- 1National Research Centre for Epidemiology and Microbiology named after Honorary Academician N F Gamaleya of the Ministry of Health of the Russian Federation, Moscow, Russia
- 2Belozersky Institute of Physico-Chemical Biology, Lomonosov Moscow State University, Moscow, Russia
- 3Faculty of Bioengineering and Bioinformatics, Lomonosov Moscow State University, Moscow, Russia
- 4Shemyakin-Ovchinnikov Institute of Bioorganic Chemistry of the Russian Academy of Sciences, Moscow, Russia
- 5Chemistry Department, Lomonosov Moscow State University, Moscow, Russia
- 6Department of Virology, Lomonosov Moscow State University, Moscow, Russia
- 7Infectiology Department, I. M. Sechenov First Moscow State Medical University, Moscow, Russia
Single-domain antibodies (sdAbs, VHHs, or nanobodies) are a promising tool for the treatment of both infectious and somatic diseases. Their small size greatly simplifies any genetic engineering manipulations. Such antibodies have the ability to bind hard-to-reach antigenic epitopes through long parts of the variable chains, the third complementarity-determining regions (CDR3s). VHH fusion with the canonical immunoglobulin Fc fragment allows the Fc-fusion single-domain antibodies (VHH-Fc) to significantly increase their neutralizing activity and serum half-life. Previously we have developed and characterized VHH-Fc specific to botulinum neurotoxin A (BoNT/A), that showed a 1000-fold higher protective activity than monomeric form when challenged with five times the lethal dose (5 LD50) of BoNT/A. During the COVID-19 pandemic, mRNA vaccines based on lipid nanoparticles (LNP) as a delivery system have become an important translational technology that has significantly accelerated the clinical introduction of mRNA platforms. We have developed an mRNA platform that provides long-term expression after both intramuscular and intravenous application. The platform has been extensively characterized using firefly luciferase (Fluc) as a reporter. An intramuscular administration of LNP-mRNA encoding VHH-Fc antibody made it possible to achieve its rapid expression in mice and resulted in 100% protection when challenged with up to 100 LD50 of BoNT/A. The presented approach for the delivery of sdAbs using mRNA technology greatly simplifies drug development for antibody therapy and can be used for emergency prophylaxis.
Introduction
Botulism is a rare but severe and often fatal disease caused by botulinum neurotoxin (BoNT) produced by bacterium Clostridium botulinum. Botulism most commonly occurs following ingestion of contaminated food, penetration of spores through the wound or when spores are ingested and then germinate in the intestinal tract (more common in infants) (1). The inhalation of the pre-formed toxin also causes disease, but this event does not happen naturally and may occur in the context of a bioterrorist attack. Botulinum toxin has a history of use as a potential bioweapon, and thus it is classified as a Category A bioterror agent (2). Among the four major human pathogenic BoNTs (BoNT/A, B, E, and F), produced by different serotypes of C. botulinum, BoNT/A poses the most serious threat for human due to its high potency and long duration of action (3). Due to the potency and rapid onset of symptoms, exposure to botulinum toxin demands immediate anti-toxin therapy, which is usually based on equine antitoxin sera. The prophylactic therapy is also required for an individual who has eaten food suspected of being infected with C. botulinum. However, side effects, such as allergic reactions, fever serum sickness, and anaphylactic reactions, often occur (4). A vaccine against botulism exists but it is rarely used as its effectiveness has not been fully evaluated and it has demonstrated negative side effects (5).
An alternative to antitoxin serum with minimal or no side effects is treatment with monoclonal antibodies (mAbs) to BoNT (6, 7). Along with conventional antibodies, single-domain antibodies (sdAb), also referred to as VHHs (variable domains of heavy-chain only antibodies) or nanobodies, opened new perspectives in the engineering of antibodies since they were discovered (8). VHHs have some advantages over classical immunoglobulins (IgG), such as simplicity of engineering, improved heat and pH stability, and better tissue penetration (9). However, the problems of widespread use of mAbs (both classical IgG and nanobodies) for the treatment of infectious diseases are mainly associated with the complexity and high cost of producing recombinant antibody proteins (i.e. production in cultured mammalian cells followed by purification from complex media). Furthermore, these proteins have complex post-translational modifications, which can strongly impact on their biological activity and therapeutic properties of the mAbs. Thus, they need costly development and implementation of numerous analytical tools for accurate downstream control (10).
Delivery of DNA or mRNA that encode mAbs is an attractive way to circumvent the problems of the recombinant mAb production and purification (11, 12). The possibility of using mRNA-encoded antibodies may bring some advantages, such as no risk of genome integration, more controlled exposure and more rapid protein production compared to DNA-based vectors (10). Over the past decade, mRNA-based drugs have demonstrated a high potential in the treatment of various diseases (13–15). During the COVID-19 pandemic, the possibility of rapid development of mRNA vaccines based on lipid nanoparticles (LNP) as a delivery system and their successful implementation were demonstrated. This breakthrough made a significant contribution to limiting the spread of the virus and reducing or stabilizing the COVID-19 incidence in countries where these vaccines were used (16, 17). These recent advances in the treatment and prevention of infectious diseases using mRNA-based technology have increased the interest in antibody production based on this method for both therapeutic and prophylactic purposes (12, 18, 19).
Here we present an LNP-mRNA platform providing efficient long-term expression of a delivered gene in vivo. First, we showed a rapid and sustained accumulation of a reporter protein (firefly luciferase, Fluc) in mice through both intramuscular and intravenous administration routes. We then applied this technology for the production of a highly protective BoNT/A-specific nanobody fused to the human Fc fragment (hereafter called “B11-Fc antibody”), which we described previously (20). We report 100% protection in mice after intramuscular administration of the LNP-mRNA preparation as early as 3.5 h before the injection of five times the lethal dose (5 LD50) of BoNT/A. In the case of LNP-mRNA encoding VHH-Fc application 48 hours before challenge a 100% of the animals were resistant to 100 LD50 dose. The described approach greatly simplifies the development and widespread use of drugs based on sdAbs and can be used for emergency prophylaxis of various toxic and infectious diseases.
Results
Design mRNA platform and its characterization in cell cultures and living mice
To develop an mRNA platform for efficient and long-term expression, we took advantage of fleeting mRNA transfection (FLERT) of cultured mammalian cells we have successfully used before (21, 22). To provide a high translation rate and stability, all in vitro transcribed mRNAs had (i) the cap-1 structure at the 5′ end; (ii) a 100-nt long poly(A)-tail at the 3′ end; (iii) the 5′ and 3′ untranslated regions (UTRs) from the human hemoglobin alpha subunit (HBA1) mRNA; (iv) codon optimized coding sequences (CDS), as schematically shown in Figure 1A. A modified nucleoside, N1-methylpseudouridines (m1Ψ), were co-transcriptionally incorporated into the mRNA instead of 100% uridines (U) to reduce mRNA immunogenicity (23, 24).
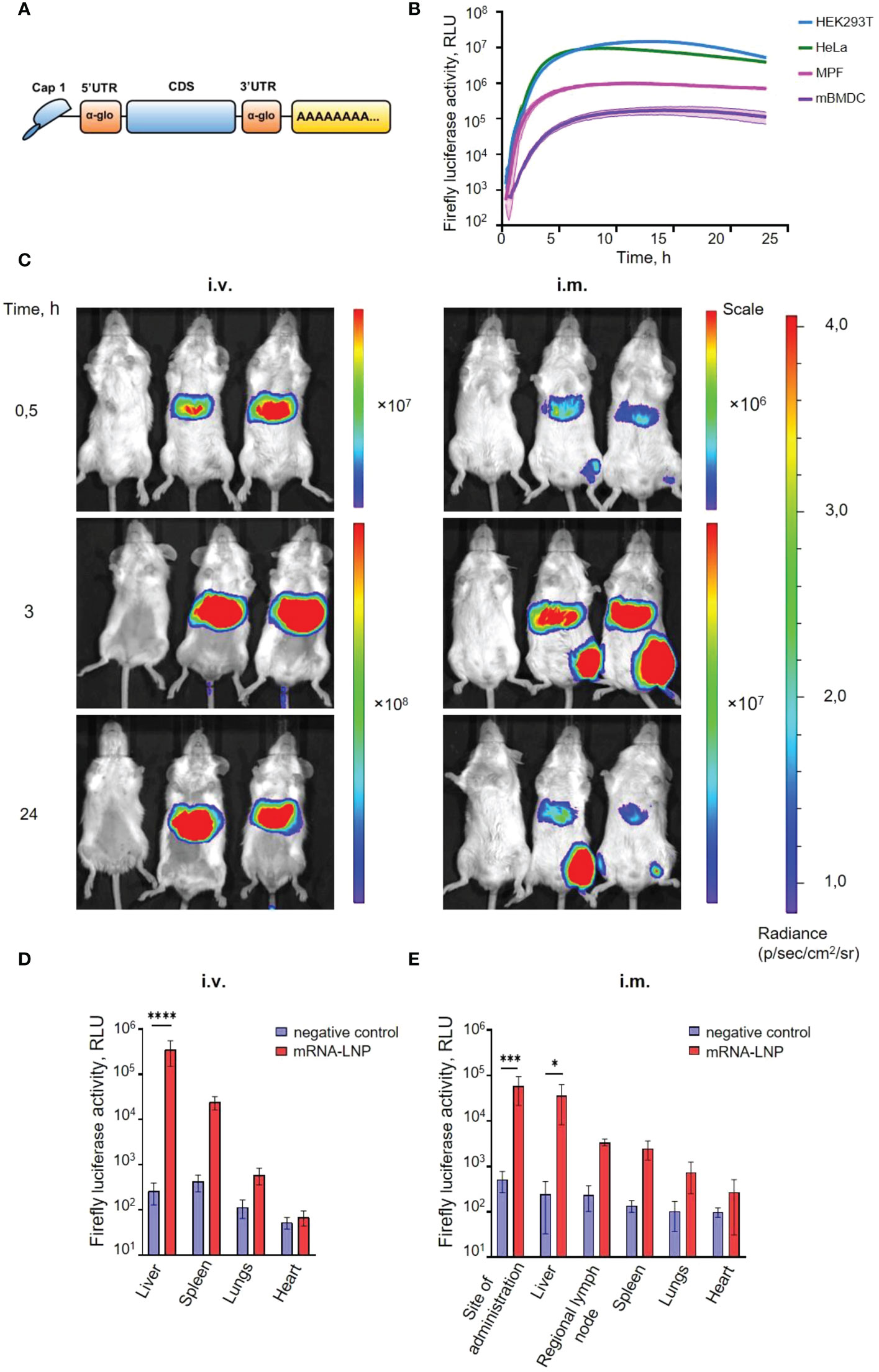
Figure 1 An mRNA platform design and characterization. (A) Schematic representation of the mRNA platform used in this study. (B) Firefly luciferase expression continuously measured after mRNA transfection of cultured mammalian cells: two human cell lines (HEK293T and HeLa), mouse primary fibroblasts (MPF), and mouse bone marrow-derived dendritic cells (mBMDC). Luciferase activity curves represent the mean (lines) and SD (shaded regions) of three technical replicates. (C) Luciferase activity in mice detected with in vivo imaging system (IVIS). BALB/c mice were injected with 5.0 µg mRNA-LNP in PBS (right and middle mouse), or PBS (left mouse) by intramuscular (i.m.) and intravenous (i.v.) routes. Relative luminescence plot is shown, and the scale of luminescence is indicated. (D, E) Luciferase activity in homogenates of different organs after 3 h after intramuscular (i.m.) or intravenous (i.v.) administration of mRNA-LNP preparation. Boxes and whiskers represent means with standard deviations (SD). p-value determined by Bonferroni’s multiple comparisons test. *p < 0.05, ***p < 0.001, ****p < 0.0001.
First, the efficiency of the designed mRNA platform was explored with firefly luciferase (Fluc). Different cell cultures, including cancer and non-cancer human cell line (HeLa and HEK293T), as well as primary cells: mouse primary fibroblasts (MPF) and mouse bone marrow-derived dendritic cells (mBMDC), were transfected with the Fluc-encoding mRNA. Luciferase activity was continuously measured within 24 h in a temperature controlled plate reader with CO2 supply, as described previously (25). In all cases, we observed a pronounced expression of the transfected mRNA, although at different levels and duration (Figure 1B). As expected (24, 26), in primary cells (in particular, in mBMDCs) the efficient expression strictly required both 100% m1Ψ incorporated instead of uridine, and the presence of cap-1 instead of cap-0 (Figure S1).
To investigate the rate and distribution of the protein production from Fluc-mRNA, mRNA-LNP were prepared (see Methods for details) and administered into mice using two delivery routes: intravenous (tail vein) and intramuscular (posterolateral thigh muscle group) injections. The luciferase activity was detected as early as 15 min in the liver after intravenous (i.v.) mRNA-LNP injection, as well as in the site of injection for intramuscular (i.m.) route of administration (data not shown). 30 min after i.m. administration, the bioluminescence was observed mainly in the site of injection and in the liver (confirming a systemic spread of the mRNA-LNPs), but at a lower level compared to the i.v. administration (Figure 1C). For i.v. injection, bioluminescence has been raised within the first three hours post injection and then decreased slightly by the 24 h. For i.m. delivery route, a sharp increase in the Fluc activity was also observed during the first 3 hours post injection, after that it was declined in the liver and was not detected by the third day (Figure S2), whereas a very long-term expression (up to 21 days post-injection) was observed in the site of injection (Figure S2).
Distribution of Fluc-mRNA expression was further confirmed by assaying luciferase activity in homogenates of isolated organs (Figures 1D, E). The highest luciferase activity was detected in the liver homogenates for i.v. route of administration, as well as in the muscle and liver homogenates for i.m. route of injection.
Passive B11-mRNA-LNP immunization and BoNT/A lethal dose protection
Based on the previous study of the camelid nanobodies (20), we designed mRNA coding for dimer of BoNT/A-specific camelid VHHs fused to human IgG Fc-fragment. The mRNA was assembled into LNP (B11-mRNA-LNP) according to the same protocol as for Fluc-mRNA before.
To assess the prophylactic effect of the single administration of B11-mRNA-LNP, the BoNT/A challenge experiments were performed. Three groups of mice (n = 3) were treated with B11-mRNA-LNP (10 μg per mouse), the fourth group was treated with recombinant antibody B11-Fc (10 μg per mouse), and the fifth one (the negative control) was treated with sterile PBS. Then all animals were challenged with 5 LD50 dose of BoNT/A according to the schedule (Figure 2A). Groups treated with B11-mRNA-LNP differed from each other in the time interval between prophylaxis and challenge (0, 3.5, 8 or 24 h). Mice in groups pre-treated with PBS or B11-mRNA-LNP at 0 h interval had been shown moderate symptoms in four hours and then died within 8 h and 12 h after BoNT/A administration, respectively. Group of mice with 3.5 h interval between prophylaxis and challenge showed mild clinical symptoms 26 h after intoxication but were survived up to 72 h without deterioration. Other groups of mice that received B11-mRNA-LNP or recombinant B11-Fc antibody and subsequently were challenged in 8 and 24 h, survived and displayed no symptoms of botulism (Figure 2B).
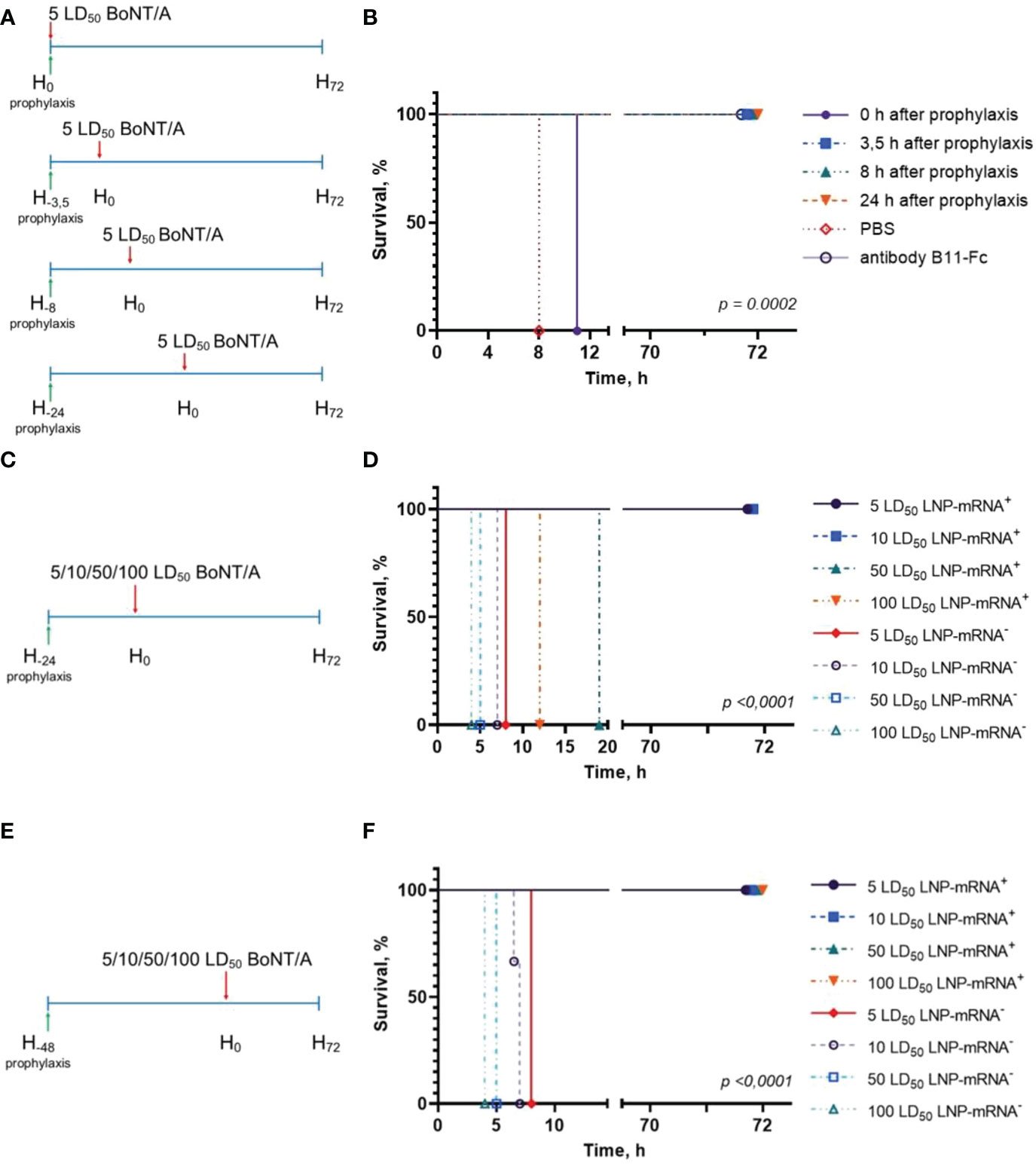
Figure 2 Study of mRNA-B11-LNP prophylactic effect. (A) Schedule of passive B11-mRNA-LNP immunization before BoNT/A challenge. A total of three mice per group were challenged and experiment was performed twice. Mice received a single intramuscular (i.m.) injection of 10 μg of B11-mRNA-LNP or recombinant B11-Fc antibody 0, 3.5, 8 or 24 h before 5 LD50 intraperitoneally injected BoNT/A challenge. Body weight, clinical score and survival were monitored for up to 72 h; (B) Survival curve of mice that were treated according to the schedule in (A). (C, E) Schedules of passive B11-mRNA-LNP immunization (10 μg of B11-mRNA-LNP per mouse) before challenge by different BoNT/A doses (5, 10, 50 and 100 LD50). A total of three mice per group were challenged and experiment was performed twice. Body weight, clinical score and survival were monitored for up to 72 h; (D, F) Survival curves of mice that were treated according to the schedules in (C, E). A total of three mice per group were challenged and experiment was performed twice. LNP-mRNA+ – mice that were treated with B11-mRNA-LNP; LNP-mRNA- – mice that did not receive B11-mRNA-LNP, but were challenged. p-value determined by log-rank (Mantel-Cox) test. “H” on (A, C, E) shows the key points of the experiment chart and means the hours.
Then, we studied the protective effect of B11-mRNA-LNP (injected 24 h and 48 h before challenge) against different BoNT/A doses (5, 10, 50 and 100 LD50) according to schedules (Figures 2C, E). Mice that were injected with B11-mRNA-LNP 24 hours before challenge did not survive the exposure of BoNT/A high doses (50 and 100 LD50) in contrast to those received B11-mRNA-LNP 48 hours before challenge (Figures 2D, F). According to the pharmacokinetic study data in mice (Figure 3) the maximum level of B11 antibodies – 99 ng/mL - was observed after 24 hours since B11-mRNA-LNP injection. B11 antibodies were being detected in mouse serum during 5 days after B11-mRNA-LNP injection.
Discussion
Recent advances in the treatment and prevention of infectious diseases using mRNA-related approaches have increased the interest in the production of antibodies based on this technology, for both therapeutic and prophylactic purposes. We have not investigated post-translational changes of our mRNA-derived antibodies. However, unlike mAbs, which go through many stages of purification from complex media and cellular components before its administration, antibodies encoded by the mRNA molecule are synthesized directly in the organism.
In this study we created and applied a new mRNA platform for the production of BoNT/A-specific nanobody fused to the human Fc fragment and showed its high protective activity in mice. To reduce immunogenicity and provide a prolonged and efficient protein synthesis, in our transcripts we replaced 100% U with m1Ψ, and used 5′ cap-1 structure, efficient human HBA1 5′ and 3′ UTRs, and a 100-nt long poly(A)-tail, as suggested in earlier studies (24, 26–29). In particular, the use of non-immunogenic mRNA is crucial, because a number of cell innate immune receptors recognizing exogenous RNA can trigger the release of type I interferons leading to activation of interferon inducible genes and inhibition of translation.
The construct was initially characterized in cultured cells (including immortalized human cell lines and primary mouse cells) using luciferase reporter assay. We demonstrated efficient reporter activity during at least 24 h. Notably, the expression was well pronounced only for cap-1 and 100%-m1Ψ containing transcripts in the case of dendritic cells, where the innate immune receptors are known to be especially active (Figure S1). These results are consistent with previous studies, where mRNA-transfected cells demonstrated a similar dynamics of the Fluc activity (30, 31). However, we would like to note that the continuous real-time measurement of the luciferase activity in living cells we applied (25) provides a more accurate and comprehensive analysis of mRNA expression than the end-point measurements (implying cell lysis) regularly used by others.
Next, we evaluated the effects of the Fluc-mRNA in vivo using LNP delivery system. Recent advances demonstrated that LNP provides a wide range of possibilities for researchers in molecular therapeutics. LNP has been specifically developed for delivery into the liver (32) and has previously been shown to be very effective for this purpose, providing robust protein synthesis (31, 33). In this study we used ionizable lipid ALC-0315 combined with DSPC, cholesterol, and a PEG–lipid, a mixture similar to the delivery system used in the Pfizer-BioNTech COVID-19 vaccine (34). We observed the high level and prolonged expression of Fluc mRNA formulated into LNPs injected through intravenous and intramuscular routes in mice.
As expected, i.v. route allowed obtaining the highest values of luciferase activity in the liver for the entire study period (Figures 1C, D). Luminescence was already observed at 15 min after injection and continued about 24 h followed by a decrease. Whereas i.m. injection led to moderate expression, it produced long lasted results: we could detect luminescence for about 3 weeks in the injection site and up to 48 h in the liver (Figures 1C, E; Figure S2). Therefore, for our further purposes related to passive immunotherapy, intramuscular administration of mRNA encoding the anti-BoNT/A antibody seemed to be the most appropriate. In general, our data are in good agreement with previously reported results (31) with difference in translation duration, due to higher mRNA-LNP dose used in our study (10 vs. 5 µg per mice).
Next, we analyzed the in vivo effects of a similar mRNA construct encoding the B11-Fc antibody. This clone of the camelid sdAb fused with the human Fc fragment was described earlier (20). Injection of B11-Fc mAb 1 and 3 h in advance or 1 h after 10 LD50 BoNT/A administration led to 100% survival (20). Pharmacokinetics study shows that 10 μg of B11-mRNA-LNP administration per mouse allows to achieve profile comparable to direct 100 μg B11-Fc antibody injection (20).
Our results of the experiment with BoNT/A challenge confirmed that mRNA technology can be used for passive immunization against botulism. According to our prophylaxis scheme, we have established the time (3.5 h) after B11-mRNA-LNP administration, at which the protective effect was observed though mild symptoms of intoxication began already to show (Figure 2C). The presence of clinical manifestations of mild severity indicates that a further reduction in 3.5-hour interval between treatment and challenge may lead to a lethal outcome. Furthermore, we demonstrated that 48-hour mRNA translation ensured the 100% survival even following 100 LD50 BoNT/A exposure (Figure 2F).
Previously, it has been demonstrated that expression of B11-Fc antibody, delivered via recombinant adeno-associated virus (rAAV-B11-Fc), can protect animals from lethal dose (10 LD50) of BoNT/A, starting only from day 3 after administration (11). Moreover, rAAV-B11-Fc did not ensure survival against higher doses of BoNT/A (50 and 100 LD50) (11). In a recent study, Thran and co-authors showed therapeutic potential of mRNA-mediated antibody expression for animal protection against a number of toxins including BoNT/A (12), challenging mice with 4 LD50 of BoNT/A. Our data showed that passive immunization with B11-mRNA-LNP provides rapid (as early as 3.5 h) and robust protective antibody production sufficient to neutralize even significantly higher dose of BoNT/A, which is crucial for emergency prevention. Taken together, these results indicate that mRNA-mediated nanobody expression can be used for both therapeutic and prophylactic purposes in BoNT/A challenge.
It’s also worth noting that during preclinical studies of a protein therapeutic agent based on B11-Fc monoclonal antibodies for the treatment of botulinum toxin intoxication, anti-drug antibodies were evaluated after systemic administration of the drug. As a result of studies, an insignificant titer of anti-drug antibodies was detected in the blood serum of animals to 60 days and was under limit of detection to 150 day of observation (11). Only Fc but not VHH portion of our sdAb was humanized. It is known that VHH humanization is additional way to improve Ab for subsequent application for human therapy (35) but it is still technically challenging because most VHHs do not have 3D structures (36).
Limitation
The performed study allows us to evaluate the prophylactic mode of antibodies action, while practical application may require therapeutic activity. Future experiments should allow to determine the possibility of using neutralizing antibodies in the form of mRNA to achieve a therapeutic effect against BoNT/A. Also we haven’t studied the immunogenicity of our mRNA delivered antibodies on the mouse model.
Conclusion
We have developed an mRNA platform for effective gene delivery in mammals. The platform allows long-term expression of mRNA after intramuscular and intravenous administration in mice. Injection of mRNA-LNP encoding the humanized single-domain VHH-Fc antibody specific for botulinum neurotoxin A provides protective activity as early as 3.5 h after administration. Thus, the mRNA-based approach for single-domain antibody delivery paves the way for the development of new immunotherapy drugs for emergency prevention.
Methods
Cloning, the production and purification of plasmid DNA, in vitro transcription
The coding regions for the firefly luciferase (from the vector pGL4.51[luc2/CMV/Neo] (Promega)) or the camelid anti-BoNT/A sdAb (clone B11) (20) were cloned into a vector for in vitro transcription (IVT) based on pJAZZ-OK linear bacterial plasmid (Figure S3A). For this, the ColE1 replication origin was first amplified from plasmid pTZ57R (Thermo Fisher Scientific), appended with the SmaI restriction site at one end, and inserted into pJAZZ-OK using BigEasy® v2.0 Linear Cloning Kit (Lucigen) to increase plasmid copy number (Figure S3B). The resulting plasmid was then digested with SmaI (Sibenzyme) and assembled with PCR product containing mRNA template elements (T7-promoter, the HBA1 5′ UTR appended with Kozak sequence (27), the HBA1 3′ UTRs, and 100-nt poly(A)-tail followed by BsmBI site) using Gibson Assembly® Ultra kit (Codex) (Figure S3C). Before the assembly, SmaI site was inserted between 5′ and 3′ UTRs using Overlap Extension PCR from pT7-5′Luc3′-pA template (unpublished) and BsmBI site in B11-Fc CDS was destroyed using the site-directed mutagenesis resulting in alternative synonymous codon for V193. Finally, after treatment of the resulting plasmid with SmaI, either Fluc or B11-Fc CDSs were cloned directly between 5′ and 3′ UTRs, also using Gibson assembly (Figure S3D), with the SmaI site elimination during this process. Cloning steps and plasmid production were performed using freshly prepared E. coli BigEasy™-TSA™ Electrocompetent Cells (Lucigen). All cloning procedures were confirmed by Sanger sequencing using BigDye® Terminator v3.1 Cycle Sequencing kit on 3500 Genetic Analyzer (Applied Biosystems). The primer sets used in this study listed in Table S1.
The pDNA for IVT were isolated and purified from a culture of the E. coli grown for 16 h in 250 ml of 2×YT medium using the Plasmid Maxi Kit (QIAGEN) in accordance with the manufacturer’s protocol. The pDNA digestion was performed using BsmBI-v2 restriction endonuclease (NEB), followed by purification of the linearized plasmid by phenol-chloroform extraction and ethanol precipitation. IVT was performed as described earlier (21), with the following modifications. 100-μl reaction volume contained 3 μg of DNA template, 3 μl T7 RNA polymerase (Biolabmix) and 10×Buffer (TriLink), 4 mM trinucleotide cap 1 analog ((3′-OMe-m7G)-5′-ppp-5′-(2′-OMeA)pG)) (Biolabmix), 5 mM m1ΨTP (Biolabmix) replacing UTP, and 5 mM GTP, ATP and CTP. After 2 h incubation at 37°C, 6 μl DNase I (Thermo Fisher Scientifiс) was added for additional 15 min, followed by mRNA precipitation with 2M LiCl (incubation for 1 h in ice and centrifugation for 10 min at 14,000 g, 4°C) and carefully washed with 80% ethanol. RNA integrity was assessed by electrophoresis in 8% denaturing PAGE.
Formulation of mRNA in LNP
In vitro transcribed mRNAs were encapsulated in LNPs using a self-assembly process in which an aqueous solution of mRNA at pH 4.0 is rapidly mixed with a solution of lipids dissolved in ethanol. LNPs used in this study were similar in composition to those described previously (18, 31, 34). Briefly, lipids were dissolved in ethanol at molar ratios of 46.3:9:42.7:1.6 (ionizable lipid:distearoyl PC:cholesterol:PEG-lipid) (34). Acuitas ionizable lipid (ALC-0315) and PEG-lipid (1,2-Dimyristoyl-sn-glycero-3-methoxypolyethylene glycol 2000) were purchased in Cayman Chemical Company. The lipid mixture was combined with an acidification buffer of 10 mM sodium citrate (pH 4.0) containing mRNA (0.2 mg/ml) at a volume ratio of 3:1 (aqueous: ethanol) using a Nanoassemblr Spark device (Precision NanoSystems). The ratio of ionizable nitrogen atoms in the ionizable lipid to the number of phosphate groups in the mRNA (N:P ratio) was set to 6 for each formulation. Formulations were dialyzed against PBS (pH 7.2) in Slide-A-Lyzer dialysis cassettes (Thermo Fisher Scientific) for at least 24 h. Formulations were concentrated using Amicon ultra-centrifugal filters (EMD Millipore), if needed, then passed through a 0.22-μm filter and stored at 4°C (PBS) until use. Formulations were tested for particle size, zeta potential, RNA encapsulation. All LNPs were found to be about 80 nm in size as measured by dynamic light scattering using a Zetasizer Nano ZS instrument (Malvern Panalytical). mRNA encapsulation was about 90% (measured by RiboGreen assay – Quant-iT™ RiboGreen™ RNA Reagent, Thermo Fisher Scientific).
Cell culture, transfection and luciferase measurements
HeLa and HEK293T cells were obtained from ATCC and cultured in DMEM (Gibco) at 37°C, in a 5% CO2 humidified atmosphere. Bone marrow derived dendritic cells (BMDCs) from C57BL/6 mice were differentiated from proliferating mouse bone marrow progenitors through induction with 20 ng/ml granulocyte macrophage colony stimulating factor (GM-CSF) (R&D Systems, USA) over 6–9 days as described (37). Briefly, mice were euthanized by CO2 overdose and the femurs and tibias were collected in ice-cold Hank’s balanced salt solution (HBSS, Sigma-Aldrich). The muscles were removed with a scalpel and by rubbing the bones with a tissue. The ends of the bones were then cut off with scissors and crushed. The bone marrow was flushed out with 2–3 ml of RPMI complete medium in a syringe with a 25-gauge needle. All bone marrow cells were collected and washed twice with HBSS. The bone marrow cells were cultured in 24-well plates containing approximately 5×105 cells/ml in 1 ml total volume. The cells were maintained at 37°C with 5% CO2 in complete RPMI medium with 10% heat inactivated fetal calf serum (PAA), 0.05 mM 2-mercaptoethanol (Thermo Fisher Scientific), 1×Non Essential Amino Acids (PanEco), 20 ng/ml GM-CSF, 2 mM glutamine, 100 U/ml penicillin, and 100 μg/ml streptomycin (all PanEco). After 24 hours, the nonadherent cells were collected and discarded, and 1 ml of fresh media was added to each well. On day 3, 5 and 7 half of the medium in each well was replaced with fresh medium. On day 7–9, the percentage of CD11c-positive cells in non-adherent population in the cultures was ~60–70%. Mouse primary fibroblasts (MPF) were derived from auricles according to the protocol (38). Briefly, the animals were euthanized, their auricles were collected and rinsed with 70% ethanol. Tissue fragments were dissected with sterile scissors in a FBS-free DMEM solution containing 1x Liberase TM Research Grade (Roche) and Antibiotic-Antimycotic (Gibco), and incubated at 37°C on a shaker for 1 h. The tissue fragments were then centrifuged thrice in 50 ml of growth media (DMEM/F12 with 1×Antibiotic-Antimycotic and 15% FBS (Gibco) for 5 min at 500 g, the pellet was resuspended in 10 ml of growth media and incubated for 7 days in a tissue culture dish at 37°C, 5% CO2. After 7 days the cells were trypsinized, plated in a new culture dish, and used for experiments within the first 12 population doublings.
Transfection with the Fluc encoding mRNA was performed essentially as described previously (25). Shortly, a day before transfection, ~3×104 cells per well were transferred into the white FB/HB 96-well plates (Greiner) in 75 μl of DMEM (Paneco) supplemented with 10% FBS (HyClone) in the presence of penicillin and streptomycin (Paneco). Next day, cell culture at ~70% confluency was transfected with the reporter mRNA. For this, 30 ng of mRNA in 20 μl Opti-MEM (Gibco) per well was mixed with a solution of 0.06 μl of GenJector™-U (Molecta) in 3 μl Opti-MEM (per well), incubated for 15 min, then supplied with 0.4 μl of 100 mM D-luciferin (Promega) per well, and then 23 μl of the mixture were added to the cells. During these steps, all manipulations were performed as described in the FLERT protocol (22), providing a rapid and non-stressful transfection procedure. Real-time luminescence measurements were carried out overnight in the CLARIOstar plate reader (BMG Labtech) equipped with Atmospheric Control Unit to maintain 5% CO2, at 37°C (signal integration time – 5 s). All the experiments were repeated at least three times (including ones with different cell passages), the mean values ± SD were calculated.
Animal experiments
All animal experiments were performed in accordance to the Directive 2010/63/EU (Directive 2010/63/EU of the European Parliament and of the Council of 22 September 2010 on the Protection of Animals Used for Scientific Purposes. Off J Eur Communities L276:33–79), FELASA recommendations (39), and the Inter-State Standard of «GLP» (GOST - 33044-2014) approved by the Institutional Animal Care and Use Committee (IACUC) of the Federal Research Centre of Epidemiology and Microbiology named after Honorary Academician N.F. Gamaleya and were performed under Protocol #22 from 15 April 2022. All persons using or caring for animals in research underwent training annually as required by the IACUC.
Females BALB/c mice, 5 – 6-week-old, weighting 18 – 20 g were randomly allocated into groups. Fluc-mRNA LNPs in PBS, or PBS (for placebo group) were injected by two ways of administration, intravenous and intramuscular, in dose 10 μg mRNA per mouse. Following 30 min, 3 and 24 h after Fluc mRNA LNPs injection, 2.5 μg of D-luciferin was injected intraperitoneally. For intramuscular administration, bioluminescence was measured for 24 days (additional points – 2, 3, 4, 7, 10, 14, 21, 24 days). Then, after 5 min exposition, bioluminescence in mice was visualized using IVIS Lumina II (Life sciences) under isoflurane anesthesia. Animals (n = 3 per group) that were not used for in vivo visualization, were euthanized by CO2 inhalation with subsequent isolation of internal organs. The firefly luciferase activity was detected in homogenates of organs by luminescence measurements using Synergy H4 hybrid reader (Bio-Tek) and Bright-Glo Luciferase Kit (Promega).
Botulinum toxin A preparation
Botulinum toxin A was obtained using C. botulinum strain A98. The strain was cultivated under anaerobic conditions for five days. Bacterial suspension was precipitated by centrifugation at 5000×g for 30 min at 10°C. Bacterial proteins from the filtrate were concentrated by trichloroacetic acid precipitation at pH = 3.8 for 45 min. The precipitate was separated by centrifugation at 12000×g for 30 min at 10°C and dissolved in 47 mM citrate-phosphate buffer with pH = 5.6. S300 gel filtration and ion-exchange chromatography were performed on the AKTA start system (GE Healthcare Life Science) with DE cellulose (Pharmacia). The toxin (150 kDa) was additionally purified by chromatography with DE cellulose (Pharmacia) in borate buffer with pH = 8 and eluted by 50 mM NaCl. The specific antigenic activity of BoNT/A was determined by reaction with monospecific antibodies to BoNT/A (Gamaleya Laboratory of Clostridiosis and commercial preparation of Scientific Centre for Expert Evaluation of Medicinal Products Russian Federation). Purified BoNT/A was checked by SDS-electrophoresis with 2-mercaptoethanol. The toxin was filtered through 0.22 μm syringe filter before injection. The BoNT/A concentration was determined spectrophotometrically (UV 280 nm) and calorimetrically (Bradford protein analysis). LD50 was determined on six-week-old female BALB/c mice (weighing 18 – 20 g). Specific activity was determined by the standard mouse lethality assay described by Lindstrom and Korkeala (40). The specific activity ranged from 10 – 30 pg/mouse among lots of BoNT/A. Variations in the specific activity of BoNT/A was associated with different batches of animals and BoNT/A. Therefore, we checked the specific toxic activity before each experiment and for each batch of animals and the toxin.
Challenge experiments
Five- to six-week-old BALB/c mice weighting 18 – 20 g were randomly allocated into groups (three animals per group). Mice from all groups were housed in standard isolator cages under standard conditions and were challenged with 5 LD50 (or 10, 50, 100 LD50) dose of intraperitoneal injection of BoNT/A. For prophylactic treatment a single intramuscular injection of LNP-formulated B11-mRNA, or PBS (for negative control group), or recombinant B11-Fc antibody (20) in a dose of 0.5 mg/kg (for positive control group) were administered 0/3.5/8/24 h before BoNT/A challenge. For high dose survival study B11-mRNA-LNP was injected 24 and 48 h before challenge. Mice were monitored for clinical symptoms at intervals: 0, 4, 8, 26, 48 and 72 h after challenge. Assessment of clinical symptoms was carried out in accordance with the scale: score of 0 (no symptoms), score of 1 (mild symptoms), score of 2 (moderate symptoms), score of 3 (severe symptoms = humane endpoint). Time of death was defined as the time at which a mouse was found dead or was euthanized via carbon dioxide asphyxiation followed by cervical dislocation at humane endpoint.
Evaluation of pharmacokinetic of B11-Fc antibody expressed via in vivo B11-mRNA-LNP injection
A total of 27 BALB/c female mice (18–20 g.) were treated with a single intramuscular injection of B11-mRNA-LNP with a dose of 10 μg/mouse, with three animals per group. The blood was collected from the facial vein before B11-mRNA-LNP injection (point 0) and 2, 4, 8, 24, 72, 96, 120 and 144 hours after B11-mRNA-LNP injection. Serum was collected and stored at−20°C until further analysis. Serum samples were taken from three animals per time point. The concentration of B11-Fc antibody in collected serum samples was measured by ELISA kit IgG total-EIA-BEST (Vector-Best, Russia); 10 μl/well of serum was added. The analysis was performed according to the manufacturer’s instructions except second for antibodies, which were changed to ECL Human IgG, HRP-linked whole Ab (Cytiva, NA933-1ML), and antibody standards, which were changed to purified B11-Fc antibody.
Statistical analysis
Data were analyzed using GraphPad Prism software version 8.
Data availability statement
The raw data supporting the conclusions of this article will be made available by the authors, without undue reservation.
Ethics statement
The animal study was reviewed and approved by Institutional Animal Care and Use Committee (IACUC) of the Federal Research Centre of Epidemiology and Microbiology named after Honorary Academician N.F. Gamaleya.
Author contributions
VAG and DYL designed and conceived the study. EAP, DAK, ENB, EPM, ASD, ANZ, AAD, IBE, IIS, EVU, IAI and TSZ performed the experiments. EVU, IAI, TSZ, DVS, ANN and SED provided methodology. EAP, DAK, ENB, EPM ASD, ANZ, AAD, IBE, IIS, TSZ, SED, VAG and DYL performed the investigation. VAG and DYL are project administrators. VAG, DYL and ALG provided funding. DAK, ENB, EPM and VAG wrote the manuscript. DAK, ENB, EPM, AAD, IBE, DVS, TSZ, SED, BSN, VAG and DYL reviewed the manuscript.
Funding
This research was funded by National Research Centre for Epidemiology and Microbiology named after Honorary Academician N F Gamaleya (from the income-generating activities) and the grant #121102500071-6 provided by the Ministry of Health of the Russian Federation, Russia.
Acknowledgments
We are grateful to Timofey A. Remizov and Anastasia A. Zakharova for their help with the purchase of reagents and paperwork for ongoing projects. We thank ANN for his valuable advice and materials necessary for setting up an BoNT/A challenge animal model. We are also grateful to Maria Konopleva for assistance with cell culture. EAP, IIS, and SED are members of the Interdisciplinary Scientific and Educational School of Moscow University “Molecular Technologies of Living Systems and Synthetic Biology”.
Conflict of interest
The authors declare that the research was conducted in the absence of any commercial or financial relationships that could be construed as a potential conflict of interest.
Publisher’s note
All claims expressed in this article are solely those of the authors and do not necessarily represent those of their affiliated organizations, or those of the publisher, the editors and the reviewers. Any product that may be evaluated in this article, or claim that may be made by its manufacturer, is not guaranteed or endorsed by the publisher.
Supplementary material
The Supplementary Material for this article can be found online at: https://www.frontiersin.org/articles/10.3389/fimmu.2023.1098302/full#supplementary-material
Supplementary Figure 1 | The influence of the presence of a cap1/cap0 structure and incorporation of modified nucleoside, N1-methylpseudouridine (m1Ψ), on the firefly luciferase mRNA expression in mouse bone marrow-derived dendritic cells (mBMDC).
Supplementary Figure 2 | Representative IVIS images of BALB/c mice injected with 5.0 µg mRNA-LNP in PBS (right and middle mouse), or PBS (left mouse) by intramuscular (i.m.) route. Relative luminescence plot is shown, and the scale of luminescence is indicated.
Supplementary Figure 3 | Cloning strategy for IVT plasmid templates based on pJAZZ-OK (Details described in Methods section 1). (A). Schematic of commercial pJAZZ-OK linear bacterial plasmid derived from N15 bacteriophage genome (telN – protelomerase gene, repA – replication factor gene and origin of replication, cB – the primary replication repressor protein, KanR – kanamycin resistance gene, black semi-circles denoting terminal hairpin loops); (B). Schematic of linear plasmid with ColE1 origin of replication (ori, yellow arrow) followed by SmaI restriction site (grey block); (C) Schematic of plasmid contained backbone sequences for IVT: T7-promoter (T7P, white triangle), human HBA1 5′ and 3′ UTRs (blue and plum blocks, respectively) cleaved by SmaI site, 2x strong translation terminator (Stop, red octagon), 100-nt poly(A)-tail (orange block) followed by BsmBI restriction site (cyan rhombus); (D) Schematic of resulted linear plasmid with open reading frame of luciferase or B11 antibody (GOI ORF, green arrow).
Supplementary Table 1 | The primer sets used in the study.
References
1. Goonetilleke A. Clostridial neurotoxins. J Neurol Neurosurg Psychiatry (2004) 75:iii35–9. doi: 10.1136/jnnp.2004.046102
2. Franz DR. Clinical recognition and management of patients exposed to biological warfare agents. JAMA: J Am Med Assoc (1997) 278:399–411. doi: 10.1001/jama.278.5.399
3. Dolly JO, Aoki KR. The structure and mode of action of different botulinum toxins. Eur J Neurol (2006) 13:1–9. doi: 10.1111/j.1468-1331.2006.01648.x
4. U.S. Food and Drug Administration. Available at: https://www.fda.gov/media/78196/download.
5. World Health Organization. Botulism (2018). Geneva, Switzerland: World Health Organization. Available at: https://www.who.int/news-room/fact-sheets/detail/botulism (Accessed 11 December 2018).
6. Rasetti-Escargueil C, Avril A, Miethe S, Mazuet C, Derman Y, Selby K, et al. The European AntibotABE framework program and its update: Development of innovative botulinum antibodies. Toxins (2017) 9:309. doi: 10.3390/toxins9100309
7. Yu R, Wang S, Yu Y-Z, Du W-S, Yang F, Yu W-Y, et al. Neutralizing antibodies of botulinum neurotoxin serotype a screened from a fully synthetic human antibody phage display library. SLAS Discov (2009) 14:991–8. doi: 10.1177/1087057109343206
8. Hamers-Casterman C, Atarhouch T, Muyldermans S, Robinson G, Hammers C, Songa EB, et al. Naturally occurring antibodies devoid of light chains. Nature (1993) 363:446–8. doi: 10.1038/363446a0
9. van der Linden RHJ, Frenken LGJ, de Geus B, Harmsen MM, Ruuls RC, Stok W, et al. Comparison of physical chemical properties of llama VHH antibody fragments and mouse monoclonal antibodies. Biochim Biophys Acta (BBA) - Protein Structure Mol Enzymol (1999) 1431:37–46. doi: 10.1016/S0167-4838(99)00030-8
10. Van Hoecke L, Roose K. How mRNA therapeutics are entering the monoclonal antibody field. J Transl Med (2019) 17:54. doi: 10.1186/s12967-019-1804-8
11. Derkaev AA, Ryabova EI, Esmagambetov IB, Shcheblyakov DV, Godakova SA, Vinogradova ID, et al. rAAV expressing recombinant neutralizing antibody for the botulinum neurotoxin type a prophylaxis. Front Microbiol (2022) 13:960937. doi: 10.3389/fmicb.2022.960937
12. Thran M, Mukherjee J, Pönisch M, Fiedler K, Thess A, Mui BL, et al. mRNA mediates passive vaccination against infectious agents, toxins, and tumors. EMBO Mol Med (2017) 9:1434–47. doi: 10.15252/emmm.201707678
13. Miao L, Zhang Y, Huang L. mRNA vaccine for cancer immunotherapy. Mol Cancer (2021) 20:41. doi: 10.1186/s12943-021-01335-5
14. Weissman D. mRNA transcript therapy. Expert Rev Vaccines (2015) 14:265–81. doi: 10.1586/14760584.2015.973859
15. Sahin U, Karikó K, Türeci Ö. mRNA-based therapeutics — developing a new class of drugs. Nat Rev Drug Discov (2014) 13:759–80. doi: 10.1038/nrd4278
16. Pardi N, Hogan MJ, Porter FW, Weissman D. mRNA vaccines — a new era in vaccinology. Nat Rev Drug Discov (2018) 17:261–79. doi: 10.1038/nrd.2017.243
17. Barbier AJ, Jiang AY, Zhang P, Wooster R, Anderson DG. The clinical progress of mRNA vaccines and immunotherapies. Nat Biotechnol (2022) 40:840–54. doi: 10.1038/s41587-022-01294-2
18. Kose N, Fox JM, Sapparapu G, Bombardi R, Tennekoon RN, de Silva AD, et al. A lipid-encapsulated mRNA encoding a potently neutralizing human monoclonal antibody protects against chikungunya infection. Sci Immunol (2019) 4:eaaw6647. doi: 10.1126/sciimmunol.aaw6647
19. Van Hoecke L, Verbeke R, De Vlieger D, Dewitte H, Roose K, Van Nevel S, et al. mRNA encoding a bispecific single domain antibody construct protects against influenza a virus infection in mice. Mol Ther - Nucleic Acids (2020) 20:777–87. doi: 10.1016/j.omtn.2020.04.015
20. Godakova, Noskov, Vinogradova, Ugriumova, Solovyev, Esmagambetov, et al. Camelid VHHs fused to human fc fragments provide long term protection against botulinum neurotoxin a in mice. Toxins (2019) 11:464. doi: 10.3390/toxins11080464
21. Dmitriev SE, Andreev DE, Terenin IM, Olovnikov IA, Prassolov VS, Merrick WC, et al. Efficient translation initiation directed by the 900-nucleotide-long and GC-rich 5’ untranslated region of the human retrotransposon LINE-1 mRNA is strictly cap dependent rather than internal ribosome entry site mediated. Mol Cell Biol (2007) 27:4685–97. doi: 10.1128/MCB.02138-06
22. Akulich KA, Andreev DE, Terenin IM, Smirnova VV, Anisimova AS, Makeeva DS, et al. Four translation initiation pathways employed by the leaderless mRNA in eukaryotes. Sci Rep (2016) 6:37905. doi: 10.1038/srep37905
23. Karikó K, Muramatsu H, Welsh FA, Ludwig J, Kato H, Akira S, et al. Incorporation of pseudouridine into mRNA yields superior nonimmunogenic vector with increased translational capacity and biological stability. Mol Ther (2008) 16:1833–40. doi: 10.1038/mt.2008.200
24. Andries O, Mc Cafferty S, De Smedt SC, Weiss R, Sanders NN, Kitada T. N(1)-methylpseudouridine-incorporated mRNA outperforms pseudouridine-incorporated mRNA by providing enhanced protein expression and reduced immunogenicity in mammalian cell lines and mice. J Control Release (2015) 217:337–44. doi: 10.1016/j.jconrel.2015.08.051
25. Osterman IA, Wieland M, Maviza TP, Lashkevich KA, Lukianov DA, Komarova ES, et al. Tetracenomycin X inhibits translation by binding within the ribosomal exit tunnel. Nat Chem Biol (2020) 16:1071–7. doi: 10.1038/s41589-020-0578-x
26. Ramanathan A, Robb GB, Chan S-H. mRNA capping: biological functions and applications. Nucleic Acids Res (2016) 44:7511–26. doi: 10.1093/nar/gkw551
27. Zeng C, Hou X, Yan J, Zhang C, Li W, Zhao W, et al. Leveraging mRNA sequences and nanoparticles to deliver SARS-CoV-2 antigens in vivo. Adv Mater (2020) 32:e2004452. doi: 10.1002/adma.202004452
28. Corbett KS, Edwards DK, Leist SR, Abiona OM, Boyoglu-Barnum S, Gillespie RA, et al. SARS-CoV-2 mRNA vaccine design enabled by prototype pathogen preparedness. Nature (2020) 586:567–71. doi: 10.1038/s41586-020-2622-0
29. Polack FP, Thomas SJ, Kitchin N, Absalon J, Gurtman A, Lockhart S, et al. Safety and efficacy of the BNT162b2 mRNA covid-19 vaccine. N Engl J Med (2020) 383:2603–15. doi: 10.1056/NEJMoa2034577
30. Phua KKL, Leong KW, Nair SK. Transfection efficiency and transgene expression kinetics of mRNA delivered in naked and nanoparticle format. J Controlled Release (2013) 166:227–33. doi: 10.1016/j.jconrel.2012.12.029
31. Pardi N, Tuyishime S, Muramatsu H, Kariko K, Mui BL, Tam YK, et al. Expression kinetics of nucleoside-modified mRNA delivered in lipid nanoparticles to mice by various routes. J Controlled Release (2015) 217:345–51. doi: 10.1016/j.jconrel.2015.08.007
32. Akinc A, Querbes W, De S, Qin J, Frank-Kamenetsky M, Jayaprakash KN, et al. Targeted delivery of RNAi therapeutics with endogenous and exogenous ligand-based mechanisms. Mol Ther (2010) 18:1357–64. doi: 10.1038/mt.2010.85
33. Sabnis S, Kumarasinghe ES, Salerno T, Mihai C, Ketova T, Senn JJ, et al. A novel amino lipid series for mRNA delivery: Improved endosomal escape and sustained pharmacology and safety in non-human primates. Mol Ther (2018) 26:1509–19. doi: 10.1016/j.ymthe.2018.03.010
34. Pfizer-BioNTech covid-19 vaccine FDA EAU letter of authorization. Available at: https://www.fda.gov/media/144412/download (Accessed 14 December 2020).
35. Conrath K, Vincke C, Stijlemans B, Schymkowitz J, Decanniere K, Wyns L, et al. Antigen binding and solubility effects upon the veneering of a camel VHH in framework-2 to mimic a VH. J Mol Biol (2005) 350(1):112–25. doi: 10.1016/j.jmb.2005.04.050
36. Vishwakarma P, Vattekatte AM, Shinada N, Diharce J, Martins C, Cadet F, et al. VHH structural modelling approaches: A critical review. Int J Mol Sci (2022) 23(7):3721. doi: 10.3390/ijms23073721
37. Inaba K, Inaba M, Romani N, Aya H, Deguchi M, Ikehara S, et al. Generation of large numbers of dendritic cells from mouse bone marrow cultures supplemented with granulocyte/macrophage colony-stimulating factor. J Exp Med (1992) 176:1693–702. doi: 10.1084/jem.176.6.1693
38. Seluanov A, Vaidya A, Gorbunova V. Establishing primary adult fibroblast cultures from rodents. J Vis Exp (2010) 44:e2033. doi: 10.3791/2033
39. FELASA working group on revision of guidelines for health monitoring of rodents and rabbits, Mähler (Convenor) M, Berard M, Feinstein R, Gallagher A, Illgen-Wilcke B, et al. FELASA recommendations for the health monitoring of mouse, rat, hamster, guinea pig and rabbit colonies in breeding and experimental units. Lab Anim (2014) 48:178–92. doi: 10.1177/0023677213516312
Keywords: single-domain antibody, mRNA platform, BoNT/A inhibitor, VHH-Fc antibody, Clostridium botulinum
Citation: Panova EA, Kleymenov DA, Shcheblyakov DV, Bykonia EN, Mazunina EP, Dzharullaeva AS, Zolotar AN, Derkaev AA, Esmagambetov IB, Sorokin II, Usachev EV, Noskov AN, Ivanov IA, Zatsepin TS, Dmitriev SE, Gushchin VA, Naroditsky BS, Logunov DY and Gintsburg AL (2023) Single-domain antibody delivery using an mRNA platform protects against lethal doses of botulinum neurotoxin A. Front. Immunol. 14:1098302. doi: 10.3389/fimmu.2023.1098302
Received: 14 November 2022; Accepted: 30 January 2023;
Published: 14 February 2023.
Edited by:
Sicai Zhang, Northwest A&F University, ChinaCopyright © 2023 Panova, Kleymenov, Shcheblyakov, Bykonia, Mazunina, Dzharullaeva, Zolotar, Derkaev, Esmagambetov, Sorokin, Usachev, Noskov, Ivanov, Zatsepin, Dmitriev, Gushchin, Naroditsky, Logunov and Gintsburg. This is an open-access article distributed under the terms of the Creative Commons Attribution License (CC BY). The use, distribution or reproduction in other forums is permitted, provided the original author(s) and the copyright owner(s) are credited and that the original publication in this journal is cited, in accordance with accepted academic practice. No use, distribution or reproduction is permitted which does not comply with these terms.
*Correspondence: Denis Y. Logunov, ldy78@yandex.ru
†These authors have contributed equally to this work