- 1Department of Microbiology and Immunology, Cornell Institute of Host-Microbe Interaction and Disease, Cornell Center for Immunology, Cornell University, Ithaca, NY, United States
- 2Department of Pathobiological Sciences, School of Veterinary Medicine, Louisiana State University, Baton Rouge, LA, United States
- 3Cornell Center for Health Equity, Cornell University, Ithaca, NY, United States
One of the most proliferative periods for T cells occurs during their development in the thymus. Increased DNA replication can result in increased DNA mutations in the nuclear genome, but also in mitochondrial genomes. A high frequency of mitochondrial DNA mutations can lead to abnormal mitochondrial function and have negative implications on human health. Furthermore, aging is accompanied by an increase in such mutations through oxidative damage and replication errors. Increased mitochondrial DNA mutations cause loss of mitochondrial protein function, and decrease energy production, substrates, and metabolites. Here we have evaluated the effect of increased mitochondrial DNA mutations on T cell development in the thymus. Using mice carrying a mutant mitochondrial DNA polymerase γ (PolG) that causes increased mitochondrial DNA mutations, we show that high fidelity replication of mitochondrial DNA is pivotal for proper T cell development. Reducing the fidelity of mitochondrial DNA replication results in a premature age-dependent reduction in the total number of CD4/CD8 double negative and double positive thymocytes. Analysis of mitochondrial density in thymocyte subpopulations suggests that this may be due to reduced proliferation in specific double negative stages. Taken together, this work suggests that T cell development is regulated by the ability of mitochondria to faithfully replicate their DNA.
Introduction
T lymphocytes are a critical part of the adaptive immune response, needed for protection from non-self, maintenance of self-tolerance, and removing abnormal cell growth in an antigen specific manner. T cells originate from stem cells in the bone marrow, which migrate to the thymus to mature, and egress into the periphery where they can perform their effector functions. Fully functioning T cells can then proliferate and differentiate according to appropriate immune responses. One of the most proliferative periods for T cells occurs during development in the thymus. Maturation of lymphoid precursors can occur in various stages marked by CD4 and CD8 expression, i.e., CD4-CD8- double negative (DN), CD4+CD8+ double positive (DP), CD4+CD8- single positive or CD4-CD8+ single positive (SP). In addition, the DN stage can be further compartmentalized into four major stages based on expression of CD25 and CD44: CD44+CD25- (DN1), CD44+CD25+ (DN2), CD44-CD25+ (DN3), and CD44-CD25- (DN4). These different stages are defined based on different phases of the T cell receptor β (TCRβ) gene selection where the most proliferative stage is just prior to the DP stage when productive TCRβ gene arrangement occurs (1). Such responses require synchronous metabolic support through glycolysis and oxidative phosphorylation which occur in the cytoplasm and the mitochondria, respectively (2).
The mitochondrion is made up of around 1,000 different proteins encoded by mitochondrial and nuclear genes (3). Approximately ~99% of the proteins in this semi-autonomous organelle are transcribed from nuclear genes. However, mitochondrial function is dependent on the mitochondrial genome. The mitochondrial genome is highly regulated to meet cellular demands and makes copies irrespective of the cell replication and in differentiating cells (4–6). Access to these genes is imperative for normal mitochondrial function as they encode for 37 different mitochondrial proteins, all of which play a critical role in normal mitochondrial physiology (7). As part of the aging process mutations accumulate in these genes, and over time these modifications of the mitochondrial genome can cause proteins to lose their functional capacity. Robust mutation repair mechanisms guard the nuclear genome of the cell (8). In contrast, there are fewer mechanisms for correcting mitochondrial gene mutations, the most important of which is carried out by the N-terminal “proofreading” exonuclease domain of the mitochondria DNA (mtDNA) polymerase γ (PolG) (9, 10). PolG corrects mismatched mitochondrial nucleotides, which if not corrected can result in increased somatic mtDNA mutations and decreased production of energy, substrates, and metabolites by the mitochondria (10–12). Immune cells, including T cells, are highly dependent on these products as they are some of the most dynamic cells in the body, being among the most proliferative cells, requiring multiple rounds of DNA replication, both nuclear and mitochondrion (13, 14).
In this study, we have evaluated the effect of increased mtDNA mutations on T cell development. Using mice carrying the PolGD257A mutation in the mtDNA polymerase γ (PolG) that causes increased mtDNA mutations (up to 500-fold higher mutation burden than WT mice) (15), we show that high fidelity replication of mtDNA is pivotal for proper T cell development. Reducing the fidelity of mtDNA replication results in a premature age-dependent reduction in the total number of CD4/CD8 double positive and negative thymocytes (16). Analysis of mitochondrial density in thymocyte subpopulations suggests that this may be due to reduced proliferation in specific double negative stages. Taken together, this work suggests that T cell development is regulated by the ability of mitochondria to faithfully replicate their DNA.
Results
Low fidelity mtDNA polymerase impairs thymocyte development in an age and genotype dependent manner
To determine the effects of increased mtDNA mutations on T cell development, we examined the low fidelity PolGD257A mouse model at different ages, analyzing age groups previously shown in the literature to represent young (1-3 months), mature (6-8 months) and old (11-13 months) mice (Figure 1A) (17, 18). We weighed the thymi and counted the total number of thymocytes from the three different age groups (young, mature, old) and genotypes; wildtype (PolG+/+ or WT), heterozygous (PolGD257A/+), and homozygous (PolGD257A/D257A). Consistent with previously published results, we found that there is decreased weight and total number of thymocytes (Figures 1B, C) in the PolGD257A/D257A relative to WT in the mature mice (15). However, these differences were not observed in the young or old groups. Furthermore, we found that the PolG+/D257A mice shared the same phenotype as WT mice in all age groups, suggesting that some WT polymerase may be sufficient for the development of these cells. Examination of RNA-Sequencing data for PolG expression in thymus samples of neonatal and adult mice by RNA-revealed no age-related difference in expression (Supplemental Figure 1).
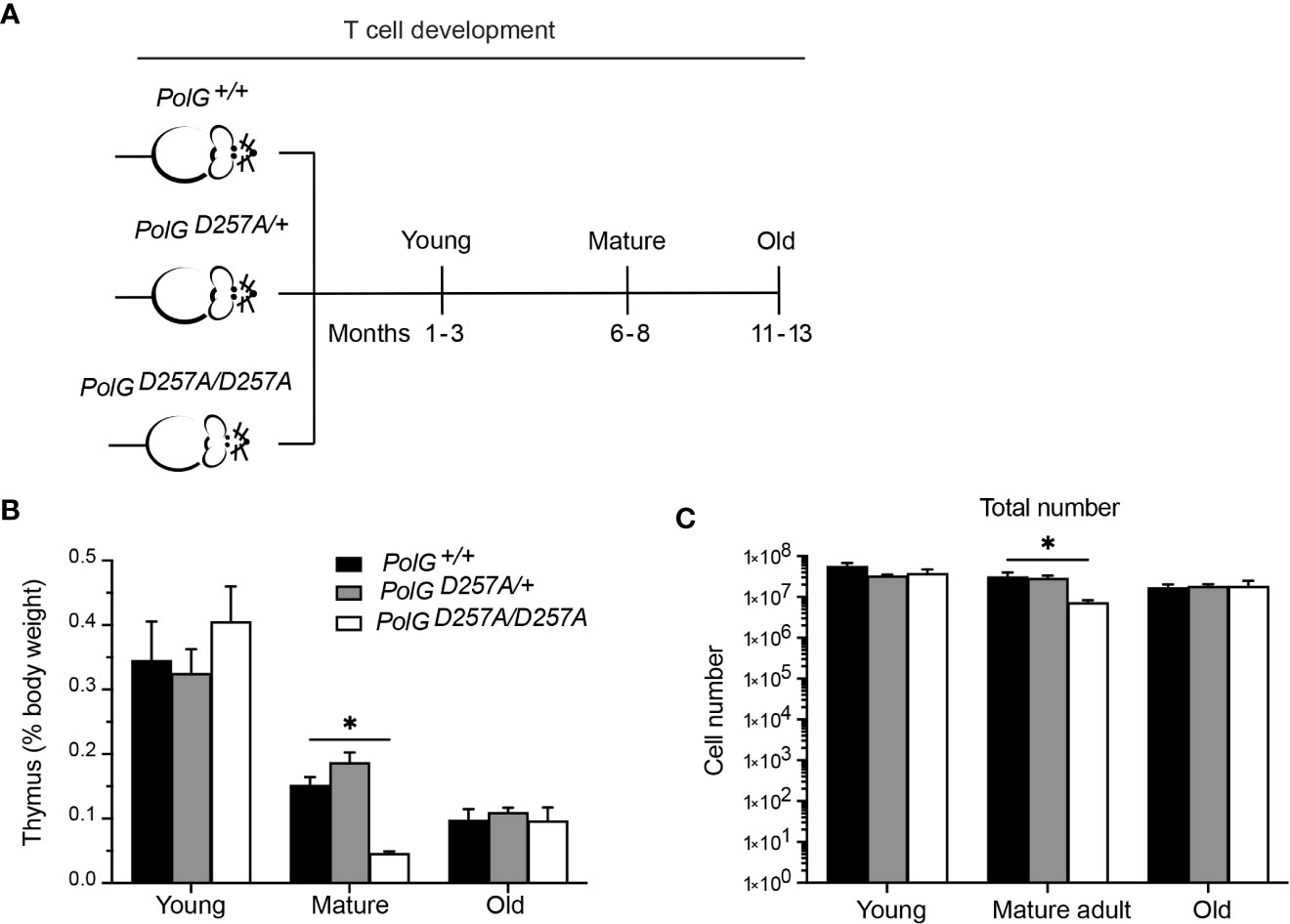
Figure 1 Fidelity of mtDNA replication is important for normal thymocyte numbers. (A) Experimental outline of PolGD257A/D257A genotypes and age groups. (B) Thymi from young, mature, and old PolGD257A/D257A mice were weighed and plotted as a percentage of body weight. (C) Thymocyte numbers from young, mature, and old PolG+/+ and PolG+/D257A were determined and plotted. Data representative 11 independent experiments, n=5-24 mice per group. *p<0.05-.01.
We next used CD4 and CD8 to differentiate the thymocyte stages utilizing flow cytometry (Figures 2A) (19). There was an increase in the percent of the DN population in the mature and old PolGD257A/D257A mice relative to WT mice (Figures 2B, C). Furthermore, the total cell numbers also indicated that there was fewer total number of DP and SP CD4+ and CD8+ T cells in the mature PolGD257A/D257A group relative to the WT (Figure 2C). Taken together, this suggests that dysfunctional PolG causes a block at the DN developmental stage.
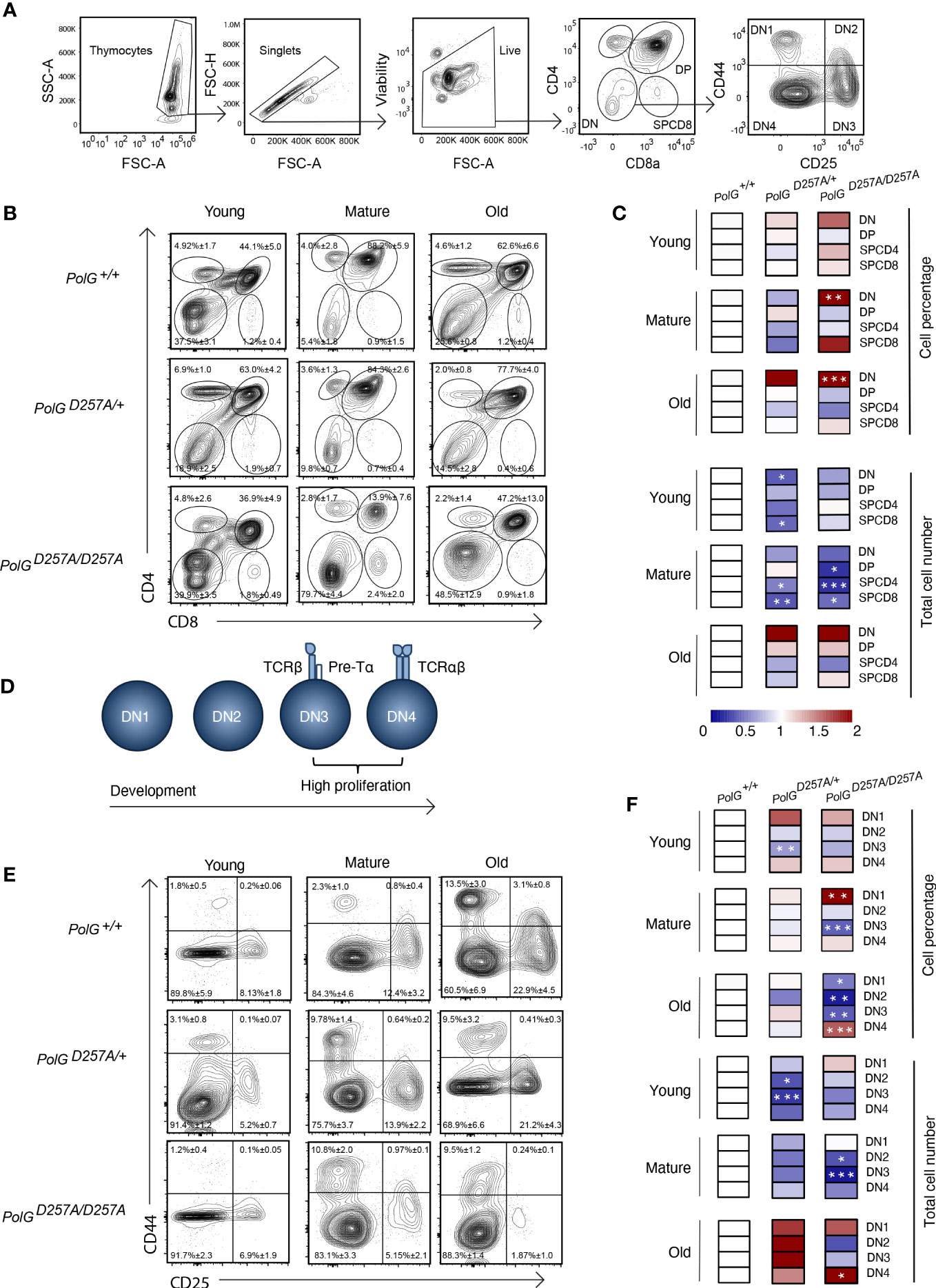
Figure 2 Error prone mtDNA replication impairs thymocyte development in mature PolGD257A/D257A mice. (A) Gating scheme used to determine the percentage (and number) of developing T cell populations. DN= double negative, DP= double positive, and SP=single positive. (B, C) Thymi from young, mature, and old PolG+/+, PolG+/D257A, and PolGD257A/D257A mice were stained for CD4 and CD8 surface markers. The gating shows DP, CD4+ and CD8+ SP thymocytes. Heatmaps are a representative quantification that are normalized by the mean of the control, where red indicates an increase and blue indicates a decrease. (D) Cartoon schematic showing the progression of T cell development in the DN stage. (E, F) Thymocytes from PolG+/+, PolG+/D257A, and PolGD257A/D257A mice were stained for CD25 and CD44 to differentiate DN subpopulations, and percentages of the DN1-4 stages determined. Data are displayed as mean ± standard error of mean and representative 11 independent experiments, n=7-24 mice per group, where *p<0.05-.01, **p<0.01-0.001, ***p<0.001 based on multiple t tests.
We sought to further investigate the DN populations with the goal of determining the stage at which these cells are being prevented from passing into the more mature stages, including the DP stage. Therefore, we investigated the four identifiable double negative populations: DN1-4, utilizing the expression patterns of CD25 and CD44 as markers (Figures 2A, D). As expected, we observed the greatest differences in the DN3 or DN4 populations, the most proliferative stages during T cell development (Figures 2E, F). We saw an increase in cell percentages of DN1, and decrease in DN3 stages, in the mature PolGD257A/D257A (Figure 2F). Furthermore, we saw a decrease in the total number of DN2 and DN3 populations in mature PolGD257A/D257A mice relative to WT mice, as well as curiously, in young PolG+/D257A mice but not in young PolGD257A/D257A mice. We also noted an increase in the number of the DN4 population in old PolGD257A/D257A mice relative to WT mice (Figure 2F). There was also what seemed like a corresponding increase in the percentage of the DN1 population, and a reduction in the percentage of DN3 population in mature PolGD257A/D257A mice relative to WT mice. The old PolGD257A/D257A mice had reduced percentages of DN1, DN2, and DN3 populations and an increase in DN4 populations. This data suggests that the presence of a low fidelity mtDNA polymerase resulted in an overall decrease in the number of DN populations and stalled T cell development, likely at the DN3 population in mature mice.
Expression of TCR transgene partially rescues the DN population in PolGD257A/D257A mice
To interrogate the effects of the fidelity of mtDNA replication in a model of T cell development expressing a fixed TCR, we crossed PolGD257A mice to OT-II transgenic mice which carry rearranged TCR alpha and beta chains that are restricted to major histocompatibility complex II (MHCII) Ab (and recognizes the peptide 323-339 from the protein Ovalbumin) (20, 21). The presence of the already rearranged TCR accelerates the developmental stages through the DN3 when gene segments encoding the TCR alpha and beta chains would usually rearrange (22, 23). Using this transgenic T cell receptor (TgTCR) model, we tracked the T cell development in the thymus, focusing on young and mature groups given their differences in T cell development.
We collected thymi from young or mature groups and analyzed thymic weights and thymocyte number. We observed a strong concordance between the TgTCR model and the non-TgTCR PolGD257A/D257A, where we saw no significant differences in the young mice, but a reduced thymic weight of mature OTII/PolGD257A/D257A mice relative to the control (Figures 3A, B). However, when we analyzed the percentage of DN and DP populations in the OTII/PolGD257A/D257A mice relative to the control, we observed an increased overall percentage of the DN population in the OTII/PolGD257A/D257A, as was found in the non-TgTCR model, along with a decrease in the percentage of the DP population (Figure 3C). Closer examination of the DN populations revealed a trend towards an overall decrease in the percentage of the cells in the DN1-3 stages (significantly decreased in DN1), unlike what we observed in the non-TgTCR model, but an increase in the percentage of the DN4 population relative to the control (Figure 3D). This data suggests that the presence of a low fidelity mtDNA polymerase resulted in stalled T cell development at the DN3 stage in mature mice, and this is altered in the transgenic model with accelerated development through these stages.
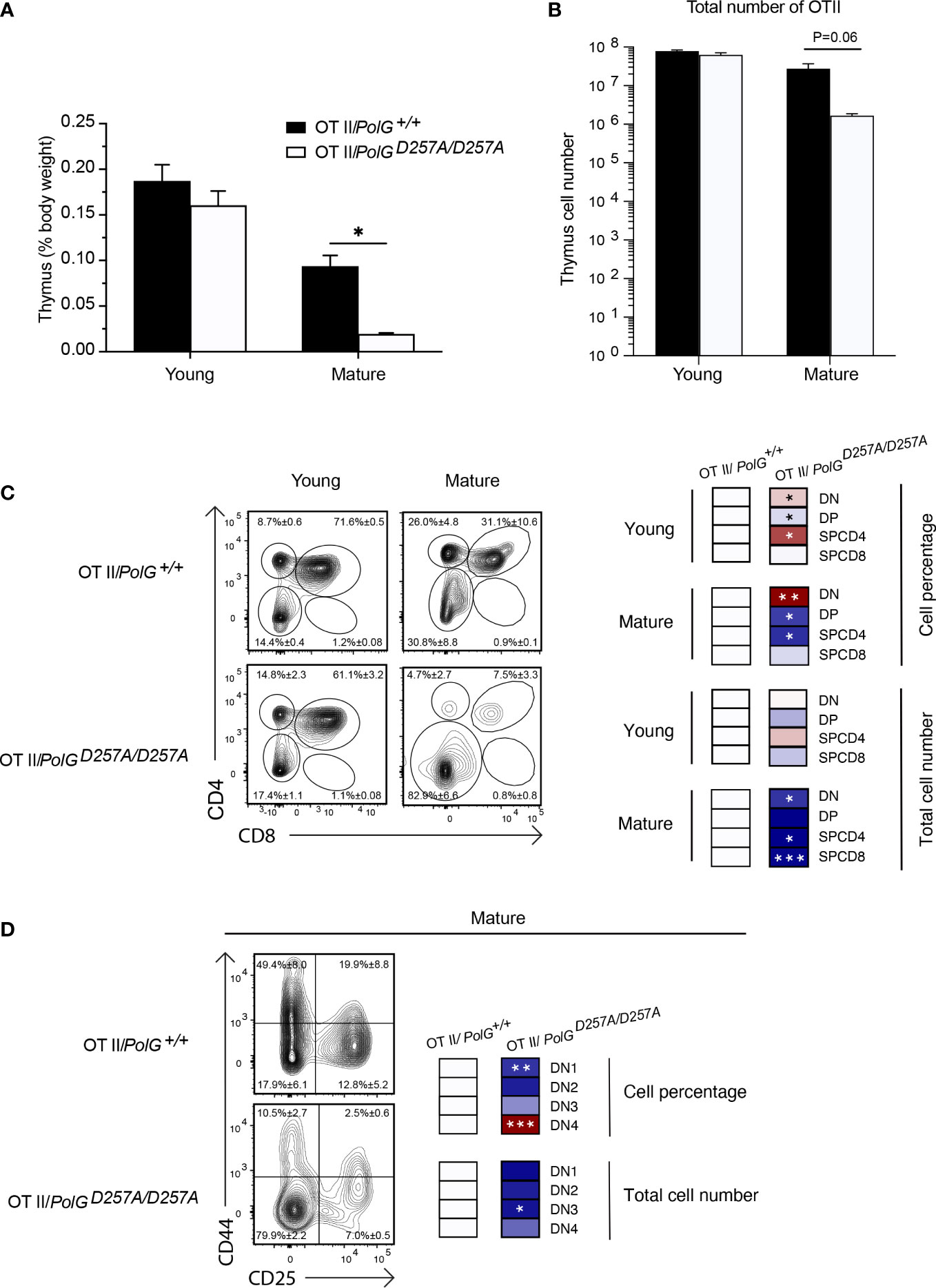
Figure 3 Mature OTII/PolGD257A/D257A mice have decreased thymi weight and increased DN populations relative to controls. (A) Thymi from mature OTII/PolG+/+ OTII/PolGD257A/D257A mice were weighed and plotted as a percentage of body weight. (B) Thymocyte numbers from mature OTII/PolG+/+ OTII/PolGD257A/D257A mice were determined and plotted. p=0.06. (C) Thymocytes from mature OTII/PolG+/+ and OTII/PolGD257A/D257A mice were stained for CD4 and CD8 and analyzed for CD4/CD8 expression. (D) Thymocytes from OTII/PolG+/+ and OTII/PolGD257A/D257A mice were stained for CD25 and CD44 to identify DN1-4 subpopulations, and percentages of the DN1-4 stages determined. Heatmaps are a representative quantification that are normalized by the mean of the control, where red indicates an increase and blue indicates a decrease. Data are displayed as mean ± standard error of mean and representative of 2 independent experiments, n=3-5 mice per group, where *p< 0.05-.01, **p< 0.01-0.001, ***p<0.001 based on multiple t tests.
Mature transgenic TCR T cells with low fidelity mtDNA polymerase have decreased mitochondrial density in DN subpopulations and exhibit less proliferation
We next wanted to explore whether the block in T cell development observed in mature non-transgenic and OTII/PolGD257A/D257A mice was associated with alterations in mitochondrial density within the double negative population. We first compared thymocytes from mature non-transgenic mice, and used a mitotracker stain, to determine mitochondrial density, gating on double negative subpopulations (DN1-4) (24). We found that the DN1 stage exhiboted and 4 stages exhibited elevated mitochondrial density, while the DN2 and 3 stages exhibited reduced mitochondrial density in PolGD257A/D257A (Figures 4A, B). This correlated with the percentage of cells in each of these stages, and for the DN2 and 3 stages, the number of these developing PolGD257A/D257A thymocytes (see Figures 2F, E)
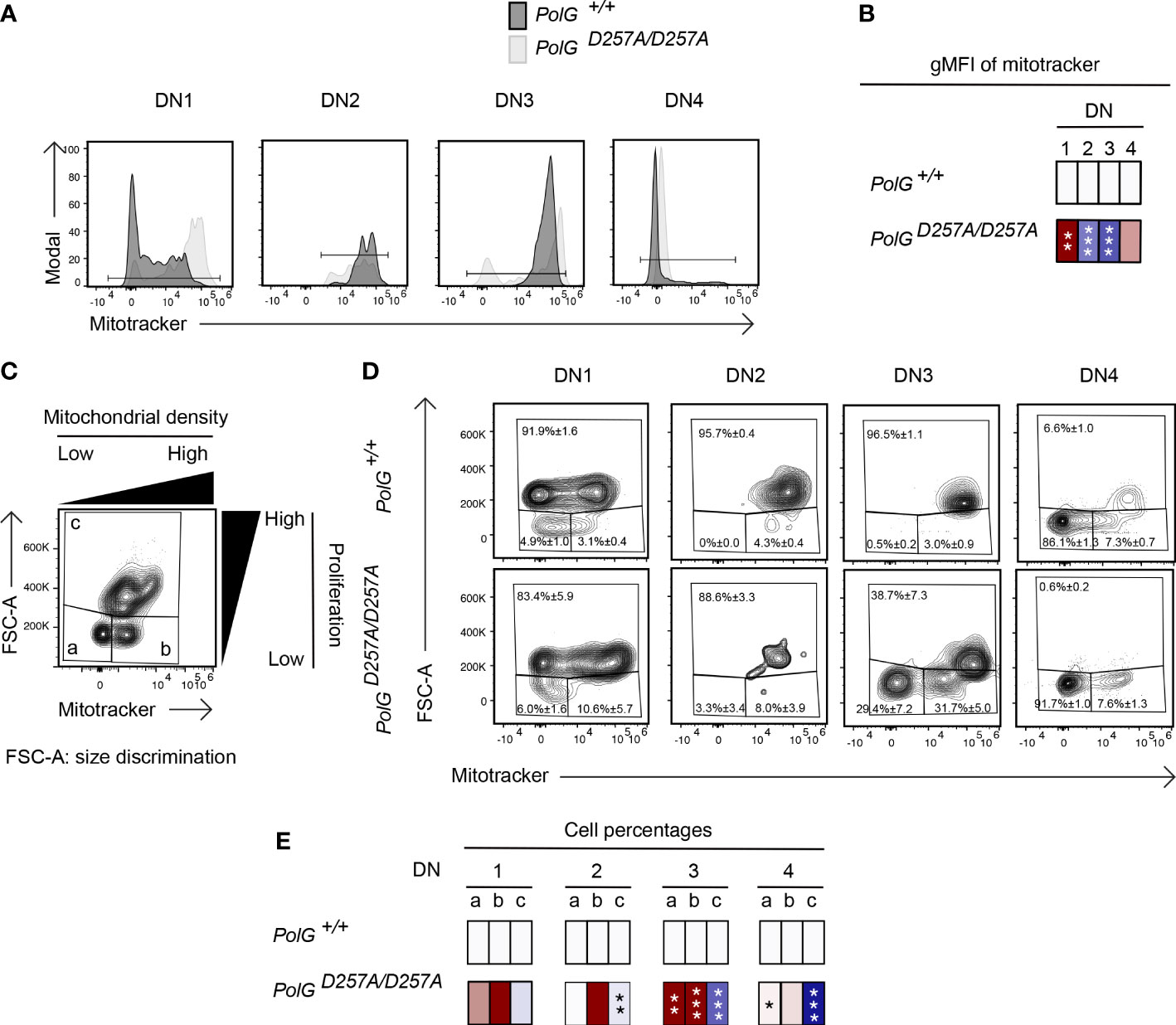
Figure 4 Reduced mitochondrial density and proliferation in DN thymocytes in mature PolGD257A/D257A mice. (A) Thymocytes from PolG+/+ and PolGD257A/D257A mice were stained for mitochondria, along with CD25 and CD44. Histograms depict mitochondria density in gated double negative subpopulations: DN1, DN2, DN3, and DN4. (B) Geometric mean fluorescence intensity (gMFI) of mitochondria density staining in DN1-4 thymocytes from PolG+/+ and PolGD257A/D257A mice. (C) Illustration of gating strategy used to discriminate larger proliferating cells and mitochondrial density and (D, E) frequencies of size (FSC-A) and mitochondrial density (mitotracker stain) in DN1-4 populations as indicated. Heatmaps are a representative quantification that are normalized by the mean of the control, where red indicates an increase and blue indicates a decrease. Data are displayed as mean ± standard error of mean and representative of 1 independent experiment, n=3 mice, where *p< 0.05-.01, **p< 0.01-0.001, ***p<0.001 based on multiple t tests.
Since these DN populations are known to be highly proliferative, we wanted to explore whether decreased mitochondrial density negatively impact these highly proliferative stages of T cell development in the mature non-transgenic mice (25–27). Figure 4D shows an analysis that determines proliferation (based on cell size using FSC-A, since the larger cell size is associated with cell cycle progression) (27, 28), where populations a and b are low proliferation populations, and population c is high (Figure 4C). The proportion of large (proliferating) cells (as determined by the increase in cell size), particularly among the DN3 cells in mature thymocytes was drastically decreased in PolGD257A/D257A mice relative to the control (Figure 4D). Additionally, there was a decrease in the mitochondrial density (shown as an increase in the cells in gate a) in the DN3 population in PolGD257A/D257A mice relative to WT (Figures 4D, E).
We next analyzed mitochondrial density within the double negative population of mature OTII/PolGD257A/D257A mice, again, using Mitotracker to determine mitochondrial density, gating on double negative subpopulations (DN1-4) (24). Here, we found that all four DN stages exhibited reduced mitochondrial density in mature OTII/PolGD257A/D257A mice (Figure 5A), with the difference increasing in the more mature stages towards DN4 (Figure 5B). Together this suggests that as thymocytes develop along the DN1-4 stages, reduced fidelity of mtDNA replication in the PolGD257A/D257A background may lead to progressively reduced mitochondrial density.
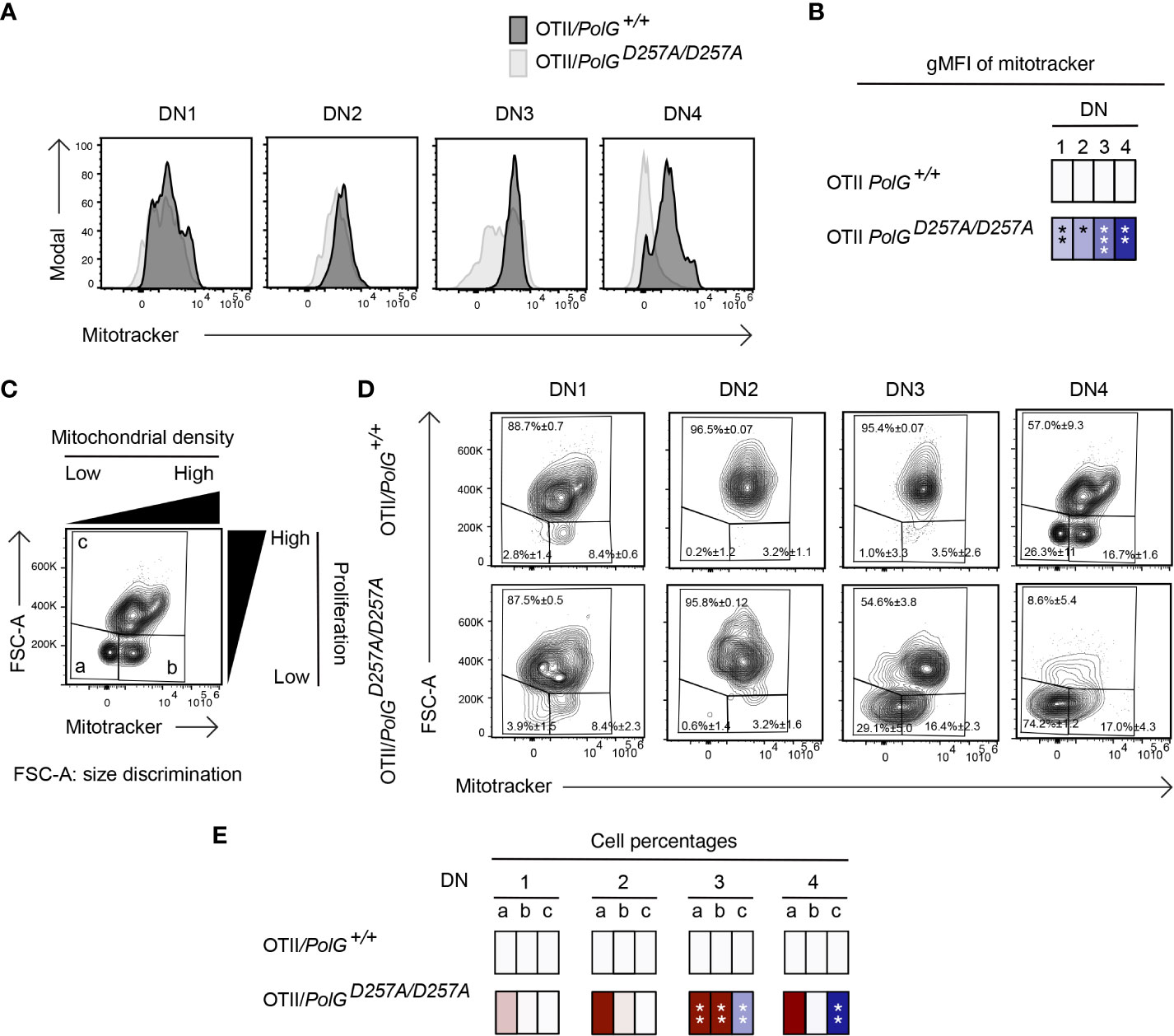
Figure 5 Reduced mitochondrial density and proliferation in DN thymocytes in mature OTII/PolGD257A/D257A mice. (A) Thymocytes from OTII/PolG+/+ and OTII/PolGD257A/D257A mice were stained for mitochondria, along with CD25 and CD44. Histograms depict mitochondria density in gated double negative subpopulations: DN1, DN2, DN3, and DN4. (B) Geometric mean fluorescence intensity (gMFI) of mitochondria density staining in DN1-4 thymocytes from OTII/PolG+/+ and OTII/PolGD257A/D257A mice. (C) Illustration of gating strategy used to discriminate larger proliferating cells and mitochondrial density and (D, E) frequencies of size (FSC-A) and mitochondrial density (mitotracker stain) in DN1-4 populations as indicated. Heatmaps are a representative quantification that are normalized by the mean of the control, where red indicates an increase and blue indicates a decrease. Data are displayed as mean ± standard error of mean and representative of 1 independent experiment, n=3 mice, where *p< 0.05-.01, **p< 0.01-0.001, ***p<0.001 based on multiple t tests.
We also analyzed these DN populations to determine whether decreased mitochondria also negatively impact this highly proliferative stage of T cell development in the mature OTII/PolGD257A/D257A mice (25–27). Figure 5D shows a similar analysis that determines proliferation (based on cell size using FSC-A, since the larger cell size is associated with cell cycle progression) (27, 28), where populations a and b are low proliferation populations, and population c is high (see Figure 5C). The proportion of large (proliferating) DN3 and DN4 cells in OTII/PolGD257A/D257A thymocytes (as determined by the increase in cell size in gate c) was drastically decreased relative to the control (Figure 5D). Additionally, there was a decrease in the mitochondrial density (shown as an increase in the cells in gate a) in the OTII/PolGD257A/D257A DN3, and again more drastically in DN4 populations relative to OTII/PolG+/+ (Figures 5D, E).
Mature OTII/PolGD257A/D257A T cells have decreased mitochondrial density and proliferation at the SP CD4+ T cell stage
Lastly, we wanted to determine whether the aberrant effects observed in the mature thymocytes continued through the rest of T cell development in the thymus. To determine this, we looked at more mature DP and SPCD4 T cell populations in the thymus. There was a decrease in the proliferating cells (larger cells in gate c) in the OTII/PolGD257A/D257A relative to the control (Figure 6A), although this was not seen in the non-transgenic PolGD257A/D257A DP cells. Examination of the more mature DP and SPCD4 OTII/PolGD257A/D257A T cell populations in the thymus revealed decreases in the mitochondrial density (gates a and b), in CD4SP cells in both non-transgenic PolGD257A/D257A and OTII/PolGD257A/D257A thymocytes, which was less apparent in DP thymocytes (cf. Figure 6B, vs A). Similar results were observed in CD4SP non-transgenic PolGD257A/D257A thymocytes (Figure 6C. note that OTII thymocytes develop into CD4SP, not CD8SP).
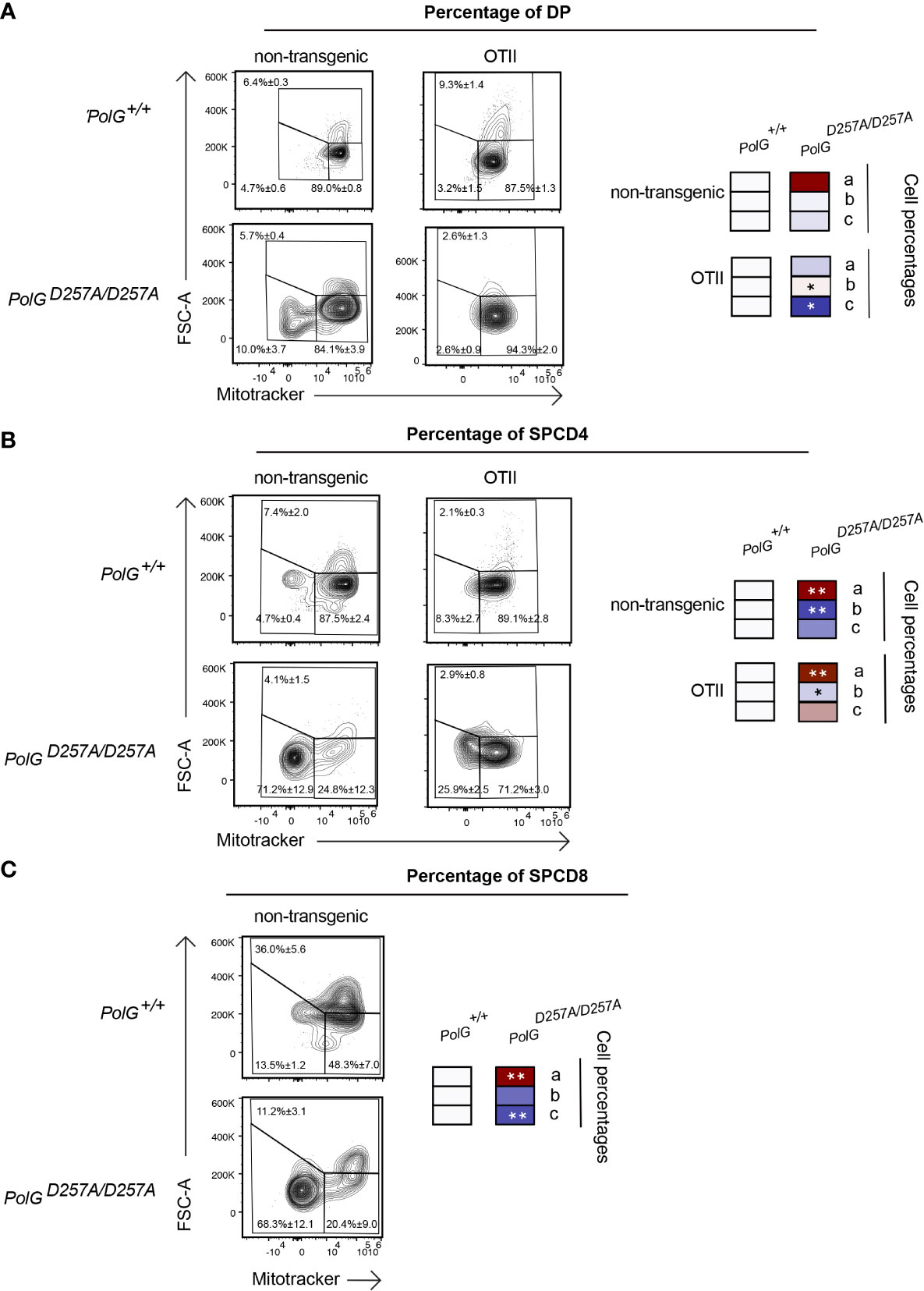
Figure 6 Single positive CD4+ T cells in mature OTII/PolGD257A/D257A mice have decreased mitochondrial density. (A) Thymocytes from PolG+/+, PolGD257A/D257A, OTII/PolG+/+ and OTII/PolGD257A/D257A mice were stained for mitotracker, along with CD4 and CD8, and the frequencies of size (FSC-A). The histograms represent mitochondrial density (mitotracker stain) in CD4/CD8 DP, (B) CD4 SP, or (C) or CD8 SP population plotted population plotted (the PolG+/+ and PolGD257A/D257A mice only in (C)). Data are displayed as mean ± standard error of mean and representative of 1 independent experiment, n=3 mice, where *p< 0.05-.01, **p< 0.01-0.001, based on multiple t tests.
Discussion
The role of mitochondria in T cell development has only recently been intensely investigated, and understanding the effects of mtDNA mutations on this process is even more limited (2). Here, we have explored the effects of reduced mtDNA replication fidelity, which leads to increase in mtDNA mutations in mice carrying the mutant PolGD257A, on T cell development. We found that reducing the fidelity of mtDNA replication results in premature age-dependent reduction in the total number of CD4/CD8 double positive and negative thymocytes. This is likely due to reduced proliferation in the highly proliferative double negative stages due to reduced mitochondrial density and the accompanying effect on mitochondrial products needed for appropriate function. Taken together, this work suggests that T cell development is regulated by the ability of mitochondria to faithfully replicate their DNA.
We examined the importance of the fidelity of the mtDNA replication in T cell development and the significance of this process in an age dependent manner, given that over time mtDNA mutations accumulate naturally in WT mice and at an accelerated rate (>500 fold) in PolGD257A mice (15). Others have previously shown that homozygous mutations in the exonuclease region of PolGD257A result in premature aging phenotypes, including the thymus, in 9-13 month old mice (15). Interestingly, 3 month old mice have an increase in apoptosis in total thymi, suggesting that there might be fewer thymocytes because of increased cell death (15). Considering the age gap between 3- and 9-month-old mice, we sought to determine whether these differences are detectable between these ages, and whether these findings depend on the dose of the PolGD275A mutant. Similar to previous findings, we saw decreased weight and total cell number of thymocytes in the mature PolGD257A/D257A mice (15, 29). That this observation was not detected in the heterozygous group suggests that one functional copy of the PolG gene may be sufficient to otherwise ameliorate negative effects.
We further investigated the effects on various T cell developmental stages. The DN stage includes TCR β chain-selection, survival, differentiation, proliferation, and allelic exclusion at the TCRβ locus (30). We predicted that we would see an increase in the DN stage in the mature PolGD257A/D257A mice relative to the control because there would be higher mutations due to the high proliferation rates, and the findings supported this prediction. There are different factors that distinguish progression through the DN stage, discriminated between DN1-4 stages. DN1-2 stages or early thymic precursors are the earliest clearly identifiable intrathymic stages (23). We observed an increase in the DN1 population in the mature PolGD257A/D257A mice relative to the controls. This suggests that there may be a developmental stall in the DN1 stage, thus reducing progression to the subsequent stages and affecting further T cell development.
T cell commitment occurs at the end of the DN2 prior to entry into the DN3, and during this stage they traverse a significant proliferative expansion (31). We observed a decrease in the cell number and percentage of DN3 population in the PolGD257A/D257A mice, suggesting that the fidelity of mtDNA replication significantly affects the highly proliferative cells in the DN3 stage. A possible reason for the reduction in the number of these cells is increased cell death, although this requires further investigation. This finding highlights the importance of functional mitochondria and mitochondrial products in this highly proliferative stage, which may be more sensitive to higher mtDNA mutational burden in the PolGD257A/D257A mice.
The DP stage, which follows the highly proliferative DN stage, is where the CD4 and CD8 co-receptors are upregulated and expression of the rearranged TCRα and β chains occur (30). Following which, T cells go through positive selection where the TCRs are tested for having appropriate avidity for the MHC/peptide. These stages do not require high proliferation like the DN stage. Therefore, we predicted that we would not see a difference in the percentage of these cells, but a decrease in the cell number in the PolGD257A/D257A mice relative to the control, and this conclusion aligned with the data.
We also examined the effect of increased mtDNA mutations on T cell development in OTII/PolGD257A/D257A transgenic mice. The rationale for our analysis of OTII/PolGD257A/D257A mice was that the presence of the already rearranged TCR accelerates the progress through the DN developmental stages, when gene segments would normally rearrange and encode the TCR alpha and beta chains. Unlike the non-transgenic mice, T cell development in the transgenic mice do not have to go through TCR gene rearrangement in the same manner as non-transgenic mice, since unlike the non-transgenic mice, T cells in the transgenic mice do not have to go through TCR VDJ recombination at the alpha and beta loci during their development (32). Thus, if the increased proliferation during these stages was inordinately affected by the low fidelity mtDNA replication, then these stages could potentially be less affected in these transgenic thymocytes. Notably, we did not see the same trend of increased numbers of thymocytes in the DN stage in the OTII/PolGD257A/D257A mice. However, we found that there was a block later in the DN stage, with an increase in the percentage of DN4 in the mature OTII/PolGD257A/D257A mice relative to the control.
We explored potential mechanisms for these observed defects in T cell development in the mutant mice by analysis of mitochondrial density and cell size, as a proxy for cell division. Abnormal mtDNA replication can lead to altered expression of mitochondrial proteins which are important for the electron transport chain and can cause decreased oxidative phosphorylation and activate mitochondrial degradation through mitophagy. If there are fewer mitochondria to make appropriate substrates for cell replication, this can alter metabolism and cause deleterious effects to cell proliferation (2).
Further analysis of the mitochondrial density indicated that there was decreased in the DN2 and 3 populations in PolGD257A/D257A mice compared to the controls. Furthermore, there is a notable reduced proliferation in the DN3 population in the PolGD257A/D257A relative to WT. This could be due to a buildup of mtDNA mutations relative to the control at this stage. Furthermore, it is possible that increased mtDNA mutation could result in decreased mitochondrial function and increased mitophagy. By contrast, the OTII/PolGD257A/D257A DN1-4 populations exhibited decreased mitochondrial density compared to the controls. Furthermore, there is a notable reduced proliferation in DN3 and DN4 populations in the OTII/PolGD257A/D257A relative to WT. This trend appeared to increase over differentiation stage, where the largest differences between mitochondrial density and proliferation were observed at the DN4 stage. This could also be a due to a larger buildup of mtDNA mutations relative to the control at this stage, and increased mitophagy. One major limitation to our findings is that it is unclear whether they are influenced by non-T cell dependent factors given that PolGD257A/D257A and OTII/PolGD257A/D257A models are globally mutated. Therefore, future experiments will need to be conducted to determine whether our observations are due to T cell intrinsic effects.
Finally, our investigation of T cell developmental stages beyond the DN stage in non-transgenic and OTII transgenic mice revealed that while proliferation of DP thymocytes in mature non-transgenic PolGD257A/D257A is not obviously affected compared to WT mice, this is impaired in OTII/PolGD257A/D257A compared to WT OTII mice. This suggests that the PolGD257A/D257A mutant cells are not all selectively inhibited from further development given their presence, but that the rearranged transgene may affect their progression, as it does in the DN stage. Notably however, there increased percentage of low mitochondrial density in the mature non-transgenic PolGD257A/D257A and OTII/PolGD257A/D257A SPCD4 (as well as SP CD8 in non-transgenic mice, note that OTII thymocytes develop into CD4 SP cells due to the nature of the rearranged TCR) with respect to the controls. The observed low mitochondrial density may be an indicator that there is a decreased capacity to quickly make new mitochondrial due to possibly aberrant mitochondrial function. There were also decreases in the total number of CD4+ and CD8+ T cells in the lymph node of mature PolGD257A/D257A mice, but interestingly, not in the spleen (Supplemental Figure 2). These findings suggest that the mature T cells may be affected by reduced fidelity of mitochondrial DNA replication, in an organ specific manner.
There are drugs with reported side effects of decreased mtDNA content and mitochondrial functional impairment similar to what is observed in the PolG mouse model (33, 34). One common observation is mitochondrial toxicity seen with the use of reverse transcriptase inhibitors which can resemble purines (adenosine and guanosine) and pyrimidines (cytidine, thymine, and uridine), known as nucleoside-analogue reverse-transcriptase inhibitor. These inhibitors are widely utilized as an antiviral treatment with patients who have viral infections (35). It is currently unclear whether such drugs induce similar alterations as we observe in the PolG mutant mice.
Altogether our data revealed that the fidelity of mtDNA replication is critical to T cell development, affecting proliferation in specific DN stages. This reduced proliferation and block in the DN stages may be due to decreased mitochondrial density, resulting in reduced proliferation and development of T cells. This study contributes to our understanding of PolG and the fidelity of mtDNA replication in T cell development, providing new insight into how mitochondria affect this process.
Materials and methods
Mice
All mice were maintained and housed in specific pathogen-free facilities and experiments were performed in accordance with protocols that were approved by the Institutional Animal Care and Use Committee at University of Cornell University. PolGD257A/D257A mice have been previously described and obtained from the Jax labs (15). Male PolG+/D257A and PolGD257A/D257A were backcrossed with WT C57BL/6J, mice, to generate male and female heterozygous and homozygous littermates which were then crossed and used for this study. The presence of PolGD257A knock-in mutation was determined by PCR using the following primers in genotyping (5’ to 3’; reverse common: AGT CCT GCG CCA ACA CAG; wildtype forward: GCT TTG CTT GAT CTC TGC TC; mutant forward: ACG AAG TTA TTA GGT CCC TCG AC). OTII transgenic mice were also obtained from the Jax labs and crossed with PolGD257A/D257A and then the OTII/PolGD257A/+ were crossed with OTII/PolGD257A/+ and the subsequent generation were used for experiments.
Antibodies for flow cytometry
The following antibodies were used for flow cytometry analysis: PerCP-Cy5.5-anti-CD25, PE-anti-CD24, CD44-FITC, PE-CF594-anti-CD4 (BD Biosciences, Inc.), PacificBlue-anti-CD4, PacificBlue-anti-CD8a, eF506-viability dye (eBioscience Inc.), and AF700-anti-CD8a, Pecy7-anti-TCRβ (BioLegend). Mitotracker green (Cell Signaling Technology) was used to detect mitochondrial density.
Flow cytometry
Organs were mechanically dissociated through 70 µM screen placed in complete RPMI media while on ice. For surface staining, cells were stained, washed, and all flow cytometry analysis was conducted in the Cornell University Flow Cytometry Core Facility using the Thermo Fisher Attune NxT and data was analyzed in FlowJo (Tree Star, Ashland, OR) where all data were performed gating on doublet excluded viable cells.
Data extraction and statistical analysis
Flow cytometry data was extracted and analyzed using code through RStudio 2022.07.1 Build 554 (GitHub repository TMPierpont/tmisBasic/Heatmap/R/timsDataLoader.R 2022). This code was used RStudio to export percentages from FlowJo analysis and to calculate total cell numbers. The flow cytometry gates show average cell percentages, and heatmaps are a representative quantification that are normalized by the mean of the control. Statistical analyses were carried out utilizing Prism 9 (GraphPad, San Diego CA) and RStudio. Statistical significance was set at p<0.05, and all data are reported as a mean, plus and minus standard error of the mean (SEM). Age and genotype were compared by multiple t-tests.
Data availability statement
The original contributions presented in the study are included in the article/Supplementary Materials. Further inquiries can be directed to the corresponding author.
Ethics statement
The animal study was reviewed and approved by Institutional Animal Care and Use Committee at Cornell University.
Author contributions
AA conceived the project, supervised experiments, and analyzed data. CL, NB, DZ, UC, ANV, and WH performed experiments. CL analyzed the data. AA and CL wrote the manuscript. All authors contributed to the article and approved the submitted version.
Funding
This work was supported in part by grants from the National Institutes of Health (AI120701 and AI138570 to AA, AI129422 to AA and WH) and a HHMI Professorship (AA). CL is a Cornell Sloan Scholar.
Acknowledgments
We thank the Cornell animal care facilities for their animal care, Amie Redko for maintaining the breeder colony and Ling Zhang for genotyping. The following Cornell University core facilities have also made this research possible: the Institute of Biotechnology for collecting the data and Statistical Consulting Unit for assistance with data analysis. Lastly, we thank members of the August lab for comments and suggestions.
Conflict of interest
AA receives research support from 3M Company, WH receives research support from MegaRobo.
The remaining authors declare that the research was conducted in the absence of any commercial or financial relationships that could be construed as a potential conflict of interest.
Publisher’s note
All claims expressed in this article are solely those of the authors and do not necessarily represent those of their affiliated organizations, or those of the publisher, the editors and the reviewers. Any product that may be evaluated in this article, or claim that may be made by its manufacturer, is not guaranteed or endorsed by the publisher.
Supplementary material
The Supplementary Material for this article can be found online at: https://www.frontiersin.org/articles/10.3389/fimmu.2023.1128626/full#supplementary-material
Supplementary Figure 1 | No difference in PolG mRNA expression in neonatal an adult thymus. RNA-Sequencing data from neonatal (6-8 days old) and adult gBT-1 thymocytes (2-4 months old, GSE80597) was analyzed for expression of polg. Results not statistically significant.
Supplementary Figure 2 | Reduced number of T cells in Lymph nodes, but not spleen, in mature PolGD257A/D257A mice. Lymph node and spleen cells were analyzed for number of CD4+ and CD8+ T cells.
References
1. Kreslavsky T, Gleimer M, Miyazaki M, Choi Y, Gagnon E, Murre C, et al. β-selection-induced proliferation is required for αβ T cell differentiation. Immunity (2012) 37:840–53. doi: 10.1016/j.immuni.2012.08.020
2. Sun V, Sharpley M, Kaczor-Urbanowicz KE, Chang P, Montel-Hagen A, Lopez S, et al. The metabolic landscape of thymic T cell development In vivo and In vitro. Front Immunol (2021) 12:716661. doi: 10.3389/fimmu.2021.716661
3. Calvo SE, Clauser KR, Mootha VK. MitoCarta2.0: an updated inventory of mammalian mitochondrial proteins. Nucleic Acids Res (2016) 44:D1251–7. doi: 10.1093/nar/gkv1003
4. Yasukawa T, Kang D. An overview of mammalian mitochondrial DNA replication mechanisms. J Biochem (Tokyo) (2018) 164:183–93. doi: 10.1093/jb/mvy058
5. Sasaki T, Sato Y, Higashiyama T, Sasaki N. Live imaging reveals the dynamics and regulation of mitochondrial nucleoids during the cell cycle in Fucci2-HeLa cells. Sci Rep (2017) 7:11257. doi: 10.1038/s41598-017-10843-8
6. Lee WTY, John J. St. the control of mitochondrial DNA replication during development and tumorigenesis. Ann N Y Acad Sci (2015) 1350:95–106. doi: 10.1111/nyas.12873
7. Quiros PM, Goyal A, Jha P, Auwerx J. Analysis of mtDNA/nDNA ratio in mice. Curr Protoc Mouse Biol (2017) 7:47–54. doi: 10.1002/cpmo.21
8. Lukas J, Lukas C, Bartek J. More than just a focus: The chromatin response to DNA damage and its role in genome integrity maintenance. Nat Cell Biol (2011) 13:1161–9. doi: 10.1038/ncb2344
9. Roy A, Kandettu A, Ray S, Chakrabarty S. Mitochondrial DNA replication and repair defects: Clinical phenotypes and therapeutic interventions. Biochim Biophys Acta Bioenerg (2022) 1863:148554. doi: 10.1016/j.bbabio.2022.148554
10. Macao B, Uhler JP, Siibak T, Zhu X, Shi Y, Sheng W, et al. The exonuclease activity of DNA polymerase γ is required for ligation during mitochondrial DNA replication. Nat Commun (2015) 6:7303. doi: 10.1038/ncomms8303
11. Hiona A, Sanz A, Kujoth GC, Pamplona R, Seo AY, Hofer T, et al. Mitochondrial DNA mutations induce mitochondrial dysfunction, apoptosis and sarcopenia in skeletal muscle of mitochondrial DNA mutator mice. PloS One (2010) 5:e11468. doi: 10.1371/journal.pone.0011468
12. Clark-Matott J, Saleem A, Dai Y, Shurubor Y, Ma X, Safdar, et al. Metabolomic analysis of exercise effects in the POLG mitochondrial DNA mutator mouse brain. Neurobiol Aging (2015) 36:2972–83. doi: 10.1016/j.neurobiolaging.2015.07.020
13. Radsak K, Schütz E. Changes of mitochondrial DNA polymerase-γ activity in synchronized mouse cell cultures. Eur J Biochem (1978) 89:3–9. doi: 10.1111/j.1432-1033.1978.tb20889.x
14. Chatre L, Ricchetti M. Prevalent coordination of mitochondrial DNA transcription and initiation of replication with the cell cycle. Nucleic Acids Res (2013) 41:3068–78. doi: 10.1093/nar/gkt015
15. Kujoth GC, Hiona A., Pugh TD, Someya S, Panzer K, Wohlgemuth SE, et al. Mitochondrial DNA mutations, oxidative stress, and apoptosis in mammalian aging. Science (2005) 309:481–4. doi: 10.1126/science.1112125
16. Vermulst M, Bielas JH, Kujoth GC, Ladiges WC, Rabinovitch PS, Prolla TA, et al. Mitochondrial point mutations do not limit the natural lifespan of mice. Nat Genet (2007) 39:540–3. doi: 10.1038/ng1988
17. Life span as a biomarker. In: The Jackson laboratory. Available at: https://www.jax.org/research-and-faculty/research-labs/the-harrison-lab/gerontology/life-span-as-a-biomarker.
18. Trifunovic A, Wredenberg A, Falkenberg M, Spelbrink JN, Rovio AT, Bruder CE, et al. Premature ageing in mice expressing defective mitochondrial DNA polymerase. Nature (2004) 429:417–23. doi: 10.1038/nature02517
19. Tan C, Taylor AA, Coburn MZ, Marino JH, Van De Wiele CJ, Teague TK. Ten-color flow cytometry reveals distinct patterns of expression of CD124 and CD126 by developing thymocytes. BMC Immunol (2011) 12:36. doi: 10.1186/1471-2172-12-36
20. Clarke S, Barnden M, Kurts C, Carbone FR, Miller JF, Heath WR. Characterization of the ovalbumin-specific TCR transgenic line OT-I: MHC elements for positive and negative selection. Immunol Cell Biol (2000) 78:110–7. doi: 10.1046/j.1440-1711.2000.00889.x
21. Barnden MJ, Allison J, Heath WR, Carbone FR. Defective TCR expression in transgenic mice constructed using cDNA-based alpha- and beta-chain genes under the control of heterologous regulatory elements. Immunol Cell Biol (1998) 76:34–40. doi: 10.1046/j.1440-1711.1998.00709.x
22. Krotkova A, Smith E, Nerz G, Falk I, Eichmann K. Delayed and restricted expression limits putative instructional opportunities of Vγ1.1/Vγ2 γδ TCR in αβ/γδ lineage choice in the thymus. J Immunol (2004) 173:25–32. doi: 10.4049/jimmunol.173.1.25
23. Rothenberg EV, Moore JE, Yui MA. Launching the T-lineage developmental programme. Nat Rev Immunol (2008) 8:9–21. doi: 10.1038/nri2232
24. Nangaku M, Sato-Yoshitake R, Okada Y, Noda Y, Takemura R, Yamazaki H, et al. KIF1B, a novel microtubule plus end-directed monomeric motor protein for transport of mitochondria. Cell (1994) 79:1209–20. doi: 10.1016/0092-8674(94)90012-4
25. Penit C, Ezine S. Cell proliferation and thymocyte subset reconstitution in sublethally irradiated mice: compared kinetics of endogenous and intrathymically transferred progenitors. Proc Natl Acad Sci U S A (1989) 86:5547–51. doi: 10.1073/pnas.86.14.5547
26. Petrie HT, Tourigny M, Burtrum DB, Livak F. Precursor thymocyte proliferation and differentiation are controlled by signals unrelated to the pre-TCR. J Immunol Baltim Md 1950 (2000) 165:3094–8. doi: 10.4049/jimmunol.165.6.3094
27. Tourigny MR, Mazel S, Burtrum DB, Petrie HT. T Cell receptor (TCR)-beta gene recombination: dissociation from cell cycle regulation and developmental progression during T cell ontogeny. J Exp Med (1997) 185:1549–56. doi: 10.1084/jem.185.9.1549
28. Miettinen TP, Björklund M. Cellular allometry of mitochondrial functionality establishes the optimal cell size. Dev Cell (2016) 39:370–82. doi: 10.1016/j.devcel.2016.09.004
29. Chen ML, Logan TD, Hochberg ML, Shelat SG, Yu X, Wilding GE, et al. Erythroid dysplasia, megaloblastic anemia, and impaired lymphopoiesis arising from mitochondrial dysfunction. Blood (2009) 114:4045–53. doi: 10.1182/blood-2008-08-169474
30. Baldwin TA, Sandau MM, Jameson SC, Hogquist KA. The timing of TCRα expression critically influences T cell development and selection. J Exp Med (2005) 202:111–21. doi: 10.1084/jem.20050359
31. Bao X, Qin Y, Lu L, Zheng M. Transcriptional regulation of early T-lymphocyte development in thymus. Front Immunol (2022) 13:884569. doi: 10.3389/fimmu.2022.884569
32. Roberts JL, Lauzurica P, Krangel MS. Developmental regulation of VDJ recombination by the core fragment of the T cell receptor α enhancer. J Exp Med (1997) 185:131–40. doi: 10.1084/jem.185.1.131
33. Setzer B, Schlesier M, Walker UA. Effects of didanosine-related depletion of mtDNA in human T lymphocytes. J Infect Dis (2005) 191:848–55. doi: 10.1086/427655
34. Ciesielska EJ, Kim S, Bisimwa HM, Grier C, Rahman MM, Young CKJ, et al. Remdesivir triphosphate blocks DNA synthesis and increases exonucleolysis by the replicative mitochondrial DNA polymerase, pol γ. Mitochondrion (2021) 61:147–58. doi: 10.1016/j.mito.2021.09.010
Keywords: DNA polymerase γ, mutator mice, T cell development, thymus, mitochondria, aging, proliferation
Citation: Limper CB, Bondah N, Zhu D, Villanueva AN, Chukwukere UK, Huang W and August A (2023) Effective differentiation of double negative thymocytes requires high fidelity replication of mitochondrial DNA in an age dependent manner. Front. Immunol. 14:1128626. doi: 10.3389/fimmu.2023.1128626
Received: 21 December 2022; Accepted: 03 March 2023;
Published: 20 March 2023.
Edited by:
Bin Zhao, Second Xiangya Hospital, Central South University, ChinaCopyright © 2023 Limper, Bondah, Zhu, Villanueva, Chukwukere, Huang and August. This is an open-access article distributed under the terms of the Creative Commons Attribution License (CC BY). The use, distribution or reproduction in other forums is permitted, provided the original author(s) and the copyright owner(s) are credited and that the original publication in this journal is cited, in accordance with accepted academic practice. No use, distribution or reproduction is permitted which does not comply with these terms.
*Correspondence: Avery August, YXZlcnlhdWd1c3RAY29ybmVsbC5lZHU=