- 1Department of General Surgery and Guangdong Provincial Key Laboratory of Precision Medicine for Gastrointestinal Tumor, Nanfang Hospital, The First School of Clinical Medicine, Southern Medical University, Guangzhou, Guangdong, China
- 2Department of Gastrointestinal and Hernia Surgery, Ganzhou Hospital-Nanfang Hospital, Southern Medical University, Ganzhou, Jiangxi, China
The tumor microenvironment (TME) is modified by its cellular or acellular components throughout the whole period of tumor development. The dynamic modulation can reprogram tumor initiation, growth, invasion, metastasis, and response to therapies. Hence, the focus of cancer research and intervention has gradually shifted to TME components and their interactions. Accumulated evidence indicates neural and immune factors play a distinct role in modulating TME synergistically. Among the complicated interactions, neurotransmitters, the traditional neural regulators, mediate some crucial regulatory functions. Nevertheless, knowledge of the exact mechanisms is still scarce. Meanwhile, therapies targeting the TME remain unsatisfactory. It holds a great prospect to reveal the molecular mechanism by which the interplay between the nervous and immune systems regulate cancer progression for laying a vivid landscape of tumor development and improving clinical treatment.
1 Introduction
Cancer, the leading cause of death worldwide, cannot simply be recognized as a single illness but as a manifold group of diseases with diverse causes. As same as blood and lymphatic vessels, nerve fibers transmit signaling molecules and convey nutrients in the tumor microenvironment (TME). Theories of angiogenesis and lymphangiogenesis in tumors thrive over the past decades, but the role of nerves in tumorigenesis is still little known. Similar to the former two, the process tumors stimulate nerve innervation is termed “neoneurogenesis” (1), yet the specific mechanism remains controversial. Some evidence demonstrates that tumor cells can exploit nerve-derived factors to create a favorable microenvironment for tumor survival. Simultaneously, tumors can also stimulate the regeneration of nerve fibers by releasing neurotrophic factors like nerve growth factor (NGF) and axon guidance molecules like netrin-1. Early in 1926, psychosocial factors were demonstrated to be involved in cancer incidence and progression (2). The released neurotransmitters and hormones from neuroendocrine cells transduce the same effects. β-adrenergic agonists or adrenaline showed dose-dependent increases in tumor metastases, while β-adrenergic antagonists and indomethacin synergistically blocked the effects of behavioral stress on lung tumor metastasis. In murine models of cancers, sympathectomy via chemical reagents or surgical way and genetic deletion of β2-adrenergic receptors (AR) repressed tumor development in the early stage. Besides, prostate tumor metastases can be abolished by blocking the stromal type 1 muscarinic receptor with medicine or genetic disruption (3), which is the same in a murine model of gastric cancer (4). Sensory neurons can play a role as well. For instance, a model of pancreatic ductal adenocarcinoma has demonstrated that sensory neuron ablation by neonatal injection of capsaicin alleviates tumorigenesis and progression (5).
The immune system is never the minor character in this tug-of-war competition. Stress or depression, the emotional feelings, always do not induce the generation of tumors directly but through psychoneuroimmunology (6). Intricate interplays between neurons and immune cells existed during pancreatitis and modulated inflammation and cancer growth (7). Under chronic stress or depression, a durative-activated hypothalamic-pituitary-adrenal(HPA) axis suppresses the immune response, contributing to tumor development and progression in multiple cancers (6). Specifically, stress and depression were both associated with decreased cytotoxic T cell and natural killer (NK) cell activities and hence influenced immune surveillance of tumors, underlying the increased clinical susceptibility to malignant tumors. In animal models, mental stress, such as swim stress, surgical stress, social confrontation, and hypothermia, led to increased lung metastasis from injected breast cancer cells by suppressing NK cell activity (8–11).
In this review, we focus on discussing the neurotransmitters in the TME and their roles in immune regulation and tumor growth, progression, metastasis, and invasion, as well as their potential opportunities in the clinical treatment of cancer.
2 Immunomodulatory neurotransmitters
Immunology has long been studied along with microbiology and pathology. It was generally identified as a self-regulated system by immunologists. Emerging evidence gradually makes it a consensus that the nervous system participates in immune modulation physiologically. As the dominant component, the central nervous system (CNS) regulates immune functions at the whole organism level, moreover, the peripheral nerve endings may also participate in modulating the CNS immune factors or the immune-related neuroendocrine mediators (12). Recently, a noteworthy shift in research focuses happened owing to the discovery that immune cells could produce and release neuroendocrine factors and neuromodulators by themselves (13).
The interactions between the neuroendocrine and immune systems imply a bidirectional circuit where the in-depth mechanism is still obscure. Neurotransmitters, the major modulators in the CNS and perineural system (PNS), have been recognized as potential signaling molecules linking the two major systems for maintaining homeostasis. A series of studies recognized the immunomodulatory function of neurotransmitters that transforms the course of cancer (Figure 1). The exact amount of neurotransmitters in total is hard to calculate, but probably over 100, meanwhile their receptors are nearly 1000 (14). Despite the diversity, these molecules can be categorized into two classes: small-molecule neurotransmitters and neuropeptides. Neuropeptides are transmitter molecules composed of 3 to 36 amino acids with neural activity. Amino acids like acetylcholine, glutamate, gamma-aminobutyric acid (GABA), and biogenic amines (including dopamine, norepinephrine, epinephrine, serotonin, and histamine) are much lower in molecular weight and recognized as the classical neurotransmitters. In general, small-molecule transmitters mediate rapid reaction, while neuropeptides are prone to modulating slower responses (15).
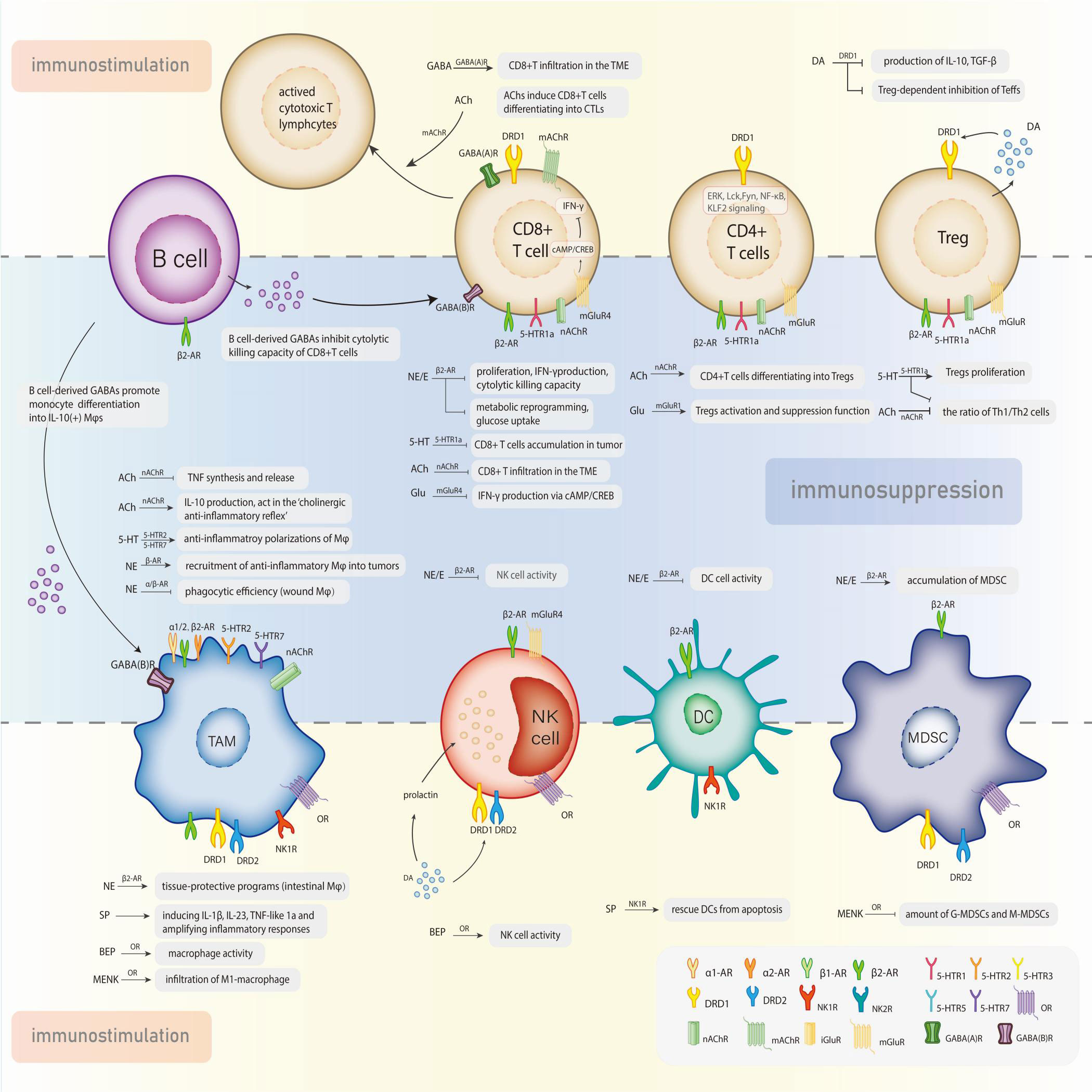
Figure 1 Neurotransmitters exert the dual effects in the modulation of tumor-associated immune cells via specific receptors.
2.1 Catecholamines
Catecholamines (CAs), the main effectors in the sympathetic nervous system (SNS), are tyrosine-originated biogenic amines and mediate the SNS-induced ‘fight-or-flight’ stress reaction. In response to psychological stress, SNS activation elevates catecholamines levels in circulation via the release of epinephrine from the adrenal medulla or norepinephrine spill-over from the neuro-muscular junction of sympathetic nerves (16–18). Generally, an acute SNS activation is beneficial but chronic stress is detrimental as it suppresses the activities of effector immune cells and activates the immunosuppressive cells (19). T cells, as well as macrophages and neutrophils, can synthesize catecholamines themselves and regulate their function in an autocrine/paracrine manner (20). Dopamine (DA), norepinephrine (noradrenaline, NE), and epinephrine (adrenaline, E) are all included.
On the other hand, growing evidence suggests that catecholamines play distinct roles in the regulation of angiogenesis (21–25), which has been clarified as DA inhibits tumor angiogenesis and stimulates tumor immunity while NE and E stimulate angiogenesis and inhibit immune functions in cancer (26).
2.1.1 Norepinephrine and epinephrine
Norepinephrine and epinephrine, known as stress excitatory neurotransmitters, are the main effectors in the sympathetic system. Activated by a stress reaction, they could stimulate muscle contraction, glycogen degradation, airway dilation, and stress-induced tumor progression as well (15).
Norepinephrine and epinephrine perform their functions through α1-,α2- and β-ARs on their target cells respectively. α1 -AR upregulates the intracellular calcium level but α2- AR decreases adenylate cyclase and inhibits intracellular cyclic AMP (cAMP), exerting the opposing functions. There are three subtypes of β-ARs, the G-protein-coupled receptors whose primary function is transmitting information from the extracellular environment to the interior cell and distributing it to the whole body (27). Associated signaling molecules have been summarized as β1- and β2- ARs increase intracellular cAMP by activating adenylate cyclase (27–33).
Both the innate and adaptive immune systems fight against the neoplasms. β-ARs are widely expressed in immune cells, including T lymphocytes, B lymphocytes, NK cells, monocyte/macrophage, and dendritic cells (DCs), of which the activation generally inhibits lymphocyte, NK cell, and DC responses (34, 35). Innate immune cells express the β2-, α1- and α2- ARs, while the β2 subtype is the main receptor on adaptive immune cells, except for Th2 cells (35, 36). Focus has long been on the influence of activated β2-ARs on CD4(+) T cells and B cells. Though CD8(+) T cells express three times the quantity of β2-ARs on CD4(+) T cells, it is still hard to elucidate how the β2-AR-mediated modulation acts in CD8(+) T cells—the backbone of adaptive immunity (37–40). Generally, β-adrenergic signaling significantly suppressed the function of antigen-specific CD8(+)T cells, including their proliferation, interferon-gamma (IFN-γ) production, and cytolytic killing capacity. This T-cell-selective inhibitory effect does not disturb innate lymphocyte responses (41). Moreover, blocked CD8(+) T cell metabolic reprogramming via β-adrenergic signaling decreased the glucose uptake of T cells and contributed to stress-induced immunosuppression (42). In addition to suppressing lymphocyte function directly, norepinephrine may downregulate anti-tumor response by favoring the accumulation of immunosuppressive cells, which can be abolished by propranolol in a murine spontaneous model of melanoma (43). As for innate immunity, activated β-AR decreases NK cell activity and permits tumor metastases in an animal model (44, 45). Physiologically, the regulation of NK cell function is closely related to SNS-mediated biological behaviors, such as circadian regulation, exercises, stress, and social engagement (46, 47), and rhythmic NE input to the rat spleen acts as the molecular clock of cellular activity in local NK cells (48). Mobilization and redistribution of NK cells can be motivated by epinephrine in the murine model with regular exercise, which depends on the secretion of IL-6 (49). Endogenous E and prostaglandins orchestrated the inhibition of cytotoxic T-lymphocyte and NK cell responses and promote leukemia progression in rats (50). The affected function of macrophages via adrenergic receptors varies under different circumstances. Both physiologic and pharmacologic doses of norepinephrine suppressed wound macrophage phagocytic efficiency through α- and β-AR signaling in a dose-dependent manner (51). With the recruitment of CD11b(+)F4/80(+) macrophages into tumors, the secretion of NE could increase the metastasis of breast cancer cells to distant sites, including the lymph nodes and lungs, without affecting the growth of primary tumors (52). However, intestinal macrophages enhanced tissue-protective programs on luminal bacterial infection via activated β2-ARs (53).
2.1.2 Dopamine
Dopamine is an inhibitory stress neurotransmitter in the brain and the precursor for norepinephrine and epinephrine synthesis as well. Though it does not translocate across the blood-brain barrier, dopamine can be detected in the urine, implying its derivation from peripheral tissues. At least three sources of dopamine have been identified: sympathetic neurons, adrenal medulla, and neuroendocrine cells.
Five different seven-transmembrane G-protein-coupled dopamine receptors(DRs) are categorized into two groups: D1 class (D1 and D5) and D2 (D2, D3, and D4) class of receptors on target cells (16, 54). Activated dopamine receptor D1 (DRD1) class increases intracellular cAMP, whereas the dopamine receptor D2 (DRD2) class inhibits intracellular cAMP (54).
Regulation mediated by diverse dopamine receptors is complicated in cancers. In breast and colon cancer preclinical models, dopamine made anti-cancer drugs efficient through an anti-angiogenic effect (55). In gastric cancer, activated DRD2 inhibits insulin-like growth factor (IGF)-I-induced tumor cell proliferation (56). However, the upregulation of DRD1 agitates tumor growth and meanwhile inhibits immunosuppression, but displays an anti-tumor effect in preclinical models (57, 58). DRD1 signaling promoted hepatocellular carcinoma (HCC) cell growth (59). Catecholamines release of CD4(+)CD25(+) regulatory T lymphocytes (Tregs) decreased interleukin-10 (IL-10) and transforming growth factor-β (TGF-β) and inhibited Treg-dependent inhibition of effector-T lymphocytes(Teffs) proliferation, which is selectively reversed by pharmacological blockade of D1-like receptors (60).
SNS has an abundant innervation in the immune system, including most secondary lymphoid organs. Most immune cells or organs express DR, including the thymus and the immune effector cells(e.g., lymphocytes, monocytes, neutrophils, and DCs), suggesting its potential role in modulating the whole immune system (16, 61–65). Both central and peripheral DA have an impact on tumor growth and progression by unbalancing immune homeostasis (66–68). DA can stimulate the peritoneal macrophages, NK cells, and cytotoxic T cells to perform its anti-tumor function (16, 26, 62). Especially, DA has unique and opposite effects on T cell functions, which depends on different DRs level, composition, or dopamine response in various cell types. It was demonstrated that DA activates naïve or resting T cells by D1, D2, D3, and D5 receptors, but inhibits activated T cells by D1, D2, D3, D4, and D5 receptors (69, 70), making their function dynamic. Dopamine itself is a potent activator of resting effector T cells (Teffs) via two independent ways: direct Teffs activation and indirect Teffs activation by suppression of Tregs. Dopamine(~10-8M) activates resting or naïve Teffs(CD8(+) far outweighs CD4(+)) and affects Th1/Th2/Th7 differentiation via ERK, Lck, Fyn, NF-κB and KLF2 signaling cascades (71). However, dopamine in a physiological concentration can significantly inhibit the proliferation and cytotoxicity of CD4(+) and CD8(+) T cells in vitro, especially for CD8(+)T cells (72, 73). Except for being an effector, immune cells can be the initiator to secrete DA, such as Tregs (74) aimed at balancing immune homeostasis and influencing the course of disease (75). Activated Tregs produce more dopamine than Teffs in general. In addition, DA can indirectly affect tumor growth by regulating the production and release of prolactin (76–78), which regulates the function of NK cells and lymphokine-activated killer cells (79).
2.2 Serotonin/5-Hydroxytryptamine
5-Hydroxytryptamine (5-HT), also named serotonin, is a monoamine neurotransmitter synthesized in the serotonergic neurons within the CNS and the enterochromaffin cells of the intestine (80). More than 90% of the body’s 5-HT is synthesized by the intestine enterochromaffin cells and then stored in platelets. Besides cognitive and behavioral works in the CNS (81), 5-HT also exerts essential roles in peripheral aggregating platelets, provoking immune responses, promoting bone development, regulating insulin secretion, and sustaining systemic energy homeostasis (82, 83). Ovarian cancer progression due to chronic stress was significantly associated with decreased serotonin and inhibited by serotonin/HTR1E signaling (84).
5-HT performs its functions via seven different subtypes of receptors (5-HT1-7) coupled to multiple signaling pathways. All of the seven belong to the family of G-protein-coupled receptors except for 5-HT3—a ligand-gated ion channel. Gi/o receptors(5-HT1 and 5-HT5) coupled to adenylyl cyclase decreased cAMP. Gq/1 receptors(5-HT2) coupled to phospholipase C (PLC) promoted intracellular Ca2+ release. Gs receptors(5-HT4, 5-HT6, and 5-HT7) coupled to adenylyl cyclase increased cAMP mostly (81, 82).
The multiple effects of 5-HT on depression and the tumor is still far from conclusion. 5-HT itself modulated the macrophage polarization with a sustained anti-inflammatory state predominantly through 5-HT2BR and 5-HT7R (85). T cell lymphoma invasion and metastasis 2 (TIAM2) promoted colorectal tumorigenesis by maintaining a pro-inflammatory state via serotonin-induced immunomodulatory effects (86). 5-HT1aR induced an immunosuppressive environment in lung adenocarcinomas patients with depression by activating the p-signal transducer and activator of transcription 3(pSTAT3) and autophagy signaling, as well as upregulating its downstream PD-L1 molecules (87). Specifically, 5-HT1aR on T cells is critical for expanding the group of CD4(+)CD25(+)Foxp3(+) Treg cells and reducing the ratio of Th1/Th2 cells, and 5-HT1aR on tumor cells is inversely related to cytotoxic lymphocytes activity. Inhibition of platelet-derived peripheral serotonin is associated with decreased pancreatic and colorectal tumor growth in mice, increased CD8(+)T cell influx, and decreased PD-L1 expression in tumors (88).
2.3 Acetylcholine
Acetylcholine (Ach), a predominant neurotransmitter of the parasympathetic system, is synthesized and secreted by neurons or nonneuronal cells, such as epithelial cells, mesothelial cells, endothelial cells, immune cells, cancer cells, etc. Apart from the brain, peripheral organs also have an abundant cholinergic innervation, involving a complicated interplay between autonomic nerves and immune cells. Gautron L. et al. found cholinergic fibers in mice gut are close to immune cells, including macrophages, plasma cells, and T cells (89), suggesting a potential role of the cholinergic system in neuroimmune interaction.
Ach receptors can be classified into the nicotinic acetylcholine receptor (nAchR) and the muscarinic receptor (mAchR) (90). Muscarinic receptors provoke immune activities, including lymphocyte mitogenesis, cytotoxic responses, and mast-cell-derived cytokines release. Zimring JC et al. demonstrated M-1 muscarinic receptors improve CD8(+) T cells differentiating into cytolytic T lymphocytes (91). Through nicotinic receptors, acetylcholine inhibited the secretion of tumor necrosis factor (TNF) (92) and stimulated IL-10 production in macrophages in an auto/paracrine manner (93), implying its functional role in immunosuppression. α7nAChR and α4β2nAChR are the evolutionarily oldest nAChRs. α7nAChRs on cytokine-producing macrophages or other immune cells are regarded as the main mediator for the ‘cholinergic anti-inflammatory reflex’’, a prototypical vagus nerve circuit where a memory phenotype T cell population producing acetylcholine was identified (94). Mashimo M et al. identified that α7nAChRs expressed on antigen-presenting cells(APCs) downregulated T cells differentiation by impairing antigen processing, while those expressed on CD4(+) T cells upregulated differentiation into Tregs and Teffs, regulating the intensity of immune responses (95, 96). Activated α7nAChR also mediated PD-L1 expression in normal human bronchial epithelial cells (HBECs) via STAT3/NRF2 pathways (97). Another classical nAChR, α4β2nAChR, play opposing roles against α7nAChR in cancer development and progression (98). The two counterparts are in a delicate balance that can be easily broken when the synthesis or release of neurotransmitters or the expression of receptors alters in cancer.
Nonneuronal Ach has been identified as a regulator participating in cell proliferation, differentiation, apoptosis, migration, angiogenesis, and immune response (99–101). Especially, tumor cell-derived Ach can promote tumor progression in an autocrine manner. Wang, ZL et al. found that acetylcholine increased the self-renewal ability of CD133(+) thyroid cancer cells and promoted the expression of PD-L1 via the CD133-Akt pathway (102). The pro-tumoral effect of cholinergic signaling was triggered by perineural invasion by sustaining an immunosuppressive environment typical of a reduced CD8(+) T cell infiltration and Th1/Th2 ratio (103). Zhu, P et al. have demonstrated the stimulation of α5nAChR promoted PD-L1 expression and thus induced immune escape via the pSTAT3, Jab1 signaling in lung adenocarcinomas (104).
2.4 Glutamate
Glutamate, the principal CNS excitatory neurotransmitter, is associated with affective, sensory, motor, and synaptic plasticity, and is also engaged in learning and memory. Abundant glutamate in the TME nourishes cell growth facilitates tumor progression and suppresses anti-tumor immunity. However, some evidence emphasizes that glutamate is also essential for the development and activation of effector T cells to exert anti-tumor function in STK11-/Lkb1-deficient lung cancer (105).
Two classes of glutamate receptors have been identified: the metabotropic receptors(mGluRs) and the ionotropic receptors(iGluRs). According to sequence homology, and pharmacological and intracellular signaling mechanisms, the mGluRs, belonging to the superfamily of GPCRs, are further categorized into three groups. Group I mGluRs(mGluR1 and mGluR5) are coupled to the Gq proteins and their activation stimulates PLC. Whereas, group II(mGluR2 and mGluR3) and III(mGluR4, mGluR6, mGluR7 and mGluR8) are negatively coupled to adenylate cyclase (106). Based on structural similarities, the iGluRs are divided into three subgroups named by the type of synthetic agonist that activates them: N-methyl-D-aspartate(NMDA) receptors, α-amino-3-hydroxy-5-methyl-4-isoxazolepropionate(AMPA) receptors, and 2-carboxy-3-carboxymethyl-4-iso-propenylpyrrolidine(kainate) receptors (107).
Functional iGluRs and mGluRs expressed on normal, tumor, and autoimmune human T cells mediate the activation of many critical cell functions(e.g., adhesion, migration, proliferation), intracellular Ca2+ fluxes, and outward K+ currents, mainly under a low physiological 10-8M to 10-5M concentration of glutamate (108). Tumor-derived glutamate leads to peritumoral excitotoxic cell death and thus vacates space for tumor expansion (109–112). Metabotropic glutamate receptor 4(GRM4) plays a novel role in suppressing anti-tumor immunity. Perturbations of GRM4 strengthened the anti-tumor immunity by activating NK, CD4(+) T, and CD8(+) T cells. Specifically, GRM4(-/-) stimulated the IFN-γ production in CD8(+) T cells through cAMP/CREB protein-mediated pathway (113). Various cancers depend on glutamate to an unusual degree for its contribution to metabolic building blocks and the energy supply. Activated mGluR2 and mGluR3 signals promote U87MG human glioma cell growth in vivo (114). Downregulation of glutaminase(GLS)—the critical enzyme converting glutamine into glutamate and regulating glutathione synthesis—diminishes cell-autonomous tumorigenesis in an HCC mouse model (115). GLS1 repression promoted the therapeutic efficacy of anti-PD-L1 therapy with less arginase 1(+) myeloid cells and more CD8(+)/IFNγ(+)/granzyme B(+) T cells, which is also effective in an immune checkpoint blockade(ICB)-resistant mouse model (116). However, GLS2, identified as a p53 target gene, contributes to the p53 tumor suppression via its antioxidant and pro-apoptotic function (117).
An antiporter system on the cell surface can import cystine into cells with a 1:1 counter-transport of glutamate, regulating the processes of redox homeostasis and cell growth. Solute Carrier Family 7 Member 11(SLC7A11) or xCT, the light chain subunit of system , serves as the primary transporter (118). Physiologically, imported cystine and intracellular glutamine are converted into cysteine and glutamate respectively, serving as precursors for glutathione(GSH) synthesis, which protects cells from oxidative stress (119). Elevated extracellular glutamate derived from glioblastoma with overexpressed SLC7A11 stimulated the activation and suppressive function of Treg, and the expression of mGlutR1 (120). SLC7A11 repression can be a synergistic anti-tumor mechanism in combination with checkpoint blockade (121). IFN-γ secreted from CD8(+) T cell reduced GSH synthesis in fibroblasts through transcriptional repression of system via the JAK/STAT1 pathway, and ultimately abolished the ovarian tumor resistance to platinum-based chemotherapy (122). Similarly, Weimin Wang et al. found that PD-L1 blockade therapy-activated CD8 (+)T cell inhibited SLC7A11 expression, diminished the cystine intake into tumor cells, and hence accelerated tumor cell lipid peroxidation and ferroptosis through IFN-γ (123).
2.5 GABA
GABA, a primary inhibitory neurotransmitter in the CNS, is produced from glutamate by the glutamate decarboxylase 1/2 (GAD1/2) enzymes and is catabolized by GABA-transaminase (ABAT). GABA is also widely expressed in the peripheral endocrine organs, including the pituitary, pancreas, gastrointestinal tract, testes, ovaries, placenta, uterus, and adrenal medulla but at a lower level than in the brain (124, 125), and is upregulated in autoimmune diseases and certain solid tumors, such as gastric, pancreatic, and breast cancers (126–129). Three types of GABA receptors include the ionotropic receptors(GABA(A) and GABA(C)) and metabotropic receptors(GABA(B)), inducing different effects on cancer growth (130).
A 2021 publication in Nature has identified B cell-derived GABA promotes monocyte differentiation into IL-10(+) macrophages and limits anti-tumor immunity by inhibiting CD8(+) T cell killer function (131), establishing a suppressive TIME via modulating macrophage differentiation. Krummel DAP, et al. demonstrated that benzodiazepines, a drug that can enhance GABA(A)R-mediated anion transport, could depolarize melanoma cells and reduce tumor growth, as well as potentiate radiation and immune checkpoint inhibitor response by provoking direct anti-tumor activity and infiltration of CD8(+) T cell (132). Activated GABA(B) receptor shows contradictory effects on human cancer progression. Baclofen, a GABA(B) receptor agonist, inhibits human HCC growth through the downregulation of intracellular cAMP level and upregulation of p21(WAF1) (133). However, GABA(B) receptor 1 signaling impaired the colorectal tumor cells migration and invasion through blocked EMT and the hippo/YAP1 pathway (134). GABA(B) receptor activated by tumor-derived GABA inhibits GSK-3β activity, enhances β-catenin signaling, and leads to stimulation of tumor cell proliferation and suppression of CD8(+) T cell intratumoral infiltration, suggesting its distinct role of being targeted pharmacologically to reverse immunosuppression beyond its traditional function as a neurotransmitter (135).
2.6 Substance P
Substance P(SP), a member of the tachykinin family, is an eleven-amino acid neurotransmitter expressed in CNS or PNS and affects emotional behavior (15). SPs are expressed on the macrophage, neuronal, endothelial, and epithelial cells (136). SP acts on neurokinin-1/2 receptors(NK1/2R), and blocking the neurokinin-1 receptor(NK1R) can inhibit NK1/2R signaling for the treatment of anxiety and depression disorders (137). As the chief receptor for the tachykinin family peptides, NK1R, an inflammation-related G protein-coupled receptor, is widely expressed in the CNS and peripheral tissues. NK1Rs participate in physiological responses such as pain transmission, vasodilation, endocrine and paracrine factors secretion, and cell proliferation (138).
Generally, the stimulatory effects of SPs on immunity consist of accelerating lymphocyte proliferation and the activation of phagocytic cells, bone marrow, and platelets for cytotoxicity (139, 140). DCs, the target of immunotherapy protocols aimed at the stimulation of cellular immune responses, do not always function ex vivo. Signaling via NK1R can rescue DCs from apoptosis due to the lack of GM-CSF and IL-4 for ex vivo generation of immune-stimulatory DCs (141). Moreover, the interaction between SP and proinflammatory cytokines modulates the activation of an immune response. NK1R signaling inhibits IL-10 secretion and thus promotes immunostimulatory DCs capable of biasing type 1 immunity (142). To amplify inflammatory responses, SP may function on memory T cells at a local level by inducing the level of IL-1β, IL-23, and TNF-like 1a in monocytes (143).
SP is also a mitogen. Concerning tumor biology, SP stimulates tumor migration in the colon (144) or breast carcinoma cells (145) and induces chemotactic properties in small-cell lung carcinoma cells (146). SP via NK1R upregulated toll-like receptor-4 (TLR-4) and contributed to the increase of tumor cell biological activity (147). Anti-SP therapy could strongly suppress cell growth and induce apoptosis in breast, colon, or prostate cancer cell lines and decrease the steady state of Her2 and EGFR (148). NK1R antagonists can also suppress inflammation and metastasis of breast carcinoma cells metastasized into the liver (149). Aprepitant, a kind of NK1R antagonist, prevents macrophages from LPS-induced oxidative stress by reducing the production of ROS and the expression of NOX-4, which may modulate the oxidative state of the TIME (150). Concerning the few available evidence, it is hard to define the exact effects of SP or NKR on anti-tumor immunity now. Clinical administration of NK1R antagonists/agonists still requires abundant examinations.
2.7 Opioid peptide
Endorphin, encephalin, and dynorphin, known as endogenous opioids or opioid peptides, are processed from the precursor proopiomelanocortin via post-translational cleavage. Leucocyte subsets express proopiomelanocortin (151) and release the products at sites of inflammation, contributing toimmune regulation in pain control (152).
Opioid substances exerted a chief immunosuppressive effect on anti-tumor immunity according to early research (153). However, views differ among the subsequent studies. β-endorphin(BEP), a chemokine for immune cells and small-cell lung carcinoma cells (146), fights against cancers via inhibited SNS function and elevates peripheral NK cell and macrophage activities. The effects also involve alterations in the TME, including altered DNA repairs, cell-matrix adhesion, angiogenesis, and epithelial-mesenchymal transition (154). Sarkar, DK et al. transplanted in-vitro-generated BEP neurons into the hypothalamic of rats enduring breast carcinogenesis. The BEP neurons-transplanted rats displayed increased immune functions and reduced growth and metastasis of mammary carcinoma, such as activated peripheral NK cells and macrophage, higher anti-inflammatory cytokines, and lower inflammatory cytokines. The opiate antagonist naloxone, beta-receptor agonist metaproterenol, or nicotine acetylcholine receptor antagonist methyllycaconitine can all inactivate NK cells and macrophages, reversing the effects of anti-tumor metastasis (155). Chronic opioid use also alters human CD8(+) T cell subsets balance, including significant decreases in T effector memory RA(+) cells (156).
A clinical investigation on two independent samples involving 1,929 and 1,569 middle-aged women found that the low fasting plasma concentration of encephalin precursor (pro-ENK) is associated with an increased risk of future breast cancer in middle-aged and postmenopausal women (157). According to existing evidence, the function of opioid peptides varies in different cancer, such as methionine enkephalin (MENK) is reported to promote breast carcinoma cells migration (145) but inhibit the cell-cycle process of pancreatic, colon, and head and neck cancer cells (158). Tumor heterogeneity cannot be exclusive of the reason, but no matter the location, the roles of MENK in tumors invariably courted controversy. Multiple pieces of evidence have clarified that MENK exerts anti-tumor effects by enhancing anti-tumor immune response or directly inhibiting tumor cell proliferation (159, 160). In CRC, MENK elevated the M1-type macrophages and T cells infiltration and reduced the groups of myeloid-derived suppressor cells(MDSCs) and M2-type macrophages (159), contributing to a pro-inflammatory state. In a CRC murine model, MENK invigorated immune response by markedly suppressing MDSCs and strengthening T cell activities, thus preventing colon carcinoma progression, which brings light to the development of adjuvant therapy for tumors (160). However, a certain report emphasizes the pro-tumor role of MENK by inhibiting T and B cell proliferation, promoting tumor cell growth, and resulting in the desensitization of lymphocytes via opioid receptors (161).
3 Clinical opportunities of neurotransmitters in anti-tumor immunity
Immune cells within the TME, named tumor-associated immune cells(TAIs), can defend against proliferation aberrances or conversely induce variations, suggesting their dual role in modulating tumor progression, which generally involves neural stimulation. A highly activated metabolic and energy-consuming state in tumors makes the neuroimmune interaction network more complicated and intensive (Figure 2). The administration of β-blockers and antidepressants on cancer patients is initially for other complications besides cancer, such as hypertension, heart disease, stress, or depression. But with the expanded application, these drugs are demonstrated to influence tumor progression or prognosis. Several typical cases are listed below:
3.1 β-blockers
β-adrenergic receptors, the chief messengers of sympathetic functions, can activate adenylyl cyclase and accumulate the second messenger cAMP (162) along with accelerated tumor growth (163–165). Overexpressed β-ARs were found in breast and ovarian cancer cells (163, 166), and β2-AR was the dominant subtype on them. According to a large case-control study about prostate cancer patients with simultaneous anti-hypertensive medication, only β-blocker-applied groups have a significant association with reduced cancer risk (167). A cardiovascular patients cohort study showed that the administration of β-blockers resulted in a 49% decrease in cancer risk to never-using relatively (168). Whereas, there is no large population-based case-control study that has confirmed altered risk in invasive breast carcinoma with β-blockers use (169). Activated β2-ARs also enhance the IgE response via a PKA-dependent, p38 MAPK-mediated pathway (170). AR regulation is important for cancer vaccine therapy. The role of β2-AR in an effective DC-based cancer vaccination was evaluated in the murine E.G7-ovalbumin(OVA) model and turns out that blocking β2-AR together with the activation of TLR2 at the position of DC inoculation could either promote tolerogenesis or enhance anti-tumor effects (171).
Drug repurposing has been a hot issue in recent years. Concerning the immunomodulatory function mentioned above, β-blockers repurposing may improve the immunotherapies’ efficacy in cancer patients. Several retrospective epidemiological studies have concluded that cancer patients administrated with β-blockers tend to reach better outcomes in prostate, breast, and colorectal cancer (172–175). Similarly, in the murine model, administrating β-blockers can reverse immunosuppression and significantly improve the efficacy of response to checkpoint inhibitor immunotherapy (19). β-blockers can also regulate immune response by modulating the activation of MDSCs and their expression of immunosuppressive molecules(arginase-I and PD-L1). The immunosuppressive effects of MDSCs tend to be alleviated by treating β-blockers or reinforced by β-adrenergic agonists (176). β-blocker for the perioperative treatment of cancer patients abolished the postoperative immune suppression and reduced the risk of tumor metastasis (177–180) by recovering the decreased NK cells cytotoxicity after surgery (181, 182). With this combined method, β-blockers are still warranted because the main factor of surgery-induced recurrence is associated with the postoperative stress response (183).
3.2 Antidepressants
Antidepressant drugs are widely used for the clinical treatment of depressive symptoms in cancer patients, modulating tumor growth partly by targeting the immune system (184–186).
Monoamine oxidase A(MAO-A), an enzyme first discovered in the brain, can promote the degradation of monoamine neurotransmitters such as serotonin and dopamine (187). By inhibiting monoamine oxidase to increase available serotonin, MAO inhibitors(MAOIs) enhance anti-tumor T cell activity via autocrine serotonin signaling (188) and depolarize alternatively activated immunosuppressive tumor-associated macrophages(TAMs) through the reduction of ROS production (189), suggesting its promising role against tumor-induced immune resistance. With depolarizing TAMs, MAOI treatment could raise the efficacy of other ICB therapies by serving as a TME-engineering therapy. Unfortunately, due to overstimulated serotonin receptors in immunotherapeutic doses, MAOIs may induce aggressive behavioral side effects, which limits their application in anti-tumor therapies (190). Thus, a recent study established a nanoformulation MAOI phenelzine(PLZ) to optimize the administration of MAOIs (191).
Several investigations reveal that SSRIs may inhibit tumor growth through their immune-modulatory actions through the modulation of monoaminergic systems. Fluoxetine, a classic SSRI, significantly inhibits melanoma tumor growth with an increased mitogen-induced T cell proliferation (192) and suppresses the progression of lymphoma via restoring NK cell activity and cytotoxic T lymphocyte activity with no noticeable systemic toxicity (193). Fluoxetine also reduced macrophage polarization in vivo by reversing tumor-induced oxidative damage and consequent oxidative stress in thymocytes (194, 195). Moreover, fluvoxamine significantly suppressed the migration and proliferation of tumor cells and prompted infiltration of T lymphocytes and M1-type macrophages with reduced PD-L1 molecules in colon cancer murine models (196). Sertraline recovered the T cell stress-induced deficiency by strengthening CD8(+) T cell infiltration, upregulating IFN-γ and Granular enzyme B(GzmB) levels, and reducing PD-1 on CD8(+) T cells, indicating its potential to raise the efficacy of ICB immunotherapy (197).
Tricyclic antidepressant imipramine enhanced autophagy in glioblastoma (GBM) cancer cells and surprisingly reprogrammed immunosuppressive TAMs by suppressing histamine receptor signaling to be immunostimulatory. The combination of imipramine with vascular endothelial growth factor (VEGF) pathway inhibitors orchestrated the infiltration and activation of T cells, supporting anti-PD-L1 therapeutic effects in several GBM mouse models (198).
3.3 DRD agonists or antagonists
DA has been demonstrated to play a protective role in cancer patients. According to several epidemiological studies, the compared incidents of cancer between Parkinson’s syndrome(a hypodopaminergic disease) (68) and schizophrenic patients with a probable hyperactive dopaminergic system (199, 200) show the decrease of dopamine are generally followed by higher cancer rates. Contrary to the controversial role of DRD1 in promoting tumor growth but also inhibiting immunosuppression, DRD1 agonists were proven to exert a major anti-tumor effect in several preclinical models (57, 58). Similarly, D1-like receptor agonists can potently inhibit the suppressive function of MDSC, suggesting that dopaminergic signaling tends to modulate tumor growth through strengthening anti-tumor immunity (201). An increased number of breast cancer has been observed in patients treated with DRD2 antagonists (202). However, paliperidone, a DRD2 antagonist, is reported to inhibit GBM growth and decrease the expression of programmed death-ligand 1(PD-L1) in GBM (203), suggesting different roles of DRD2 in different types of cancer.
3.4 Cancer immunotherapy and neurotransmitters
Cancer immunotherapy with ICB is based on the inhibition of tumor-mediated immune resistance, instead of directly exerting cytotoxic effects on tumor cells (204). Anti-programmed death-1(PD-1)/programmed death ligand-1(PD-L1) therapy, which circumvents T cell exhaustion due to the immunosuppressive TME by blocking PD-1/PD-L1 checkpoints binding, has been approved by the FDA as a clinical treatment for solid tumors. Considering the major role of T cells in immune defense, the scope of anti-PD-1/PD-L1 therapy is expanding rapidly in clinical practice. However, tumor immune resistance diminishes the efficacy of ICB considerably and becomes an urgent problem to be solved (205).
Neurotransmitters, which prompt immunosuppression, can be potential targets for abolishing immune resistance. For example, cholinergic signaling mainly upregulated the expression of PD-L1 and thus mediated immune escape in vitro, inducing an immunosuppressive environment characterized by impaired CD8(+) T cell infiltration and a reduced Th1/Th2 ratio (102, 104). Benzodiazepines, a GABA(A)R activator, potentiated radiation, and ICB response by promoting direct anti-tumor activity and infiltration of CD8(+) T cell (132). Several neural signals show the potential to improve the efficacy of ICB as an adjuvant therapy. In breast cancer, sympathetic denervation surprisingly downregulated the expression of immune checkpoint molecules (PD-1, PD-L1, and FOXP3) (206). In an experimental murine model, the inhibition of β-AR signaling favored an immune-active TME with increased infiltration of CD8(+) T cells, elevated Teffs cell to Tregs cell ratio, and decreased expression of PD-1, which raises the efficacy of anti-PD-1 checkpoint blockade (207). Further research on the involvement of neurotransmitters in TME immunomodulation will be of great interest in improving the efficiency of cancer immunotherapies in the future.
In this review, we discussed the modulatory function of the neurotransmitters in the tumor immune microenvironment (TIME) and their promising application in tumor treatment (Table 1). With the further exploration of neuroimmune interactions in the TME, we expect to approach the opportunities for the clinical application of related inhibitors or agonists.
Author contributions
LX, XL, CF, JY, and TC contributed to the conception and design of the study. LX wrote the first draft of the manuscript. XL, JY, and TC wrote sections of the manuscript. LX, XL, and CF designed the table and figures. All authors contributed to manuscript revision, read, and approved the submitted version.
Funding
The National Natural Science Foundation General Project (82272087), Guangdong Natural Science Foundation Outstanding Youth Project (2021B1515020055), Guangdong Provincial Key Laboratory of Precision Medicine for Gastrointestinal Cancer (2020B121201004).
Conflict of interest
The authors declare that the research was conducted in the absence of any commercial or financial relationships that could be construed as a potential conflict of interest.
Publisher’s note
All claims expressed in this article are solely those of the authors and do not necessarily represent those of their affiliated organizations, or those of the publisher, the editors and the reviewers. Any product that may be evaluated in this article, or claim that may be made by its manufacturer, is not guaranteed or endorsed by the publisher.
References
1. Mancino M, Ametller E, Gascon P, Almendro V. The neuronal influence on tumor progression. Biochim Et Biophys Acta-Reviews Cancer (2011) 1816(2):105–18. doi: 10.1016/j.bbcan.2011.04.005
3. Magnon C, Hall SJ, Lin J, Xue X, Gerber L, Freedland SJ, et al. Autonomic nerve development contributes to prostate cancer progression. Science (2013) 341(6142):1236361. doi: 10.1126/science.1236361
4. Zhao CM, Hayakawa Y, Kodama Y, Muthupalani S, Westphalen CB, Andersen GT, et al. Denervation suppresses gastric tumorigenesis. Sci Transl Med (2014) 6(250):250ra115. doi: 10.1126/scitranslmed.3009569
5. Saloman JL, Albers KM, Li D, Hartman DJ, Crawford HC, Muha EA, et al. Ablation of sensory neurons in a genetic model of pancreatic ductal adenocarcinoma slows initiation and progression of cancer. Proc Natl Acad Sci USA (2016) 113(11):3078–83. doi: 10.1073/pnas.1512603113
6. Reiche EM, Nunes SO, Morimoto HK. Stress, depression, the immune system, and cancer. Lancet Oncol (2004) 5(10):617–25. doi: 10.1016/S1470-2045(04)01597-9
7. Demir IE, Friess H, Ceyhan GO. Neural plasticity in pancreatitis and pancreatic cancer. Nat Rev Gastroenterol Hepatol (2015) 12(11):649–59. doi: 10.1038/nrgastro.2015.166
8. Ben-Eliyahu S, Yirmiya R, Liebeskind JC, Taylor AN, Gale RP. Stress increases metastatic spread of a mammary tumor in rats: evidence for mediation by the immune system. Brain Behav Immun (1991) 5(2):193–205. doi: 10.1016/0889-1591(91)90016-4
9. Ben-Eliyahu S, Page GG, Yirmiya R, Shakhar G. Evidence that stress and surgical interventions promote tumor development by suppressing natural killer cell activity. Int J Cancer (1999) 80(6):880–8. doi: 10.1002/(SICI)1097-0215(19990315)80:6<880::AID-IJC14>3.0.CO;2-Y
10. Page GG, Ben-Eliyahu S. A role for NK cells in greater susceptibility of young rats to metastatic formation. Dev Comp Immunol (1999) 23(1):87–96. doi: 10.1016/S0145-305X(98)00040-8
11. Melamed R, Rosenne E, Shakhar K, Schwartz Y, Abudarham N, Ben-Eliyahu S. Marginating pulmonary-NK activity and resistance to experimental tumor metastasis: suppression by surgery and the prophylactic use of a beta-adrenergic antagonist and a prostaglandin synthesis inhibitor. Brain Behav Immun (2005) 19(2):114–26. doi: 10.1016/j.bbi.2004.07.004
12. Dantzer R. Neuroimmune interactions: from the brain to the immune system and vice versa. Physiol Rev (2018) 98(1):477–504. doi: 10.1152/physrev.00039.2016
13. Zhang B, Vogelzang A, Miyajima M, Sugiura Y, Wu Y, Chamoto K, et al. B cell-derived GABA elicits IL-10(+) macrophages to limit anti-tumour immunity. Nature (2021) 599(7885):471–6. doi: 10.1038/s41586-021-04082-1
14. Spitzer NC, Borodinsky LN. Implications of activity-dependent neurotransmitter-receptor matching. Philos Trans R Soc Lond B Biol Sci (2008) 363(1495):1393–9. doi: 10.1098/rstb.2007.2257
15. Entschladen F, Drell TL, Lang K, Joseph J, Zaenker KS. Tumour-cell migration, invasion, and metastasis: navigation by neurotransmitters. Lancet Oncol (2004) 5(4):254–8. doi: 10.1016/S1470-2045(04)01431-7
16. Sarkar C, Basu B, Chakroborty D, Dasgupta PS, Basu S. The immunoregulatory role of dopamine: an update. Brain Behav Immun (2010) 24(4):525–8. doi: 10.1016/j.bbi.2009.10.015
17. Bergquist J, Tarkowski A, Ekman R, Ewing A. Discovery of endogenous catecholamines in lymphocytes and evidence for catecholamine regulation of lymphocyte function via an autocrine loop. Proc Natl Acad Sci U S A. (1994) 91(26):12912–6. doi: 10.1073/pnas.91.26.12912
18. Goldstein DS, Eisenhofer G, Kopin IJ. Sources and significance of plasma levels of catechols and their metabolites in humans. J Pharmacol Exp Ther (2003) 305(3):800–11. doi: 10.1124/jpet.103.049270
19. Qiao GX, Chen MH, Bucsek MJ, Repasky EA, Hylander BL. Adrenergic signaling: a targetable checkpoint limiting development of the antitumor immune response. Front Immunol (2018) 9:15. doi: 10.3389/fimmu.2018.00164
20. Flierl MA, Rittirsch D, Huber-Lang M, Sarma JV, Ward PA. Catecholamines-crafty weapons in the inflammatory arsenal of Immune/Inflammatory cells or opening pandora’s box? Mol Med (2008) 14(3-4):195–204. doi: 10.2119/2007-00105.Flierl
21. Basu S, Nagy JA, Pal S, Vasile E, Eckelhoefer IA, Bliss VS, et al. The neurotransmitter dopamine inhibits angiogenesis induced by vascular permeability factor/vascular endothelial growth factor. Nat Med (2001) 7(5):569–74. doi: 10.1038/87895
22. Basu S, Sarkar C, Chakroborty D, Nagy J, Mitra RB, Dasgupta PS, et al. Ablation of peripheral dopaminergic nerves stimulates malignant tumor growth by inducing vascular permeability factor/vascular endothelial growth factor-mediated angiogenesis. Cancer Res (2004) 64(16):5551–5. doi: 10.1158/0008-5472.CAN-04-1600
23. Xia Y, Wei Y, Li ZY, Cai XY, Zhang LL, Dong XR, et al. Catecholamines contribute to the neovascularization of lung cancer via tumor-associated macrophages. Brain Behav Immun (2019) 81:111–21. doi: 10.1016/j.bbi.2019.06.004
24. Chakroborty D, Sarkar C, Basu B, Dasgupta PS, Basu S. Catecholamines regulate tumor angiogenesis. Cancer Res (2009) 69(9):3727–30. doi: 10.1158/0008-5472.CAN-08-4289
25. Peters MA, Walenkamp AM, Kema IP, Meijer C, de Vries EG, Oosting SF. Dopamine and serotonin regulate tumor behavior by affecting angiogenesis. Drug Resist Update (2014) 17(4-6):96–104. doi: 10.1016/j.drup.2014.09.001
26. Sarkar C, Chakroborty D, Basu S. Neurotransmitters as regulators of tumor angiogenesis and immunity: the role of catecholamines. J Neuroimmune Pharmacol (2013) 8(1):7–14. doi: 10.1007/s11481-012-9395-7
27. Cole SW, Sood AK. Molecular pathways: beta-adrenergic signaling in cancer. Clin Cancer Res (2012) 18(5):1201–6. doi: 10.1158/1078-0432.CCR-11-0641
28. Schuller HM. Neurotransmitter receptor-mediated signaling pathways as modulators of carcinogenesis. Prog Exp Tumor Res (2007) 39:45–63. doi: 10.1159/000100045
29. Thaker PH, Lutgendorf SK, Sood AK. The neuroendocrine impact of chronic stress on cancer. Cell Cycle (2007) 6(4):430–3. doi: 10.4161/cc.6.4.3829
30. Mayer SE. Adenyl cyclase as a component of the adrenergic receptor. in: molecular properties of drug receptors. Ciba Found Symp (1970) 43–58. doi: 10.1002/9780470719763.ch4
31. Thaker PH, Han LY, Kamat AA, Arevalo JM, Takahashi R, Lu C, et al. Chronic stress promotes tumor growth and angiogenesis in a mouse model of ovarian carcinoma. Nat Med (2006) 12(8):939–44. doi: 10.1038/nm1447
32. Lutgendorf SK, Cole S, Costanzo E, Bradley S, Coffin J, Jabbari S, et al. Stress-related mediators stimulate vascular endothelial growth factor secretion by two ovarian cancer cell lines. Clin Cancer Res (2003) 9(12):4514–21.
33. Kamp T, Liebl B, Haen E, Emmerich B, Hallek M. Defects of beta 2-adrenergic signal transduction in chronic lymphocytic leukaemia: relationship to disease progression. Eur J Clin Invest (1997) 27(2):121–7. doi: 10.1046/j.1365-2362.1997.700623.x
34. Marino F, Cosentino M. Adrenergic modulation of immune cells: an update. Amino Acids (2013) 45(1):55–71. doi: 10.1007/s00726-011-1186-6
35. Nance DM, Sanders VM. Autonomic innervation and regulation of the immune system (1987-2007). Brain Behav Immun (2007) 21(6):736–45. doi: 10.1016/j.bbi.2007.03.008
36. Sanders VM, Baker RA, Ramer-Quinn DS, Kasprowicz DJ, Fuchs BA, Street NE. Differential expression of the beta2-adrenergic receptor by Th1 and Th2 clones: implications for cytokine production and b cell help. J Immunol (1997) 158(9):4200–10. doi: 10.4049/jimmunol.158.9.4200
37. Raskov H, Orhan A, Christensen JP, Gogenur I. Cytotoxic CD8(+)T cells in cancer and cancer immunotherapy. Br J Cancer (2021) 124(2):359–67. doi: 10.1038/s41416-020-01048-4
38. Farhood B, Najafi M, Mortezaee K. CD8(+) cytotoxic T lymphocytes in cancer immunotherapy: a review. J Cell Physiol (2019) 234(6):8509–21. doi: 10.1002/jcp.27782
39. Gajewski TF, Schreiber H, Fu YX. Innate and adaptive immune cells in the tumor microenvironment. Nat Immunol (2013) 14(10):1014–22. doi: 10.1038/ni.2703
40. Fan XL, Wang YD. Beta 2 adrenergic receptor on T lymphocytes and its clinical implications. Prog Nat Sci (2009) 19(1):17–23. doi: 10.1016/j.pnsc.2008.10.001
41. Nissen MD, Sloan EK, Mattarollo SR. Beta-adrenergic signaling impairs antitumor CD8(+) T-cell responses to b-cell lymphoma immunotherapy. Cancer Immunol Res (2018) 6(1):98–109. doi: 10.1158/2326-6066.CIR-17-0401
42. Qiao G, Bucsek MJ, Winder NM, Chen M, Giridharan T, Olejniczak SH, et al. Beta-adrenergic signaling blocks murine CD8(+) T-cell metabolic reprogramming during activation: a mechanism for immunosuppression by adrenergic stress. Cancer Immunol Immunother (2019) 68(1):11–22. doi: 10.1007/s00262-018-2243-8
43. Wrobel LJ, Bod L, Lengagne R, Kato M, Prevost-Blondel A, Le Gal FA. Propranolol induces a favourable shift of anti-tumor immunity in a murine spontaneous model of melanoma. Oncotarget (2016) 7(47):77825–37. doi: 10.18632/oncotarget.12833
44. Shakhar G, Ben-Eliyahu S. In vivo Beta-adrenergic stimulation suppresses natural killer activity and compromises resistance to tumor metastasis in rats. J Immunol (1998) 160(7):3251–8. doi: 10.4049/jimmunol.160.7.3251
45. Ben-Eliyahu S, Shakhar G, Page GG, Stefanski V, Shakhar K. Suppression of NK cell activity and of resistance to metastasis by stress: a role for adrenal catecholamines and beta-adrenoceptors. Neuroimmunomodulation (2000) 8(3):154–64. doi: 10.1159/000054276
46. Tarr AJ, Powell ND, Reader BF, Bhave NS, Roloson AL, Carson WE, et al. Beta-adrenergic receptor mediated increases in activation and function of natural killer cells following repeated social disruption. Brain Behav Immunity (2012) 26(8):1226–38. doi: 10.1016/j.bbi.2012.07.002
47. Kanemi O, Zhang X, Sakamoto Y, Ebina M, Nagatomi R. Acute stress reduces intraparenchymal lung natural killer cells via beta-adrenergic stimulation. Clin Exp Immunol (2005) 139(1):25–34. doi: 10.1111/j.1365-2249.2005.02672.x
48. Logan RW, Arjona A, Sarkar DK. Role of sympathetic nervous system in the entrainment of circadian natural-killer cell function. Brain Behav Immunity (2011) 25(1):101–9. doi: 10.1016/j.bbi.2010.08.007
49. Pedersen L, Idorn M, Olofsson GH, Lauenborg B, Nookaew I, Hansen RH, et al. Voluntary running suppresses tumor growth through epinephrine- and IL-6-Dependent NK cell mobilization and redistribution. Cell Metab (2016) 23(3):554–62. doi: 10.1016/j.cmet.2016.01.011
50. Inbar S, Neeman E, Avraham R, Benish M, Rosenne E, Ben-Eliyahu S. Do stress responses promote leukemia progression? an animal study suggesting a role for epinephrine and prostaglandin-E2 through reduced NK activity. PloS One (2011) 6(4):e19246. doi: 10.1371/journal.pone.0019246
51. Gosain A, Muthu K, Gamelli RL, DiPietro LA. Norepinephrine suppresses wound macrophage phagocytic efficiency through alpha- and beta-adrenoreceptor dependent pathways. Surgery (2007) 142(2):170–9. doi: 10.1016/j.surg.2007.04.015
52. Sloan EK, Priceman SJ, Cox BF, Yu S, Pimentel MA, Tangkanangnukul V, et al. The sympathetic nervous system induces a metastatic switch in primary breast cancer. Cancer Res (2010) 70(18):7042–52. doi: 10.1158/0008-5472.CAN-10-0522
53. Gabanyi I, Muller PA, Feighery L, Oliveira TY, Costa-Pinto FA, Mucida D. Neuro-immune interactions drive tissue programming in intestinal macrophages. Cell (2016) 164(3):378–91. doi: 10.1016/j.cell.2015.12.023
54. Missale C, Nash SR, Robinson SW, Jaber M, Caron MG. Dopamine receptors: from structure to function. Physiol Rev (1998) 78(1):189–225. doi: 10.1152/physrev.1998.78.1.189
55. Sarkar C, Chakroborty D, Chowdhury UR, Dasgupta PS, Basu S. Dopamine increases the efficacy of anticancer drugs in breast and colon cancer preclinical models. Clin Cancer Res (2008) 14(8):2502–10. doi: 10.1158/1078-0432.CCR-07-1778
56. Ganguly S, Basu B, Shome S, Jadhav T, Roy S, Majumdar J, et al. Dopamine, by acting through its D2 receptor, inhibits insulin-like growth factor-I (IGF-i)-induced gastric cancer cell proliferation via up-regulation of krüppel-like factor 4 through down-regulation of IGF-IR and AKT phosphorylation. Am J Pathol (2010) 177(6):2701–7. doi: 10.2353/ajpath.2010.100617
57. Sobczuk P, Lomiak M, Cudnoch-Jedrzejewska A. Dopamine D1 receptor in cancer. Cancers (2020) 12(11):22. doi: 10.3390/cancers12113232
58. Yang L, Yao Y, Yong L, Feng Y, Su H, Yao Q, et al. Dopamine D(1) receptor agonists inhibit lung metastasis of breast cancer reducing cancer stemness. Eur J Pharmacol (2019) 859:172499. doi: 10.1016/j.ejphar.2019.172499
59. Yan Y, Pan J, Chen Y, Xing W, Li Q, Wang D, et al. Increased dopamine and its receptor dopamine receptor D1 promote tumor growth in human hepatocellular carcinoma. Cancer Commun (Lond) (2020) 40(12):694–710. doi: 10.1002/cac2.12103
60. Cosentino M, Fietta AM, Ferrari M, Rasini E, Bombelli R, Carcano E, et al. Human CD4(+)CD25(+) regulatory T cells selectively express tyrosine hydroxylase and contain endogenous catecholamines subserving an autocrine/paracrine inhibitory functional loop. Blood (2007) 109(2):632–42. doi: 10.1182/blood-2006-01-028423
61. Basu S, Dasgupta PS, Lahiri T, Chowdhury JR. Uptake and biodistribution of dopamine in bone marrow, spleen and lymph nodes of normal and tumor bearing mice. Life Sci (1993) 53(5):415–24. doi: 10.1016/0024-3205(93)90645-J
62. Basu S, Dasgupta PS. Dopamine, a neurotransmitter, influences the immune system. J Neuroimmunol (2000) 102(2):113–24. doi: 10.1016/S0165-5728(99)00176-9
63. Ferrari M, Cosentino M, Marino F, Bombelli R, Rasini E, Lecchini S, et al. Dopaminergic D1-like receptor-dependent inhibition of tyrosine hydroxylase mRNA expression and catecholamine production in human lymphocytes. Biochem Pharmacol (2004) 67(5):865–73. doi: 10.1016/j.bcp.2003.10.004
64. McKenna F, McLaughlin PJ, Lewis BJ, Sibbring GC, Cummerson JA, Bowen-Jones D, et al. Dopamine receptor expression on human T- and b-lymphocytes, monocytes, neutrophils, eosinophils and NK cells: a flow cytometric study. J Neuroimmunol (2002) 132(1-2):34–40. doi: 10.1016/S0165-5728(02)00280-1
65. Nakano K, Higashi T, Takagi R, Hashimoto K, Tanaka Y, Matsushita S. Dopamine released by dendritic cells polarizes Th2 differentiation. Int Immunol (2009) 21(6):645–54. doi: 10.1093/intimm/dxp033
66. Basu S, Dasgupta PS. Role of dopamine in malignant tumor growth. Endocrine (2000) 12(3):237–41. doi: 10.1385/ENDO:12:3:237
67. Barnes NM, Gordon J. Harnessing serotonergic and dopaminergic pathways for lymphoma therapy: evidence and aspirations. Semin Cancer Biol (2008) 18(3):218–25. doi: 10.1016/j.semcancer.2007.12.007
68. Rubí B, Maechler P. Minireview: new roles for peripheral dopamine on metabolic control and tumor growth: let’s seek the balance. Endocrinology (2010) 151(12):5570–81. doi: 10.1210/en.2010-0745
69. Sarkar C, Basu B, Chakroborty D, Dasgupta PS, Basu S. The immunoregulatory role of dopamine: an update. Brain Behav Immunity (2010) 24(4):525–8. doi: 10.1016/j.bbi.2009.10.015
70. Sarkar C, Das S, Chakroborty D, Chowdhury UR, Basu B, Dasgupta PS, et al. Cutting edge: stimulation of dopamine d-4 receptors induce T cell quiescence by up-regulating kruppel-like factor-2 expression through inhibition of ERK1/ERK2 phosphorylation. J Immunol (2006) 177(11):7525–9. doi: 10.4049/jimmunol.177.11.7525
71. Levite M. Dopamine and T cells: dopamine receptors and potent effects on T cells, dopamine production in T cells, and abnormalities in the dopaminergic system in T cells in autoimmune, neurological and psychiatric diseases. Acta Physiol (Oxf). (2016) 216(1):42–89. doi: 10.1111/apha.12476
72. Saha B, Mondal AC, Majumder J, Basu S, Dasgupta PS. Physiological concentrations of dopamine inhibit the proliferation and cytotoxicity of human CD4+ and CD8+ T cells in vitro: a receptor-mediated mechanism. Neuroimmunomodulation (2001) 9(1):23–33. doi: 10.1159/000049004
73. Nasi G, Ahmed T, Rasini E, Fenoglio D, Marino F, Filaci G, et al. Dopamine inhibits human CD8+Treg function through d-1-like dopaminergic receptors. J Neuroimmunol (2019) 332:233–41. doi: 10.1016/j.jneuroim.2019.02.007
74. Cosentino M, Fietta AM, Ferrari M, Rasini E, Bombelli R, Carcano E, et al. Human CD4+CD25+ regulatory T cells selectively express tyrosine hydroxylase and contain endogenous catecholamines subserving an autocrine/paracrine inhibitory functional loop. Blood (2007) 109(2):632–42. doi: 10.1182/blood-2006-01-028423
75. Beyer M, Schultze JL. Regulatory T cells in cancer. Blood (2006) 108(3):804–11. doi: 10.1182/blood-2006-02-002774
76. Lissoni P, Vaghi M, Pescia S, Rovelli F, Ardizzola A, Valtulina F, et al. Biological response modifiers of cancer-related neuroendocrine disorders: efficacy of the long-term dopaminergic agonist cabergoline in the treatment of breast cancer-induced hyperprolactinemia. J Biol Regul Homeost Agents (2004) 18(3-4):291–4.
77. Flückiger E. Dopaminergic control of prolactin secretion. Bull Schweiz Akad Med Wiss (1978) 34(1-3):191–6.
78. Clemens JA, Shaar CJ, Smalstig EB. Dopamine, PIF, and other regulators of prolactin secretion. Fed Proc (1980) 39(11):2907–11.
79. Redelman D, Welniak LA, Taub D, Murphy WJ. Neuroendocrine hormones such as growth hormone and prolactin are integral members of the immunological cytokine network. Cell Immunol (2008) 252(1-2):111–21. doi: 10.1016/j.cellimm.2007.12.003
80. De Ponti F. Pharmacology of serotonin: what a clinician should know. Gut (2004) 53(10):1520–35. doi: 10.1136/gut.2003.035568
81. Walstab J, Rappold G, Niesler B. 5-HT3 receptors: role in disease and target of drugs. Pharmacol Ther (2010) 128(1):146–69. doi: 10.1016/j.pharmthera.2010.07.001
82. Jonnakuty C, Gragnoli C. What do we know about serotonin? J Cell Physiol (2008) 217(2):301–6. doi: 10.1002/jcp.21533
83. Mammadova-Bach E, Mauler M, Braun A, Duerschmied D. Autocrine and paracrine regulatory functions of platelet serotonin. Platelets (2018) 29(6):541–8. doi: 10.1080/09537104.2018.1478072
84. Qin X, Li J, Wang S, Lv J, Luan F, Liu Y, et al. Serotonin/HTR1E signaling blocks chronic stress-promoted progression of ovarian cancer. Theranostics (2021) 11(14):6950–65. doi: 10.7150/thno.58956
85. de las Casas-Engel M, Corbi AL. Serotonin modulation of macrophage polarization: inflammation and beyond. In: Camps J, editor. Oxidative stress and inflammation in non-communicable diseases - molecular mechanisms and perspectives in therapeutics. advances in experimental medicine and biology, vol. 824 . Cham: Springer International Publishing (2014). p. 89–115.
86. Chan YL, Lai WC, Chen JS, Tseng JTC, Chuang PC, Jou J, et al. TIAM2S mediates serotonin homeostasis and provokes a pro-inflammatory immune microenvironment permissive for colorectal tumorigenesis. Cancers (2020) 12(7):18. doi: 10.3390/cancers12071844
87. Liu Y, Zhang H, Wang ZY, Wu P, Gong W. 5-Hydroxytryptamine1a receptors on tumour cells induce immune evasion in lung adenocarcinoma patients with depression via autophagy/pSTAT3. Eur J Cancer (2019) 114:8–24. doi: 10.1016/j.ejca.2019.03.017
88. Schneider MA, Heeb L, Beffinger MM, Pantelyushin S, Linecker M, Roth L, et al. Attenuation of peripheral serotonin inhibits tumor growth and enhances immune checkpoint blockade therapy in murine tumor models. Sci Trans Med (2021) 13(611):16. doi: 10.1126/scitranslmed.abc8188
89. Gautron L, Rutkowski JM, Burton MD, Wei W, Wan Y, Elmquist JK. Neuronal and nonneuronal cholinergic structures in the mouse gastrointestinal tract and spleen. J Comp Neurol (2013) 521(16):3741–67. doi: 10.1002/cne.23376
90. Giastas P, Zouridakis M, Tzartos SJ. Understanding structure-function relationships of the human neuronal acetylcholine receptor: insights from the first crystal structures of neuronal subunits. Br J Pharmacol (2018) 175(11):1880–91. doi: 10.1111/bph.13838
91. Zimring JC, Kapp LM, Yamada M, Wess J, Kapp JA. Regulation of CD8(+) cytolytic T lymphocyte differentiation by a cholinergic pathway. J Neuroimmunol (2005) 164(1-2):66–75. doi: 10.1016/j.jneuroim.2005.03.018
92. Wang H, Yu M, Ochani M, Amella CA, Tanovic M, Susarla S, et al. Nicotinic acetylcholine receptor alpha7 subunit is an essential regulator of inflammation. Nature (2003) 421(6921):384–8. doi: 10.1038/nature01339
93. Chernyavsky AI, Arredondo J, Skok M, Grando SA. Auto/paracrine control of inflammatory cytokines by acetylcholine in macrophage-like U937 cells through nicotinic receptors. Int Immunopharmacol (2010) 10(3):308–15. doi: 10.1016/j.intimp.2009.12.001
94. Rosas-Ballina M, Olofsson PS, Ochani M, Valdes-Ferrer SI, Levine YA, Reardon C, et al. Acetylcholine-synthesizing T cells relay neural signals in a vagus nerve circuit. Science (2011) 334(6052):98–101. doi: 10.1126/science.1209985
95. Mashimo M, Fujii T, Ono S, Moriwaki Y, Misawa H, Kawashima K. Minireview: divergent roles of alpha 7 nicotinic acetylcholine receptors expressed on antigen-presenting cells and CD4+ T cells in the regulation of T cell differentiation. Int Immunopharmacol (2020) 82:7. doi: 10.1016/j.intimp.2020.106306
96. Fujii T, Mashimo M, Moriwaki Y, Misawa H, Ono S, Horiguchi K, et al. Physiological functions of the cholinergic system in immune cells. J Pharmacol Sci (2017) 134(1):1–21. doi: 10.1016/j.jphs.2017.05.002
97. Kwok HH, Gao BN, Chan KH, Ip MSM, Minna JD, Lam DCL. Nicotinic acetylcholine receptor subunit alpha 7 mediates cigarette smoke-induced PD-L1 expression in human bronchial epithelial cells. Cancers (2021) 13(21):14. doi: 10.3390/cancers13215345
98. Schuller HM. Is cancer triggered by altered signalling of nicotinic acetylcholine receptors? Nat Rev Cancer (2009) 9(3):195–205. doi: 10.1038/nrc2590
99. Kawashima K, Fujii T. Expression of non-neuronal acetylcholine in lymphocytes and its contribution to the regulation of immune function. Front Biosci (2004) 9:2063–85. doi: 10.2741/1390
100. Wessler IK, Kirkpatrick CJ. Non-neuronal acetylcholine involved in reproduction in mammals and honeybees. J Neurochem (2017) 142 Suppl 2:144–50. doi: 10.1111/jnc.13953
101. Razani-Boroujerdi S, Boyd RT, Dávila-García MI, Nandi JS, Mishra NC, Singh SP, et al. T Cells express alpha7-nicotinic acetylcholine receptor subunits that require a functional TCR and leukocyte-specific protein tyrosine kinase for nicotine-induced Ca2+ response. J Immunol (2007) 179(5):2889–98. doi: 10.4049/jimmunol.179.5.2889
102. Wang ZL, Liu W, Wang C, Li YA, Ai ZL. Acetylcholine promotes the self-renewal and immune escape of CD133+thyroid cancer cells through activation of CD133-akt pathway. Cancer Letters. (2020) 471:116–24. doi: 10.1016/j.canlet.2019.12.009
103. Yang MW, Tao LY, Jiang YS, Yang JY, Huo YM, Liu DJ, et al. Perineural invasion reprograms the immune microenvironment through cholinergic signaling in pancreatic ductal adenocarcinoma. Cancer Res (2020) 80(10):1991–2003. doi: 10.1158/0008-5472.CAN-19-2689
104. Zhu P, Jin ZX, Kang GY, Jia YF, Liu DR, Zhang Q, et al. Alpha5 nicotinic acetylcholine receptor mediated immune escape of lung adenocarcinoma via STAT3/Jab1-PD-L1 signalling. Cell Commun Signal (2022) 20(1):17. doi: 10.1186/s12964-022-00934-z
105. Best SA, Gubser PM, Sethumadhavan S, Kersbergen A, Negrón Abril YL, Goldford J, et al. Glutaminase inhibition impairs CD8 T cell activation in STK11-/Lkb1-deficient lung cancer. Cell Metab (2022) 34(6):874–87.e6. doi: 10.1016/j.cmet.2022.04.003
106. Nicoletti F, Battaglia G, Storto M, Ngomba RT, Iacovelli L, Arcella A, et al. Metabotropic glutamate receptors: beyond the regulation of synaptic transmission. Psychoneuroendocrinology (2007) 32:S40–S5. doi: 10.1016/j.psyneuen.2007.04.015
107. Jiang SH, Hu LP, Wang X, Li J, Zhang ZG. Neurotransmitters: emerging targets in cancer. Oncogene (2020) 39(3):503–15. doi: 10.1038/s41388-019-1006-0
108. Ganor Y, Levite M. The neurotransmitter glutamate and human T cells: glutamate receptors and glutamate-induced direct and potent effects on normal human T cells, cancerous human leukemia and lymphoma T cells, and autoimmune human T cells. J Neural Transm (2014) 121(8):983–1006. doi: 10.1007/s00702-014-1167-5
109. Ye ZC, Sontheimer H. Glioma cells release excitotoxic concentrations of glutamate. Cancer Res (1999) 59(17):4383–91.
110. Ye ZC, Rothstein JD, Sontheimer H. Compromised glutamate transport in human glioma cells: reduction-mislocalization of sodium-dependent glutamate transporters and enhanced activity of cystine-glutamate exchange. J Neurosci (1999) 19(24):10767–77. doi: 10.1523/JNEUROSCI.19-24-10767.1999
111. Behrens PF, Langemann H, Strohschein R, Draeger J, Hennig J. Extracellular glutamate and other metabolites in and around RG2 rat glioma: an intracerebral microdialysis study. J Neurooncol (2000) 47(1):11–22. doi: 10.1023/A:1006426917654
112. Takano T, Lin JH, Arcuino G, Gao Q, Yang J, Nedergaard M. Glutamate release promotes growth of malignant gliomas. Nat Med (2001) 7(9):1010–5. doi: 10.1038/nm0901-1010
113. Wan ZY, Sun RZ, Liu YW, Li SH, Sun JJ, Li J, et al. Targeting metabotropic glutamate receptor 4 for cancer immunotherapy. Sci Advances (2021) 7(50):19. doi: 10.1126/sciadv.abj4226
114. Arcella A, Carpinelli G, Battaglia G, D’Onofrio M, Santoro F, Ngomba RT, et al. Pharmacological blockade of group II metabotropic glutamate receptors reduces the growth of glioma cells in vivo. Neuro-Oncology (2005) 7(3):236–45. doi: 10.1215/S1152851704000961
115. Xiang Y, Stine ZE, Xia JS, Lu YQ, O’Connor RS, Altman BJ, et al. Targeted inhibition of tumor-specific glutaminase diminishes cell-autonomous tumorigenesis. J Clin Invest (2015) 125(6):2293–306. doi: 10.1172/JCI75836
116. Wu WC, Sun HW, Chen J, OuYang HY, Yu XJ, Chen HT, et al. Immunosuppressive immature myeloid cell generation is controlled by glutamine metabolism in human cancer. Cancer Immunol Res (2019) 7(10):1605–18. doi: 10.1158/2326-6066.CIR-18-0902
117. Suzuki S, Tanaka T, Poyurovsky MV, Nagano H, Mayama T, Ohkubo S, et al. Phosphate-activated glutaminase (GLS2), a p53-inducible regulator of glutamine metabolism and reactive oxygen species. Proc Natl Acad Sci United States America (2010) 107(16):7461–6. doi: 10.1073/pnas.1002459107
118. Lewerenz J, Hewett SJ, Huang Y, Lambros M, Gout PW, Kalivas PW, et al. The Cystine/Glutamate antiporter system x(c)(-) in health and disease: from molecular mechanisms to novel therapeutic opportunities. Antioxid Redox Signal (2013) 18(5):522–55. doi: 10.1089/ars.2011.4391
119. Jyotsana N, Ta KT, DelGiorno KE. The role of Cystine/Glutamate antiporter SLC7A11/xCT in the pathophysiology of cancer. Front Oncol (2022) 12:13. doi: 10.3389/fonc.2022.858462
120. Long Y, Tao HP, Karachi A, Grippin AJ, Jin LC, Chang Y, et al. Dysregulation of glutamate transport enhances treg function that promotes VEGF blockade resistance in glioblastoma. Cancer Res (2020) 80(3):499–509. doi: 10.1158/0008-5472.CAN-19-1577
121. Lang X, Green MD, Wang W, Yu J, Choi JE, Jiang L, et al. Radiotherapy and immunotherapy promote tumoral lipid oxidation and ferroptosis via synergistic repression of SLC7A11. Cancer Discovery (2019) 9(12):1673–85. doi: 10.1158/2159-8290.CD-19-0338
122. Wang WM, Kryczek I, Dostal L, Lin H, Tan LJ, Zhao LL, et al. Effector T cells abrogate stroma-mediated chemoresistance in ovarian cancer. Cell (2016) 165(5):1092–105. doi: 10.1016/j.cell.2016.04.009
123. Wang W, Green M, Choi JE, Gijón M, Kennedy PD, Johnson JK, et al. CD8+ T cells regulate tumour ferroptosis during cancer immunotherapy. Nature (2019) 569(7755):270–4. doi: 10.1038/s41586-019-1170-y
124. Li ZJ, Cho CH. Neurotransmitters, more than meets the eye–neurotransmitters and their perspectives in cancer development and therapy. Eur J Pharmacol (2011) 667(1-3):17–22. doi: 10.1016/j.ejphar.2011.05.077
125. Gladkevich A, Korf J, Hakobyan VP, Melkonyan KV. The peripheral GABAergic system as a target in endocrine disorders. Auton Neurosci (2006) 124(1-2):1–8. doi: 10.1016/j.autneu.2005.11.002
126. Matuszek M, Jesipowicz M, Kleinrok Z. GABA content and GAD activity in gastric cancer. Med Sci Monit (2001) 7(3):377–81.
127. Yang YH, Ren LW, Li S, Zheng XJ, Liu JY, Li W, et al. GABRP is a potential prognostic biomarker and correlated with immune infiltration and tumor microenvironment in pancreatic cancer. Transl Cancer Res (2022) 11(4):649. doi: 10.21037/tcr-21-2021
128. Mazurkiewicz M, Opolski A, Wietrzyk J, Radzikowski C, Kleinrok Z. GABA level and GAD activity in human and mouse normal and neoplastic mammary gland. J Exp Clin Cancer Res (1999) 18(2):247–53.
129. Brzozowska A, Burdan F, Duma D, Solski J, Mazurkiewicz M. Gamma-amino butyric acid (GABA) level as an overall survival risk factor in breast cancer. Ann Agr Env Med (2017) 24(3):435–9. doi: 10.26444/aaem/75891
130. Ortega A. A new role for GABA: inhibition of tumor cell migration. Trends Pharmacol Sci (2003) 24(4):151–4. doi: 10.1016/S0165-6147(03)00052-X
131. Zhang BH, Vogelzang A, Miyajima M, Sugiura Y, Wu YB, Chamoto K, et al. B cell-derived GABA elicits IL-10(+) macrophages to limit anti-tumour immunity. Nature (2021) 599(7885):471. doi: 10.1038/s41586-021-04082-1
132. Krummel DAP, Nasti TH, Kaluzova M, Kallay L, Bhattacharya D, Melms JC, et al. Melanoma cell intrinsic GABA(A) receptor enhancement potentiates radiation and immune checkpoint inhibitor response by promoting direct and T cell-mediated antitumor activity. Int J Radiat Oncol Biol Phys (2021) 109(4):1040–53. doi: 10.1016/j.ijrobp.2020.10.025
133. Wang T, Huang W, Chen F. Baclofen, a GABAB receptor agonist, inhibits human hepatocellular carcinoma cell growth in vitro and in vivo. Life Sci (2008) 82(9-10):536–41. doi: 10.1016/j.lfs.2007.12.014
134. Wang H, Zhang H, Sun Z, Chen W, Miao C. GABAB receptor inhibits tumor progression and epithelial-mesenchymal transition via the regulation of Hippo/YAP1 pathway in colorectal cancer. Int J Biol Sci (2021) 17(8):1953–62. doi: 10.7150/ijbs.58135
135. Huang D, Wang Y, Thompson JW, Yin T, Alexander PB, Qin DY, et al. Cancer-cell-derived GABA promotes beta-catenin-mediated tumour growth and immunosuppression. Nat Cell Biol (2022) 24(2):230. doi: 10.1038/s41556-021-00820-9
136. Palma C. Tachykinins and their receptors in human malignancies. Curr Drug Targets (2006) 7(8):1043–52. doi: 10.2174/138945006778019282
137. Mantyh PW. Neurobiology of substance p and the NK1 receptor. J Clin Psychiatry (2002) 63 (Suppl 11):6–10.
138. Garcia-Recio S, Gascon P. Biological and pharmacological aspects of the NK1-receptor. BioMed Res Int (2015) 2015:14. doi: 10.1155/2015/495704
139. Foreman JC. Neuropeptides and the pathogenesis of allergy. Allergy (1987) 42(1):1–11. doi: 10.1111/j.1398-9995.1987.tb02180.x
140. Shanahan F, Lee TD, Bienenstock J, Befus AD. Mast cell heterogeneity: effect of anti-allergic compounds on neuropeptide-induced histamine release. Int Arch Allergy Appl Immunol (1986) 80(4):424–6. doi: 10.1159/000234092
141. Janelsins BM, Mathers AR, Tkacheva OA, Erdos G, Shufesky WJ, Morelli AE, et al. Proinflammatory tachykinins that signal through the neurokinin 1 receptor promote survival of dendritic cells and potent cellular immunity. Blood (2009) 113(13):3017–26. doi: 10.1182/blood-2008-06-163121
142. Janelsins BM, Sumpter TL, Tkacheva OA, Rojas-Canales DM, Erdos G, Mathers AR, et al. Neurokinin-1 receptor agonists bias therapeutic dendritic cells to induce type 1 immunity by licensing host dendritic cells to produce IL-12. Blood (2013) 121(15):2923–33. doi: 10.1182/blood-2012-07-446054
143. Cunin P, Caillon A, Corvaisier M, Garo E, Scotet M, Blanchard S, et al. The tachykinins substance p and hemokinin-1 favor the generation of human memory Th17 cells by inducing IL-1 beta, IL-23, and TNF-like 1A expression by monocytes. J Immunol (2011) 186(7):4175–82. doi: 10.4049/jimmunol.1002535
144. Entschladen F, Lang K, Drell TL, Joseph J, Zaenker KS. Neurotransmitters are regulators for the migration of tumor cells and leukocytes. Cancer Immunol Immunother (2002) 51(9):467–82. doi: 10.1007/s00262-002-0300-8
145. Drell TL, Joseph J, Lang K, Niggemann B, Zaenker KS, Entschladen F. Effects of neurotransmitters on the chemokinesis and chemotaxis of MDA-MB-468 human breast carcinoma cells. Breast Cancer Res Treat (2003) 80(1):63–70. doi: 10.1023/A:1024491219366
146. Ruff M, Schiffmann E, Terranova V, Pert CB. Neuropeptides are chemoattractants for human tumor cells and monocytes: a possible mechanism for metastasis. Clin Immunol Immunopathol (1985) 37(3):387–96. doi: 10.1016/0090-1229(85)90108-4
147. Yang C, Sun YH, Ouyang XY, Li J, Zhu Z, Yu RH, et al. Pain may promote tumor progression via substance p-dependent modulation of toll-like receptor-4. Pain Med (2020) 21(12):3443–50. doi: 10.1093/pm/pnaa265
148. Mayordomo C, Garcia-Recio S, Ametller E, Fernandez-Nogueira P, Pastor-Arroyo EM, Vinyals L, et al. Targeting of substance p induces cancer cell death and decreases the steady state of EGFR and Her2. J Cell Physiol (2012) 227(4):1358–66. doi: 10.1002/jcp.22848
149. Nizam E, Koksoy S, Erin N. NK1R antagonist decreases inflammation and metastasis of breast carcinoma cells metastasized to liver but not to brain; phenotype-dependent therapeutic and toxic consequences. Cancer Immunol Immunother (2020) 69(8):1639–50. doi: 10.1007/s00262-020-02574-z
150. Zhao XN, Bai ZZ, Li CH, Sheng CL, Li HY. The NK-1R antagonist aprepitant prevents LPS-induced oxidative stress and inflammation in RAW264. 7 Macrophages Drug Des Dev Ther (2020) 14:1943–51. doi: 10.2147/DDDT.S244099
151. Mechanick JI, Levin N, Roberts JL, Autelitano DJ. Proopiomelanocortin gene expression in a distinct population of rat spleen and lung leukocytes. Endocrinology (1992) 131(1):518–25. doi: 10.1210/endo.131.1.1612033
152. Cabot PJ. Immune-derived opioids and peripheral antinociception. Clin Exp Pharmacol Physiol (2001) 28(3):230–2. doi: 10.1046/j.1440-1681.2001.03425.x
153. Manfredi B, Sacerdote P, Bianchi M, Locatelli L, Veljic-Radulovic J, Panerai AE. Evidence for an opioid inhibitory effect on T cell proliferation. J Neuroimmunol (1993) 44(1):43–8. doi: 10.1016/0165-5728(93)90266-2
154. Sarkar DK, Murugan S, Zhang CQ, Boyadjieva N. Regulation of cancer progression by beta-endorphin neuron. Cancer Res (2012) 72(4):836–40. doi: 10.1158/0008-5472.CAN-11-3292
155. Sarkar DK, Zhang C, Murugan S, Dokur M, Boyadjieva NI, Ortiguela M, et al. Transplantation of beta-endorphin neurons into the hypothalamus promotes immune function and restricts the growth and metastasis of mammary carcinoma. Cancer Res (2011) 71(19):6282–91. doi: 10.1158/0008-5472.CAN-11-1610
156. Mazahery C, Benson BL, Cruz-Lebron A, Levine AD. Chronic methadone use alters the CD8(+) T cell phenotype In Vivo and modulates its responsiveness ex vivo to opioid receptor and TCR stimuli. J Immunol (2020) 204(5):1188–200. doi: 10.4049/jimmunol.1900862
157. Melander O, Orho-Melander M, Manjer J, Svensson T, Almgren P, Nilsson PM, et al. Stable peptide of the endogenous opioid enkephalin precursor and breast cancer risk. J Clin Oncol (2015) 33(24):2632–U81. doi: 10.1200/JCO.2014.59.7682
158. Zagon IS, Roesener CD, Verderame MF, Ohlsson-Wilhelm BM, Levin RJ, McLaughlin PJ. Opioid growth factor regulates the cell cycle of human neoplasias. Int J Oncol (2000) 17(5):1053–61. doi: 10.3892/ijo.17.5.1053
159. Wang XA, Li SL, Yan SQ, Shan YY, Wang X, Jingbo Z, et al. Methionine enkephalin inhibits colorectal cancer by remodeling the immune status of the tumor microenvironment. Int Immunopharmacol (2022) 111:13. doi: 10.1016/j.intimp.2022.109125
160. Tuo YL, Zhang ZJ, Tian C, Hu QY, Xie R, Yang J, et al. Anti-inflammatory and metabolic reprogramming effects of MENK produce antitumor response in CT26 tumor-bearing mice. J Leukoc Biol (2020) 108(1):215–28. doi: 10.1002/JLB.3MA0120-578R
161. Tuo YL, Tian C, Lu LL, Xiang M. The paradoxical role of methionine enkephalin in tumor responses. Eur J Pharmacol (2020) 882:8. doi: 10.1016/j.ejphar.2020.173253
162. McDonald PH, Lefkowitz RJ. Beta-arrestins: new roles in regulating heptahelical receptors’ functions. Cell Signal (2001) 13(10):683–9. doi: 10.1016/S0898-6568(01)00203-0
163. Badino GR, Novelli A, Girardi C, Di Carlo F. Evidence for functional beta-adrenoceptor subtypes in CG-5 breast cancer cell. Pharmacol Res (1996) 33(4–5):255–60. doi: 10.1006/phrs.1996.0036
164. Vandewalle B, Revillion F, Lefebvre J. Functional beta-adrenergic receptors in breast cancer cells. J Cancer Res Clin Oncol (1990) 116(3):303–6. doi: 10.1007/BF01612908
165. Marchetti B, Spinola PG, Pelletier G, Labrie F. A potential role for catecholamines in the development and progression of carcinogen-induced mammary tumors: hormonal control of beta-adrenergic receptors and correlation with tumor growth. J Steroid Biochem Mol Biol (1991) 38(3):307–20. doi: 10.1016/0960-0760(91)90102-B
166. Sood AK, Bhatty R, Kamat AA, Landen CN, Han L, Thaker PH, et al. Stress hormone-mediated invasion of ovarian cancer cells. Clin Cancer Res (2006) 12(2):369–75. doi: 10.1158/1078-0432.CCR-05-1698
167. Perron L, Bairati I, Harel F, Meyer F. Antihypertensive drug use and the risk of prostate cancer (Canada). Cancer Causes Control (2004) 15(6):535–41. doi: 10.1023/B:CACO.0000036152.58271.5e
168. Algazi M, Plu-Bureau G, Flahault A, Dondon MG, Lê MG. [Could treatments with beta-blockers be associated with a reduction in cancer risk]? Rev Epidemiol Sante Publique (2004) 52(1):53–65. doi: 10.1016/S0398-7620(04)99022-0
169. Li CI, Malone KE, Weiss NS, Boudreau DM, Cushing-Haugen KL, Daling JR. Relation between use of antihypertensive medications and risk of breast carcinoma among women ages 65-79 years. Cancer (2003) 98(7):1504–13. doi: 10.1002/cncr.11663
170. Padro CJ, Sanders VM. Neuroendocrine regulation of inflammation. Semin Immunol (2014) 26(5):357–68. doi: 10.1016/j.smim.2014.01.003
171. Botta F, Maestroni GJ. Adrenergic modulation of dendritic cell cancer vaccine in a mouse model: role of dendritic cell maturation. J Immunother (2008) 31(3):263–70. doi: 10.1097/CJI.0b013e318160995e
172. Grytli HH, Fagerland MW, Fosså SD, Taskén KA. Association between use of β-blockers and prostate cancer-specific survival: a cohort study of 3561 prostate cancer patients with high-risk or metastatic disease. Eur Urol (2014) 65(3):635–41. doi: 10.1016/j.eururo.2013.01.007
173. Lemeshow S, Sørensen HT, Phillips G, Yang EV, Antonsen S, Riis AH, et al. β-blockers and survival among Danish patients with malignant melanoma: a population-based cohort study. Cancer Epidemiol Biomarkers Prev (2011) 20(10):2273–9. doi: 10.1158/1055-9965.EPI-11-0249
174. Melhem-Bertrandt A, Chavez-Macgregor M, Lei X, Brown EN, Lee RT, Meric-Bernstam F, et al. Beta-blocker use is associated with improved relapse-free survival in patients with triple-negative breast cancer. J Clin Oncol (2011) 29(19):2645–52. doi: 10.1200/JCO.2010.33.4441
175. Jansen L, Hoffmeister M, Arndt V, Chang-Claude J, Brenner H. Stage-specific associations between beta blocker use and prognosis after colorectal cancer. Cancer (2014) 120(8):1178–86. doi: 10.1002/cncr.28546
176. Mohammadpour H, MacDonald CR, Qiao GX, Chen MH, Dong BW, Hylander BL, et al. Beta 2 adrenergic receptor-mediated signaling regulates the immunosuppressive potential of myeloid-derived suppressor cells. J Clin Invest (2019) 129(12):5537–52. doi: 10.1172/JCI129502
177. Goldfarb Y, Sorski L, Benish M, Levi B, Melamed R, Ben-Eliyahu S. Improving postoperative immune status and resistance to cancer metastasis a combined perioperative approach of immunostimulation and prevention of excessive surgical stress responses. Ann Surg (2011) 253(4):798–810. doi: 10.1097/SLA.0b013e318211d7b5
178. Neeman E, Ben-Eliyahu S. Surgery and stress promote cancer metastasis: new outlooks on perioperative mediating mechanisms and immune involvement. Brain Behav Immunity (2013) 30:S32–40. doi: 10.1016/j.bbi.2012.03.006
179. Neeman E, Zmora O, Ben-Eliyahu S. A new approach to reducing postsurgical cancer recurrence: perioperative targeting of catecholamines and prostaglandins. Clin Cancer Res (2012) 18(18):4895–902. doi: 10.1158/1078-0432.CCR-12-1087
180. Behrenbruch C, Shembrey C, Paquet-Fifield S, Molck C, Cho HJ, Michael M, et al. Surgical stress response and promotion of metastasis in colorectal cancer: a complex and heterogeneous process. Clin Exp Metastasis (2018) 35(4):333–45. doi: 10.1007/s10585-018-9873-2
181. Benish M, Bartal I, Goldfarb Y, Levi B, Avraham R, Raz A, et al. Perioperative use of beta-blockers and COX-2 inhibitors may improve immune competence and reduce the risk of tumor metastasis. Ann Surg Oncol (2008) 15(7):2042–52. doi: 10.1245/s10434-008-9890-5
182. Hiller JG, Perry NJ, Poulogiannis G, Riedel B, Sloan EK. Perioperative events influence cancer recurrence risk after surgery. Nat Rev Clin Oncol (2018) 15(4):205–18. doi: 10.1038/nrclinonc.2017.194
183. Bakos O, Lawson C, Rouleau S, Tai LH. Combining surgery and immunotherapy: turning an immunosuppressive effect into a therapeutic opportunity. J Immunother Cancer (2018) 6:11. doi: 10.1186/s40425-018-0398-7
184. Pyter LM, Pineros V, Galang JA, McClintock MK, Prendergast BJ. Peripheral tumors induce depressive-like behaviors and cytokine production and alter hypothalamic-pituitary-adrenal axis regulation. Proc Natl Acad Sci USA (2009) 106(22):9069–74. doi: 10.1073/pnas.0811949106
185. Lebeña A, Vegas O, Gómez-Lázaro E, Arregi A, Garmendia L, Beitia G, et al. Melanoma tumors alter proinflammatory cytokine production and monoamine brain function, and induce depressive-like behavior in male mice. Behav Brain Res (2014) 272:83–92. doi: 10.1016/j.bbr.2014.06.045
186. Yang M, Kim J, Kim JS, Kim SH, Kim JC, Kang MJ, et al. Hippocampal dysfunctions in tumor-bearing mice. Brain Behav Immun (2014) 36:147–55. doi: 10.1016/j.bbi.2013.10.022
187. Pintar JE, Breakefield XO. Monoamine oxidase (MAO) activity as a determinant in human neurophysiology. Behav Genet (1982) 12(1):53–68. doi: 10.1007/BF01065740
188. Wang X, Li B, Kim YJ, Wang YC, Li Z, Yu JJ, et al. Targeting monoamine oxidase a for T cell-based cancer immunotherapy. Sci Immunol (2021) 6(59):16. doi: 10.1126/sciimmunol.abh2383
189. Wang YC, Wang X, Yu J, Ma F, Li Z, Zhou Y, et al. Targeting monoamine oxidase a-regulated tumor-associated macrophage polarization for cancer immunotherapy. Nat Commun (2021) 12(1):3530. doi: 10.1038/s41467-021-23164-2
190. Tiihonen J, Rautiainen MR, Ollila HM, Repo-Tiihonen E, Virkkunen M, Palotie A, et al. Genetic background of extreme violent behavior. Mol Psychiatry (2015) 20(6):786–92. doi: 10.1038/mp.2014.130
191. Brown J, Li Z, Wang X, Kim YJ, Wang YC, Zuo YN, et al. Nanoformulation improves antitumor efficacy of MAOI immune checkpoint blockade therapy without causing aggression-related side effects. Front Pharmacol (2022) 13. doi: 10.3389/fphar.2022.970324
192. Grygier B, Arteta B, Kubera M, Basta-Kaim A, Budziszewska B, Leskiewicz M, et al. Inhibitory effect of antidepressants on B16F10 melanoma tumor growth. Pharmacol Rep (2013) 65(3):672–81. doi: 10.1016/S1734-1140(13)71045-4
193. Di Rosso ME, Sterle HA, Cremaschi GA, Genaro AM. Beneficial effect of fluoxetine and sertraline on chronic stress-induced tumor growth and cell dissemination in a mouse model of lymphoma: crucial role of antitumor immunity. Front Immunol (2018) 9:16. doi: 10.3389/fimmu.2018.01341
194. Ghosh S, Mukherjee S, Choudhury S, Gupta P, Adhikary A, Baral R, et al. Reactive oxygen species in the tumor niche triggers altered activation of macrophages and immunosuppression: role of fluoxetine. Cell Signal (2015) 27(7):1398–412. doi: 10.1016/j.cellsig.2015.03.013
195. Ghosh S, Choudhury S, Mukherjee S, Gupta P, Chowdhury O, Baral R, et al. Fluoxetine triggers selective apoptosis in inflammation-induced proliferating (Ki-67(high)) thymocytes. Immunol Cell Biol (2019) 97(5):470–84. doi: 10.1111/imcb.12227
196. Wei PK, Jia HJ, Li RP, Zhang CL, Guo SS, Wei SJ, et al. Fluvoxamine prompts the antitumor immune effect via inhibiting the PD-L1 expression on mice-burdened colon tumor. Cell Biol Int (2023) 47(2):439–50. doi: 10.1002/cbin.11936
197. Zhou S, Ye D, Xia HW, Xu HJ, Tang WP, Tang QL, et al. Sertraline inhibits stress-induced tumor growth through regulating CD8(+) T cell-mediated anti-tumor immunity. Anti-Cancer Drugs (2022) 33(9):935–42. doi: 10.1097/CAD.0000000000001383
198. Chryplewicz A, Scotton J, Tichet M, Zomer A, Shchors K, Joyce JA, et al. Cancer cell autophagy, reprogrammed macrophages, and remodeled vasculature in glioblastoma triggers tumor immunity. Cancer Cell (2022) 40(10):1111. doi: 10.1016/j.ccell.2022.08.014
199. Seeman P, Kapur S. Schizophrenia: more dopamine, more D2 receptors. Proc Natl Acad Sci U S A. (2000) 97(14):7673–5. doi: 10.1073/pnas.97.14.7673
200. Bushe CJ, Hodgson R. Schizophrenia and cancer: in 2010 do we understand the connection? Can J Psychiatry (2010) 55(12):761–7. doi: 10.1177/070674371005501203
201. Wu J, Zhang RH, Tang N, Gong ZZ, Zhou JF, Chen YW, et al. Dopamine inhibits the function of gr-1(+)CD115(+) myeloid-derived suppressor cells through D1-like receptors and enhances anti-tumor immunity. J Leukoc Biol (2015) 97(1):191–200. doi: 10.1189/jlb.5A1113-626RR
202. Wang PS, Walker AM, Tsuang MT, Orav EJ, Glynn RJ, Levin R, et al. Dopamine antagonists and the development of breast cancer. Arch Gen Psychiatry (2002) 59(12):1147–54. doi: 10.1001/archpsyc.59.12.1147
203. Liu YS, Huang BR, Lin CJ, Shen CK, Lai SW, Chen CW, et al. Paliperidone inhibits glioblastoma growth in mouse brain tumor model and reduces PD-L1 expression. Cancers (2021) 13(17):17. doi: 10.3390/cancers13174357
204. Nishino M, Ramaiya NH, Hatabu H, Hodi FS. Monitoring immune-checkpoint blockade: response evaluation and biomarker development. Nat Rev Clin Oncol (2017) 14(11):655–68. doi: 10.1038/nrclinonc.2017.88
205. Kalbasi A, Ribas A. Tumour-intrinsic resistance to immune checkpoint blockade. Nat Rev Immunol (2020) 20(1):25–39. doi: 10.1038/s41577-019-0218-4
206. Kamiya A, Hayama Y, Kato S, Shimomura A, Shimomura T, Irie K, et al. Genetic manipulation of autonomic nerve fiber innervation and activity and its effect on breast cancer progression. Nat Neurosci (2019) 22(8):1289. doi: 10.1038/s41593-019-0430-3
207. Bucsek MJ, Qiao GX, MacDonald CR, Giridharan T, Evans L, Niedzwecki B, et al. Beta-adrenergic signaling in mice housed at standard temperatures suppresses an effector phenotype in CD8(+) T cells and undermines checkpoint inhibitor therapy. Cancer Res (2017) 77(20):5639–51. doi: 10.1158/0008-5472.CAN-17-0546
Keywords: neurotransmitter, tumor microenvironment, neuroimmune interaction, cancer immunology, immune modulator
Citation: Xiao L, Li X, Fang C, Yu J and Chen T (2023) Neurotransmitters: promising immune modulators in the tumor microenvironment. Front. Immunol. 14:1118637. doi: 10.3389/fimmu.2023.1118637
Received: 07 December 2022; Accepted: 12 April 2023;
Published: 05 May 2023.
Edited by:
Roi Gazit, Ben Gurion University of the Negev, IsraelReviewed by:
David Askew, Case Western Reserve University, United StatesAbhilasha Purohit, National Institutes of Health (NIH), United States
Copyright © 2023 Xiao, Li, Fang, Yu and Chen. This is an open-access article distributed under the terms of the Creative Commons Attribution License (CC BY). The use, distribution or reproduction in other forums is permitted, provided the original author(s) and the copyright owner(s) are credited and that the original publication in this journal is cited, in accordance with accepted academic practice. No use, distribution or reproduction is permitted which does not comply with these terms.
*Correspondence: Tao Chen, drchentao@163.com; Jiang Yu, balbc@163.com