- 1School and Hospital of Stomatology, China Medical University, Shenyang, China
- 2Liaoning Provincial Key Laboratory of Oral Diseases, China Medical University, Shenyang, China
- 3Department of Orthodontics, Shenyang Stomatological Hospital, Shenyang, China
- 4College of Life and Health Sciences, Northeastern University, Shenyang, China
- 5Shenyang National Laboratory for Materials Science, Northeastern University, Shenyang, China
Implant surgery is followed by a series of inflammatory reactions that directly affect its postoperative results. The inflammasome plays a vital role in the inflammatory response by inducing pyroptosis and producing interleukin-1β, which plays a critical role in inflammation and tissue damage. Therefore, it is essential to study the activation of the inflammasome in the bone healing process after implant surgery. As metals are the primary implant materials, metal-induced local inflammatory reactions have received significant attention, and there has been more and more research on the activation of the NLRP3 (NOD-like receptor protein-3) inflammasome caused by these metals. In this review, we consolidate the basic knowledge on the NLRP3 inflammasome structures, the present knowledge on the mechanisms of NLRP3 inflammasome activation, and the studies of metal-induced NLRP3 inflammasome activation.
1 Introduction
Implantation has been widely used in orthopedic and dental treatments to replace the non-regenerative part of the human body (1, 2). Surgical implantation is accompanied by hemorrhage, which causes various cells and proteins between the bone and surrounding soft tissue to participate in a series of biological processes, including protein deposition, coagulation, inflammation, and tissue formation (3). Leukocytes migrate into the peri-implant site, and the activation of leukocytes results in the release of inflammatory mediators, including interleukin-1 beta (IL-1β), IL-6, tumor necrosis factor alpha (TNF-α), and macrophage colony-stimulating factor (3). The initial reaction of inflammatory cells to foreign materials influences the bone healing process. Thus, it is essential to study biomaterial-induced inflammation in the osteogenesis field (4). The inflammasome is an essential part of the innate immune system that is involved in metal-induced hypersensitivity, bacterial infection-induced peri-implantitis, and other possible side effects of implantation (5, 6). The activation and assembly of the inflammasome are complicated programmed processes that involve the upstream sensors, the adaptors, and the downstream effectors (7). Therefore, studies on implantation-induced inflammasome activation have constantly increased over the years.
Broadly, biomaterials are grouped into natural precursors or synthetic materials (8, 9). Suitable implant materials must satisfy the biochemical, physiological, and antibacterial property requirements of implantations. The implant surface chemistry and topography influence the process of osteogenesis (10). There are two main methods to improve osseointegration: 1) sintering of the metallic beads or fibers over the implant surface and 2) plasma spray deposition of the metallics or ceramics onto the implant surface (3). Metal implants apply load-bearing sections, such as in long bone and dental implants. Metal implantation can constantly precipitate metal ions, which are components of cellular proteins, bone structures, and intracellular or extracellular matrices (11). In addition, the release of metal ions into the surrounding bone tissues participates in osseointegration, which has been used to describe the successful healing of an implant within a host bone (12).
Peri-implant tissue healing starts with an inflammatory response after the implant is inserted into the bone cavity (13); however, inflammation at the peri-implantation site is also the leading cause of implantation failure (14). Therefore, it is essential to study the immune response to implant biomaterials. However, the role of implant-released metal ions in inflammasome activation, a critical component of the host immune system, is still unclear. Recent studies on metal-activated inflammasomes have mainly focused on the NOD-like receptor protein-3 (NLRP3) inflammasome. In this review, we first present an overview of the series of NLRP3 inflammasome activation mechanisms and then summarize recently published research on various metal ions, metal particles, and metalloproteins that activate the NLRP3 inflammasome signals.
2 NLRP3 inflammasome
The inflammasome, identified by Tschopp and co-workers in 2002, is described as a high-molecular-weight complex present in the cytosol of stimulated immune cells that mediates the activation of inflammatory caspases (15). The activated caspases convert IL-1β and IL-18 from their inactive to their active forms. At the same time, full-length gasdermin D (FL-GSDMD) is cleaved to the N-terminal GSDMD (GSDMD-NT) and forms membrane pores, resulting in cytokine release and/or programmed cell death called pyroptosis (16, 17). The inflammasomes are named after the pattern recognition receptors (PRRs) that sense the pathogen-associated molecular patterns (PAMPs) and the damage-associated molecular patterns (DAMPs) in the cytosol and initiate downstream responses. In general, inflammasomes are classified into canonical and non-canonical inflammasomes.
Canonical inflammasomes comprise a sensor molecule, the adaptor ASC (apoptosis-associated speck-like protein containing a C-terminal caspase recruitment domain (CARD), and the effector caspase-1. Several sensors have been identified. There are sensors that consist of the nucleotide-binding oligomerization domain (NOD) and leucine-rich repeat (LRR)-containing protein (NLR) family members, including NLRP1, NLRP3, NLRC4, NLRP6, NLRP7, and NLRP12. These sensors, which activate inflammasomes, are classified as NLR N-terminal domains. Another class of inflammasomes assembling sensors is represented by the PYHIN protein family members, such as melanoma 2 (AIM2) and pyrin (7). The ASC adaptor contains two death-fold domains: one pyrin domain (PYD) and one caspase recruitment domain (CARD). On the one hand, PYD forms PYD–PYD interactions with the PYD domain in activated sensors. On the other hand, the CARD domain interacts with the CARD domain in pro-caspase-1. Therefore, ASC serves as a bridge between the sensors and pro-caspase-1, forming inflammasomes as a result of recognition of the PAMPs and DAMPs in canonical inflammasomes (6).
The NLRP3 inflammasome plays critical roles in various inflammatory disorders, including Alzheimer’s disease, diabetes, and other inflammation-related diseases (18–20). It consists of an N-terminal PYD, a central NOD (also called the NACHT domain), and a C-terminal LRR domain (21). The ATPase activity of NOD is essential for NLRP3 oligomerization and is targeted by MCC950, a commonly used NLRP3 inhibitor (22). In addition, it has been determined that the NIMA-related kinase 7 (NEK7) interacts with the LRR domain to promote the activation of NLRP3 (23). The structure of the NLRP3 inflammasome is shown in Figure 1.
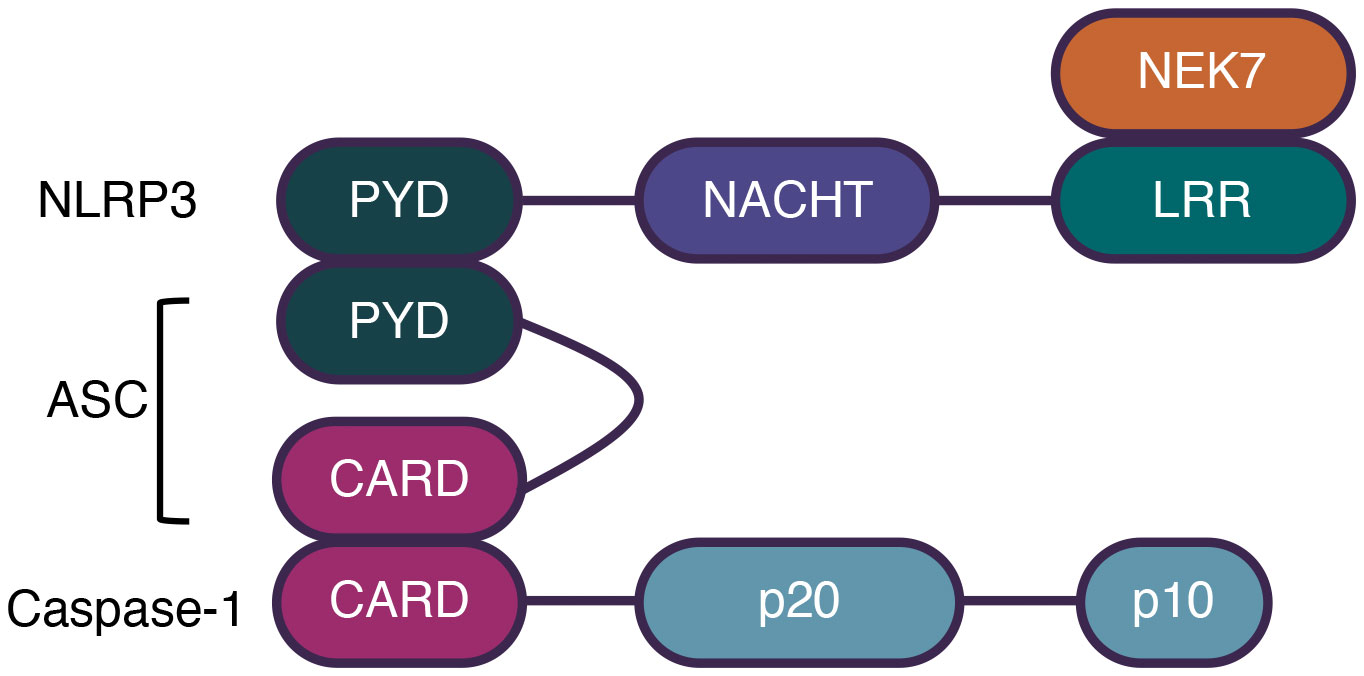
Figure 1 The structure of NLRP3 inflammasome. Canonical inflammasomes comprise a sensor molecule, the adaptor ASC, and the effector Caspase-1. NLRP3 comprises a N-terminal PYD, a central NOD, and a C-terminal LRR domain. NEK7 interacts with LRR domain to promote NLRP3 activation. ASC comprises a PYD and a CARD domain. Caspase-1 comprises a CARD and two subunits, p10 and p20. Sensors interact with ASC by PYD domains and ASC interacts with caspases-1 by CARD domains.
The NLRP3 inflammasome has been extensively studied for its broad spectrum of stimuli and complicated activation signaling. The stimulators of NLRP3 include crystalline materials, extracellular ATP, pore-forming toxins, RNA–DNA hybrids, peptide aggregates, and viral, fungal, and bacterial pathogens (6, 24). It is generally accepted that the activation of the NLRP3 inflammasome involves a two-step process. Signal 1, also known as the priming signal, is triggered by PRR signaling, such as Toll-like receptors (TLRs) or cytokine receptors, e.g., TNF and IL-1 receptors. The activation of signal 1 leads to the transcriptional activation of nuclear factor kappa B (NF-κB), which upregulates the gene expression of NLRP3, IL-1β, and IL-18. Signal 2, also known as the activation signal, is induced by various PAMPs and DAMPs. Multiple molecular and cellular signaling events have been proposed to activate the NLRP3 inflammasome, as follows: 1) ionic flux, including K+ efflux, Ca2+ mobilization, Cl− efflux, and Na+ influx. As a DAMP, ATP activates the P2X7 receptor on the cell membrane, inducing K+ efflux that triggers the activation signal; 2) mitochondrial dysfunction and the production of reactive oxygen species (ROS); and 3) lysosome damage. However, none of these is recognized as a common event induced by all the NLRP3 inflammasome stimuli (21, 25). To date, studies on metal-activated inflammasomes have mainly focused on the NLRP3 inflammasome (26, 27). Details of these studies are discussed in the following sections and are summarized in Figure 2.
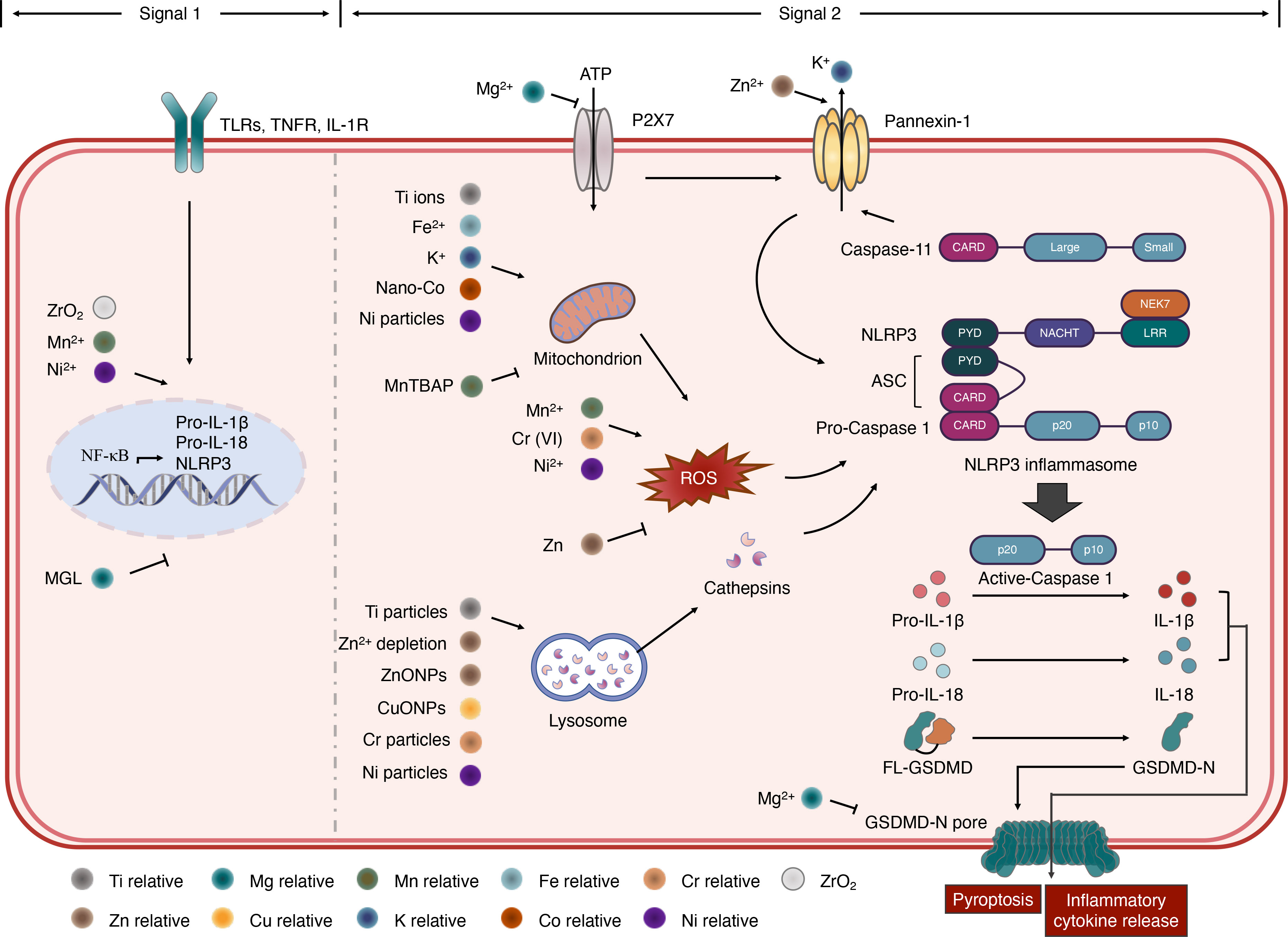
Figure 2 Roles of metals in NLRP3 inflammasome activation. NLRP3 inflammasome activation requires a two-step process. In signal 1, NF-κB transcriptional activation can be accelerated by Mn2+ binding prion fibrils, Ni2+ ions, ZrO2 and inhibited by magnesium isoglycyrrhizinate (MGL). In signal 2, ionic flux activating NLRP3 inflammasome is controlled by the P2X7 and pannexin-1 receptors. The pannexin-1 activity can be regulated by Zn2+. Mg2+ can inhibit the ATP-gated Ca2+ channel P2X7 and limits the oligomerization and membrane localization of GSDMD-NT, which form the GSDMD-N pore. In addition, caspase-11 cleave pannexin-1, resulting in K+ efflux and NLRP3 inflammasome activation. Mitochondrion dysfunction and ROS production can induce NLRP3 inflammasome activation. Ti ions, Fe2+, K+, Nano-Co and Ni particles promote this process, while MnTBAP inhibits it. Zinc can attenuate ROS production while Mn2+, Cr (VI) and Ni2+ induce the release of ROS, triggering NLRP3 inflammasome. Ti particles, Zn2+ depletion, ZnONPs, CuONPs, Cr pariticles and Ni particles can induce lysosome damage, which induced cathepsins release and result in NLRP3 inflammasome activation.
3 Metals involved in NLRP3 inflammasome activation
As metals are the primary implant materials, metal-induced local inflammatory reactions have received significant attention, and there has been more and more research on the activation of inflammasomes caused by metals (28, 29). One of the present trends of biomaterials is the development of strategies and solutions that modulate the immune cell biology to avoid or minimize the undesired side effects in regenerative medicine (30). In the host response to biomaterials, the inflammasome is the bridge between inflammation and tissue regeneration. As the widest studied inflammasome, the NLRP3 inflammasome has become a major topic in the development of immunomodulatory biomaterials. Modulation of NLRP3 inflammasome activation in response to biomaterials in order to minimize the tissue inflammatory response to implants can promote tissue regeneration around the implant and determine the outcomes post-implantation (31). The reaction and mechanisms of metals activating the NLRP3 inflammasome depend on the different metal elements and metal states. We summarize recent studies on the roles of metals in NLRP3 inflammasome activation in Table 1. Details of specific metals are discussed as follows.
3.1 Titanium
Titanium (Ti) and its alloys have recently attracted significant interest (75). They are the most widely used metals for implantation due to their advantageous characteristics, including their excellent corrosion resistance and bone-bonding ability (76). The interface between metals and the surrounding tissues is also critical as insufficient bonding provides a bacterial invasion route, resulting in peri-implantitis. Titanium ions are frequently detected in the implant region, especially in peri-implantitis tissue (75). It has been reported that Ti ions can promote the production of ROS, and N-acetyl-l-cysteine (NAC), a ROS scavenger, decreased the Ti ion-induced NLRP3 gene expression and IL-1β release, suggesting that Ti ions activated the NLRP3 inflammasome in an ROS-dependent manner (32).
However, research has shown that Ti ions alone cannot stimulate the transcription of the inflammasome components, but they form particles that stimulate inflammasome activation and, consequently, IL-1β release (77). It is widely accepted that Ti particles can activate the NLRP3 inflammasome (78, 79). However, the mechanisms of Ti-induced inflammasome activation in inflammatory diseases are still controversial. Several studies have suggested that Ti particle-induced activation of the NLRP3 inflammasome is dependent on lysosomes and lysosomal cathepsins. St. Pierre et al. found that the macrophage uptake of Ti particles was cathepsin B-dependent and induced acute inflammation by activating the NLRP3 inflammasome, resulting in IL-1β release and neutrophil recruitment (33). In a rat peri-implant osteolysis model, Ti particles induced NLRP3 inflammasome activation depending on mitochondrial function. Sirtuin 3, an NAD+-dependent deacetylase of the mitochondria that regulates its function, suppressed the Ti particle-induced NLRP3 inflammasome activation via the GSK-3β/β-catenin pathway (35). In addition, Ti particles can also induce cell death. However, NLRP3 and gasdermin D did not participate in the cell death process, suggesting that the Ti particle-induced cell death was not pyroptosis (80). An in vitro study also showed that Ti particles alone were insufficient at inducing the IL-1β release in macrophages; an additional priming signal, such as bacterial lipopolysaccharide (LPS), was required to enable inflammasome activation (63). Therefore, reducing the amount of particles produced in the process of implant surgery and application is critical to suppressing the activation of the NLRP3 inflammasome.
3.2 Zinc
Nearly 90% of zinc (Zn) is found in muscles and bones. Zn has a stimulatory effect on bone metabolism and the ability to promote bone formation and mineralization (81). It is accepted that the immune system is regulated by Zn homeostasis, and Zn2+ functions as a second passenger in innate immunity (27). Zn homeostasis is maintained by the Zn2+ transporter proteins, including SLC30A (ZnT) and SLC39A (ZIP, Zir/Irt-like proteins) (82). The effects of Zn in NLRP3 inflammasome activation have been proven in series studies. Brough et al. explored pretreating the macrophage with N,N,N′,N′-tetrakis(2-pyridylmethyl)ethylenediamine (TPEN), the Zn chelator, and found significant inhibition of the activity of pannexin-1, thus suppressing the activation of the NLRP3 inflammasome and decreasing the production of IL-1β (36). Pretreatment of primary mouse macrophages with TPEN also damaged the integrity of the lysosome, thus suppressing the NLRP3 inflammasome activation and IL-1β secretion (37). In addition, Zn can inhibit high glucose-induced NLRP3 inflammasome activation in peritoneal mesothelial cells by attenuating ROS production (38). Zn also played a neuroprotective role by suppressing NLRP3 inflammasome activation through autophagy and ubiquitination in an experimental spinal cord injury model (39, 83). Another study also showed that Zn suppressed NLRP3 activation by inducing microglia autophagy and played a neuroprotective role in spinal cord injury (40). Moreover, Zn participated in LPS and hypoxia inducing NLRP3 inflammasome activation in the microglia (41). Zinc oxide nanoparticles (ZnONPs) can suppress the NLRP3 inflammasome using the NLRP3 inflammasome–autophagy–exosomal pathway (42).
3.3 Magnesium
Magnesium (Mg) is a degradable and absorbable biomaterial. It is widely used in the clinic as its structural and mechanical characteristics are similar to those of the trabecular bone, which is beneficial to obtaining early fixation (84). Mg homeostasis in cells is maintained by Mg channels and transporters, including Mrs2, SLC41A1, SLC41A2, and TRPM6 (82).
It has been reported that Mg ions can suppress both the canonical and non-canonical pyroptotic pathways in macrophages by inhibiting the ATP-gated Ca2+ channel P2X7 and limiting the oligomerization and membrane localization of GSDMD-NT, thus blocking the GSDMD-NT-induced pyroptosis (43). In addition, magnesium isoglycyrrhizinate (MGL), a new stereoisomer of glycyrrhizic acid, performs immune modulation through its anti-inflammatory effect and is clinically used as a hepatoprotective agent for the treatment of liver diseases. The anti-inflammatory effects of MGL may involve suppressing the activation of inflammasomes (44, 45, 85). MGL inhibited both NF-κB activation and NLRP3 inflammasome formation, thus alleviating liver inflammation in fructose-fed rats with metabolic syndrome (44). MGL was also used to treat chronic obstructive pulmonary disease by suppressing NLRP3 and cleaving caspase-1 expression (85). In addition, MGL administration can decrease the expression of NLRP3, NLRP6, and caspase-3 in mice, suggesting its downregulatory inflammasome expression effect in liver tissue (45).
3.4 Copper
Copper (Cu), an indispensable trace element in organisms, plays a crucial role in a lot of physiological activities, including respiration, iron metabolism, antioxidant activity, and tissue integrity (86). It is well known that the addition of Cu can endow biomaterials with antibacterial properties, osteogenesis, and angiogenesis (87). In organisms, Cu exists in two states: Cu(I)/Cu+ (cuprous ion) and Cu(II)/Cu2+ (cupric ion). Cu2+ is the predominant redox state in blood, whereas Cu+ is the form found in the reducing environment of the cell cytosol (88). Cu2+ can be reduced to Cu+ through the plasma membrane reductase of the STEAP (six-transmembrane epithelial antigen of prostate) family or DCYTB (duodenal cytochrome B) and then transported into cells by CTR1 (copper transporter 1) or DMT1 (divalent metal ion transporter 1), which are located in the membrane (89). In addition, proteins called metallochaperones also distribute Cu to specific sites in cells (90).
The dyshomeostasis of Cu has been reported to trigger inflammasome activation. Metal chelators remove metal ions from the body, reducing the metal concentration. Tetrathiomolybdate (TTM) is a highly specific, clinically approved Cu chelator (91) that can be used as an anti-inflammatory agent to prevent LPS-induced inflammatory reactions in vivo. Deigendesch et al. showed that TTM could prevent the activation of NLRP3 by removing Cu from the active site of superoxide dismutase 1 (SOD1) in macrophages. This regulation targets macrophages, not monocytes, in both mice and humans (46). In addition, TTM did not block the NF-κB and mitogen-activated protein kinase (MAPK) pathways or the other major antimicrobial inflammasomes such as NLRC4, NLRP1, and AIM2 (44). In vivo, depletion of bioavailable Cu led to a decreased caspase-1-dependent inflammation and reduced the susceptibility to LPS-induced endotoxic shock (44). Exposure to copper oxide nanoparticles (CuONPs) also resulted in NLRP3 activation by inducing lysosomal damage and the release of cathepsin B. Moreover, after lysosomal deposition, CuONPs released Cu2+ due to the acidic environment of lysosomes. Cu2+ then induced cellular oxidative stress and further mediated the activation of the NLRP3 inflammasome (47).
3.5 Iron
Iron (Fe) is involved in many critical biological processes, such as oxygen transport, ATP generation, and DNA biosynthesis. With regard to tissue engineering, Fe has excellent mechanical properties, making it a good candidate for implants requiring high structural strength, such as bone defect repair and vascular stents (92). Fe has redox activity, and a high Fe level can induce ROS, leading to oxidative stress and signal pathways critical to cell survival and death (93). The homeostasis of Fe metabolism in the human body is strictly regulated by a variety of proteins, including, among others, ferritin (FTH1 and FTL), a protein complex that stores Fe in cells for future use; transferrin (TF), an Fe-binding serum protein; transferrin receptor 1 (TfR1, TFRC), a plasma membrane protein that allows cells to ingest transferrin; divalent metal transporter 1 (DMT1, SLC11A2), a critical metal transporter for TfR1-mediated Fe uptake and dietary Fe absorption; and ferroportin (Fpn, SLC40A1), the only known cell Fe efflux pump (94).
The multi-target Fe chelator M30 has an Fe chelating/free radical scavenging effect. It inhibits lipid peroxidation, which has been proven to inhibit oxidative stress and inflammation in many diseases, such as type 2 diabetes and Alzheimer’s disease. In an in vitro ethanol-induced hepatocyte injury model, M30 inhibited the activation of the AC/cAMP/PKA/HIF-1/NLRP3 inflammasome pathway, ameliorated oxidative cell stress, and reduced cell damage (48). Fe2+-specific chelators can also rescue peripheral blood mononuclear cells from an LPS stimulation-induced Fe2+ increase following an Fe2+ dose-dependent IL-1β production, which results from NLRP3 inflammasome activation (49). Gelfand et al. found that an Fe overload caused retinal degeneration by enhancing the stability of Alu RNAs, thereby promoting retinal pigmented epithelium (RPE) degeneration, thus inducing NLRP3 inflammasome activation (95). Moreover, Liu et al. explored how morphology affects the NLRP3 inflammasome-activating property of iron oxide nanoparticles (IONPs). Research indicates that morphology is a critical determinant of IONP-induced IL-1β release and pyroptosis, and this process is not all mediated by NLRP3 (96).
3.6 Manganese
Manganese (Mn) is an essential metal required for proper immune function, regulation of blood sugar and cellular energy, reproduction, digestion, bone growth, blood coagulation and hemostasis, and defense against ROS (27). Mn is used as an additive of biomaterials because of its advantages in stabilizing and promoting osteoblast differentiation and bone metabolism (97). In biological systems, Mn exists in two oxidation states, Mn2+ and Mn3+, which mediate the redox cycling of Mn and are involved in the biological effects of metals, including the Fenton reaction, transferrin-mediated transport, and interference, as well as interference with other divalent metals (98). In addition, Mn forms various Mn-dependent enzymes, including oxidoreductases, isomerases, transferases, ligases, lyases, and hydrolases (27). Mn2+ is also an essential component of some metalloenzymes, such as SOD, glutamate synthetase, pyruvate carboxylase, arginase, hydrolases, phosphatases, transferases, dehydrogenases, kinases, peptidases, and decarboxylases (99). However, excessive levels of Mn can cause cellular toxicity, which leads to oxidative stress, genotoxicity, membrane perturbation, and protein dysfunction by catalyzing the conversion of hydrogen peroxide (H2O2) into oxygen radical species via the Fenton reaction (99). There are several Mn2+ importers in the plasma membrane, including SLC39A14, SLC39A8, SLC30A10, and DMT1. In addition, NRAMP1 transports Mn2+ from the phagosome to the cytoplasm (99, 100).
Excessive Mn exposure can activate the NLRP3 inflammasome in the microglia, the principal central nervous system (CNS) immune cells, and may result in neurodegenerative disorders. Mn2+ activated the NLRP3 inflammasome in the striatum of adult rats and induced the release of ROS, triggering the NLRP3 inflammasome (50). In addition, Mn exposure also resulted in mitochondrial defects that drove the NLRP3 inflammasome signal amplification and propagation and the exosomal release of ASC in microglial cells (51). Another study also showed that Mn2+ binding on prion fibrils was critical to inducing the priming signal in NLRP3 inflammasome activation in the microglia (52). Sodium para-aminosalicylic acid inhibited Mn-induced NLRP3 inflammasome by inhibiting NF-κB activation and oxidative stress in the microglia (101, 102). On the other hand, the SIRT3 activating enzyme manganese superoxide dismutase (MnSOD) in the mitochondria significantly enhanced the ability to scavenge ROS and suppressed the activation of the NLRP3 inflammasome to protect the heart against oxidative stress (53). Anakinra, the recombinant form of the IL-1 receptor antagonist, dampened the NLRP3 activity by increasing the MnSOD protein longevity (103). Manganese tetrakis porphyrin chloride (MnTBAP), a mitochondrial SOD2 mimic, suppressed NLRP3 inflammasome activation by blocking the albumin-induced mitochondrial dysfunction in renal tubular injury (54). MnTE-2-PyP, another artificial mitochondrial SOD2 mimic, was also proven to suppress the pulmonary hypertension-induced NLRP3 inflammasome activation in macrophages (55). Mn-TAT PTD-Ngb, an artificial metalloprotein containing a TAT protein transduction domain (TAT PTD), which was recombined from apo-neuroglobin (apo-Ngb) with Mn porphyrin, suppressed both NF-κB and ROS-NLRP3 inflammasome activation in the microglia (27, 57).
To date, the role of Mn in NLRP3 inflammasome activation has been examined mainly in relation to the brain neuroscience field. The effect of Mn and the Mn-dependent enzymes on NLRP3 inflammasome activation in the bone healing process needs further exploration, which is beneficial to the application of Mn in bone tissue engineering.
3.7 Potassium
Potassium (K) is an essential element in the human body. As a monovalent cation, the potassium ion directly controls other ionic signaling pathways by regulating the membrane potential. In addition, the balance between the intracellular and extracellular fluids is maintained by Na+/K+ ATPase, which pumps sodium ion (Na+) to extra cells while it uptakes K+ into the cell in reverse concentration gradients (27, 104).
Activating the NLRP3 inflammasome inevitably leads to potassium efflux, which can also induce NLRP3 inflammasome activation. The molecular mechanisms underlying NLRP3 inflammasome activation through potassium efflux have been extensively investigated. In this review, we only briefly summarized the main mechanisms. For details of the studies, please refer to an elegant review published previously (59). Two potential mechanisms are acknowledged. Potassium efflux may be related to the interaction of NLRP3–NEK7, which is essential to the activation of the NLRP3 inflammasome (58). Another theory is that potassium efflux might promote NLRP3 inflammasome activation by inducing mitochondrial dysfunction and mitochondrial ROS (mtROS) production (105). P2X7 has been reported to be expressed in immune cells such as macrophages, lymphocytes, mast cells, and neutrophils. P2X7 appears to play a critical role in inflammation and autoimmune diseases (82). Extracellular ATP can activate the P2X7 receptor and lead to the activation of the NLRP3 inflammasome. Furthermore, it has also been reported that the non-canonical inflammasome caspase-11 can cleave pannexin-1, followed by ATP release, P2X7 receptor activation, potassium efflux, and NLRP3 inflammasome activation (60). Another non-canonical inflammasome activating the NLRP3 inflammasome mechanism is potassium efflux through the GSDMD-NT-forming pores induced by non-canonical inflammasomes, which further activates the NLRP3 inflammasome (61). The two-pore domain potassium (K2P) is responsible for maintenance of the resting membrane potential in almost all cells. It has been suggested to cooperate with the P2X7 receptor mechanistically (59).
3.8 Cobalt
Cobalt (Co) is essential for humans in the form of cobalamin (coenzyme B12), which is tightly bound to a corrin ring and serves as a methyl group carrier with Co oxidation states (106). Co-based alloys are considered one of the most successful materials used for implants as they have satisfactory corrosion, wear, and mechanical properties (107). The homeostasis of Co is maintained by Co2+ transporter proteins, including CbiMNQO, NiCoT, HupE/UreJ, CorA, and TBDT. Human serum albumin is considered the primary transporter of Co2+ in the blood (108). Although humans are exposed to Co2+, the most stable form under ambient conditions, in the course of normal nutrition (109), a high level of Co2+ is toxic to cells (104). Co2+ has also been reported to induce an immune response by stimulating TLR4 (110).
Exposure to high levels of CoCl2 significantly increased the NLRP3 expression, caspase-1 activity, and IL-1β secretion (64). Although a high CoCl2 level can induce apoptosis in T lymphocytes, CoCl2-treated monocytes did not undergo apoptosis as the effect of p53 was counteracted by the anti-apoptotic activity of the activation of NF-κB and the inflammasome danger signaling pathway leading to the production of pro-inflammatory cytokines (111). However, a study demonstrated that CoCl2-induced hypoxia may negatively regulate the NLRP3 inflammasome signaling in brain glial cells (65). Feng et al. found that exposure to Co nanoparticles (Nano-Co) promoted intracellular oxidative stress damage and mtROS, which activated the NLRP3 inflammasome in hepatocytes, suggesting an essential role of the ROS/NLRP3 pathway in Nano-Co-induced hepatotoxicity (66). A study also showed that hemin and cobalt protoporphyrin (CoPP) inhibited NLRP3 inflammasome assembly by reducing the amount of intracellular ASC in cultured macrophages (67).
3.9 Chromium
Chromium (Cr), which belongs to the group of trace elements, exists in many different oxidation states in the environment and is essential in numerous functions of the human body. Cr deficiency can cause various physical dysfunctions, while exposure to Cr at higher concentrations is also toxic and may lead to neoplastic diseases. The Co–Cr–Mo alloy is the most widely used combination of Co-based alloys due to its unique combination of strength and ductility (107). Cr(VI) and Cr(III) are the most stable forms of Cr. Cr(VI), the most cancer-related among all Cr oxidation states, enters cells through the sulfate anion transporter system and is reduced to the intermediate oxidation states, e.g., Cr(V) and Cr(IV), in the process of forming stable Cr(III) forms (112).
Adam et al. found the indirect effects of Cr(VI) compounds in pro-inflammation activation. Cr(VI) compounds can induce NLRP3 inflammasome activation and IL-1β production, amplifying the innate immune activation inflammatory response. The authors also confirmed the production of mtROS upstream of the NLRP3 inflammasome assembly by treatment with NAC, suggesting that Cr(VI) induces the production of mtROS and thus activates the NLRP3 inflammasome. In addition, the Cr(III) compounds were also examined. However, the Cr(III) compounds failed to induce these reactions in cells, suggesting that oxidation state-specific differences in mitochondrial reactivity may determine the activation of the inflammasome (62). Jämsen et al. observed that Cr particles alone were insufficient to induce NLRP3 inflammasome activation. Priming human primary macrophages with LPS and exposing the cells to Cr particles were discovered to induce the production of IL-1β, which was significantly reduced by the NLRP3 inflammasome or cathepsin B inhibitor, suggesting that Cr-induced NLRP3 activation is lysosome/cathepsin B-dependent (63). In addition, Cr3+ can activate both priming signaling and activation signaling of the NLRP3 inflammasome by inducing ROS accumulation (28).
3.10 Nickel
Nickel (Ni) is an abundant element in the earth’s core and is a commonly used implant material as it grants necessary strength and durability to the implant. However, it is also associated with metal hypersensitivity reactions and can be found in trace amounts in “commercially pure” Ti materials used in surgical implants (113, 114). The uptake of Ni has a toxic effect on cell metabolism and physiology in humans. The toxicity of Ni is dependent on the solubility of the Ni compounds. Insoluble Ni compounds are phagocytosed by cells, while Ni ions are delivered into cells and induce the production of free radicals (68). Ni2+ triggers an inflammatory response by activating human TLR4 (115).
Xin et al. found that Ni-refining fume particles can induce the decrease of the mitochondrial membrane potential (MMP) and the increase of the opening rate of the mitochondrial permeability transition pore (MPTP) in human lung epithelial BEAS-2B cells, and activation of the NLRP3 inflammasome induced by Ni-refining fume particles can be significantly suppressed by NAC, an effective ROS remover, suggesting that Ni-refining fume particles activate the NLRP3 inflammasome by causing mitochondrial dysfunction and ROS production (68). Another study also showed that Ni-refining fumes promoted the expression of the NLRP3 inflammasome by inducing the Warburg effect in BEAS-2B cells (69). In addition, it has also been confirmed that activation of the NLRP3 inflammasome by Ni ions is independent of the phagolysosome–cathepsin B pathway (70). However, in lung pathology, Ni-contaminated particles activated the NLRP3 inflammasome by disrupting macrophage phagolysosomes, which resulted in prolonged inflammation (71). In a study of Ni ions, NiCl2 induced the accumulation of ROS and mitochondrial DNA, resulting in the activation of the NLRP3 inflammasome. It was also found that NiCl2-induced apoptosis is dependent on ROS generation, suggesting that NiCl2 can induce both apoptosis and pyroptosis (72).
3.11 Metal element-bearing ceramic materials
Ceramic materials are widely used in orthopedic implantations because of their low osteolytic potential and friction coefficients and high biocompatibility. The particles of ceramic materials can be taken up by immune cells and can induce an immune response, including inflammasome activation (31, 116). Titanium dioxide (TiO2), zirconium oxide (ZrO2), and aluminum oxide (Al2O3) are widely applied in bioceramic implantation due to their satisfactory properties of wear, tear, hardness, biocompatibility, and corrosion resistance (107). Cytotoxicity significantly increases when macrophages are exposed to high concentrations of ZrO2 particles (≥107 particles/ml). However, compared to TiO2, ZrO2 particles produce less inflammatory cytokines, suggesting that they are less toxic than TiO2 (117). Jamieson et al. found that Al2O3 and ZrO2 treatment can enhance the gene expression of IL-1β, which is TLR4-dependent. Priming cells with LPS following Al2O3 or ZrO2 treatment can induce cell death. In addition, LPS and ZrO2 treatment can also induce IL-1β protein secretion, while treatment with LPS and Al2O3 was insufficient to induce it. These results suggest that ZrO2, but not Al2O3, may activate the inflammasome (73). In another in vivo study, intraperitoneal injection of a water-soluble supernatant with Al2O3 in mice revealed that the mRNA expression of NLRP3 decreased while that of caspase-1 did not change (74).
4 Conclusion and perspective
Metals are widely used in the fabrication of implants due to their advantage of having good mechanical properties and ductility compared to other biomaterials. However, metal corrosion inducing ion release and metal debris production is inevitable. At the same time, the amount of metal released is highest after the operation, which is also the acute inflammatory phase initiated by injury to the tissue and is one of the factors that define the outcome of the implant (118). A growing number of studies has provided new insights into how these metals affect the early inflammatory response of bone regeneration after metal implantation. However, whether the specific ion activates inflammasomes synergistically or singly is still unclear. The detailed mechanisms of metal ions activating inflammasomes are still under investigation. Additional studies are also needed to further understand the roles of metal implantation debris and the metal ions released from implantation in mediating the process of inflammasome activation. Immunomodulatory alloy biomaterials based on the NLRP3 inflammasome activation mechanism could be developed, but the immune response of tissues to these biomaterials needs to be further confirmed.
Author contributions
WH, ZZ, and YQ wrote the manuscript and created the figures and the table. QZ and QW reviewed and edited the manuscript and provided guidance. YG and YF provided important perspective of the manuscript. All authors contributed to the article and approved the submitted version.
Funding
This work is supported by the Natural Science Foundation Project of Liaoning Province (2019-ZD-0775 and 2020-MS-150).
Conflict of interest
The authors declare that the research was conducted in the absence of any commercial or financial relationships that could be construed as a potential conflict of interest.
Publisher’s note
All claims expressed in this article are solely those of the authors and do not necessarily represent those of their affiliated organizations, or those of the publisher, the editors and the reviewers. Any product that may be evaluated in this article, or claim that may be made by its manufacturer, is not guaranteed or endorsed by the publisher.
Glossary
References
1. Guglielmotti MB, Olmedo DG, Cabrini RL. Research on implants and osseointegration. Periodontol 2000 (2019) 79(1):178–89. doi: 10.1111/prd.12254
2. Kaur M, Singh K. Review on titanium and titanium based alloys as biomaterials for orthopaedic applications. Mater Sci Eng C Mater Biol Appl (2019) 102:844–62. doi: 10.1016/j.msec.2019.04.064
3. Kuzyk PR, Schemitsch EH. The basic science of peri-implant bone healing. Indian J Orthop (2011) 45:108–15. doi: 10.4103/0019-5413.77129
4. Glenske K, Donkiewicz P, Köwitsch A, Milosevic-Oljaca N, Rider P, Rofall S, et al. Applications of metals for bone regeneration. Int J Mol Sci (2018) 19:e826. doi: 10.3390/ijms19030826
5. McKee AS, Fontenot AP. Interplay of innate and adaptive immunity in metal-induced hypersensitivity. Curr Opin Immunol (2016) 42:25–30. doi: 10.1016/j.coi.2016.05.001
6. Broz P, Dixit VM. Inflammasomes: mechanism of assembly, regulation and signalling. Nat Rev Immunol (2016) 16:407–20. doi: 10.1038/nri.2016.58
7. Zheng D, Liwinski T, Elinav E. Inflammasome activation and regulation: toward a better understanding of complex mechanisms. Cell Discov (2020) 6:36. doi: 10.1038/s41421-020-0167-x
8. Chen H, Agrawal DK, Thankam FG. Biomaterials-driven sterile inflammation. Tissue Eng Part B Rev (2022) 28:22–34. doi: 10.1089/ten.TEB.2020.0253
9. Insuasti-Cruz E, Suárez-Jaramillo V, Mena Urresta KA, Pila-Varela KO, Fiallos-Ayala X, Dahoumane SA, et al. Natural biomaterials from biodiversity for healthcare applications. Adv Healthc Mater (2022) 11:e2101389. doi: 10.1002/adhm.202101389
10. Witte F. The history of biodegradable magnesium implants: A review. Acta Biomater (2010) 6:1680–92. doi: 10.1016/j.actbio.2010.02.028
11. Vasconcelos DM, Santos SG, Lamghari M, Barbosa MA. The two faces of metal ions: From implants rejection to tissue repair/regeneration. Biomaterials (2016) 84:262–75. doi: 10.1016/j.biomaterials.2016.01.046
12. Davies JE. Bone bonding at natural and biomaterial surfaces. Biomaterials (2007) 28:5058–67. doi: 10.1016/j.biomaterials.2007.07.049
13. Marco F, Milena F, Gianluca G, Vittoria O. Peri-implant osteogenesis in health and osteoporosis. Micron (2005) 36:630–44. doi: 10.1016/j.micron.2005.07.008
14. Schwarz F, Derks J, Monje A, Wang H-L. Peri-implantitis. J Clin Periodontol (2018) 45 Suppl 20:S246–66. doi: 10.1111/jcpe.12954
15. Martinon F, Burns K, Tschopp J. The inflammasome: a molecular platform triggering activation of inflammatory caspases and processing of proIL-beta. Mol Cell (2002) 10:417–26. doi: 10.1016/s1097-2765(02)00599-3
16. Kesavardhana S, Malireddi RKS, Kanneganti T-D. Caspases in cell death, inflammation, and pyroptosis. Annu Rev Immunol (2020) 38:567–95. doi: 10.1146/annurev-immunol-073119-095439
17. Lu F, Lan Z, Xin Z, He C, Guo Z, Xia X, et al. Emerging insights into molecular mechanisms underlying pyroptosis and functions of inflammasomes in diseases. J Cell Physiol (2020) 235:3207–21. doi: 10.1002/jcp.29268
18. Swanson KV, Deng M, Ting JP-Y. The NLRP3 inflammasome: molecular activation and regulation to therapeutics. Nat Rev Immunol (2019) 19:477–89. doi: 10.1038/s41577-019-0165-0
19. Huang Y, Xu W, Zhou R. NLRP3 inflammasome activation and cell death. Cell Mol Immunol (2021) 18:2114–27. doi: 10.1038/s41423-021-00740-6
20. Huang W, Zeng F, Gu Y, Jiang M, Zhang X, Yan X, et al. Porphyromonas gingivalis infection induces synaptic failure via increased IL-1β production in leptomeningeal cells. JAD (2021) 83:665–81. doi: 10.3233/JAD-210031
21. Paik S, Kim JK, Silwal P, Sasakawa C, Jo E-K. An update on the regulatory mechanisms of NLRP3 inflammasome activation. Cell Mol Immunol (2021) 18:1141–60. doi: 10.1038/s41423-021-00670-3
22. Coll RC, Hill JR, Day CJ, Zamoshnikova A, Boucher D, Massey NL, et al. MCC950 directly targets the NLRP3 ATP-hydrolysis motif for inflammasome inhibition. Nat Chem Biol (2019) 15:556–9. doi: 10.1038/s41589-019-0277-7
23. Sharif H, Wang L, Wang WL, Magupalli VG, Andreeva L, Qiao Q, et al. Structural mechanism for NEK7-licensed activation of NLRP3 inflammasome. Nature (2019) 570:338–43. doi: 10.1038/s41586-019-1295-z
24. Latz E, Xiao TS, Stutz A. Activation and regulation of the inflammasomes. Nat Rev Immunol (2013) 13:397–411. doi: 10.1038/nri3452
25. Kelley N, Jeltema D, Duan Y, He Y. The NLRP3 inflammasome: An overview of mechanisms of activation and regulation. Int J Mol Sci (2019) 20:e3328. doi: 10.3390/ijms20133328
26. Pettersson M, Almlin S, Romanos GE, Johansson A. Ti Ions induce IL-1β release by activation of the NLRP3 inflammasome in a human macrophage cell line. Inflammation (2022) 45(5):2027–37. doi: 10.1007/s10753-022-01672-7
27. Wang C, Zhang R, Wei X, Lv M, Jiang Z. Metalloimmunology: The metal ion-controlled immunity. Adv Immunol (2020) 145:187–241. doi: 10.1016/bs.ai.2019.11.007
28. Ma F IC. Effects of metal ions on caspase-1 activation and interleukin-1β release in murine bone marrow-derived macrophages. PLos One (2018) 13(8):e0199936. doi: 10.1371/journal.pone.0199936
29. Samelko L, Caicedo M, McAllister K, Jacobs J, Hallab NJ. Metal-induced delayed type hypersensitivity responses potentiate particle induced osteolysis in a sex and age dependent manner. PLos One (2021) 16:e0251885. doi: 10.1371/journal.pone.0251885
30. Vishwakarma A, Bhise NS, Evangelista MB, Rouwkema J, Dokmeci MR, Ghaemmaghami AM, et al. Engineering immunomodulatory biomaterials to tune the inflammatory response. Trends Biotechnol (2016) 34:470–82. doi: 10.1016/j.tibtech.2016.03.009
31. Vasconcelos DP, Águas AP, Barbosa MA, Pelegrín P, Barbosa JN. The inflammasome in host response to biomaterials: Bridging inflammation and tissue regeneration. Acta Biomater (2019) 83:1–12. doi: 10.1016/j.actbio.2018.09.056
32. Li X, Tang L, Thu Ye M, Chen D. Titanium ions play a synergistic role in the activation of NLRP3 inflammasome in jurkat T cells. Inflammation (2020) 43:1269–78. doi: 10.1007/s10753-020-01206-z
33. St Pierre CA, Chan M, Iwakura Y, Ayers DC, Kurt-Jones EA, Finberg RW. Periprosthetic osteolysis: characterizing the innate immune response to titanium wear-particles. J Orthop Res (2010) 28:1418–24. doi: 10.1002/jor.21149
34. Jessop F, Hamilton RF, Rhoderick JF, Fletcher P, Holian A. Phagolysosome acidification is required for silica and engineered nanoparticle-induced lysosome membrane permeabilization and resultant NLRP3 inflammasome activity. Toxicol Appl Pharmacol (2017) 318:58–68. doi: 10.1016/j.taap.2017.01.012
35. Zheng K, Bai J, Li N, Li M, Sun H, Zhang W, et al. Protective effects of sirtuin 3 on titanium particle-induced osteogenic inhibition by regulating the NLRP3 inflammasome via the GSK-3β/β-catenin signalling pathway. Bioact Mater (2021) 6:3343–57. doi: 10.1016/j.bioactmat.2021.02.039
36. Brough D, Pelegrin P, Rothwell NJ. Pannexin-1-dependent caspase-1 activation and secretion of IL-1beta is regulated by zinc. Eur J Immunol (2009) 39:352–8. doi: 10.1002/eji.200838843
37. Summersgill H, England H, Lopez-Castejon G, Lawrence CB, Luheshi NM, Pahle J, et al. Zinc depletion regulates the processing and secretion of IL-1β. Cell Death Dis (2014) 5:e1040. doi: 10.1038/cddis.2013.547
38. Fan Y, Zhang X, Yang L, Wang J, Hu Y, Bian A, et al. Zinc inhibits high glucose-induced NLRP3 inflammasome activation in human peritoneal mesothelial cells. Mol Med Rep (2017) 16:5195–202. doi: 10.3892/mmr.2017.7236
39. Lin J-Q, Tian H, Zhao X-G, Lin S, Li D-Y, Liu Y-Y, et al. Zinc provides neuroprotection by regulating NLRP3 inflammasome through autophagy and ubiquitination in a spinal contusion injury model. CNS Neurosci Ther (2021) 27:413–25. doi: 10.1111/cns.13460
40. Zhao X, Sun J, Yuan Y, Lin S, Lin J, Mei X. Zinc promotes microglial autophagy through NLRP3 inflammasome inactivation via XIST/miR-374a-5p axis in spinal cord injury. Neurochem Res (2022) 47:372–81. doi: 10.1007/s11064-021-03441-8
41. Park H-S, Yoo MH, Koh J-Y. Role of zinc dyshomeostasis in inflammasome formation in cultured cortical cells following lipopolysaccharide or oxygen-glucose deprivation/reperfusion exposure. Neurobiol Dis (2020) 137:104771. doi: 10.1016/j.nbd.2020.104771
42. Chen Y-Y, Lee Y-H, Wang B-J, Chen R-J, Wang Y-J. Skin damage induced by zinc oxide nanoparticles combined with UVB is mediated by activating cell pyroptosis via the NLRP3 inflammasome-autophagy-exosomal pathway. Part Fibre Toxicol (2022) 19:2. doi: 10.1186/s12989-021-00443-w
43. Wang D, Zheng J, Hu Q, Zhao C, Chen Q, Shi P, et al. Magnesium protects against sepsis by blocking gasdermin d n-terminal-induced pyroptosis. Cell Death Differ (2020) 27:466–81. doi: 10.1038/s41418-019-0366-x
44. Zhao X-J, Yang Y-Z, Zheng Y-J, Wang S-C, Gu H-M, Pan Y, et al. Magnesium isoglycyrrhizinate blocks fructose-induced hepatic NF-κB/NLRP3 inflammasome activation and lipid metabolism disorder. Eur J Pharmacol (2017) 809:141–50. doi: 10.1016/j.ejphar.2017.05.032
45. Yang Q, Wang J, Liu R, Wang Z, Li Y, Zhang Y, et al. Amelioration of concanavalin a-induced autoimmune hepatitis by magnesium isoglycyrrhizinate through inhibition of CD4(+)CD25(-)CD69(+) subset proliferation. Drug Des Devel Ther (2016) 10:443–53. doi: 10.2147/DDDT.S92440
46. Deigendesch N, Zychlinsky A, Meissner F. Copper regulates the canonical NLRP3 inflammasome. J Immunol (2018) 200(5):1607–17. doi: 10.4049/jimmunol.1700712
47. Tao X, Wan X, Wu D, Song E, Song Y. A tandem activation of NLRP3 inflammasome induced by copper oxide nanoparticles and dissolved copper ion in J774A.1 macrophage. J Hazardous Materials (2021) 411:125134. doi: 10.1016/j.jhazmat.2021.125134
48. Xiao J, Lv Y, Lin B, Tipoe GL, Youdim MBH, Xing F, et al. A novel antioxidant multitarget iron chelator M30 protects hepatocytes against ethanol-induced injury. Oxid Med Cell Longev (2015) 2015:607271. doi: 10.1155/2015/607271
49. Nakamura K, Kawakami T, Yamamoto N, Tomizawa M, Fujiwara T, Ishii T, et al. Activation of the NLRP3 inflammasome by cellular labile iron. Exp Hematol (2016) 44:116–24. doi: 10.1016/j.exphem.2015.11.002
50. Zhao X, Yin L, Wu Y, Han M, Zhuang Y, Cong Y, et al. Manganese induces neuroinflammation via NF-κB/ROS NLRP3 pathway in rat brain striatum and HAPI cells. Mol Cell Toxicol (2019) 15:173–83. doi: 10.1007/s13273-019-0021-0
51. Sarkar S, Rokad D, Malovic E, Luo J, Harischandra DS, Jin H, et al. Manganese activates NLRP3 inflammasome signaling and propagates exosomal release of ASC in microglial cells. Sci Signal (2019) 12:eaat9900. doi: 10.1126/scisignal.aat9900
52. Jen H-I, Lin Z-Y, Guo J-X, Lee C-I. The effects of divalent cation-chelated prion fibrils on the immune response of EOC 13. 31 Microglia Cells Cells (2020) 9:e2285. doi: 10.3390/cells9102285
53. Darwesh AM, Bassiouni W, Adebesin AM, Mohammad AS, Falck JR, Seubert JM. A synthetic epoxydocosapentaenoic acid analogue ameliorates cardiac Ischemia/Reperfusion injury: The involvement of the sirtuin 3-NLRP3 pathway. Int J Mol Sci (2020) 21:e5261. doi: 10.3390/ijms21155261
54. Zhuang Y, Yasinta M, Hu C, Zhao M, Ding G, Bai M, et al. Mitochondrial dysfunction confers albumin-induced NLRP3 inflammasome activation and renal tubular injury. Am J Physiol Renal Physiol (2015) 308:F857–866. doi: 10.1152/ajprenal.00203.2014
55. Villegas LR, Kluck D, Field C, Oberley-Deegan RE, Woods C, Yeager ME, et al. Superoxide dismutase mimetic, MnTE-2-PyP, attenuates chronic hypoxia-induced pulmonary hypertension, pulmonary vascular remodeling, and activation of the NALP3 inflammasome. Antioxid Redox Signal (2013) 18:1753–64. doi: 10.1089/ars.2012.4799
56. Zhang C, Hao X, Chang J, Geng Z, Wang Z. Mn-TAT PTD-ngb attenuates oxidative injury by an enhanced ROS scavenging ability and the regulation of redox signaling pathway. Sci Rep (2019) 9:20103. doi: 10.1038/s41598-019-56595-5
57. Zhang C, Yang R, Hao X, Geng Z, Wang Z. Mn-TAT PTD-ngb ameliorates inflammation through the elimination of damaged mitochondria and the activation of Nrf2-antioxidant signaling pathway. Biochem Pharmacol (2020) 178:114055. doi: 10.1016/j.bcp.2020.114055
58. He Y, Zeng MY, Yang D, Motro B, Núñez G. NEK7 is an essential mediator of NLRP3 activation downstream of potassium efflux. Nature (2016) 530:354–7. doi: 10.1038/nature16959
59. Xu Z, Chen Z, Wu X, Zhang L, Cao Y, Zhou P. Distinct molecular mechanisms underlying potassium efflux for NLRP3 inflammasome activation. Front Immunol (2020) 11:609441. doi: 10.3389/fimmu.2020.609441
60. Yang D, He Y, Muñoz-Planillo R, Liu Q, Núñez G. Caspase-11 requires the pannexin-1 channel and the purinergic P2X7 pore to mediate pyroptosis and endotoxic shock. Immunity (2015) 43:923–32. doi: 10.1016/j.immuni.2015.10.009
61. Kayagaki N, Stowe IB, Lee BL, O’Rourke K, Anderson K, Warming S, et al. Caspase-11 cleaves gasdermin d for non-canonical inflammasome signalling. Nature (2015) 526:666–71. doi: 10.1038/nature15541
62. Adam C, Wohlfarth J, Haußmann M, Sennefelder H, Rodin A, Maler M, et al. Allergy-inducing chromium compounds trigger potent innate immune stimulation Via ROS-dependent inflammasome activation. J Invest Dermatol (2017) 137:367–76. doi: 10.1016/j.jid.2016.10.003
63. Jämsen E, Pajarinen J, Kouri V-P, Rahikkala A, Goodman SB, Manninen M, et al. Tumor necrosis factor primes and metal particles activate the NLRP3 inflammasome in human primary macrophages. Acta Biomater (2020) 108:347–57. doi: 10.1016/j.actbio.2020.03.017
64. Klasson M, Lindberg M, Westberg H, Bryngelsson I-L, Tuerxun K, Persson A, et al. Dermal exposure to cobalt studied in vitro in keratinocytes - effects of cobalt exposure on inflammasome activated cytokines, and mRNA response. Biomarkers (2021) 26:674–84. doi: 10.1080/1354750X.2021.1975823
65. Kim E-H, Won J-H, Hwang I, Yu J-W. Cobalt chloride-induced hypoxia ameliorates NLRP3-mediated caspase-1 activation in mixed glial cultures. Immune Netw (2013) 13:141–7. doi: 10.4110/in.2013.13.4.141
66. Feng S, Zhang Z, Mo Y, Tong R, Zhong Z, Chen Z, et al. Activation of NLRP3 inflammasome in hepatocytes after exposure to cobalt nanoparticles: The role of oxidative stress. Toxicol In Vitro (2020) 69:104967. doi: 10.1016/j.tiv.2020.104967
67. Nurmi K, Kareinen I, Virkanen J, Rajamäki K, Kouri V-P, Vaali K, et al. Hemin and cobalt protoporphyrin inhibit NLRP3 inflammasome activation by enhancing autophagy: A novel mechanism of inflammasome regulation. J Innate Immun (2017) 9:65–82. doi: 10.1159/000448894
68. Xin R, Pan Y-L, Wang Y, Wang S-Y, Wang R, Xia B, et al. Nickel-refining fumes induce NLRP3 activation dependent on mitochondrial damage and ROS production in beas-2B cells. Arch Biochem Biophys (2019) 676:108148. doi: 10.1016/j.abb.2019.108148
69. Wang R, Wang S-Y, Wang Y, Xin R, Xia B, Xin Y, et al. The warburg effect promoted the activation of the NLRP3 inflammasome induced by Ni-refining fumes in BEAS-2B cells. Toxicol Ind Health (2020) 36:580–90. doi: 10.1177/0748233720937197
70. Li X, Zhong F. Nickel induces interleukin-1β secretion via the NLRP3-ASC-caspase-1 pathway. Inflammation (2014) 37:457–66. doi: 10.1007/s10753-013-9759-z
71. Hamilton RF, Buford M, Xiang C, Wu N, Holian A. NLRP3 inflammasome activation in murine alveolar macrophages and related lung pathology is associated with MWCNT nickel contamination. Inhal Toxicol (2012) 24:995–1008. doi: 10.3109/08958378.2012.745633
72. Guo H, Liu H, Jian Z, Cui H, Fang J, Zuo Z, et al. Nickel induces inflammatory activation via NF-κB, MAPKs, IRF3 and NLRP3 inflammasome signaling pathways in macrophages. Aging (Albany NY) (2019) 11:11659–72. doi: 10.18632/aging.102570
73. Jamieson S, Mawdesley A, Deehan D, Kirby J, Holland J, Tyson-Capper A. Inflammatory responses to metal oxide ceramic nanopowders. Sci Rep (2021) 11:10531. doi: 10.1038/s41598-021-89329-7
74. Ogino K, Zhang R, Takahashi H, Takemoto K, Kubo M, Murakami I, et al. Allergic airway inflammation by nasal inoculation of particulate matter (PM2.5) in NC/Nga mice. PLos One (2014) 9:e92710. doi: 10.1371/journal.pone.0092710
75. Agarwal R, García AJ. Biomaterial strategies for engineering implants for enhanced osseointegration and bone repair. Adv Drug Delivery Rev (2015) 94:53–62. doi: 10.1016/j.addr.2015.03.013
76. Hanawa T. Titanium-tissue interface reaction and its control with surface treatment. Front Bioeng Biotechnol (2019) 7:170. doi: 10.3389/fbioe.2019.00170
77. Pettersson M, Kelk P, Belibasakis GN, Bylund D, Molin Thorén M, Johansson A. Titanium ions form particles that activate and execute interleukin-1β release from lipopolysaccharide-primed macrophages. J Periodontal Res (2017) 52:21–32. doi: 10.1111/jre.12364
78. Yazdi AS, Guarda G, Riteau N, Drexler SK, Tardivel A, Couillin I, et al. Nanoparticles activate the NLR pyrin domain containing 3 (Nlrp3) inflammasome and cause pulmonary inflammation through release of IL-1α and IL-1β. Proc Natl Acad Sci U.S.A. (2010) 107:19449–54. doi: 10.1073/pnas.1008155107
79. Kolling J, Tigges J, Hellack B, Albrecht C, Schins RPF. Evaluation of the NLRP3 inflammasome activating effects of a Large panel of TiO2 nanomaterials in macrophages. Nanomaterials (Basel) (2020) 10:E1876. doi: 10.3390/nano10091876
80. Fort BP, Dubyak GR, Greenfield EM. Lysosomal disruption by orthopedic wear particles induces activation of the NLRP3 inflammasome and macrophage cell death by distinct mechanisms. J Orthop Res (2021) 39:493–505. doi: 10.1002/jor.24826
81. Tao Z-S, Zhou W-S, He X-W, Liu W, Bai B-L, Zhou Q, et al. A comparative study of zinc, magnesium, strontium-incorporated hydroxyapatite-coated titanium implants for osseointegration of osteopenic rats. Materials Sci Engineering: C (2016) 62:226–32. doi: 10.1016/j.msec.2016.01.034
82. Feske S, Wulff H, Skolnik EY. Ion channels in innate and adaptive immunity. Annu Rev Immunol (2015) 33:291–353. doi: 10.1146/annurev-immunol-032414-112212
83. Yu T, Ao Q, Ao T, Ahmad MA, Wang A, Xu Y, et al. Preparation and assessment of an optimized multichannel acellular nerve allograft for peripheral nerve regeneration. Bioengineering Transla Med (2022). doi: 10.1002/btm2.10435
84. Nie X, Zhang X, Lei B, Shi Y, Yang J. Regulation of magnesium matrix composites materials on bone immune microenvironment and osteogenic mechanism. Front Bioeng Biotechnol (2022) 10:842706. doi: 10.3389/fbioe.2022.842706
85. Yang Y, Huang L, Tian C, Qian B. Magnesium isoglycyrrhizinate inhibits airway inflammation in rats with chronic obstructive pulmonary disease. BMC Pulm Med (2021) 21:371. doi: 10.1186/s12890-021-01745-7
86. Chellan P, Sadler PJ. The elements of life and medicines. Philos Trans A Math Phys Eng Sci (2015) 373:20140182. doi: 10.1098/rsta.2014.0182
87. Liu H, Zhang X, Jin S, Zhao Y, Ren L, Yang K. Effect of copper-doped titanium nitride coating on angiogenesis. Materials Lett (2020) 269:127634. doi: 10.1016/j.matlet.2020.127634
88. Ge EJ, Bush AI, Casini A, Cobine PA, Cross JR, DeNicola GM, et al. Connecting copper and cancer: from transition metal signalling to metalloplasia. Nat Rev Cancer (2022) 22:102–13. doi: 10.1038/s41568-021-00417-2
89. Ohgami RS, Campagna DR, McDonald A, Fleming MD. The steap proteins are metalloreductases. Blood (2006) 108:1388–94. doi: 10.1182/blood-2006-02-003681
90. Bertinato J, L’Abbé MR. Maintaining copper homeostasis: regulation of copper-trafficking proteins in response to copper deficiency or overload. J Nutr Biochem (2004) 15:316–22. doi: 10.1016/j.jnutbio.2004.02.004
91. Fang T, Chen W, Sheng Y, Yuan S, Tang Q, Li G, et al. Tetrathiomolybdate induces dimerization of the metal-binding domain of ATPase and inhibits platination of the protein. Nat Commun (2019) 10:186. doi: 10.1038/s41467-018-08102-z
92. Li Y, Jahr H, Pavanram P, Bobbert FSL, Puggi U, Zhang X-Y, et al. Additively manufactured functionally graded biodegradable porous iron. Acta Biomaterialia (2019) 96:646–61. doi: 10.1016/j.actbio.2019.07.013
93. van Beers EJ, Yang Y, Raghavachari N, Tian X, Allen DT, Nichols JS, et al. Iron, inflammation, and early death in adults with sickle cell disease. Circ Res (2015) 116:298–306. doi: 10.1161/CIRCRESAHA.116.304577
94. Bogdan AR, Miyazawa M, Hashimoto K, Tsuji Y. Regulators of iron homeostasis: New players in metabolism, cell death, and disease. Trends Biochem Sci (2016) 41:274–86. doi: 10.1016/j.tibs.2015.11.012
95. Gelfand BD, Wright CB, Kim Y, Yasuma T, Yasuma R, Li S, et al. Iron toxicity in the retina requires alu RNA and the NLRP3 inflammasome. Cell Rep (2015) 11:1686–93. doi: 10.1016/j.celrep.2015.05.023
96. Liu L, Sha R, Yang L, Zhao X, Zhu Y, Gao J, et al. Impact of morphology on iron oxide nanoparticles-induced inflammasome activation in macrophages. ACS Appl Mater Interfaces (2018) 10:41197–206. doi: 10.1021/acsami.8b17474
97. Liu Y-T, Kung K-C, Lee T-M, Lui T-S. Enhancing biological properties of porous coatings through the incorporation of manganese. J Alloys Compounds (2013) 581:459–67. doi: 10.1016/j.jallcom.2013.07.106
98. Aguirre JD, Culotta VC. Battles with iron: Manganese in oxidative stress protection. J Biol Chem (2012) 287:13541–8. doi: 10.1074/jbc.R111.312181
99. Wu Q, Mu Q, Xia Z, Min J, Wang F. Manganese homeostasis at the host-pathogen interface and in the host immune system. Semin Cell Dev Biol (2021) 115:45–53. doi: 10.1016/j.semcdb.2020.12.006
100. Tinkov AA, Paoliello MMB, Mazilina AN, Skalny AV, Martins AC, Voskresenskaya ON, et al. Molecular targets of manganese-induced neurotoxicity: A five-year update. Int J Mol Sci (2021) 22:4646. doi: 10.3390/ijms22094646
101. Peng D, Li J, Deng Y, Zhu X, Zhao L, Zhang Y, et al. Sodium para-aminosalicylic acid inhibits manganese-induced NLRP3 inflammasome-dependent pyroptosis by inhibiting NF-κB pathway activation and oxidative stress. J Neuroinflamm (2020) 17:343. doi: 10.1186/s12974-020-02018-6
102. Fang Y, Peng D, Liang Y, Lu L, Li J, Zhao L, et al. Sodium p-aminosalicylic acid inhibits manganese-induced neuroinflammation in BV2 microglial cells via NLRP3-CASP1 inflammasome pathway. Biol Trace Elem Res (2021) 199:3423–32. doi: 10.1007/s12011-020-02471-7
103. Pariano M, Pieroni S, De Luca A, Iannitti RG, Borghi M, Puccetti M, et al. Anakinra activates superoxide dismutase 2 to mitigate inflammasome activity. IJMS (2021) 22:6531. doi: 10.3390/ijms22126531
104. Li C, Chen M, He X, Ouyang D. A mini-review on ion fluxes that regulate NLRP3 inflammasome activation. Acta Biochim Biophys Sin (Shanghai) (2021) 53:131–9. doi: 10.1093/abbs/gmaa155
105. Tang T, Lang X, Xu C, Wang X, Gong T, Yang Y, et al. CLICs-dependent chloride efflux is an essential and proximal upstream event for NLRP3 inflammasome activation. Nat Commun (2017) 8:202. doi: 10.1038/s41467-017-00227-x
106. Cheung AC, Banerjee S, Cherian JJ, Wong F, Butany J, Gilbert C, et al. Systemic cobalt toxicity from total hip arthroplasties: review of a rare condition part 1 - history, mechanism, measurements, and pathophysiology. Bone Joint J (2016) 98-B(1):6–13. doi: 10.1302/0301-620X.98B1.36374
107. Amirtharaj Mosas KK, Chandrasekar AR, Dasan A, Pakseresht A, Galusek D. Recent advancements in materials and coatings for biomedical implants. Gels (2022) 8:323. doi: 10.3390/gels8050323
108. Nandedkar AK, Hong MS, Friedberg F. Co2+ binding by plasma albumin. Biochem Med (1974) 9:177–83. doi: 10.1016/0006-2944(74)90050-7
109. Lu J, Stewart AJ, Sleep D, Sadler PJ, Pinheiro TJT, Blindauer CA. A molecular mechanism for modulating plasma zn speciation by fatty acids. J Am Chem Soc (2012) 134:1454–7. doi: 10.1021/ja210496n
110. Raghavan B, Martin SF, Esser PR, Goebeler M, Schmidt M. Metal allergens nickel and cobalt facilitate TLR4 homodimerization independently of MD2. EMBO Rep (2012) 13:1109–15. doi: 10.1038/embor.2012.155
111. Paladini F, Cocco E, Potolicchio I, Fazekasova H, Lombardi G, Fiorillo MT, et al. Divergent effect of cobalt and beryllium salts on the fate of peripheral blood monocytes and T lymphocytes. Toxicol Sci (2011) 119:257–69. doi: 10.1093/toxsci/kfq328
112. Kimura T. Molecular mechanism involved in chromium(VI) toxicity. Yakugaku Zasshi (2007) 127:1957–65. doi: 10.1248/yakushi.127.1957
113. Baumann CA, Crist BD. Nickel allergy to orthopaedic implants: A review and case series. J Clin Orthopaedics Trauma (2020) 11:S596–603. doi: 10.1016/j.jcot.2020.02.008
114. Harloff T, Hönle W, Holzwarth U, Bader R, Thomas P, Schuh A. Titanium allergy or not? “Impurity” of titanium implant materials. Health (2010) 02:306–10. doi: 10.4236/health.2010.24045
115. Schmidt M, Raghavan B, Müller V, Vogl T, Fejer G, Tchaptchet S, et al. Crucial role for human toll-like receptor 4 in the development of contact allergy to nickel. Nat Immunol (2010) 11:814–9. doi: 10.1038/ni.1919
116. Beraudi A, Stea S, De Pasquale D, Bordini B, Catalani S, Apostoli P, et al. Metal ion release: also a concern for ceramic-on-ceramic couplings? Hip Int (2014) 24:321–6. doi: 10.5301/hipint.5000132
117. Ramenzoni LL, Flückiger LB, Attin T, Schmidlin PR. Effect of titanium and zirconium oxide microparticles on pro-inflammatory response in human macrophages under induced sterile inflammation: An In vitro study. Materials (Basel) (2021) 14:4166. doi: 10.3390/ma14154166
Keywords: inflammasome, metal, inflammation, implant, osteoimmunology
Citation: Huang W, Zhang Z, Qiu Y, Gao Y, Fan Y, Wang Q and Zhou Q (2023) NLRP3 inflammasome activation in response to metals. Front. Immunol. 14:1055788. doi: 10.3389/fimmu.2023.1055788
Received: 28 September 2022; Accepted: 16 January 2023;
Published: 10 February 2023.
Edited by:
Luiz Eduardo Baggio Savio, Federal University of Rio de Janeiro, BrazilReviewed by:
Kívia De Andrade, University of São Paulo, BrazilDechun Geng, The First Affiliated Hospital of Soochow University, China
Copyright © 2023 Huang, Zhang, Qiu, Gao, Fan, Wang and Zhou. This is an open-access article distributed under the terms of the Creative Commons Attribution License (CC BY). The use, distribution or reproduction in other forums is permitted, provided the original author(s) and the copyright owner(s) are credited and that the original publication in this journal is cited, in accordance with accepted academic practice. No use, distribution or reproduction is permitted which does not comply with these terms.
*Correspondence: Qing Zhou, enFmb3JzdHVkZW50QDE2My5jb20=; Qiang Wang, bWZxd2FuZ0BjbXUuZWR1LmNu
†These authors have contributed equally to this work