- 1Center for Immunology and Inflammatory Diseases, Massachusetts General Hospital, Boston, MA, United States
- 2Division of Rheumatology, Allergy, and Immunology, Massachusetts General Hospital, Boston, MA, United States
- 3Harvard Medical School, Boston, MA, United States
- 4Center for Vaccine Innovation, La Jolla Institute for Immunology, La Jolla, CA, United States
- 5Ragon Institute of MGH, MIT, and Harvard, Cambridge, MA, United States
- 6Program in Health Sciences and Technology, Harvard Medical School and Massachusetts Institute of Technology, Boston, MA, United States
- 7Endocrine Division, MGH, Boston, MA, United States
- 8Division of Gastroenterology, MGH, Boston, MA, United States
- 9Department of Medicine, University of California San Diego, La Jolla, CA, United States
- 10Division of Pulmonary and Critical Care Medicine, MGH, Boston, MA, United States
Understanding adaptive immunity against SARS-CoV-2 is a major requisite for the development of effective vaccines and treatments for COVID-19. CD4+ T cells play an integral role in this process primarily by generating antiviral cytokines and providing help to antibody-producing B cells. To empower detailed studies of SARS-CoV-2-specific CD4+ T cell responses in mouse models, we comprehensively mapped I-Ab-restricted epitopes for the spike and nucleocapsid proteins of the BA.1 variant of concern via IFNγ ELISpot assay. This was followed by the generation of corresponding peptide:MHCII tetramer reagents to directly stain epitope-specific T cells. Using this rigorous validation strategy, we identified 6 immunogenic epitopes in spike and 3 in nucleocapsid, all of which are conserved in the ancestral Wuhan strain. We also validated a previously identified epitope from Wuhan that is absent in BA.1. These epitopes and tetramers will be invaluable tools for SARS-CoV-2 antigen-specific CD4+ T cell studies in mice.
Introduction
Severe acute respiratory syndrome coronavirus 2 (SARS-CoV-2) is the causative pathogen of the coronavirus disease 2019 (COVID-19) pandemic that has become an epic global health crisis (1). The unprecedented effort to develop vaccines and treatments for this disease has put forth an urgency to better understand how adaptive immunity develops to the virus.
As with most other viral pathogens, effective immunity to SARS-CoV-2 involves a combination of humoral responses from B cells and antibodies as well as cellular responses from CD4+ and CD8+ T cells (2, 3). While the significance of virus-specific antibodies and particularly their neutralizing function in COVID-19 is unquestioned, there is growing appreciation for the parallel protective role provided by virus-specific T cells, due in part to their greater resistance to immune evasion by viral evolution and perhaps greater stability over time (4).
T cells recognize peptides processed from antigenic proteins and presented by major histocompatibility complex (MHC) proteins on the surface of antigen-presenting cells (APCs). Identification of the sequences of such peptide epitopes from SARS-CoV-2 proteins enables detailed analyses of SARS-CoV-2-specific T cell populations by informing the design of in vitro stimulation assays as well as the generation of peptide:MHC tetramer reagents that can directly stain SARS-CoV-2-specific T cells. Considerable progress has been made in the identification of human T cell epitopes for SARS-CoV-2 antigens, resulting in the generation and use of SARS-CoV-2-specific peptide:MHC tetramers for studies of viral epitope-specific T cell responses (5). However, there are only a few reported sequences of mouse T cell epitopes that would benefit immunological studies in mouse models (6–11).
In this report, we performed a comprehensive mapping of CD4+ T cell epitopes in SARS-CoV-2 spike and nucleocapsid proteins for C57BL/6 (B6) mice, the most widely used strain for immunological studies. Overlapping peptide libraries for these proteins were screened for reactivity to T cells in immunized mice by interferon-γ (IFNγ) ELISpot assay. Peptide : MHCII tetramer reagents were then generated for candidate peptide epitopes and used to measure the expansion of T cell populations with specificity for these epitopes and compare their relative immunogenicity. In all, we identified 6 novel epitopes in spike and 3 in nucleocapsid, which will be useful for future studies of T cell responses to SARS-CoV-2 in mice.
Materials and methods
Mice
C57BL/6 mice were purchased from Jackson Laboratory and housed under specific-pathogen-free conditions at Massachusetts General Hospital. Male and female mice were immunized between 6-16 weeks of age.
Proteins
SARS-CoV-2 Wuhan-Hu-1 (D614G) or SARS-CoV-2 BA.1 spike was expressed in the “HexaPro” background (12) as previously reported (13, 14). Briefly, ExpiCHO-S cells (Thermo Fisher) were maintained and transfected according to the manufacturer’s protocol. Transfected cells were harvested 7-8 days after transfection. Cultures were clarified by centrifugation and BioLock (IBA Life Sciences) added. The Twin-StrepII-Tagged-proteins were purified over a StrepTrap-HP column equilibrated with 25mM Tris pH 7.5, 200mM NaCl (TBS). After extensive washing, bound proteins were eluted in TBS buffer supplemented with 5mM d-desthiobiotin (Sigma Aldrich). Affinity-purified proteins were incubated with HRV-3C protease to remove purification tags and subsequently purified by size-exclusion-chromatography on a Superose 6 Increase column (GE Healthcare) in TBS.
Alternatively, HexaPro spike proteins were expressed in Drosophila S2 cells as previously described for the production of peptide:MHCII tetramers (15). Briefly, the same sequences were cloned into the pR expression vector and expressed in stably transfected S2 cells. Protein was harvested from cell culture supernatants and purified by binding to a nickel-nitrilotriacetic acid (Ni-NTA) column (Novagen) followed by elution with 1M imidazole containing 0.2% octyl β-D-glucopyranoside. After concentration through 100kD centrifugation filters (Millipore), the protein was further purified by size-exclusion chromatography on a Sephacryl 300 column (GE Healthcare) in PBS.
For memory T cell experiments, the VFLIP prefusion stabilized version of SARS-CoV-2 Wuhan spike was used instead (16). This protein was generated in ExpiCHO-S cells as described above.
Recombinant SARS-CoV-2 BA.1 nucleocapsid protein was subcloned into the pET46 vector (Novagen) with an upstream 6xHis tag and expressed in Rosetta2 pLysS E. coli (Novagen). Cells were lysed with an M-110P microfluidizer (Microfluidics) in 50 mM HEPES pH 7.5, 500 mM NaCl, 10% glycerol, 20 mM imidazole, 6M urea, 1 μl Benzonase Nuclease (Millipore). Recombinant protein was purified by binding to Ni-NTA beads (Qiagen) and eluted in the same buffer supplemented with 300 mM imidazole. The resulting sample was then dialyzed overnight in Snakeskin dialysis tubing with 10-kDa pore size in refolding buffer (50 mM HEPES pH 7.5, 500 mM NaCl, 10% glycerol) and further purified by size-exclusion chromatography on a Superose 200 Increase column (GE Healthcare).
Peptides
Peptide libraries for BA.1 spike and nucleocapsid (15-mers with 4aa overlap) were synthesized at a purity of at least 70% by the MGH Peptide Core (17). All others were custom ordered through Genscript.
Immunizations
100 μg of recombinant protein or peptide was emulsified in a 1:1 mix of 0.9% saline and complete Freund’s adjuvant (CFA)(Sigma-Aldrich) and injected subcutaneously at the back of the neck and base of the tail (50 μl each site). For memory T cell studies, a priming dose of 10 μg of spike protein was mixed with 20 μg of polyinosinic:polycytidylic acid (poly(I:C))(InvivoGen) in 50 μl of 0.9% saline and injected intramuscularly in the back leg. A boost immunization was administered intranasally under anesthesia three weeks later using the same dose of spike protein in 30 μl of 0.9% saline.
T cells and APCs for ELISpot assays
Secondary lymphoid organs (SLO) including the spleen and various skin-draining lymph nodes (e.g., brachial, axillary, inguinal, mandibular, accessory mandibular, parotid, iliac, and mesenteric) (18), were harvested from mice 9-10 days post-immunization and processed into single cell suspensions by mechanical disruption over nylon mesh. CD4+ T cells were then isolated via a negative selection CD4+ T cell isolation kit (Miltenyi). For APCs, splenocytes were isolated from naïve mice, subjected to red cell lysis with ACK lysis buffer (Corning) at RT for 5 min, washed, and then irradiated at 2500 rads.
ELISpot assays
IFNγ ELISpot assays were performed using a commercially available kit in accordance with its instructions (Mabtech). Briefly, 2.5 x 105 CD4+ T cells were incubated with 2.5 x 105 irradiated APCs in 200 μl R10 media (RPMI 1640 + 10% FBS, pen/strep, 2-ME) in each well of a 96-well pre-coated ELISpot plate. Single peptides from the spike and nucleocapsid libraries were added to each well at a final concentration of 20 μg/ml. Positive control wells were set up with 10 μg/ml Concanavalin A (ConA)(Sigma-Aldrich) and negative control wells with no stimulus. After overnight (16-18h) incubation at 37C with 5% CO2, plates were washed and developed according to kit instructions. Dried plates were then analyzed on a Mabtech ASTOR ELISpot reader for quantification of spot-forming units (SFU), which were then expressed as SFU per 106 CD4+ T cells after subtraction of background signal determined by the average of negative control (cells + media alone) wells. Machine settings for spot size and brightness were preserved as best possible across experiments.
Tetramers
The generation of peptide:MHCII tetramers has been described in detail (15). In brief, soluble heterodimeric I-Ab molecules covalently linked to peptide epitopes were expressed and biotinylated in stably transfected Drosophila S2 cells. Following immunoaffinity purification, these biotinylated peptide:MHCII complexes were titrated and tetramerized to streptavidin pre-conjugated to R-phycoerythrin (PE), allophycocyanin (APC), or R-phycoerythrin-cyanine-7 (PE-Cy7) fluorochromes (Prozyme, Thermo-Fisher). All three fluorochrome versions were used in calculations of epitope-specific T cell frequencies.
Tetramer-based T cell enrichment and flow cytometry
SLO were harvested from mice 7-8 days post-immunization and processed into single cell suspensions by mechanical disruption over nylon mesh. For memory T cell studies, SLO also included lung draining mediastinal lymph nodes and were harvested 4 weeks post-boost. Tetramer-based enrichment of epitope-specific T cells from these samples was performed as described in detail (19). Flow cytometry was performed with the Aurora spectral flow cytometer (Cytek). In general, ~0.5 - 2.0 x 106 total enriched cells containing ~2000 - 8000 CD4+ T cells were collected per sample. Data analysis was performed with FlowJo software (Treestar).
Statistical analysis
All statistical analysis was performed on Prism (GraphPad).
Results
Screening of peptide libraries by IFNγ ELISpot
To facilitate detailed studies of SARS-CoV-2-specific CD4+ T cell responses in B6 mice, we performed a comprehensive screen of potential I-Ab-restricted epitopes in SARS-CoV-2 spike and nucleocapsid proteins, which have been shown to be major immunogenic targets of CD4+ T cells during infection in humans (20). The sequences used in our study were from the Omicron (BA.1) variant strain due to the timing of when our studies began.
B6 mice were immunized subcutaneously with recombinant spike or nucleocapsid protein emulsified in complete Freund’s adjuvant (CFA), and 7-10 days later, expanded CD4+ T cells were isolated from pooled secondary lymphoid organs (SLO) consisting of spleen and skin-draining lymph nodes. To identify epitopes, the T cells were restimulated in vitro with individual peptides from a library of overlapping peptides (15-mer with 4 residue overlap) covering the entire sequence of each protein. Epitope-specific responses were gauged by the production of interferon γ (IFNγ) via ELISpot assay (Figure 1 and Supplementary File 1). Based on an initial arbitrary threshold signal of ~20 spot-forming units (SFU) per million CD4+ T cells, a total of 13 regions representing putative epitopes were identified in spike, and 3 were identified in nucleocapsid (Table 1).
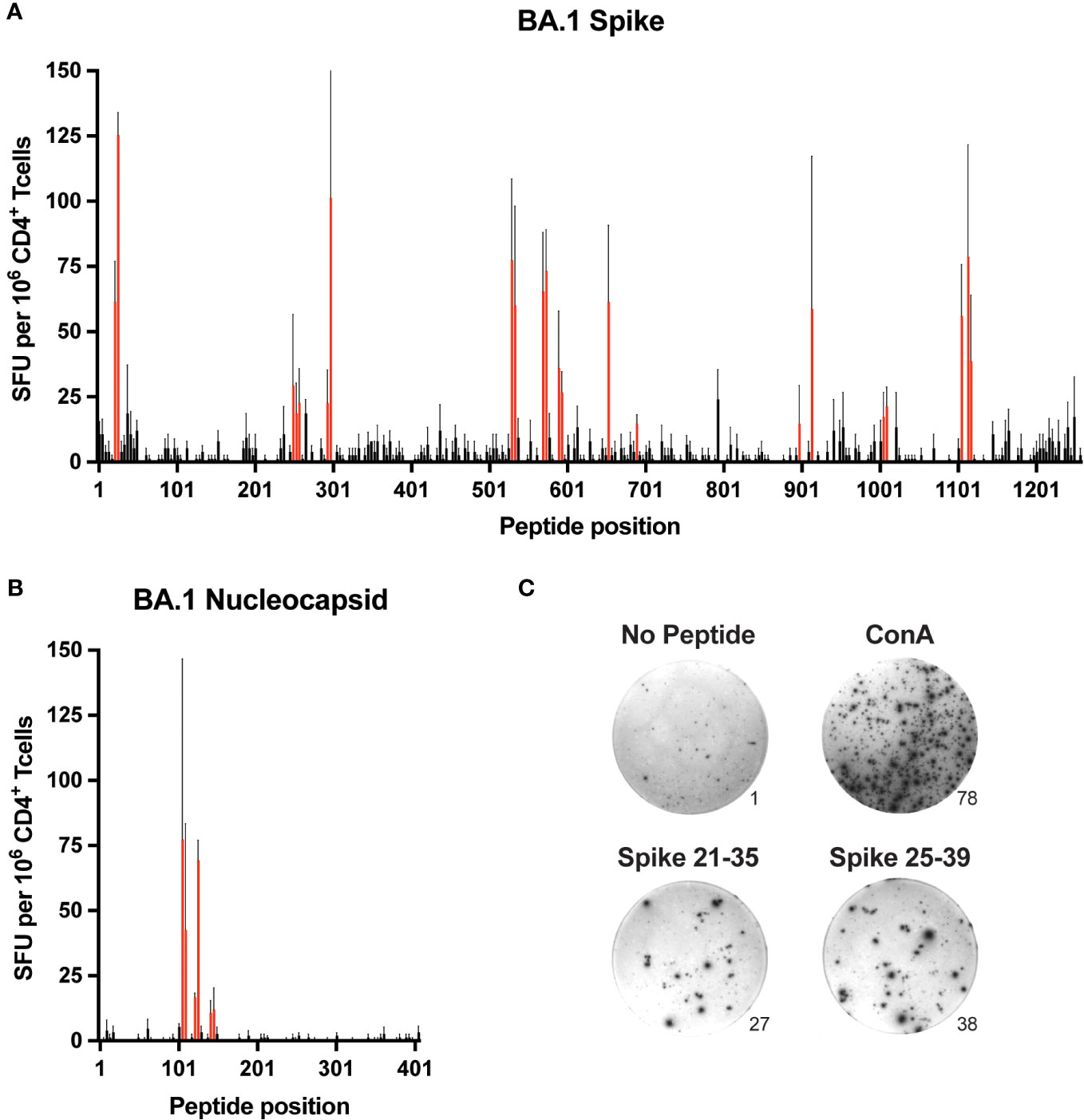
Figure 1 Comprehensive mapping of CD4+ T cell epitopes in SARS-CoV-2 BA.1 spike and nucleocapsid proteins C57BL/6 mice were immunized subcutaneously (s.c.) with BA.1 spike or nucleocapsid proteins plus CFA as adjuvant, and 9-10 days later, CD4+ T cells from secondary lymphoid organs (SLO) were assessed for IFNγ production by ELISpot following in vitro restimulation with peptide libraries covering the entire sequence of (A) spike and (B) nucleocapsid. Mean values ± SEM (thin black lines extending above bars) are shown for n=3 independent experiments. Red bars indicate putative epitopes that were subsequently evaluated by peptide:MHCII tetramer staining. (C) Representative images of ELISpot samples stimulated with PBS alone (No peptide, negative control), Concanavalin A (Con A, positive control), or overlapping peptides encompassing the S-26 epitope of spike (Spike 21-25 and Spike 25-39). Numbers at the lower right edges of images indicate the raw number of spot-forming units counted per well.
Tetramer-based validation of epitopes
To further validate these epitopes, we generated I-Ab tetramer reagents to directly stain epitope-specific CD4+ T cells. Tetramers were constructed with minimal peptide sequences (10-11 residues) representing each putative epitope as inferred from the overlap between neighboring immunogenic peptides as well as the use of computational tools that predict peptide binding registers in I-Ab (21, 22) (Table 1). In some cases where minimal epitope sequences were not obvious, multiple tetramers were generated to cover the best possibilities from each immunogenic region (data not shown).
Mice were immunized with each minimal peptide, and 7 days later the expansion of CD4+ T cells specific for that peptide was assessed by corresponding tetramer staining and magnetic cell enrichment (Figure 2). Six of the 13 spike epitopes (S-26, S-298, S-571, 6-690, S-1008, and S-1104) and all three of the nucleocapsid epitopes (N-107, N-125, N-143) exhibited immunogenicity based on observed increases in tetramer-positive T cell frequencies following immunization. Despite having excellent MHC-binding prediction scores, tetramers for spike epitopes S-592 and S-655 were consistently fraught with issues of high background staining and the immunogenicity of these epitopes could not be ascertained (data not shown).
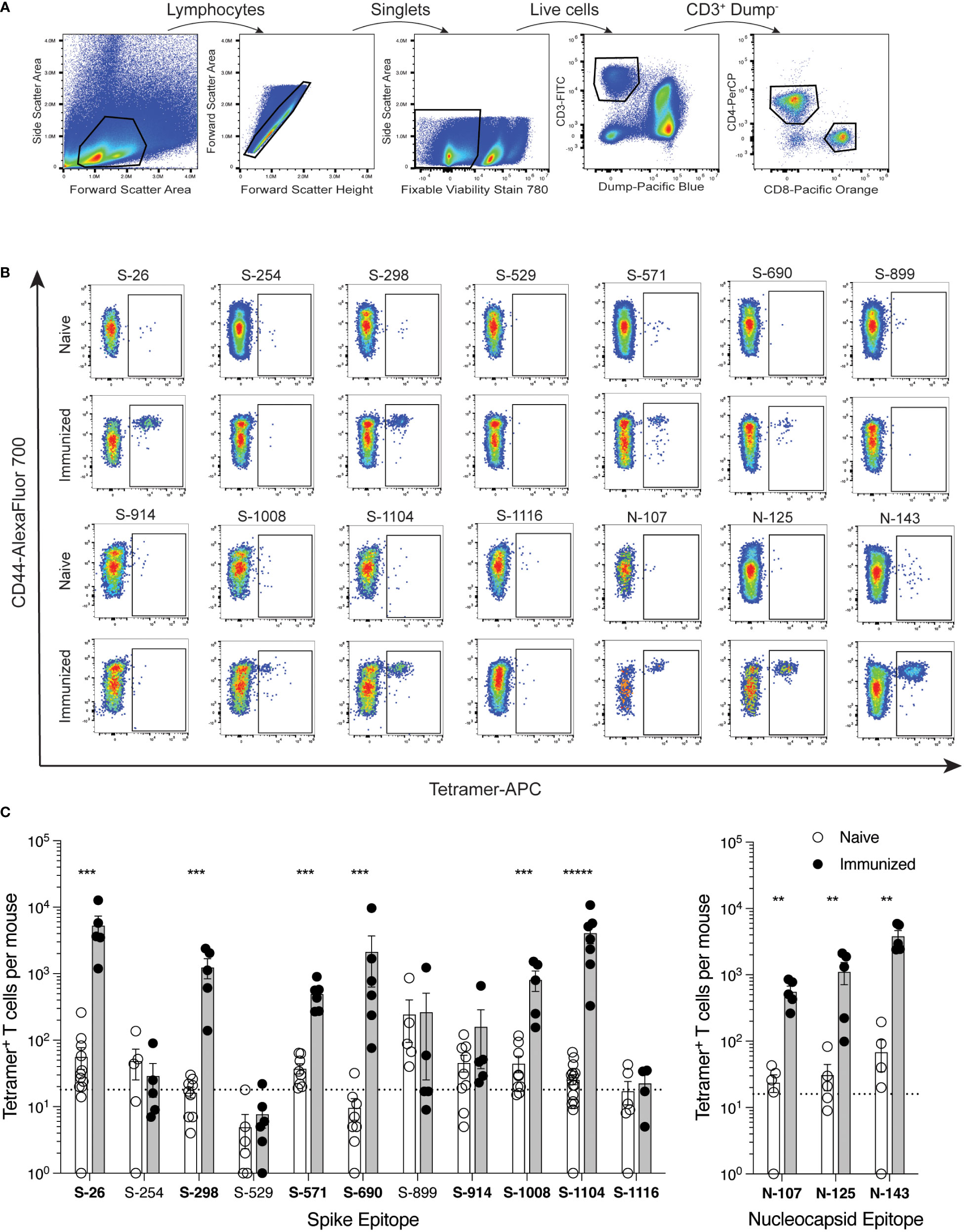
Figure 2 Identification of SARS-CoV-2 epitope-specific CD4+ T cells by peptide:MHCII tetramers C57BL/6 mice were immunized s.c. with candidate epitope peptides plus CFA as adjuvant, and 7 days later, epitope-specific CD4+ T cells from the SLO were detected by peptide:MHCII tetramer-based cell enrichment and flow cytometry. (A) Flow cytometry gating strategy for analysis of lymphocyte+ single cell+ live+ dump (B220, CD11b, CD11c, F4/80)− CD3+ CD4+ events. (B) Representative flow cytometry plots of CD4+ gated events illustrating tetramer staining of epitope-specific T cells from naïve and corresponding peptide-immunized mice. APC-conjugated tetramer staining is shown exclusively for comparison purposes, but all three fluorochrome versions were used to generate data. (C) Quantification of epitope-specific CD4+ T cells from naïve and immunized mice. Mean values ± SEM are shown for n=4-16 mice per epitope across multiple independent experiments. Dotted lines represent the limit of detection as defined by the mean numbers of CD8+tetramer+ events per mouse. Bold font indicates epitopes subsequently evaluated in protein immunization experiments. Statistical significance was calculated via Mann-Whitney tests (B, C); **p < 0.01, ***p < 0.001, *****p < 0.00001.
Peptides were chosen for these immunizations to provide maximal levels of peptide:MHCII complex presentation that could test whether the epitope was capable of evoking a T cell response. Once epitopes were validated in this way, tetramers were then used to detect epitope-specific T cells following immunization of mice with whole spike or nucleocapsid protein (Figure 3). This additional step addressed the immunogenicity of each epitope when the efficiency by which it is processed from protein and presented by antigen-presenting cells is also considered. Eight spike epitopes and 3 nucleocapsid epitopes were chosen for testing by protein immunization based on their initial promise of immunogenicity in early peptide immunization experiments, albeit only 6 of the spike epitopes and all 3 nucleocapsid epitopes were ultimately validated by peptide immunization as described above. With the exception of S-1008, all 9 of the epitopes that were validated by peptide immunizations also elicited a reliable response by protein immunization. Responses ranged from minimal to robust, and they reflected the same general hierarchy of epitope immunodominance seen in the peptide studies.
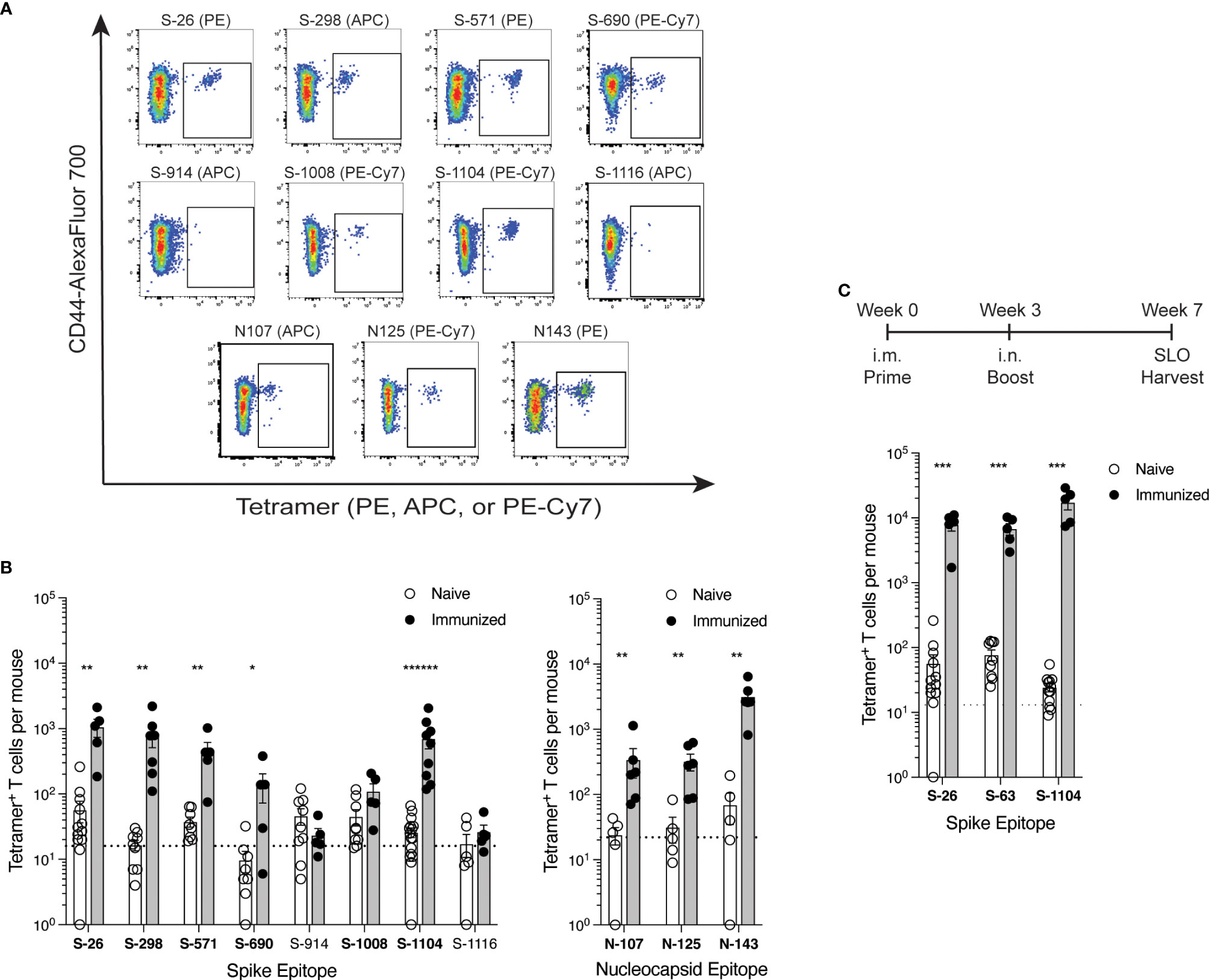
Figure 3 Comparison of SARS-CoV-2 epitope-specific CD4+ T cell responses after protein immunization (A, B) C57BL/6 mice were immunized s.c. with spike or nucleocapsid protein plus CFA as adjuvant, and 7 days later, epitope-specific CD4+ T cells from the SLO were detected by tetramer-based cell enrichment and flow cytometry. (A) Representative flow cytometry plots of CD4+ gated events illustrating tetramer staining of epitope-specific T cells from protein-immunized mice. (B) Quantification of epitope-specific CD4+ T cells from naïve and immunized mice. Naive mice data is the same as in Figure 2C. Mean values ± SEM are shown for n=5-16 mice per epitope across multiple independent experiments. Bold font indicates epitopes validated by statistically significant increases in frequency upon immunization. (C) C57BL/6 mice were primed intramuscularly (i.m.) with Wuhan spike protein plus poly (I:C) as adjuvant, and 3 weeks later, boosted intranasally (i.n.) with the same dose. Four weeks later, epitope-specific memory CD4+ T cells from the SLO were enumerated by tetramer-based cell enrichment and flow cytometry. Naive data is the same as in Figure 2C and Supplementary Figure S2. Mean values ± SEM are shown for n=5 mice per epitope. Dotted lines represent the limit of detection as defined by the mean numbers of CD8+tetramer+ events per mouse. Statistical significance was calculated via Mann-Whitney tests (B) and (C); *p < 0.05, **p < 0.01, ***p < 0.001, ******p < 0.000001.
Validation of additional epitopes
The spike protein used in our studies was the previously described HexaPro construct engineered to remain in a prefusion trimer complex due to the introduction of 6 stabilizing proline substitutions (12). To determine whether any potential epitopes were affected by these mutations, we immunized mice with peptides corresponding to native sequences at these locations and assessed T cell responses by IFNγ ELISpot (Supplementary Figure S1, Supplementary File 1). One of these peptides generated a weak response, which was supported by tetramer staining experiments showing a trend of expanded T cells in mice immunized with a minimal epitope sequence from this region (S-883, Table 1).
All of the identified SARS-CoV-2 BA.1 spike and nucleocapsid epitopes in our study are completely conserved from the ancestral Wuhan-Hu-1 strain. However, there are two previously reported epitopes in Wuhan, spike residues 62-78 and nucleocapsid residues 9-23 (10), that are mutated in BA.1 and therefore were not identified in our screen. To compare the immunogenicity of these epitopes to the new ones identified in our current study, and to find any other potential epitopes in Wuhan spike and nucleocapsid, we performed further ELISpot experiments using Wuhan spike and nucleocapsid proteins and a set of Wuhan peptides covering all the sites that are mutated in BA.1 (Supplementary Figures S2A, B, Supplementary File 1). Consistent with earlier reports, we found a very strong response to the spike 62-78 region and a very weak response to the nucleocapsid 9-23 region. We also generated a corresponding tetramer with a minimal sequence for the strong spike epitope (S-63, Table 1) and tested it in mice immunized with peptide or Wuhan spike protein (Supplementary Figures S2C, D). Responses to this epitope were very robust and superior to the responses for any of the newly identified epitopes.
Validation of epitopes in memory T cell populations
To further assess the relevance of these epitopes in T cell immunity to spike antigen, we used tetramers to detect the frequencies of epitope-specific T cells among memory CD4+ T cells generated after a 7-week prime-boost immunization scheme. Because subcutaneous immunization with CFA creates an antigen depot unsuitable for the study of memory T cells, we primed mice intramuscularly with a soluble dose of HexaPro Wuhan spike protein and poly(I:C) as adjuvant, and then three weeks later, boosted them intranasally with the same dose. At 4 weeks post-boost, we found robust frequencies of T cells specific for the newly identified S-26 and S-1104 epitopes in addition to the previously known S-63 epitope in the SLO (Figure 3C), demonstrating the contribution of these new epitopes in long term CD4+ T cell responses to SARS-CoV-2 spike.
Discussion
In this study, we provide a comprehensive mapping and evaluation of mouse I-Ab-restricted CD4+ T cell epitopes in spike and nucleocapsid, two of the major immunogens of the SARS-CoV-2 virus. This was done systematically by screening a library of overlapping peptides covering the entire sequences of these proteins by IFNγ ELISpot assay. This was followed up with the generation of peptide:MHCII tetramer reagents, which were used to validate candidate epitopes as well as evaluate their relative immunogenicity The result of our labor-intensive empirical approach was a more rigorous identification of epitopes than what computational approaches of epitope prediction alone can provide. However, we did use computational approaches to help narrow down minimal peptide epitope sequences during the design of tetramers.
In total, we discovered 6 immunogenic epitopes in spike (S-26, S-298, S-571, 6-690, S-1008, and S-1104) and 3 in nucleocapsid (N-107, N-125, N-143) (summarized in Table 1), which to the best of our knowledge are all novel. The weak S-1008 epitope was only reliably immunogenic in response to peptide and not protein immunization. One additional weak spike epitope (S-883) lies in a region affected by a proline substitution (A889P) used in the prefusion stabilization of the HexaPro construct (but not current vaccines in the U.S.), highlighting potential caveats of using HexaPro and other engineered versions of viral proteins for immunological studies.
We also validated a previously identified immunodominant spike epitope (S-63) that is present in the Wuhan strain but absent in the BA.1 variant that was studied in our report (10). A tetramer representing this epitope (residues 62-76) was successfully used in a previous study (23). The tetramer created in our current study differs by containing a smaller, minimal peptide sequence (residues 63-74) for this epitope, which limits potential issues that may arise from alternative stretches of amino acids occupying the MHC-binding groove (24). Our ELISpot experiments also confirmed a very weak response to the previously identified nucleocapsid epitope (residues 9-23) of Wuhan that is also absent in BA.1 (10), but we did not investigate this further with tetramers. Interestingly, the two previous studies mapping CD4+ T cell epitopes in C57BL/6 mice only identified these two epitopes and not any of our novel ones (10, 11). As the S-63 epitope is considerably more immunodominant than the others in primary immune responses, this discrepancy could simply be due to differences in the sensitivity of our methods, or perhaps differences in epitope immunodominance patterns resulting from the different ways in which mice were immunized with antigen.
The frequencies of epitope-specific T cells determined by tetramer staining were in the general range of 101 - 102 cells per naive mouse, increasing variably up to as much as 3 x 104 cells in immunized mice, which is consistent with past observations of other epitope-specific CD4+ T cell populations (25). Assuming that there are approximately 2 x 107 total CD4+ T cells in the tissues we sampled per mouse, this translates into frequencies of 0.5 - 5 per million CD4+ T cells and 1500 per million CD4+ T cells in naive and immunized mice, respectively. Frequencies in protein immunized mice were more typically in the range of 102 - 103 cells (5 - 50 per million), which generally correlated with frequencies determined by ELISpot.
The most notable limitation of our study is that despite our comprehensive efforts, additional epitopes may have been missed. Several candidates identified by the ELISpot assay were not successfully validated by tetramers. It is not clear whether this was due to inaccurate prediction of minimal epitope sequences that were used in tetramer design or other technical issues related to tetramer generation. Although we screened potential epitopes masked by proline substitutions in HexaPro spike, we did not screen the truncated 63 amino acids of the C-terminus that are replaced with a Foldon domain in this construct (12). Finally, our assay relies specifically on T cell production of IFNγ, and although unlikely, it is possible that other epitopes preferentially generating other non-Th1 cell lineages were missed.
All of the newly identified epitopes are conserved between BA.1 and the ancestral Wuhan strain as well as Alpha, Gamma, Delta, BA.2, BA.5, XBB.1.5, EG.5.1, and the mouse adapted strain MA10 (26), and differences with Beta, BA.4, and BQ.1.1 are limited to just one epitope each (S-690, N-143, N-125, respectively). The S-63 epitope notwithstanding, this is not entirely surprising as there is unlikely to be immune selective pressure against mouse T cell epitopes in a human virus. However, it is noteworthy that human T cell epitopes have also been generally conserved across variants of concern, suggesting that immune evasion is driven predominantly by antibody rather than T cell recognition (17, 27). A welcome consequence of this conservation is that the epitopes described here for BA.1 can also be used for studies of Wuhan and other variant strains. It will be interesting to see if further epitope mapping for other variants will identify additional epitopes discordant with BA.1.
The identification of these I-Ab-restricted epitopes will be very useful for studies of CD4+ T cell immunity in C57BL/6 mice, the most commonly used strain in immunological research and the background for most hACE2 transgenic mice used in SARS-CoV-2 infection studies (28, 29). There is an appreciable difference in immunogenicity between the two strongest epitopes (S-26, S-1104) and the rest, so we propose the use of these epitopes along with S-63, if applicable, for robust studies of antigen-specific CD4+ T cells, especially if tetramers are to be used.
Data availability statement
The raw data supporting the conclusions of this article will be made available by the authors, without undue reservation.
Ethics statement
The animal study was approved by Massachusetts General Hospital IACUC. The study was conducted in accordance with the local legislation and institutional requirements.
Author contributions
LB: Conceptualization, Data curation, Formal analysis, Investigation, Methodology, Validation, Writing – review & editing. JB: Conceptualization, Data curation, Formal analysis, Investigation, Methodology, Validation, Writing – original draft, Writing – review & editing. JN: Data curation, Investigation, Methodology, Validation, Writing – review & editing. TN: Data curation, Investigation, Methodology, Validation, Writing – review & editing. LK: Investigation, Methodology, Writing – review & editing. YY: Investigation, Writing – review & editing. KH: Resources, Writing – review & editing. SL: Resources, Writing – review & editing. EO: Methodology, Resources, Writing – review & editing. CH: Resources, Writing – review & editing. AN: Resources, Writing – review & editing. MG: Resources, Writing – review & editing. AG: Resources, Writing – review & editing. AK: Resources, Writing – review & editing. GG: Funding acquisition, Resources, Writing – review & editing. EO: Funding acquisition, Project administration, Resources, Writing – review & editing. AL: Conceptualization, Formal analysis, Funding acquisition, Project administration, Resources, Supervision, Writing – review & editing. JM: Conceptualization, Data curation, Formal analysis, Funding acquisition, Investigation, Methodology, Project administration, Resources, Supervision, Validation, Writing – original draft, Writing – review & editing.
Funding
The author(s) declare financial support was received for the research, authorship, and/or publication of this article. This work was supported by the National Institutes of Health (P01 AI165072 for EOS, AL, and JM, DP2 AI154421, R01 AI176533, DP1 DA058476 for GG), the Massachusetts Consortium on Pathogen Readiness (GG and JM), Bill and Melinda Gates Foundation (GG), Burroughs Wellcome Career Award for Medical Scientists (GG), Howard Goodman Fellowship (GG), and the Massachusetts General Hospital Executive Committee on Research (AL and JM).
Acknowledgments
We thank Tianyi Bai for assistance with tetramer and protein production, Hugh Chen for help with ELISpot assays, and Daniel Shin, Ryan Nelson, Maisa Takenaka, and Stephen Elledge for helpful discussions.
Conflict of interest
GG receives research funding from Merck and Moderna.
The remaining authors declare that the research was conducted in the absence of any commercial or financial relationships that could be construed as a potential conflict of interest.
Publisher’s note
All claims expressed in this article are solely those of the authors and do not necessarily represent those of their affiliated organizations, or those of the publisher, the editors and the reviewers. Any product that may be evaluated in this article, or claim that may be made by its manufacturer, is not guaranteed or endorsed by the publisher.
Supplementary material
The Supplementary Material for this article can be found online at: https://www.frontiersin.org/articles/10.3389/fimmu.2024.1329846/full#supplementary-material
References
1. ;Li J, Lai S, Gao GF, Shi W. The emergence, genomic diversity and global spread of SARS-CoV-2. Nature. (2021) 600(7889):408–18. doi: 10.1038/s41586-021-04188-6
2. Röltgen K, Boyd SD. Antibody and B cell responses to SARS-CoV-2 infection and vaccination: the end of the beginning. Annu Rev Pathol. (2023) 19:69–97. doi: 10.1146/annurev-pathmechdis-031521-042754
3. Sette A, Sidney J, Crotty S. T cell responses to SARS-CoV-2. Annu Rev Immunol. (2023) 41:343–73. doi: 10.1146/annurev-immunol-101721-061120
4. Petrone L, Sette A, de Vries RD, Goletti D. The importance of measuring SARS-CoV-2-specific T-cell responses in an ongoing pandemic. Pathogens. (2023) 12(7):862. doi: 10.3390/pathogens12070862
5. Nelson RW, Chen Y, Venezia OL, Majerus RM, Shin DS,, MGH COVID-19 Collection & Processing Team, et al. SARS-CoV-2 epitope-specific CD4+ memory T cell responses across COVID-19 disease severity and antibody durability. Sci Immunol. (2022) 7(73):eabl9464. doi: 10.1126/sciimmunol.abl9464
6. Davenport BJ, Morrison TE, Kedl RM, Klarquist J. Conserved and novel mouse CD8 T cell epitopes within SARS-CoV-2 spike receptor binding domain protein identified following subunit vaccination. J Immunol. (2021) 206(11):2503–7. doi: 10.4049/jimmunol.2100195
7. Joag V, Wijeyesinghe S, Stolley JM, Quarnstrom CF, Dileepan T, Soerens AG, et al. Cutting edge: mouse SARS-CoV-2 epitope reveals infection and vaccine-elicited CD8 T cell responses. J Immunol. (2021) 206(5):931–5. doi: 10.4049/jimmunol.2001400
8. Smith CC, Olsen KS, Gentry KM, Sambade M, Beck W, Garness J, et al. Landscape and selection of vaccine epitopes in SARS-CoV-2. Genome Med. (2021) 13(1):101. doi: 10.1186/s13073-021-00910-1
9. Yang J, Kim E, Lee JS, Poo H. A murine CD8+ T cell epitope identified in the receptor-binding domain of the SARS-CoV-2 spike protein. Vaccines (Basel). (2021) 9(6):641. doi: 10.3390/vaccines9060641
10. Zhuang Z, Lai X, Sun J, Chen Z, Zhang Z, Dai J, et al. Mapping and role of T cell response in SARS-CoV-2-infected mice. J Exp Med. (2021) 218(4):e20202187. doi: 10.1084/jem.20202187
11. Wang Y, Wang B, Zhao Z, Xu J, Zhang Z, Zhang J, et al. Effects of SARS-CoV-2 omicron BA.1 spike mutations on T-cell epitopes in mice. Viruses. (2023) 15(3):763. doi: 10.3390/v15030763
12. Hsieh CL, Goldsmith JA, Schaub JM, DiVenere AM, Kuo HC, Javanmardi K, et al. Structure-based design of prefusion-stabilized SARS-CoV-2 spikes. Science. (2020) 369(6510):1501–5. doi: 10.1126/science.abd0826
13. Hastie KM, Li H, Bedinger D, Schendel SL, Dennison SM, Li K, et al. Defining variant-resistant epitopes targeted by SARS-CoV-2 antibodies: A global consortium study. Science. (2021) 374(6566):472–8. doi: 10.1126/science.abh2315
14. Hastie KM, Yu X, Ana-Sosa-Batiz F, Zyla DS, Harkins SS, Hariharan C, et al. Potent Omicron-neutralizing antibodies isolated from a patient vaccinated 6 months before Omicron emergence. Cell Rep. (2023) 42(5):112421. doi: 10.1016/j.celrep.2023.112421
15. Moon JJ, Pepper M. Generation of allergen-specific tetramers for a murine model of airway inflammation. Methods Mol Biol. (2018) 1799:165–81. doi: 10.1007/978-1-4939-7896-0_14
16. Olmedillas E, Mann JC, Peng W, Wang Y, Diaz Avalos R, Bedinger D, et al. Structure-based design of a highly stable, covalently-linked SARS-CoV-2 spike trimer with improved structural properties and immunogenicity. bioRxiv. (2021), 2021.05.06.441046. doi: 10.1101/2021.05.06.441046
17. Naranbhai V, Nathan A, Kaseke C, Berrios C, Khatri A, Choi S, et al. T cell reactivity to the SARS-CoV-2 Omicron variant is preserved in most but not all individuals. Cell. (2022) 185(7):1041–51. doi: 10.1016/j.cell.2022.01.029
18. Van den Broeck W, Derore A, Simoens P. Anatomy and nomenclature of murine lymph nodes: Descriptive study and nomenclatory standardization in BALB/cAnNCrl mice. J Immunol Methods. (2006) 312(1-2):12–9. doi: 10.1016/j.jim.2006.01.022
19. Legoux FP, Moon JJ. Peptide:MHC tetramer-based enrichment of epitope-specific T cells. J Vis Exp. (2012) 68):e4420. doi: 10.3791/4420
20. Grifoni A, Weiskopf D, Ramirez SI, Mateus J, Dan JM, Moderbacher CR, et al. Targets of T cell responses to SARS-CoV-2 coronavirus in humans with COVID-19 disease and unexposed individuals. Cell. (2020) 181(7):1489–501. doi: 10.1016/j.cell.2020.05.015
21. Lee SJ, McLachlan JB, Kurtz JR, Fan D, Winter SE, Baumler AJ, et al. Temporal expression of bacterial proteins instructs host CD4 T cell expansion and Th17 development. PloS Pathog. (2012) 8(1):e1002499. doi: 10.1371/journal.ppat.1002499
22. Dhanda SK, Mahajan S, Paul S, Yan Z, Kim H, Jespersen MC, et al. IEDB-AR: immune epitope database-analysis resource in 2019. Nucleic Acids Res. (2019) 47(W1):W502–6. doi: 10.1093/nar/gkz452
23. Mao T, Israelow B, Peña-Hernández MA, Suberi A, Zhou L, Luyten S, et al. Unadjuvanted intranasal spike vaccine elicits protective mucosal immunity against sarbecoviruses. Science. (2022) 378(6622):eabo2523. doi: 10.1126/science.abo2523
24. Landais E, Romagnoli PA, Corper AL, Shires J, Altman JD, Wilson IA, et al. New design of MHC class II tetramers to accommodate fundamental principles of antigen presentation. J Immunol. (2009) 183(12):7949–57. doi: 10.4049/jimmunol.0902493
25. Jenkins MK, Moon JJ. The role of naive T cell precursor frequency and recruitment in dictating immune response magnitude. J Immunol. (2012) 188(9):4135–40. doi: 10.4049/jimmunol.1102661
26. Leist SR, Dinnon KH, Schäfer A, Tse LV, Okuda K, Hou YJ, et al. A mouse-adapted SARS-CoV-2 induces acute lung injury and mortality in standard laboratory mice. Cell. (2020) 183(4):1070–85. doi: 10.1016/j.cell.2020.09.050
27. Tarke A, Sidney J, Methot N, Yu ED, Zhang Y, Dan JM, et al. Impact of SARS-CoV-2 variants on the total CD4+ and CD8+ T cell reactivity in infected or vaccinated individuals. Cell Rep Med. (2021) 2(7):100355. doi: 10.1016/j.xcrm.2021.100355
28. McCray PB, Pewe L, Wohlford-Lenane C, Hickey M, Manzel L, Shi L, et al. Lethal infection of K18-hACE2 mice infected with severe acute respiratory syndrome coronavirus. J Virol. (2007) 81(2):813–21. doi: 10.1128/JVI.02012-06
Keywords: C57BL/6, epitope mapping, HexaPro, vFLIP, immunodominance
Citation: Bricio-Moreno L, Barreto de Albuquerque J, Neary JM, Nguyen T, Kuhn LF, Yeung Y, Hastie KM, Landeras-Bueno S, Olmedillas E, Hariharan C, Nathan A, Getz MA, Gayton AC, Khatri A, Gaiha GD, Ollmann Saphire E, Luster AD and Moon JJ (2024) Identification of mouse CD4+ T cell epitopes in SARS-CoV-2 BA.1 spike and nucleocapsid for use in peptide:MHCII tetramers. Front. Immunol. 15:1329846. doi: 10.3389/fimmu.2024.1329846
Received: 30 October 2023; Accepted: 29 January 2024;
Published: 11 March 2024.
Edited by:
Laura Maggi, University of Florence, ItalyReviewed by:
Ravi Kumar Sharma, Karolinska Institutet (KI), SwedenLuka Cicin-Sain, Helmholtz Association of German Research Centers (HZ), Germany
Copyright © 2024 Bricio-Moreno, Barreto de Albuquerque, Neary, Nguyen, Kuhn, Yeung, Hastie, Landeras-Bueno, Olmedillas, Hariharan, Nathan, Getz, Gayton, Khatri, Gaiha, Ollmann Saphire, Luster and Moon. This is an open-access article distributed under the terms of the Creative Commons Attribution License (CC BY). The use, distribution or reproduction in other forums is permitted, provided the original author(s) and the copyright owner(s) are credited and that the original publication in this journal is cited, in accordance with accepted academic practice. No use, distribution or reproduction is permitted which does not comply with these terms.
*Correspondence: James J. Moon, jjmoon@mgh.harvard.edu
†Present address: Sara Landeras-Bueno, Universidad Cardenal Herrera-CEU, CEU Universities, Valencia, Spain
‡ These authors share first authorship
§These authors share last authorship