge of the cellular pathways that mediate efficacy and toxicity, may enable the development of additional cell- and tumor-selective agonists with an improved therapeutic window.
Data Availability Statement
The original contributions presented in the study are included in the article/supplementary material. Further inquiries can be directed to the corresponding author.
Author Contributions
All authors listed have made a substantial, direct, and intellectual contribution to the work and approved it for publication.
Conflict of Interest
RD is inventor in patents covering Fc-engineered CD40 mAbs. RS and RD are inventors in a PCT patent application covering CD40 bispecific antibodies discussed in this article.
Publisher’s Note
All claims expressed in this article are solely those of the authors and do not necessarily represent those of their affiliated organizations, or those of the publisher, the editors and the reviewers. Any product that may be evaluated in this article, or claim that may be made by its manufacturer, is not guaranteed or endorsed by the publisher.
References
1. Hahn AW, Gill DM, Pal SK, Agarwal N. The Future of Immune Checkpoint Cancer Therapy After PD-1 and CTLA-4. Immunotherapy (2017) 9:681–92. doi: 10.2217/imt-2017-0024
2. Sharmea P. The Future of Immue Checkpoint Therapy. Science (2014) 348:56–61. doi: 10.1126/science.aaa8172
3. Ribas A, Wolchok JD. Cancer Immunotherapy Using Checkpoint Blockade. Science (2018). doi: 10.1126/science.aar4060
4. Topalian SL, Drake CG, Pardoll DM. Immune Checkpoint Blockade: A Common Denominator Approach to Cancer Therapy. Cancer Cell (2015) 27:451–61. doi: 10.1016/j.ccell.2015.03.001
5. Vonderheide RH. The Immune Revolution: A Case for Priming, Not Checkpoint. Cancer Cell (2018) 33:563–9. doi: 10.1016/j.ccell.2018.03.008
6. Vonderheide RH. CD40 Agonist Antibodies in Cancer Immunotherapy. Annu Rev Med (2020) 71:annurev-med-062518-045435. doi: 10.1146/annurev-med-062518-045435
7. Van Mierlo GJD, Den BAT, JP M, van der Voort EIH, Fransen MF, Offringa R, et al. CD40 Stimulation Leads to Effective Therapy of CD40- Tumors Through Induction of Strong Systemic Cytotoxic T Lymphocyte Immunity. Proc Natl Acad Sci U.S.A. (2002). doi: 10.1073/pnas.082107699
8. Luheshi NM, Coates-Ulrichsen J, Harper J, Mullins S, Sulikowski MG, Martin P, et al. Transformation of the Tumour Microenvironment by a CD40 Agonist Antibody Correlates With Improved Responses to PD-L1 Blockade in a Mouse Orthotopic Pancreatic Tumour Model. Oncotarget (2016). doi: 10.18632/oncotarget.7610
9. Byrne KT, Vonderheide RH. CD40 Stimulation Obviates Innate Sensors and Drives T Cell Immunity in Cancer. Cell Rep (2016) 15:2719–32. doi: 10.1016/j.celrep.2016.05.058
10. Caux BC, Massacrier C, Vanbervliet B, Dubois B, Van Kooten C, Durand I, et al. Sllmm~’y. CD40-CD40 Ligand. J Leuk Biol (2000) 67:2–17. doi: 10.1002/jlb.67.1.2
11. Clark EA. A Short History of the B-Cell-Associated Surface Molecule. Front Immunol (2014) 5:472. doi: 10.3389/fimmu.2014.00472
12. Alexandroff AB, Jackson AM, Paterson T, Haley JL, Ross JA, Longo DL, et al. Role for CD40-CD40 Ligand Interactions in the Immune Response to Solid Tumours. Mol Immunol (2000) 37:515–26. doi: 10.1016/S0161-5890(00)00079-1
13. Henn V, Steinbach S, Bu K, Presek P, Kroczek RA. The Inflammatory Action of CD40 Ligand ( CD154 ) Expressed on Activated Human Platelets Is Temporally Limited by Coexpressed CD40. Blood (2001) 98:1047–54. doi: 10.1182/blood.v98.4.1047
14. Yellin BMJ, Brett J, Baum D, Matsushima A, Szabolcs M, Stern D, et al. Functional Interactions ofT Cells With Endothelial Cells : The Role of CD40L-CD40-Mediated Signals. J. Exp Med (1995) 182:1857–64. doi: 10.1084/jem.182.6.1857
15. Vonderheide RH, Glennie MJ. Agonistic CD40 Antibodies and Cancer Therapy. Clin Cancer Res (2013) 19:1035–43. doi: 10.1158/1078-0432.CCR-12-2064
17. Diehl L, den Boer AT, Schoenberger SP, van der Voort EI, Schumacher TN, Melief CJ, et al. CD40 Activation In Vivo Overcomes Peptide-Induced Peripheral Cytotoxic T-Lymphocyte Tolerance and Augments Anti-Tumor Vaccine Efficacy. Nat Med (1999) 5:1–6.
18. Uno T, Takeda K, Kojima Y, Yoshizawa H, Akiba H, Mittler RS, et al. Eradication of Established Tumors in Mice by a Combination Antibody-Based Therapy. Nat Med (2006) 12:693–8. doi: 10.1038/nm1405
19. Vonderheide RH, Flaherty KT, Khalil M, Stumacher MS, Bajor DL, Hutnick NA, et al. Clinical Activity and Immune Modulation in Cancer Patients Treated With CP-870,893, A Novel CD40 Agonist Monoclonal Antibody. J Clin Oncol (2007) 25:876–83. doi: 10.1200/JCO.2006.08.3311
20. Rüter J, Antonia SJ, Burris HA, Huhn RD, Vonderheide RH. Immune Modulation With Weekly Dosing of an Agonist CD40 Antibody in a Phase I Study of Patients With Advanced Solid Tumors. Cancer Biol Ther (2010) 10:983–93. doi: 10.4161/cbt.10.10.13251
21. Furman RR, Forero-Torres A, Shustov A, Drachman JG. A Phase I Study of Dacetuzumab (SGN-40, a Humanized Anti-CD40 Monoclonal Antibody) in Patients With Chronic Lymphocytic Leukemia. Leuk Lymphoma (2010). doi: 10.3109/10428190903440946
22. Johnson PW, Steven NM, Chowdhury F, Dobbyn J, Hall E, Ashton-Key M, et al. A Cancer Research UK Phase I Study Evaluating Safety, Tolerability, and Biological Effects of Chimeric Anti-CD40 Monoclonal Antibody (MAb), Chi Lob 7/4. J Clin Oncol (2010) 28:2507. doi: 10.1200/jco.2010.28.15_suppl.2507
23. Vonderheide RH, Bajor DL, Winograd R, Evans RA, Bayne LJ, Beatty GL. CD40 Immunotherapy for Pancreatic Cancer. Cancer Immunol Immunother (2013) 62:949–54. doi: 10.1007/s00262-013-1427-5
24. Vonderheide RH, Burg JM, Mick R, Trosko JA, Li D, Shaik MN, et al. Phase I Study of the CD40 Agonist Antibody CP-870,893 Combined With Carboplatin and Paclitaxel in Patients With Advanced Solid Tumors. Oncoimmunology (2013) 2:1–10. doi: 10.4161/onci.23033
25. Dahan R, Barnhart BC, Li F, Yamniuk AP, Korman AJ, Ravetch JV. Therapeutic Activity of Agonistic, Human Anti-CD40 Monoclonal Antibodies Requires Selective Fcγr Engagement. Cancer Cell (2016) 29:820–31. doi: 10.1016/j.ccell.2016.05.001
26. Chowdhury F, Johnson PW, Glennie MJ, Williams AP. Ex Vivo Assays of Dendritic Cell Activation and Cytokine Profiles as Predictors of In Vivo Effects in an Anti-Human CD40 Monoclonal Antibody ChiLob 7/4 Phase I Trial. Cancer Immunol Res (2014) 2:229–40. doi: 10.1158/2326-6066.CIR-13-0070
27. Li F, Ravetch JV. Inhibitory Fcγ Receptor Engagement Drives Adjuvant and Anti-Tumor Activities of Agonistic CD40 Antibodies. Science (2011) 333:1034–0. doi: 10.1126/science.1206954
28. White AL, Chan HTC, Roghanian A, French RR, Mockridge CI, Tutt AL, et al. Interaction With Fc RIIB Is Critical for the Agonistic Activity of Anti-CD40 Monoclonal Antibody. J Immunol (2011) 187:1754–63. doi: 10.4049/jimmunol.1101135
29. Li F, Ravetch JV. Antitumor Activities of Agonistic Anti-TNFR Antibodies Require Differential Fcγriib Coengagement. vivo. Proc Natl Acad Sci USA (2013) 110:19506–1. doi: 10.1073/pnas.1319502110
30. Knorr DA, Dahan R, Ravetch JV. Toxicity of an Fc-Engineered Anti-CD40 Antibody is Abrogated by Intratumoral Injection and Results in Durable Antitumor Immunity [Immunology and Inflammation]. Proc Natl Acad Sci U.S.A. (2018) 115:11053–48. doi: 10.1073/pnas.1810566115
31. Garris CS, Wong JL, Ravetch JV, Knorr DA. Dendritic Cell Targeting With Fc-Enhanced CD40 Antibody Agonists Induces Durable Antitumor Immunity in Humanized Mouse Models of Bladder Cancer. Sci Transl Med (2021) 13:eabd1346.
32. Hara MHO, Reilly EMO, Varadhachary G, Wolff RA, Wainberg ZA, Ko AH, et al. CD40 Agonistic Monoclonal Antibody APX005M ( Sotigalimab ) and Chemotherapy, With or Without Nivolumab, for the Treatment of Metastatic Pancreatic Adenocarcinoma: An Open-Label, Multicentre, Phase 1b Study. Lancet Oncol (2021) 22:131–18. doi: 10.1016/S1470-2045(20)30532-5
33. O’Hara M, Mick R, Lyman J, Xu J, Hosseini M, LaVallee T, et al. A Phase 1b/2 Study of CD40 Agonistic Monoclonal Antibody (APX005M) Together With Gemcitabine and Nab-Paclitaxel With or Without Nivolumab in Untreated Metastatic Pancreatic Adenocarcinoma Patients. Cancer Res (2019) 79:CT004. doi: 10.1158/1538-7445.AM2019-CT004
34. White AL, Chan HTC, French RR, Willoughby J, Mockridge CI, Roghanian A, et al. Conformation of the Human Immunoglobulin G2 Hinge Imparts Superagonistic Properties to Immunostimulatory Anticancer Antibodies. Cancer Cell (2015) 27:138–48. doi: 10.1016/j.ccell.2014.11.001
35. Yu X, Chan HTC, Fisher H, Tews I, Glennie MJ, Cragg MS, et al. Article Isotype Switching Converts Anti-CD40 Antagonism to Agonism to Elicit Potent Antitumor Activity Isotype Switching Converts Anti-CD40 Antagonism to Agonism to Elicit Potent Antitumor Activity. Cancer Cell (2020) 37:866–50. doi: 10.1016/j.ccell.2020.04.013
36. Vonderheide RH, Dutcher JP, Anderson JE, Eckhardt SG, Stephans KF, Razvillas B, et al. Phase I Study of Recombinant Human CD40 Ligand in Cancer Patients. J Clin Oncol (2001) 19:3280–7. doi: 10.1200/JCO.2001.19.13.3280
37. Divine R, Dang HV, Ueda G, Fallas JA, Vulovic I, Sheffler W, et al. Designed Proteins Assemble Antibodies Into Modular Nanocages. Sci (80- ) (2021) 372:1–32. doi: 10.1126/science.abd9994
38. Liu X, Zhao Y, Shi H, Zhang Y, Yin X, He Y, et al. Antitumour Activities Through Biophysical Fl Exibility. Nat Commun (2019) 10:4206. doi: 10.1038/s41467-019-12097-6
39. Johnson P, Challis R, Chowdhury F, Gao Y, Harvey M, Geldart T, et al. Clinical and Biological Effects of an Agonist Anti-CD40 Antibody a Cancer Research UK Phase I Study. Clin Cancer Res (2015) 21:1328–21. doi: 10.1158/1078-0432.CCR-14-2355
40. Berner V, Liu H, Zhou Q, Alderson KL, Sun K, Weiss JM, et al. IFN-γ Mediates CD4+ T-Cell Loss and Impairs Secondary Antitumor Responses After Successful Initial Immunotherapy. Nat Med (2007) 13:3–6. doi: 10.1038/nm1554
41. Chiodoni C, Iezzi M, Guiducci C, Sangaletti S, Alessandrini I, Ratti C, et al. Triggering CD40 on Endothelial Cells Contributes to Tumor Growth. J Exp Med (2006) 203:2441–50. doi: 10.1084/jem.20060844
42. Siwicki M, Gort-freitas NA, Messemaker M, Bill R, Gungabeesoon J, Engblom C, et al. Resident Kupffer Cells and Neutrophils Drive Liver Toxicity in Cancer Immunotherapy. Sci Immunol (2021) 6:eabi7083. doi: 10.1126/sciimmunol.abi7083
43. Bonnans C, Thomas G, He W, Jung B, Chen W, Liao M, et al. CD40 Agonist- Induced IL- 12p40 Potentiates Hepatotoxicity. J Immunother Cancer (2020) 8:e000624. doi: 10.1136/jitc-2020-000624
44. Salomon R, Rotem H, Katzenelenbogen Y, Weiner A, Saban NC, Feferman T, et al. Bispecific Antibodies Increase the Therapeutic Window of CD40 Agonists Through Selective Dendritic Cell Targeting. Nat Cancer (2022) 3:287–302. doi: 10.1038/s43018-022-00329-6
45. Ferris ST, Durai V, Wu R, Theisen DJ, Ward JP, Bern MD, et al. Cdc1 Prime and are Licensed by CD4 + T Cells to Induce Anti-Tumour Immunity. Nature (2020) 584:629–4. doi: 10.1038/s41586-020-2611-3
46. Fransen MF, Sluijter M, Morreau H, Arens R, Melief CJM. Local Activation of CD8 T Cells and Systemic Tumor Eradication Without Toxicity via Slow Release and Local Delivery of Agonistic CD40 Antibody. Clin Cancer Res (2011) 17:2280–70. doi: 10.1158/1078-0432.CCR-10-2888
47. Sandin LC, Orlova A, Gustafsson E, Ellmark P, Tolmachev V, Tötterman TH, et al. Locally Delivered CD40 Agonist Antibody Accumulates in Secondary Lymphoid Organs and Eradicates Experimental Disseminated Bladder Cancer. Cancer Immunol Res (2014) 2:90–80. doi: 10.1158/2326-6066.CIR-13-0067
48. Irenaeus SMM, Nielsen D, Ellmark P, Yachnin J, Deronic A, Nilsson A, et al. First-In-Human Study With Intratumoral Administration of a CD40 Agonistic Antibody, ADC-1013, in Advanced Solid Malignancies. Int J Cancer (2019) 145:1189–99. doi: 10.1002/ijc.32141
49. Ye S, Cohen D, Belmar NA, Choi D, Tan SS, Sho M, et al. A Bispecific Molecule Targeting CD40 and Tumor Antigen Mesothelin Enhances Tumor-Specific Immunity. Cancer Immunol Res (2019) 7:1864–75. doi: 10.1158/2326-6066.CIR-18-0805
50. Bano JD, Florès-Florès R, Josselin E, Goubard A, Ganier L, Castellano R, et al. A Bispecific Antibody-Based Approach for Targeting Mesothelin in Triple Negative Breast Cancer. Front Immunol (2019) 10:1593. doi: 10.3389/fimmu.2019.01593
51. Luke JJ, Barlesi F, Chung K, Tolcher AW, Kelly K, Hollebecque A, et al. Phase I Study of ABBV-428, a Mesothelin-CD40 Bispecific, in Patients With Advanced Solid Tumors. J Immunother Cancer (2021) 9:1–10. doi: 10.1136/jitc-2020-002015
52. Ellmark P, Hägerbrand K, Levin M, Von Schantz L, Deronic A, Vara L, et al. A Bispecific Antibody Targeting CD40 and EPCAM Induces Superior Anti-Tumor Effects Compered to the Combination of the Monospecific Antibodies. Nuevos Sist Comun e Inf (2021) 8:A1–A559. doi: 10.1136/jitc-2020-SITC2020.0858
Keywords: CD40, fc receptor, agonistic antibody, therapeutic antibody, cancer immunotherapy, bispecific antibodies (BsAbs)
Citation: Salomon R and Dahan R (2022) Next Generation CD40 Agonistic Antibodies for Cancer Immunotherapy. Front. Immunol. 13:940674. doi: 10.3389/fimmu.2022.940674
Received: 10 May 2022; Accepted: 21 June 2022;
Published: 13 July 2022.
Edited by:
Peter Boross, Genmab, NetherlandsReviewed by:
Ramon Arens, Leiden University Medical Center, NetherlandsSara Mangsbo, Uppsala University, Sweden
Fubin Li, Shanghai Jiao Tong University, China
Copyright © 2022 Salomon and Dahan. This is an open-access article distributed under the terms of the Creative Commons Attribution License (CC BY). The use, distribution or reproduction in other forums is permitted, provided the original author(s) and the copyright owner(s) are credited and that the original publication in this journal is cited, in accordance with accepted academic practice. No use, distribution or reproduction is permitted which does not comply with these terms.
*Correspondence: Rony Dahan, cm9ueS5kYWhhbkB3ZWl6bWFubi5hYy5pbA==