- 1ARUP Laboratories Institute for Clinical and Experimental Pathology, Salt Lake City, UT, United States
- 2Department of Internal Medicine, Spencer Fox Eccles School of Medicine, University of Utah, Salt Lake City, UT, United States
- 3Department of Pathology, Spencer Fox Eccles School of Medicine, University of Utah, Salt Lake City, UT, United States
Introduction: The clinical manifestations of acute severe acute respiratory syndrome coronavirus type 2 (SARS-CoV-2) infection and coronavirus disease 2019 (COVID-19) suggest a dysregulation of the host immune response that leads to inflammation, thrombosis, and organ dysfunction. It is less clear whether these dysregulated processes persist during the convalescent phase of disease or during long COVID. We sought to examine the effects of SARS-CoV-2 infection on the proportions of classical, intermediate, and nonclassical monocytes, their activation status, and their functional properties in convalescent COVID-19 patients.
Methods: Peripheral blood mononuclear cells (PBMCs) from convalescent COVID-19 patients and uninfected controls were analyzed by multiparameter flow cytometry to determine relative percentages of total monocytes and monocyte subsets. The expression of activation markers and proinflammatory cytokines in response to LPS treatment were measured by flow cytometry and ELISA, respectively.
Results: We found that the percentage of total monocytes was decreased in convalescent COVID-19 patients compared to uninfected controls. This was due to decreased intermediate and non-classical monocytes. Classical monocytes from convalescent COVID-19 patients demonstrated a decrease in activation markers, such as CD56, in response to stimulation with bacterial lipopolysaccharide (LPS). In addition, classical monocytes from convalescent COVID-19 patients showed decreased expression of CD142 (tissue factor), which can initiate the extrinsic coagulation cascade, in response to LPS stimulation. Finally, we found that monocytes from convalescent COVID-19 patients produced less TNF-α and IL-6 in response to LPS stimulation, than those from uninfected controls.
Conclusion: SARS-CoV-2 infection exhibits a clear effect on the relative proportions of monocyte subsets, the activation status of classical monocytes, and proinflammatory cytokine production that persists during the convalescent phase of disease.
Introduction
Severe acute respiratory syndrome coronavirus type 2 (SARS-CoV-2) is the causative agent of coronavirus infectious disease 19 (COVID-19) As of August 2023, it has affected over 770 million people, resulting in nearly 7 million deaths worldwide (WHO Coronavirus (COVID) dashboard). The symptoms of the disease vary from mild to severe, depending on age, gender, physical health, and other host factors. Notably, a dysregulated host immune response is observed in COVID-19 patients, leading to complications like hyperinflammation, thrombosis, and organ damage (1, 2). Cytokine storm, characterized by elevated levels of circulating cytokines and hyperactivation of immune cells (3, 4), and coagulopathy, an alteration in the normal blood clotting process (5, 6), are the two major mechanisms contributing to COVID-19 pathogenesis. Myeloid cells, including circulating monocytes and tissue macrophages, are pivotal in mediating these pathologies.
Alterations in myeloid cells, particularly monocytes, are evident during acute COVID-19 infection. Specifically, total monocyte counts are decreased, especially in severe disease (7, 8). In addition, multiple studies demonstrate differences in the proportions of classical (CD14+ CD16-), intermediate (CD14+ CD16+), and non-classical monocytes (CD14lo CD16+), with a notable decrease in circulating non-classical monocytes in moderate and severe COVID-19 (9–12). Phenotypic changes in monocytes in patients with moderate and severe COVID-19 infection have also been described, including increased CD83 expression (9) and decreased HLA-DR expression (9–13). During acute infection, monocytes and tissue macrophages are characterized by increased activation marker and inflammatory gene expression as measured by single cell transcriptomics (14, 15). This increase in activated monocytes and macrophages corresponds to increased levels of chemokines and cytokines (15), including TNF-α and IL-1β, and increased interferon-stimulated gene (ISG) expression (16–18). Together, these studies suggest that SARS-CoV-2 infection induces myeloid cells to generate a hyperinflammatory state similar to cytokine storm during acute infection.
The fate of monocytes during the convalescent phase of COVID-19 is less well understood. In some studies, patients who fully recovered from acute COVID-19 infection were found to have increased circulating non-classical monocytes and decreased classical monocytes during convalescence (19–21). In addition, these studies showed that monocytes in convalescent individuals display increased levels of inflammatory genes (21–24), antigen presentation molecules, including HLA-DQA and HLA-DPA (20), and the activation marker CD169 (21). However, other studies have demonstrated a decrease in monocyte percentages in convalescent individuals including those who had severe disease (25, 26) or comorbid conditions (27). While a growing body of research has shed light on monocyte behavior during the convalescent phase, contrasting findings underscore the need for more detailed studies to unravel these complexities.
Similar findings have been seen with long COVID, a heterogeneous syndrome characterized by persistent significant health issues lasting, recurring, or developing three months after initial infection (28–31). Although the mechanisms driving long COVID are poorly understood (32), myeloid cells are thought to play a significant role. A number of studies have demonstrated that patients experiencing long COVID have persistently elevated levels of IFN-β, IFN-Λ1, IFN-γ, TNF-α, IL-1β, IL-6, IL-10, IL-12, and IL-17 (33–36); however, more recent studies have demonstrated that long COVID is associated with cytokine deficiencies (37). In addition, Phetsouphanh et al. described elevated numbers of circulating activated monocytes and plasmacytoid dendritic cells (pDCs) (36). Despite these studies, the contribution of myeloid cells in long COVID, much like in the convalescent phase of the disease, are not well understood.
To further clarify the role and behavior of monocytes post-COVID, our study used a comprehensive approach involving multiparameter flow cytometry and functional assays. We focused on the phenotypic and functional characteristics of monocytes in the circulating blood from convalescent COVID-19 patients. Specifically, we examined the proportions of classical, intermediate, and non-classical monocytes, evaluated their activation status in response to bacterial lipopolysaccharide (LPS), and examined their ability to produce inflammatory cytokines. Our studies revealed several phenotypic and functional alterations in monocytes during the convalescent phase of SARS-CoV-2 infection.
Materials and methods
Study subjects
We obtained donor blood samples from individuals who were recruited under University of Utah Institutional Review Board (IRB) protocol 131664. These individuals were recruited from Salt Lake City, UT and the surrounding metropolitan area between May of 2020 and December of 2021. Informed consent was obtained from all individual participants included in the study. The de-identified specimens were blinded during collection and analysis. Whole blood samples were drawn from 18 convalescent COVID-19 patients and 31 healthy individuals in parallel. The clinical samples were obtained from convalescent patients who exhibited a mild form of the disease and did not require hospitalization. For convalescent patients, specimens were collected approximately two to four weeks after disease onset. Donors reported no symptoms at the time of sample collection. The COVID-19 patients ranged in age from 19 to 65 years old (43.3 y/o mean), representing different races and genders. The healthy control individuals ranged in age from 19 to 71 years old (38.5 y/o mean), representing different races and genders (Table 1). SARS-CoV-2 infection was assessed by one or more immunologic (Abbott Architect SARS-CoV-2 IgG, Euroimmun Anti-SARS-CoV-2 ELISA, or Siemens SARS-CoV-2 IgG) (38) or molecular assays.
Blood samples
Fifteen mL of whole blood was collected by phlebotomy-certified research staff into two BD Vacutainer EDTA Additive Blood Collection Tubes. Tubes were gently inverted eight times to mix the blood and EDTA and were then centrifuged at 150 x g for 20 minutes at room temperature. Peripheral blood mononuclear cells (PBMCs) were isolated by Ficoll density gradient (Histopaque-1077, Sigma), and were cryopreserved in 1 ml aliquots in 90% complete culture media [RPMI 1640 with 10% Fetal Bovine Serum (FBS)] and 10% dimethyl sulfoxide (DMSO) in sterile cryovials. PBMCs were stored in liquid nitrogen prior to use.
Isolation of peripheral blood monocytes
Total monocytes were isolated by negative selection using the EasySep Human Monocyte Enrichment Kit without CD16 Depletion (StemCell Technologies) using the manufacturer’s instructions. Briefly, PBMCs were resuspended at 5 x 107 cells/mL in isolation buffer (PBS with 2% FBS and 1 mM EDTA) and incubated with the enrichment cocktail (50 μL/mL) at 4°C for 10 minutes. Next, vortexed magnetic particles were added, and the sample was mixed and incubated at 4°C for 5 minutes. Isolation media was then added to a final volume of 2.5 mL and the sample was placed in an EasySep magnet at room temperature for 2.5 minutes. The enriched cell suspension (non-monocytes) was poured off into a new tube, and the remaining cells (monocytes) were washed three times with isolation buffer. The tube was then removed from the magnet and purified monocytes were decanted into a new tube for use.
Flow cytometry
Two antibody panels were used in this study. The first antibody panel was designed to identify total monocytes, classical monocytes, non-classical monocytes, intermediate monocytes, B cells, T cells, NK cells, conventional or myeloid dendritic cells (mDCs), and plasmacytoid dendritic cells (pDCs). The cell subsets were identified using the following antibodies: CD64 PE-Cy7 (Cat. # B06025, Beckman Coulter); CD45 PerCP (Cat. # 340665, BD Biosciences); Fixable Viability eFluorTM 506 (Cat. # 65-0866-18, Thermo Fisher Scientific); CD14 V450 (Cat. # 655114, BD Biosciences); CD16 FITC (Cat. # 656147, BD Biosciences); CD3 APC R700 (Cat. # 659110, BD Biosciences); CD19 BV786 (Cat. # 563325, BD Biosciences); HLA-DR APC-H7 (Cat. # 641402, BD Biosciences); CD123 PE (Cat. # 649453, BD Biosciences); CD11b APC (Cat. # 340936, BD Biosciences); CD11c BV605 (Cat. # 663799, BD Biosciences). The gating strategy started with a time gate, followed by exclusion of dead cells (CD45 vs Fixable Viability Dye), and exclusion of doublets (FSC-A vs FSC-H). Monocytes were then selected as CD64+CD3-CD19- gated cells. The initially identified monocytes were then refined as HLA-DR+, CD123-, CD11bhi, and CD11chi cells to exclude DCs. Monocytes were then analyzed for classical, non-classical, and intermediate monocytes by comparing CD14 and CD16 expression. The mDCs were identified from the total WBC population as CD3-, CD19-, HLA-DR+, CD123-, CD11c+, CD11b-, CD14-, and CD16- cells. The pDCs were identified as CD14-, CD16-, HLA-DR-, and CD123+ cells. In addition, our gating also included identification of B cells, T cells, and NK cells as CD45+CD64-CD19+, CD45+CD64-CD3+, and CD45+CD64-CD19-CD3-CD16+ cells, respectively.
The second antibody panel was designed examine the major monocyte subsets (classical, non-classical, and intermediate) and expression of their activation markers, including a marker associated with the extrinsic coagulation pathway (CD142). The antibody panel was used for staining of both short time-stimulated (2-3 hours) and unstimulated samples. The stimulation was carried out at 37°C in CO2 incubator in the presence of 1.0 μg/mL E. coli liposaccharide (LPS, Invivogen) in RPMI 1640 media supplemented with 10% heat-inactivated Fetal Bovine Serum (Gibco Fetal Bovine Serum, Cat. No. 26140087), L-glutamine (200 mM L-Glutamine, ThermoFisher Scientific Cat. No. 25030149), penicillin-streptomycin (Gibco Penicillin-Streptomycin, Cat. No. 15140148). After incubation with and without LPS, cells were washed with 1% FBS in PBS antibody staining buffer and stained with the following antibodies: CD64 PE-Cy7 (Cat. # B06025, Beckman Coulter); CD45 PerCP (Cat. # 340665, BD Biosciences); Fixable Viability eFluorTM 506 (Cat. # 65-0866-18, Thermo Fisher Scientific); CD14 V450 (Cat. # 655114, BD Biosciences); CD16 FITC (Cat. # 656147, BD Biosciences); CD4 APC-H7 (Cat. # 641407, BD Biosciences); CD56 APC R700 (Cat. # 657887, BD Biosciences); CD69 APC (Cat. # 654663, BD Biosciences); CD83 BV786 (Cat. # 565336, BD Biosciences); CD86 BV605 (Cat. # 562999, BD Biosciences); CD142 PE (Cat. # 550312, BD Biosciences). The gating strategy started with a time gate, followed by exclusion of dead cells (CD45 vs Fixable Viability Dye), and exclusion of doublets (FSC-A vs FSC-H). Total monocytes were subsequently selected as CD64+ cells. The identified monocytes were then analyzed for classical, non-classical, and intermediate monocytes by CD14 and CD16 expression. Each cell subset was examined for the expression of CD4, CD56, CD69, CD83, and CD86 activation markers and the marker for the extrinsic coagulation pathway (CD142).
Determination of cytokine concentration
Isolated monocytes were plated at 1x105 cells/well in 96-well plates in 200 μL of complete culture medium (RPMI 1640 with 10% FBS, 100 U/mL penicillin, 100 μg/mL streptomycin, and 0.29 mg/mL L-glutamine). Monocytes were treated with E. coli LPS (Invivogen) at 100 ng/mL. After 18 hours, the plates were briefly centrifuged, and cell-free supernatant was harvested for analysis. TNFα and IL-6 were measured in culture supernatant by ELISA (R&D Systems) according to the manufacturer’s instructions.
Statistical analysis
Demographic data of donors were analyzed for statistical significance using either unpaired t-test with Welch’s correction or Fisher’s exact test. Flow cytometry data and individual cytokine values for uninfected and convalescent COVID-19 (unpaired) specimens were analyzed for statistical significance using Mann-Whitney tests. Flow cytometry data and individual cytokine values for untreated and LPS-treated (paired) specimens were analyzed for statistical significance using Wilcoxon rank tests. The level of significance was determined as p < 0.05. Statistical analyses and graphic presentation were performed using Prism 9.01 software (GraphPad Software, Inc., San Diego, California).
Results
Study population description
Peripheral blood specimens were obtained from uninfected individuals and from convalescent patients who exhibited a mild form of the disease. Specimens were collected between May 2020 and January 2021, during the initial SARS-CoV-2 outbreak in the United States. The median age of the participants was 36 years (range 19-65) for uninfected subjects (n=31) and 42.5 years (range 19-71) for convalescent COVID-19 subjects (n=18). None of the participants had received a COVID vaccine at the time of sample collection. All individuals from the convalescent COVID-19 population had mild symptoms, including fever, headache, rhinorrhea, sore throat, cough, fatigue, and/or myalgias, which did not require medical intervention or hospitalization during illness. Their symptoms resolved within two weeks of disease onset and specimens were collected approximately two to four weeks after disease onset. The convalescent patients and uninfected donors reported no symptoms at the time of sample collection. Participant characteristics, including self-reported co-morbidities, are listed in Table 1.
The proportion of circulating monocytes is decreased in convalescent COVID-19 patients
To determine the effect of COVID-19 infection on blood monocytes during the convalescent stage of disease, peripheral blood mononuclear cells were isolated from convalescent COVID-19 patients (n=18) and uninfected controls (n=31) and were analyzed by flow cytometry. The gating strategy for identifying total monocytes, total lymphocytes, myeloid dendritic cells (mDCs), and plasmacytoid dendritic cells (pDCs) is shown in Supplementary Figure S1. We found that the percentage of total circulating monocytes (relative to total PBMCs) was lower in convalescent COVID-19 patients (23.7%) compared to uninfected controls (38.5%) (Figure 1A). Conversely, the percentage of lymphocytes was increased in the convalescent population (70% in COVID-19 patients vs. 54.5% in controls) (Figure 1B). There was no significant difference in the percentages of mDCs or pDCs between the two populations (Figures 1C, D). The increase in lymphocytes in the convalescent population was driven by an increase in T cells, without a significant difference in the percentages of B cells between the two populations (Figure 2). There was a slight, but significant, decrease in the percentage of NK cells in convalescent COVID-19 patients (Figure 2C). These findings are consistent with prior studies demonstrating a decrease in circulating monocytes during convalescent COVID-19 infection (25, 26).
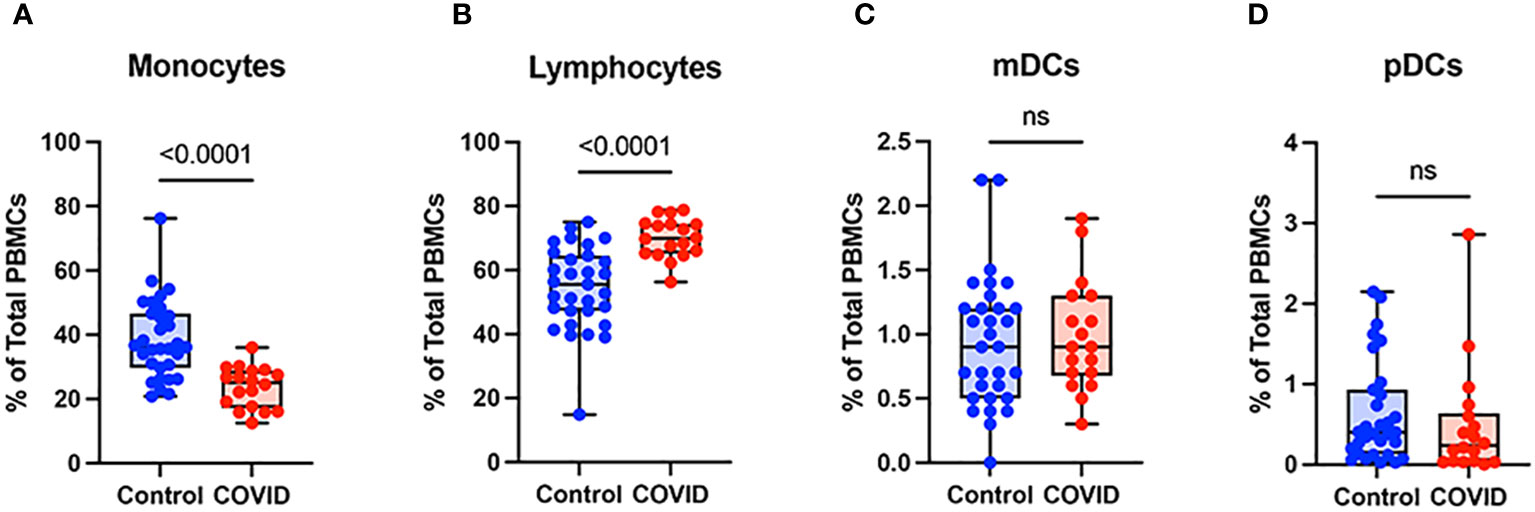
Figure 1 Monocytes are decreased and lymphocytes increased in convalescent COVID-19 patients. (A) Total monocyte percentage, (B) Total lymphocyte percentage, (C) Myeloid dendritic cell percentage, and (D) Plasmacytoid dendritic cell percentage (of PBMCs) in convalescent COVID-19 (red, n=18) and control (blue, n=31) groups. Mann-Whitney U test. ns, not significant.
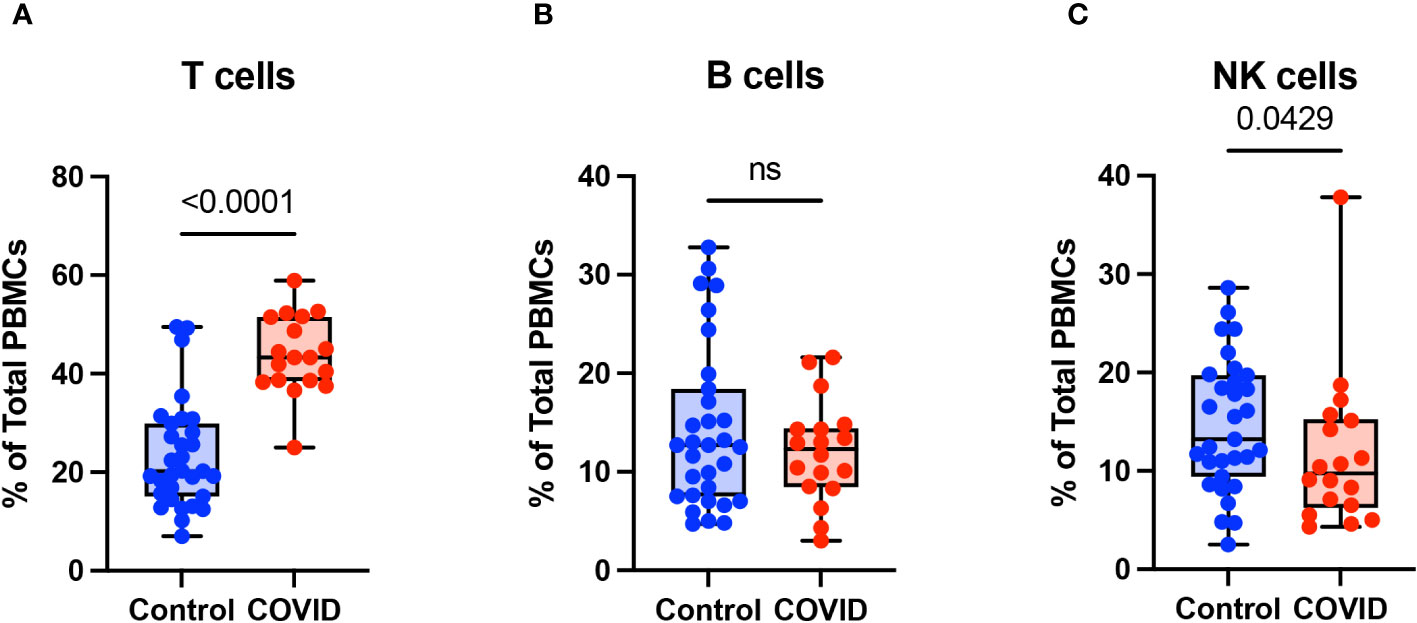
Figure 2 T cells are increased in convalescent COVID-19 patients. (A) T cell percentage, (B) B cell percentage, and (C) NK cell percentage (of PBMCs) in convalescent COVID-19 (red, n=18) and control (blue, n=31) groups. Mann-Whitney U test. ns, not significant.
Monocyte subsets including classical monocytes (CD14+ CD16-), intermediate monocytes (CD14+ CD16+), and non-classical (CD14lo CD16+) were defined by CD14 and CD16 expression within the total monocyte population (Supplementary Figure S1). Whereas the number of classical monocytes was unchanged between the uninfected and convalescent groups (Figure 3A), the percentage of intermediate and non-classical monocytes were significantly decreased in convalescent patients compared to uninfected donors (2.8% vs. 3.9% and 1.5% vs. 2.6%, respectively) (Figures 3B, C). Together, these observations suggest that circulating monocyte percentages decrease in convalescent patients, with significant decreases in intermediate and non-classical monocytes.
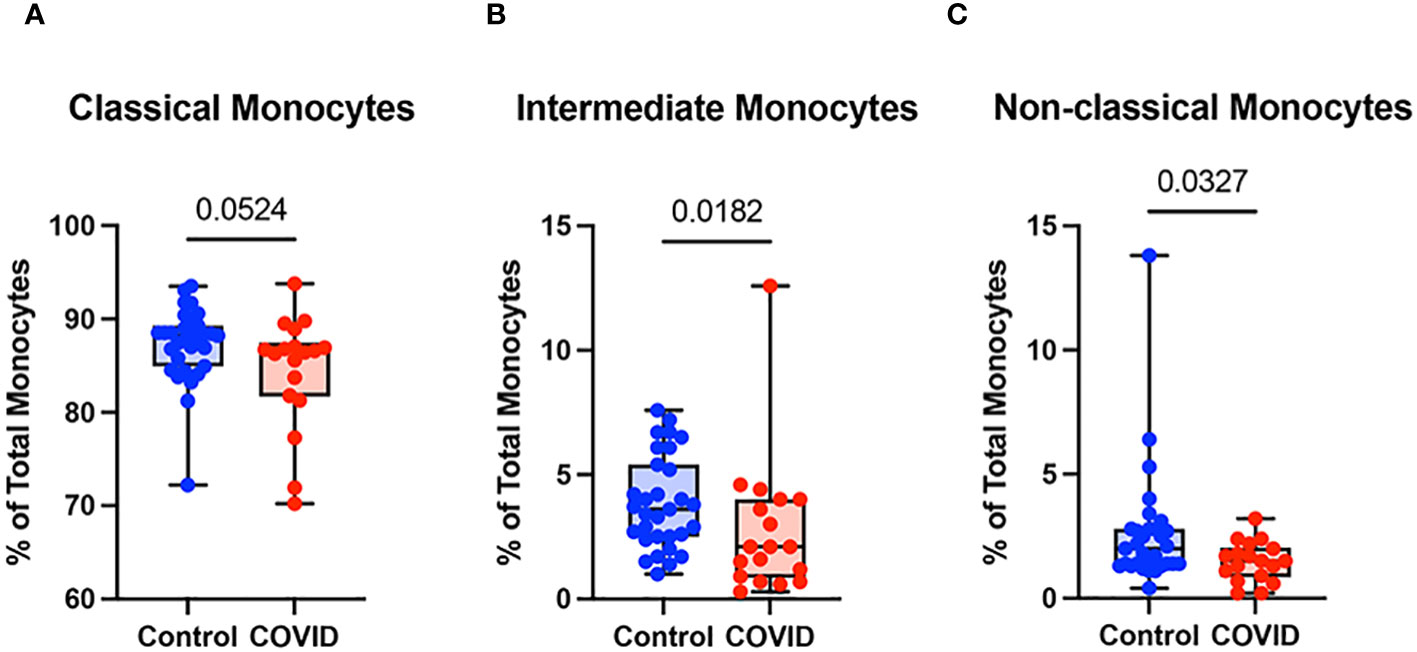
Figure 3 Classical (CD14+ CD16-) monocytes from convalescent COVID-19 patients express less CD142 (tissue factor) in response to LPS. (A) CD142 percentage (of classical monocytes) in convalescent COVID-19 (red, n=18) and control (blue, n=31) groups in the absence (empty) or presence (filled) of 100 ng/mL LPS. (B) CD142 percentage (of intermediate monocytes) in convalescent COVID-19 (blue) and control (red) groups in the absence (empty) or presence (filled) of 100 ng/mL LPS. (C) CD142 percentage (of non-classical monocytes) in convalescent COVID-19 (blue) and control (red) groups in the absence (empty) or presence (filled) of 100 ng/mL LPS. Comparison between groups (control vs. COVID-19) Mann-Whitney U test. Comparison within groups (untreated vs. LPS-treated) Wilcoxon ranked test. ns, not significant.
Expression of monocyte activation markers is altered in convalescent COVID-19 patients
To further investigate differences in monocyte activation between the study populations, we assessed the expression of a number of activation markers including: CD4, a glycoprotein expressed at low levels on the surface of monocytes which may stimulate cytokine expression and differentiation to macrophages (39) and which is downregulated in response to LPS stimulation (40, 41); CD56, an adhesion molecule that is expressed at high levels on monocytes in inflammatory conditions (42–44), including severe COVID-19 infection (45, 46); CD69, a type II C-lectin receptor upregulated on monocytes in response to stimulation (47); CD83, a transmembrane protein involved in the regulation of immune responses (48); and CD86, a co-stimulatory molecule expressed in monocytes whose expression can be altered by LPS treatment (49). We chose to activate monocytes with E. coli LPS, which is considered an initiator of classical activation in monocytes and leads to the production of proinflammatory cytokines and the generation of other immune mediators such as nitric oxide (reviewed in reference 50). The gating strategy for identifying total monocytes and monocyte subsets for the examination of activation markers is shown in Supplementary Figure S2.
When comparing classical monocytes from convalescent COVID-19 patients (n=18) and uninfected controls (n=31), we found no significant differences in the percentages of cells expressing bright (T-cell intensity) CD4, CD56, CD69, CD83, or CD86 in the absence of LPS stimulation (Figure 4). The expression patterns of these markers observed in response to LPS stimulation in these two populations were more complex. In response to stimulation with LPS, we found a statistically significant increase in the percentage of classical monocytes expressing CD56 and CD83, a significant decrease in the percentage of classical monocytes expressing CD69 and CD86, and no change in the percentage of classical monocytes expressing bright CD4 in uninfected control subjects (Figure 4). In contrast, in convalescent COVID-19 patients, LPS treatment led to a statistically significant increase in the percentage of classical monocytes expressing bright (T-cell intensity) CD4 and CD83, a decrease in the percentage of classical monocytes expressing CD86, and no change in the percentage of classical monocytes expressing CD56 or CD69 from convalescent COVID-19 patients in response to LPS (Figure 4). When directly comparing LPS-stimulated classical monocytes from convalescent COVID-19 patients to those from uninfected subjects, there was a statistically significant increase in the percentage of cells expressing bright CD4 and CD69, a significant decrease in the percentage of cells expressing CD56, and no difference in the percentage of cells expressing CD83 or CD86. Taken together, these findings suggest that classical monocytes from convalescent COVID-19 patients are hyporesponsive to stimulation with LPS.
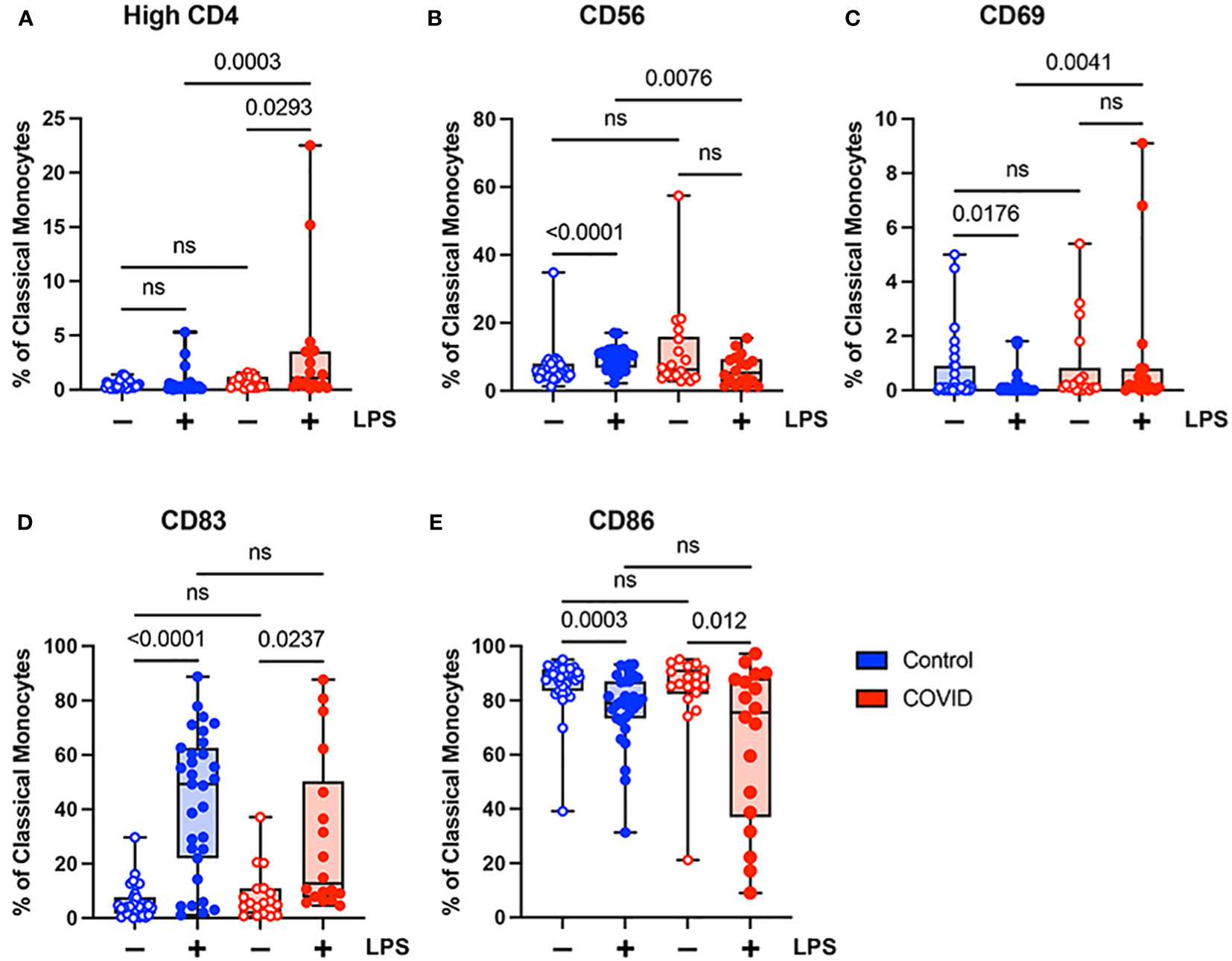
Figure 4 Expression of activation markers is altered in classical (CD14+ CD16-) monocytes from convalescent COVID-19 patients. (A) CD4 percentage, (B) CD56 percentage, (C) CD69 percentage, (D) CD83 percentage, and (E) CD86 percentage (of classical monocytes) in convalescent COVID-19 (red, n=18) and control (blue, n=31) groups in the absence (empty) or presence (filled) of 100 ng/mL LPS. Comparison between groups (control vs. COVID-19) Mann-Whitney U test. Comparison within groups (untreated vs. LPS-treated) Wilcoxon ranked test. ns, not significant.
Unlike classical monocytes, which showed a complex pattern of activation marker expression, intermediate monocytes demonstrated uniformly decreased expression of bright CD4, CD56, CD69, CD83, and CD86 in response to stimulation with LPS (Figure 5). This was true for both convalescent and uninfected subjects. In addition, there were no significant differences in any of the activation markers tested when comparing intermediate monocytes from convalescent and uninfected populations, either at baseline or with LPS stimulation (Figure 5). The differences in activation marker expression between classical and intermediate monocytes in response to LPS stimulation is somewhat surprising given that both monocyte populations express CD14, which is necessary for binding LPS (51). These observed differences may result from differential expression of other components of LPS signaling, such as MD2 and TLR4, or downstream effector molecules.
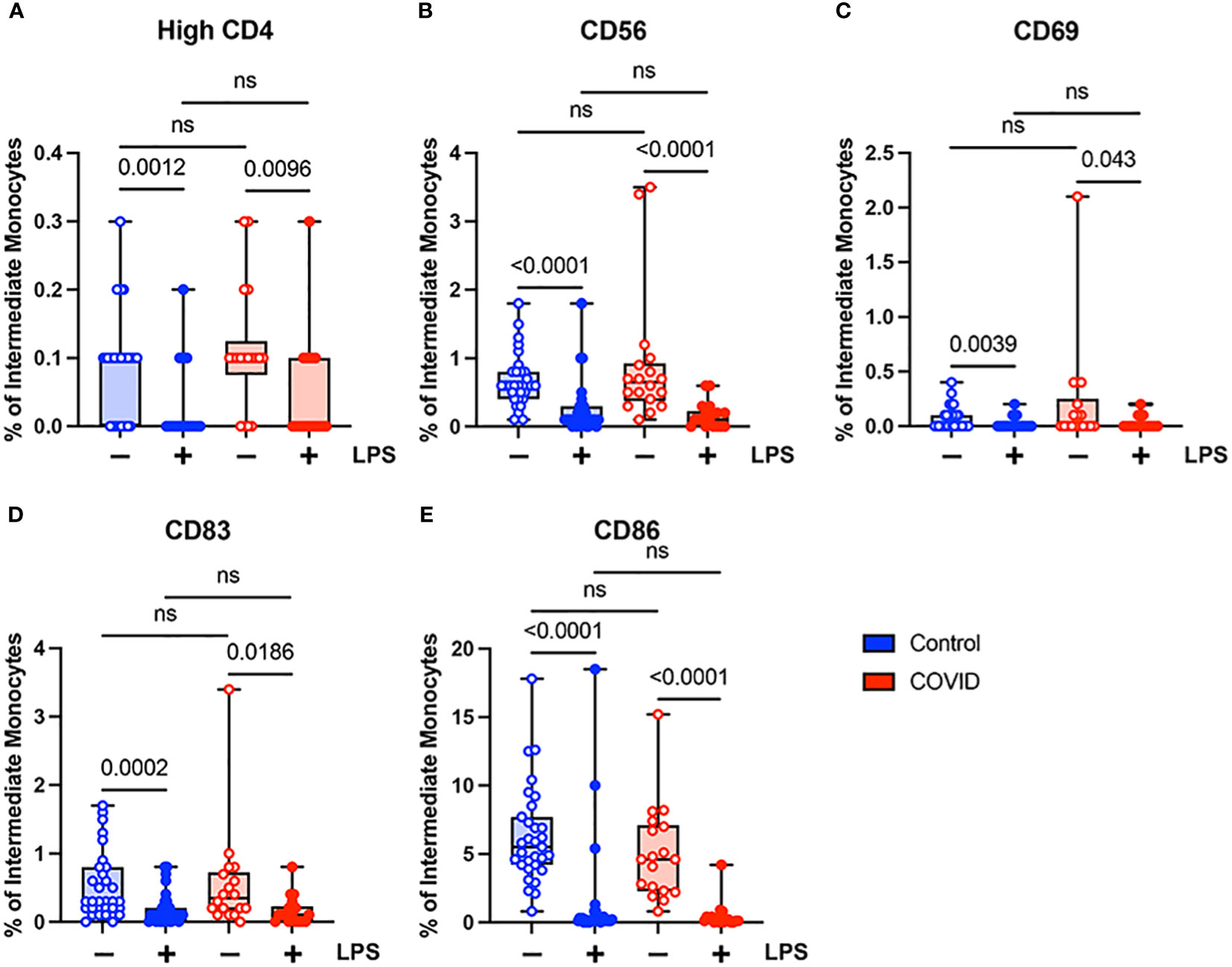
Figure 5 Activation marker expression is not altered in intermediate (CD14+ CD16+) monocytes from convalescent COVID-19 patients. (A) CD4 percentage, (B) CD56 percentage, (C) CD69 percentage, (D) CD83 percentage, and (E) CD86 percentage (of intermediate monocytes) in convalescent COVID-19 (red, n=18) and control (blue, n=31) groups in the absence (empty) or presence (filled) of 100 ng/mL LPS. Comparison between groups (control vs. COVID-19) Mann-Whitney U test. Comparison within groups (untreated vs. LPS-treated) Wilcoxon ranked test. ns, not significant.
In contrast to classical and intermediate monocytes, which showed no difference in baseline expression of CD56, a higher percentage of non-classical monocytes from convalescent patients expressed CD56 compared to those from uninfected subjects in the absence of LPS stimulation (Supplementary Figure S3B). This was the only significant difference in non-classical monocytes between the two study populations. Non-classical monocytes from both convalescent COVID-19 patients and uninfected controls demonstrated decreased CD56 and CD86 expression in response to LPS treatment (Supplementary Figures S3Bb, S3E). In addition, the percentage of non-classical monocytes expressing bright CD4 from uninfected control subjects decreased in response to LPS (Supplementary Figure S3A) and the percentage of non-classical monocytes expressing CD83 from convalescent patients decreased in response to LPS treatment (Supplementary Figure S3D) The muted response of non-classical monocytes to LPS stimulation is not entirely surprising given that, by definition, these cells express low levels of surface CD14; however, it is possible that the stimulatory effects of LPS could be potentiated by soluble CD14 present in the total monocyte culture.
In addition, we found that LPS stimulation leads to changes in the relative proportions of classical, intermediate, and non-classical monocytes in both healthy and COVID donors. We see a significant decrease in the percentages of intermediate and non-classical monocytes with a concurrent increase in the percentage of classical monocytes in both cohorts (Supplementary Figure S4). This may reflect a transition from one state to another or perhaps decreased viability of intermediate and non-classical monocytes. It is unlikely to reflect the preferential replication of classical monocytes, given that the treatment period with LPS is relatively short (2-3 hours) and that the percentage of monocytes (of total PBMCs) did not change significantly in response to LPS. These findings are similar to those published by other groups who demonstrated a relative increase in classical monocytes post-treatment with LPS in an experimental model of human endotoxemia (52–54).
Expression of CD142 (tissue factor) is decreased in convalescent COVID-19 patients in response to LPS stimulation
The extrinsic coagulation cascade can be triggered by soluble or cell-surface CD142 (tissue factor). Prior studies have demonstrated increased CD142 expression in the setting of viral infections (55–58) and in response to LPS (55, 59). In addition, there is a correlation between higher levels of CD142 and COVID-19 disease severity (57, 58). Given the role of coagulopathy in COVID-19 pathogenesis, we wanted to evaluate the expression of CD142 by the various monocyte subsets in our uninfected (n=31) and convalescent COVID-19 (n=18) populations. We found that although convalescent subjects had a higher percentage of classical monocytes expressing CD142 compared to uninfected subjects (mean 0.68% vs. 0.32%, p=0.0064) at baseline, they had a significantly lower percentage of CD142-expressing classical monocytes in response to LPS treatment (7.2% vs. 10.1%) (Figure 6A). This was expected given our findings demonstrating decreased activation marker expression in classical monocytes from convalescent COVID-19 patients in response to LPS stimulation (Figures 4B, C). No differences in CD142 expression were observed between intermediate monocytes or non-classical monocytes from convalescent COVID-19 patients and uninfected controls (Figures 6B, C). Similar to our findings with other activation markers, this suggests that classical monocytes from convalescent subjects are less responsive to LPS stimulation.
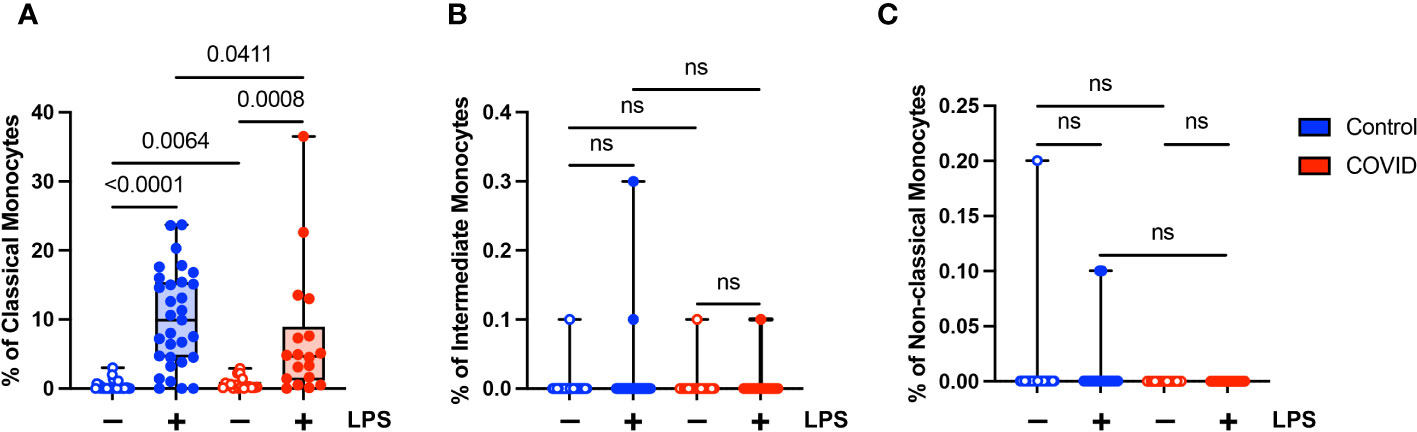
Figure 6 Classical (CD14+ CD16-) monocytes from convalescent COVID-19 patients express less CD142 (tissue factor) in response to LPS. (A) CD142 percentage (of classical monocytes) in convalescent COVID-19 (red, n=18) and control (blue, n=31) groups in the absence (empty) or presence (filled) of 100 ng/mL LPS. (B) CD142 percentage (of intermediate monocytes) in convalescent COVID-19 (blue) and control (red) groups in the absence (empty) or presence (filled) of 100 ng/mL LPS. (C) CD142 percentage (of non-classical monocytes) in convalescent COVID-19 (blue) and control (red) groups in the absence (empty) or presence (filled) of 100 ng/mL LPS. Comparison between groups (control vs. COVID-19) Mann-Whitney U test. Comparison within groups (untreated vs. LPS-treated) Wilcoxon ranked test. ns, not significant.
Expression of proinflammatory cytokines is decreased in convalescent COVID-19 patients
Finally, we wanted to determine whether there were functional differences between monocytes in convalescent COVID-19 patients (n=10) and uninfected controls (n=10). It has been shown previously that monocytes from acutely infected patients produce higher levels of proinflammatory cytokines and type I IFNs (14–18). Similarly, monocytes in convalescent individuals have been shown to express increased levels of inflammatory genes (21–24). There have been conflicting reports as to whether long COVID is associated with increased levels of proinflammatory cytokines (33–36) or whether it is characterized by cytokine deficiencies (37). Given these disparate study results, as well as our findings suggesting a hyporesponsive monocyte phenotype in convalescent COVID-19 patients, we wanted to determine whether monocytes from convalescent COVID-19 patients were capable of producing proinflammatory cytokines in response to stimuli. We treated purified total monocytes from uninfected and convalescent COVID-19 subjects with LPS and measured TNF-α and IL-6 production by ELISA. We found that monocytes from convalescent COVID-19 patients expressed significantly lower levels of both TNF-α (Figure 7A) and IL-6 (Figure 7B) in response to LPS stimulation, consistent with our findings regarding the expression of activation markers (Figure 4) and cellular CD142 (Figure 6). In addition, unstimulated monocytes from convalescent patients expressed less IL-6 than those from uninfected controls. Taken together, our findings suggest that monocytes from convalescent COVID-19 patients are hyporesponsive to LPS stimulation.
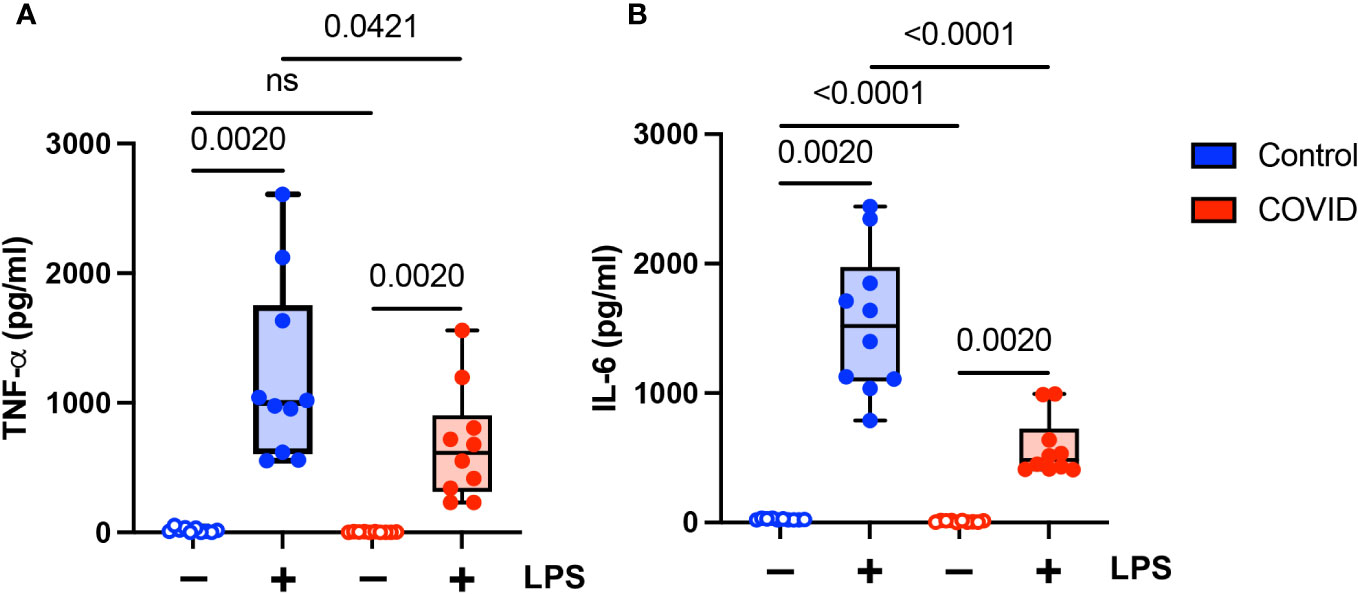
Figure 7 Proinflammatory cytokine expression is decreased in monocytes from convalescent COVID-19 patients. (A) TNF-α and (B) IL-6 expression in convalescent COVID-19 (red, n=10) and control (blue, n=10) groups in the absence (empty) or presence (filled) of 100 ng/mL LPS. Comparison between groups (control vs. COVID-19) Mann-Whitney U test. Comparison within groups (untreated vs. LPS-treated) Wilcoxon ranked test. ns, not significant.
Discussion
Several studies examining peripheral blood from COVID-19 patients demonstrated a complex immune response in which myeloid cells play a central role (60, 61). The contributions of monocytes to disease pathogenesis varies depending upon the stage of infection, and relatively little is known about their role during the convalescent stage of disease. We aimed to comprehensively analyze the phenotypic and functional changes of monocytes in convalescent COVID-19 patients with an eye toward the potential implications for disease progression.
We show that the percentage of total monocytes is decreased in COVID-19 patients, with significant decreases in intermediate and non-classical monocytes. These results contrast sharply with a previously published study by Park et al. that demonstrated increased circulating total monocytes and increased classical and intermediate monocytes (21). The differences between our study and the previously published study by Park et al. may be due to differences in the study populations (primarily Caucasian vs. primarily Asian/Pacific Islander), timing (2-4 weeks post resolution of symptoms vs. >30 days after infection), vaccination status (not vaccinated vs. predominantly vaccinated), or possibly viral strain. One limitation of the present study is that it compares samples from a single time point during the convalescent phase of COVID-19 infection, two to four weeks after the resolution of symptoms. It is certainly possible that this study may not capture changes that would become evident in a more comprehensive longitudinal study that follows patients throughout the acute to convalescent phases of disease.
Samples were collected during the early phase of the COVID-19 pandemic and, therefore, likely represent a primary exposure to the initial strain (wild-type or lineage A) of SARS-CoV-2 present in the United States. Our findings suggest that the convalescent phase of infection with wild-type SARS-CoV-2 is associated with decreased monocytes, increased lymphocytes, and a hyporesponsive monocyte phenotype characterized by decreased expression of activation markers and proinflammatory cytokines in response to stimulation. The relevance of these findings to subsequent infections with SARS-CoV-2 variants of concern, such as Alpha, Beta, Gamma, Delta, and Omicron, is unknown. There are several differences in the humoral, cellular, and innate immune responses to these variants of concern (reviewed in (62). For example, the SARS-CoV-2 Alpha variant evades innate immune detection better than prior SARS-CoV-2 isolates, due at least in part to increased expression of ORF9B (63). Given these differences, it is possible that there are differences in monocyte function during the convalescent phase of disease. It will be important to determine whether the observed changes in monocyte frequency and function following infection with wild-type SARS-CoV-2 infection are _following infection with other SARS-CoV-2 variants.
Circulating monocytes are comprised of three phenotypically and functionally distinct subsets: classical monocytes, intermediate monocytes, and non-classical (64, 65). Classical monocytes account for about 80–95% of circulating monocytes. These cells are highly phagocytic, and play major roles in innate immune responses, antibody-dependent cellular cytotoxicity (ADCC), and coagulation. Intermediate monocytes account for 2–10% of circulating monocytes. These cells are important producers of reactive oxygen species (ROS), in addition to processing and presenting antigen for T-cell stimulation. Non-classical monocytes account for about 2–10% of circulating monocytes. They are primarily responsible for clearance of debris and apoptotic cells, but also secrete inflammatory cytokines. Due to their varying abilities to phagocytose, process and present antigen, and produce proinflammatory cytokines, all three monocyte subtypes play a role during viral infection (65, 66). However, non-classical monocytes are thought to play a primary role during the antiviral response due to their expression of pattern recognition receptors that recognize viral nuclei acids (67). We found that there were significant decreases in the relative levels of intermediate and non-classical monocytes in convalescent COVID-19 patients. Given their purported functions, their relative decrease may be associated with decreased T-cell activation and/or decreased proinflammatory cytokine production in convalescent patients.
In addition to finding that the percentage of monocytes was decreased in convalescent patients, we demonstrated that they were hyporesponsive to LPS stimulation. Classical monocytes from convalescent COVID-19 patients demonstrate significantly lower levels of CD56 expression in response to LPS stimulation compared to uninfected controls. This finding was not observed in intermediate or classical monocytes. In addition, classical monocytes from convalescent patients show no change in CD69 expression in response to LPS stimulation. Furthermore, monocytes from convalescent patients express significantly less TNF-α and IL-6 when treated with LPS. These findings suggest that, as patients transition from acute to convalescent stages of disease, monocytes shift towards a hyporesponsive phenotype.
The biological consequences of these changes in the frequencies of monocyte subtypes and monocyte function are unclear. It is possible that this shift to a hyporesponsive phenotype may play a role in dampening the inflammatory response and promoting tissue repair. Tolerogenic myeloid cells, in particular mDCs, have been shown to suppress inflammation in other settings by means of decreased T-cell activation (68). Recent studies have also demonstrated immune exhaustion in murine monocytes following stimulation (69, 70). These exhausted monocytes may play a role in immune suppression following inflammatory conditions (69), though data in human monocytes is lacking. Our studies did not delve into the mechanism(s) by which classical monocytes become hyporesponsive in the setting of convalescent COVID-19. Studies of monocytes and macrophages that become hyporesponsive in the setting of repeated LPS stimulation, exposure to commensal microbes, and systemic inflammation suggest roles for anti-inflammatory cytokines (71), the downregulation of receptors such as TLR4 (72, 73), epigenetic changes (74), and/or altered intracellular signaling cascades (75). It is possible that SARS-CoV-2 acts in a similar manner to promote a hyporesponsive phenotype in monocytes. The hyporesponsive nature of monocytes in convalescent COVID-19 patients may play a deleterious role in the immune response to subsequent bacterial infections. COVID-19 patients at increased risk for bacterial co-infections and superinfections, which are associated with adverse outcomes (76, 77). Interestingly, Smith et al. showed that there is a time-dependent increase in disease severity in a murine model of SARS-CoV-2 and bacterial superinfection (78). It is possible that this observed increase in disease severity correlates with decreased monocyte function as there was no increase in monocyte or macrophage recruitment to the lungs of mice co-infected with SARS-CoV-2 and pneumococcus compared to mice infected with SARS-CoV-2 alone. Finally, our findings in convalescent patients are similar to those reported in a cohort of long COVID patients (37). In that study, PBMCs from long COVID patients produced lower levels of proinflammatory cytokines compared to uninfected controls. It should be noted that other studies of long COVID patients have demonstrated a dysregulated immune response characterized by increased inflammation (33–36). Despite these differences, our findings reinforce the notion that the immune response is dysregulated following SARS-CoV-2 infection and that this dysregulation may contribute to long COVID.
Consistent with prior publications (52–54), we found that stimulation with LPS led to changes in the relative proportions of classical, intermediate, and non-classical monocytes, with a decrease in the percentages of intermediate and non-classical monocytes and an increase in the percentage of classical monocytes (Supplementary Figure S4). This shift in the relative proportions of the monocyte subsets occurred in both healthy donors and convalescent COVID-19 patients. It has been proposed that intermediate monocytes may represent a transition state between non-classical and classical monocytes (79). If this is true, our findings suggest that LPS treatment may promote the transition from intermediate monocytes to classical monocytes, rather than to non-classical monocytes as is proposed to occur under steady state conditions (54). Additional considerations for the observed effects of LPS include decreased viability of intermediate and non-classical monocytes.
There is a correlation between expression of cell surface (57) and extracellular, vesicular (58) CD142 (tissue factor) and COVID-19 disease severity. Interestingly, in a study by Stephenson et al., circulating monocytes displaying the highest levels of ISG expression were found to express ligands and receptors for platelets (80). This may explain the increased propensity for coagulopathy in acutely ill COVID-19 patients. We found a significant decrease in classical monocytes expressing CD142 in response to LPS stimulation in convalescent patients compared to uninfected subjects. This finding is consistent with our data showing that classical monocytes from convalescent COVID-19 patients demonstrate significantly lower levels of CD56 and proinflammatory cytokines in response to LPS stimulation.
Our results indicate that there is a relative decrease in monocytes in convalescent COVID-19 patients following mild disease compared to control, uninfected subjects. Intermediate and non-classical monocytes are decreased in COVID-19 patients with no significant difference in classical monocytes. Furthermore, classical monocytes from COVID-19 patients demonstrate decreased CD56 expression and decreased CD142 expression in response to LPS stimulation compared to control subjects. Finally, monocytes from convalescent COVID-19 patients demonstrate significantly decreased TNF-α and IL-6 expression in response to LPS stimulation compared to control subjects. Taken together, these findings suggest that SARS-CoV-2 infection exhibits a clear effect on both monocyte subset development and the response to physiological stimuli, as reflected in their relative numbers, activation state, and function, which lasts into the convalescent phase of disease.
Data availability statement
The raw data supporting the conclusions of this article will be made available by the authors, without undue reservation.
Ethics statement
The studies involving humans were approved by the University of Utah Institutional Review Board. The studies were conducted in accordance with local legislation and institutional requirements. The participants provided their written informed consent to participate in this study.
Author contributions
ER: Conceptualization, Data curation, Formal analysis, Investigation, Methodology, Writing – original draft, Writing – review & editing. EW: Conceptualization, Data curation, Writing – review & editing, Funding acquisition. ME: Conceptualization, Writing – review & editing, Funding acquisition. AB: Conceptualization, Writing – review & editing. VP: Conceptualization, Funding acquisition, Writing – review & editing. AS: Conceptualization, Data curation, Writing – review & editing. JD: Conceptualization, Funding acquisition, Writing – review & editing. LL: Conceptualization, Writing – review & editing. TH: Conceptualization, Data curation, Formal analysis, Funding acquisition, Investigation, Methodology, Project administration, Supervision, Writing – original draft, Writing – review & editing.
Funding
The author(s) declare financial support was received for the research, authorship, and/or publication of this article. This work was supported by funding from ARUP Laboratories Institute for Clinical and Experimental Pathology, the Department of Pathology at the Spencer Fox Eccles School of Medicine, University of Utah (TH), the University of Utah Inflammation, Immunology, and Infectious Disease (3i) Initiative’s Emerging Infectious Diseases Fellowship (ESCPW), a seed grant from the University of Utah Vice President for Research and the Immunology, Inflammation, and Infectious Disease (3i) Initiative (VP), and NIH GRANT 5R01-AI143567 (VP).
Conflict of interest
The authors declare that the research was conducted in the absence of any commercial or financial relationships that could be construed as a potential conflict of interest.
Publisher’s note
All claims expressed in this article are solely those of the authors and do not necessarily represent those of their affiliated organizations, or those of the publisher, the editors and the reviewers. Any product that may be evaluated in this article, or claim that may be made by its manufacturer, is not guaranteed or endorsed by the publisher.
Supplementary material
The Supplementary Material for this article can be found online at: https://www.frontiersin.org/articles/10.3389/fimmu.2023.1329026/full#supplementary-material
References
1. Harrison AG, Lin T, Wang P. Mechanisms of sars-cov-2 transmission and pathogenesis. Trends Immunol (2020) 41(12):1100–15. doi: 10.1016/j.it.2020.10.004
2. Lamers MM, Haagmans BL. Sars-cov-2 pathogenesis. Nat Rev Microbiol (2022) 20(5):270–84. doi: 10.1038/s41579-022-00713-0
3. Vanderbeke L, Van Mol P, Van Herck Y, De Smet F, Humblet-Baron S, Martinod K, et al. Monocyte-driven atypical cytokine storm and aberrant neutrophil activation as key mediators of covid-19 disease severity. Nat Commun (2021) 12(1):4117. doi: 10.1038/s41467-021-24360-w
4. Guo C, Li B, Ma H, Wang X, Cai P, Yu Q, et al. Single-cell analysis of two severe covid-19 patients reveals a monocyte-associated and tocilizumab-responding cytokine storm. Nat Commun (2020) 11(1):3924. doi: 10.1038/s41467-020-17834-w
5. Subramaniam S, Kothari H, Bosmann M. Tissue factor in covid-19-associated coagulopathy. Thromb Res (2022) 220:35–47. doi: 10.1016/j.thromres.2022.09.025
6. Conway EM, Mackman N, Warren RQ, Wolberg AS, Mosnier LO, Campbell RA, et al. Understanding covid-19-associated coagulopathy. Nat Rev Immunol (2022) 22(10):639–49. doi: 10.1038/s41577-022-00762-9
7. Paliogiannis P, Zinellu A, Scano V, Mulas G, De Riu G, Pascale RM, et al. Laboratory test alterations in patients with covid-19 and non covid-19 interstitial pneumonia: A preliminary report. J Infect Dev Ctries (2020) 14(7):685–90. doi: 10.3855/jidc.12879
8. Neeland MR, Bannister S, Clifford V, Dohle K, Mulholland K, Sutton P, et al. Innate cell profiles during the acute and convalescent phase of sars-cov-2 infection in children. Nat Commun (2021) 12(1):1084. doi: 10.1038/s41467-021-21414-x
9. Schulte-Schrepping J, Reusch N, Paclik D, Bassler K, Schlickeiser S, Zhang B, et al. Severe covid-19 is marked by a dysregulated myeloid cell compartment. Cell (2020) 182(6):1419–40 e23. doi: 10.1016/j.cell.2020.08.001
10. Wilk AJ, Lee MJ, Wei B, Parks B, Pi R, Martinez-Colon GJ, et al. Multi-omic profiling reveals widespread dysregulation of innate immunity and hematopoiesis in covid-19. J Exp Med (2021) 218(8). doi: 10.1084/jem.20210582
11. Silvin A, Chapuis N, Dunsmore G, Goubet AG, Dubuisson A, Derosa L, et al. Elevated calprotectin and abnormal myeloid cell subsets discriminate severe from mild covid-19. Cell (2020) 182(6):1401–18 e18. doi: 10.1016/j.cell.2020.08.002
12. Su Y, Chen D, Yuan D, Lausted C, Choi J, Dai CL, et al. Multi-omics resolves a sharp disease-state shift between mild and moderate covid-19. Cell (2020) 183(6):1479–95 e20. doi: 10.1016/j.cell.2020.10.037
13. Lombardi A, Trombetta E, Cattaneo A, Castelli V, Palomba E, Tirone M, et al. Early phases of covid-19 are characterized by a reduction in lymphocyte populations and the presence of atypical monocytes. Front Immunol (2020) 11:560330. doi: 10.3389/fimmu.2020.560330
14. Melms JC, Biermann J, Huang H, Wang Y, Nair A, Tagore S, et al. A molecular single-cell lung atlas of lethal covid-19. Nature (2021) 595(7865):114–9. doi: 10.1038/s41586-021-03569-1
15. Delorey TM, Ziegler CGK, Heimberg G, Normand R, Yang Y, Segerstolpe A, et al. Covid-19 tissue atlases reveal sars-cov-2 pathology and cellular targets. Nature (2021) 595(7865):107–13. doi: 10.1038/s41586-021-03570-8
16. Lee JS, Park S, Jeong HW, Ahn JY, Choi SJ, Lee H, et al. Immunophenotyping of covid-19 and influenza highlights the role of type I interferons in development of severe covid-19. Sci Immunol (2020) 5(49). doi: 10.1126/sciimmunol.abd1554
17. Combes AJ, Courau T, Kuhn NF, Hu KH, Ray A, Chen WS, et al. Global absence and targeting of protective immune states in severe covid-19. Nature (2021) 591(7848):124–30. doi: 10.1038/s41586-021-03234-7
18. Li Z, Chen X, Dan J, Hu T, Hu Y, Liu S, et al. Innate immune imprints in sars-cov-2 omicron variant infection convalescents. Signal Transduct Target Ther (2022) 7(1):377. doi: 10.1038/s41392-022-01237-y
19. Rajamanickam A, Kumar NP, Pandiarajan AN, Selvaraj N, Munisankar S, Renji RM, et al. Dynamic alterations in monocyte numbers, subset frequencies and activation markers in acute and convalescent covid-19 individuals. Sci Rep (2021) 11(1):20254. doi: 10.1038/s41598-021-99705-y
20. Zhang B, Zhang Z, Koeken V, Kumar S, Aillaud M, Tsay HC, et al. Altered and allele-specific open chromatin landscape reveals epigenetic and genetic regulators of innate immunity in covid-19. Cell Genom (2023) 3(2):100232. doi: 10.1016/j.xgen.2022.100232
21. Park J, Dean LS, Jiyarom B, Gangcuangco LM, Shah P, Awamura T, et al. Elevated circulating monocytes and monocyte activation in covid-19 convalescent individuals. Front Immunol (2023) 14:1151780. doi: 10.3389/fimmu.2023.1151780
22. Theobald SJ, Simonis A, Georgomanolis T, Kreer C, Zehner M, Eisfeld HS, et al. Long-lived macrophage reprogramming drives spike protein-mediated inflammasome activation in covid-19. EMBO Mol Med (2021) 13(8):e14150. doi: 10.15252/emmm.202114150
23. Singh R, Hemati H, Bajpai M, Yadav P, Maheshwari A, Kumar S, et al. Sustained expression of inflammatory monocytes and activated T cells in covid-19 patients and recovered convalescent plasma donors. Immun Inflamm Dis (2021) 9(4):1279–90. doi: 10.1002/iid3.476
24. Wen W, Su W, Tang H, Le W, Zhang X, Zheng Y, et al. Immune cell profiling of covid-19 patients in the recovery stage by single-cell sequencing. Cell Discov (2020) 6:31. doi: 10.1038/s41421-020-0168-9
25. Hoffmann AD, Weinberg SE, Swaminathan S, Chaudhuri S, Mubarak HF, Schipma MJ, et al. Unique molecular signatures sustained in circulating monocytes and regulatory T cells in convalescent covid-19 patients. Clin Immunol (2023), 252:109634. doi: 10.1016/j.clim.2023.109634
26. Chu CF, Sabath F, Fibi-Smetana S, Sun S, Ollinger R, Noessner E, et al. Convalescent Covid-19 Patients without Comorbidities Display Similar Immunophenotypes over Time Despite Divergent Disease Severities. Front Immunol (2021) 12:601080. doi: 10.3389/fimmu.2021.601080
27. Bumbea V, Ardelean L, Radulescu L, Damian L, Bumbea H, Dumitru I, et al. Proinflammatory role of monocytes in sars-cov-2 infection in chronic hemodialysis patients. Front Immunol (2023) 14:1210961. doi: 10.3389/fimmu.2023.1210961
28. Lopez-Leon S, Wegman-Ostrosky T, Ayuzo Del Valle NC, Perelman C, Sepulveda R, Rebolledo PA, et al. Long-covid in children and adolescents: A systematic review and meta-analyses. Sci Rep (2022) 12(1):9950. doi: 10.1038/s41598-022-13495-5
29. Fernandez-de-Las-Penas C, Palacios-Cena D, Gomez-Mayordomo V, Cuadrado ML, Florencio LL. Defining post-covid symptoms (Post-acute covid, long covid, persistent post-covid): an integrative classification. Int J Environ Res Public Health (2021) 18(5). doi: 10.3390/ijerph18052621
30. Nabavi N. Long covid: how to define it and how to manage it. BMJ (2020) 370:m3489. doi: 10.1136/bmj.m3489
31. Peluso MJ, Donatelli J, Henrich TJ. Long-term immunologic effects of sars-cov-2 infection: leveraging translational research methodology to address emerging questions. Transl Res (2022) 241:1–12. doi: 10.1016/j.trsl.2021.11.006
32. Merad M, Blish CA, Sallusto F, Iwasaki A. The immunology and immunopathology of covid-19. Science (2022) 375(6585):1122–7. doi: 10.1126/science.abm8108
33. Peluso MJ, Lu S, Tang AF, Durstenfeld MS, Ho HE, Goldberg SA, et al. Markers of immune activation and inflammation in individuals with postacute sequelae of severe acute respiratory syndrome coronavirus 2 infection. J Infect Dis (2021) 224(11):1839–48. doi: 10.1093/infdis/jiab490
34. Schultheiss C, Willscher E, Paschold L, Gottschick C, Klee B, Henkes SS, et al. The il-1beta, il-6, and tnf cytokine triad is associated with post-acute sequelae of covid-19. Cell Rep Med (2022) 3(6):100663. doi: 10.1016/j.xcrm.2022.100663
35. Bellan M, Apostolo D, Albe A, Crevola M, Errica N, Ratano G, et al. Determinants of long covid among adults hospitalized for sars-cov-2 infection: A prospective cohort study. Front Immunol (2022) 13:1038227. doi: 10.3389/fimmu.2022.1038227
36. Phetsouphanh C, Darley DR, Wilson DB, Howe A, Munier CML, Patel SK, et al. Immunological dysfunction persists for 8 months following initial mild-to-moderate sars-cov-2 infection. Nat Immunol (2022) 23(2):210–6. doi: 10.1038/s41590-021-01113-x
37. Williams ES, Martins TB, Shah KS, Hill HR, Coiras M, Spivak AM, et al. Cytokine deficiencies in patients with long-covid. J Clin Cell Immunol (2022) 13(6). doi: 10.35248/2155-9899.22.13.672
38. Rychert J, Couturier MR, Elgort M, Lozier BK, La’ulu S, Genzen JR, et al. Evaluation of 3 sars-cov-2 igg antibody assays and correlation with neutralizing antibodies. J Appl Lab Med (2021) 6(3):614–24. doi: 10.1093/jalm/jfaa188
39. Zhen A, Krutzik SR, Levin BR, Kasparian S, Zack JA, Kitchen SG. Cd4 ligation on human blood monocytes triggers macrophage differentiation and enhances hiv infection. J Virol (2014) 88(17):9934–46. doi: 10.1128/JVI.00616-14
40. Neate EV, Greenhalgh AM, McPhee DA, Crowe SM. Bacterial lipopolysaccharide mediates the loss of cd4 from the surface of purified peripheral blood monocytes. Clin Exp Immunol (1992) 90(3):539–44. doi: 10.1111/j.1365-2249.1992.tb05879.x
41. Herbein G, Doyle AG, Montaner LJ, Gordon S. Lipopolysaccharide (Lps) down-regulates cd4 expression in primary human macrophages through induction of endogenous tumour necrosis factor (Tnf) and il-1 beta. Clin Exp Immunol (1995) 102(2):430–7. doi: 10.1111/j.1365-2249.1995.tb03801.x
42. Sconocchia G, Keyvanfar K, El Ouriaghli F, Grube M, Rezvani K, Fujiwara H, et al. Phenotype and function of a cd56+ Peripheral blood monocyte. Leukemia (2005) 19(1):69–76. doi: 10.1038/sj.leu.2403550
43. Grip O, Bredberg A, Lindgren S, Henriksson G. Increased subpopulations of cd16(+) and cd56(+) blood monocytes in patients with active crohn’s disease. Inflamm Bowel Dis (2007) 13(5):566–72. doi: 10.1002/ibd.20025
44. Krasselt M, Baerwald C, Wagner U, Rossol M. Cd56+ Monocytes have a dysregulated cytokine response to lipopolysaccharide and accumulate in rheumatoid arthritis and immunosenescence. Arthritis Res Ther (2013) 15(5):R139. doi: 10.1186/ar4321
45. Dutt TS, LaVergne SM, Webb TL, Baxter BA, Stromberg S, McFann K, et al. Comprehensive immune profiling reveals cd56(+) monocytes and cd31(+) endothelial cells are increased in severe covid-19 disease. J Immunol (2022) 208(3):685–96. doi: 10.4049/jimmunol.2100830
46. Campana S, De Pasquale C, Sidoti Migliore G, Pezzino G, Cavaliere R, Venanzi Rullo E, et al. Cutting edge: hyperinflammatory monocytes expressing cd56 abound in severe covid-19 patients. J Immunol (2022) 209(4):655–9. doi: 10.4049/jimmunol.2200021
47. Ziegler SF, Ramsdell F, Alderson MR. The activation antigen cd69. Stem Cells (1994) 12(5):456–65. doi: 10.1002/stem.5530120502
48. Grosche L, Knippertz I, Konig C, Royzman D, Wild AB, Zinser E, et al. The cd83 molecule - an important immune checkpoint. Front Immunol (2020) 11:721. doi: 10.3389/fimmu.2020.00721
49. Vishnyakova P, Poltavets A, Karpulevich E, Maznina A, Vtorushina V, Mikhaleva L, et al. The response of two polar monocyte subsets to inflammation. BioMed Pharmacother (2021) 139:111614. doi: 10.1016/j.biopha.2021.111614
50. Rossol M, Heine H, Meusch U, Quandt D, Klein C, Sweet MJ, et al. Lps-induced cytokine production in human monocytes and macrophages. Crit Rev Immunol (2011) 31(5):379–446. doi: 10.1615/critrevimmunol.v31.i5.20
51. Dobrovolskaia MA, Vogel SN. Toll receptors, cd14, and macrophage activation and deactivation by lps. Microbes Infect (2002) 4(9):903–14. doi: 10.1016/s1286-4579(02)01613-1
52. Thaler B, Hohensinner PJ, Krychtiuk KA, Matzneller P, Koller L, Brekalo M, et al. Differential in vivo activation of monocyte subsets during low-grade inflammation through experimental endotoxemia in humans. Sci Rep (2016) 6:30162. doi: 10.1038/srep30162
53. Tak T, van Groenendael R, Pickkers P, Koenderman L. Monocyte subsets are differentially lost from the circulation during acute inflammation induced by human experimental endotoxemia. J Innate Immun (2017) 9(5):464–74. doi: 10.1159/000475665
54. Patel AA, Zhang Y, Fullerton JN, Boelen L, Rongvaux A, Maini AA, et al. The fate and lifespan of human monocyte subsets in Steady state and systemic inflammation. J Exp Med (2017) 214(7):1913–23. doi: 10.1084/jem.20170355
55. Funderburg NT, Mayne E, Sieg SF, Asaad R, Jiang W, Kalinowska M, et al. Increased tissue factor expression on circulating monocytes in chronic hiv infection: relationship to in vivo coagulation and immune activation. Blood (2010) 115(2):161–7. doi: 10.1182/blood-2009-03-210179
56. Schechter ME, Andrade BB, He T, Richter GH, Tosh KW, Policicchio BB, et al. Inflammatory monocytes expressing tissue factor drive siv and hiv coagulopathy. Sci Transl Med (2017) 9(405). doi: 10.1126/scitranslmed.aam5441
57. Hottz ED, Azevedo-Quintanilha IG, Palhinha L, Teixeira L, Barreto EA, Pao CRR, et al. Platelet activation and platelet-monocyte aggregate formation trigger tissue factor expression in patients with severe covid-19. Blood (2020) 136(11):1330–41. doi: 10.1182/blood.2020007252
58. Burrello J, Caporali E, Gauthier LG, Pianezzi E, Balbi C, Rigamonti E, et al. Risk stratification of patients with sars-cov-2 by tissue factor expression in circulating extracellular vesicles. Vascul Pharmacol (2022) 145:106999. doi: 10.1016/j.vph.2022.106999
59. Holstein K, Matysiak A, Witt L, Sievers B, Beckmann L, Haddad M, et al. Lps-induced expression and release of monocyte tissue factor in patients with haemophilia. Ann Hematol (2020) 99(7):1531–42. doi: 10.1007/s00277-020-04075-6
60. Knoll R, Schultze JL, Schulte-Schrepping J. Monocytes and macrophages in covid-19. Front Immunol (2021) 12:720109. doi: 10.3389/fimmu.2021.720109
61. Grant RA, Morales-Nebreda L, Markov NS, Swaminathan S, Querrey M, Guzman ER, et al. Circuits between infected macrophages and T cells in sars-cov-2 pneumonia. Nature (2021) 590(7847):635–41. doi: 10.1038/s41586-020-03148-w
62. Torbati E, Krause KL, Ussher JE. The immune response to sars-cov-2 and variants of concern. Viruses (2021) 13(10). doi: 10.3390/v13101911
63. Thorne LG, Bouhaddou M, Reuschl AK, Zuliani-Alvarez L, Polacco B, Pelin A, et al. Evolution of enhanced innate immune evasion by sars-cov-2. Nature (2022) 602(7897):487–95. doi: 10.1038/s41586-021-04352-y
64. Sampath P, Moideen K, Ranganathan UD, Bethunaickan R. Monocyte subsets: phenotypes and function in tuberculosis infection. Front Immunol (2018) 9:1726. doi: 10.3389/fimmu.2018.01726
65. Kapellos TS, Bonaguro L, Gemund I, Reusch N, Saglam A, Hinkley ER, et al. Human monocyte subsets and phenotypes in major chronic inflammatory diseases. Front Immunol (2019) 10:2035. doi: 10.3389/fimmu.2019.02035
66. Ziegler-Heitbrock L. The cd14+ Cd16+ Blood monocytes: their role in infection and inflammation. J Leukoc Biol (2007) 81(3):584–92. doi: 10.1189/jlb.0806510
67. Cros J, Cagnard N, Woollard K, Patey N, Zhang SY, Senechal B, et al. Human cd14dim monocytes patrol and sense nucleic acids and viruses via tlr7 and tlr8 receptors. Immunity (2010) 33(3):375–86. doi: 10.1016/j.immuni.2010.08.012
68. Marin E, Bouchet-Delbos L, Renoult O, Louvet C, Nerriere-Daguin V, Managh AJ, et al. Human tolerogenic dendritic cells regulate immune responses through lactate synthesis. Cell Metab (2019) 30(6):1075–90 e8. doi: 10.1016/j.cmet.2019.11.011
69. Pradhan K, Yi Z, Geng S, Li L. Development of exhausted memory monocytes and underlying mechanisms. Front Immunol (2021) 12:778830. doi: 10.3389/fimmu.2021.778830
70. Naler LB, Hsieh YP, Geng S, Zhou Z, Li L, Lu C. Epigenomic and transcriptomic analyses reveal differences between low-grade inflammation and severe exhaustion in lps-challenged murine monocytes. Commun Biol (2022) 5(1):102. doi: 10.1038/s42003-022-03035-2
71. Ueda Y, Kayama H, Jeon SG, Kusu T, Isaka Y, Rakugi H, et al. Commensal microbiota induce lps hyporesponsiveness in colonic macrophages via the production of il-10. Int Immunol (2010) 22(12):953–62. doi: 10.1093/intimm/dxq449
72. Bosisio D, Polentarutti N, Sironi M, Bernasconi S, Miyake K, Webb GR, et al. Stimulation of toll-like receptor 4 expression in human mononuclear phagocytes by interferon-gamma: A molecular basis for priming and synergism with bacterial lipopolysaccharide. Blood (2002) 99(9):3427–31. doi: 10.1182/blood.v99.9.3427
73. Skinner NA, MacIsaac CM, Hamilton JA, Visvanathan K. Regulation of toll-like receptor (Tlr)2 and tlr4 on cd14dimcd16+ Monocytes in response to sepsis-related antigens. Clin Exp Immunol (2005) 141(2):270–8. doi: 10.1111/j.1365-2249.2005.02839.x
74. El Gazzar M, Yoza BK, Hu JY, Cousart SL, McCall CE. Epigenetic silencing of tumor necrosis factor alpha during endotoxin tolerance. J Biol Chem (2007) 282(37):26857–64. doi: 10.1074/jbc.M704584200
75. Freise N, Burghard A, Ortkras T, Daber N, Imam Chasan A, Jauch SL, et al. Signaling mechanisms inducing hyporesponsiveness of phagocytes during systemic inflammation. Blood (2019) 134(2):134–46. doi: 10.1182/blood.2019000320
76. Musuuza JS, Watson L, Parmasad V, Putman-Buehler N, Christensen L, Safdar N. Prevalence and outcomes of co-infection and superinfection with sars-cov-2 and other pathogens: A systematic review and meta-analysis. PloS One (2021) 16(5):e0251170. doi: 10.1371/journal.pone.0251170
77. Shafran N, Shafran I, Ben-Zvi H, Sofer S, Sheena L, Krause I, et al. Secondary bacterial infection in covid-19 patients is a stronger predictor for death compared to influenza patients. Sci Rep (2021) 11(1):12703. doi: 10.1038/s41598-021-92220-0
78. Smith AP, Williams EP, Plunkett TR, Selvaraj M, Lane LC, Zalduondo L, et al. Time-dependent increase in susceptibility and severity of secondary bacterial infections during sars-cov-2. Front Immunol (2022) 13:894534. doi: 10.3389/fimmu.2022.894534
79. Cormican S, Griffin MD. Human monocyte subset distinctions and function: insights from gene expression analysis. Front Immunol (2020) 11:1070. doi: 10.3389/fimmu.2020.01070
Keywords: monocytes, COVID-19, SARS-CoV-2, tissue factor, TNF-α, IL-6 69, 70
Citation: Ravkov EV, Williams ESCP, Elgort M, Barker AP, Planelles V, Spivak AM, Delgado JC, Lin L and Hanley TM (2024) Reduced monocyte proportions and responsiveness in convalescent COVID-19 patients. Front. Immunol. 14:1329026. doi: 10.3389/fimmu.2023.1329026
Received: 27 October 2023; Accepted: 06 December 2023;
Published: 04 January 2024.
Edited by:
Tanushree Dangi, Northwestern University, United StatesReviewed by:
Marta Bermejo Jambrina, Innsbruck Medical University, AustriaGüliz Tuba Barut, Institute of Virology and Immunology, Switzerland
Copyright © 2024 Ravkov, Williams, Elgort, Barker, Planelles, Spivak, Delgado, Lin and Hanley. This is an open-access article distributed under the terms of the Creative Commons Attribution License (CC BY). The use, distribution or reproduction in other forums is permitted, provided the original author(s) and the copyright owner(s) are credited and that the original publication in this journal is cited, in accordance with accepted academic practice. No use, distribution or reproduction is permitted which does not comply with these terms.
*Correspondence: Timothy M. Hanley, timothy.hanley@hsc.utah.edu