- Key Laboratory of Major Chronic Diseases of Nervous System of Liaoning Province, Health Sciences Institute of China Medical University, Shenyang, China
Neurodegenerative diseases (NDs) are chronic conditions that result in progressive damage to the nervous system, including Alzheimer’s disease (AD), Parkinson’s disease (PD), Huntington’s disease (HD), and Amyotrophic lateral sclerosis (ALS). Age is a major risk factor for NDs. Telomere shortening is a biological marker of cellular aging, and telomerase reverse transcriptase (TERT) has been shown to slow down this process by maintaining telomere length. The blood-brain barrier (BBB) makes the brain a unique immune organ, and while the number of T cells present in the central nervous system is limited, they play an important role in NDs. Research suggests that NDs can be influenced by modulating peripheral T cell immune responses, and that TERT may play a significant role in T cell senescence and NDs. This review focuses on the current state of research on TERT in NDs and explores the potential connections between TERT, T cells, and NDs. Further studies on aging and telomeres may provide valuable insights for developing therapeutic strategies for age-related diseases.
1 Introduction
Neurodegenerative diseases (NDs) are a group of neurological disorders characterized by the loss of neurons or myelin sheaths, leading to progressive motor and cognitive impairment. The most common forms of these diseases include Alzheimer’s Disease (AD), Parkinson’s Disease (PD), Huntington’s Disease (HD), and Amyotrophic Lateral Sclerosis (ALS). The development of NDs is linked to a range of factors, including aging, oxidative stress, inflammation, mitochondrial dysfunction, and protein aggregate accumulation (1–5). Aging is the most significant risk factor and is characterized by metabolic dysfunction, mitochondrial dysfunction, and telomere wear (6). Understanding and addressing the aging process has the potential to improve many age-related conditions, including neurodegenerative diseases. Immune senescence, marked by a decline in T cell immune function, is a common feature of aging. However, it is not very clear whether immune senescence is associated with NDs, and T-cell aging drives aging of other non-immune organs (7). Additionally, Natural Killer (NK) cells increase progressively in the brain with age. Studies have found that clearing immune cells in the aging brain promotes neuroblastoma cell survival and improve cognitive function (8). T-lymphocyte senescence is primarily caused by shortened telomere length or impaired telomerase function (9). Telomeres, widely recognized as hallmarks of aging, have also been implicated in the process of neurodegeneration in neurodegenerative diseases (10, 11).
Telomeres are repetitive DNA sequences (TTAGGG)n located at the ends of eukaryotic linear chromosomes. They protect cells during replication, prevent DNA damage, and regulate the cell division cycle through their telomeric DNA and telomere binding proteins (12, 13). As cells divide, telomeres gradually shorten, but when this reaches a critical point, telomerase can be activated to lengthen telomeres, allowing cells to survive a crisis state or replicate indefinitely (14, 15). Telomerase activity, which is essential for maintaining telomere length, is primarily composed of the Telomerase reverse transcriptase (TERT) protein, telomerase RNA component (TERC), and telomerase-related catalytic proteins (16, 17). TERT is the primary determinant of telomerase activity, and it is normally found in germ cells, neurons, and stem cells, but is inactive in somatic cells (18–22). Exploring telomere biology offers the potential to search for effective strategies for treating neurodegenerative diseases. The expression of TERT can be influenced by various factors, such as cycloastragenol (CAG), a telomerase activator, which has been shown to promote TERT expression in rat neurons (21–23). GRN510, a novel small molecule compound, is another example of a telomerase activator that has protective effects against neurological diseases by upregulating TERT expression (24). TERT levels remain constant during embryonic development, despite decreasing telomerase activity (25). TERT has been found to play multiple roles, including neuronal protection, anti-inflammation, antioxidant, tumor suppression, immunomodulation, and reduction of toxic proteins (26). TERT can also reduce oxidative stress by transporting from the nucleus into mitochondria and reducing the production of reactive oxygen species (ROS) (27, 28) Upregulated TERT expression in microglia has also been shown to inhibit the production of inflammatory factors and have anti-inflammatory effects (29).
Oxidative stress is a significant contributor to the development of neurodegenerative diseases and TERT has been found to play a role in addressing these conditions. As such, TERT has emerged as a promising strategy for the treatment of age-related NDs (30, 31) Research has shown that telomerase activation promotes lymphocyte proliferation and slows down cellular senescence, while TERT upregulation prevents or reverses tissue and organ degeneration and premature aging in mice (11). Neurodegenerative diseases are characterized by an altered balance of oxidation-reduction, leading to disruptions in cellular signaling pathways and the regulation of the immune response and inflammatory processes through ROS (32). In NDs, the intracellular accumulation of amyloid β protein and neuronal death is accompanied by increased Th1-Th17 responses and decreased Th2 responses (33). In the central nervous system, pro-inflammatory factor-mediated neuroinflammation in glial cells can be regulated by peripheral T cells, while CD8+ T cells and CD4+ T cells are capable of inducing significant neuronal death (34, 35). IL-4-deficient mice with inflammatory myeloid cells exhibit cognitive deficits, but wild-type T cells can reverse this effect (36).
In conclusion, TERT has the potential to play a role in NDs by regulating oxidative stress and inflammatory responses, which can impact Treg cells. The progress of research on the function of TERT in NDs is discussed in this paper.
2 TERT and T cells
The role of T lymphocytes in the immune system and the relationship between TERT and T cells have been widely studied. T lymphocytes are a type of immune cell that mature in the thymus and are the main component of lymphocytes. T lymphocytes are the most numerous and complex in terms of function, and aging can decrease and abnormalize the body’s immune function by affecting T lymphocytes (37). Regulatory T cells (Treg cells) are a subset of T cells that play an important role in maintaining self-tolerance, suppressing autoimmunity, and regulating inflammation in neurodegeneration. It has been shown that Treg cells are involved in an adaptive response when the cellular environment is altered, in order to maintain the tissue internal environment’s homeostasis (38).
TERT is an antigen that is closely related to the development of immunotherapies, and T cells are at the center of the “immune surveillance” process (39). The expression of TERT has been found to be closely associated with immunosuppressive features in the form of Th2 cells, Treg cells, CD56dim natural killer cells, and myeloid-derived suppressor cells (40). Additionally, overexpression of the TERT gene has been shown to enhance the proliferative capacity of T cells, which provides a potential therapeutic strategy for diseases such as tumors (41). TERT is expressed in all stages of tumor differentiation and is a crucial target for immunotherapy, with CD4 T cells playing a role in combating TERT in cancer (39). Inflammation affects the body’s immune response, and numerous studies have confirmed that several inflammatory factors, including IL-2 and IL-6, can promote the expression of telomerase in T cells and keep it at high levels (42). The NF-κB signaling pathway is an important pathway that regulates the inflammatory response, and its downstream target genes include inflammatory factors such as IL-6, IL-8, and TNF-α, the overexpression of which leads to immune dysfunction. TERT has a forward feedback regulation with the NF-κB signaling pathway, allowing it to regulate the inflammatory immune response of cells (43). The transcriptional regulation of NF-κB by TERT has been shown to be responsible for the expression of genes in metabolic syndrome and the regulation of immune cell function (44, 45). Additionally, TERT gene-transduced T cells have an enhanced ability to resist oxidative stress (46), and there is evidence that T cells overexpressing TERT have a stronger rate of proliferation and are not malignant (47). Importantly, siRNA mediated TERT knockdown increased cellular ROS levels, while TERT overexpression inhibited endogenous ROS production (48). In vitro experiments showed that reducing TERT activity promoted oxidative stress, leading to cell death (26, 49). With aging, telomere length shortening occurs in various organs, causing more cells to enter the senescent phase and lose their proliferative capacity (50).
The role of T cells in the brain is increasingly being recognized and as the relationship between aging and neurodegenerative diseases becomes more evident, there is growing interest in studying T cells for potential therapeutic insights into these aging-related conditions. Although there is limited research on TERT and T cells, the potential for further exploration is significant.
A recent study found that T cells play an important role in the brain and considering the relationship between aging and neurodegenerative diseases (51), it is not difficult to imagine that a deeper study of T cells may provide important therapeutic clues for aging-related diseases, including neurodegenerative diseases. Despite the relatively few studies related to TERT and T cells, there is great value for exploration.
3 Role of TERT in neurodegenerative diseases
As previously mentioned, the aging process is closely tied to the development of neurodegenerative diseases. This is often characterized by oxidative stress, neuroinflammation, and an altered immune response. Research has shown that prolonged exposure to pro-oxidants leads to the production of reactive oxygen species (ROS) which, in turn, causes cellular damage through inflammation and mitochondrial dysfunction. In short, there is an interaction between oxidative stress and inflammatory responses, and the inflammatory factors produced by the body can potentially cause an immune response that can affect the progression of NDs. This results in increased expression of IL-17 and Syntaxin5 in the hippocampus of the brain, leading to cognitive impairment (32). Given the anti-aging and antioxidant effects of TERT, it is essential to further examine the relationship between TERT and various neurodegenerative diseases.
3.1 TERT and AD
Alzheimer’s disease (AD) is a common neurodegenerative disorder that increases in incidence and prevalence with age (52–55). It is predominantly found in individuals over 65 years old (56). The underlying pathological features of AD are the presence of large numbers of senile plaques (SPs) formed by beta-amyloid peptide (Aβ) deposits outside the neuros (57) in the brain, as well as neurofibrillary tangles (NFTs) formed by tau-hyperphosphorylation (58, 59), oxidative stress (60), and alterations in cholinergic signaling (61). Studies have demonstrated that oxidative stress plays a critical role in the development and progression of AD, promoting Aβ deposition, Tau protein hyperphosphorylation, metal ion imbalances, synaptic and neuronal loss, and mitochondrial dysfunction (62, 63). Thus, targeting oxidative stress holds great potential as a therapeutic strategy for AD.
Studies have shown that patients with Alzheimer’s disease (AD) have elevated levels of CD4+/CD8+ T cells in peripheral blood, which is related to the permeability of the blood-brain barrier, which increases as the disease progresses, promoting the infiltration of T cells into the brain tissue (64–66). There are conflicting results regarding the role of T cells in AD, with some studies suggesting a neuroprotective role for Treg cells and an improvement in cognitive function with higher levels of Treg cells and IL-35 (67). On the other hand, elevated levels of TEMRA CD8 T cells, which are cytotoxic and secrete inflammatory molecules, have been found to impair cognitive performance in AD patients (68, 69). Furthermore, peripheral T cell activation and the subsequent release of pro-inflammatory factors such as IL-6, tumor necrosis factor α (TNFα), and IL-1, have also been linked to AD pathology (70). While multiple studies have suggested a role for T cells in AD development, their specific mechanisms of action and impact on the disease remain unclear.
The length of telomeres, which serve as a marker of cellular aging, are critical to normal brain function (71, 72). Given this importance, the potential benefits of TERT in preventing and treating Alzheimer’s disease (AD) have garnered significant attention. AD is predominantly seen in the elderly population, but studies have shown that TERT levels in the brains of AD patients remain unchanged with disease progression (73). TERT has been found to have multiple biological roles in adult brain neurons, including the accumulation in the mitochondria of AD patients’ brains and the prevention of neuronal damage from pathological proteins (74). Additionally, TERT has been shown to alleviate memory impairment in AD (75). AGS treatment was found to temporarily increase TERT gene expression in hippocampal primary cell cultures, either with or without Aβ, and ultimately protect neurons from Aβ-induced neuronal degeneration by increasing expression of neurotrophic factors, neuronal plasticity genes, and activating the Wnt/beta-catenin pathway (76). The telomerase protein TERT confers neuronal resistance to pathological tau by reducing the production of oxidative species and improving mitochondrial function rather than altering tau protein (77). Furthermore, neurons treated with TERT inhibitors were found to be more susceptible to Aβ damage, while TERT was able to protect neurons from Aβ-induced apoptosis in an experimental model of AD (78, 79).In conclusion, TERT may be a promising target for AD treatment and provide a new direction for research. However, its specific mechanisms of action require further investigation.
PD is a neurodegenerative disease that is second only to AD in prevalence worldwide (80) The aging population is leading to a growing number of PD patients (81). PD primarily affects the elderly, causing progressively worsening and irreversible symptoms, which can be divided into motor and non-motor symptoms (82). Pathologically, PD is characterized by the loss of neurons in the substantia nigra, the presence of eosinophilic protein deposits in the cytoplasm, and a reduction in dopaminergic neurons in the striatum of patients (83, 84). Lipid peroxidation, protein carbonylation, and 8-hydroxyguanosine have been found in the dopamine-producing neurons of PD patients (85). Neuroinflammation is also considered to play a crucial role in the development of PD. In PD model mice, the protective effect of the substantia nigra and striatum is mainly due to activated Treg cells (86). Pro-inflammatory cytokines such as tumor necrosis factor (TNF-α), interferon (IFN)-γ, interleukin (IL)-1β and IL-6 have been found to be elevated in the nigrostriatal region of PD patients (87). In the peripheral blood of PD patients, the levels of naive T cells and anti-inflammatory regulatory T cells are reduced, and the ratio of IFN-γ to IL-4-producing T cells is increased, creating a pro-inflammatory environment (88). α-Syn-reactive T cells have been found in PD patients, and they are most abundant in those with motor PD (89). Innate and adaptive immune changes occur in both the brain and periphery in PD, including adaptive T-lymphocyte responses (90). Additionally, a reduction in replicative senescence of CD8+ T cells in the early stages of PD has been shown to promote PD progression (91).
There is evidence that telomerase activators can have neuroprotective effects by increasing TERT expression in the brains of PD model mice and enhancing their locomotor abilities (92). Studies have shown that after CAG treatment in PD mice, there was a significant reduction of alpha-synuclein in the hippocampus and cortex, and a significant improvement in the mice’s motor function, suggesting that TERT can alleviate the symptoms of PD by inhibiting the aggregation of alpha-synuclein (74). It has been shown that the presence of estrogen receptor (ER) control elements in the promoter sequence of TERT elements, the integrity of ER is required for the regulation of hTERT, and the expression and distribution of ER are simultaneously regulated by the ratio of androgen to estrogen in the internal environment (93). TERT has a known association with estrogen, and the results of the rotating bar test after CAG treatment in PD model mice showed that motor function was significantly improved in females compared to males, which may be due to the protective effect of TERT (94). After 1‐Methyl‐4‐phenyl‐1,2,3,6‐tetrahydropyridine (MPTP) intraperitoneal injection in wild mice and TERT knockout mice, it was found that TERT-deficient mice displayed more severe symptoms, demonstrating that TERT-deficient mice are more susceptible to the neurotoxic effects of MPTP (95). Additionally, knockdown of TERT in the hippocampal dentate gyrus impaired the formation of spatial memory in mice (96). These findings suggest that the pathogenesis of PD could be improved by targeting TERT.
3.3 TERT and HD
Huntington’s disease (HD) is a debilitating neurodegenerative disorder that is characterized by the progressive decline of cognitive and motor functions, psychiatric disorders, and metabolic abnormalities (97). It typically presents in individuals between the ages of 30 and 40 years, although 5% of patients may present before the age of 21 years (97, 98). The disease is caused by the repetitive amplification of the first exon of the HTT gene, which encodes the Huntington protein. Research has shown that leukocyte telomere length is shorter in HD patients compared to healthy individuals, suggesting that telomere shortening may play a role in the pathogenesis of HD (99). The peripheral immune dysfunction in Huntington’s disease appears to be primarily mediated by the innate immune system, rather than the adaptive immune system (100). Additionally, therapies that decrease T cell-driven inflammation have been shown to delay or prevent the onset of HD (101). The results of relevant investigations have also suggested a potential association between telomere shortening and HD, which may be related to oxidative stress (102). As TERT is known to be a key component in maintaining telomere length and has antioxidant effects, it may be a potential target for alleviating HD and warrant further study.
3.4 TERT and ALS
Amyotrophic Lateral Sclerosis (ALS) is a type of motor neuron disease that affects mostly sporadic patients, with around 10% of cases being hereditary (103). The disease typically begins to develop between the ages of 40 and 60, with a high incidence rate (104). ALS is a rapid and fatal neurodegenerative disease characterized by the death and dysfunction of motor neurons in the patient’s brainstem, cerebral cortex, and spinal cord (105). Although the disease is influenced by multiple factors, it is currently incurable. Some studies have shown that immune system-related genes can play a role in ALS pathogenesis, including Treg cells that have a protective effect. For example, Foxp3, TGF-β, IL-4, and Gata3 mRNA levels were found to be reduced in patients with rapidly progressing ALS (106). In addition, research in mice expressing SOD1 suggests that deleting the gene in astrocytes or bone marrow compartments slows ALS progression (107). Moreover, peripheral immune cells have been shown to contribute to neurodegeneration (108). The study also found that the presence of clonally expanded TEMRA CD8 T cells in the peripheral blood of ALS4 patients may serve as a potential biomarker for exploring the disease’s pathogenesis (109). Furthermore, the levels of CD4+ T lymphocytes in the blood of ALS patients have been linked to evaluating cognitive impairment and age (110). TERT expression was found to be reduced in ALS patients (111) and a study showed that the AGS-499 compound improved motor neuron survival and delayed ALS progression in mice by promoting TERT expression in the brain in a dose-dependent manner (112). This suggests that TERT could be a promising target for the development of ALS therapies.
In conclusion, neurodegenerative diseases such as AD, PD, HD, and ALS can impact individuals at different life stages and pose a significant burden on affected individuals and society. Despite extensive research, current treatments for these diseases are limited and ineffective, making it crucial to better understand the underlying pathological mechanisms and causes of neurodegenerative diseases. Upon reviewing the literature, it is evident that there is a scarcity of studies that focus on the role of TERT in neurodegenerative diseases, especially in the context of immune aging. Thus, exploring the potential connection between TERT’s antioxidant effects and T cell senescence could be a promising avenue for future research and could offer new insights into the treatment of neurodegenerative diseases.
4 Conclusion and future prospects
The search for effective solutions to neurodegenerative diseases has become increasingly urgent due to the slow progress in research on these diseases. Aging is the main risk factor and the pathological changes it causes are irreversible. T cell senescence has emerged as an important area of research due to its role in aging and the recent discovery of its role in neurodegenerative diseases. TERT, which maintains telomere length and delays cellular senescence, has been shown to have neuroprotective effects (Figure 1). However, there is limited research on the relationship between T cell senescence and neurodegenerative diseases, which has led to a growing interest in exploring the potential link between TERT and T cells in this context. This review summarizes the relevant roles of TERT and the potential relationship between T cell senescence and neurodegenerative diseases, in order to provide insights into the pathogenesis of these diseases and pave the way for the development of new therapeutic agents.
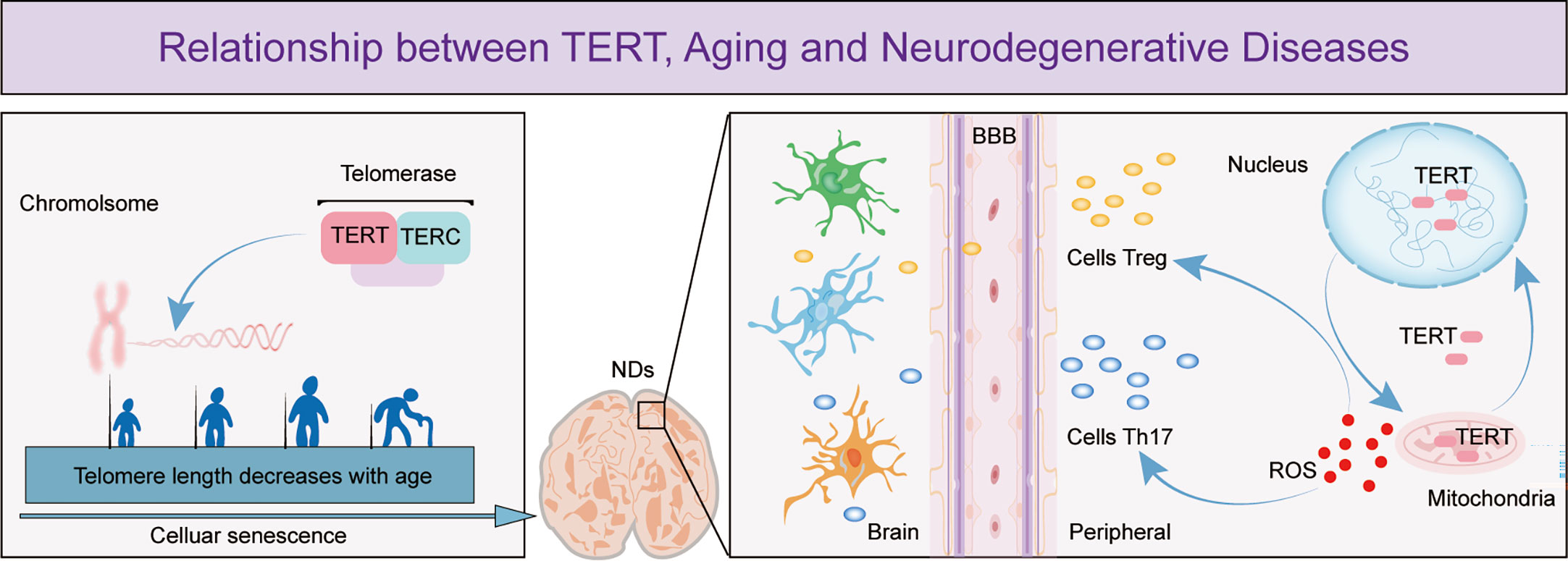
Figure 1 The figure depicts the important role of TERT in telomere maintenance and regulation of T cell effects in neurodegenerative diseases. The figure shows that as cells age, telomeres shorten in length and TERT, an essential component of telomerase, has a crucial function in preserving telomere length. Cellular senescence is a leading cause of neurodegenerative diseases and research has demonstrated that as these diseases progress, the permeability of the blood-brain barrier increases, enabling more peripheral T cells to enter the brain. TERT helps to regulate T cell expression by maintaining the optimal balance of intracellular oxidative stress, thereby exerting a neuroprotective effect.
Author contributions
XY and M-ML wrote the manuscript. T-YL and ZW contribution to the figure. Y-CZ reviewed the manuscript. XY and Z-YW edited and finalized the manuscript. All authors contributed to the article and approved the submitted version.
Funding
This work was supported by the National Natural Sciences Foundation of China (81901116, 92049106), China Postdoctoral Science Foundation (Grant No. 2019M661161) and The Science Research Foundation of Liaoning Province (NO. 2020-MS-169).
Conflict of interest
The authors declare that the research was conducted in the absence of any commercial or financial relationships that could be construed as a potential conflict of interest.
Publisher’s note
All claims expressed in this article are solely those of the authors and do not necessarily represent those of their affiliated organizations, or those of the publisher, the editors and the reviewers. Any product that may be evaluated in this article, or claim that may be made by its manufacturer, is not guaranteed or endorsed by the publisher.
References
1. Catanesi M, d'Angelo M, Tupone MG, Benedetti E, Giordano A, Castelli V, et al. MicroRNAs dysregulation and mitochondrial dysfunction in neurodegenerative diseases. Int J Mol Sci (2020) 21(17):5986. doi: 10.3390/ijms21175986
2. Singh A, Kukreti R, Saso L, Kukreti S. Oxidative stress: A key modulator in neurodegenerative diseases. Molecules. (2019) 24(8):1583. doi: 10.3390/molecules24081583
3. Yu YC, Kuo CL, Cheng WL, Liu CS, Hsieh M. Decreased antioxidant enzyme activity and increased mitochondrial DNA damage in cellular models of machado-Joseph disease. J Neurosci Res (2009) 87(8):1884–91. doi: 10.1002/jnr.22011
4. Shafi O. Inverse relationship between alzheimer's disease and cancer, and other factors contributing to alzheimer's disease: a systematic review. BMC Neurol (2016) 16(1):236. doi: 10.1186/s12883-016-0765-2
5. Bieschke J, Zhang Q, Bosco DA, Lerner RA, Powers ET, Wentworth P Jr., et al. Small molecule oxidation products trigger disease-associated protein misfolding. Acc Chem Res (2006) 39(9):611–9. doi: 10.1021/ar0500766
6. López-Otín C, Blasco MA, Partridge L, Serrano M, Kroemer G. The hallmarks of aging. Cell. (2013) 153(6):1194–217. doi: 10.1016/j.cell.2013.05.039
7. Kennedy BK, Berger SL, Brunet A, Campisi J, Cuervo AM, Epel ES, et al. Geroscience: linking aging to chronic disease. Cell. (2014) 159(4):709–13. doi: 10.1016/j.cell.2014.10.039
8. Hyman BT, Phelps CH, Beach TG, Bigio EH, Cairns NJ, Carrillo MC, et al. National institute on aging-alzheimer's association guidelines for the neuropathologic assessment of alzheimer's disease. Alzheimers Dement (2012) 8(1):1–13. doi: 10.1016/j.jalz.2011.10.007
9. Bernadotte A, Mikhelson VM, Spivak IM. Markers of cellular senescence. telomere shortening as a marker of cellular senescence. Aging (Albany NY) (2016) 8(1):3–11. doi: 10.18632/aging.100871
10. Flanary BE, Sammons NW, Nguyen C, Walker D, Streit WJ. Evidence that aging and amyloid promote microglial cell senescence. Rejuvenation Res (2007) 10(1):61–74. doi: 10.1089/rej.2006.9096
11. Jaskelioff M, Muller FL, Paik JH, Thomas E, Jiang S, Adams AC, et al. Telomerase reactivation reverses tissue degeneration in aged telomerase-deficient mice. Nature. (2011) 469(7328):102–6. doi: 10.1038/nature09603
12. Lu W, Zhang Y, Liu D, Songyang Z, Wan M. Telomeres-structure, function, and regulation. Exp Cell Res (2013) 319(2):133–41. doi: 10.1016/j.yexcr.2012.09.005
13. Moyzis RK, Buckingham JM, Cram LS, Dani M, Deaven LL, Jones MD, et al. A highly conserved repetitive DNA sequence, (TTAGGG)n, present at the telomeres of human chromosomes. Proc Natl Acad Sci U S A (1988) 85(18):6622–6. doi: 10.1073/pnas.85.18.6622
14. Dunham MA, Neumann AA, Fasching CL, Reddel RR. Telomere maintenance by recombination in human cells. Nat Genet (2000) 26(4):447–50. doi: 10.1038/82586
15. Gunes C, Avila AI, Rudolph KL. Telomeres in cancer. Differentiation. (2018) 99:41–50. doi: 10.1016/j.diff.2017.12.004
16. Ahmed S, Passos JF, Birket MJ, Beckmann T, Brings S, Peters H, et al. Telomerase does not counteract telomere shortening but protects mitochondrial function under oxidative stress. J Cell Sci (2008) 121(Pt 7):1046–53. doi: 10.1242/jcs.019372
17. Coutelier H, Xu Z, Morisse MC, Lhuillier-Akakpo M, Pelet S, Charvin G, et al. Adaptation to DNA damage checkpoint in senescent telomerase-negative cells promotes genome instability. Genes Dev (2018) 32(23-24):1499–513. doi: 10.1101/gad.318485.118
18. Bersani FS, Lindqvist D, Mellon SH, Penninx BW, Verhoeven JE, Révész D, et al. Telomerase activation as a possible mechanism of action for psychopharmacological interventions. Drug Discovery Today (2015) 20(11):1305–9. doi: 10.1016/j.drudis.2015.06.016
19. González-Giraldo Y, Forero DA, Echeverria V, Gonzalez J, Ávila-Rodriguez M, Garcia-Segura LM, et al. Neuroprotective effects of the catalytic subunit of telomerase: A potential therapeutic target in the central nervous system. Ageing Res Rev (2016) 28:37–45. doi: 10.1016/j.arr.2016.04.004
20. Tsoukalas D, Buga AM, Docea AO, Sarandi E, Mitrut R, Renieri E, et al. Reversal of brain aging by targeting telomerase: A nutraceutical approach. Int J Mol Med (2021) 48(5):199. doi: 10.3892/ijmm.2021.5032
21. Molgora B, Bateman R, Sweeney G, Finger D, Dimler T, Effros RB, et al. Functional assessment of pharmacological telomerase activators in human T cells. Cells. (2013) 2(1):57–66. doi: 10.3390/cells2010057
22. Fernandez ML, Thomas MS, Lemos BS, DiMarco DM, Missimer A, Melough M, et al. TA-65, a telomerase activator improves cardiovascular markers in patients with metabolic syndrome. Curr Pharm Des (2018) 24(17):1905–11. doi: 10.2174/1381612824666180316114832
23. Ip FC, Ng YP, An HJ, Dai Y, Pang HH, Hu YQ, et al. Cycloastragenol is a potent telomerase activator in neuronal cells: implications for depression management. Neurosignals. (2014) 22(1):52–63. doi: 10.1159/000365290
24. Le Saux CJ, Davy P, Brampton C, Ahuja SS, Fauce S, Shivshankar P, et al. A novel telomerase activator suppresses lung damage in a murine model of idiopathic pulmonary fibrosis. PloS One (2013) 8(3):e58423. doi: 10.1371/journal.pone.0058423
25. Klapper W, Shin T, Mattson MP. Differential regulation of telomerase activity and TERT expression during brain development in mice. J Neurosci Res (2001) 64(3):252–60. doi: 10.1002/jnr.1073
26. Whittemore K, Derevyanko A, Martinez P, Serrano R, Pumarola M, Bosch F, et al. Telomerase gene therapy ameliorates the effects of neurodegeneration associated to short telomeres in mice. Aging (Albany NY) (2019) 11(10):2916–48. doi: 10.18632/aging.101982
27. Zheng Q, Huang J, Wang G. Mitochondria, telomeres and telomerase subunits. Front Cell Dev Biol (2019) 7:274. doi: 10.3389/fcell.2019.00274
28. Muzza M, Colombo C, Cirello V, Perrino M, Vicentini L, Fugazzola L. Oxidative stress and the subcellular localization of the telomerase reverse transcriptase (TERT) in papillary thyroid cancer. Mol Cell Endocrinol (2016) 431:54–61. doi: 10.1016/j.mce.2016.05.005
29. Zhou Q, Lin L, Li H, Wang H, Jiang S, Huang P, et al. Melatonin reduces neuroinflammation and improves axonal hypomyelination by modulating M1/M2 microglia polarization via JAK2-STAT3-Telomerase pathway in postnatal rats exposed to lipopolysaccharide. Mol Neurobiol (2021) 58(12):6552–76. doi: 10.1007/s12035-021-02568-7
30. Boccardi V, Arosio B, Cari L, Bastiani P, Scamosci M, Casati M, et al. Beta-carotene, telomerase activity and alzheimer's disease in old age subjects. Eur J Nutr (2020) 59(1):119–26. doi: 10.1007/s00394-019-01892-y
31. Ma PK, Wei BH, Cao YL, Miao Q, Chen N, Guo CE, et al. Pharmacokinetics, metabolism, and excretion of cycloastragenol, a potent telomerase activator in rats. Xenobiotica. (2017) 47(6):526–37. doi: 10.1080/00498254.2016.1204568
32. Rivas-Arancibia S, Hernández-Orozco E, Rodríguez-Martínez E, Valdés-Fuentes M, Cornejo-Trejo V, Pérez-Pacheco N, et al. Ozone pollution, oxidative stress, regulatory T cells and antioxidants. Antioxidants (Basel) (2022) 11(8). doi: 10.3390/antiox11081553
33. Velázquez-Pérez R, Rodríguez-Martínez E, Valdés-Fuentes M, Gelista-Herrera N, Gómez-Crisóstomo N, Rivas-Arancibia S. Oxidative stress caused by ozone exposure induces changes in P2X7 receptors, neuroinflammation, and neurodegeneration in the rat hippocampus. Oxid Med Cell Longev (2021) 2021:3790477. doi: 10.1155/2021/3790477
34. Town T, Tan J, Flavell RA, Mullan M. T-Cells in alzheimer's disease. Neuromolecular Med (2005) 7(3):255–64. doi: 10.1385/NMM:7:3:255
35. Giuliani F, Goodyer CG, Antel JP, Yong VW. Vulnerability of human neurons to T cell-mediated cytotoxicity. J Immunol (2003) 171(1):368–79. doi: 10.4049/jimmunol.171.1.368
36. Derecki NC, Cardani AN, Yang CH, Quinnies KM, Crihfield A, Lynch KR, et al. Regulation of learning and memory by meningeal immunity: a key role for IL-4. J Exp Med (2010) 207(5):1067–80. doi: 10.1084/jem.20091419
37. Akbar AN, Henson SM, Lanna A. Senescence of T lymphocytes: Implications for enhancing human immunity. Trends Immunol (2016) 37(12):866–76. doi: 10.1016/j.it.2016.09.002
38. Burzyn D, Kuswanto W, Kolodin D, Shadrach JL, Cerletti M, Jang Y, et al. A special population of regulatory T cells potentiates muscle repair. Cell. (2013) 155(6):1282–95. doi: 10.1016/j.cell.2013.10.054
39. Dosset M, Castro A, Carter H, Zanetti M. Telomerase and CD4 T cell immunity in cancer. Cancers (Basel) (2020) 12(6):1687. doi: 10.3390/cancers12061687
40. Mao J, Zhang Q, Wang Y, Zhuang Y, Xu L, Ma X, et al. TERT activates endogenous retroviruses to promote an immunosuppressive tumour microenvironment. EMBO Rep (2022) 23(4):e52984. doi: 10.15252/embr.202152984
41. Barsov EV. Telomerase and primary T cells: biology and immortalization for adoptive immunotherapy. Immunotherapy. (2011) 3(3):407–21. doi: 10.2217/imt.10.107
42. Weng NP, Levine BL, June CH, Hodes RJ. Regulated expression of telomerase activity in human T lymphocyte development and activation. J Exp Med (1996) 183(6):2471–9. doi: 10.1084/jem.183.6.2471
43. Ghosh A, Saginc G, Leow SC, Khattar E, Shin EM, Yan TD, et al. Telomerase directly regulates NF-κB-dependent transcription. Nat Cell Biol (2012) 14(12):1270–81. doi: 10.1038/ncb2621
44. Rentoukas E, Tsarouhas K, Kaplanis I, Korou E, Nikolaou M, Marathonitis G, et al. Connection between telomerase activity in PBMC and markers of inflammation and endothelial dysfunction in patients with metabolic syndrome. PloS One (2012) 7(4):e35739. doi: 10.1371/journal.pone.0035739
45. Ogoshi M, Takashima A, Taylor RS. Mechanisms regulating telomerase activity in murine T cells. J Immunol (1997) 158(2):622–8. doi: 10.4049/jimmunol.158.2.622
46. Luiten RM, Péne J, Yssel H, Spits H. Ectopic hTERT expression extends the life span of human CD4+ helper and regulatory T-cell clones and confers resistance to oxidative stress-induced apoptosis. Blood. (2003) 101(11):4512–9. doi: 10.1182/blood-2002-07-2018
47. Röth A, Baerlocher GM, Schertzer M, Chavez E, Dührsen U, Lansdorp PM. Telomere loss, senescence, and genetic instability in CD4+ T lymphocytes overexpressing hTERT. Blood. (2005) 106(1):43–50. doi: 10.1182/blood-2004-10-4144
48. Indran IR, Hande MP, Pervaiz S. hTERT overexpression alleviates intracellular ROS production, improves mitochondrial function, and inhibits ROS-mediated apoptosis in cancer cells. Cancer Res (2011) 71(1):266–76. doi: 10.1158/0008-5472.CAN-10-1588
49. Ait-Aissa K, Kadlec AO, Hockenberry J, Gutterman DD, Beyer AM. Telomerase reverse transcriptase protects against angiotensin II-induced microvascular endothelial dysfunction. Am J Physiol Heart Circ Physiol (2018) 314(5):H1053–h1060. doi: 10.1152/ajpheart.00472.2017
50. Bojesen SE. Telomeres and human health. J Intern Med (2013) 274(5):399–413. doi: 10.1111/joim.12083
51. Yousefzadeh MJ, Flores RR, Zhu Y, Schmiechen ZC, Brooks RW, Trussoni CE, et al. An aged immune system drives senescence and ageing of solid organs. Nature. (2021) 594(7861):100–5. doi: 10.1038/s41586-021-03547-7
52. Weller J, Budson A. Current understanding of alzheimer's disease diagnosis and treatment. F1000Res (2018) 7:F1000 Faculty Rec-1161. doi: 10.12688/f1000research.14506.1
53. McHugh D, Gil J. Senescence and aging: Causes, consequences, and therapeutic avenues. J Cell Biol (2018) 217(1):65–77. doi: 10.1083/jcb.201708092
54. Gomersall C. A rapid response intermediate care service for older people with mental health problems. Nurs Times (2009) 105(17):12–3.
55. Alzheimer's Association. 2016 alzheimer's disease facts and figures. Alzheimers Dement (2016) 12(4):459–509. doi: 10.1016/j.jalz.2016.03.001
56. Brookmeyer R, Johnson E, Ziegler-Graham K, Arrighi HM. Forecasting the global burden of alzheimer's disease. Alzheimers Dement (2007) 3(3):186–91. doi: 10.1016/j.jalz.2007.04.381
57. Mucke L, Selkoe DJ. Neurotoxicity of amyloid β-protein: synaptic and network dysfunction. Cold Spring Harb Perspect Med (2012) 2(7):a006338. doi: 10.1101/cshperspect.a006338
58. Jouanne M, Rault S, Voisin-Chiret AS. Tau protein aggregation in alzheimer's disease: An attractive target for the development of novel therapeutic agents. Eur J Med Chem (2017) 139:153–67. doi: 10.1016/j.ejmech.2017.07.070
59. Polydoro M, de Calignon A, Suárez-Calvet M, Sanchez L, Kay KR, Nicholls SB, et al. Reversal of neurofibrillary tangles and tau-associated phenotype in the rTgTauEC model of early alzheimer's disease. J Neurosci (2013) 33(33):13300–11. doi: 10.1523/JNEUROSCI.0881-13.2013
60. Rey F, Ottolenghi S, Zuccotti GV, Samaja M, Carelli S. Mitochondrial dysfunctions in neurodegenerative diseases: role in disease pathogenesis, strategies for analysis and therapeutic prospects. Neural Regener Res (2022) 17(4):754–8. doi: 10.4103/1673-5374.322430
61. Lazarevic-Pasti T, Leskovac A, Momic T, Petrovic S, Vasic V. Modulators of acetylcholinesterase activity: From alzheimer's disease to anti-cancer drugs. Curr Med Chem (2017) 24(30):3283–309. doi: 10.2174/0929867324666170705123509
62. Alberdi E, Sánchez-Gómez MV, Ruiz A, Cavaliere F, Ortiz-Sanz C, Quintela-López T, et al. Mangiferin and morin attenuate oxidative stress, mitochondrial dysfunction, and neurocytotoxicity, induced by amyloid beta oligomers. Oxid Med Cell Longev (2018) 2018:2856063. doi: 10.1155/2018/2856063
63. Chen Z, Zhong C. Oxidative stress in alzheimer's disease. Neurosci Bull (2014) 30(2):271–81. doi: 10.1007/s12264-013-1423-y
64. St-Amour I, Bosoi CR, Paré I, Ignatius Arokia Doss PM, Rangachari M, Hébert SS, et al. Peripheral adaptive immunity of the triple transgenic mouse model of alzheimer's disease. J Neuroinflammation (2019) 16(1):3. doi: 10.1186/s12974-018-1380-5
65. Carrano A, Hoozemans JJ, van der Vies SM, Rozemuller AJ, van Horssen J, de Vries HE. Amyloid beta induces oxidative stress-mediated blood-brain barrier changes in capillary amyloid angiopathy. Antioxid Redox Signal (2011) 15(5):1167–78. doi: 10.1089/ars.2011.3895
66. Goldeck D, Larbi A, Pellicanó M, Alam I, Zerr I, Schmidt C, et al. Enhanced chemokine receptor expression on leukocytes of patients with alzheimer's disease. PloS One (2013) 8(6):e66664. doi: 10.1371/journal.pone.0066664
67. Fu J, Duan J, Mo J, Xiao H, Huang Y, Chen W, et al. Mild cognitive impairment patients have higher regulatory T-cell proportions compared with alzheimer's disease-related dementia patients. Front Aging Neurosci (2020) 12:624304. doi: 10.3389/fnagi.2020.624304
68. Williams JL, Manivasagam S, Smith BC, Sim J, Vollmer LL, Daniels BP, et al. Astrocyte-T cell crosstalk regulates region-specific neuroinflammation. Glia. (2020) 68(7):1361–74. doi: 10.1002/glia.23783
69. Gate D, Saligrama N, Leventhal O, Yang AC, Unger MS, Middeldorp J, et al. Clonally expanded CD8 T cells patrol the cerebrospinal fluid in alzheimer's disease. Nature. (2020) 577(7790):399–404. doi: 10.1038/s41586-019-1895-7
70. Mietelska-Porowska A, Wojda U. T Lymphocytes and inflammatory mediators in the interplay between brain and blood in alzheimer's disease: Potential pools of new biomarkers. J Immunol Res (2017) 2017:4626540. doi: 10.1155/2017/4626540
71. Mather KA, Jorm AF, Parslow RA, Christensen H. Is telomere length a biomarker of aging? a review. J Gerontol A Biol Sci Med Sci (2011) 66(2):202–13. doi: 10.1093/gerona/glq180
72. Boccardi V, Pelini L, Ercolani S, Ruggiero C, Mecocci P. From cellular senescence to alzheimer's disease: The role of telomere shortening. Ageing Res Rev (2015) 22:1–8. doi: 10.1016/j.arr.2015.04.003
73. Iannilli F, Zalfa F, Gartner A, Bagni C, Dotti CG. Cytoplasmic TERT associates to RNA granules in fully mature neurons: Role in the translational control of the cell cycle inhibitor p15INK4B. PloS One (2013) 8(6):e66602. doi: 10.1371/journal.pone.0066602
74. Wan T, Weir EJ, Johnson M, Korolchuk VI, Saretzki GC. Increased telomerase improves motor function and alpha-synuclein pathology in a transgenic mouse model of parkinson's disease associated with enhanced autophagy. Prog Neurobiol (2021) 199:101953. doi: 10.1016/j.pneurobio.2020.101953
75. Bernardes de Jesus B, Vera E, Schneeberger K, Tejera AM, Ayuso E, Bosch F, et al. Telomerase gene therapy in adult and old mice delays aging and increases longevity without increasing cancer. EMBO Mol Med (2012) 4(8):691–704. doi: 10.1002/emmm.201200245
76. Baruch-Eliyahu N, Rud V, Braiman A, Priel E. Telomerase increasing compound protects hippocampal neurons from amyloid beta toxicity by enhancing the expression of neurotrophins and plasticity related genes. Sci Rep (2019) 9(1):18118. doi: 10.1038/s41598-019-54741-7
77. Spilsbury A, Miwa S, Attems J, Saretzki G. The role of telomerase protein TERT in alzheimer's disease and in tau-related pathology in vitro. J Neurosci (2015) 35(4):1659–74. doi: 10.1523/JNEUROSCI.2925-14.2015
78. Lukens JN, Van Deerlin V, Clark CM, Xie SX, Johnson FB. Comparisons of telomere lengths in peripheral blood and cerebellum in alzheimer's disease. Alzheimers Dement (2009) 5(6):463–9. doi: 10.1016/j.jalz.2009.05.666
79. Zhu H, Fu W, Mattson MP. The catalytic subunit of telomerase protects neurons against amyloid beta-peptide-induced apoptosis. J Neurochem (2000) 75(1):117–24. doi: 10.1046/j.1471-4159.2000.0750117.x
80. Silva RC, Domingues HS, Salgado AJ, Teixeira FG. From regenerative strategies to pharmacological approaches: can we fine-tune treatment for parkinson's disease? Neural Regener Res (2022) 17(5):933–6. doi: 10.4103/1673-5374.324827
81. Kowal SL, Dall TM, Chakrabarti R, Storm MV, Jain A. The current and projected economic burden of parkinson's disease in the united states. Mov Disord (2013) 28(3):311–8. doi: 10.1002/mds.25292
82. Fearnley JM, Lees AJ. Ageing and parkinson's disease: substantia nigra regional selectivity. Brain. (1991) 114(Pt 5):2283–301. doi: 10.1093/brain/114.5.2283
83. Svenningsson P, Westman E, Ballard C, Aarsland D. Cognitive impairment in patients with parkinson's disease: diagnosis, biomarkers, and treatment. Lancet Neurol (2012) 11(8):697–707. doi: 10.1016/S1474-4422(12)70152-7
84. Schneider SA, Obeso JA. Clinical and pathological features of parkinson's disease. Curr Top Behav Neurosci (2015) 22:205–20. doi: 10.1007/7854_2014_317
85. Filograna R, Beltramini M, Bubacco L, Bisaglia M. Anti-oxidants in parkinson's disease therapy: A critical point of view. Curr Neuropharmacol (2016) 14(3):260–71. doi: 10.2174/1570159X13666151030102718
86. Reynolds AD, Banerjee R, Liu J, Gendelman HE, Mosley RL. Neuroprotective activities of CD4+CD25+ regulatory T cells in an animal model of parkinson's disease. J Leukoc Biol (2007) 82(5):1083–94. doi: 10.1189/jlb.0507296
87. Mogi M, Harada M, Kondo T, Riederer P, Inagaki H, Minami M, et al. Interleukin-1 beta, interleukin-6, epidermal growth factor and transforming growth factor-alpha are elevated in the brain from parkinsonian patients. Neurosci Lett (1994) 180(2):147–50. doi: 10.1016/0304-3940(94)90508-8
88. Karikari AA, McFleder RL, Ribechini E, Blum R, Bruttel V, Knorr S, et al. Neurodegeneration by α-synuclein-specific T cells in AAV-A53T-α-synuclein parkinson's disease mice. Brain Behav Immun (2022) 101:194–210. doi: 10.1016/j.bbi.2022.01.007
89. Lindestam Arlehamn CS, Dhanwani R, Pham J, Kuan R, Frazier A, Rezende Dutra J, et al. α-synuclein-specific T cell reactivity is associated with preclinical and early parkinson's disease. Nat Commun (2020) 11(1):1875. doi: 10.4049/jimmunol.204.Supp.64.8
90. Mosley RL, Hutter-Saunders JA, Stone DK, Gendelman HE. Inflammation and adaptive immunity in parkinson's disease. Cold Spring Harb Perspect Med (2012) 2(1):a009381. doi: 10.1101/cshperspect.a009381
91. Kouli A, Jensen M, Papastavrou V, Scott KM, Kolenda C, Parker C, et al. T Lymphocyte senescence is attenuated in parkinson's disease. J Neuroinflammation (2021) 18(1):228. doi: 10.1186/s12974-021-02287-9
92. Saretzki G, Wan T. Telomerase in brain: The new kid on the block and its role in neurodegenerative diseases. Biomedicines. (2021) 9(5):490. doi: 10.3390/biomedicines9050490
93. Grasselli A, Nanni S, Colussi C, Aiello A, Benvenuti V, Ragone G, et al. Estrogen receptor-alpha and endothelial nitric oxide synthase nuclear complex regulates transcription of human telomerase. Circ Res (2008) 103(1):34–42. doi: 10.1161/CIRCRESAHA.107.169037
94. Vera E, Bosco N, Studer L. Generating late-onset human iPSC-based disease models by inducing neuronal age-related phenotypes through telomerase manipulation. Cell Rep (2016) 17(4):1184–92. doi: 10.1016/j.celrep.2016.09.062
95. Jackson-Lewis V, Przedborski S. Protocol for the MPTP mouse model of parkinson's disease. Nat Protoc (2007) 2(1):141–51. doi: 10.1038/nprot.2006.342
96. Zhou QG, Liu MY, Lee HW, Ishikawa F, Devkota S, Shen XR, et al. Hippocampal TERT regulates spatial memory formation through modulation of neural development. Stem Cell Rep (2017) 9(2):543–56. doi: 10.1016/j.stemcr.2017.06.014
97. Panes JD, Wendt A, Ramirez-Molina O, Castro PA, Fuentealba J. Deciphering the role of PGC-1α in neurological disorders: from mitochondrial dysfunction to synaptic failure. Neural Regener Res (2022) 17(2):237–45. doi: 10.4103/1673-5374.317957
98. Bakels HS, Roos RAC, van Roon-Mom WMC, de Bot ST. Juvenile-onset huntington disease pathophysiology and neurodevelopment: A review. Mov Disord (2022) 37(1):16–24. doi: 10.1002/mds.28823
99. Scarabino D, Veneziano L, Peconi M, Frontali M, Mantuano E, Corbo RM. Leukocyte telomere shortening in huntington's disease. J Neurol Sci (2019) 396:25–9. doi: 10.1016/j.jns.2018.10.024
100. Miller JR, Träger U, Andre R, Tabrizi SJ. Mutant huntingtin does not affect the intrinsic phenotype of human huntington's disease T lymphocytes. PloS One (2015) 10(11):e0141793. doi: 10.1371/journal.pone.0141793
101. Lemprière S. T Helper cells implicated in early huntington disease. Nat Rev Neurol (2020) 16(1):4. doi: 10.1038/s41582-019-0297-7
102. PerezGrovas-Saltijeral A, Ochoa-Morales A, Miranda-Duarte A, Martínez-Ruano L, Jara-Prado A, Camacho-Molina A, et al. Telomere length analysis on leukocytes derived from patients with huntington disease. Mech Ageing Dev (2020) 185:111189. doi: 10.1016/j.mad.2019.111189
103. Boillée S, Vande Velde C, Cleveland DW. ALS: a disease of motor neurons and their nonneuronal neighbors. Neuron. (2006) 52(1):39–59. doi: 10.1016/j.neuron.2006.09.018
104. Wei QQ, Hou YB, Zhang LY, Ou RW, Cao B, Chen YP, et al. Neutrophil-to-lymphocyte ratio in sporadic amyotrophic lateral sclerosis. Neural Regener Res (2022) 17(4):875–80. doi: 10.4103/1673-5374.322476
105. Nayak MS, Kim YS, Goldman M, Keirstead HS, Kerr DA. Cellular therapies in motor neuron diseases. Biochim Biophys Acta (2006) 1762(11-12):1128–38. doi: 10.1016/j.bbadis.2006.06.004
106. Henkel JS, Beers DR, Wen S, Rivera AL, Toennis KM, Appel JE, et al. Regulatory T-lymphocytes mediate amyotrophic lateral sclerosis progression and survival. EMBO Mol Med (2013) 5(1):64–79. doi: 10.1002/emmm.201201544
107. Yamanaka K, Chun SJ, Boillee S, Fujimori-Tonou N, Yamashita H, Gutmann DH, et al. Astrocytes as determinants of disease progression in inherited amyotrophic lateral sclerosis. Nat Neurosci (2008) 11(3):251–3. doi: 10.1038/nn2047
108. Butovsky O, Siddiqui S, Gabriely G, Lanser AJ, Dake B, Murugaiyan G, et al. Modulating inflammatory monocytes with a unique microRNA gene signature ameliorates murine ALS. J Clin Invest (2012) 122(9):3063–87. doi: 10.1172/JCI62636
109. Campisi L, Chizari S, Ho JSY, Gromova A, Arnold FJ, Mosca L, et al. Clonally expanded CD8 T cells characterize amyotrophic lateral sclerosis-4. Nature. (2022) 606(7916):945–52. doi: 10.1038/s41586-022-04844-5
110. Yang Y, Pan D, Gong Z, Tang J, Li Z, Ding F, et al. Decreased blood CD4+ T lymphocyte helps predict cognitive impairment in patients with amyotrophic lateral sclerosis. BMC Neurol (2021) 21(1):157. doi: 10.1186/s12883-021-02185-w
111. De Felice B, Annunziata A, Fiorentino G, Manfellotto F, D'Alessandro R, Marino R, et al. Telomerase expression in amyotrophic lateral sclerosis (ALS) patients. J Hum Genet (2014) 59(10):555–61. doi: 10.1038/jhg.2014.72
Keywords: telomerase reverse transcriptase, aging, neurodegenerative diseases, T cell, nervous system
Citation: Yu X, Liu MM, Zheng CY, Liu YT, Wang Z and Wang ZY (2023) Telomerase reverse transcriptase and neurodegenerative diseases. Front. Immunol. 14:1165632. doi: 10.3389/fimmu.2023.1165632
Received: 14 February 2023; Accepted: 15 March 2023;
Published: 29 March 2023.
Edited by:
Mingzhu Zheng, Southeast University, ChinaCopyright © 2023 Yu, Liu, Zheng, Liu, Wang and Wang. This is an open-access article distributed under the terms of the Creative Commons Attribution License (CC BY). The use, distribution or reproduction in other forums is permitted, provided the original author(s) and the copyright owner(s) are credited and that the original publication in this journal is cited, in accordance with accepted academic practice. No use, distribution or reproduction is permitted which does not comply with these terms.
*Correspondence: Zhan-You Wang, d2FuZ3p5QGNtdS5lZHUuY24=
†These authors have contributed equally to this work