- 1Clinic for Pediatric and Adolescent Medicine, University Hospital Schleswig-Holstein, Lübeck, Germany
- 2Department for Infectious Diseases and Microbiology, University Hospital Schleswig-Holstein, Lübeck, Germany
- 3German Center for Infection Research, Partner Site Hamburg-Lübeck-Borstel-Riems, Lübeck, Germany
- 4Medical Clinic III, University Hospital Schleswig-Holstein, Lübeck, Germany
- 5Clinic for Gynecology and Obstetrics, University Hospital Schleswig-Holstein, Lübeck, Germany
- 6Pediatric Clinic and Policlinic, University Hospital Würzburg, Würzburg, Germany
Introduction: Group B Streptococcus is a significant cause of early-onset disease in term newborns, with a global incidence of 0.41/1000 live births. Intrapartum antibiotic prophylaxis (IAP) has reduced EOD incidence by over 80%, but concerns exist about its impact on the neonatal gut microbiome and potential long-term health effects.
Methods: This single center study examines the effects of IAP on the fecal infant microbiome in the first year of age and on the T cell phenotype in the first days after birth among 22 infants receiving IAP with penicillin due to maternal GBS colonization and 26 infants not exposed to IAP. The fecal microbiome was analyzed at birth, one month and one year of age through 16S rRNA gene sequencing. Additionally, a T cell phenotyping of peripheral blood was performed between the second and fifth day of age.
Results: At one month, IAP exposed infants had a significantly lower relative abundance of Bifidobacterium longum in fecal samples, an effect which was sustained at one year. In IAP exposed infants we found a proinflammatory T-helper cell profile, characterized by higher IL-17A, RORgt, and TGF-b expression.
Discussion: This study proposes a sustained impact of IAP on the neonatal microbiome and T cell repertoire.
Introduction
Group B Streptococcus (GBS) remains the leading cause of early onset disease (EOD) in term newborns (1) (2). Globally, the incidence of early onset disease by GBS is estimated to be 0.41/1000 live births (3), contributing substantially to neonatal morbidity and mortality. For Germany, an incidence of 0.15/1000 live births was reported by a prospective active surveillance study covering the years 2009 and 2010 (4). Vertical transmission of GBS occurs during birth in 35-63% of colonized women, if no IAP was administered (5) (6) (7). Globally, Russell et al. estimated 18% of pregnant women to have evidence for GBS in rectovaginal cultures (8), while estimates for Germany vary between 16% and 21%. Rectovaginal colonization is usually asymptomatic but can be a cause of chorioamnionitis in pregnant women (9) (10) (11).
Since the introduction of intrapartum antibiotic prophylaxis (IAP) in the 1990s, the incidence of EOD by GBS has decreased by more than 80% in the United States (12) (13), while late-onset GBS risk remains largely unchanged (12). Noteworthy, early antibiotic exposure can be associated with several adverse sequelae, e.g. obesity and overweight (14) (15), asthma, eczema and allergy (15) and Crohns disease in children (16). Therefore, concerns have arisen about the potential impact of IAP on the neonatal gut microbiome. The gut microbiome exerts a variety of functions within the human organism, such as biosynthesis of vitamins (17) and short-chain fatty acids providing additional energy to the host and lowering the luminal pH (17) (18) (19) (20), (21), protection against pathogens (18) (21), and regulation of intestinal permeability (22) (23). Furthermore, the gut microbiome interacts with the immune system influencing for instance the Treg/TH17-balance (24) (25) (26). Alterations of the gut microbiome in early life have implications for immune-related diseases such as atopy and asthma (27) as well as autoimmune diseases (28) later in life. Numerous studies have investigated the impact of IAP in vaginally delivered term infants on neonatal gut microbiota with conflicting results (29–44). However, one consistent effect of IAP in vaginally delivered full-term infants is the reduction of Bifidobacterium and Bacteroides, whereas on phylum-level Proteobacteria are increased in gut microbiota within the first three months following IAP (45) (46) (47). A meta-analysis found a non-significant reduction in infant α-diversity following IAP exposure including studies with fecal sampling between one week and three months (48). However, only few studies have investigated this impact beyond the first weeks after birth. Immune-microbiome interactions following IAP have not yet been examined to our knowledge.
In this study, we investigated the impact of the IAP on the infant microbiome immediately after birth and during the first year of age, as well as concomitant alterations of the immunophenotype of T cells within the first days after birth. We focused on T cells, as adaptive immune system-alterations are well described in animals and humans following microbial pertubations (49) (27), possibly due to the close interactions between the gut microbiome and Tregs or TH17 cells (24) (25) (26).
Methods
Study population
Between May 2019 and October 2020 n = 48 (n = 22 GBS positive; n = 26 GBS unknown (5) or negative (21)) mothers and their newborns were recruited in a single center study at the University Hospital of Lübeck, Germany. Written informed consent was obtained. Inclusion criteria were: at least 37 completed weeks of gestation, maternal age ≥ 18 years, informed consent of both parents, singleton pregnancy and vaginal birth. Exclusion criteria were antibiotic therapy within 8 weeks before birth, maternal smoking, insulin dependent diabetes mellitus, clinical signs of amniotic infection (maternal fever > 37.8°C without other cause or maternal tachycardia > 120 beats per minute (bpm) or fetal tachycardia > 180 bpm or purulent and foul-smelling amniotic fluid/discharge or maternal leukocytosis > 15,000/µl) or penicillin allergy. The study was approved by the Ethics committee of the University of Lübeck (reference numbers 19-022 and 15-304).
Stratification
Participants were assigned to one of two groups according to maternal GBS status. Mothers with cultural proof of rectovaginal GBS colonization – obtained between 35 and 37 weeks of gestation and cultured on selective media - received IAP according to national guidelines (5 million IU Benzylpenicillin from the onset of labor or rupture of membranes, followed by 2.5 million IU every 4h until birth intravenously (50)). Mothers without proof of rectovaginal GBS colonization (either negative culture or not tested and no risk factors for EOD by GBS) did not receive any intrapartum antibiotic prophylaxis, here referred to as “control group”.
Sample collection
Rectal swabs were obtained from the mothers perinatally and after birth from the newborn. Meconium was collected, immediately stored at -20°C and timely transferred to -80°C. Cord blood was collected immediately after birth for assessment of penicillin concentrations. For the T cell panel, 100 µl EDTA-blood were obtained once between the second and fifth day after birth in a subset of 22 infants during routine blood sampling (sepsis workup or newborn screening) by venipuncture of a peripheral vein. At one month and one year of age, stool collection tubes and instructions were mailed to the families. Stool samples were then collected at home by the parents, stored in a freezer and picked up by study personnel. The samples were transported into the lab on ice and stored at -80°C. Health data (concerning e.g. nutrition, infections, body measurements, medication and diseases) were collected via a standardized questionnaire at birth, one month and one year by study personnel.
DNA isolation
Samples were thawed at room temperature and approximately 100 mg of stool or 200 mg of meconium, were processed using the DNeasy PowerSoil Kit or DNeasy PowerSoil Pro Kit (Qiagen GmbH, Hilden, Germany) following the manufacturer´s instruction with some modifications: 20 µl Proteinase K were added to solution C1. For the DNA-extraction of meconium samples, DNA was washed twice with the C5-solution and eluted in 60 µl C6-solution. A negative extraction control was performed with each batch. Extracted DNA was stored at -20°C until subsequent use. For three children no meconium samples were available, therefore rectal swabs obtained immediately after birth were used for DNA-extraction and analysis in these three cases.
16S rRNA gene amplification, sequencing and bioinformatic processing
Partial sequences of the 16S rRNA gene in DNA samples were amplified using primers for V3/V4 hypervariable regions of the 16S rRNA gene (Supplementary Table 1) as described earlier (51). Polymerase chain reaction (PCR) was performed with the following parameters: 98°C for 30 seconds, 30 cycles with 98°C for 9 seconds, 55°C for 60 seconds and 72°C for 90 seconds. Meconium samples passed 35 PCR-cycles. In each PCR, a negative and a positive control were included, only PCRs with a clean negative control were subsequently used. Amplicons were run in gel electrophoresis and concentrations were estimated using Bio 1D software (Vilber Lourmat, Eberhardzell, Germany) against the 100 bp DNA ladder (Thermo Fischer Scientific, Waltham, USA). Equimolar amounts of each amplicon were pooled into a library and – after a run on an agarose gel – purified using the MiniElute Gel Extraction Kit (Qiagen GmbH, Hilden, Germany) and quantified with the NEBNExt Library Quant Kit (New England Biolabs, Ipswich, USA). The library was sequenced using the MiSeq platform (Illumina, San Diego, USA) and the MiSeq reagent Kit V3 for 600 cycles. PhiX library served as positive control. Negative extraction controls were incorporated to prove lack of contamination.
Fastq files were processed using mothur version 1.43.0 (mothur.org) (52) (53). Quality control steps included removal of sequences with homopolymers of more than 12 bases or sizes longer than 500 bp, removal of non-aligned sequences after alignment against EzBioCloud reference data base (54) and removal of chimeric sequences as identified by the VSEARCH algorithm (55). Taxonomic assignment followed using EzBioCloud reference (54). Mitochondrial, archaeal and eukaryotic sequences were removed. Operational taxonomic units (OTU) based analyses were performed on a random subset of 1360 reads per sample with a cutoff level of 0.03 or based on taxonomic assignment.
Flow cytometry analysis
100 µl EDTA blood were stained with 1 µl Fixable Viability Dye and with antibodies for T-cell subpopulation differentiation (CD3, CD4, CD25, Foxp3) and intracellular cytokines, chemokines and transcription factors (RORγt, IFN-γ, IL-2, IL-8, IL-17A, IL-10 and TGF-β, Supplementary Table 2) using Foxp3/Transcription Factor Staining Buffer Set (Thermo Fisher Scientific, Waltham, USA), which includes a lysis buffer for red blood cell lysis, and 50 µl Brilliant Stain Buffer (BD Biosciences, Becton, Dickinson and Company, Franklin Lakes, USA). Stained cells were resuspended in 500 µl Flow cytometry staining buffer (Thermo Fisher Scientific, Waltham, USA) and analyzed via flow cytometry (BD LSR II, BD Biosciences, Becton, Dickinson and Company, Franklin Lakes, USA) within four days. Compensation controls were carried out using beads (Invitrogen, Thermo Fisher Scientific, Waltham, USA) for generating a compensation matrix. The gating strategy included gating for singlets, alive cells, T-lymphocytes, T helper cells (CD3+ CD4+), cytotoxic T cells (CD3+ CD4-) and regulatory T cells (CD3+ CD4+, Foxp3+ CD25+). Fluorescence minus one controls were used to determine gates for cytokines, chemokines and transcription factors.
Statistical analysis
Microbiome data – Statistical analyses and data visualization were performed in R version 4.0.1 (r-project.org). Alpha diversity was compared using Shannons diversity index computed in psych package (56) and by calculating number of species in vegan package (57). Differences between groups were analyzed via Kruskal-Wallis-Test and pairwise Wilcoxon rank-sum test. To further assess differences between groups, indicator species were derived using Linear Discriminant Analysis Effect Size (LEfSe) (58) powered by Galaxy Project Platform (59). Score for differentiating discriminative features was set at 2.0. Beta diversity was analyzed applying principal coordinate analysis based on Bray-Curtis dissimilarities in labdsv package (60) and constrained correspondence analysis in vegan package (57). Differences between the groups were assessed via analysis of variance permutation testing in vegan package (57). Correlations between alpha diversity and antibiotic exposure degree were derived via Pearsons correlation coefficient in psych package (56).
Flow cytometry data – Relative expression as well as median fluorescence intensity (MdFI) of cytokines, chemokines and transcription factors were statistically analyzed with the Mann-Whitney-U-Test in R (version 4.3.2, packages tidyverse, dplyr, ggpubr). Statistical significance was defined as alpha-error < 5%. Because of alpha error accumulation, the significance level was adjusted for multiple testing using the Bonferroni correction.
Descriptive cohort data – Analysis was conducted in R (version 4.3.2) using Fishers exact test or Pearsons Chi-squared test with Yates continuity correction or Mann-Whitney-U-Test, statistical significance was defined as alpha-error < 5%. The influence of the count of penicillin doses on penicillin concentrations in cord blood was determined via linear regression, therefore the packages tidyverse, sandwich, ggfortify and car were applied.
Results
Study population
48 mothers and their infants were enrolled, 26 were allocated to the control group and 22 to the IAP exposed group. At birth, the two groups did not differ with respect to main perinatal parameters (Table 1, Supplementary Table 3). Follow up rate at one month and one year was 100% including a full set of samples.
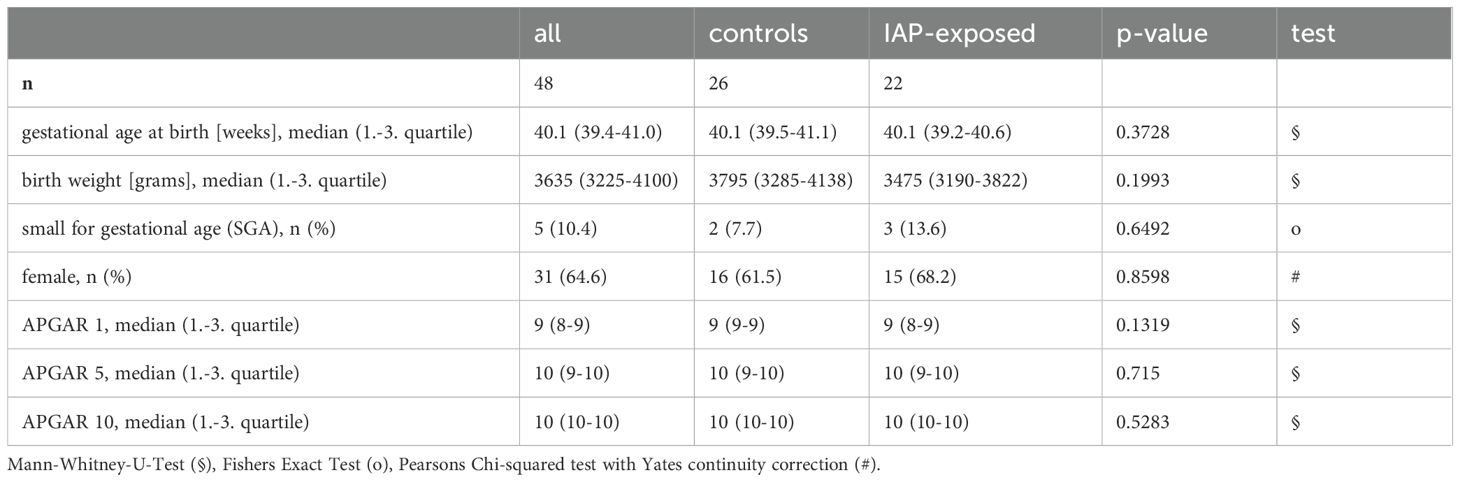
Table 1. Main characteristics of participating infants did not differ between IAP-exposed and control neonates at birth.
IAP exposed vs. control infants showed no differences in feeding type, antibiotic use or probiotic supplementation and hospitalization at one month and one year. Secondary outcomes such as weight, z-scores for weight and body mass index (BMI), occurrence of infections and other health-related outcomes were not different among IAP exposed and control infants during the first year of age (Supplementary Table 3).
Age dependent change of microbiota
Analysis of V3/V4 hypervariable region of the 16S rRNA gene revealed age dependent and age specific microbiome compositions at birth, one month of age and one year of age. Figure 1 illustrates data of the entire cohort, including both groups and all feeding types. In meconium samples, Proteobacteria dominated the infant gut, whereas at the age of one month a pronounced abundance of Actinobacteria (particularly Bifidobacterium (B.) longum) was notable. At the age of one year, the infant gut microbiome was dominated by Firmicutes (Figure 1A, species level data in Supplementary Figure 1). Both microbiome diversity and number of species increased with age (Figures 1B, C). Principal component analysis revealed distinct clusters for each age group as well as maternal swabs (Figure 1D).
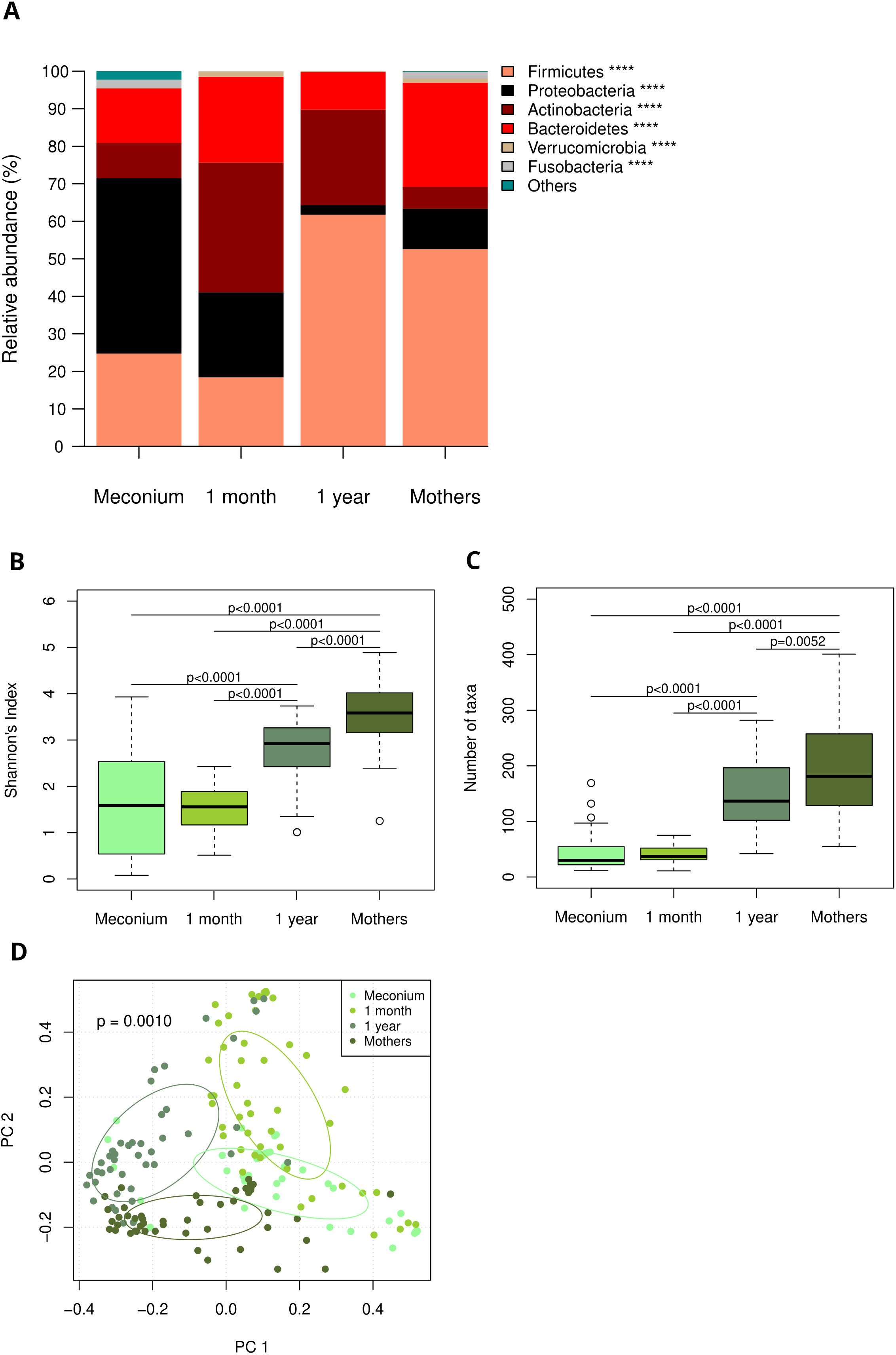
Figure 1. (A) Age dependent and age specific microbiome composition at birth, one month and one year of age and in maternal swabs, depicted as relative abundances of most abundant phyla. Taxa are depicted in the legend with their respective significance value (Kruskal-Wallis test: ****p<0.0001) (B) Shannons diversity index at birth, one month and one year of age as well as in maternal swabs (Pairwise Wilcoxon rank-sum test). (C) Number of detected species at birth, one month and one year of age as well as in maternal swabs (Pairwise Wilcoxon rank-sum test). (D) Principal component analysis reveals distinct clusters for each age group and maternal samples.
IAP with penicillin has limited effects on meconium microbiome
The microbiome of meconium samples showed minimal alterations with regard to IAP. Linear discriminant analysis Effect Size (LEfSe) revealed that Lactobacillales were a signature taxon in control infants. Negativicutes (species Veillonella dispar and Dialister invisus) and Stigonematales as well as the species Enterococcus gilvus, Bacteroides uniformis and B. bifidum and the genera Sporobacter, Citrobacter and Kluyvera were associated with exposure to IAP (Supplementary Figure 2C). Global measures of microbiome diversity and relative abundances did not differ significantly between groups (Supplementary Figures 2 A, B, D, E).
IAP leads to reduced expansion of Bifidobacterium longum at one month of age
In contrast to meconium samples, a stronger effect of IAP was detected in stool samples at the age of one month. The relative abundance of B. longum was significantly higher in control infants compared to exposed infants (23.8% vs. 12.0%, p = 0.02, Figure 2A). Other bifidobacterial species, such as B. breve and B. adolescentis, were nonsignificantly reduced in IAP-exposed infants at one month (Figure 2A). There was a significant impact of nutrition on the relative abundance of B. longum (breastfed neonates 10.9%, formula-fed neonates 4.2%, mixed [i.e. feeding of breast milk and formula] 20.1%, Kruskal-Wallis-Test p = 0.042, Figure 2D), whereas testing for specific feeding habits revealed no significant impact, possibly due to correction for multiple testing. In line with the relative abundances, B. longum was identified as a signature taxon for control infants at one month. Additionally, the species B. sanguini, the genus Collinsella and family, order and class Coriobacteriaceae, Coriobacteriales and Coriobacteriia were detected as signature taxa in control infants (Figure 2C). Indicator species for IAP exposure at one month of age were Deltaproteobacteria (with species Bilophila wadsworthia), Fusobacteria (genus Fusobacterium), Leclercia (Leclercia adecarboxylata) as well as the species Cutibacterium avidum, Staphylococcus lugudunensis, Lactobacillus reuteri and Veillonella parvula (Figure 2C). Global measures of microbial diversity were not affected by IAP (Figure 2B, Supplementary Figures 3A, B).
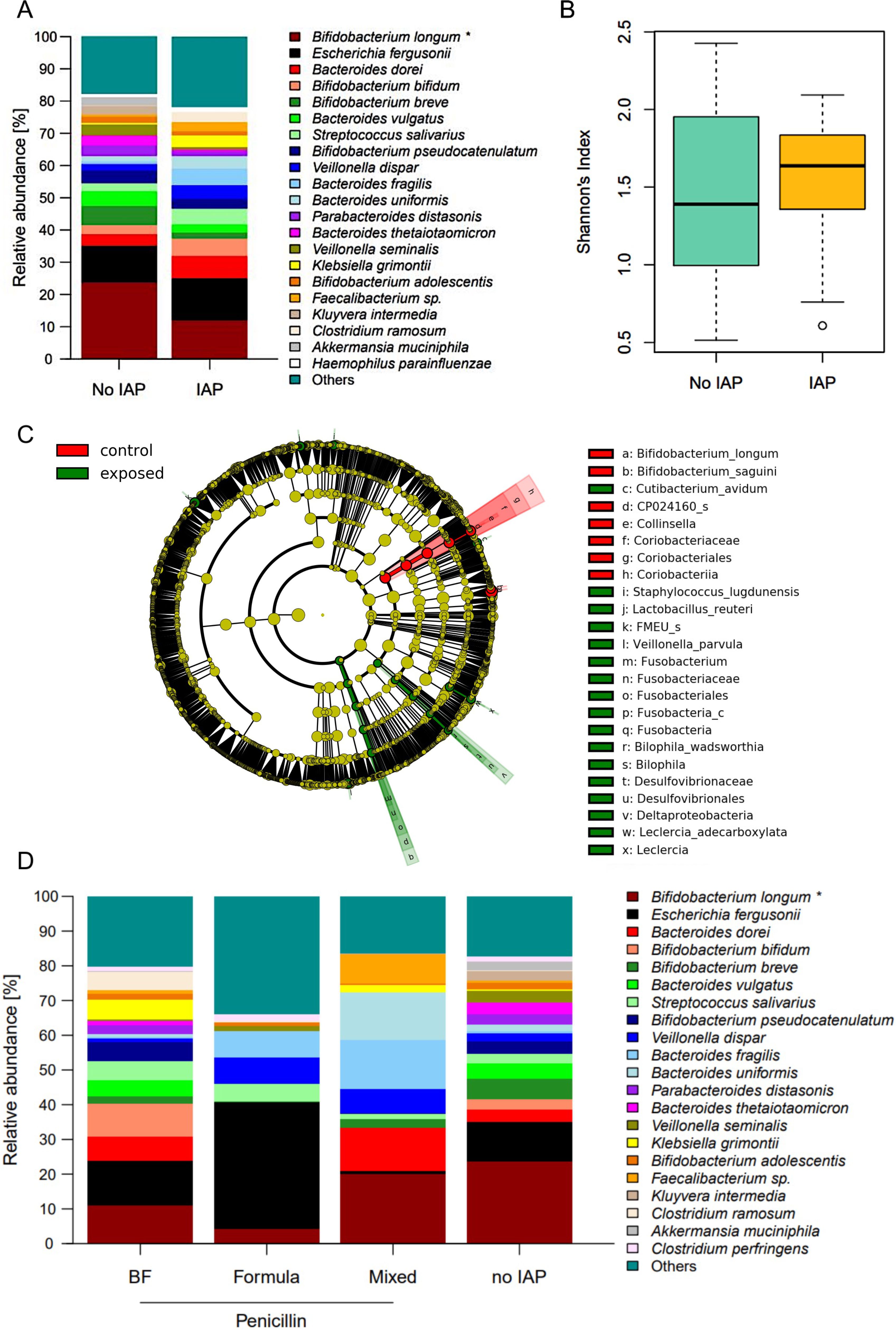
Figure 2. (A) Relative abundances of the most abundant species in fecal samples at one month. Significantly higher abundance of B. longum in control infants compared to IAP exposed infants. Taxa are depicted in the legend with their respective significance value (Mann-Whitney-U-Test: *p = 0.02). (B) Shannons diversity index of fecal mibrobiome samples at one month did not differ between exposed and control infants. (C) Influence of nutrition on relative abundances in fecal microbiome at one mont. Taxa are depicted in the legend with their respective significance value (Kruskal-Wallis test: *p = 0.042). BF = exclusively breast fed infants, formula = exclusively formula fed infants, mixed = infants fed with breast milk and formula. (D) .
Effects of IAP are sustained through the first year of age
As in one month samples, the relative abundance of B. longum was persistently reduced in IAP exposed infants at the age of one year (9.5% vs. 11.4%, p = 0.044, Figure 3A). Consistent with the results at one month, indicator species analysis revealed B. longum as a signature taxon for control infants. Moreover, Bacillales, Pasteurellales, Merdimonas as well as the species Parabacteroides distasonis and Lactobacillus acidophilus and Clostridium celatum were identified as signature taxa for control infants.
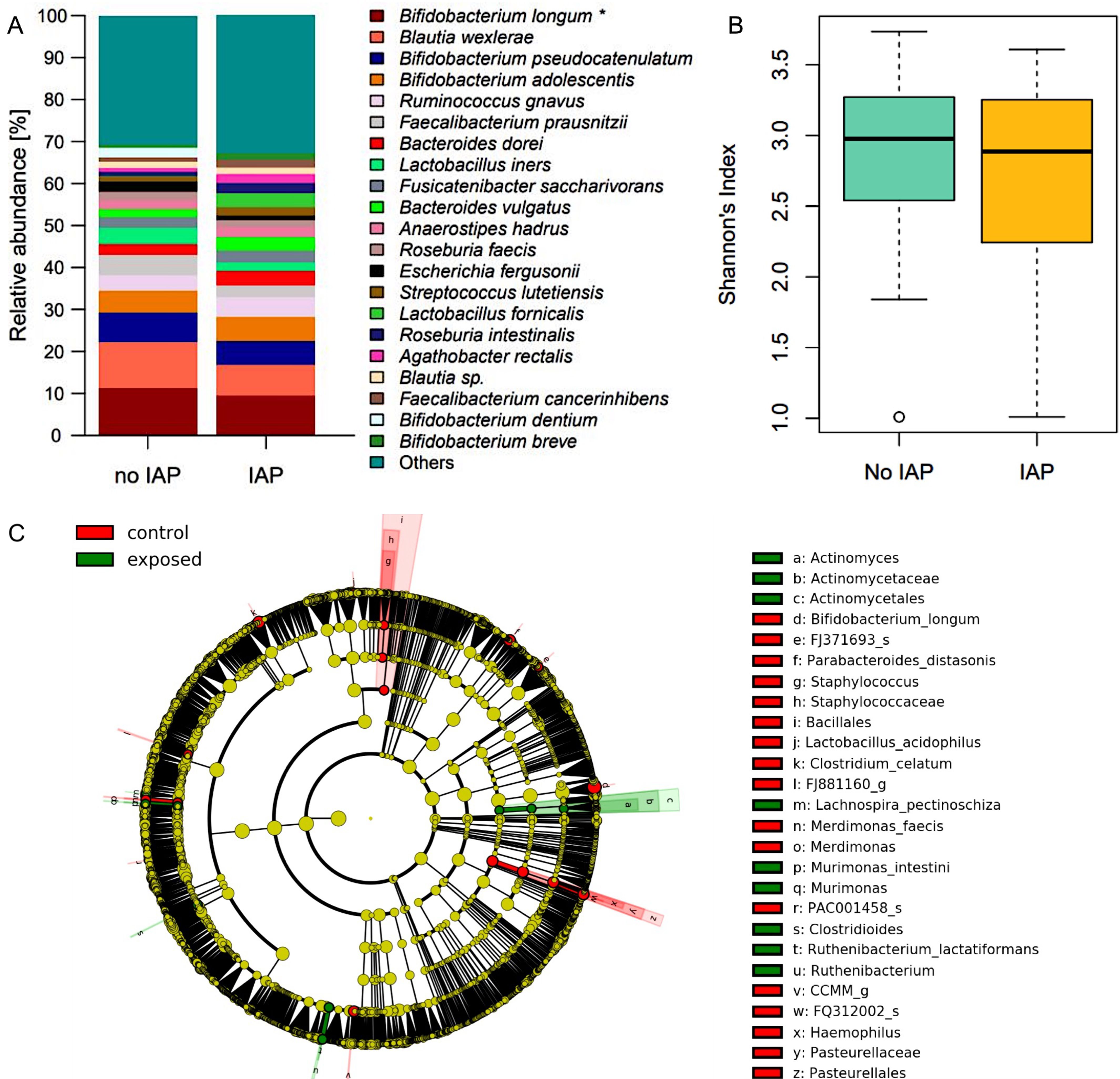
Figure 3. (A) Relative abundances of the most abundant species in fecal samples at one year. Significantly higher abundance of B. longum in control infants. Taxa are depicted in the legend with their respective significance value (Mann-Whitney-U-Test: *p = 0.044). (B) Shannons diversity index of fecal mibrobiome samples at one year did not differ between exposed and control infants. (C) LEfSe analysis of fecal microbiome at one year identified signature taxa in IAP exposed and control infants.
On the contrary, Actinomycetales, Clostridioides, Murimonas and the species Lachnospira pectinoschiza and Ruthenibacterium lactatiformans were associated with IAP in these samples (Figure 3C). Global measures of microbiome diversity did not differ between groups (Figure 3B, Supplementary Figures 3C, D).
IAP exposed infants display a proinflammatory T helper cell repertoire
The subset of the whole cohort which provided blood samples (22 of 48 infants, n = 9 IAP exposed and n = 13 control infants) showed no significant differences among IAP exposed and control infants concerning important characteristics such as gestational age, occurrence of early onset disease, birth weight and sex (data not shown). IAP was associated with a higher expression of IL-17A (p = 0.013), RORγt (p = 0.036) and TGF-β (p = 0.034) in T helper cells (Figures 4A–C) in peripheral blood during the first days after birth. Interestingly, regulatory T cells expressed less IL-17A in IAP exposed infants compared to control infants (p = 0.05, Figure 4D). Cytotoxic T cells (defined as CD3+ CD4- lymphocytes) expressed less IFN-γ (p = 0.01), IL-2 (p = 0.017), IL-8 (p = 0.028) and IL-10 (p = 0.021) in IAP exposed infants, whereas a higher expression of RORγt (p = 0.023) was observed (Figures 5A–E). Flow cytometry data did not remain significant after Bonferroni-correction for multiple testing.
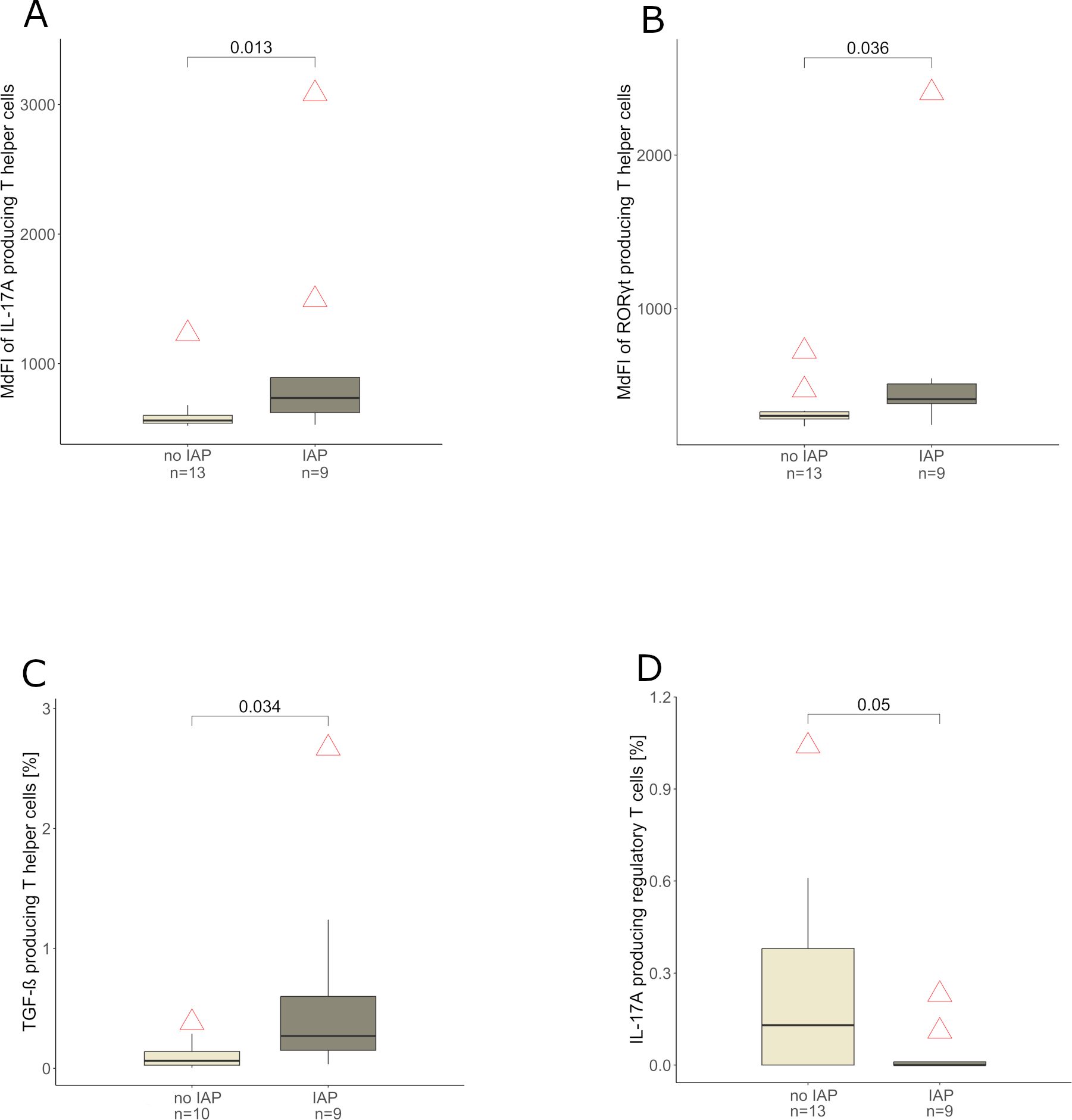
Figure 4. (A, B) Median Fluorescence Intensity (MdFI) of T helper cells producing IL-17A and RORγt derived from peripheral blood of infants without and with IAP respectively, light grey: no IAP, dark grey: IAP exposure. (C) T helper cell frequencies [%] producing TGF-β derived from peripheral blood of infants without and with IAP respectively, light grey: no IAP, dark grey: IAP exposure. (D) Regulatory T cell frequencies [%] producing IL-17A derived from peripheral blood of without and with IAP respectively, light grey: no IAP, dark grey: IAP exposure.
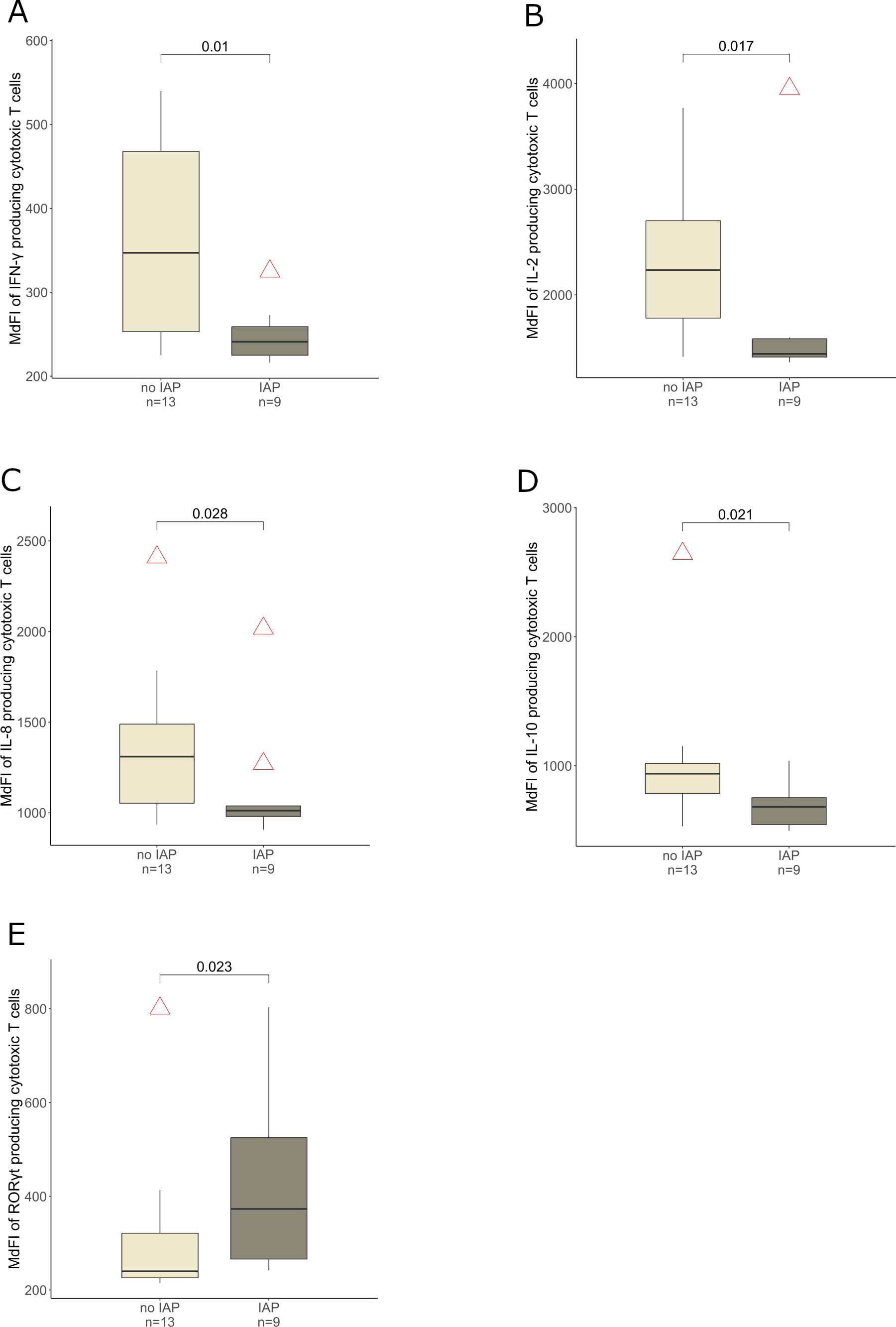
Figure 5. MdFI of cytotoxic T cells producing (A) IFN-γ, (B) IL-2, (C) IL-8, (D) IL-10 and (E) RORγt derived from peripheral blood of infants without and with IAP respectively, light grey: no IAP, dark grey: IAP exposure.
Repetitive penicillin doses have no cumulative effect on microbiome diversity
The median penicillin concentration of 12 available cord blood samples was 6.3 µg/ml. We observed no cumulation of penicillin-concentrations in cord blood with increasing penicillin administrations (Supplementary Figure 4B). In a subgroup analysis at one month of age, the Shannons diversity index did not correlate with the number of penicillin doses (Supplementary Figure 4A).
Discussion
GBS is a leading cause of EOD in term newborns (1) (2). Its vertical transmission during birth can be prevented with IAP. In order to explore effects of an IAP on the fecal infant microbiome in the first year of age as well as the T cell phenotype in the first days after birth, we conducted a prospective cohort study of 48 mothers and their term infants, among them 22 with IAP (here defined as penicillin G for maternal GBS-colonization) and 26 without any intrapartum antibiotics (control group).
We found that IAP significantly reduced the relative abundance of B. longum in the infant gut at one month and one year of age. In infant blood, a predominantly proinflammatory T-helper-phenotype in penicillin-exposed neonates was observed with a higher production of IL-17A, RORγt and TGF-β.
In meconium, multiple facultative anaerobic colonizers were identified, such as Escherichia, Enterococcus and Staphylococcus, which are typical pioneer colonizers of the infant gut (61). Lactobacillus and Prevotella were also present in meconium, most likely as a result of vaginal birth (62). B. longum occurred with a relative abundance of 0.4% and 2.3% in control vs. exposed infants, respectively.
Strict anaerobic colonizers such as Bifidobacterium and Bacteroides became more abundant at one month postpartum, consistent with previous findings (35). B. longum abundance was significantly reduced in IAP exposed infants.
At one year, mainly Bifidobacterium and Bacteroides dominated the infant gut. As described by Bäckhed et al., signature taxa at 12 months, such as Bacteroides, Anaerostipes, and Roseburia were also present in our cohort (61). Notably, one third of the cohort was still breastfed at one year, maintaining taxa like Bifidobacterium and Lactobacillus (61) (63). The relative abundance of B. longum remained significantly lower in IAP exposed infants compared to control infants at one year.
Bifidobacteria dominate the gut microbiome of breastfed infants during the first year and B. longum declines in abundance throughout life (64). It is known to mediate multiple beneficial effects in early infancy: B. longum subspecies infantis is the champion metabolizer of human milk oligosaccharides (65), converts these into acetate and provides hereby additional energy for the infant (19) (65) (66). B. longum protects against enteropathogenic infections by producing acetate (18) and by lowering fecal pH (20). Bifidobacteriaceae promote intestinal tolerance by inducing regulatory T cells and anti-inflammatory cytokines like IL-10 and IL-27 (67), which could be particularly important in the immune regulation of microbiota-induced immune responses during colonization of the newborn. Bifidobacteria-derived indol-3-lactic acid (68) suppresses TH17 responses via Galectin-1 (67), providing a possible functional link between the proinflammatory immune phenotype of T helper cells (IL-17A, RORγt and TGF-β) and diminished Bifidobacterium longum observed in IAP exposed infants in our study. Galectin-1, a β-galactoside binding lectin (69), modulates T-cell functions by reducing cell adhesion to extracellular matrix (70) and endothelial cells (71) and through an altered cytokine production towards antiinflammation (70) (72).
Reduced bifidobacterial counts are a feature of dysbiosis (73) and linked to intestinal inflammation in celiac disease (74) (75), colic (76), obesity (77), and autoimmunity (28). Reduced bifidobacterial abundances could also compromise vaccine responses as these were positively associated with Bifidobacterium and Bifidobacterium longum subspecies infantis in a Bangladeshi cohort at 15 weeks of age (78). Thus, reduced B. longum abundances might have relevant short term as well as long term consequences for the infant health and development.
The effects of IAP on the infant microbiome in vaginally delivered term infants have been investigated extensively, with studies focusing on the first weeks after birth and some following up to one year (29–44). A reduction in Bifidobacterium following IAP has been observed between one and twelve weeks (30) (31) (33) (34), (35), (37), (39), (42). In the largest cohort of vaginally delivered, IAP-exposed (n=375) and not exposed infants (n=876) studied to date, a reduction of Bifidobacterium was described in partially or fully breastfed infants at the age of three months in both, 16S rRNA-Analysis and qPCR (42). At family-level, a reduction of Bifidobacteriaceae after IAP exposure was observed between the age of two days and four weeks in vaginally delivered terms (36) (38), including one study showing remarkably increased Bifidobacteriaceae at the age of 12 month (36).
Furthermore, recent research regarding microbial deviations following IAP in vaginally delivered term infants consistently reports a reduction in Bacteroides (between the second day after birth and 12 months) (29) (33) (40) (44). Also, elevation of Clostridium in the infant feces (between one and 12 month of age) (29) (40) (41), was observed, whereas one study detected lower Clostridium at 12 months (33).
We identified several signature taxa for IAP exposed and non-exposed infants during the first year of age. B. longum, consistently identified in non-exposed infants at one month and one year, is associated with numerous beneficial effects, as described above. In meconium, Lactobacillales, typical early colonizers in vaginally born infants, are a signature taxon for non-exposed infants, indicating a reduced transmission of Lactobacillales during parturition from the maternal vaginal microbiome in IAP-exposed newborns (62). Lactobacillales typically reside in the intestines of vaginally delivered, breastfed infants (62) (63), exerting beneficial effects such as enhancing tight junction integrity and reducing the adhesion of enteropathogenic bacteria (23). At one month, Coriobacteriaceae was a signature taxon in non-exposed infants fecal samples, which may protect against intestinal inflammation (79). In contrast, Fusobacterium was a signature taxon in IAP exposed infants at one month. This is intriguing, as Fusobacterium promotes a proinflammatory microenvironment (80) and Fusobacteriaceae can be indicative of disease status in treatment-naïve Crohns disease in pediatric patients (81).
The proinflammatory T helper cell phenotype (TGF-β, RORγt, IL-17A, Figure 4A-C) among IAP exposed neonates in our small sample study generates the hypothesis that TH17-cell responses might be pronounced in IAP-exposed neonates. TH17 cells are involved in acute inflammatory reactions (82), strengthen the mucosal barrier function (83) and ward off pathogens (84). Depending on IL-23, they can induce autoimmunity (85) and adopt a pathological phenotype (86). TH17-cells are involved in the pathogenesis of psoriasis (87) and are frequently present in patients with multiple sclerosis (88). In mice, delayed microbial maturation increased TH17-frequency and led to IL-17A dependent aggravated allergic asthma, even with allergen exposure at the time of normalized microbial diversity (89). Consistently, a higher RORγt expression in CD3+ CD4- cells among IAP exposed neonates (Figure 5E) could indicate an increased Tc17 cell response, defined as CD8 positive T cells producing IL-17 (90), which play an important role in inflammatory diseases (91) (88) (92).
RORγt positive regulatory T cells are induced in mice by microbiota and their metabolites (93) (94), and are also found in human colonic biopsies (94). Human RORγt positive regulatory T cells can produce IL-17 under proinflammatory conditions in vitro, temporarily losing their suppressive functions (95). Al Nabhani et al. describe a “weaning reaction” in mice, an intestinal immune response during a defined time window, where disturbances like antibiotics can cause lifelong inflammatory imprinting. During this reaction, microbiota and their metabolites induce RORγt positive Tregs (96). We observed a reduction in IL-17A-producing Tregs in IAP exposed infants, possibly due to an impaired microbiota-dependent induction of RORγt positive Tregs. In vitro, TGF-β inhibits IL-17 production in Foxp3+ cells (95), possibly explaining the reduction in IL-17A-producing Tregs (Figure 4D), whereas CD4 positive cells exhibited a higher TGF-β production (Figure 4C) in IAP exposed infants in our study.
We observed a reduced activity of cytotoxic T cells in IAP exposed infants e.g. in the production of IFN-γ and IL-2 (Figure 5A, B). Although interferon responses are important to combat viral infections, our T cell findings did not translate into differences in the occurrence of infections during the first year of age.
In an available subgroup of 12 cord blood samples, 10/12 of penicillin-concentrations were above the minimal inhibitory concentration for GBS-Meningitis of 0.125 µg/ml (97), indicating efficacious concentrations in mothers and infants. A median penicillin concentration in cord blood of 6.3 µg/ml was reached, penicillin concentrations measured in this study are in line with the published cord blood concentrations of penicillin as IAP (98) (99).
A major strength of this study lies in the prospective study design and a balanced composition of the cohort consisting of vaginally born term-infants at least partially breastfed over 80% at the age of one month and approximately one third of the cohort at the age of one year. No family was lost to follow-up and even over 80% of the meconium samples provided by IAP exposed and control infants were included in the final microbiome analysis. There are only a few studies investigating effects of IAP on the infant microbiome during the entire first year of age (29) (32) (33) (36), (44), none of them integrating immune responses of the infant.
Limitations, on the other hand, lie in a rather small sample size and possible confounding factors such as postpartum applied antibiotics (13% at the age of one month, 21% at the age of one year, there were no significant differences between IAP exposed and control infants, Supplementary Table 3).
We used partial 16S sequencing, which is known to have limited precision in species level assignment in analyzing the microbiota (100). More precise and accurate classification could have been achieved using full length 16S sequencing (101). While we are aware of that species level assignments are to be taken with caution, we believe species level assignment to be inevitable in neonatal research and we have outlined usability of our approach in recent studies (e.g (102–104)) and believe this to be an adequate strategy in a setting with a focus on neonatal microbial communities which provide less diversity and thus less complexity. Examination of T cell panels during the first days after birth was performed only in a subset of infants (n = 22) and might therefore be underpowered. A longer follow-up period of a larger cohort during early childhood could account for dysbiosis-mediated diseases as a possible result of inflammatory imprinting of the immune system, such as obesity and diseases with intestinal inflammation such as colic, celiac disease or Crohns disease.
Increasing the abundance of B. longum subsp. infantis by probiotic supplementation might be an approach to prevent or alleviate IAP induced dysbiosis and potential harmful long-term consequences in infants at risk. Twenty-one days of B. longum subsp. infantis strain EVC001-supplementation in breastfed newborns led to a sustained increase in bifidobacterial counts and a reduction of inflammatory surrogate parameters (less fecal IL-17A, higher anti-inflammatory indol-3-lactic acid and less fecal calprotectin) (20) (67) (105).
Following IAP in GBS-colonized mothers, the relative abundance of B. longum was significantly reduced at the age of one month and this reduction persisted up to one year. IAP was furthermore associated with a proinflammatory T-helper-phenotype during the first days of age. Our findings suggest that intrapartum antibiotic prophylaxis can have a sustained impact on the infant gut microbiome and early immune development. These observations may help to better understand the potential consequences of early-life interventions and their role in shaping microbiota and immune function. Further research with adequate power and high-quality investigation methods in the field of IAP-induced dysbiosis and health-related outcomes with a longer follow-up than one year is needed.
Data availability statement
The raw sequencing data are freely available online at the European Nucleotide Archive at https://www.ebi.ac.uk/ena/browser/home under accession number PRJEB80487.
Ethics statement
The studies involving humans were approved by Ethics committee of the University of Lübeck. The studies were conducted in accordance with the local legislation and institutional requirements. Written informed consent for participation in this study was provided by the participants legal guardians/next of kin.
Author contributions
JT: Data curation, Investigation, Visualization, Writing – original draft, Writing – review & editing. ML: Data curation, Formal analysis, Validation, Visualization, Writing – review & editing. SG: Data curation, Formal analysis, Supervision, Validation, Visualization, Writing – review & editing. SJ: Resources, Writing – review & editing. AB: Resources, Writing – review & editing. EH: Resources, Writing – review & editing. GS: Supervision, Writing – review & editing. VB: Resources, Writing – review & editing. JR: Resources, Supervision, Writing – review & editing. CH: Conceptualization, Resources, Supervision, Writing – review & editing. MD: Conceptualization, Data curation, Funding acquisition, Investigation, Methodology, Resources, Supervision, Writing – original draft, Writing – review & editing.
Funding
The author(s) declare that financial support was received for the research and/or publication of this article. The work was supported by the German Research Foundation (DFG: IRTG 1911).
Conflict of interest
The authors declare that the research was conducted in the absence of any commercial or financial relationships that could be construed as a potential conflict of interest.
Generative AI statement
The author(s) declare that no Generative AI was used in the creation of this manuscript.
Publisher’s note
All claims expressed in this article are solely those of the authors and do not necessarily represent those of their affiliated organizations, or those of the publisher, the editors and the reviewers. Any product that may be evaluated in this article, or claim that may be made by its manufacturer, is not guaranteed or endorsed by the publisher.
Supplementary material
The Supplementary Material for this article can be found online at: https://www.frontiersin.org/articles/10.3389/fimmu.2025.1540979/full#supplementary-material
Supplementary Figure 1 | Relative abundances of the most abundant species in meconium samples as well as samples at one month and one year of age and maternal swabs.
Supplementary Figure 2 | (A) Relative abundances of the most abundant species in meconium samples showed little differences between IAP exposed and control newborns. (B) Shannons diversity index of meconium samples did not differ between exposed and control infants. (C) LEfSe analysis of fecal microbiome in meconium samples identified signature taxa in IAP exposed and control infants. (D) Beta diversity showed no significant differences between IAP exposed and control infants in meconium samples (E) Constrained correspondence analysis indicated that IAP exposure explained 2.3% of compositional variance.
Supplementary Figure 3 | (A) Beta diversity showed no significant differences in fecal samples at one month between IAP exposed and control infants. (B) Constraining for IAP exposure displayed 2.23% of compositional variance explained by the selected variable at the age of one month. (C) Beta diversity showed no significant differences in fecal samples at one year between IAP exposed and control infants. (D) Constrained correspondence analysis showed 1.7% of variance explained by IAP exposure at the age of one year.
Supplementary Figure 4 | (A) Shannons diversity index and number of penicillin applications at one month of age showed no significant Pearsons product-moment correlation. (B) Penicillin concentrations in cord blood and number of penicillin applications show no significant correlation in linear regression.
Supplementary Figure 5 | Gating strategy. After singlet discrimination, gating of live cells and CD3+ lymphocytes (light green), T helper cells (CD4+) and cytotoxic T cells (here defined as CD3+CD4-) were discriminated. Within each T cell subtype different antigens were detected (exemplary shown here with T helper cells).
References
1. Joshi NS, Huynh K, Lu T, Lee HC, and Frymoyer A. Epidemiology and trends in neonatal early onset sepsis in California, 2010–2017. J Perinatol. (2022) 42:940–6. doi: 10.1038/s41372-022-01393-7
2. Stoll BJ, Puopolo KM, Hansen NI, Sánchez PJ, Bell EF, Carlo WA, et al. Early-onset neonatal sepsis 2015 to 2017, the rise of escherichia coli, and the need for novel prevention strategies. JAMA Pediatr. (2020) 174:e200593. doi: 10.1001/jamapediatrics.2020.0593
3. Madrid L, Seale AC, Kohli-Lynch M, Edmond KM, Lawn JE, Heath PT, et al. Infant group B streptococcal disease incidence and serotypes worldwide: systematic review and meta-analyses. Clin Infect Dis. (2017) 65:S160–72. doi: 10.1093/cid/cix656
4. Wicker E, Lander F, Weidemann F, Hufnagel M, Berner R, and Krause G. Group B streptococci: declining incidence in infants in Germany. Pediatr Infect Dis J. (2019) 38:516–9. doi: 10.1097/INF.0000000000002115
5. Easmon CSF, Hastings MJG, Deeley J, Bloxham B, Rivers RPA, and Marwood R. The effect of intrapartum chemoprophylaxis on the vertical transmission of group B streptococci. BJOG Int J Obstet. Gynaecol. (1983) 90:633–5. doi: 10.1111/j.1471-0528.1983.tb09280.x
6. Boyer KM and Gotoff SP. Prevention of early-onset neonatal group B streptococcal disease with selective intrapartum chemoprophylaxis. N Engl J Med. (1986) 314:1665–9. doi: 10.1056/NEJM198606263142603
7. Gizachew M, Tiruneh M, Moges F, Adefris M, Tigabu Z, and Tessema B. Proportion of Streptococcus agalactiae vertical transmission and associated risk factors among Ethiopian mother-newborn dyads, Northwest Ethiopia. Sci Rep. (2020) 10:1–8. doi: 10.1038/s41598-020-60447-y
8. Russell NJ, Seale AC, O'Driscoll M, O'Sullivan C, Bianchi-Jassir F, Gonzalez-Guarin J, et al. Maternal colonization with group B streptococcus and serotype distribution worldwide: systematic review and meta-analyses. Clin Infect Dis. (2017) 65:S100–11. doi: 10.1093/cid/cix658
9. Brimil N, Barthell E, Heindrichs U, Kuhn M, Lütticken R, and Spellerberg B. Epidemiology of Streptococcus agalactiae colonization in Germany. Int J Med Microbiol. (2006) 296:39–44. doi: 10.1016/j.ijmm.2005.11.001
10. Kunze M, Zumstein K, Markfeld-Erol F, Elling R, Lander F, Prömpeler H, et al. Comparison of pre- and intrapartum screening of group B streptococci and adherence to screening guidelines: a cohort study. Eur J Pediatr. (2015) 174:827–35. doi: 10.1007/s00431-015-2548-y
11. Kunze M, Ziegler A, Fluegge K, Hentschel R, Proempeler H, and Berner R. Colonization, serotypes and transmission rates of group B streptococci in pregnant women and their infants born at a single University Center in Germany. J Perinat. Med. (2011) 39:417–22. doi: 10.1515/jpm.2011.037
12. Schrag SJ and Verani JR. Intrapartum antibiotic prophylaxis for the prevention of perinatal group B streptococcal disease: Experience in the United States and implications for a potential group B streptococcal vaccine. Vaccine. (2013) 31:D20–6. doi: 10.1016/j.vaccine.2012.11.056
13. Schrag SJ, Farley MM, Petit S, Reingold A, Weston EJ, Pondo T, et al. Epidemiology of invasive early-onset neonatal sepsis, 2005 to 2014. Pediatrics. (2016) 138:1–9:e20162013. doi: 10.1542/peds.2016-2013
14. Rasmussen SH, Shrestha S, Bjerregaard LG, Ängquist LH, Baker JL, Jess T, et al. Antibiotic exposure in early life and childhood overweight and obesity: A systematic review and meta-analysis. Diabetes Obes. Metab. (2018) 20:1508–14. doi: 10.1111/dom.13230
15. Bejaoui S and Poulsen M. The impact of early life antibiotic use on atopic and metabolic disorders. E Med Public Health. (2020) 2020(1):279–89. doi: 10.1093/emph/eoaa039
16. Hviid A, Svanstrom H, and Frisch M. Antibiotic use and inflammatory bowel diseases in childhood. Gut. (2011) 60:49–54. doi: 10.1136/gut.2010.219683
17. Koenig JE, Spor A, Scalfone N, Fricker AD, Stombaugh J, Knight R, et al. Succession of microbial consortia in the developing infant gut microbiome. Proc Natl Acad Sci. (2011) 108:4578–85. doi: 10.1073/pnas.1000081107
18. Fukuda S, Toh H, Hase K, Oshima K, Nakanishi Y, Yoshimura K, et al. Bifidobacteria can protect from enteropathogenic infection through production of acetate. Nature. (2011) 469:543–7. doi: 10.1038/nature09646
19. Sela DA, Chapman J, Adeuya A, Kim JH, Chen F, Whitehead TR, et al. The genome sequence of Bifidobacterium longum subsp. infantis reveals adaptations for milk utilization within the infant microbiome. Proc Natl Acad Sci. (2008) 105:18964–9. doi: 10.1073/pnas.0809584105
20. Frese SA, Hutton AA, Contreras LN, Shaw CA, Palumbo MC, Casaburi G, et al. Persistence of Supplemented Bifidobacterium longum subsp. infantis EVC001 in Breastfed Infants. mSphere. (2017) 2:e00501–17. doi: 10.1128/mSphere.00501-17
21. Mercer EM and Arrieta M-C. Probiotics to improve the gut microbiome in premature infants: are we there yet? Gut Microbes. (2023) 15:. 1–27. doi: 10.1080/19490976.2023.2201160
22. Ewaschuk JB, Diaz H, Meddings L, Diederichs B, Dmytrash A, Backer J, et al. Secreted bioactive factors from Bifidobacterium infantis enhance epithelial cell barrier function. Am J Physiol.-Gastrointest. Liver Physiol. (2008) 295:G1025–34. doi: 10.1152/ajpgi.90227.2008
23. Anderson RC, Cookson AL, McNabb WC, Kelly WJ, and Roy NC. Lactobacillus plantarum DSM 2648 is a potential probiotic that enhances intestinal barrier function. FEMS Microbiol Lett. (2010) 309:184–92. doi: 10.1111/j.1574-6968.2010.02038.x
24. Ivanov II, Frutos Rde L, Manel N, Yoshinaga K, Rifkin DB, Sartor RB, et al. Specific microbiota direct the differentiation of IL-17-producing T-helper cells in the mucosa of the small intestine. Cell Host Microbe. (2008) 4:337–49. doi: 10.1016/j.chom.2008.09.009
25. Atarashi K, Tanoue T, Shima T, Imaoka A, Kuwahara T, Momose Y, et al. Induction of colonic regulatory T cells by indigenous clostridium species. Science. (2011) 331:337–41:6015. doi: 10.1126/science.1198469
26. Furusawa Y, Obata Y, Fukuda S, Endo TA, Nakato G, Takahashi D, et al. Commensal microbe-derived butyrate induces the differentiation of colonic regulatory T cells. Nature. (2013) 504:446–50. doi: 10.1038/nature12721
27. Fujimura KE, Sitarik AR, Havstad S, Lin DL, Levan S, Fadrosh D, et al. Neonatal gut microbiota associates with childhood multisensitized atopy and T-cell differentiation. Nat Med. (2016) 22:1187–91. doi: 10.1038/nm.4176
28. Vatanen T, Kostic AD, d'Hennezel E, Siljander H, Franzosa EA, Yassour M, et al. Variation in microbiome LPS immunogenicity contributes to autoimmunity in humans. Cell. (2016) 165:842–53. doi: 10.1016/j.cell.2016.04.007
29. Ainonen S, Tejesvi MV, Mahmud MR, Paalanne N, Pokka T, Li W, et al. Antibiotics at birth and later antibiotic courses: effects on gut microbiota. Pediatr Res. (2022) 91:154–62. doi: 10.1038/s41390-021-01494-7
30. Aloisio I, Quagliariello A, De Fanti S, Luiselli D, De Filippo C, Albanese D, et al. Evaluation of the effects of intrapartum antibiotic prophylaxis on newborn intestinal microbiota using a sequencing approach targeted to multi hypervariable 16S rDNA regions. Appl Microbiol Biotechnol. (2016) 100:5537–46. doi: 10.1007/s00253-016-7410-2
31. Aloisio I, Mazzola G, Corvaglia LT, Tonti G, Faldella G, Biavati B, et al. Influence of intrapartum antibiotic prophylaxis against group B Streptococcus on the early newborn gut composition and evaluation of the anti-Streptococcus activity of Bifidobacterium strains. Appl Microbiol Biotechnol. (2014) 98:6051–60. doi: 10.1007/s00253-014-5712-9
32. Azad M, Konya T, Persaud RR, Guttman DS, Chari RS, Field CJ, et al. Impact of maternal intrapartum antibiotics, method of birth and breastfeeding on gut microbiota during the first year of life: a prospective cohort study. BJOG Int J Obstet. Gynaecol. (2016) 123:983–93. doi: 10.1111/1471-0528.13601
33. Coker M, Hoen AG, Dade E, Lundgren S, Li Z, Wong AD, et al. Specific class of intrapartum antibiotics relates to maturation of the infant gut microbiota: a prospective cohort study. BJOG Int J Obstet. Gynaecol. (2020) 127:217–27. doi: 10.1111/1471-0528.15799
34. Corvaglia L, Tonti G, Martini S, Aceti A, Mazzola G, Aloisio I, et al. Influence of intrapartum antibiotic prophylaxis for group B streptococcus on gut microbiota in the first month of life. J Pediatr Gastroenterol Nutr. (2016) 62:304–8. doi: 10.1097/MPG.0000000000000928
35. Imoto N, Morita H, Amanuma F, Maruyama H, Watanabe S, and Hashiguchi N. Maternal antimicrobial use at delivery has a stronger impact than mode of delivery on bifidobacterial colonization in infants: a pilot study. J Perinatol. (2018) 38:1174–81. doi: 10.1038/s41372-018-0172-1
36. Jokela R, Korpela K, Jian C, Dikareva E, Nikkonen A, Saisto T, et al. Quantitative insights into effects of intrapartum antibiotics and birth mode on infant gut microbiota in relation to well-being during the first year of life. Gut Microbes. (2022) 14:1–16. doi: 10.1080/19490976.2022.2095775
37. Mazzola G, Murphy K, Ross RP, Di Gioia D, Biavati B, Corvaglia LT, et al. Early gut microbiota perturbations following intrapartum antibiotic prophylaxis to prevent group B streptococcal disease. PloS One. (2016) 11:1–11:e015752. doi: 10.1371/journal.pone.0157527
38. Nogacka A, Salazar N, Suárez M, Milani C, Arboleya S, Solís G, et al. Impact of intrapartum antimicrobial prophylaxis upon the intestinal microbiota and the prevalence of antibiotic resistance genes in vaginally delivered full-term neonates. Microbiome. (2017) , 5:1–10. doi: 10.1186/s40168-017-0313-3
39. Stearns JC, Simioni J, Gunn E, McDonald H, Holloway AC, Thabane L, et al. Intrapartum antibiotics for GBS prophylaxis alter colonization patterns in the early infant gut microbiome of low risk infants. Sci Rep. (2017) 7:1–7. doi: 10.1038/s41598-017-16606-9
40. Tapiainen T, Koivusaari P, Brinkac L, Lorenzi HA, Salo J, Renko M, et al. Impact of intrapartum and postnatal antibiotics on the gut microbiome and emergence of antimicrobial resistance in infants. Sci Rep. (2019) 9:. 1–11. doi: 10.1038/s41598-019-46964-5
41. Turta O, Selma-Royo M, Kumar H, Collado MC, Isolauri E, Salminen S, et al. Maternal intrapartum antibiotic treatment and gut microbiota development in healthy term infants. Neonatology. (2022) 119:. 93–102. doi: 10.1159/000519574
42. Chen YY, Zhao X, Moeder W, Tun HM, Simons E, Mandhane PJ, et al. Impact of maternal intrapartum antibiotics, and caesarean section with and without labour on bifidobacterium and other infant gut microbiota. Microorganisms. (2021) 9:1847. doi: 10.3390/microorganisms9091847
43. Qi Q, Wang L, Zhu Y, Li S, Gebremedhin MA, Wang B, et al. Unraveling the microbial symphony: impact of antibiotics and probiotics on infant gut ecology and antibiotic resistance in the first six months of life. Antibiotics. (2024) 13:602. doi: 10.3390/antibiotics13070602
44. Shao Y., Forster SC, Tsaliki E, Vervier K, Strang A, Simpson N, et al. Stunted microbiota and opportunistic pathogen colonization in caesarean-section birth. Nature. (2019) 574:117–21. doi: 10.1038/s41586-019-1560-1
45. Arboleya S, Saturio S, and Gueimonde M. Impact of intrapartum antibiotics on the developing microbiota: a review. Microbiome Res Rep. (2022) 1:22. doi: 10.20517/mrr.2022.04
46. Zimmermann P and Curtis N. Effect of intrapartum antibiotics on the intestinal microbiota of infants: a systematic review. Arch Dis Child. - Fetal Neonatal Ed. (2020) 05:201–8. doi: 10.1136/archdischild-2018-316659
47. Dierikx Y., Visser DH, Benninga MA, van Kaam AHLC, de Boer NKH, de Vries R, et al. The influence of prenatal and intrapartum antibiotics on intestinal microbiota colonisation in infants: A systematic review. J Infect. (2020) 81:190–204. doi: 10.1016/j.jinf.2020.05.002
48. Grech A, Collins CE, Holmes A, Lal R, Duncanson K, Taylor R, et al. Maternal exposures and the infant gut microbiome: a systematic review with meta-analysis. Gut Microbes. (2021) 13:1897210. doi: 10.1080/19490976.2021.1897210
49. Cox LM. et al., Altering the intestinal microbiota during a critical developmental window has lasting metabolic consequences. Cell. (2014) 158:705–21. doi: 10.1016/j.cell.2014.05.052
50. Franz A, Härtel C, Herting E, Kehl S, Gille C, Doubek K, et al. S2k-Leitlinie 024-020 “Prophylaxe der Neugeborenensepsis - frühe Form - durch Streptokokken der Gruppe B. AWMF Online. (2016) 221(3):122–9. doi: 10.1055/s-0043-105207
51. Graspeuntner S, Loeper N, Künzel S, Baines JF, and Rupp J. Selection of validated hypervariable regions is crucial in 16S-based microbiota studies of the female genital tract. Sci Rep. (2018) 8:1–7. doi: 10.1038/s41598-018-27757-8
52. Kozich JJ, Westcott SL, Baxter NT, Highlander SK, and Schloss PD. Development of a dual-index sequencing strategy and curation pipeline for analyzing amplicon sequence data on the miSeq illumina sequencing platform. Appl Environ Microbiol. (2013) 79:5112–20. doi: 10.1128/AEM.01043-13
53. Schloss PD, Westcott SL, Ryabin T, Hall JR, Hartmann M, Hollister EB, et al. Introducing mothur: open-source, platform-independent, community-supported software for describing and comparing microbial communities. Appl Environ Microbiol. (2009) 75:7537–41. doi: 10.1128/AEM.01541-09
54. Yoon S-H, Ha SM, Kwon S, Lim J, Kim Y, Seo H, et al. Introducing EzBioCloud: a taxonomically united database of 16S rRNA gene sequences and whole-genome assemblies. Int J Syst E Microbiol. (2017) 67:1613–7. doi: 10.1099/ijsem.0.001755
55. Edgar RC, Haas BJ, Clemente JC, Quince C, and Knight R. UCHIME improves sensitivity and speed of chimera detection. Bioinformatics. (2011) 27:. 2194–2200. doi: 10.1093/bioinformatics/btr381
56. Revelle W. psych: procedures for psychological, psychometric and personality research. Evanston, Illinois: Northwestern University (2023). Available at: https://CRAN.R-Project.org/package=psych (Accessed September 09, 2024).
57. Oksanen J, et al. vegan: community ecology package (2019). Available online at: https://CRAN.R-project.org/package=vegan (Accessed September 09, 2024).
58. Segata N, Izard J, Waldron L, Gevers D, Miropolsky L, Garrett WS, et al. Metagenomic biomarker discovery and explanation. Genome Biol. (2011) 12. doi: 10.1186/gb-2011-12-6-r60
59. Afgan E, Baker D, van den Beek M, Blankenberg D, Bouvier D, Čech M, et al. The Galaxy platform for accessible, reproducible and collaborative biomedical analyses: 2016 update. Nucleic Acids Res. (2016) 44:W3–W10. doi: 10.1093/nar/gkw343
60. Roberts DW. labdsv: ordination and multivariate analysis for ecology. (2013). Available online at: https://CRAN.R-project.org/package=labdsv (Accessed September 09, 2024).
61. Bäckhed F, Roswall J, Peng Y, Feng Q, Jia H, Kovatcheva-Datchary P, et al. Dynamics and stabilization of the human gut microbiome during the first year of life. Cell Host Microbe. (2015) 17:690–703. doi: 10.1016/j.chom.2015.04.004
62. Dominguez-Bello MG, Costello EK, Contreras M, Magris M, Hidalgo G, Fierer N, et al. Delivery mode shapes the acquisition and structure of the initial microbiota across multiple body habitats in newborns. Proc Natl Acad Sci. (2010) 107:11971–5. doi: 10.1073/pnas.1002601107
63. Bergström A, Skov TH, Bahl MI, Roager HM, Christensen LB, Ejlerskov KT, et al. Establishment of intestinal microbiota during early life: a longitudinal, explorative study of a large cohort of danish infants. Appl Environ Microbiol. (2014) 80:2889–900. doi: 10.1128/AEM.00342-14
64. Yatsunenko T, Rey FE, Manary MJ, Trehan I, Dominguez-Bello MG, Contreras M, et al. Human gut microbiome viewed across age and geography. Nature. (2012) 486:222–7. doi: 10.1038/nature11053
65. Sela DA and Mills DA. The marriage of nutrigenomics with the microbiome: the case of infant-associated bifidobacteria and milk. Am J Clin Nutr. (2014) 99(3):697S–703S. doi: 10.3945/ajcn.113.071795
66. LoCascio RG, Desai P, Sela DA, Weimer B, and Mills DA. Broad Conservation of Milk Utilization Genes in Bifidobacterium longum subsp. infantis as Revealed by Comparative Genomic Hybridization. Appl Environ Microbiol. (2010) 76(22):7373–81. doi: 10.1128/AEM.00675-10
67. Henrick BM, Rodriguez L, Lakshmikanth T, Pou C, Henckel E, Arzoomand A, et al. Bifidobacteria-mediated immune system imprinting early in life. Cell. (2021) 184:3884–3898.e11. doi: 10.1016/j.cell.2021.05.030
68. Meng D, Sommella E, Salviati E, Campiglia P, Ganguli K, Djebali K, et al. Indole-3-lactic acid, a metabolite of tryptophan, secreted by Bifidobacterium longum subspecies infantis is anti-inflammatory in the immature intestine. Pediatr Res. (2020) 88(2):209–17. doi: 10.1038/s41390-019-0740-x
69. Barondes SH, Castronovo V, Cooper DN, Cummings RD, Drickamer K, Feizi T, et al. Galectins: A family of animal β-galactoside-binding lectins. Cell. (1994) 76:597–8. doi: 10.1016/0092-8674(94)90498-7
70. Rabinovich GA, Ariel A, Hershkoviz R, Hirabayashi J, Kasai K-I, and Lider O. Specific inhibition of T-cell adhesion to extracellular matrix and proinflammatory cytokine secretion by human recombinant galectin-1. Immunology. (1999) 97:100–6. doi: 10.1046/j.1365-2567.1999.00746.x
71. Norling LV, Sampaio ALF, Cooper D, and Perretti M. Inhibitory control of endothelial galectin-1 on in vitro and in vivo lymphocyte trafficking. FASEB J. (2008) 22:682–90. doi: 10.1096/fj.07-9268com
72. van der Leij J, van den Berg A, Blokzijl T, Harms G, van Goor H, Zwiers P, et al. Dimeric galectin-1 induces IL-10 production in T-lymphocytes: an important tool in the regulation of the immune response. J Pathol. (2004) 204:511–8. doi: 10.1002/path.1671
73. Xiang Q, Yan X, Shi W, Li H, and Zhou K. Early gut microbiota intervention in premature infants: Application perspectives. J Adv Res. (2023) 51:. 59–72. doi: 10.1016/j.jare.2022.11.004
74. Collado MC, Donat E, Ribes-Koninckx C, Calabuig M, and Sanz Y. Imbalances in faecal and duodenal Bifidobacterium species composition in active and non-active coeliac disease. BMC Microbiol. (2008) 8:1–9. doi: 10.1186/1471-2180-8-232
75. De Palma G, Capilla A, Nova E, Castillejo G, Varea V, Pozo T, et al. Influence of milk-feeding type and genetic risk of developing coeliac disease on intestinal microbiota of infants: the PROFICEL study. PloS One. (2012) 7:1–10:e30791. doi: 10.1371/journal.pone.0030791
76. Rhoads JM, Collins J, Fatheree NY, Hashmi SS, Taylor CM, Luo M, et al. Infant colic represents gut inflammation and dysbiosis. J Pediatr. (2018) 203:55–61.e3. doi: 10.1016/j.jpeds.2018.07.042
77. Gao X, Jia R, Xie L, Kuang L, Feng L, and Wan C. Obesity in school-aged children and its correlation with Gut E.coli and Bifidobacteria: a case–control study. BMC Pediatr. (2015) 15:1–4. doi: 10.1186/s12887-015-0384-x
78. Huda MN, Lewis Z, Kalanetra KM, Rashid M, Ahmad SM, Raqib R, et al. Stool microbiota and vaccine responses of infants. Pediatrics. (2014) 134:e362–72. doi: 10.1542/peds.2013-3937
79. Pittayanon R, Lau JT, Leontiadis GI, Tse F, Yuan Y, Surette M, et al. Differences in gut microbiota in patients with vs without inflammatory bowel diseases: A systematic review. Gastroenterology. (2020) 158:930–946.e1. doi: 10.1053/j.gastro.2019.11.294
80. Kostic AD, Chun E, Robertson L, Glickman JN, Gallini CA, Michaud M, et al. Fusobacterium nucleatum potentiates intestinal tumorigenesis and modulates the tumor-immune microenvironment. Cell Host Microbe. (2013) 14:207–15. doi: 10.1016/j.chom.2013.07.007
81. Gevers D, Kugathasan S, Denson LA, Vázquez-Baeza Y, Van Treuren W, Ren B, et al. The treatment-naive microbiome in new-onset crohns disease. Cell Host Microbe. (2014) 15:382–92. doi: 10.1016/j.chom.2014.02.005
82. Park H, Li Z, Yang XO, Chang SH, Nurieva R, Wang YH, et al. A distinct lineage of CD4 T cells regulates tissue inflammation by producing interleukin 17. Nat Immunol. (2005) 6:1133–41. doi: 10.1038/ni1261
83. Honda K and Littman DR. The microbiota in adaptive immune homeostasis and disease. Nature. (2016) 535:75–84. doi: 10.1038/nature18848
84. Conti HR, Shen F, Nayyar N, Stocum E, Sun JN, Lindemann MJ, et al. Th17 cells and IL-17 receptor signaling are essential for mucosal host defense against oral candidiasis. J Exp Med. (2009) 206:299–311. doi: 10.1084/jem.20081463
85. Langrish CL, Chen Y, Blumenschein WM, Mattson J, Basham B, Sedgwick JD, et al. IL-23 drives a pathogenic T cell population that induces autoimmune inflammation. J Exp Med. (2005) 201:233–40. doi: 10.1084/jem.20041257
86. Jain R, Chen Y, Kanno Y, Joyce-Shaikh B, Vahedi G, Hirahara K, et al. Interleukin-23-induced transcription factor blimp-1 promotes pathogenicity of T helper 17 cells. Immunity. (2016) 44:131–42. doi: 10.1016/j.immuni.2015.11.009
87. Shellard EM, Rane SS, Eyre S, and Warren RB. Functional genomics and insights into the pathogenesis and treatment of psoriasis. Biomolecules. (2024) 14:1–20. doi: 10.3390/biom14050548
88. Wang HH, Dai YQ, Qiu W, Lu ZQ, Peng FH, Wang YG, et al. Interleukin-17-secreting T cells in neuromyelitis optica and multiple sclerosis during relapse. J Clin Neurosci. (2011) 18:1313–7. doi: 10.1016/j.jocn.2011.01.031
89. Wilburn AN, McAlees JW, Haslam DB, Graspeuntner S, Schmudde I, Laumonnier Y, et al. Delayed microbial maturation durably exacerbates th17-driven asthma in mice. Am J Respir Cell Mol Biol. (2023) 68:498–510. doi: 10.1165/rcmb.2022-0367OC
90. Hipp AV, Bengsch B, and Globig A-M. Friend or Foe – Tc17 cell generation and current evidence for their importance in human disease. Discov Immunol. (2023) 2:1–20. doi: 10.1093/discim/kyad010
91. Teunissen MBM, Yeremenko NG, Baeten DLP, Chielie S, Spuls PI, de Rie MA, et al. The IL-17A-producing CD8 + T-cell population in psoriatic lesional skin comprises mucosa-associated invariant T cells and conventional T cells. J Invest. Dermatol. (2014) 134:2898–907. doi: 10.1038/jid.2014.261
92. Globig AM, Hipp AV, Otto-Mora P, Heeg M, Mayer LS, Ehl S, et al. High-dimensional profiling reveals Tc17 cell enrichment in active Crohns disease and identifies a potentially targetable e signature. Nat Commun. (2022) 13:1–15. doi: 10.1038/s41467-022-31229-z
93. Ohnmacht C, Park JH, Cording S, Wing JB, Atarashi K, Obata Y, et al. The microbiota regulates type 2 immunity through RORγt + T cells. Science. (2015) 349:989–93. doi: 10.1126/science.aac4263
94. Sefik E, Geva-Zatorsky N, Oh S, Konnikova L, Zemmour D, McGuire AM, et al. Individual intestinal symbionts induce a distinct population of RORγ + regulatory T cells. Science. (2015) 349:993–7. doi: 10.1126/science.aaa9420
95. Beriou G, Costantino CM, Ashley CW, Yang L, Kuchroo VK, Baecher-Allan C, et al. IL-17–producing human peripheral regulatory T cells retain suppressive function. Blood. (2009) 113:4240–9. doi: 10.1182/blood-2008-10-183251
96. Nabhani Z, Dulauroy S, Marques R, Cousu C, Al Bounny S, Déjardin F, et al. A weaning reaction to microbiota is required for resistance to immunopathologies in the adult. Immunity. (2019) 50:1276–1288.e5. doi: 10.1016/j.immuni.2019.02.014
97. European Committee on Antimicrobial Susceptibility Testing. Breakpoint tab les for interpretation of MICs and zone diameters, Version 14.0. (2024) EUCAST Development Laboratory for Antimicrobial Susceptibility Testing of bacteria c/o Clinical Microbiology Central Hospital, Växjö Sweden.
98. Scasso S, Laufer J, Rodriguez G, Alonso JG, and Sosa CG. Vaginal group B streptococcus status during intrapartum antibiotic prophylaxis. Int J Gynecol. Obstet. (2015) 129:9–12. doi: 10.1016/j.ijgo.2014.10.018
99. McCoy JA, Elovitz MA, Alby K, Koelper NC, Nissim I, and Levine LD. Association of obesity with maternal and cord blood penicillin levels in women with group B streptococcus colonization. Obstet. Gynecol. (2020) 136:756–64. doi: 10.1097/AOG.0000000000004020
100. Janda JM and Abbott SL. 16S rRNA gene sequencing for bacterial identification in the diagnostic laboratory: pluses, perils, and pitfalls. J Clin Microbiol. (2007) 45(9):2761–4. doi: 10.1128/JCM.01228-07
101. Johnson JS, Spakowicz DJ, Hong BY, Petersen LM, Demkowicz P, Chen L, et al. Evaluation of 16S rRNA gene sequencing for species and strain-level microbiome analysis. Nat Commun. (2019) 10:5029. doi: 10.1038/s41467-019-13036-1
102. Graspeuntner S, Lupatsii M, van Zandbergen V, Dammann MT, Pagel J, Nguyen DN, et al. Infants < 90 days of age with late-onset sepsis display disturbances of the microbiome-immunity interplay. Infection. (2024) 11(3). doi: 10.1007/s15010-024-02396-6
103. Graspeuntner S, Lupatsii M, Dashdorj L, Rody A, Rupp J, Bossung V, et al. First-day-of-life rectal swabs fail to represent meconial microbiota composition and underestimate the presence of antibiotic resistance genes. Microbiol Spectr. (2023) 11(3). doi: 10.1128/spectrum.05254-22
104. Bossung V, Lupatsii M, Dashdorj L, Tassiello O, Jonassen S, Pagel J, et al. Timing of antimicrobial prophylaxis for cesarean section is critical for gut microbiome development in term born infants. Gut Microbes. (2022) 14:2038855. doi: 10.1080/19490976.2022.2038855
Keywords: intrapartum antibiotic prophylaxis (IAP), group B Streptococcus, early life microbiota, neonatal immunity, intestinal microbiome
Citation: Teuscher JL, Lupatsii M, Graspeuntner S, Jonassen S, Bringewatt A, Herting E, Stichtenoth G, Bossung V, Rupp J, Härtel C and Demmert M (2025) Persistent reduction of Bifidobacterium longum in the infant gut microbiome in the first year of age following intrapartum penicillin prophylaxis for maternal GBS colonization. Front. Immunol. 16:1540979. doi: 10.3389/fimmu.2025.1540979
Received: 06 December 2024; Accepted: 23 April 2025;
Published: 15 May 2025.
Edited by:
Luigi Marano, Academy of Applied Medical and Social Sciences, PolandReviewed by:
Yuying Liu, University of Texas Health Science Center at Houston, United StatesBabak Pakbin, Texas A and M University, United States
Copyright © 2025 Teuscher, Lupatsii, Graspeuntner, Jonassen, Bringewatt, Herting, Stichtenoth, Bossung, Rupp, Härtel and Demmert. This is an open-access article distributed under the terms of the Creative Commons Attribution License (CC BY). The use, distribution or reproduction in other forums is permitted, provided the original author(s) and the copyright owner(s) are credited and that the original publication in this journal is cited, in accordance with accepted academic practice. No use, distribution or reproduction is permitted which does not comply with these terms.
*Correspondence: Martin Demmert, bWFydGluLmRlbW1lcnRAdWtzaC5kZQ==
†These authors have contributed equally to this work and share first authorship