- 1Department of Orthopedics, The Second Hospital of Jilin University, Changchun, Jilin, China
- 2Joint International Research Laboratory of Ageing Active Strategy and Bionic Health in Northeast Asia of Ministry of Education, Jilin University, Changchun, Jilin, China
Orthopedic diseases pose significant challenges to public health due to their high prevalence, debilitating effects, and limited treatment options. Additionally, orthopedic tumors, such as osteosarcoma, chondrosarcoma, and Ewing sarcoma, further complicate the treatment landscape. Current therapies, including pharmacological treatments and joint replacement, address symptoms but fail to promote true tissue regeneration. Cell-based therapies, which have shown successful clinical results in cancers and other diseases, have emerged as a promising solution to repair damaged tissues and restore function in orthopedic diseases and tumors. This review discusses the advances and potential application of cell therapy for orthopedic diseases, with a particular focus on osteoarthritis, bone fractures, cartilage degeneration, and the treatment of orthopedic tumors. We explore the potential of mesenchymal stromal cells (MSCs), chondrocyte transplantation, engineered immune cells and induced pluripotent stem cells to enhance tissue regeneration by modulating the immune response and addressing inflammation. Ultimately, the integration of cutting-edge cell therapy, immune modulation, and molecular targeting strategies could revolutionize the treatment of orthopedic diseases and tumors, providing hope for patients seeking long-term solutions to debilitating conditions.
1 Introduction
1.1 Orthopedic diseases and the need for regenerative treatments
Orthopedic diseases, including osteoarthritis (OA), bone fractures, cartilage degeneration, and orthopedic tumors, are among the most prevalent conditions worldwide (1–3). These diseases significantly impact the quality of life of millions, particularly in aging populations. Osteoarthritis alone affects over 500 million people globally, with its incidence expected to rise as the population ages (1). Bone cancers such as osteosarcoma, chondrosarcoma, and Ewing sarcoma, although rarer, present significant clinical challenges in both diagnosis and treatment, requiring more specialized care. These diseases are characterized by the progressive deterioration of joints, cartilage, and bone, which leads to pain, reduced mobility, and impaired function (Table 1). Orthopedic tumors, though often less common, complicate treatment regimens with the need for aggressive therapies that include surgery, chemotherapy, and radiation. Current pharmacological treatments for pain management in degenerative joint diseases include nonsteroidal anti-inflammatory drugs (NSAIDs), opioids, corticosteroids, and disease-modifying osteoarthritis drugs (DMOADs). These agents aim to reduce pain and inflammation, although they do not address the underlying degeneration of joint tissues. While conventional treatments like pharmacological management and joint replacement surgeries help address symptoms, they fail to promote true tissue regeneration (4, 5). These treatments can be effective in alleviating pain and restoring some joint function, but they do not address the underlying tissue loss or repair the natural anatomy of the affected areas.
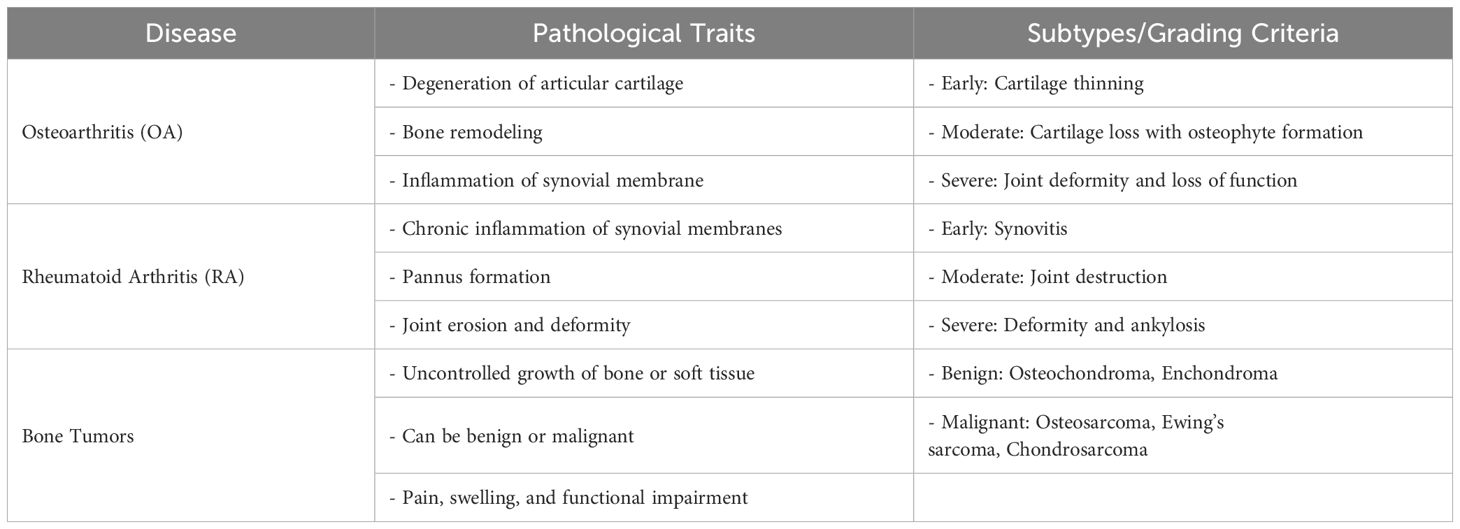
Table 1. Pathological traits, subtypes, and grading criteria of key diseases affecting joints and bones.
Regenerative medicine, particularly cell-based therapies, has gained attention as a promising alternative to traditional treatments. By harnessing the body’s own regenerative potential, these therapies aim to repair or regenerate damaged tissues and restore function (6, 7). This approach is being explored not only for degenerative diseases like osteoarthritis but also for orthopedic tumors, where regenerative treatments may aid in rebuilding bone tissue after tumor excision or radiation (8). However, despite significant advances, challenges remain in optimizing the efficacy and long-term outcomes of cell therapies in orthopedic diseases. The inability to fully regenerate damaged tissues, control inflammation, and address immune responses within the local tissue microenvironment remains a significant barrier to the successful application of these therapies (9, 10).
1.2 Engineered cell therapies
Cell therapy has emerged as a promising solution to address the limitations of conventional treatments for orthopedic diseases, including degenerative conditions and tumors. Mesenchymal stromal cells (MSCs), which have the ability to differentiate into multiple cell types, such as chondrocytes, osteoblasts, and adipocytes, are a key focus of research in this field (11, 12). MSCs can be sourced from various tissues, such as bone marrow, adipose tissue, and synovial fluid, and have shown potential in repairing cartilage defects, promoting bone healing, and reducing inflammation (10, 13, 14). Clinical studies have demonstrated the safety and feasibility of MSC-based therapies for conditions like osteoarthritis, with improvements in pain reduction and function observed in many cases (15). For orthopedic tumors, MSCs may also play a role in regenerating bone and cartilage after tumor resection. MSC-based treatments have been explored in preclinical models for osteosarcoma and other bone cancers, where they may assist in filling bone defects left after surgery and aid in the regeneration of normal bone tissue (16). Another promising approach is chondrocyte transplantation, where autologous chondrocytes are harvested, expanded, and re-implanted into damaged cartilage (17, 18). It has shown success in treating focal cartilage defects, although challenges remain in maintaining the functional integrity of the graft long-term (19). Furthermore, the engineering of immune cells, such as T-cells or macrophages, to enhance their regenerative potential and modulate the inflammatory response within the orthopedic disease microenvironment represents an exciting avenue for research (20). In orthopedic tumors, engineered immune cells also help in targeting residual tumor cells or modulating the immune response to prevent relapse (21). The key to success in these therapies lies in overcoming the inflammatory environment that characterizes many orthopedic diseases and finding ways to enhance tissue regeneration while preventing immune rejection (22, 23). Additionally, induced pluripotent stem cells (iPSCs) have gained attention in orthopedic disease therapy due to their unique ability to differentiate into any cell type, including chondrocytes and osteoblasts, providing the potential for autologous tissue repair. Although iPSCs offer significant promise, challenges in controlling differentiation and preventing tumorigenesis remain (24).
1.3 Key molecular pathways modulate the cell therapy effect in orthopedic diseases
A critical factor in the success of cell therapies for orthopedic diseases is the modulation of the immune response and the regulation of local inflammation (25, 26). The immune microenvironment of tissues affected by osteoarthritis, bone fractures, and tumors is often characterized by a pro-inflammatory state that hinders healing and tissue regeneration (27). Toll-like receptors (TLRs), a family of pattern recognition receptors that play a central role in innate immunity, are key regulators of this inflammatory response (28, 29). TLRs are expressed on various cells, including chondrocytes, macrophages, synoviocytes, and even cancer cells, and their activation leads to the production of pro-inflammatory cytokines and chemokines, contributing to cartilage degradation, bone resorption, and tumor progression (30, 31). Among the TLRs, TLR3 has emerged as a particularly interesting target in orthopedic disease therapy (32–34). TLR3 is primarily activated by double-stranded RNA, a pathogen-associated molecular pattern typically associated with viral infections (35). Upon activation, TLR3 initiates a signaling cascade involving the transcription factors NF-κB and interferon regulatory factor 3 (IRF3), which leads to the production of pro-inflammatory cytokines and type I interferons (36, 37). In orthopedic diseases and tumors, TLR3 plays a dual role by modulating the immune response and influencing tissue repair processes. Recent studies suggest that TLR3 signaling may be involved in the regulation of cartilage degeneration, bone remodeling, and immune responses to bone tumors. TLR3 activation promotes joint degeneration in osteoarthrosis (34).
2 Advances in cell-based therapies for orthopedic diseases
Cell-based therapies have shown considerable promise in the field of regenerative medicine, offering potential solutions for treating orthopedic diseases, such as osteoarthritis, cartilage degeneration, bone fractures, and even orthopedic tumors. These therapies aim to repair, regenerate, or replace damaged tissues by harnessing the regenerative properties of different cell types. This section explores several key approaches, including MSC therapy, chondrocyte-based approaches, engineered immune cell therapies and iPSCs, each of which presents unique benefits and challenges (Figure 1).
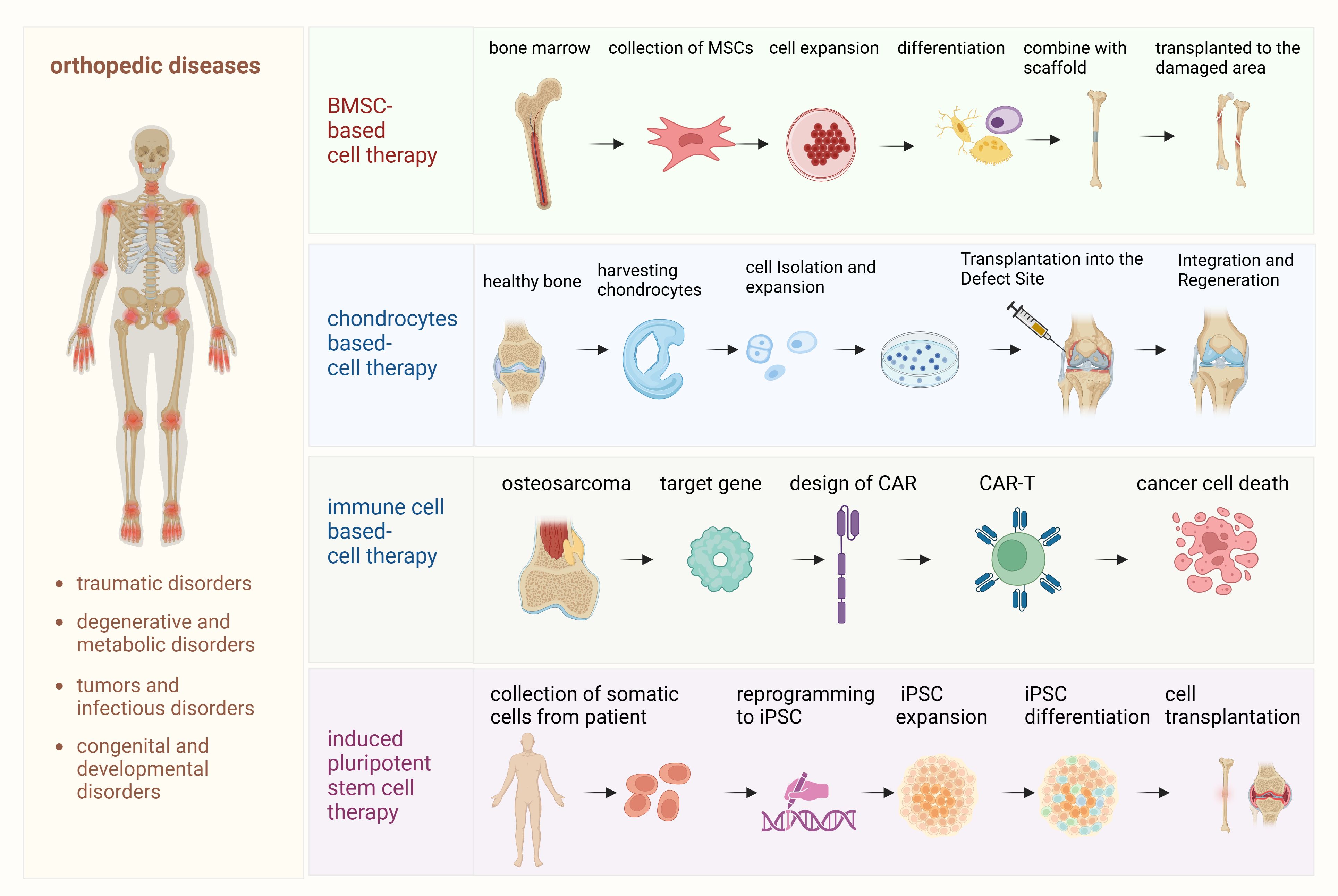
Figure 1. Detailed processes of cell-based therapies for orthopedic diseases. This diagram outlines the step-by-step processes involved in various cell-based therapies used for the treatment of orthopedic diseases. (1) Mesenchymal stem cell (MSC) therapy: This process begins with the isolation of MSCs from bone marrow or adipose tissue. The MSCs are then expanded in culture and differentiated into osteoblasts, chondrocytes, or other cell types, depending on the specific tissue regeneration needed. These differentiated cells are transplanted into the damaged bone or cartilage to promote healing and regeneration. (2) Chondrocyte therapy: In this process, autologous chondrocytes are harvested from the patient’s own cartilage, expanded in vitro, and then re-implanted into the damaged cartilage area. This therapy is designed to repair cartilage defects, particularly in cases of osteoarthritis or traumatic injuries. (3) Immune cell therapy (osteosarcoma and CAR-T therapy): This section illustrates the use of chimeric antigen receptor T (CAR-T) cell therapy specifically for osteosarcoma treatment. Patient-derived T cells are engineered to express CARs targeting tumor antigens on osteosarcoma cells. These engineered CAR-T cells are expanded and infused back into the patient, where they recognize and attack tumor cells, modulating the immune response and potentially improving outcomes for patients with osteosarcoma. (4) Induced pluripotent stem cell (iPSC) therapy: iPSCs are generated by reprogramming somatic cells, such as skin fibroblasts, back into pluripotent cells. These iPSCs are then differentiated into osteoblasts or chondrocytes to regenerate bone and cartilage tissues, offering a potential therapeutic approach for a wide range of orthopedic disorders.
2.1 Mesenchymal stroma cell therapy
In this review, the term mesenchymal stromal cells (MSCs) is used in accordance with the International Society for Cell and Gene Therapy (ISCT) nomenclature guidelines (38). MSCs are a population of cells with immunomodulatory and secretory functions, as opposed to ‘mesenchymal stem cells,’ which are defined by their demonstrated self-renewal and multilineage differentiation capabilities. Key markers used to identify MSCs, such as CD73, CD90, and CD105, are essential for characterizing MSCs in regenerative therapies. These markers help define the identity and functional properties of MSCs, ensuring their purity and effectiveness in clinical applications.
MSCs are a promising cell type for regenerative therapies due to their ability to differentiate into various mesodermal cell types, including chondrocytes, osteoblasts, and adipocytes. MSCs are primarily derived from adult tissues, such as bone marrow, adipose tissue, and synovial fluid, and can also be sourced from umbilical cord blood or placenta. Their multipotent differentiation capacity, coupled with their immunomodulatory properties, makes them an ideal candidate for treating orthopedic diseases. MSCs exerts therapeutic effects via paracrine signaling (39–41). When MSCs are transplanted into damaged tissues, they secrete a wide range of bioactive molecules, including growth factors, cytokines, and extracellular matrix components, that help modulate inflammation, stimulate tissue repair, and promote regeneration (42, 43). In osteoarthritis, for example, MSCs secrete anti-inflammatory cytokines such as IL-10 and TGF-β, which help to counteract the inflammatory mediators that contribute to cartilage degradation (44). Moreover, MSCs stimulate the proliferation and differentiation of endogenous progenitor cells, thereby enhancing tissue regeneration. In addition to their regenerative capabilities, MSCs are also capable of modulating the immune response. MSCs interact with various immune cells, such as T cells, B cells, and macrophages, to suppress inflammatory responses and reduce immune-mediated damage in tissues (12, 45, 46). This immunomodulatory effect is particularly important in autoimmune and inflammatory diseases like osteoarthritis, where chronic inflammation is a key driver of disease progression (47, 48). For example, bone marrow MSC (BMSC)-derived exosomes prevent osteoarthritis by regulating synovial macrophage polarization, promoting macrophages from pro-inflammatory (M1-like) phenotype to anti-inflammatory (M2-like) phenotype (39).
MSC-based therapies for orthopedic diseases have shown promising results in preclinical studies and early-phase clinical trials. MSCs are being investigated as a potential therapy for OA, with clinical trials in phase I/II showing pain relief and functional improvement (49, 50). However, challenges like low cell survival in inflammatory joints and limited therapeutic duration remain. Two clinical studies highlight the importance of stem cell delivery sites (44, 51). In one study, bilateral knee OA patients received BMSCs either via intra-articular (I.A.) injection or directly to subchondral bone lesions. After 15 years, 70% of knees in the I.A. group required total knee arthroplasty (TKA), compared to only 20% in the subchondral group, emphasizing the role of BML (bone marrow lesion)-targeted therapy in preventing OA progression (51). Another study on 140 late-stage OA patients compared subchondral BMSC delivery to TKA (44). Both groups had similar knee scores (~80) and TKA rates (~1%/year) after 15 years, suggesting BML regeneration delay knee replacement by over a decade. These findings underscore the potential of BMSC therapy and the critical role of subchondral bone in OA treatment (52).
For bone fractures, MSCs have been used to promote bone healing, particularly in cases of non-union fractures or those associated with large bone defects (53, 54). Hypoxic MSC-derived exosomes promote bone fracture healing by the transfer of miR-126 (40). Moreover, in orthopedic tumors, MSCs have shown potential for assisting in the regeneration of bone tissue after tumor excision or radiation, particularly in bone cancers like osteosarcoma. Osteosarcoma is a malignant bone tumor that arises from mesenchymal cells, which are also the source of MSCs. While MSCs play a role in bone regeneration, they also influence the tumor microenvironment in osteosarcoma. Metallothionein 1G (MT1G) regulates the cell’s antioxidant status and metal ion balance, influencing the proliferation and differentiation of MSCs, thereby affecting bone regeneration and repair processes, MT1G has been verified as a target for osteosarcoma (55). In addition, MSC-derived exosomes as targeted nanocarriers for Doxorubicin delivery, enhancing osteosarcoma therapy through the SDF1-CXCR4 axis (56). However, challenges remain in optimizing MSC therapy, including issues related to cell survival, engraftment, and long-term efficacy.
Allogeneic MSC transplantation offers several advantages, making it an attractive option for regenerative therapies (57, 58). One key benefit is the off-the-shelf availability of allogeneic MSCs, which eliminates the delays and complications associated with autologous MSC sourcing. Since these cells can be readily harvested from a donor, the need for patient-specific cell harvesting is bypassed, facilitating more timely treatments (58). In addition, allogeneic MSCs have immunomodulatory properties that enable them to modulate the immune response, which is crucial for reducing inflammation and promoting healing in various tissues. These properties allow them to be effective in treating diseases characterized by chronic inflammation, such as osteoarthritis, by helping to suppress immune-mediated tissue damage.
Furthermore, fetal-derived MSCs have garnered attention due to their higher proliferative capacity and enhanced differentiation potential compared to adult-derived MSCs (59). These cells are known to proliferate more rapidly and have a greater ability to differentiate into a wider range of cell types. As a result, fetal-derived MSCs present a promising alternative source for regenerative medicine, particularly in the context of orthopedic diseases where rapid tissue regeneration is essential (60). Their superior growth and differentiation potential could lead to more effective MSC-based therapies, improving the outcomes for patients with conditions such as osteoarthritis or bone fractures.
2.2 Chondrocyte-based cell therapy
Chondrocyte-based therapies involve the transplantation of autologous or allogenic chondrocytes to repair damaged cartilage (61, 62). It has been widely used in the treatment of focal cartilage defects, particularly in younger patients (63). The process typically involves harvesting healthy chondrocytes from non-weight-bearing regions of the joint, expanding them in vitro, and then re-implanting them into the damaged area, thus to restore the normal cartilage structure and function, thereby alleviating symptoms and preventing further joint degeneration (64). Autologous chondrocyte implantation (ACI) is a well-established procedure for treating large cartilage defects and has been shown to improve joint function and reduce pain in many patients (65). The key advantage of ACI is the use of the patient’s own cells, which minimizes the risk of immune rejection (66). Both ACI and matrix-assisted chondrocyte implantation (MACI) procedures have demonstrated long-term success in treating knee cartilage lesions related to osteoarthritis, helping to delay the need for arthroplasty (66).
However, technique has limitations, such as the need for a two-step procedure and the potential for donor site morbidity. Additionally, the newly generated cartilage may not fully replicate the mechanical properties of native cartilage, leading to a potential risk of graft failure or degeneration over time. Using scaffold materials combined with chondrocytes to provide structural support and promote the growth of new cartilage (67). These scaffolds are made from a variety of materials, including natural polymers, synthetic hydrogels, or even decellularized tissues, and they are designed to mimic the biomechanical properties of cartilage (68–70). Silk fibroin-based materials have been used for cartilage and osteochondral repair too (71). Advances in 3D bioprinting have enabled the creation of more complex scaffolds that better support chondrocyte growth and tissue regeneration (72). Additionally, the use of stroma/stem cells, such as MSCs or iPSCs, in combination with chondrocytes or scaffolds may offer a promising strategy to enhance cartilage regeneration and improve long-term outcomes.
A significant challenge in cartilage therapies is the in vitro culture and expansion of autologous chondrocytes. During culture, chondrocytes can dedifferentiate, losing their ability to produce cartilage-specific extracellular matrix components (73). Moreover, the need to expand sufficient numbers of cells for clinical applications remains a challenge, as does maintaining the differentiated state of the chondrocytes. One key question regarding autologous chondrocyte transplantation is whether it leads to true regeneration of articular cartilage or primarily facilitates tissue repair. While some studies report the formation of hyaline-like cartilage, others suggest that the newly formed tissue may more closely resemble fibrocartilage, which lacks the durability and biomechanical properties of genuine hyaline cartilage.
2.3 Engineered immune cell therapy
Engineered immune cells, such as chimeric antigen receptor T cells (CAR-T), CAR-natural killer (CAR-NK) cells, and CAR-macrophages (CAR-M), represent an exciting frontier in cell-based therapies. Traditionally used in cancer immunotherapy, these engineered immune cells have also shown potential for modulating inflammation and promoting tissue repair in orthopedic diseases. They focus on harnessing the immune system’s ability to target and eliminate specific cells, such as inflammatory macrophages or damaged tissue, and to enhance tissue repair processes.
Engineered immune cell therapy has been widely studied in orthopedic cancers such as osteosarcoma, chondrosarcoma, Ewing’s sarcoma, fibrosarcoma, etc (74, 75). Phase I trial (NCT02107963) of GD2 CAR-Ts (GD2-CAR.OX40.28.z.iC9), has demonstrated feasibility and safety of administration in children and young adults with osteosarcoma and neuroblastoma (76). GGD2-C7R CAR-T therapy clinical trial (NCT03635632) are conducted in solid tumors with GD2 target including relapsed Ewing Sarcoma and osteosarcoma (77). These trials highlight the promising potential of engineered immune cell therapies to treat difficult-to-treat orthopedic cancers.
The ability of CAR-T cells to specifically target inflammatory cells, such as activated macrophages or T cells, could be utilized to reduce inflammation and promote tissue healing in diseases like osteoarthritis. Additionally, CAR-T cells could be engineered to target specific molecules involved in cartilage degradation or bone resorption, such as matrix metalloproteinases (MMPs) or receptor activator of nuclear factor kappa-B ligand (RANKL). CAR-NK cells are engineered to enhance the cytotoxic activity of NK cells, and their application in orthopedic diseases could involve targeting and eliminating activated immune cells that contribute to chronic inflammation. Additionally, CAR-NK cells may promote tissue regeneration by enhancing the activity of other immune cells, such as MSCs, within the tissue microenvironment (78). In addition, macrophages play a dual role in inflammation and tissue repair. Macrophages could be polarized in vitro in two extreme phenotypes: the pro-inflammatory (M1-like) phenotype, associated with the release of pro-inflammatory cytokines and promotion of tissue destruction, and the anti-inflammatory (M2-like) phenotype, which supports tissue regeneration and repair (79). During the resolution phase of inflammation, pro-resolving macrophages play a crucial role in promoting tissue healing by producing growth factors such as vascular endothelial growth factor (VEGF) and platelet-derived growth factor (PDGF), which enhance angiogenesis and collagen synthesis (80, 81). Given their ability to modulate immune responses, CAR-macrophages could be explored as a potential approach to attenuate inflammation and support tissue regeneration in orthopedic diseases. Future studies may investigate their capacity to target pro-inflammatory cytokines or signaling pathways involved in cartilage degradation.
2.4 Induced pluripotent stem cells therapy
IPSCs offer an abundant and renewable source of cells for tissue engineering, serving as an appealing alternative to primary cells. With their remarkable plasticity and differentiation capabilities, iPSCs hold significant promise for cell-based therapies. Patient-specific iPSCs can be tailored to reduce the risk of autoimmune reactions, making them a nearly ideal candidate for regenerative medicine. Research on iPSCs in cartilage tissue engineering has highlighted their potential for functional cartilage repair and as valuable models for understanding cartilage-related diseases. For instance, iPSCs derived from somatic cells generate patient-specific stem cells for studying osteoarthritis and testing therapeutic agents (82, 83). Furthermore, iPSCs obtained from OA patients’ tissues can differentiate into cartilage, creating new opportunities for investigating cartilage pathology and treatment (84). Despite these advancements, no clinical trials using iPSC-derived cartilage cells for therapy have been reported. iPSCs share the proliferative and differentiation advantages of other stem cells while avoiding issues related to immune rejection and ethical concerns (24). However, further research is essential to advance the use of iPSC-derived chondrocytes in OA treatment and joint repair. In addition to cartilage repair, iPSC-based therapies are showing potential in the treatment of bone-related disorders. For example, iPSCs have been successfully differentiated into osteoblasts, the cells responsible for bone formation, and used in preclinical studies to promote bone regeneration in critical-sized bone defects. In a study involving a mouse model, iPSC-derived osteoblasts seeded onto bioengineered scaffolds not only enhanced bone regeneration but also demonstrated superior integration with the host tissue compared to traditional stem cell therapies (85). This approach highlights the versatility of iPSCs in addressing complex orthopedic challenges such as large bone defects and non-union fractures.
As outlined in the previous sections, a range of cell-based therapies has been developed to address orthopedic diseases, each offering distinct mechanisms and therapeutic potentials. To fully assess the advantages and limitations of these approaches, it is essential to conduct a comparative analysis of several key therapies, including MSC therapy, chondrocyte therapy, immune cell therapy (specifically CAR-T cell therapy for osteosarcoma), and iPSC therapy. This comparison enables a comprehensive evaluation of their effectiveness in promoting tissue regeneration, targeting tumors, and achieving optimal therapeutic outcomes (Table 2).
3 Immune modulation in orthopedic repair and regeneration
3.1 Balancing inflammation and regeneration
The immune microenvironment plays a crucial role in bone and cartilage repair and recovery following orthopedic tumor treatments (86, 87). Effective healing requires a well-regulated immune response to clear damaged cells and pathogens while promoting tissue regeneration. Acute inflammation initiates repair in the early stages of injury, but excessive or chronic inflammation can hinder recovery, leading to cartilage degradation, fibrosis, impaired bone healing, and delayed recovery after tumor surgery (88). Achieving a balance between inflammation and regeneration is critical in orthopedic conditions like osteoarthritis, bone fractures, cartilage degeneration, and tumor-related treatments. Key immune mediators, including cytokines and immune cells, influence this balance. Pro-inflammatory cytokines like TNF-α, IL-1β, and IL-6 are essential for initiating repair but can exacerbate tissue damage if persistently elevated (89). In contrast, anti-inflammatory cytokines such as IL-10 and TGF-β resolve inflammation and support tissue healing by promoting cell migration and differentiation. In orthopedic tumors, regulatory T cells (Tregs) suppress excessive inflammation, creating a regenerative microenvironment conducive to tissue healing after surgery (90). Modulating the immune response is therefore a promising strategy to enhance tissue repair and improve cell therapy outcomes.
3.2 The role of TLR3 in immune modulation
TLR3, a pattern recognition receptor, is critical for modulating inflammation and repair (91). Expressed on immune cells, chondrocytes, and synovial cells, TLR3 detects double-stranded RNA from pathogens or tissue damage (92, 93). Its activation triggers NF-κB and IRF3 signaling, leading to the production of pro-inflammatory cytokines and type I interferons. Activating TLR3 selectively in MSCs enhances their regenerative properties, improving integration and functionality in damaged tissues, thus promote joint degeneration in osteoarthritis (34).While TLR3-induced inflammation supports innate immunity, it also impacts tissue homeostasis and repair. In orthopedic diseases, TLR3 signaling plays a dual role. It contributes to osteoarthritis by promoting pro-inflammatory cytokine production, exacerbating cartilage degradation and inflammation. Targeting TLR3 by alleviate osteoarthritis (19). However, TLR3 also enhances the regenerative potential of MSCs, improving their migration and differentiation. Preconditioning MSCs with TLR3 agonists boosts chondrogenic differentiation and migration, which enhances cartilage repair. This dual role makes TLR3 an attractive target for therapeutic modulation.
4 Challenges and potential strategies of cell therapy for orthopedic diseases
Key challenges in cell therapy for orthopedic diseases include improving cell survival, enhancing engraftment within damaged tissues, and mitigating immunological and inflammatory responses that impair tissue repair, particularly in the complex tumor microenvironment of orthopedic cancers (94). Additionally, the lack of standardized protocols for cell production, expansion, and administration poses significant hurdles to ensuring consistency and reproducibility. Variability in cell sources, techniques, and delivery methods further complicates the translation of these therapies into clinical practice. Regulatory barriers remain another obstacle, with approval processes for cell-based therapies being both time-consuming and demanding due to stringent safety and efficacy requirements. To address these challenges, emerging technologies such as advanced biomaterials, nanocarriers, and bioengineering tools are being explored. Biomaterials like 3D-printed scaffolds, injectable hydrogels, and electrospun fibers provide mechanical support for transplanted cells while mimicking the natural extracellular matrix to promote cellular adhesion, proliferation, and differentiation (95–97). Nanotechnology further supports cell therapy by enabling targeted delivery of therapeutic agents or enhancing the bioactivity of transplanted cells. Nanoparticles and nanofibers encapsulate and deliver drugs, genes, or signaling molecules directly to the injury site, reducing off-target effects and improving therapeutic outcomes (98). For example, nanocarriers loaded with anti-inflammatory cytokines or immunosuppressive agents modulate the immune response to improve engraftment and tissue repair in inflammatory environments (99). Furthermore, preconditioning strategies are also emerging as a solution to improve cell survival and functionality. Exposing cells to hypoxic conditions, pro-inflammatory cytokines, or specific signaling molecules prior to transplantation can enhance their resilience in hostile microenvironments and increase their regenerative potential (100). Moreover, genetic engineering of cells, such as modifying MSCs to express anti-apoptotic or pro-regenerative factors, is being explored to further boost their therapeutic efficacy. Successful regenerative therapy for articular cartilage must achieve specific cellular and morphological characteristics, including the restoration of a well-organized cartilage structure, proper integration with subchondral bone, and the formation of extracellular matrix (ECM) components like collagen type II and proteoglycans. These features are essential for the functionality and durability of the regenerated cartilage in joint repair.
5 Conclusions and perspectives
In summary, cell-based therapies have made significant strides in the treatment of orthopedic diseases, offering potential solutions for conditions such as osteoarthritis, cartilage degeneration, bone fractures, and orthopedic tumors like osteosarcoma, chondrosarcoma, and Ewing sarcoma. These therapies promote tissue repair, restore function, and reduce inflammation, providing a promising alternative to conventional treatments that primarily manage symptoms. MSCs, chondrocyte transplantation, and engineered immune cell therapies have shown great potential in preclinical studies and early clinical trials. Despite these advancements, several challenges remain in translating these therapies into widespread clinical use.
Looking forward, a multi-faceted approach combining advanced cell therapies with immune modulation, molecular targeting, and emerging technologies is essential to improving clinical outcomes in orthopedic diseases and tumors. However, several unresolved questions remain that need to be addressed in future research:
1. How can we improve cell survival after transplantation? Ensuring the survival and function of transplanted cells, especially in hostile environments like the tumor microenvironment, is challenging.
2. How can we balance inflammation and regeneration? Inflammation is crucial for repair but excessive immune responses hinder regeneration.
3. How can we scale up cell therapies for clinical use? Standardized protocols for producing and administering cell therapies are lacking.
4. What molecular pathways can enhance regeneration? Pathways like TLR3 are linked to inflammation and repair, but their roles are not fully understood.
In conclusion, while cell-based therapies hold immense promise for the treatment of orthopedic diseases and tumors, overcoming the remaining challenges will require a multidisciplinary approach. The integration of immune modulation, advanced technologies, and a deeper understanding of molecular pathways will be key to enhancing the efficacy of these therapies and achieving better clinical outcomes. The future of orthopedic disease and tumor treatment will likely involve a personalized, tailored approach, combining the best of cell therapy, immune modulation, and emerging technologies to provide optimal solutions for patients.
Author contributions
JW: Writing – original draft. SX: Resources, Visualization, Writing – review & editing. BC: Resources, Validation, Writing – review & editing. YQ: Conceptualization, Investigation, Supervision, Writing – review & editing.
Funding
The author(s) declare that no financial support was received for the research and/or publication of this article.
Acknowledgments
We thank BioRender for generation of figures in this article.
Conflict of interest
The authors declare that the research was conducted in the absence of any commercial or financial relationships that could be construed as a potential conflict of interest.
Generative AI statement
The author(s) declare that no Generative AI was used in the creation of this manuscript.
Publisher’s note
All claims expressed in this article are solely those of the authors and do not necessarily represent those of their affiliated organizations, or those of the publisher, the editors and the reviewers. Any product that may be evaluated in this article, or claim that may be made by its manufacturer, is not guaranteed or endorsed by the publisher.
Abbreviations
ACI, Autologous chondrocyte implantation; BML, bone marrow lesion; BMSC, bone marrow mesenchymal stem cell; CAR-M, CAR-macrophages; CAR-NK, CAR-natural killer; CAR-T, chimeric antigen receptor T cells; IRF3, interferon regulatory factor 3; iPSCs, induced pluripotent stem cells; MACI, matrix-assisted chondrocyte implantation; MMPs, matrix metalloproteinases; MSCs, Mesenchymal stromal cells; MT1G, Metallothionein 1G; OA, osteoarthritis; PDGF, platelet-derived growth factor; RANKL, receptor activator of nuclear factor kappa-B ligand; TKA, total knee arthroplasty; TLRs, Toll-like receptors; Tregs, regulatory T cells; VEGF, vascular endothelial growth factor.
References
1. Yao Q, Wu X, Tao C, Gong W, Chen M, Qu M, et al. Osteoarthritis: pathogenic signaling pathways and therapeutic targets. Signal Transduct Target Ther. (2023) 8:56. doi: 10.1038/s41392-023-01330-w
2. Yu S, Yao X. Advances on immunotherapy for osteosarcoma. Mol Cancer. (2024) 23:192. doi: 10.1186/s12943-024-02105-9
3. Fine N, Lively S, Seguin CA, Perruccio AV, Kapoor M, Rampersaud R. Intervertebral disc degeneration and osteoarthritis: a common molecular disease spectrum. Nat Rev Rheumatol. (2023) 19:136–52. doi: 10.1038/s41584-022-00888-z
4. Nabizadeh Z, Nasrollahzadeh M, Daemi H, Baghaban Eslaminejad M, Shabani AA, Dadashpour M, et al. Micro- and nanotechnology in biomedical engineering for cartilage tissue regeneration in osteoarthritis. Beilstein J Nanotechnol. (2022) 13:363–89. doi: 10.3762/bjnano.13.31
5. Cardoneanu A, Macovei LA, Burlui AM, Mihai IR, Bratoiu I, Rezus II, et al. Temporomandibular joint osteoarthritis: pathogenic mechanisms involving the cartilage and subchondral bone, and potential therapeutic strategies for joint regeneration. Int J Mol Sci. (2022) 24(1):171. doi: 10.3390/ijms24010171
6. Anastasio AT, Paniagua A, Diamond C, Ferlauto HR, Fernandez-Moure JS. Nanomaterial nitric oxide delivery in traumatic orthopedic regenerative medicine. Front Bioeng Biotechnol. (2020) 8:592008. doi: 10.3389/fbioe.2020.592008
7. Im GI, Henrotin Y. Regenerative medicine for early osteoarthritis. Ther Adv Musculoskelet Dis. (2023) 15:1759720X231194813. doi: 10.1177/1759720X231194813
8. Chang X, Ma Z, Zhu G, Lu Y, Yang J. New perspective into mesenchymal stem cells: Molecular mechanisms regulating osteosarcoma. J Bone Oncol. (2021) 29:100372. doi: 10.1016/j.jbo.2021.100372
9. Goudarzi R, Dehpour AR, Partoazar A. Nanomedicine and regenerative medicine approaches in osteoarthritis therapy. Aging Clin Exp Res. (2022) 34:2305–15. doi: 10.1007/s40520-022-02199-5
10. Giorgino R, Albano D, Fusco S, Peretti GM, Mangiavini L, Messina C. Knee osteoarthritis: epidemiology, pathogenesis, and mesenchymal stem cells: what else is new? An update. Int J Mol Sci. (2023) 24(7):6405. doi: 10.3390/ijms24076405
11. Lan T, Luo M, Wei X. Mesenchymal stem/stromal cells in cancer therapy. J Hematol Oncol. (2021) 14:195. doi: 10.1186/s13045-021-01208-w
12. Wang Y, Fang J, Liu B, Shao C, Shi Y. Reciprocal regulation of mesenchymal stem cells and immune responses. Cell Stem Cell. (2022) 29:1515–30. doi: 10.1016/j.stem.2022.10.001
13. Le H, Xu W, Zhuang X, Chang F, Wang Y, Ding J. Mesenchymal stem cells for cartilage regeneration. J Tissue Eng. (2020) 11:2041731420943839. doi: 10.1177/2041731420943839
14. Huang J, Liu Q, Xia J, Chen X, Xiong J, Yang L, et al. Modification of mesenchymal stem cells for cartilage-targeted therapy. J Transl Med. (2022) 20:515. doi: 10.1186/s12967-022-03726-8
15. Yu H, Huang Y, Yang L. Research progress in the use of mesenchymal stem cells and their derived exosomes in the treatment of osteoarthritis. Ageing Res Rev. (2022) 80:101684. doi: 10.1016/j.arr.2022.101684
16. Sarhadi VK, Daddali R, Seppanen-Kaijansinkko R. Mesenchymal stem cells and extracellular vesicles in osteosarcoma pathogenesis and therapy. Int J Mol Sci. (2021) 22(20):11035. doi: 10.3390/ijms222011035
17. Dekker TJ, Aman ZS, DePhillipo NN, Dickens JF, Anz AW, LaPrade RF. Chondral lesions of the knee: an evidence-based approach. J Bone Joint Surg Am. (2021) 103:629–45. doi: 10.2106/JBJS.20.01161
18. Fuggle NR, Cooper C, Oreffo ROC, Price AJ, Kaux JF, Maheu E, et al. Alternative and complementary therapies in osteoarthritis and cartilage repair. Aging Clin Exp Res. (2020) 32:547–60. doi: 10.1007/s40520-020-01515-1
19. Salzmann GM, Ossendorff R, Gilat R, Cole BJ. Autologous minced cartilage implantation for treatment of chondral and osteochondral lesions in the knee joint: an overview. Cartilage. (2021) 13:1124S–36S. doi: 10.1177/1947603520942952
20. Zhu J, Simayi N, Wan R, Huang W. CAR T targets and microenvironmental barriers of osteosarcoma. Cytotherapy. (2022) 24:567–76. doi: 10.1016/j.jcyt.2021.12.010
21. Lu Y, Zhang J, Chen Y, Kang Y, Liao Z, He Y, et al. Novel immunotherapies for osteosarcoma. Front Oncol. (2022) 12:830546. doi: 10.3389/fonc.2022.830546
22. Yu T, Jiang W, Wang Y, Zhou Y, Jiao J, Wu M. Chimeric antigen receptor T cells in the treatment of osteosarcoma (Review). Int J Oncol. (2024) 64(4):40. doi: 10.3892/ijo.2024.5628
23. Clemente O, Ottaiano A, Di Lorenzo G, Bracigliano A, Lamia S, Cannella L, et al. Is immunotherapy in the future of therapeutic management of sarcomas? J Transl Med. (2021) 19:173. doi: 10.1186/s12967-021-02829-y
24. Moradi S, Mahdizadeh H, Saric T, Kim J, Harati J, Shahsavarani H, et al. Research and therapy with induced pluripotent stem cells (iPSCs): social, legal, and ethical considerations. Stem Cell Res Ther. (2019) 10:341. doi: 10.1186/s13287-019-1455-y
25. Sanchez-Lopez E, Coras R, Torres A, Lane NE, Guma M. Synovial inflammation in osteoarthritis progression. Nat Rev Rheumatol. (2022) 18:258–75. doi: 10.1038/s41584-022-00749-9
26. Knights AJ, Redding SJ, Maerz T. Inflammation in osteoarthritis: the latest progress and ongoing challenges. Curr Opin Rheumatol. (2023) 35:128–34. doi: 10.1097/BOR.0000000000000923
27. Nedunchezhiyan U, Varughese I, Sun AR, Wu X, Crawford R, Prasadam I. Obesity, inflammation, and immune system in osteoarthritis. Front Immunol. (2022) 13:907750. doi: 10.3389/fimmu.2022.907750
28. Fitzgerald KA, Kagan JC. Toll-like receptors and the control of immunity. Cell. (2020) 180:1044–66. doi: 10.1016/j.cell.2020.02.041
29. Kawai T, Ikegawa M, Ori D, Akira S. Decoding Toll-like receptors: Recent insights and perspectives in innate immunity. Immunity. (2024) 57:649–73. doi: 10.1016/j.immuni.2024.03.004
30. Cao LL, Kagan JC. Targeting innate immune pathways for cancer immunotherapy. Immunity. (2023) 56:2206–17. doi: 10.1016/j.immuni.2023.07.018
31. Chou WC, Rampanelli E, Li X, Ting JP. Impact of intracellular innate immune receptors on immunometabolism. Cell Mol Immunol. (2022) 19:337–51. doi: 10.1038/s41423-021-00780-y
32. Tominari T, Matsumoto C, Tanaka Y, Shimizu K, Takatoya M, Sugasaki M, et al. Roles of toll-like receptor signaling in inflammatory bone resorption. Biol (Basel). (2024) 13(9):692. doi: 10.3390/biology13090692
33. Qian Y, Chu G, Zhang L, Wu Z, Wang Q, Guo JJ, et al. M2 macrophage-derived exosomal miR-26b-5p regulates macrophage polarization and chondrocyte hypertrophy by targeting TLR3 and COL10A1 to alleviate osteoarthritis. J Nanobiotechnology. (2024) 22:72. doi: 10.1186/s12951-024-02336-4
34. Stolberg-Stolberg J, Boettcher A, Sambale M, Stuecker S, Sherwood J, Raschke M, et al. Toll-like receptor 3 activation promotes joint degeneration in osteoarthritis. Cell Death Dis. (2022) 13:224. doi: 10.1038/s41419-022-04680-5
35. Liu Z, Garcia Reino EJ, Harschnitz O, Guo H, Chan YH, Khobrekar NV, et al. Encephalitis and poor neuronal death-mediated control of herpes simplex virus in human inherited RIPK3 deficiency. Sci Immunol. (2023) 8:eade2860. doi: 10.1126/sciimmunol.ade2860
36. Chen Y, Lin J, Zhao Y, Ma X, Yi H. Toll-like receptor 3 (TLR3) regulation mechanisms and roles in antiviral innate immune responses. J Zhejiang Univ Sci B. (2021) 22:609–32. doi: 10.1631/jzus.B2000808
37. Crossley MP, Song C, Bocek MJ, Choi JH, Kousouros JN, Sathirachinda A, et al. R-loop-derived cytoplasmic RNA-DNA hybrids activate an immune response. Nature. (2023) 613:187–94. doi: 10.1038/s41586-022-05545-9
38. Viswanathan S, Shi Y, Galipeau J, Krampera M, Leblanc K, Martin I, et al. Mesenchymal stem versus stromal cells: International Society for Cell & Gene Therapy (ISCT(R)) Mesenchymal Stromal Cell committee position statement on nomenclature. Cytotherapy. (2019) 21:1019–24. doi: 10.1016/j.jcyt.2019.08.002
39. Liu Y, Chen J, Liang H, Cai Y, Li X, Yan L, et al. Human umbilical cord-derived mesenchymal stem cells not only ameliorate blood glucose but also protect vascular endothelium from diabetic damage through a paracrine mechanism mediated by MAPK/ERK signaling. Stem Cell Res Ther. (2022) 13:258. doi: 10.1186/s13287-022-02927-8
40. Liu W, Li L, Rong Y, Qian D, Chen J, Zhou Z, et al. Hypoxic mesenchymal stem cell-derived exosomes promote bone fracture healing by the transfer of miR-126. Acta Biomater. (2020) 103:196–212. doi: 10.1016/j.actbio.2019.12.020
41. Teixeira-Pinheiro LC, Toledo MF, Nascimento-Dos-Santos G, Mendez-Otero R, Mesentier-Louro LA, Santiago MF. Paracrine signaling of human mesenchymal stem cell modulates retinal microglia population number and phenotype in vitro. Exp Eye Res. (2020) 200:108212. doi: 10.1016/j.exer.2020.108212
42. Holan V, Hermankova B, Krulova M, Zajicova A. Cytokine interplay among the diseased retina, inflammatory cells and mesenchymal stem cells - a clue to stem cell-based therapy. World J Stem Cells. (2019) 11:957–67. doi: 10.4252/wjsc.v11.i11.957
43. Song N, Scholtemeijer M, Shah K. Mesenchymal stem cell immunomodulation: mechanisms and therapeutic potential. Trends Pharmacol Sci. (2020) 41:653–64. doi: 10.1016/j.tips.2020.06.009
44. Hernigou P, Delambre J, Quiennec S, Poignard A. Human bone marrow mesenchymal stem cell injection in subchondral lesions of knee osteoarthritis: a prospective randomized study versus contralateral arthroplasty at a mean fifteen year follow-up. Int Orthop. (2021) 45:365–73. doi: 10.1007/s00264-020-04571-4
45. Sarsenova M, Issabekova A, Abisheva S, Rutskaya-Moroshan K, Ogay V, Saparov A. Mesenchymal stem cell-based therapy for rheumatoid arthritis. Int J Mol Sci. (2021) 22(21):11592. doi: 10.3390/ijms222111592
46. Xu W, Yang Y, Li N, Hua J. Interaction between mesenchymal stem cells and immune cells during bone injury repair. Int J Mol Sci. (2023) 24(19):14484. doi: 10.3390/ijms241914484
47. Marinescu CI, Preda MB, Burlacu A. A procedure for in vitro evaluation of the immunosuppressive effect of mouse mesenchymal stem cells on activated T cell proliferation. Stem Cell Res Ther. (2021) 12:319. doi: 10.1186/s13287-021-02344-3
48. Kushioka J, Chow SK, Toya M, Tsubosaka M, Shen H, Gao Q, et al. Bone regeneration in inflammation with aging and cell-based immunomodulatory therapy. Inflammation Regener. (2023) 43:29. doi: 10.1186/s41232-023-00279-1
49. Gupta PK, Chullikana A, Rengasamy M, Shetty N, Pandey V, Agarwal V, et al. Efficacy and safety of adult human bone marrow-derived, cultured, pooled, allogeneic mesenchymal stromal cells (Stempeucel(R)): preclinical and clinical trial in osteoarthritis of the knee joint. Arthritis Res Ther. (2016) 18:301. doi: 10.1186/s13075-016-1195-7
50. Lu L, Dai C, Zhang Z, Du H, Li S, Ye P, et al. Treatment of knee osteoarthritis with intra-articular injection of autologous adipose-derived mesenchymal progenitor cells: a prospective, randomized, double-blind, active-controlled, phase IIb clinical trial. Stem Cell Res Ther. (2019) 10:143. doi: 10.1186/s13287-019-1248-3
51. Hernigou P, Bouthors C, Bastard C, Flouzat Lachaniette CH, Rouard H, Dubory A. Subchondral bone or intra-articular injection of bone marrow concentrate mesenchymal stem cells in bilateral knee osteoarthritis: what better postpone knee arthroplasty at fifteen years? A randomized study. Int Orthop. (2021) 45:391–9. doi: 10.1007/s00264-020-04687-7
52. Jiang Y. Osteoarthritis year in review 2021: biology. Osteoarthritis Cartilage. (2022) 30:207–15. doi: 10.1016/j.joca.2021.11.009
53. Deguchi M, Tsuji S, Katsura D, Kasahara K, Kimura F, Murakami T. Current overview of osteogenesis imperfecta. Medicina (Kaunas). (2021) 57(5):464. doi: 10.3390/medicina57050464
54. Zhang S, Lee Y, Liu Y, Yu Y, Han I. Stem cell and regenerative therapies for the treatment of osteoporotic vertebral compression fractures. Int J Mol Sci. (2024) 25(9):4979. doi: 10.3390/ijms25094979
55. Zheng S, Cheng X, Ke S, Zhang L, Wu H, He D, et al. Bioinformatics analysis and validation of mesenchymal stem cells related gene MT1G in osteosarcoma. Aging (Albany NY). (2024) 16:8155–70. doi: 10.18632/aging.205809
56. Wei H, Chen F, Chen J, Lin H, Wang S, Wang Y, et al. Mesenchymal stem cell derived exosomes as nanodrug carrier of doxorubicin for targeted osteosarcoma therapy via SDF1-CXCR4 axis. Int J Nanomedicine. (2022) 17:3483–95. doi: 10.2147/IJN.S372851
57. Alvarado-Velez M, Enam SF, Mehta N, Lyon JG, LaPlaca MC, Bellamkonda RV. Immuno-suppressive hydrogels enhance allogeneic MSC survival after transplantation in the injured brain. Biomaterials. (2021) 266:120419. doi: 10.1016/j.biomaterials.2020.120419
58. Ankrum JA, Ong JF, Karp JM. Mesenchymal stem cells: immune evasive, not immune privileged. Nat Biotechnol. (2014) 32:252–60. doi: 10.1038/nbt.2816
59. Gencer EB, Lor YK, Abomaray F, El Andaloussi S, Pernemalm M, Sharma N, et al. Transcriptomic and proteomic profiles of fetal versus adult mesenchymal stromal cells and mesenchymal stromal cell-derived extracellular vesicles. Stem Cell Res Ther. (2024) 15:77. doi: 10.1186/s13287-024-03683-7
60. Boss AL, Brooks AES, Chamley LW, James JL. Influence of culture media on the derivation and phenotype of fetal-derived placental mesenchymal stem/stromal cells across gestation. Placenta. (2020) 101:66–74. doi: 10.1016/j.placenta.2020.09.002
61. Mobasheri A, Kalamegam G, Musumeci G, Batt ME. Chondrocyte and mesenchymal stem cell-based therapies for cartilage repair in osteoarthritis and related orthopaedic conditions. Maturitas. (2014) 78:188–98. doi: 10.1016/j.maturitas.2014.04.017
62. Brittberg M, Lindahl A, Nilsson A, Ohlsson C, Isaksson O, Peterson L. Treatment of deep cartilage defects in the knee with autologous chondrocyte transplantation. N Engl J Med. (1994) 331:889–95. doi: 10.1056/NEJM199410063311401
63. Yue L, Lim R, Owens BD. Latest advances in chondrocyte-based cartilage repair. Biomedicines. (2024) 12(6):1367. doi: 10.3390/biomedicines12061367
64. Mumme M, Barbero A, Miot S, Wixmerten A, Feliciano S, Wolf F, et al. Nasal chondrocyte-based engineered autologous cartilage tissue for repair of articular cartilage defects: an observational first-in-human trial. Lancet. (2016) 388:1985–94. doi: 10.1016/S0140-6736(16)31658-0
65. Mistry H, Connock M, Pink J, Shyangdan D, Clar C, Royle P, et al. Autologous chondrocyte implantation in the knee: systematic review and economic evaluation. Health Technol Assess. (2017) 21:1–294. doi: 10.3310/hta21060
66. Colombini A, Libonati F, Lopa S, Peretti GM, Moretti M, de Girolamo L. Autologous chondrocyte implantation provides good long-term clinical results in the treatment of knee osteoarthritis: a systematic review. Knee Surg Sports Traumatol Arthrosc. (2023) 31:2338–48. doi: 10.1007/s00167-022-07030-2
67. Vinikoor T, Dzidotor GK, Le TT, Liu Y, Kan HM, Barui S, et al. Injectable and biodegradable piezoelectric hydrogel for osteoarthritis treatment. Nat Commun. (2023) 14:6257. doi: 10.1038/s41467-023-41594-y
68. Liu D, Wang X, Gao C, Zhang Z, Wang Q, Pei Y, et al. Biodegradable piezoelectric-conductive integrated hydrogel scaffold for repair of osteochondral defects. Adv Mater. (2024) 36:e2409400. doi: 10.1002/adma.202409400
69. Zhang FX, Liu P, Ding W, Meng QB, Su DH, Zhang QC, et al. Injectable Mussel-Inspired highly adhesive hydrogel with exosomes for endogenous cell recruitment and cartilage defect regeneration. Biomaterials. (2021) 278:121169. doi: 10.1016/j.biomaterials.2021.121169
70. Sun K, Tao C, Wang DA. Scaffold-free approaches for the fabrication of engineered articular cartilage tissue. BioMed Mater. (2022) 17(2). doi: 10.1088/1748-605X/ac51b9
71. Zhou Z, Cui J, Wu S, Geng Z, Su J. Silk fibroin-based biomaterials for cartilage/osteochondral repair. Theranostics. (2022) 12:5103–24. doi: 10.7150/thno.74548
72. Sun T, Feng Z, He W, Li C, Han S, Li Z, et al. Novel 3D-printing bilayer GelMA-based hydrogel containing BP,beta-TCP and exosomes for cartilage-bone integrated repair. Biofabrication. (2023) 16. doi: 10.1088/1758-5090/ad04fe
73. Charlier E, Deroyer C, Ciregia F, Malaise O, Neuville S, Plener Z, et al. Chondrocyte dedifferentiation and osteoarthritis (OA). Biochem Pharmacol. (2019) 165:49–65. doi: 10.1016/j.bcp.2019.02.036
74. Li S, Zhang H, Shang G. Current status and future challenges of CAR-T cell therapy for osteosarcoma. Front Immunol. (2023) 14:1290762. doi: 10.3389/fimmu.2023.1290762
75. Hidalgo L, Somovilla-Crespo B, Garcia-Rodriguez P, Morales-Molina A, Rodriguez-Milla MA, Garcia-Castro J. Switchable CAR T cell strategy against osteosarcoma. Cancer Immunol Immunother. (2023) 72:2623–33. doi: 10.1007/s00262-023-03437-z
76. Kaczanowska S, Murty T, Alimadadi A, Contreras CF, Duault C, Subrahmanyam PB, et al. Immune determinants of CAR-T cell expansion in solid tumor patients receiving GD2 CAR-T cell therapy. Cancer Cell. (2024) 42:35–51.e38. doi: 10.1016/j.ccell.2023.11.011
77. Strauss SJ, Berlanga P, McCabe MG. Emerging therapies in Ewing sarcoma. Curr Opin Oncol. (2024) 36:297–304. doi: 10.1097/CCO.0000000000001048
78. Saxena Y, Routh S, Mukhopadhaya A. Immunoporosis: role of innate immune cells in osteoporosis. Front Immunol. (2021) 12:687037. doi: 10.3389/fimmu.2021.687037
79. Mills CD, Kincaid K, Alt JM, Heilman MJ, Hill AM. M-1/M-2 macrophages and the Th1/Th2 paradigm. J Immunol. (2000) 164:6166–73. doi: 10.4049/jimmunol.164.12.6166
80. Spiller KL, Anfang RR, Spiller KJ, Ng J, Nakazawa KR, Daulton JW, et al. The role of macrophage phenotype in vascularization of tissue engineering scaffolds. Biomaterials. (2014) 35:4477–88. doi: 10.1016/j.biomaterials.2014.02.012
81. Wynn TA, Vannella KM. Macrophages in tissue repair, regeneration, and fibrosis. Immunity. (2016) 44:450–62. doi: 10.1016/j.immuni.2016.02.015
82. Diekman BO, Christoforou N, Willard VP, Sun H, Sanchez-Adams J, Leong KW, et al. Cartilage tissue engineering using differentiated and purified induced pluripotent stem cells. Proc Natl Acad Sci U S A. (2012) 109:19172–7. doi: 10.1073/pnas.1210422109
83. Lietman SA. Induced pluripotent stem cells in cartilage repair. World J Orthop. (2016) 7:149–55. doi: 10.5312/wjo.v7.i3.149
84. Nam Y, Rim YA, Lee J, Ju JH. Current therapeutic strategies for stem cell-based cartilage regeneration. Stem Cells Int. (2018) 2018:8490489. doi: 10.1155/2018/8490489
85. Lamande SR, Ng ES, Cameron TL, Kung LHW, Sampurno L, Rowley L, et al. Modeling human skeletal development using human pluripotent stem cells. Proc Natl Acad Sci U S A. (2023) 120:e2211510120. doi: 10.1073/pnas.2211510120
86. Li M, Yin H, Yan Z, Li H, Wu J, Wang Y, et al. The immune microenvironment in cartilage injury and repair. Acta Biomater. (2022) 140:23–42. doi: 10.1016/j.actbio.2021.12.006
87. Wu CL, Harasymowicz NS, Klimak MA, Collins KH, Guilak F. The role of macrophages in osteoarthritis and cartilage repair. Osteoarthritis Cartilage. (2020) 28:544–54. doi: 10.1016/j.joca.2019.12.007
88. Lu X, Dai S, Huang B, Li S, Wang P, Zhao Z, et al. Exosomes loaded a smart bilayer-hydrogel scaffold with ROS-scavenging and macrophage-reprogramming properties for repairing cartilage defect. Bioact Mater. (2024) 38:137–53. doi: 10.1016/j.bioactmat.2024.04.017
89. Fernandes TL, Gomoll AH, Lattermann C, Hernandez AJ, Bueno DF, Amano MT. Macrophage: A potential target on cartilage regeneration. Front Immunol. (2020) 11:111. doi: 10.3389/fimmu.2020.00111
90. Li F, Tang H, Luo X, Li X, Luo K, Liu S, et al. Interaction gene set between osteoclasts and regulatory CD4(+) T cells can accurately predict the prognosis of patients with osteosarcoma. Cancer Sci. (2023) 114:3014–26. doi: 10.1111/cas.15821
91. Justynski O, Bridges K, Krause W, Forni MF, Phan QM, Sandoval-Schaefer T, et al. Apoptosis recognition receptors regulate skin tissue repair in mice. Elife. (2023) 12:e2207050. doi: 10.7554/eLife.86269
92. Lu R, Qu Y, Wang Z, He Z, Xu S, Cheng P, et al. TBK1 pharmacological inhibition mitigates osteoarthritis through attenuating inflammation and cellular senescence in chondrocytes. J Orthop Translat. (2024) 47:207–22. doi: 10.1016/j.jot.2024.06.001
93. Wan Z, Wang X, Fu Z, Ma Y, Dai G, Gong X, et al. Toll-like receptor activation regulates the paracrine effect of adipose-derived mesenchymal stem cells on reversing osteoarthritic phenotype of chondrocytes. Mol Biol Rep. (2024) 51:550. doi: 10.1007/s11033-024-09499-1
94. Yin P, Jiang Y, Fang X, Wang D, Li Y, Chen M, et al. Cell-based therapies for degenerative musculoskeletal diseases. Adv Sci (Weinh). (2023) 10:e2207050. doi: 10.1002/advs.202207050
95. Han X, Saiding Q, Cai X, Xiao Y, Wang P, Cai Z, et al. Intelligent vascularized 3D/4D/5D/6D-printed tissue scaffolds. Nanomicro Lett. (2023) 15:239. doi: 10.1007/s40820-023-01187-2
96. Jing Z, Yuan W, Wang J, Ni R, Qin Y, Mao Z, et al. Simvastatin/hydrogel-loaded 3D-printed titanium alloy scaffolds suppress osteosarcoma via TF/NOX2-associated ferroptosis while repairing bone defects. Bioact Mater. (2024) 33:223–41. doi: 10.1016/j.bioactmat.2023.11.001
97. Li P, Fu L, Liao Z, Peng Y, Ning C, Gao C, et al. Chitosan hydrogel/3D-printed poly(epsilon-caprolactone) hybrid scaffold containing synovial mesenchymal stem cells for cartilage regeneration based on tetrahedral framework nucleic acid recruitment. Biomaterials. (2021) 278:121131. doi: 10.1016/j.biomaterials.2021.121131
98. Zou J, Yang W, Cui W, Li C, Ma C, Ji X, et al. Therapeutic potential and mechanisms of mesenchymal stem cell-derived exosomes as bioactive materials in tendon-bone healing. J Nanobiotechnology. (2023) 21:14. doi: 10.1186/s12951-023-01778-6
99. Ashrafizadeh M, Zarrabi A, Bigham A, Taheriazam A, Saghari Y, Mirzaei S, et al. (Nano)platforms in breast cancer therapy: Drug/gene delivery, advanced nanocarriers and immunotherapy. Med Res Rev. (2023) 43:2115–76. doi: 10.1002/med.21971
Keywords: cell therapy, orthopedic diseases, immunotherapy, MSCs, iPSCs
Citation: Wang J, Xu S, Chen B and Qin Y (2025) Advances in cell therapy for orthopedic diseases: bridging immune modulation and regeneration. Front. Immunol. 16:1567640. doi: 10.3389/fimmu.2025.1567640
Received: 13 February 2025; Accepted: 24 March 2025;
Published: 10 April 2025.
Edited by:
Philippe Saas, Etablissement Français du Sang AuRA, FranceReviewed by:
Jose Gregorio Marchan Alvarez, Karolinska Institutet (KI), SwedenCopyright © 2025 Wang, Xu, Chen and Qin. This is an open-access article distributed under the terms of the Creative Commons Attribution License (CC BY). The use, distribution or reproduction in other forums is permitted, provided the original author(s) and the copyright owner(s) are credited and that the original publication in this journal is cited, in accordance with accepted academic practice. No use, distribution or reproduction is permitted which does not comply with these terms.
*Correspondence: Yanguo Qin, UWlueWFuZ3VvMTk3NkAxMjYuY29t