- 1Laboratorio Institucional de Investigación, Facultad de Ciencias de la Naturaleza, Universidad San Sebastián, Puerto Montt, Chile
- 2Laboratorio de Inmunología y Estrés de Organismos Acuáticos, Facultad de Ciencias Veterinarias, Universidad Austral de Chile, Valdivia, Chile
- 3Escuela de Tecnología Médica, Facultad de la Salud, Universidad Santo Tomás, Osorno, Chile
- 4Centro de Investigaciones Biológicas Aplicadas (CIBA), Puerto Montt, Chile
- 5Centro de Investigación y Desarrollo i~mar, Universidad de los Lagos, Puerto Montt, Chile
- 6Instituto de Ciencias Marinas y Limnológicas, Facultad de Ciencias, Universidad Austral de Chile, Valdivia, Chile
- 7Centro Fondap de Investigación de Altas Latitudes (IDEAL), Universidad Austral de Chile, Valdivia, Chile
- 8Millennium Institute Biodiversity of Antarctic and Subantarctic Ecosystems, Biodiversity of Antarctic and Subantarctic Ecosystems (BASE), University Austral of Chile, Valdivia, Chile
- 9Centro Fondap Interdisciplinary Center for Aquaculture Research (INCAR), Universidad de Concepción, Concepción, Chile
Nutritional immunity regulates the homeostasis of micronutrients such as iron, manganese, and zinc at the systemic and cellular levels, preventing the invading microorganisms from gaining access and thereby limiting their growth. Therefore, the objective of this study was to evaluate the activation of nutritional immunity in specimens of Atlantic salmon (Salmo salar) that are intraperitoneally stimulated with both live and inactivated Piscirickettsia salmonis. The study used liver tissue and blood/plasma samples on days 3, 7, and 14 post-injections (dpi) for the analysis. Genetic material (DNA) of P. salmonis was detected in the liver tissue of fish stimulated with both live and inactivated P. salmonis at 14 dpi. Additionally, the hematocrit percentage decreased at 3 and 7 dpi in fish stimulated with live P. salmonis, unchanged in fish challenged with inactivated P. salmonis. On the other hand, plasma iron content decreased during the experimental course in fish stimulated with both live and inactivated P. salmonis, although this decrease was statistically significant only at 3 dpi. Regarding the immune-nutritional markers such as tfr1, dmt1, and ireg1 were modulated in the two experimental conditions, compared to zip8, ft-h, and hamp, which were down-regulated in fish stimulated with live and inactivated P. salmonis during the course experimental. Finally, the intracellular iron content in the liver increased at 7 and 14 dpi in fish stimulated with live and inactivated P. salmonis, while the zinc content decreased at 14 dpi under both experimental conditions. However, stimulation with live and inactivated P. salmonis did not alter the manganese content in the fish. The results suggest that nutritional immunity does not distinguish between live and inactivated P. salmonis and elicits a similar immune response. Probably, this immune mechanism would be self-activated with the detection of PAMPs, instead of a sequestration and/or competition of micronutrients by the living microorganism.
Introduction
Aquaculture in Chile focuses on the industrial-scale production of salmonids fish, mainly Atlantic salmon (Salmo salar). However, the aquaculture companies suffer significant economic losses due to the high prevalence of infectious diseases, of which, Piscirickettsiosis caused by the bacterium Piscirickettsia salmonis is the most important disease (1, 2). Despite the fact that the identification of this bacterium dates back more than 30 years (3), the antimicrobial treatments so far were not effective in eliminating or in the least regulating the disease. It is estimated that this might be due to the ability of the bacterium to modulate the immune system of Atlantic salmon (Salmo salar) for the benefit of its permanence and replication (4). Similarly, the host-pathogen interaction modulates the regulation of micronutrients such as iron, as reported by Pulgar et al. (5), who demonstrated that families of Atlantic salmon (Salmo salar) that are resistant to infection with P. salmonis decrease the content of this micronutrient in the head kidney, compared to families with high susceptibility, suggesting that the regulation of iron-associated nutritional immunity could have a key role in fish survival.
Nutritional immunity involves the regulation of the availability of micronutrients such as iron, manganese, and zinc at systemic and cellular levels so that pathogenic microorganisms cannot access them (6). The activation of this mechanism has not only been evaluated in Atlantic salmon (Salmo salar) (5), and this mechanism has previously been described in Patagonian blennie (Eleginops maclovinus) challenged with P. salmonis (7, 8), as well as in Antarctic fish “Notothenia rossii – Notothenia coriiceps” stimulated with LPS (9) and, in vitro in Atlantic salmon head kidney cells (SHK-1) stimulated with several Pathogen-Associated Molecular Patterns (PAMPs) from P. salmonis (10). From a genomic point of view, orthologous genes involved in metabolism and iron uptake in P. salmonis have been identified as markers involved in the classical components of the tonB system, tonB-dependent siderophore synthesis/receptor, membrane exporters, ABC transporter, ferrous ion transport, tonB-dependent heme/siderophore receptor, and Fur family transcriptional regulator (5, 11, 12). In addition, it has been functionally demonstrated that P. salmonis can be cultured in artificial media supplemented with both ferric ammonium citrate and ferric nitrate inducing the synthesis of siderophores under experimental conditions (13).
The nutritional immunity also regulates other critical micronutrients such as manganese and zinc (6, 14). However, the uptake systems of these micronutrients by P. salmonis and how Atlantic salmon (Salmo salar) could regulate its homeostasis under infection conditions have not yet been investigated, although Pulgar et al. (5) reported no differences in the zinc content in the head kidney of Atlantic salmon (Salmo salar) between the families resistant and susceptible to P. salmonis were found after 14 dpi. Despite that micronutrients such as iron, manganese, and zinc can be captured from the water by fish (gills), the most important route of acquisition is from the diet (gastrointestinal tract) (15, 16). Thus, once assimilated they can be used in numerous biological functions within the cells (17, 18). Therefore, it is not surprising that their deficiencies or imbalances have repercussions on the immune response (19, 20) and much less that they are also considered micronutrients. that other bacteria require to induce their pathogenesis mechanisms (21–33).
Studies reveal that microorganisms have evolved sophisticated strategies to capture these micronutrients, and thus the proteins involved in their homeostasis in the host tissues must be correctly regulated. So far, iron-associated nutritional immunity has been evaluated in S. salar challenged with live P. salmonis (5) and in the SHK-1 cell line stimulated with outer membrane vesicles (OMVs), lipopolysaccharides (LPS) and total proteins (TP) of P. salmonis (10). Yet it is still unknown whether this antimicrobial defense mechanism discriminates between: live pathogens that require micronutrients and dead pathogens (inactivated bacteria) that do not. Therefore, the current research aimed to evaluate the activation of nutritional immunity through an in vivo approach using Atlantic salmon (Salmo salar) challenged with live and inactivated P. salmonis at different times post-injection (dpi).
Material and methods
Piscirickettsia salmonis
P. salmonis strain type LF-89 (ATCC VR-1361) was grown under standard conditions in Austral-SRS broth medium for 5 days at 18°C and 50 rpm of agitation (34). The identity was confirmed by Gram staining, polymerase chain reaction (PCR), and immunofluorescence antibody test (IFAT) (IFAT, SRS-Bios Chile), following the instruction manual. Subsequently, the inoculum used for the salmon treatment with inactivated P. salmonis was obtained by applying the previously described methodology for bacterial inactivation by thermal shock at 100°C for 30 min (35). The inactivation of the culture was confirmed by cultivating 100 μL of the inactivated inoculum in Austral-SRS broth solid medium without colony formation.
Experimental challenge
Post-smolt specimens of Atlantic salmon (Salmo salar) (700 g) were obtained from local fish cultures (Valdivia, Chile) and transferred to Salmon Clinical Trials Facility, Institute of Animal Pathology, Faculty of Veterinary Medicine, Universidad Austral de Chile. The fish were kept in 1000 L ponds at 16°C, 12:12 photoperiod, 33 PSU salinity, 90-100% oxygen saturation, and fed once a day with Biomar pellets at 1% of their body weight (Proximate food analysis was 45-50% crude protein, 21-23% lipids, 9.5% carbohydrates, 12% ashes, 10% water, and 2.5% fiber). After the acclimation period, the fish (n=54) were randomly distributed into six 1000 L ponds for the implementation of the experimental treatments by intraperitoneal injection (i.p) and in duplicate: control (i.p fish with 100 μL of bacterial culture medium), live Ps (i.p fish with 100 μL of live P. salmonis at 1x104 bact/μL) and inactivated Ps (i.p fish with 100 μL of inactivated P. salmonis at 1x104 bact/μL). Three fish were randomly extracted from each pond (six for each treatment) at 3, 7, and 14 days post-injections (dpi) for blood/plasma and liver extraction. The bacterial dose applied was the same one used in a previous trial (5) and the experimental conditions in terms of temperature, salinity, oxygen, photoperiod and feeding were the same as those detailed in the acclimation period.
Total DNA extraction
Total DNA extraction was from 60 mg of sample pool (liver) per experimental condition at 14 dpi, using a commercial Bacterial DNA isolation kit (Matchery-Nagel) and following the manufacturer’s instructions. Subsequently, the total DNA pellet was dissolved in diethylpyrocarbonate water, quantified by spectrophotometry (NanoDrop Technologies), and used for the detection of P. salmonis by qPCR with specific primers described in the literature (36). The last day (14 dpi) was selected to increase the sensitivity of the qPCR due to the bacterial load administered and the experimental times evaluated.
Total RNA extraction
Total RNA was extracted from 50 mg of each tissue sample (liver) using the commercial kit E.Z.N.A ® Total RNA Kit I (Omega) and Rnase-free Dnase I, following the manufacturer’s guidelines. Then the total RNA pellet was dissolved in diethylpyrocarbonate water, quantified by spectrophotometry (NanoDrop Technologies), and its quality was evaluated using 1% agarose gel. Subsequently, 1 μg of total RNA was used as a template for cDNA synthesis, using MMLV-RT reverse transcriptase (Promega) and oligo-dT primer (Invitrogen), according to the standard procedure (10).
qPCR analysis
For the qPCR analysis, the QuantStudio™ equipment, Master Mix SYBRGreen (Life Technology, Thermo Scientific), and cDNA at 100 ng were used. Reactions were performed in triplicates in a total volume of 12 μL (6 μL SYBRGreen, 0.5 μL forward primers, 0.5 μL reverse primers, 3 μL PCR-grade water and 2 μL cDNA). Primers were designed for: transferrin receptor 1 (tfr1), divalent metal transporter 1 (dmt1), ferroportin 1 (ireg1), hepcidin (hamp), ferritin heavy-chain (ft-h), zinc transporter 8 (zip8), and small ribosomal subunit (18s) (10). The qPCR cycle conditions were 95°C for 10 min followed by 40 cycles of 95°C for 10 s and 60°C for 1 min. At the end of each reaction, the melting curve was evaluated to confirm the amplification and detection of a single PCR product, and the respective expression levels were analyzed using the comparative Ct method (2-ΔΔCT) (37). The data are presented as fold changes in gene expression normalized to an endogenous reference gene (18s) and relative to the uninfected fish (Control). The specific primers are listed in Table 1.
Plasma iron
Plasma iron levels were analyzed following the procedure indicated by Quilapi et al. (39), using the photometric technique of the Fer-color AA reaction kit (Wiener lab.) and the automatic biochemical analyzer (Erba XL-100). Briefly, 200 µL of plasma were mixed with 1 mL of reagent A (200 mM citric acid solution, 34 mM ascorbic acid, 100 mM thiourea and surfactant) and then, the absorbance was read by spectrophotometry at 600 nm. Subsequently, 200 µL of reagent B (ferene stabilized solution > 3 mM) were added to the previous mix and the absorbance was read after 5 minutes at 600 nm. The obtained absorbance is directly proportional to the iron concentration in the sample.
Micronutrients in liver
The liver samples (1 g) were freeze-dried for 24 h in the Sumtrom model CT-FOL-12P freeze-dryer, belonging to the Center for Applied Biological Research (CIBA). Subsequently, they were sent to the Service Laboratory, Solespectro Ltda. (Santiago, Chile) for the quantification of micronutrients such as iron, manganese, and zinc using flame atomic absorption spectroscopy (FAAS). The concentration of micronutrients was expressed as µg metal/g DW.
Statistical analysis
Two-way ANOVA was applied using injection type (Control, Live Ps, and Inactivated Ps) and time (3, 7, and 14 dpi) as variation factors. Data were transformed when necessary to meet parametric assumptions and post hoc Tuckey was applied to identify differences among groups. Each value is the mean ± S.E.M (n = 6). Different letters indicate statistical differences in the same treatment over time and symbols indicate statistical differences between the three conditions (Control, Live Ps, and Inactivated Ps) at the same time. Statistical differences were considered for a value of P < 0.05.
Results
Mortality and detection of P. salmonis DNA
No mortality or behavioral changes were observed for any fish group during the experimental period (3-14 dpi). As estimated, the genetic material of P. salmonis (DNA) was detected at 14 dpi in the liver of fish stimulated with live and inactivated P. salmonis. No evidence of P. salmonis DNA was detected in the control group (Figure 1).
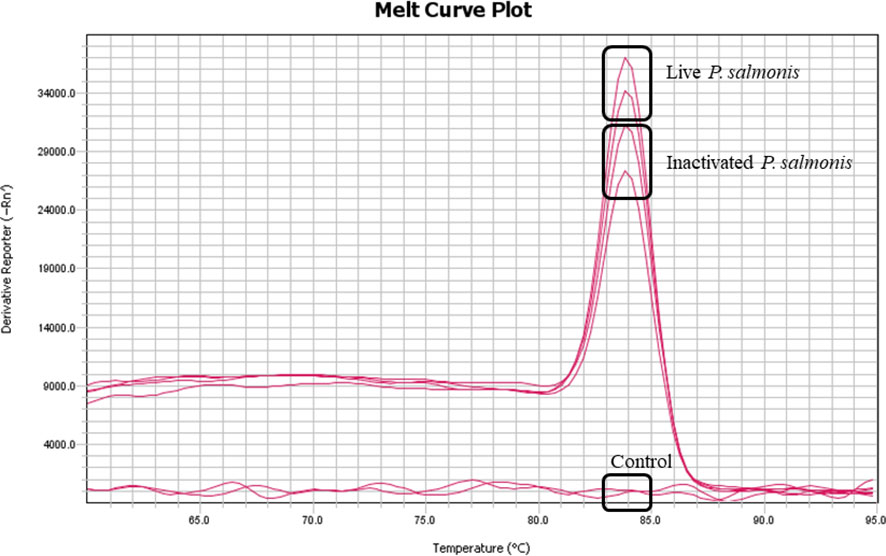
Figure 1 Detection of genetic material (DNA) of P. salmonis in the liver tissue at 14 dpi. Melting curve (Tm) of the 16s gene from P. salmonis amplified by qPCR of pooled DNA from each experimental condition.
Hematocrit
The hematocrit percentage decreased in a statistically significant manner at 3 [37.89 ± 0.72] and 7 [46.0 ± 2.33] dpi in fish stimulated with live P. salmonis, compared to the control group [48.11 ± 1.21 (3 dpi)/54.68 ± 0.96 (7 dpi)]. On the other hand, no statistical differences between control fish group and the fish group stimulated with inactivated P. salmonis were observed. However, statistical differences between the different times for this treatment were determined (Figure 2).
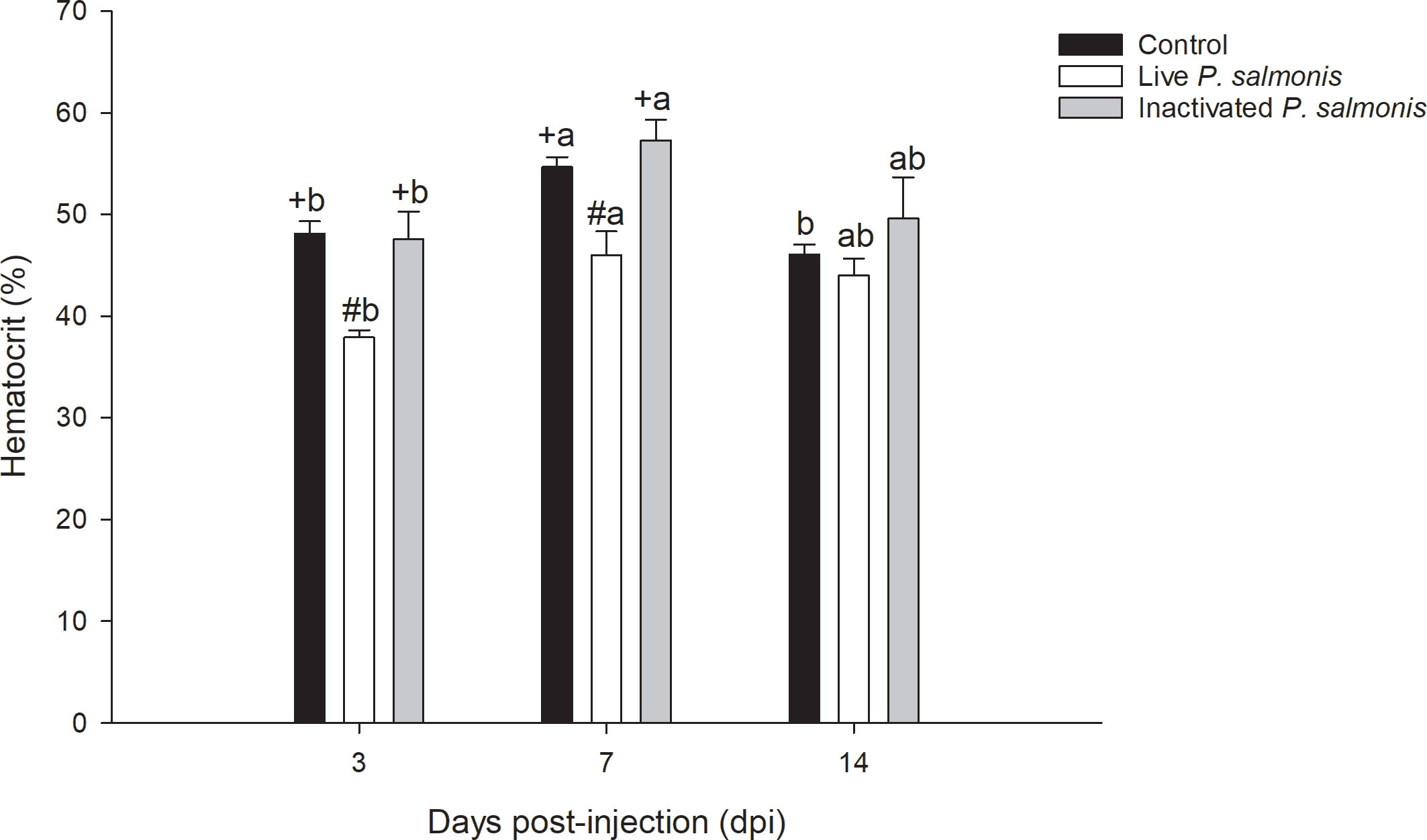
Figure 2 The hematocrit percentage in the blood of S. salar. The samples were taken at 3, 7, and 14 days after injection (dpi). Black bar: control condition, white bar (fish stimulated with live P. salmonis), and gray bar (fish stimulated with inactivated P. salmonis). Symbols (+, #) over the bars indicate statistical differences between the different treatments at the same time points. Different letters (A, B) indicate statistical differences in the same treatment at different times. Two-way ANOVA, p < 0.05; n=6.
Plasma iron
Plasma iron levels decreased statistically at 3 dpi in both the groups of fish stimulated with live P. salmonis [38.58 ± 6.48 µmol/L] and inactivated [43.97 ± 4.93 µmol/L], compared to the control group [88.28 ± 10.34 µmol/L]. In addition, a decrease in the iron levels at 7 and 14 dpi was also observed in both treatments; However, this decrease was not statistically significant (Figure 3).
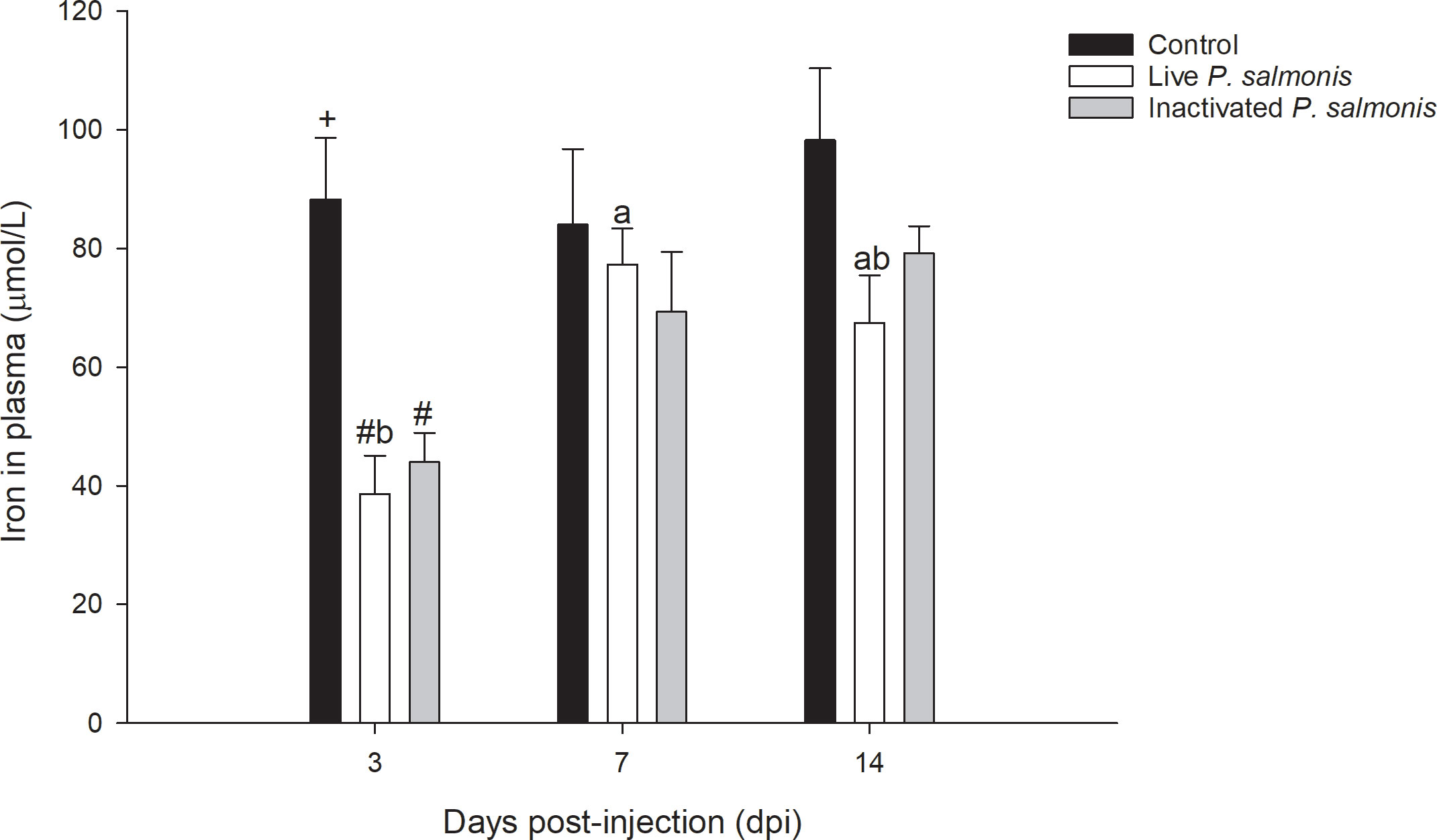
Figure 3 Plasma iron levels in S. salar. The samples were taken at 3, 7, and 14 days after injection (dpi). Black bar: control condition, white bar (fish stimulated with live P. salmonis), and gray bar (fish stimulated with inactivated P. salmonis). Symbol (#) over the bars indicates statistical differences between the different treatments at the same time points. Different letters a, b indicate statistical differences in the same treatment at different times. Two-way ANOVA, p < 0.05; n=6.
Gene expression of markers involved in nutritional immunology
The tfr1 marker involved in micronutrient uptake exhibited an increase in its transcription at 3 dpi [0.90 ± 0.03 Log2 FC] and decreased at 7 dpi [-1.36 ± 0.12 Log2 FC] in fish stimulated with live P. salmonis. On the contrary, fish stimulated with inactivated P. salmonis showed a decrease in the transcription of this marker at 3-7 dpi [-0.69 ± 0.02 (3 dpi)/-1.41 ± 0.06 (7 dpi) Log2 FC] with a statistically significant increase at 14 dpi [1.77 ± 0.18 Log2 FC] (Figure 4A).
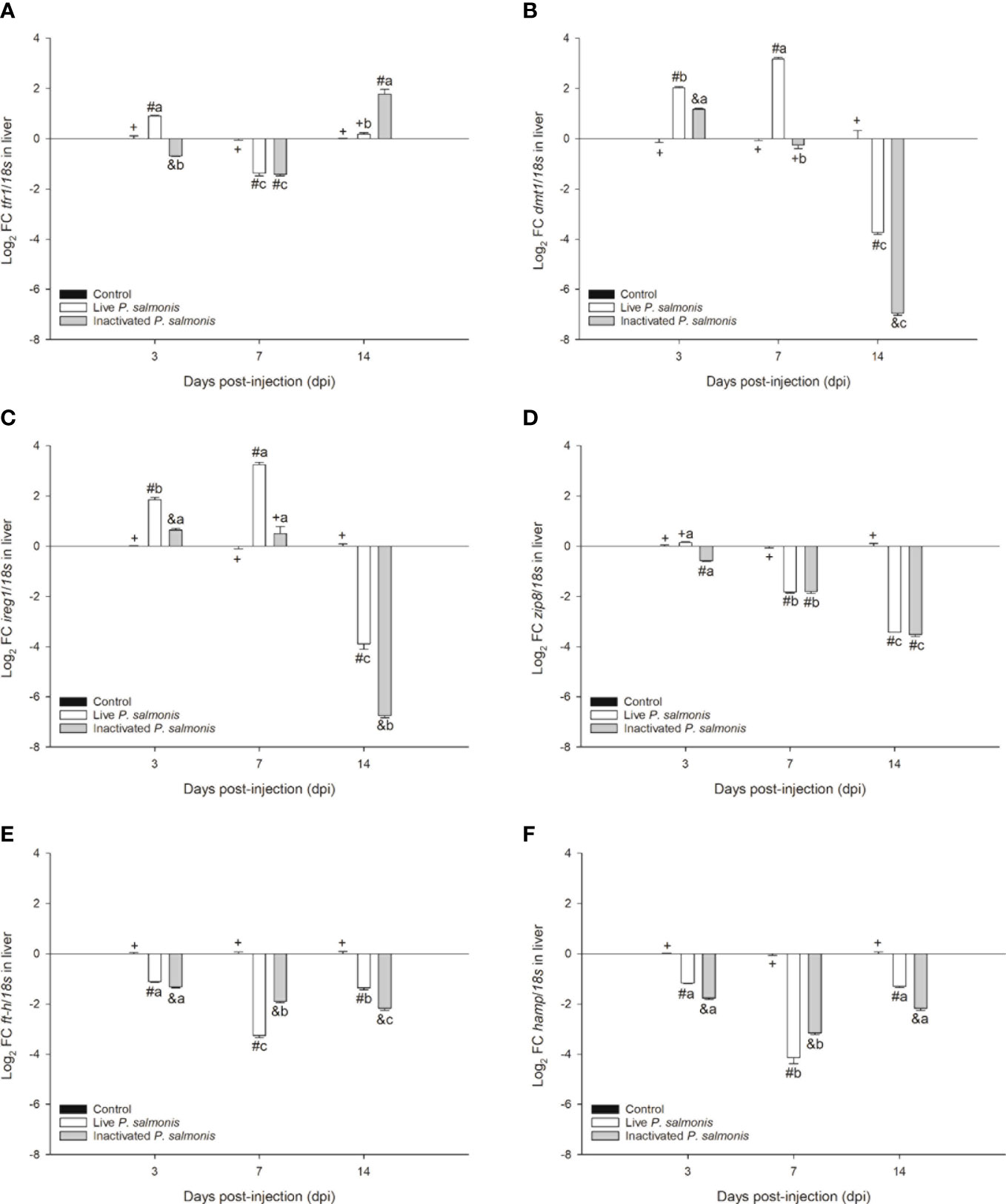
Figure 4 Gene expression of markers involved in nutritional immunology in S. salar. The samples were taken at 3, 7, and 14 days after injection (dpi). (A): transferrin receptor 1. (B): divalent metal transporter 1. (C): ferroportin 1. (D): zinc transporter 8. (E): ferritin heavy chain. (F): hepcidin. Symbols (+, #, &) over the bars indicate statistical differences between the different treatments at the same time points. Different letters a, b, c indicate statistical differences in the same treatment at different times. Two-way ANOVA, p < 0.05; n=6.
Markers involved in the transport of micronutrients such as dmt1 and ireg1 displayed the same expression profile, i.e., increasing statistically at 3 dpi in fish stimulated with live [2.02 ± 0.04 (dmt1)/1.85 ± 0.09 (ireg1) Log2 FC] and inactivated P. salmonis [1.17 ± 0.04 (dmt1)/0.64 ± 0.07 (ireg1) Log2 FC]; however, at 7 dpi, the increase was statistically significant only in fish stimulated with live P. salmonis [3.17 ± 0.07 (dmt1)/3.23 ± 0.10 (ireg1) Log2 FC], compared to at 14 dpi, where the transcription of both markers decreased significantly in fish stimulated with live P. salmonis [-3.74 ± 0.07 (dmt1)/-3.89 ± 0.22 (ireg1) Log2 FC] and inactivated [-6.94 ± 0.09 (dmt1)/-6.74 ± 0.09 (ireg1) Log2 FC] (Figures 4B, C).
The zip8 marker, which is also involved in the transport of micronutrients, significantly decreased its transcription in fish stimulated with live P. salmonis [-1.82 ± 0.03 (7 dpi)/-3.43 ± 0.05 (14 dpi) Log2 FC] and inactivated bacteria [-0.57 ± 0.02 (3 dpi)/-1.80 ± 0.07 (7 dpi)/- 3.52 ± 0.07 (14 dpi) Log2 FC]. At 3 dpi, fish stimulated with live P. salmonis did not show statistical differences compared to the control group (Figure 4D).
Finally, the markers involved in the storage (ft-h) and regulation (hamp) of micronutrients showed a statistically significant decrease in their transcription levels at 3, 7, and 14 dpi in fish stimulated with live P. salmonis [-1.11 ± 0.03 (3 dpi, ft-h)/-3.26 ± 0.08 (7 dpi, ft-h)/-1.36 ± 0.07 (14 dpi, ft-h)/-0.17 ± 0.02 (3 dpi, hamp)/-4.14 ± 0.23 (7 dpi, hamp)/-1.30 ± 0.04 (14 dpi, hamp) Log2 FC] and inactivated [-1.33 ± 0.03 (3 dpi, ft-h)/-1.90 ± 0.04 (7 dpi, ft-h)/-2.17 ± 0.09 (14 dpi, ft-h)/-1.77 ± 0.05 (3 dpi, hamp)/-3.16 ± 0.07 (7 dpi, hamp)/-2.17 ± 0.08 (14 dpi, hamp) Log2 FC] (Figures 4E, F).
Micronutrients in liver
The concentration of iron in the liver tissue did not reflect any statistical differences with respect to the control group at 3 dpi; however, at 7 and 14 dpi, the tissue concentration of this micronutrient increased significantly in fish stimulated with live P. salmonis [1923.40 ± 85.07 (7 dpi)/1526.83 ± 13.92 (14 dpi) µg Fe/g DW] and inactivated [2127.00 ± 59.87 (7 dpi)/1736.00 ± 20.87 (14 dpi) µg Fe/g DW], compared to the control group [1444.00 ± 32.31 (7 dpi)/1402.83 ± 12.42 (14 dpi) µg Fe/g DW] (Figure 5A).
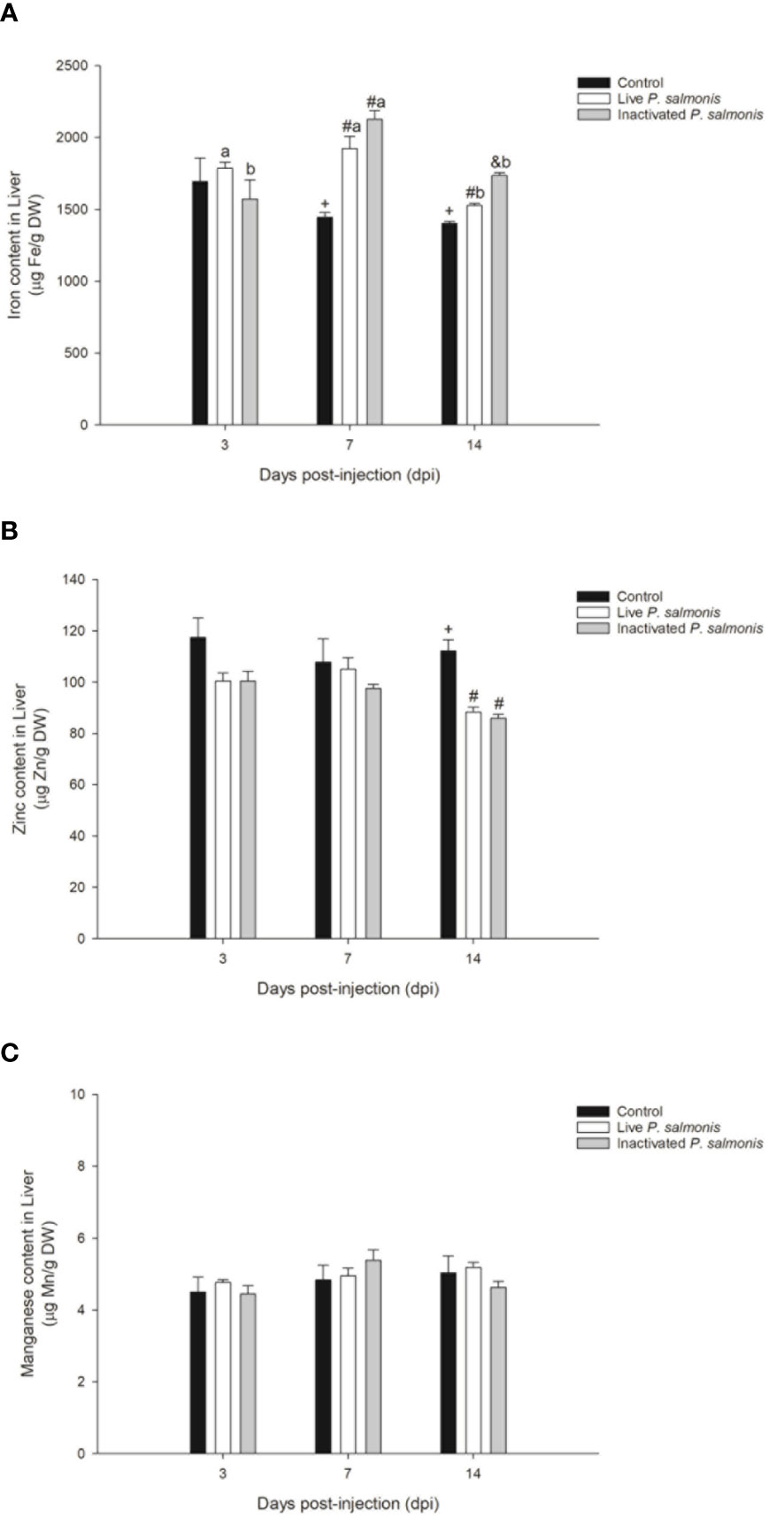
Figure 5 Micronutrient content in the liver of S. salar. The samples were taken at 3, 7 and 14 days after injection (dpi). Black bar: control condition, white bar (fish stimulated with live P. salmonis) and gray bar (fish stimulated with inactivated P. salmonis). (A): iron. (B): zinc. (C): manganese. Symbols (+, #, &) over the bars indicate statistical differences between the different treatments at the same time points. Different letters a, b indicate statistical differences in the same treatment at different times. Two-way ANOVA, p < 0.05; n=6.
On the other hand, the zinc concentration did not show statistically significant changes at 3 and 7 dpi but decreased significantly at 14 dpi in fish stimulated with live P. salmonis [88.33 ± 1.89 µg Zn/g DW] and inactivated [86.00 ± 1.48 µg Zn/g DW], compared to the control group [112.16 ± 4.31 µg Zn/g DW] (Figure 5B).
Finally, the manganese content did not show statistically significant changes among the different treatments evaluated during the experimental course (Figure 5C).
Discussion
This study evaluated the activation of nutritional immunity in Atlantic salmon (Salmo salar) specimens challenged intraperitoneally with P. salmonis, which causes high mortality rates in the Chilean salmon industry. We detected genetic material (DNA) of this bacterium in the liver of fish after 14 days of stimulation with both live and inactivated P. salmonis, indicating that the live bacterium could be established in this tissue, and that fish injected with the inactivated bacterium still retain remnants of genetic material that must be eliminated from their system. This may correlate with the bacterial dose administered (medium dose) into the tissue and the experimental time evaluated (3-14 dpi) since in fish the innate (early and non-specific) and adaptive (late and specific) immune responses have activation time ranges and both are necessary to combat pathogens and/or PAMPs at the cellular and systemic levels (40). In fact, Isla et al. (41) reported that IgM levels increase from day 28 in S. salar that are challenged with P. salmonis. Interestingly, an increase in IgM Anti-P. salmonis was observed from day 14 in E. maclovinus exposed to two strains of P. salmonis (42), suggesting that the immune response could be dependent on the species, stage of fish development, as well as doses and time of exposure to the pathogen.
Regarding the hematocrit, in the fish stimulated with live P. salmonis, it decreased in a statistically significant manner its percentage at 3 and 7 dpi while no changes were detected at 14 dpi. On the other hand, the fish stimulated with inactivated P. salmonis did not show changes in this parameter over the experimental course. The latter was to be expected since only a living pathogen provides its functional cellular machinery for the synthesis of hemolysins to be induced in P. salmonis, and consequently, the lysis of erythrocytes in Atlantic salmon (Salmo salar) (5), which could corroborate the anemia generated by Piscirickettsiosis (2). These results are not in agreement with what was previously published by Isla et al. (41), who reported a decrease in hematocrit from day 7 to 28 post-inoculation with live P. salmonis. However, this may be correlated to the bacterial dose they administered (low dose) and the size of the used smolt specimens (60-70 g), compared with the post-smolt specimens (700 g) used in this study, according to the ranges determined by Rozas-Serri et al. (43).
The lysis of erythrocytes is a mechanism of pathogenicity of P. salmonis that culminates in the release of iron that is contained in the heme group from these cell types (5). Interestingly, genomic (sequencing/annotation) and functional studies indicate that P. salmonis possesses the necessary machinery to sequester this micronutrient from different sources (5, 11–13, 44). Similarly, fish have developed mechanisms to avoid the sequestration of micronutrients, decreasing the levels of micronutrients at a systemic level (6). Our research demonstrated a decrease in the levels of plasma iron in fish stimulated with live and inactivated P. salmonis (3 dpi); however, this decrease was not statistically significant at 7 and 14 dpi. These findings agree with previous reports on smolt specimens of Atlantic salmon (Salmo salar) inoculated with a low dose of live P. salmonis (41) and in Patagonian blennie (E. maclovinus) challenged with Francisella noatunensis subsp. Noatunensis (39), which is a facultative intracellular bacterial pathogen that have similarities in terms of pathogenesis with P. salmonis (45).
The findings suggest that nutritional immunity is not capable of differentiating between live pathogens and dead or inactivated ones. Thus, the activation of this response could be induced by the detection of specific PAMPs rather than by the establishment of functional bacterial machinery. This was previously suggested in an in vitro assay using the SHK-1 cells stimulated with LPS, OMVs, and TP extracted from P. salmonis, where markers such as zip8, zip14, dmt1, ireg1, tfr1, ft-h, ft-m, il6, hamp, irp1, and irp2 were modulated between 15 and 120 min post-stimulation with these different PAMPs (10), although in Antarctic fish such as Notothenia rossii and Notothenia coriiceps, the stimulation with commercial LPS did not alter plasma iron levels on day 5 post-intraperitoneal stimulation (9).
Immuno-nutritional markers that participate in the homeostasis of iron, manganese, and zinc are fundamental so that these are not sequestered by microorganisms (6, 14). In our research, these markers showed different expression profiles based on the type of bacterial stimulation (live and inactivated P. salmonis) and the experimental time evaluated (3-14 dpi). Specifically, tfr1 involved in micronutrient uptake, showed a modulation in its transcription in fish stimulated with live and inactivated P. salmonis, while the transcription of the dmt1 and ireg1 transporters presented the same expression profile, increasing at 3 dpi and decreasing at 14 dpi in the fish stimulated with live and inactivated P. salmonis. On the other hand, zip8 involved in the transport of Zn2+, Fe2+, HseO3- y Mn2+ (46), ft-h involved in storage (47), and hamp involved in the regulation of micronutrients (48) showed down-regulation in their transcription levels in the liver of fish stimulated with both live and inactivated P. salmonis throughout the experimental course. The results suggest that stimulation with live and inactivated P. salmonis induces the transcriptional modulation of immune-nutritional markers in the liver of S. salar and that this modulation could be related to the decrease in plasma iron levels. These transcriptional findings have been corroborated by previous studies, which describe the activation of these markers in the head kidney of S. salar families with high and low susceptibility to P. salmonis (5), as well as in the liver and brain of E. maclovinus challenged with P. salmonis (7, 8), F. noatunensis subsp. Noatunensis (39), and in Antarctic fish such as Black rockcod (Notothenia coriiceps) and Marbled rockcod (Notothenia rossii) stimulated with commercial LPS (9).
Finally, the micronutrient content in the liver of Atlantic salmon (Salmo salar) revealed that the intracellular iron increased at 7 and 14 dpi in fish stimulated with live and inactive P. salmonis, while zinc content decreased at 14 dpi in both experimental conditions. However, stimulation with live and inactivated P. salmonis did not modulate the manganese content during the experiment. These results indicate that the decrease in iron in plasma could be related to the increase of this micronutrient in the liver and, more importantly, this mechanism could be a pathogenesis strategy used by P. salmonis, focused on concentrating the availability of intracellular iron necessary for its replication, similar to performed by Francisella spp (49). This was corroborated by Pulgar et al. (5), who indicated that families of Atlantic salmon (Salmo salar) susceptible to P. salmonis infection did not reduce the iron content in the head kidney unlike by the families resistant to this bacterial pathogen. In addition, these researchers concluded that the levels of zinc in the head kidney did not present any statistical differences between the families susceptible and resistant to P. salmonis. However, we observed a decrease in the zinc content at 14 dpi in the liver of fish stimulated with live and inactivated P. salmonis, which suggest that there could also be competition for this micronutrient, similar to that observed in other bacterial pathogens such as Escherichia coli (50), Staphylococcus aureus (51), Salmonella typhimurium (52) y Mycobacterium tuberculosis (53).
In conclusion, this study is the first to reveal the activation of nutritional immunity associated with micronutrient such as iron, manganese, and zinc at the systemic and cellular levels in S. salar when challenged with live and inactivated P. salmonis. The study results suggest that nutritional immunity in S. salar would not distinguish between live P. salmonis that needs micronutrients for its replication and inactivated P. salmonis which does not. The immune-nutritional markers involved in this response were modulated in both treatments, live and inactivated bacteria, during the experimental course (3, 7 and 14 dpi). This indicates that the nutritional immunity, being part of innate immunity, could be activated through the detection of PAMPs rather than a real sequestration and/or competition for the micronutrient by the live bacteria.
Data availability statement
The original contributions presented in the study are included in the article/supplementary materials. Further inquiries can be directed to the corresponding authors.
Ethics statement
The animal study was reviewed and approved by Ethical Committee of Universidad Austral de Chile (383/2020) in agreement with the National Agency for Research and Development (ANID, Chile).
Author contributions
DM, LV-C, and AR conception and design of research; DM, RO-S, JC, and CV-L performed the experiments; DM, AQ, JC, and NS analyzed the samples; DM and RO-S analyzed the data; DM, RO-S, and CO interpreted the results of experiments; DM prepared the figures; DM drafted the manuscript; DM, MG, JM, LV-C, and AR edited and revised the manuscript; DM, RO-S, CO, and LV-C approved the final version of the manuscript.
Funding
This study received financial assistance from Fondecyt-Postdoctoral N° 3200418 to DM, Fondap-Ideal Grant N° 15150003 to LV-C, ANID-Millennium Science Initiative Program-Center ICM-ANID ICN2021_002 to LV-C, and Fondap-ANID N° 1522A0004 to AR.
Conflict of interest
The authors declare that the research was conducted in the absence of any commercial or financial relationships that could be construed as a potential conflict of interest.
Publisher’s note
All claims expressed in this article are solely those of the authors and do not necessarily represent those of their affiliated organizations, or those of the publisher, the editors and the reviewers. Any product that may be evaluated in this article, or claim that may be made by its manufacturer, is not guaranteed or endorsed by the publisher.
References
2. Rozas M, Enríquez R. Piscirickettsiosis and Piscirickettsia salmonis in fish: a review. J Fish Dis (2014) 37:163–88. doi: 10.1111/jfd.12211
4. Munang’andu HM. Intracellular bacterial infections: a challenge for developing cellular mediated immunity vaccines for farmed fish. Microorganisms (2018) 6:33. doi: 10.3390/microorganisms6020033
5. Pulgar R, Hödar C, Travisany D, Zuñiga A, Domínguez C, Maass A, et al. Transcriptional response of Atlantic salmon families to Piscirickettsia salmonis infection highlights the relevance of the iron-deprivation defence system. BMC Genomics (2015) 16:495. doi: 10.1186/s12864-015-1716-9
6. Hood I, Skaar E. Nutritional immunity: transition metals at the pathogen-host interface. Nat Rev Microbiol (2012) 10:525–37. doi: 10.1038/nrmicro2836
7. Martínez D, Oyarzún R, Vargas-Lagos C, Pontigo JP, Soto-Dávila M, Saravia J, et al. Identification, characterization and modulation of ferritin-h in the sub-Antarctic notothenioid. Eleginops maclovinus challenged Piscirickettsia salmonis. Dev Comp Immunol (2017) 73:88–96. doi: 10.1016/j.dci.2017.03.015
8. Martínez D, Oyarzún R, Pontigo JP, Romero A, Yáñez A, Vargas-Chacoff L. Nutritional immunity triggers the modulation of iron metabolism genes in the Sub-Antarctic notothenioid Eleginops maclovinus in response to Piscirickettsia salmonis. Front Immunol (2017) 8:1–12. doi: 10.3389/fimmu.2017.01153
9. Martínez D, Sousa C, Oyarzún R, Pontigo JP, Canario A, Power D, et al. LPS modulates the expression of iron-related immune genes in two Antarctic notothenoids. Front Physiol (2020) 11:1–13. doi: 10.3389/fphys.2020.00102
10. Martínez D, Oliver C, Santibañez N, Coronado JL, Oyarzún-Salazar R, Enriquez R, et al. PAMPs of Piscirickettsia salmonis trigger the transcription of genes involved in nutritional immunity in a salmon macrophage-like cell line. Front Immunol (2022) 13:1–8. doi: 10.3389/fimmu.2022.849752
11. Almarza O, Valderrama K, Ayala M, Segovia C, Santander J. A functional ferric uptake regulator (Fur) protein in the fish pathogen Piscirickettsia salmonis. Int Microbiol (2016) 19:49–55. doi: 10.2436/20.1501.01.263
12. Machuca A, Martinez V. Transcriptome analysis of the intracellular facultative pathogen Piscirickettsia salmonis: expression of putative groups of genes associated with virulence and iron metabolism. PloS One (2016) 11:1–17. doi: 10.1371/journal.pone.0168855
13. Calquín P, Ruiz P, Oliver C, Sánchez P, Haro R, Oliva H, et al. Physiological evidence that Piscirickettsia salmonis produces siderophores and uses iron from different sources. J Fish Dis (2018) 41:553–8. doi: 10.1111/jfd.12745
14. Kehl-fie TE, Skaar EP. Nutritional immunity beyond iron: a role for manganese and zinc. Curr Opin Chem Biol (2010) 14:218–24. doi: 10.1016/j.cbpa.2009.11.008
15. Clearwater SJ, Farag AM, Meyer JS. Bioavailability and toxicity of dietborne copper and zinc to fish. Comp Biochem Physiol - C Toxicol Pharmacol (2002) 132:269–313. doi: 10.1016/S1532-0456(02)00078-9
16. Taylor EW. Toxicology of aquatic pollution: physiological, molecular and cellular approaches. (2009).
17. Hentze MW, Muckenthaler MU, Andrews NC. Balancing acts: molecular control of mammalian iron metabolism. Cell (2004) 117:285–97. doi: 10.1016/S0092-8674(04)00343-5
18. Andreini C, Bertini I, Cavallaro G, Holliday GL, Thornton JM. Metal ions in biological catalysis: from enzyme databases to general principles. J Biol Inorg Chem (2008) 13:1205–18. doi: 10.1007/s00775-008-0404-5
19. Wintergerst ES, Maggini S, Hornig DH. Contribution of selected vitamins and trace elements to immune function. Ann Nutr Metab (2007) 51:301–23. doi: 10.1159/000107673
20. Kitamura H, Morikawa H, Kamon H, Iguchi M, Hojyo S, Fukada T, et al. Toll-like receptor-mediated regulation of zinc homeostasis influences dendritic cell function. Nat Immunol (2006) 7:971–7. doi: 10.1038/ni1373
21. Waldron KJ, Robinson NJ. How do bacterial cells ensure that metalloproteins get the correct metal? Nat Rev Microbiol (2009) 7:25–35. doi: 10.1038/nrmicro2057
22. Kehres DG, Janakiraman A, Slauch JM, Maguire ME. SitABCD is the alkaline Mn (2+) transporter of Salmonella enterica serovar typhimurium. J Bacteriol (2002) 184:3159–66. doi: 10.1128/JB.184.12.3159-3166.2002
23. Corbett D, Wang J, Schuler S, Gloria LC, Glenn S, Brough D, et al. Two zinc uptake systems contribute to the full virulence of Listeria monocytogenes during growth in vitro and in vivo. Infect Immun (2012) 80:14–21. doi: 10.1128/IAI.05904-11
24. Bayle L, Chimalapati S, Schoehn G, Brown J, Vernet T, Durmort C. Zinc uptake by Streptococcus pneumoniae depends on both AdcA and AdcAII and is essential for normal bacterial morphology and virulence. Mol Microbiol (2011) 82:904–16. doi: 10.1111/j.1365-2958.2011.07862.x
25. Hohle TH, Franck WL, Stacey G, O’Brian MR. Bacterial outer membrane channel for divalent metal ion acquisition. Proc Natl Acad Sci (2011) 108:15390–5. doi: 10.1073/pnas.1110137108
26. Ammendola S, Pasquali P, Pistoia C, Petrucci P, Petrarca P, Rotilio G, et al. High-affinity Zn2+ uptake system ZnuABC is required for bacterial zinc homeostasis in intracellular environments and contributes to the virulence of. Salmonella enterica. Infect Immun (2007) 75:5867–76. doi: 10.1128/IAI.00559-07
27. Campoy S, Jara M, Busquets N, Ana M, De RP, Badiola I, et al. Role of the high-affinity zinc uptake znuABC system in Salmonella enterica serovar typhimurium virulence. Infect Immun (2002) 70:4721–5. doi: 10.1128/IAI.70.8.4721-4725.2002
28. Davis LM, Kakuda T, DiRita VJ. A campylobacter jejuni znuA orthologue is essential for growth in low-zinc environments and chick colonization. J Bacteriol (2009) 191:1631–40. doi: 10.1128/JB.01394-08
29. Bearden SW, Perry RD. The yfe system of Yersinia pestis transports iron and manganese and is required for full virulence of plague. Mol Microbiol (1999) 32:403–14. doi: 10.1046/j.1365-2958.1999.01360.x
30. Perry RD, Craig SK, Abney J, Bobrov AG, Kirillina O, Mier I, et al. Manganese transporters yfe and MntH are fur-regulated and important for the virulence of. Yersinia pestis. Microbiol (2012) 158:804–15. doi: 10.1099/mic.0.053710-0
31. Champion OL, Karlyshev A, Cooper IAM, Ford DC, Wren BW, Duffield M, et al. Yersinia pseudotuberculosis mntH functions in intracellular manganese accumulation, which is essential for virulence and survival in cells expressing functional Nramp1. Microbiology (2011) 157:1115–22. doi: 10.1099/mic.0.045807-0
32. Anderson ES, Paulley JT, Gaines JM, Valderas MW, Martin DW, Menscher E, et al. The manganese transporter MntH is a critical virulence determinant for Brucella abortus 2308 in experimentally infected mice. Infect Immun (2009) 77:3466–74. doi: 10.1128/IAI.00444-09
33. Rosadini CV, Gawronski JD, Raimunda D, Argüello JM, Akerley BJ. A novel zinc-binding system, zevAB, is critical for survival of non-typeable Haemophilus influenzae in a murine lung infection model. Infect Immun (2011) 79:3366–76. doi: 10.1128/IAI.05135-11
34. Yañez AJ, Valenzuela K, Silva H, Retamales J, Romero A, Enriquez R, et al. Broth medium for the successful culture of the fish pathogen. Piscirickettsia salmonis. Dis Aquat Organ (2012) 97:197–205. doi: 10.3354/dao02403
35. Birkbeck TH, Rennie S, Hunter D, Laidler LA, Wadsworth S. Infectivity of a Scottish isolate of Piscirickettsia salmonis for Atlantic salmon Salmo salar and immune response of salmon to this agent. Dis Aquat Organ (2004) 60:97–103. doi: 10.3354/dao060097
36. Karatas S, Mikalsen J, Steinum TM, Taksdal T, Bordevik M, Colquhoun DJ. Real-time PCR detection of Piscirickettsia salmonis from formalin-fixed paraffin-embedded tissues. J Fish Dis (2008) 31:747–53. doi: 10.1111/j.1365-2761.2008.00948.x
37. Livak KJ, Schmittgen TD. Analysis of relative gene expression data using real-time quantitative PCR and the 2-ΔΔct method. Methods (2001) 25:402–8. doi: 10.1006/meth.2001.1262
38. Valenzuela-Muñoz V, Gallardo-Escárate C. Iron metabolism modulation in Atlantic salmon infested with the sea lice Lepeophtheirus salmonis and Caligus rogercresseyi: a matter of nutritional immunity? Fish Shellfish Immunol (2017) 60:97–102. doi: 10.1016/j.fsi.2016.11.045
39. Quilapi AM, Vargas-Lagos C, Martínez D, Muñoz JL, Spies J, Esperguel I, et al. Brain immunity response of fish Eleginops maclovinus to infection with Francisella noatunensis: the brain´s immunity response. Fish Shellfish Immunol (2022) 120:695–705. doi: 10.1016/j.fsi.2021.11.026
40. Smith NC, Rise ML, Christian SL. A comparison of the innate and adaptive immune systems in cartilaginous fish, ray-finned fish, and lobe-finned fish. Front Immunol (2019) 10:2292. doi: 10.3389/fimmu.2019.02292
41. Isla A, Sánchez P, Ruiz P, Albornoz R, Pontigo JP, Rauch MC, et al. Effect of low-dose Piscirickettsia salmonis infection on haematological-biochemical blood parameters in Atlantic salmon (Salmo salar). J Fish Biol (2022) 4:1–12. doi: 10.1111/jfb.15167
42. Martínez D, Díaz-Ibarrola D, Vargas-Lagos C, Oyarzún R, Pontigo J-P, Muñoz J-L, et al. Immunological response of the Sub-Antarctic notothenioid fish Eleginops maclovinus injected with two strains of Piscirickettsia salmonis. Fish Shellfish Immunol (2018) 75:139–48. doi: 10.1016/j.fsi.2018.01.012
43. Rozas-Serri M, Correa R, Walker-Vergara R, Coñuecar D, Barrientos S, Leiva C, et al. Reference intervals for blood biomarkers in farmed Atlantic salmon, coho salmon and rainbow trout in Chile: promoting a preventive approach in aquamedicine. Biol (Basel) (2022) 11:1066. doi: 10.3390/biology11071066
44. Ortiz-severín J, Travisany D, Maass A, Cambiazo V, Chávez F. Global proteomic profiling of Piscirickettsia salmonis and salmon macrophage-like cells during intracellular infection. Microorganisms (2020) 8:1845. doi: 10.3390/microorganisms8121845
45. Colquhoun DJ, Duodu S. Francisella Infections in farmed and wild aquatic organisms. Vet Res (2011) 42:1–15. doi: 10.1186/1297-9716-42-47
46. Nebert DW, Liu Z. SLC39A8 gene encoding a metal ion transporter: discovery and bench to bedside. Human Genomics (2019) 13:1–21. doi: 10.1186/s40246-019-0233-3
47. Torti FM, Torti SV. Regulation of ferritin genes and protein. Blood (2002) 99:3505–16. doi: 10.1182/blood.V99.10.3505
48. Nemeth E, Tuttle MS, Powelson J, Vaughn MD, Donovan A, Ward DMV, et al. Hepcidin regulates cellular iron efflux by binding to ferroportin and inducing its internalization. Sci (80- ) (2004) 306:2090–3. doi: 10.1126/science.1104742
49. Jones C, Napier B, Sampson T, Llewellyn A, Schroeder M, Weiss D. Subversion of host recognition and defense systems by Francisella spp. Microbiol Mol Biol Rev (2012) 76:383–404. doi: 10.1128/MMBR.05027-11
50. Gläser R, Harder J, Lange H, Bartels J, Christophers E, Schröder JM. Antimicrobial psoriasin (S100A7) protects human skin from Escherichia coli infection. Nat Immunol (2005) 6:57–64. doi: 10.1038/ni1142
51. Corbin BD, Seeley EH, Raab A, Feldmann J, Miller MR, Torres VJ, et al. Bacterial growth in tissue abscesses. Sci (80- ) (2008) 319:962–6. doi: 10.1126/science.1152449
52. Liu JZ, Jellbauer S, Poe AJ, Ton V, Pesciaroli M, Kehl-Fie TE, et al. Zinc sequestration by the neutrophil protein calprotectin enhances Salmonella growth in the inflamed gut. Cell Host Microbe (2012) 11:227–39. doi: 10.1016/j.chom.2012.01.017
Keywords: nutritional immunity, Piscirickettsia salmonis, Salmo salar, iron, zinc, manganese, Atlantic salmon
Citation: Martínez D, Oyarzún-Salazar R, Quilapi AM, Coronado J, Enriquez R, Vargas-Lagos C, Oliver C, Santibañez N, Godoy M, Muñoz JL, Vargas-Chacoff L and Romero A (2023) Live and inactivated Piscirickettsia salmonis activated nutritional immunity in Atlantic salmon (Salmo salar). Front. Immunol. 14:1187209. doi: 10.3389/fimmu.2023.1187209
Received: 15 March 2023; Accepted: 18 April 2023;
Published: 28 April 2023.
Edited by:
Julio Villena, CONICET Centro de Referencia para Lactobacilos (CERELA), ArgentinaReviewed by:
Shivendra Kumar, Dr. Rajendra Prasad Central Agricultural University, IndiaStefania Dentice Maidana, National University of Tucumán, Argentina
Copyright © 2023 Martínez, Oyarzún-Salazar, Quilapi, Coronado, Enriquez, Vargas-Lagos, Oliver, Santibañez, Godoy, Muñoz, Vargas-Chacoff and Romero. This is an open-access article distributed under the terms of the Creative Commons Attribution License (CC BY). The use, distribution or reproduction in other forums is permitted, provided the original author(s) and the copyright owner(s) are credited and that the original publication in this journal is cited, in accordance with accepted academic practice. No use, distribution or reproduction is permitted which does not comply with these terms.
*Correspondence: Danixa Martínez, danixa.martinez@uss.cl; Luis Vargas-Chacoff, luis.vargas@uach.cl; Alex Romero, alexromero@uach.cl