- 1Institute of Molecular Pathogenesis, Friedrich-Loeffler-Institut, Jena, Germany
- 2Faculty of Biological Sciences, Friedrich-Schiller-University, Jena, Germany
- 3Institute of Bacterial Infections and Zoonoses, Friedrich-Loeffler-Institut, Jena, Germany
- 4Reproductive Biotechnology, TUM School of Life Sciences, Technical University of Munich, Freising, Germany
- 5Center for Infection Prevention (ZIP), Technical University of Munich, Freising, Germany
Avian γδ T lymphocytes are highly abundant in the intestinal mucosa and play a critical role in immune defense against infectious diseases in chickens. However, their specific contributions to infection control remain poorly understood. To investigate the role of γδ T cells and their possible compensation, we studied wild-type and γδ T cell knockout chickens following infection with Salmonella Enteritidis. Bacterial loads in the liver, cecal content, and cecal wall were quantified. Immune cell populations in blood, spleen, and cecum were analyzed using flow cytometry. Immune gene transcription in sorted γδ (TCR1+) and TCR1- cell subsets as well as cecal tissue was measured by RT-qPCR. Strikingly, chickens lacking γδ T cells had significantly higher bacterial loads in the liver and more extensive Salmonella invasion in the cecal wall during the early stages of infection compared to wild-type birds. In blood, infected γδ T cell knockout chickens displayed a significantly increased percentage of CD25+ NK-like cells. In both blood and tissue, infected wild-type chickens demonstrated an increased absolute number of CD8αα+hi γδ T cells (CD4-). Conversely, γδ T cell knockout chickens exhibited an augmented cell count of a CD8αα+hiCD4-TCR1- cell population after infection, which might include αβ T cells. At 7 days post infection (dpi), gene expression analysis revealed elevated transcription of the activation marker IL-2Rα and proinflammatory cytokines (IL-17A, IFN-γ) in CD8αα+hiCD4-TCR1- cells from γδ T cell knockout chickens compared to CD8αα+hi γδ T cells from wild-type birds. By 12 dpi, these differences diminished as transcription levels increased in γδ T cells of wild-type animals. Our findings demonstrate that γδ T cells play a role in early immune protection against Salmonella Enteritidis infection in chickens. In later stages of the infection, the γδ T cells and their functions appear to be replaced by other cells.
1 Introduction
Salmonella (S.) spp. is a predominant cause of foodborne gastrointestinal infection within the European Union, with 65,208 confirmed cases of human salmonellosis reported in 2022 (1). Notably, S. Enteritidis was identified as prominent pathogen, accounting for around 67% of reported cases and posing a global health concern. The main sources are poultry-derived products, mainly eggs and egg products but also chicken meat (1). For this reason, the primary aim of Salmonella control is to prevent these organisms from entering the food chain. This is achieved by stringent hygiene regimes, novel vaccine programs and selection of chicken breeds with enhanced colonization resistance. However, susceptibility to S. infection varies with age in poultry, with chicks younger than 3 days showing increased vulnerability due to their not yet fully developed gut microbiota and immune system (2–5). This underlines the crucial need for in-depth research into the avian immune response to Salmonella (S.) infection for improving chicken protection and minimizing human exposure to the pathogen in the future.
S. Enteritidis is now as ever highly prevalent in poultry, especially in laying hens. This serovar is able to induce both extensive intestinal colonization as well as pronounced invasion and potential systemic spread to different organs including the reproductive tract. The avian immune response in cecum, spleen, bursa of Fabricius and blood is marked by a significant influx of immune cells. Heterophils act as initial effector response, whereas macrophages modulate innate immunity and facilitate development of adaptive immune mechanisms (6–11).
T lymphocytes are key players in mediating immune responses against a broad spectrum of pathogens. Common to their mammalian counterparts, avian T cells exhibit heterodimeric T cell receptors (TCR), which consist of a constant and variable region with either alpha and beta chains (αβ T cells) or gamma and delta chains (γδ T cells) (12, 13). The functionality of αβ T cells, defined by the two co-receptors CD4 and CD8, is well-documented in many species, including chickens (14–16). CD4+ αβ T cells are activated upon recognition of pathogen-derived peptides presented by MHC II molecules, leading to a humoral immune response (14), or function as regulatory T cells (CD4+CD25+FoxP3+), contributing to immune tolerance (16, 17). CD8+ αβ T cells exert cytotoxic activity following antigen recognition via MHC I molecules. Avian CD8+ T cells are split into two subsets, characterized by surface expression of either CD8αα homo- or CD8αβ heterodimers (15). While the functional distinctions between these subsets still remain postulated, CD8αβ expression is presumed to mark cytotoxic T cells (15, 18), whereas cells with the CD8αα homodimer have been suggested to contribute to T cell activation and immune regulation (19).
In chickens, γδ T cells represent up to 50% of the peripheral T cells (20, 21), while in humans, mouse and rats, the frequency of γδ T cells is relatively low, ranging from 1 to 10% (22). Different species-specific subpopulations of γδ T cells have been described, depending on their localization, antigen expression and cytokine production. In chickens, γδ T cells (TCR1+ cells) are categorized into CD8α+ (high, CD8α+hi), CD8α+ (dim), and CD8α- (negative) populations, displaying substantial subset and functional heterogeneity (23).
The γδ T cells are of special importance at mucosal sites of the body (24–26). They stimulate early immune defense mechanisms (27, 28) and may serve as a bridge between the innate and adaptive reactions (29). The γδ T cells are crucial for immune regulation, tissue healing, inflammation, and protection against intra- and extracellular pathogens. Moreover, γδ T cells have memory abilities and produce a variety of cytokines (30–35).
Despite the high frequency of γδ T cells in chickens, our understanding of their functions is limited. Studies have shown a rapid expansion of γδ T cells in response to bacterial and viral infections (36, 37). Avian γδ T cells can produce cytokines and interferons such as IL-2, IL-17, IL-10, and IFN-γ (37–39), and demonstrate cytotoxic activities (40).
In Salmonella-infected chickens, distinct CD8α-expressing γδ T cell subsets quickly appear at inflammatory sites (9, 41). Especially the percentage of CD8αα+hi γδ T cells expand (37, 41). These cells express specific activation markers including CD25 and can produce immune effectors, such as IFN-γ and IL-2 (23, 42). However, it remains unclear whether γδ T cells counteract transepithelial invasion and dissemination of Salmonella, and whether different γδ T cell subsets exert distinct functions during the immune defense against Salmonella in chickens.
Progress in understanding the immunological role of γδ T cells was limited by the lack of genetically modified avian models, which restricted the study of distinct immune cell populations. Therefore, a chicken line lacking γδ T cells has been developed. Complete absence of γδ T cells was achieved through genomic deletion of the constant region of the T cell receptor γ (TCR Cy-/-). The TCR Cy-/- chickens show no pathological phenotype and are indistinguishable from wild-type birds in both behavior and appearance (43).
The absence of γδ T cells can have divergent consequences at different stages of the host immune response. Mice with γδ T cell deficiencies (due to gene targeting or antibody-mediated depletion) exhibit inadequate early innate resistance to infections such as L. monocytogenes and C. albicans. This has been attributed to the reduced production of IFN-γ and IL-17A and a decreased recruitment of innate immune cells (44–47). Other authors report an exaggerated inflammatory response after L. monocytogenes infection of γδ T cell-deficient mice, probably mediated by reduced regulation of macrophage homeostasis, T cell activity and reduced IL-10 production (31, 48–50). Remarkably, in uninfected mice, other immune cells, such as αβ T cells, appear to occupy the niches left by γδ T cells and partially compensate for their functions (51, 52).
To date, there have been no studies to determine whether and to what extent γδ T cells have a decisive influence on the course of the infection and the recovery of chickens after Salmonella exposure. In addition, it remains to be clarified whether the immunological functions of γδ T cells can somehow be compensated for in Salmonella-infected TCR Cy-/- chickens. Studies on γδ T cell function after Salmonella infection of γδ T cell knockout mice have produced conflicting results. Weintraub et al. (53) reported that intraepithelial γδ T cells (γδ IELs) and other intestinal γδ T cells do not play a significant role in controlling Salmonella invasion or replication in the mouse intestine. Conversely, Edelblum et al. (54) demonstrated that mice lacking γδ IELs exhibited increased Salmonella invasion and severity of systemic salmonellosis.
In this study, we set to address the role of γδ T cells in protecting chickens against the Salmonella infection by utilizing TCR Cy-/- chickens lacking γδ T cells and wild-type chickens. Information on the dynamics of Salmonella dissemination in infected TCR Cy-/- and wild-type animals, alongside comparative analyses of the immunological responses in blood and tissues should be gained, contributing to a better understanding of γδ T cells and their possible redundancy in avian immune defense. Our data suggest that γδ T cells contribute to the control of S. Enteritidis infection in young chickens, and identified compensatory mechanisms in knockout chickens that maintain immune protection post infection by partially replacing functional properties of γδ T cells.
2 Materials and methods
2.1 Chickens
White Leghorn chickens with different genetic modifications were used for the experiments: Chickens with a homozygous knockout of the constant region of the T cell receptor-γ chain (TCR Cγ-/- chickens (43)) and their non-modified hatch-siblings (wild-type chickens). The embryonated chicken eggs were produced at the TUM Animal Research Center, and hatched at the facilities of the Friedrich-Loeffler-Institut Jena. Genotyping was performed as described before (43). The animals were kept in cages. To effectively prevent cross-contamination between the groups, the individual groups were reared and kept in separate negative pressure rooms under standardized conditions and in accordance with the European Community Guidelines for Animal Welfare throughout the experiments. Antibiotic-free commercial feed in powder and pellet form and drinking water were available ad libitum. The study was conducted in strict compliance with the German Animal Welfare Act. The protocol was approved by the Ethics Committee for Animal Experiments and Animal Welfare of the State of Thuringia, Germany (registration number: BFI-20-001). Furthermore, the study was conducted under the supervision of the institutional animal welfare officer.
2.2 Bacteria
Oral infection of the wild-type and TCR Cy-/- animals was carried out with a rifampicin (R) resistant variant of the comprehensively characterized strain Salmonella (S.) enterica subspecies enterica serovar Enteritidis 147 (SE147, phage type 4). The potential of this strain to colonize the cecum and invade internal organs of chickens has been reported in detail (9, 55–57). The strain had been stored in a cryobank system (Mast Diagnostica, Reinfeld, Germany) at -20°C. The infection doses were estimated by measuring the absorbance at 600 nm using a calibration graph and subsequently confirmed by plate counting on nutrient agar (SIFIN, Berlin, Germany).
2.3 Experimental design
Two animal experiments were conducted separately (A, B). In each experiment, two groups of chickens were included, one group of wild-type and one group of TCR Cy-/- chickens. In experiment A, the chickens (64 wild-type and 37 TCR Cγ-/- animals) were orally infected with S. Enteritidis 147 R at a dose of 2 x 107 cfu/bird on day 3 of age. Oral administration was performed by instillation into the birds’ crop using syringes with an attached flexible tube. The volume of bacterial suspension used was 0.1 mL per bird. Three-day-old chickens of the control group (experiment B; 58 wild-type and 45 TCR Cγ-/- animals) were mock-infected with 0.1 mL phosphate-buffered saline (PBS).
At eight distinct time points post infection, 1–13 birds per group were sacrificed and dissected. From each animal, the cecal content and tissue samples of spleen, liver, cecum and peripheral blood (EDTA coated Monovette®, Sarstedt, Nümbrecht, Germany) were taken. Samples were either analyzed immediately (cecal content, cecal tissue, liver, blood, spleen) or frozen and stored in liquid nitrogen or RNA-later (Qiagen, Hilden, Germany) for subsequent analysis (cecal tissue).
2.4 Bacteriology
Bacterial counts of S. Enteritidis 147 R were determined in cecal contents and in liver of all birds at each time point by a standard plating method (56–59). Briefly, homogenized liver tissue and cecal content were diluted in PBS, plated on deoxycholate-citrate agar (SIFIN) supplemented with rifampicin (100 µg/mL) and incubated at 37°C for 18–24 h to detect bacterial colonization. Liver tissue and cecal content were also pre-enriched in buffered peptone water (SIFIN), incubated at 37°C for 18–24 h and streaked onto deoxycholate-citrate agar supplemented with rifampicin (SIFIN).
2.5 Immune cell isolation
Immune cells were isolated from peripheral blood (PBMCs) for cell sorting and analysis of natural killer-like cells (NK-like cells) as well as from spleen and cecum for determination of absolute (leukocytes and T cell subsets) and relative (NK-like cells) immune cell numbers by flow cytometry.
To obtain the PBMCs, 1 mL EDTA-blood was mixed with 1 mL PBS plus 1% bovine serum albumin (BSA: Bio&Sell, Feucht, Germany; PBS+) and transferred on Pancoll animal separation medium (1.077 g/mL; Pan-Biotech, Aidenbach, Germany; Pancoll), followed by centrifugation at 800 x g for 20 min. The cell layer at the interface between PBS+ and Pancoll was transferred to a new centrifuge tube and washed with 5 mL PBS+ at 400 x g for 15 min. After removal of the supernatant, the cells were adjusted to 5 x 106 cells/mL in PBS+ for subsequent flow cytometric staining and cell sorting.
To isolate splenic cells, the spleen was first weighed. The tissue was then placed in a petri dish containing 1 mL of sterile PBS+ and minced into small pieces using scalpel and forceps. Excess connective tissue was discarded. The remaining cell suspension was homogenized by slowly pipetting up and down using a 23G cannula (BD Bioscience, Heidelberg, Germany) with a syringe (B. Braun SE, Melsungen, Germany). After adding 1 mL fresh PBS+, the cell suspension was overlaid on Pancoll (1.077 g/mL) in a 12 mL tube and centrifuged at 800 x g for 20 min. The cell layer was transferred to a new centrifuge tube and washed with 5 mL PBS+ at 400 x g for 10 min. After removal of the supernatant, the cells were resuspended in 1 mL PBS+ and further diluted if necessary (to 5 x 106 cells/mL) for subsequent flow cytometric staining.
To obtain the immune cells from the cecum, the tubular cecum was cut open, spread out for area measurement and washed thoroughly in PBS+ to remove the remaining cecal content. Epithelial and lamina propria cells were carefully scraped from the remaining muscular and serosa layers, the latter were subsequently removed. One mL of PBS+ was added, and the cell material was mechanically shredded with a scalpel. Next, 1 mL of collagenase type V solution from Clostridium histolyticum (2 mg/mL; Merck, Darmstadt, Germany) was added to the cells and incubated for 30 min at 37°C on a shaker. The cells were then gently pipetted three times through a 1 mL pipette tip, transferred on 2 mL of Pancoll (1.077 g/mL; Pan-Biotech) and centrifuged at 800 x g for 20 min. The cell layer was transferred to a new tube and washed twice with 3 mL of fresh PBS+ at 400 x g for 10 min. Finally, the cell pellet was resuspended in 1 mL PBS+ and further diluted if necessary (to 5 x 106 cells/mL) for subsequent flow cytometric staining.
2.6 Flow cytometry
To assess immune cell numbers in whole blood, PBMCs, spleen and cecum, we conducted flow cytometric analyses as previously described (60–62).
Four distinct antibody panels were employed for immune cell characterization (Table 1). Antibody mix 1 was designed to detect absolute numbers of leukocytes (thrombocytes, monocytes, T cells, B cells, granulocytes) of infected and mock-infected animals (61). Antibody mix 2 comprised different monoclonal antibodies to distinguish absolute numbers of γδ (TCR1+) and TCR1- cell subpopulations and to quantify CD25 activation marker expression on these cells in infected and mock-infected animals. The antibody combination in mix 3 was used to identify absolute counts of αβ T cell subsets (Vβ1/Vβ2) and to phenotypically characterize the TCR1- cells in more detail. Antibody mix 4 was used to examine the percentage of NK-like cells in PBMC, spleen and cecum of infected wild-type and TCR Cy-/- chickens only.
For the flow cytometric determination of the absolute immune cell numbers in whole blood, spleen and cecum, 20 µL of the whole blood samples were diluted in 980 µL PBS+ and used for cell labelling. Fifty microliters of the diluted whole blood or 50 µl of isolated immune cell suspensions from spleen and cecum were mixed with 20 µL of the appropriate monoclonal antibody combination (antibody mixes 1-3, Table 1) directly into Trucount tubes (BD Biosciences).
To assess the relative number of NK-like cells, 50 µL of the isolated immune cells from PBMC, spleen and cecum were incubated with 20 µL of the antibody mix 4 in a FACS tube without beads (Falcon®, Corning Inc., Corning, NY, USA).
All samples were incubated in the dark for 60 min. Shortly before flow cytometric analysis, 300 µL PBS+ and DAPI (1 µg/mL; Sigma, Taufkirchen, Germany) were added to each sample. Measurements were performed on a FACSCanto II (BD Biosciences) equipped with 488 nm, 633 nm and 405 nm lasers. Prior to the study, optimal antibody concentrations and compensations were determined for each antibody in the different mixes. Appropriate fluorescence-minus one controls were conducted to confirm specificity of antibody binding.
Data acquisition was performed using the FACSDiva v9.0.1 software (BD Biosciences). For absolute cell analysis either 10,000 (mix 1) or 30,000 (mix 2 and 3) Trucount beads were acquired together with the cells. For mix 1, all CD45+ (UM16-6, IgG2a) cells as well as thrombocytes (K1+, IgG1; Prof. Kaspers) and monocytes (KUL01+, IgG1) were measured and data stored (61). For the mixes 2 and 3, the storage gate contained all γδ T cells (TCR1+, IgG1) and TCR1- cells (mix 2), Vβ1+ and Vβ2+ T cells (TCR2+ and TCR3+, IgG1; mix 3) as well as the low and high-positive CD8α+ (CT-8, IgG1), CD8β+ (EP42, IgG2a), CD4+ (CT-4, IgG1) and CD25+ (28-4, IgG3) T lymphocytes. For mix 4, 30,000-150,000 (PBMC), 30,000-50,000 (spleen) and 10,000-30,000 (cecum) CD45+ cells were acquired and all events recorded per sample.
Data analysis was performed by using the FlowJo software (version 10, FlowJo, BD Biosciences). Before immune cell analysis, doublets were excluded (FSC-A versus FSC-W) and viable cells selected (DAPI-negative cells).
For mix 1 (Supplementary Figure S1), absolute numbers of thrombocytes and monocytes were measured from the K1/KUL01 dot plot against CD45. CD45-positive leukocytes were further used to distinguish Bu1-positive B lymphocytes (AV-20) and TCR1/CD4/CD8-positive T lymphocytes. Blood heterophils were selected by back-gating from the CD45+ versus SSC dot plot (61, 63).
In mix 2, distinct subsets of T cells were identified based on their expression or absence of the TCR1 marker (64). These included the CD8α-TCR1+, CD8α+hiTCR1+ and TCR1- cell populations, as depicted on the CD8α versus TCR1 dot plot. The TCR1- cells were further separated into CD8α+hiCD4- and CD8α+CD4+ T cell subsets by plotting CD8α against CD4. The CD8α+hiTCR1+ and CD8α+hiCD4-TCR1- cell populations were further differentiated into the CD8αα homo- and CD8αβ heterodimer subsets. All lymphocyte populations were back-gated against SSC/FSC (Supplementary Figure S2).
For mix 3 (Supplementary Figure S3), T cell populations were gated based on their TCR2 and TCR3 expression as described for mix 2, respectively. For the CD8αα+hiCD4-TCR1-, CD8αα+hiTCR1+ and CD8αα+hiCD4-TCR2+/TCR3+ lymphocyte subpopulations of the mixes 2-3, the absolute numbers of CD25-expressing cells were additionally determined.
Absolute cell numbers were calculated using following equation:
Absolute cell counts of isolated tissue cells were calculated for 1 mL and at the appropriate dilution and expressed as cells per 1 g of spleen or per 1 cm2 of cecum.
In mix 4, the relative frequency of CD8α+ leukocytes (CD45+) lacking lineage-specific markers for T or B cells, referred to as NK-like cells in this study, was determined by plotting CD8α against CD45 (65). The lymphocyte subpopulation was confirmed by back-gating using an SSC/FSC dot plot. Cells expressing lineage-specific markers for TCR+ cells, B cells, CD4+ T cells, monocytes and thrombocytes (detected with FITC-conjugated antibodies) were excluded from the CD8α+CD45+ subset, as illustrated on CD8α versus FITC dot plots. Within the CD8α+CD45+FITC- subpopulation, the relative proportion of CD25-expressing cells was additionally quantified to evaluate activation status of NK-like cells (Supplementary Figure S4).
Thus, the CD8αα+hiCD4-TCR1- cell subset identified in our study excludes both γδ T cells and CD4+ T helper cells, as it lacks expression of the γδ T cell receptor TCR1 and the T cell marker CD4. However, this population may include other αβ T cell subsets or NK-like cells.
2.7 Fluorescence-activated cell sorting of T cell subsets
To investigate the transcriptional activity of various immune-related genes in T cells, fluorescence-activated cell sorting (BD FACS Aria II, BD Biosciences) was used with a 70 μm nozzle. We collected γδ (TCR1+) and TCR1- cell subsets from PBMC of Salmonella-infected chickens at 7, 9 and 12 dpi. Isolated blood cells (PBMC) were incubated with the specific monoclonal antibodies (CD8α-PE, CD8β-unlabeled, anti-IgG2a-BV510, CD4-PerCP-Cy5.5, TCR1-FITC) for 30 min in the dark and immediately sorted. Prior to sorting, the drop delay was adjusted using FACS AccuDrops (BD Biosciences). A test sort was performed to ensure a purity level exceeding 95% in the sorted cell subsets. CD8αα+hi and CD8αβ+hi γδ T cells as well as CD8αα+hiCD4- and CD8αβ+hiCD4-TCR1- cell subsets were collected separately. The sorted cell populations from each bird were immediately stored in RNAlater (Qiagen) at -20°C until RNA isolation.
2.8 Immunohistochemistry
To compare the Salmonella invasion of the wild-type and TCR Cγ−/− chickens, frozen sections of the cecum of Salmonella-infected chickens were prepared. The immunohistochemical staining method has been described previously (9). Briefly, tissue sections (7 µm thickness) were fixed in acetone and subsequently incubated with the monoclonal antibodies against Salmonella enterica lipopolysaccharide (clone 5D12A 1:800 in PBS, Bio-Rad, Feldkirchen, Germany). Next, the peroxidase-anti-peroxidase conjugated secondary goat-anti-mouse immunoglobulin (Sigma, Deisenhoven, Germany) was applied. The enzyme-linked antibody was visualized by reaction with 3,3’-diaminobinzidine (DAB, Merck, Darmstadt, Germany) and hydrogen peroxide. The sections were counterstained with hematoxylin (Agilent Dako, Waldbronn, Germany) and finally mounted with Canada balsam (Carl Roth, Karlsruhe, Germany). As a negative control, the primary antibodies were replaced by PBS.
2.9 Image analysis
The Salmonella invasion into the cecal mucosa was determined by analyzing the percentage of Salmonella positively-stained areas in the cecal epithelial lining and lamina propria of infected TCR Cy-/- and wild-type chickens. Analysis was performed using the image analysis system cellSens Dimension software (Olympus, Hamburg, Germany) as previously described (9). Regions of interest (ROIs) were drawn and at least 2 mm2 of cecal mucosa (epithelial layer and lamina propria) were analyzed per animal.
2.10 Quantitative RT-PCR for chemokine, cytokine and iNOS expression
To investigate the transcriptional dynamics of important immune genes after Salmonella infection, total RNA was extracted from cecal tissue and sorted cells using the RNeasy Mini Kit (Qiagen, Hilden, Germany) according to the manufacturer’s protocol. The RNase-free DNase kit (Qiagen) was used to digest any residual DNA. Quantitative RT-PCR was carried out using QuantiFast® SYBR® Green RT-PCR kit (Qiagen), as described by the manufacturer and in previous studies (9, 23). Sequences, annealing temperatures and accession numbers of the primers for IFN-γ, inducible nitric oxide synthase (iNOS (66); cecum only), glycerinaldehyd-3-phosphat-dehydrogenase (GAPDH), lipopolysaccharide-induced tumor necrosis factor-alpha factor (LITAF (9); cecum only), IL-10 (cecum only), IL-1β (cecum only), IL-17A, IL-22 (67), IL-2Rα (23) have been previously described. Newly designed primers used in this study are provided in Table 2. The expression of target genes was normalized to the housekeeping gene glycerinaldehyde-3-phosphate (GAPDH). For expression analysis of sorted cells, results are presented as 40-ΔCt values. For cecum, the expression levels of the tested genes were calculated by 2-△△Ct (68).
2.11 Statistical analysis
For analysis of microbiological data, viable bacterial counts were converted into logarithmic form. Samples with a viable count below the detection limit for direct plating (log10< 1.47) but positive after enrichment were assigned a value of log10 = 1.0. Samples with no detectable Salmonella growth after enrichment was assigned a log10 value of 0. Data were evaluated using multifactorial variance analysis with group and time as factors. P values< 0.05 were considered as statistically significant (software: Statgraphics Plus, Inc., Rockville, MD, USA).
Normally distributed data of flow cytometry, RT qPCR and histochemistry (Shapiro-Wilk test 0.05) were analyzed by 2-way ANOVA followed by Tukey’s multiple comparison test (GraphPad Prism 7, GraphPad Software, Inc, Boston, MA, USA). Mann-Whitney U test was applied for not normally distributed data. Infected and mock-infected chickens as well as wild-type and TCR Cy-/- animals were compared to each other at multiple time points. P values< 0.05 were considered significant.
3 Results
3.1 Increased numbers of Salmonella in liver of TCR Cγ−/− chickens compared to wild-type chickens
To examine the propagation of S. Enteritidis in infected wild-type and TCR Cγ−/− chickens, the cfu of the bacteria were determined in liver and cecal content. The oral administration of 2 x 107 cfu of S. Enteritidis per bird resulted in robust cecal colonization and systemic spread into the liver, without inducing clinical signs. Notably, at 1–2 days post infection (dpi), we observed equivalent high Salmonella loads in the cecal content of both chicken lines, indicating a comparable infectious pressure at the beginning of the disease (Figure 1A). No significant differences in cfu were detected in cecal contents of wild-type and TCR Cγ−/− chickens at later stages of infection. In the liver, significant differences in S. Enteritidis colonization were observed between the genetically different chicken lines. Compared to wild-type birds, significantly higher cfu of Salmonella were found in the liver of TCR Cγ−/− chickens at 5 dpi (Figure 1B). The dynamics of Salmonella counts in the liver also differed between the two chicken lines during the course of the infection. In the absence of γδ T cells, the number of cfu in the liver increased rapidly after infection, peaking at 5 dpi. Thereafter, the bacterial load decreased quickly. In contrast, the Salmonella load in the liver of wild-type animals remained relatively low and stable throughout the infection. At 15 dpi, hardly any Salmonella were detectable in livers of wild-type and knockout chickens. No Salmonella was detected in the liver or cecum of mock-infected chickens at any time point.
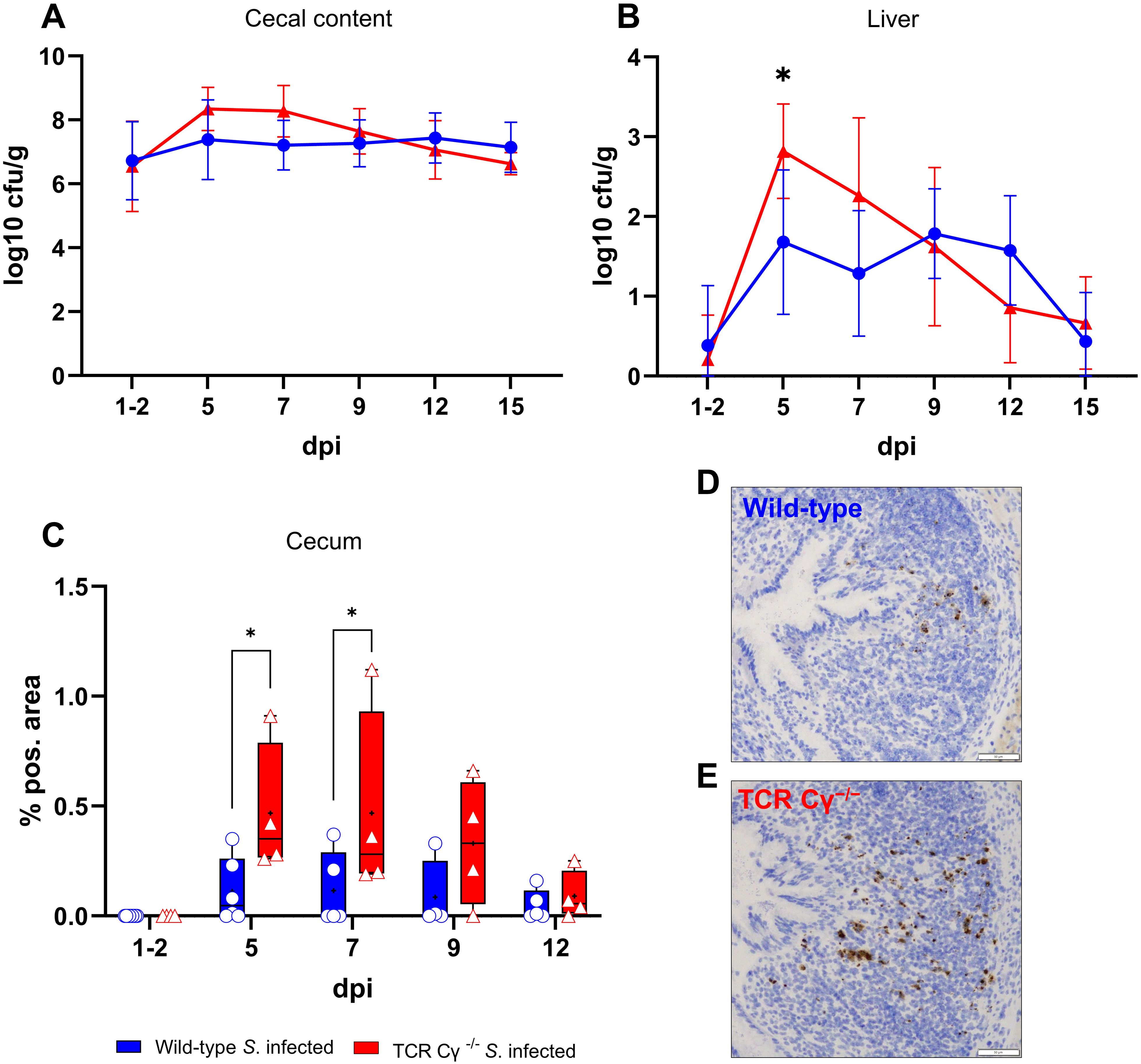
Figure 1. Detection of Salmonella Enteritidis in wild-type and TCR Cγ−/− chickens post infection. Logarithmic bacterial counts of Salmonella in cecal content (A) and liver (B) of wild-type and TCR Cγ−/− chickens (infection on day 3 of age). Data represent the mean ± standard deviation; n = 3-9. The box plot diagram displays the percentage of Salmonella-positive area in cecal mucosa (C) of infected wild-type and TCR Cγ−/− chickens. Data are presented as minimum and maximum cell counts, with median indicated; n = 4-8. Representative immunohistochemical staining of Salmonella (brown) in cecal tissue from infected wild-type (D) and TCR Cγ−/− chickens (E) at 5 dpi. Scale bar indicates 50 µm. * indicates significant differences between chicken groups, p< 0.05 (2-way ANOVA-Tukey’s multiple comparison test).
3.2 Increased Salmonella invasion into the cecum wall of TCR Cγ−/− chickens compared to wild-type chickens
The invasion of S. Enteritidis into the cecal wall was assessed using immunohistochemistry and image analysis. The results demonstrated that Salmonella was able to invade the cecal mucosa in both chicken lines, albeit at different levels. At 5 and 7 dpi, a significantly higher percentage of Salmonella-positive areas were observed in the cecal wall of TCR Cγ−/− chickens compared to wild-type birds. A trend towards increased Salmonella invasion into the cecal mucosa was also noted in the absence of γδ T cells at 9 dpi (Figures 1C–E).
3.3 Elevated numbers of monocytes in blood of wild-type and TCR Cγ−/− chickens after Salmonella infection
Dynamic changes in absolute leukocyte numbers in whole blood of Salmonella-infected TCR Cy-/- and wild-type chickens were quantified by flow cytometry (Figure 2). Compared with mock-infected controls, S. Enteritidis exposure induced a rapid and significant increase in monocytes at 5 dpi in both wild-type and TCR Cγ−/− chickens, with elevated levels persisting until at least 12 dpi in both chicken lines. In the absence of γδ T cells, knockout chickens displayed a trend towards higher monocyte counts at 5 and 7 dpi compared to wild-type birds, accompanied by large individual differences. Additionally, a significant increase in circulating thrombocyte numbers in both infected wild-type and TCR Cγ−/− chickens was observed compared to mock controls. No relevant differences were detected in the counts of heterophils and B cells, whereas TCR Cγ−/− chickens showed a trend towards lower blood T cell numbers (Supplementary Figure S5).
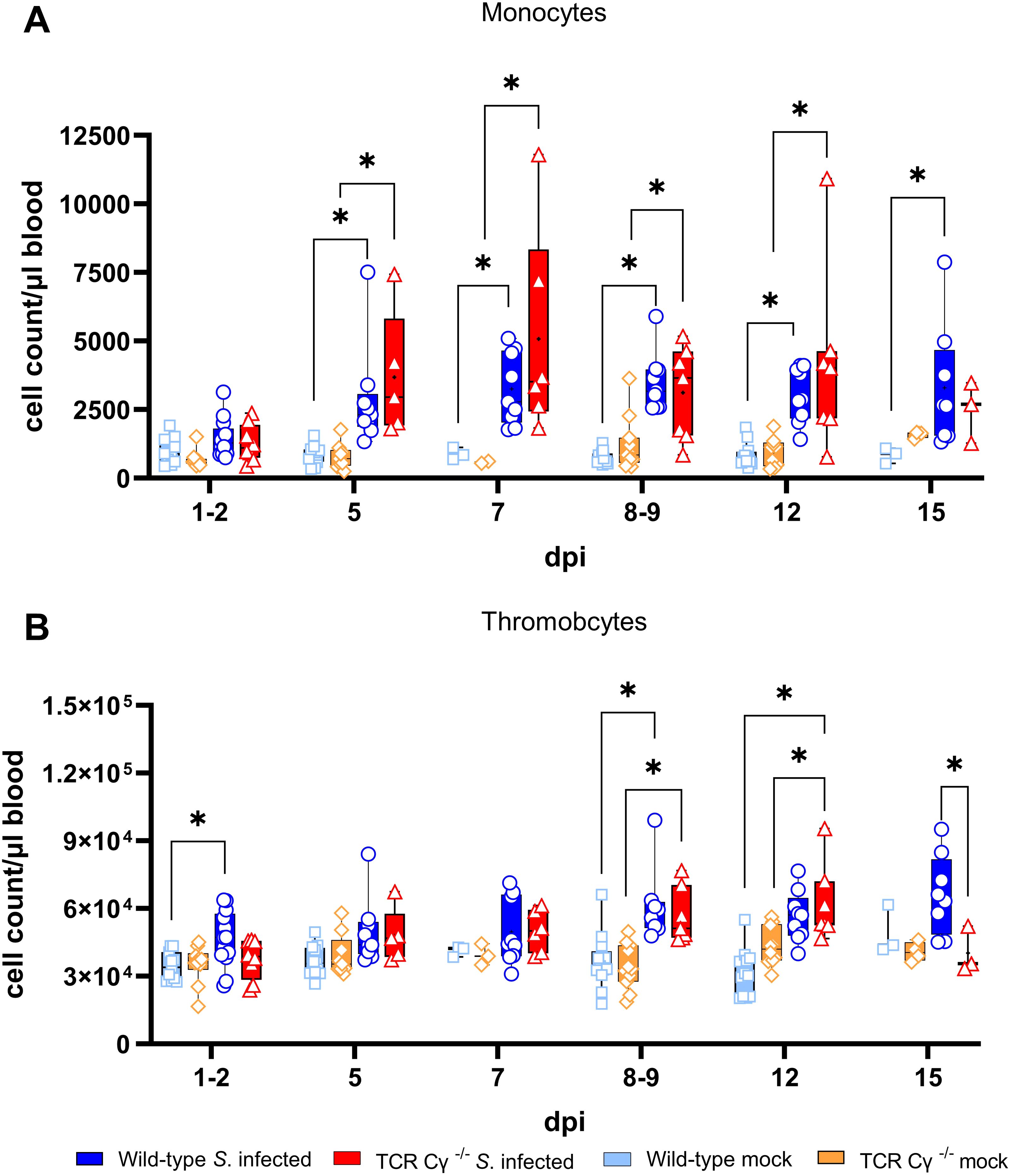
Figure 2. Flow cytometric analysis of leukocytes in avian whole blood following Salmonella Enteritidis infection. Absolute numbers of viable monocytes (A) and thrombocytes (B) were measured over time in blood from wild-type and TCR Cγ−/− chickens following Salmonella or mock infection on day 3 of age. Data are presented as minimum and maximum cell counts, with median indicated; n = 2-13. * indicates significant differences between chicken groups, p< 0.05 (2-way ANOVA-Tukey’s multiple comparison test).
3.4 Absolute numbers of γδ T cell subsets increased in whole blood, spleen and cecum after Salmonella infection of wild-type chickens
Determination of absolute numbers of γδ T cell subsets (TCR1+) in whole blood, spleen and cecum revealed an increase of CD8αα+hi γδ T cells across all compartments (Figure 3A). In whole blood, significantly enhanced numbers of CD8αα+hi γδ T cells were observed at 5, 8-9, 12 and 15 dpi. At 8–9 and 12 dpi, the number of CD8αα+hi γδ T cells co-expressing the CD25 antigen also increased significantly (Figure 3B). In the spleen of Salmonella-infected wild-type chickens, significantly elevated numbers of CD8αα+hi γδ T cells were detected at 9 and 15 dpi. A significant increase in CD25+CD8αα+hi γδ T cells was observed at 9 dpi, with a similar trend at 12 and 15 dpi. Comparable results were found in the cecum, where CD8αα+hi γδ T cells increased significantly at 9 dpi, with a similar trend noted at 7, 12 and 15 dpi compared to mock-infected controls.
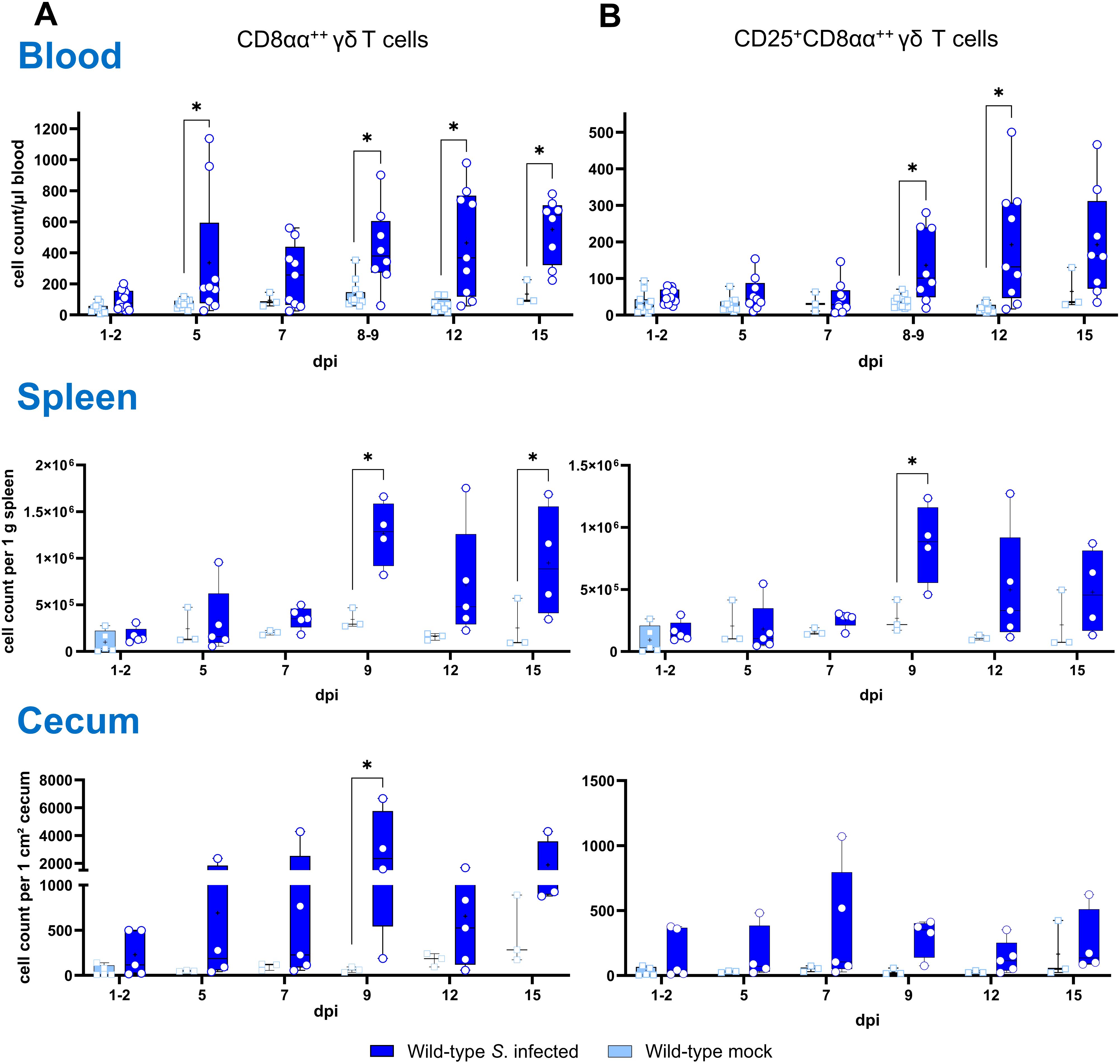
Figure 3. Flow cytometric analysis of γδ T cell subsets in wild-type chickens after Salmonella Enteritidis infection. Absolute numbers of CD8αα+hi (A) and CD25+CD8αα+hi (B) γδ T cells (TCR1+) are shown of blood (n = 3-13), spleen (n = 3-5) and cecum (n = 3-5) from Salmonella-infected and mock-infected wild-type chickens (infection on day 3 of age). Data are presented as minimum and maximum cell counts, with median indicated. * indicates significant differences between chicken groups, p< 0.05 (2-way ANOVA-Tukey’s multiple comparison test; cecum: Mann–Whitney U test).
3.5 Compensation of the lack of γδ T cells in TCR Cγ−/− chickens after Salmonella infection
Flow cytometric analysis revealed an increased emergence of a CD8αα+hiCD4-TCR1- cell population in TCR Cγ−/− chickens (Figure 4). Following Salmonella infection, the absolute numbers of these cells were significantly increased in peripheral blood and spleen in the absence of γδ T cells (8–9 and 12 dpi), but not in wild-type birds (Figure 4A). Moreover, higher absolute numbers of a CD25+CD8αα+hiCD4-TCR1- cell phenotype were found in blood (8–9 and 12 dpi) and spleen (9 dpi) of TCR Cγ−/− chickens compared to wild-type (Figure 4B). Notably, these TCR1- cells identified in the infected TCR Cγ−/− chickens exhibited an identical sub-phenotype (CD8αα+hi and CD25+) as the γδ T cells that were elevated in infected wild-type chickens (Figure 3). Analysis of cecal tissue showed no significant differences between the chicken groups.
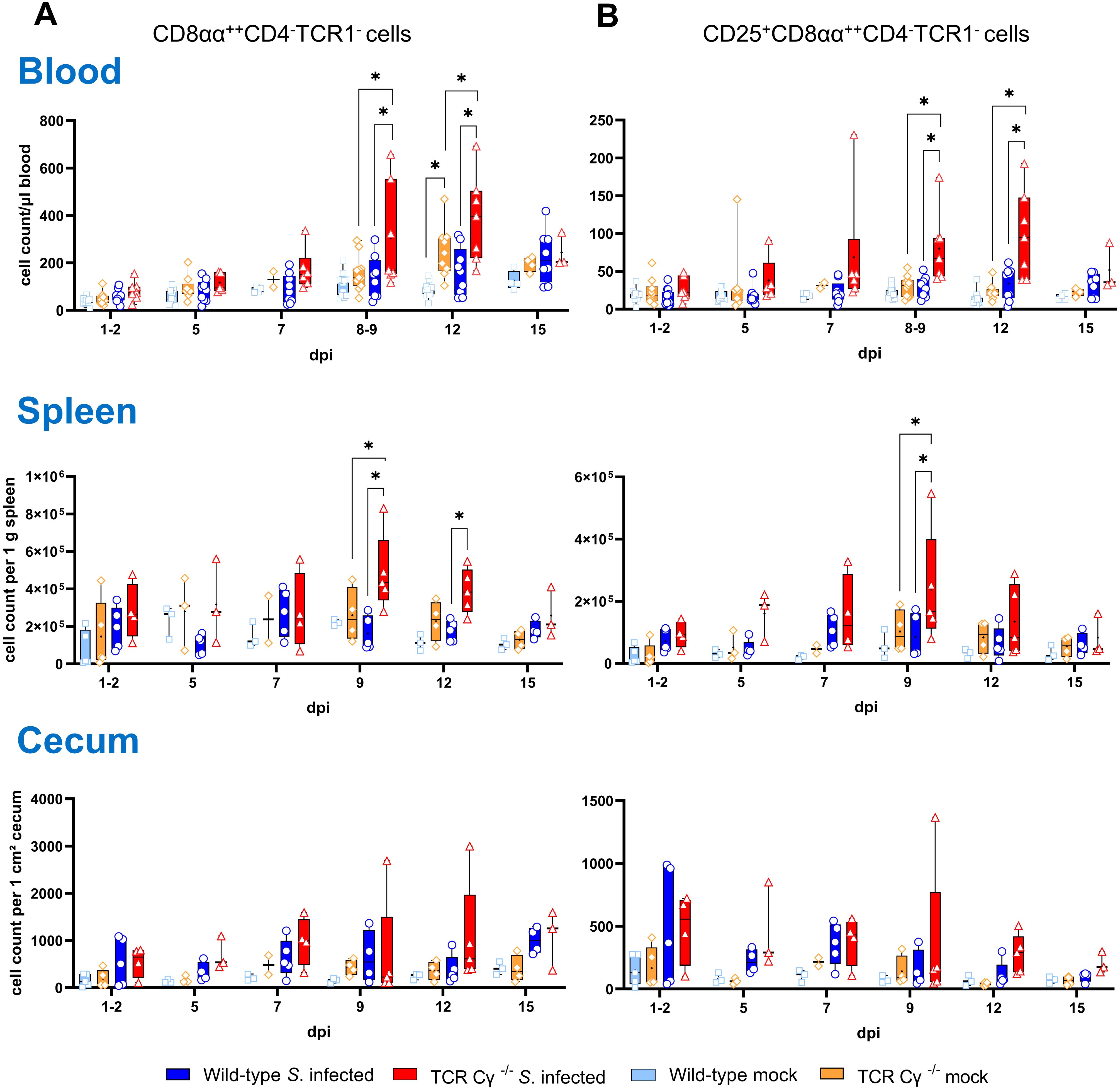
Figure 4. Flow cytometric analysis of TCR1- cell subsets in wild-type and TCR Cγ−/− chickens after Salmonella Enteritidis infection. Absolute numbers of CD8αα+hiCD4-TCR1- (A) and CD25+CD8αα+hiCD4-TCR1- cells (B) are shown for blood (n = 2-13), spleen (n = 2-5) and cecum (n = 2-5) from Salmonella-infected and mock-infected wild-type and TCR Cγ−/− chickens (infection on day 3 of age). Data are presented as minimum and maximum cell counts, with median indicated. * indicates significant differences between chicken groups, p< 0.05 (2-way ANOVA-Tukey’s multiple comparison test).
3.6 CD8αα+hiCD4-TCR1- cells of Salmonella-infected TCR Cγ−/− chickens consist at least partially of αβ T cells
To further characterize the phenotype of the CD8αα+hiCD4-TCR1- cells observed in TCR Cγ−/− animals, we investigated the emergence of Vβ1 (TCR2)/Vβ2 (TCR3) αβ T cells in blood, spleen and cecum (Figure 5). In the spleen, a significant increase in Vβ1/Vβ2 cells expressing CD8αα+hi was detected in TCR Cγ−/− chickens at 9 dpi compared to both infected wild-type and mock-infected TCR Cγ−/− controls, with a similar trend observed at 12 and 15 dpi (Figure 5A). In blood, the findings were consistent with those in the spleen. A significant increase in CD8αα+hiCD4- αβ T cells was detected in infected TCR Cγ−/− birds at 12 dpi compared to infected wild-type animals. However, no significant differences were observed compared to mock-infected TCR Cγ−/− controls (Figure 5B). In cecum, CD8αα+hiCD4- αβ T cells were significantly elevated in both infected wild-type and TCR Cγ−/− chickens at 15 dpi compared to mock-infected controls (Figure 5C).
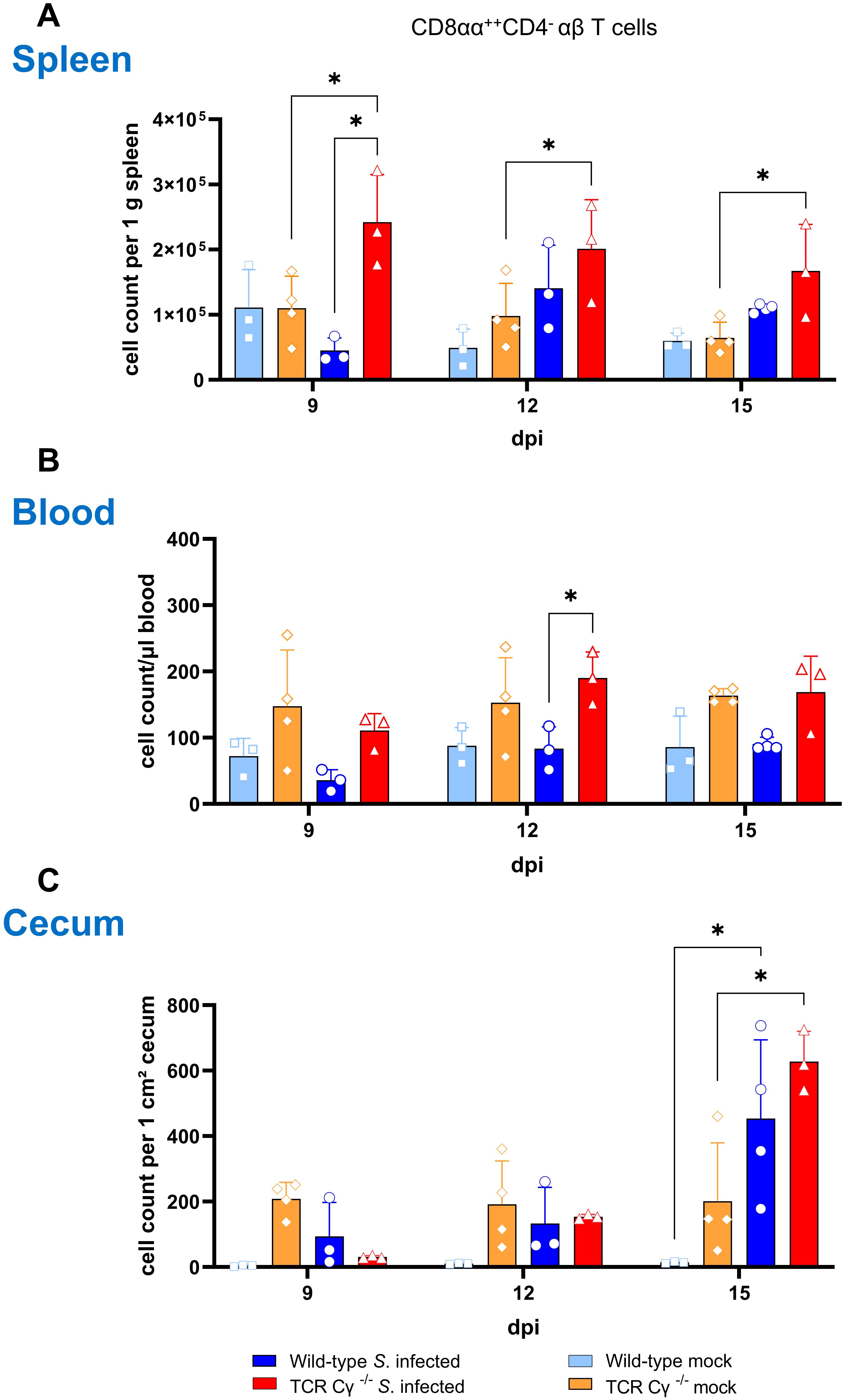
Figure 5. Emergence of CD8αα+hiCD4- αβ T cells in TCR Cγ−/− chickens following Salmonella Enteritidis infection. Absolute numbers of CD8αα+hiCD4- Vβ1/Vβ2 αβ T cell subsets (TCR2/TCR3+) are shown for spleen (A), blood (B) and cecum (C) of Salmonella-infected and mock-infected wild-type and TCR Cγ−/− chickens (infection on day 3 of age). Data represent the mean ± standard deviation; n = 3-4. * indicates significant differences between chicken groups, p< 0.05 (2-way ANOVA-Tukey’s multiple comparison test).
3.7 Higher percentages of NK-like cells expressing CD25 in blood of TCR Cγ−/− than wild-type chickens after Salmonella infection
Further, we explored the compensatory potential of the TCR1- cell subset in the absence of γδ T cells by analyzing the occurrence of CD8α+ NK-like cells in PBMCs, spleen, and cecum of chickens following Salmonella infection. Our findings showed a low relative abundance of NK-like cells in PBMCs and spleen (2–8%), whereas a notably higher proportion (30–40%) of NK-like cells was detected in the cecum of both wild-type and TCR Cγ−/− chickens (Figure 6A). No significant differences in the frequency of NK-like cells were observed between the two Salmonella-infected animal groups. However, the activation status of the cells showed a significant increase of CD25+ NK-like cells in PBMCs of TCR Cγ−/− chickens compared to wild-type birds between 9 and 12 dpi (Figure 6B). In chickens of both genotypes, a high proportion of NK-like cells in the cecum demonstrated a CD25 expression (activated phenotype) which decreased over time. Nevertheless, a trend towards more CD25+ NK-like cells was obvious in the spleen and cecum of TCR Cγ-/- compared to wild-type chickens between 9 and 15 dpi.
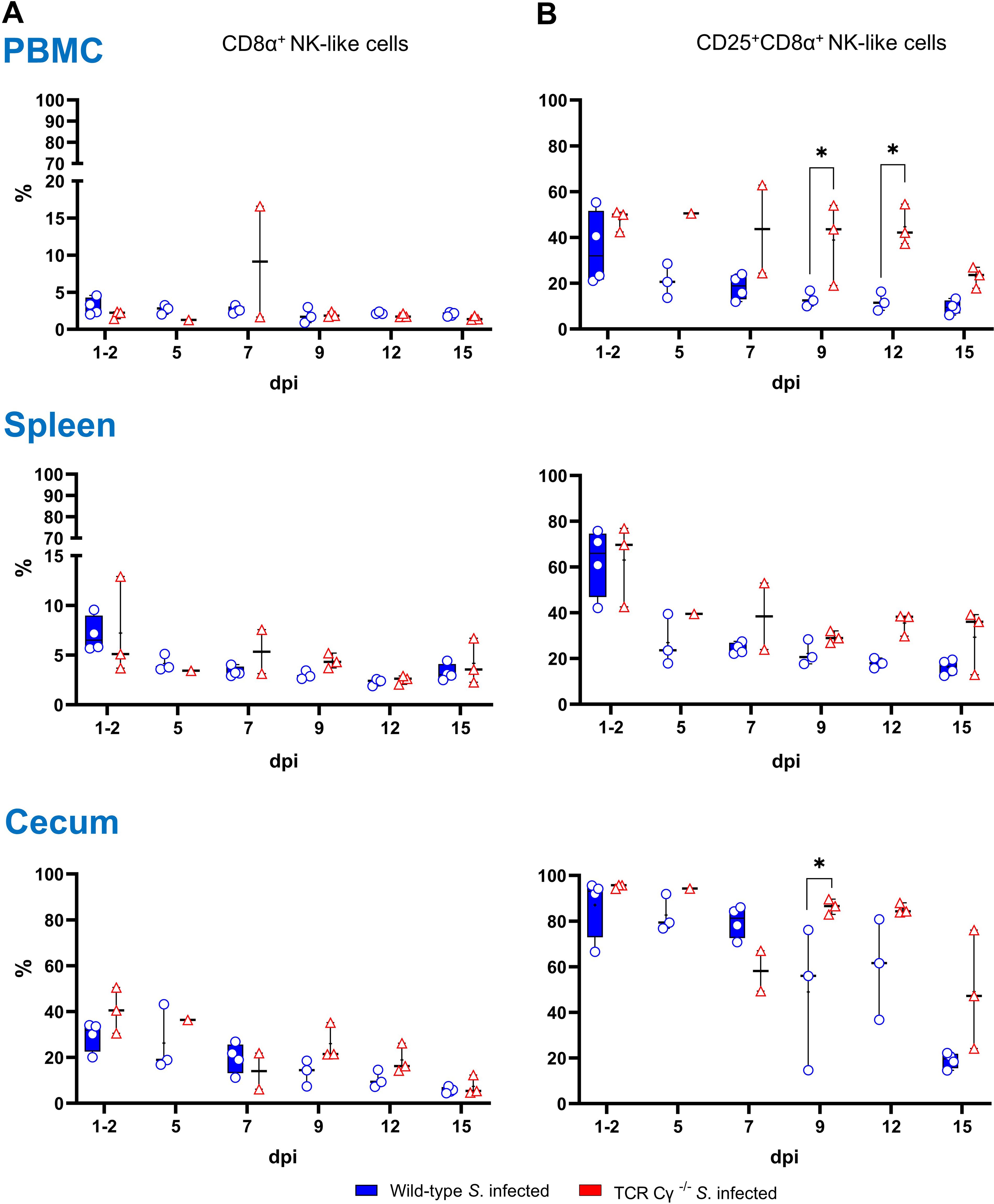
Figure 6. Frequency and activation status (CD25 expression) of NK-like cells in wild-type and TCR Cγ−/− chickens after Salmonella Enteritidis infection. (A) Relative frequency of CD8α+ NK-like cells within the CD45+ lymphocyte population lacking T and B cell lineage as well as monocyte markers (TCR1, TCR2, TCR3, CD4, Bu1, K1) in PBMCs, spleen and cecum of Salmonella-infected wild-type and TCR Cγ−/− chickens (infection on day 3 of age). (B) Percentage of CD8α+ NK-like cells expressing CD25 (marker of cell activation) relative to all CD8α+ NK-like cells. Data are presented as minimum and maximum cell counts, with median indicated; n = 1-4. * indicates significant differences between chicken groups, p< 0.05 (2-way ANOVA-Tukey’s multiple comparison test).
3.8 Increase of CD8α+CD4+TCR1- cells in TCR Cγ−/− chickens and wild-type chickens after Salmonella infection
To investigate additional compensatory mechanisms in the absence of γδ T cells, we analyzed the emergence of CD4+ T cells in blood, spleen, and cecum of Salmonella-infected and mock-infected chicken. Our data revealed a significant expansion in the absolute number of CD8α+CD4+TCR1- cells in peripheral blood following Salmonella infection (Figure 7A). This increase was particularly pronounced between 8–9 and 12 dpi in both TCR Cy-/- and wild-type chickens. At 12 dpi, the number of CD25-expressing CD8α+CD4+TCR1- cells was significantly higher in infected TCR Cγ−/− chickens compared to both the infected wild-type and mock-infected control groups (Figure 7B). In the spleen, CD25-expressing CD8α+CD4+TCR1- cells were significantly elevated at 9, 12 and 15 dpi in infected TCR Cγ−/− chickens compared to non-infected knockout controls. Additionally, at 9 dpi, their numbers were also significantly higher in the infected knockout chickens compared to the infected wild-type group. In the cecum, the number of CD8α+CD4+ TCR1- cells was increased at 15 dpi following Salmonella exposure.
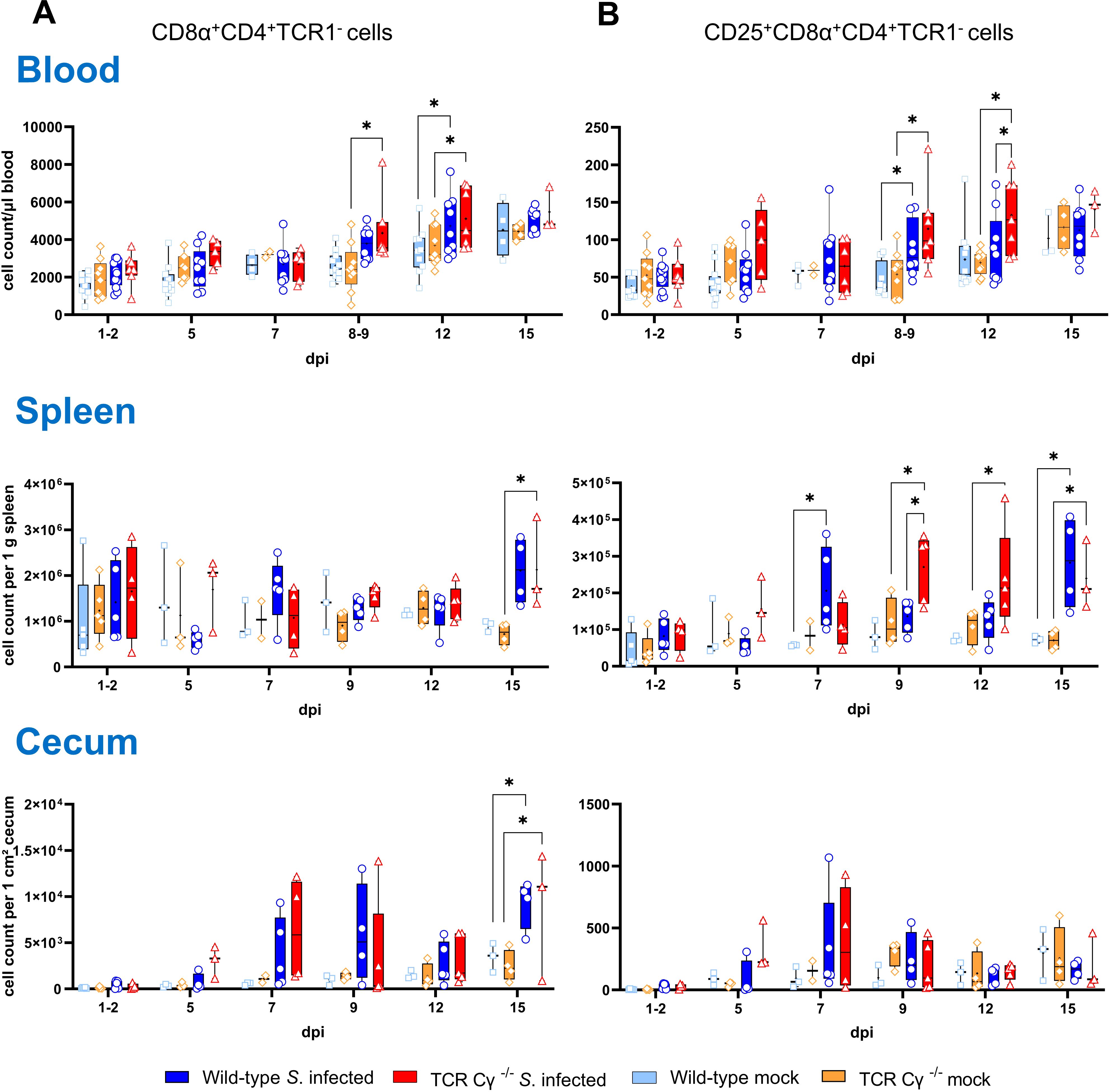
Figure 7. Flow cytometric analysis of CD8α+CD4+ T cells in Salmonella Enteritidis infected chickens. Absolute numbers of CD8α+CD4+TCR1- (A) and CD25+CD8α+CD4+TCR1- cells (B) are shown for blood (n = 2-13), spleen (n = 2-5), and cecum (n = 2-5) from wild-type and TCR Cγ−/− chickens following Salmonella and mock infection on day 3 of age. Data are presented as minimum and maximum cell counts, with median indicated. * indicates significant differences between chicken groups, p< 0.05 (2-way ANOVA-Tukey’s multiple comparison test).
3.9 Blood-derived CD8αα+hiCD4-TCR1- cells of Salmonella-infected TCR Cγ−/− chickens exhibit transcription levels indicative of a pre-activated state
To compare the functional characteristics of CD8αα+hiCD4-TCR1- cells from TCR Cγ−/− birds with their counterparts — CD8αα+hiCD4-TCR1- and CD8αα+hi γδ T cells (TCR1+, CD4-) — from wild-type chickens after infection, we analyzed the transcription levels of immune-related genes in sorted cells using quantitative real-time RT-PCR. Our results revealed that CD8αα+hiCD4-TCR1- cells from both Salmonella-infected wild-type and TCR Cγ−/− chickens exhibited resembling gene expression profiles. No significant differences were observed for the transcription levels of IL-17A, IL-2Rα, IL-22, IFN-γ, TGFβ, perforin and granzyme (Figure 8). This indicates that these cells are not only phenotypically but also functionally comparable. CD8αα+hiCD4-TCR1- cells of infected TCR Cγ−/− chickens exhibited significantly elevated transcription of IL-17A, IL-2Rα, and IL-22 at 7 dpi compared to CD8αα+hi γδ T cells (always CD4-) of infected wild-type chickens. These transcriptional differences diminished by 12 dpi, driven by a significant increase in transcriptional activity in CD8αα+hi γδ T cells over time (Figures 8B–D). The same trend was observed for IFN-γ, but without significant differences (Figure 8A).
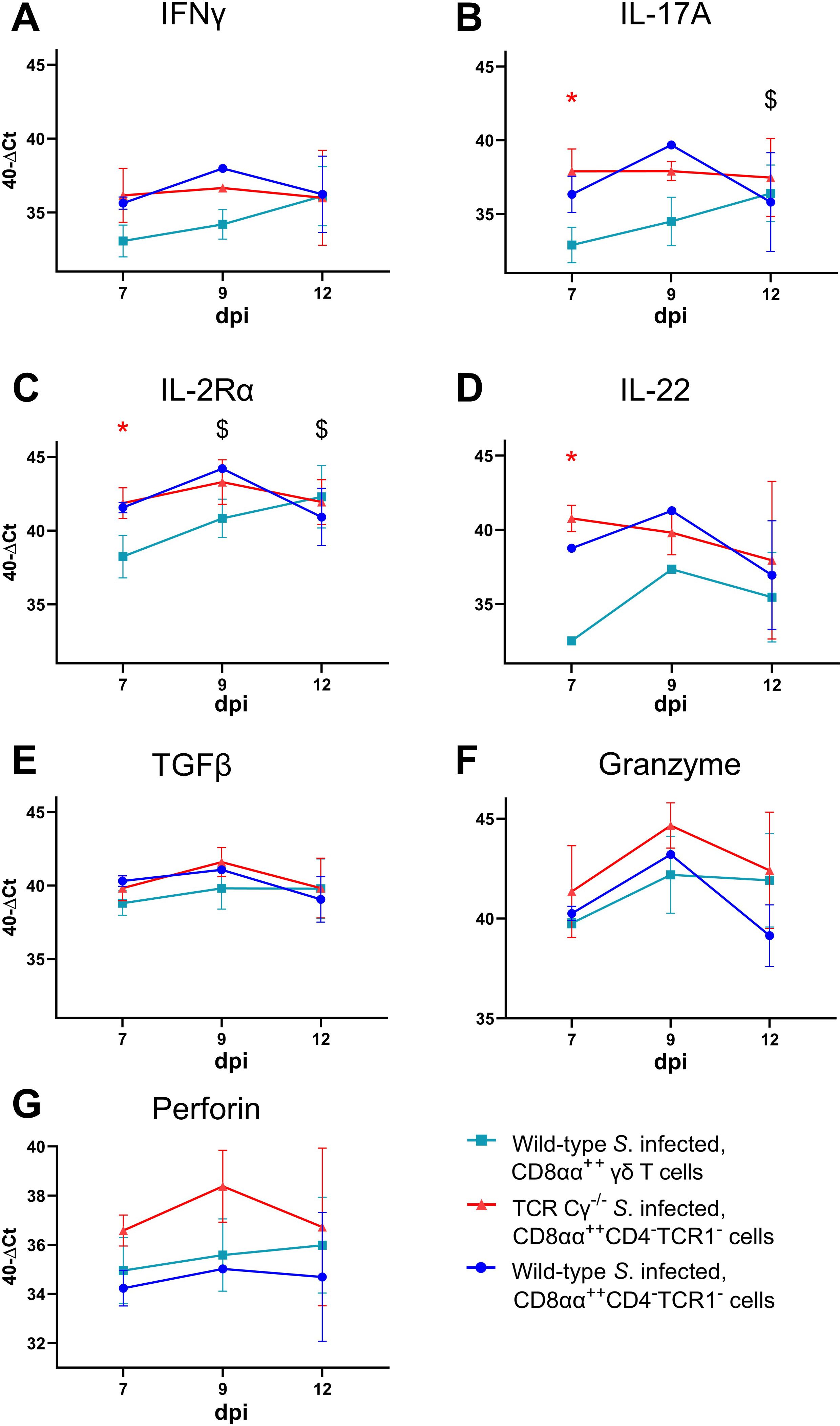
Figure 8. RT qPCR analysis of sorted CD8αα+hi γδ and TCR1- cells from Salmonella Enteritidis-infected wild-type and TCR Cγ−/− chickens. Transcription levels of IFN-γ, IL-17A, IL-2Rα, IL-22, TGFβ, granzyme, and perforin (A-G) were measured in CD8αα+hi γδ (n = 4-6; IL-22: n7dpi = 3; n9dpi = 1; n12dpi = 6) and CD8αα+hiCD4-TCR1- cells (n7dpi = 2; n9dpi = 1; n12dpi = 6) from wild-type chickens, as well as CD8αα+hiCD4-TCR1- cells (n7dpi = 3¸ n9dpi = 2; n12dpi = 3-4) from TCR Cγ−/− animals. Data represent the mean 40-ΔCt ± standard deviation. * indicates significant difference between CD8αα+hi γδ T cells of wild-type chickens and CD8αα+hiCD4-TCR1- cells of TCR Cγ−/− birds. $ indicates significant different transcription levels of CD8αα+hi γδ T cells compared to 7 dpi, p< 0.05 (2-way ANOVA-Tukey’s multiple comparison test).
No significant transcriptional differences were detected for TGFβ, granzyme or perforin between T cell subsets (Figures 8E–G).
3.10 Significant differences in gene transcription of IL-17A and IL-2Rα in blood-derived CD8αα+hiCD4-TCR1- and CD8αβ+hiCD4-TCR1- cells of Salmonella-infected TCR Cγ−/− chickens
In Salmonella-infected TCR Cγ−/− chickens, CD8αα+hiCD4-TCR1- cells showed higher transcription of IL-17A (at 7 and 12 dpi) and IL-2Rα (at 7 and 12 dpi) compared to CD8αβ+hiCD4-TCR1- cells, indicating the activated state of CD8αα+hiCD4-TCR1- cells (Figures 9A–F). Moreover, IL-2Rα transcription was significantly increased in CD8αβ+hi γδ T cells (TCR1+) from infected wild-type chickens compared to CD8αβ+hiCD4-TCR1- cells in infected TCR Cγ−/− birds at 7 and 12 dpi, suggesting distinct functional capabilities between these cell populations. Additionally, IL-2Rα was significantly higher in CD8αα+hi γδ T cells than CD8αβ+hi γδ T cells of infected wild-type chickens at 12 dpi.
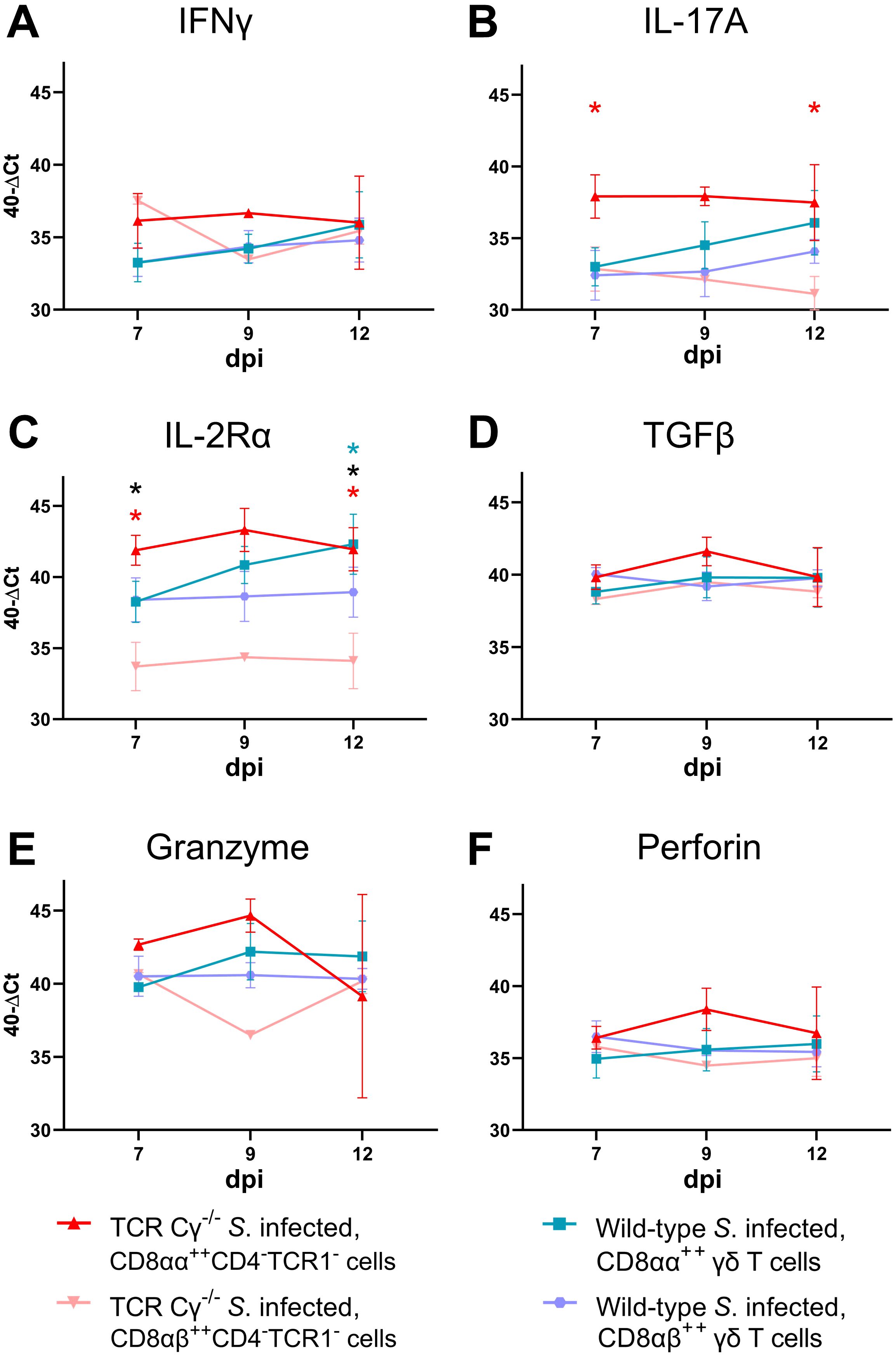
Figure 9. RT qPCR analysis of sorted CD8αα+hi and CD8αβ+hi T cell subsets from Salmonella Enteritidis-infected wild-type and TCR Cγ−/− chickens. Transcription levels of IFN-γ, IL-17A, IL-2Rα, TGFβ, granzyme, and perforin (A-F) were measured in CD8αα+hi (n = 4-6) and CD8αβ+hi γδ T cells (n = 4-6) from wild-type chickens, as well as CD8αα+hiCD4-TCR1- (n7dpi = 3¸ n9dpi = 2; n12dpi = 3-4) and CD8αβ+hiCD4-TCR1- cells (n7dpi = 3¸ n9dpi = 1; n12dpi = 4) from TCR Cγ−/− animals. Data represent the mean 40-ΔCt ± standard deviation. * indicates significant difference between CD8αα+hiCD4-TCR1- and CD8αβ+hiCD4-TCR1- cells of TCR Cγ−/− animals. * indicates significant difference between CD8αα+hi and CD8αβ+hi γδ T cells of wild-type animals. * indicates a significant difference between CD8αβ+hiCD4-TCR1-cells from TCR Cγ−/− animals and CD8αβ+hi γδ T cells from wild-type birds, p< 0.05 (2-way ANOVA-Tukey’s multiple comparison test).
3.11 Significant differences in gene transcription of IFN-γ and iNOS in cecal tissue of Salmonella-infected wild-type and TCR Cγ−/− chickens
To elucidate the impact of γδ T cells on the temporal regulation of immune gene transcription during the course of infection, we assessed the relative expression levels of key cytokines in the cecum of the Salmonella-infected wild-type and TCR Cγ−/− chickens, in comparison to their mock-infected controls. Both wild-type and knockout chickens showed significant upregulation of pro-inflammatory cytokines after Salmonella infection, including IFN-γ, IL-1β, IL-22, LITAF, and the chemokine K203, compared to their mock-infected controls (Figure 10). Upregulation generally occurred between 5 and 12 dpi. Notably, IFN-γ expression was significantly elevated at 1–2 dpi in TCR Cγ−/− chickens, exceeding the transcriptional response observed in infected wild-type birds (Figure 10A). Additionally, iNOS was upregulated in both groups at 5 and 7 dpi, with TCR Cγ−/− chickens showing significantly stronger iNOS transcription at 7 dpi than wild-type chickens (Figure 10B). At 9 dpi, IL-17A and IL-22 transcription in response to Salmonella infection were significantly elevated in TCR Cγ−/− compared to wild-type chickens (Figures 10, 11). In contrast, anti-inflammatory cytokine transcription in TCR Cγ−/− chickens was increased in the early infection phase (IL-10) and during the later stage (TGFβ) of immune regulation (Figure 11). However, the immunomodulatory cytokine IL-21 showed increased expression in both chicken lines at 9 and 12 dpi. Analysis of cytotoxic effector molecules revealed significant differences in granzyme and perforin transcription levels between infected and control birds at 7 and 9 dpi (Figure 11).
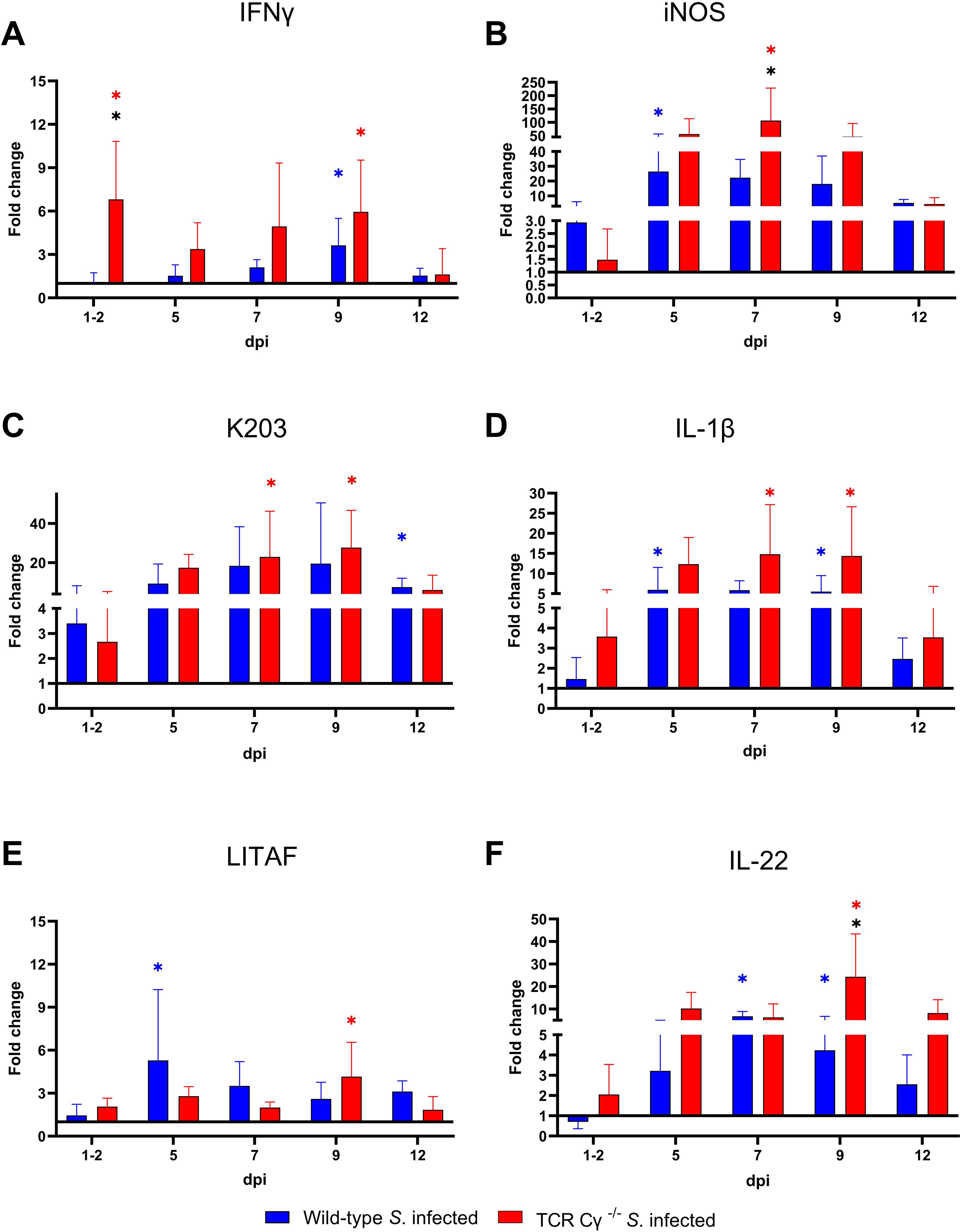
Figure 10. RT qPCR analysis of pro-inflammatory genes in cecal tissue of wild-type and TCR Cγ−/− chickens following Salmonella Enteritidis infection. Fold change expression levels of IFN-γ, iNOS, K203, IL-1β, LITAF, and IL-22 (A-F) in Salmonella-infected wild-type and TCR Cγ−/− chickens relative to their respective mock-infected controls. Data represent the means ± standard deviation; n = 6-9 (infected wild-type), n = 3-4 (infected TCR Cγ−/−, wild-type control and TCR Cγ−/− control). * indicates significant difference between Salmonella-infected wild-type and TCR Cγ−/− chickens. */* indicate a significant difference between Salmonella-infected birds to their respective mock-infected controls, p< 0.05 (2-way ANOVA-Tukey’s multiple comparison test).
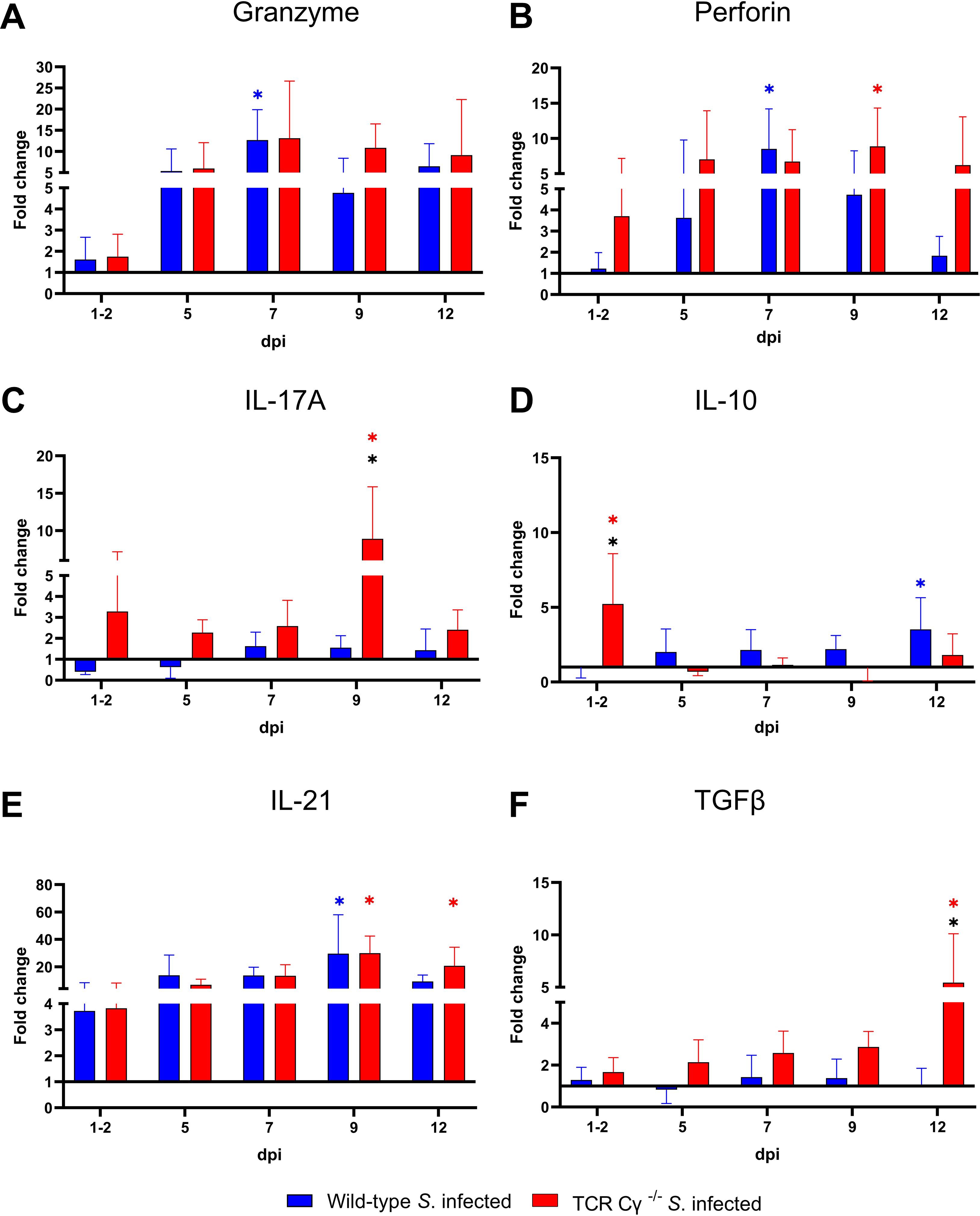
Figure 11. RT qPCR of immune related genes in cecal tissue of wild-type and TCR Cγ−/− chickens following Salmonella Enteritidis infection. Fold change expression levels of granzyme, perforin, IL-17A, IL-10, IL-21, and TGFβ (A-F) in Salmonella-infected wild-type and TCR Cγ−/− chickens relative to their respective mock-infected controls. Data represent the means ± standard deviation; n = 6-9 (infected wild-type), n = 3-4 (infected TCR Cγ−/−, wild-type control and TCR Cγ−/− control). * indicates a significant difference between Salmonella-infected wild-type and TCR Cγ−/− chickens. */* indicate a significant difference between Salmonella-infected birds to their respective mock-infected controls, p< 0.05 (2-way ANOVA-Tukey’s multiple comparison test).
4 Discussion
Previous studies with Salmonella-infected wild-type chickens revealed an increased percentage of γδ T cells and therefore postulated a function of these enigmatic cells during the immune defense against Salmonella (38). However, the actual significance of γδ T cells in eliminating Salmonella from the avian host remained unclear. In this study, our data suggest for the first time that γδ T cells can contribute to the reduction of Salmonella invasion in chickens. Moreover, the CD8αα+hi γδ T cell population (TCR1+), which has been observed to increase in wild-type chickens after Salmonella infection, appears to be compensated by a TCR1- cell population, which is predominantly composed of αβ T cells in TCR Cγ−/− chickens.
In our animal experiments, three-day-old TCR Cy-/- and wild-type chickens were infected with Salmonella Enteritidis. The infectious dose used resulted in a stable Salmonella colonization of the cecal lumen without significant differences between the chicken lines. Compared to previous studies, in which one day old wild-type chicks were infected with the same Salmonella strain and dose (9), we observed lower Salmonella colonization within the cecal lumen and reduced bacterial invasion into the liver of the chickens in this study. This disparity in results may be due to the age difference of the animals at the time of infection. The immune system and cecal microbiota of young chicks develop rapidly after hatching, and 3-day-old chickens are better protected against intestinal infections than newly hatched birds (4–6, 69). Nonetheless, our results showed a significantly higher Salmonella invasion into the cecal wall and liver of Salmonella-infected TCR Cγ−/− chickens than of wild-type animals, particularly at the onset of infection. This finding is consistent with studies in mice, which similarly reported increased translocation of Salmonella across the intestinal epithelium in the absence of γδ T cells during the early stages of infection (54, 70). The cfu in the liver of knockout animals even reached levels comparable to those observed in infected day-old wild-type chicks of previous studies (9). Thus, our results suggest that the presence of γδ T cells can influence the invasion and dissemination of Salmonella in young chickens. The γδ T cells may exert protective effects in multiple ways. Firstly, γδ T cells possess innate immune cell characteristics, can directly kill infected cells, recruit neutrophils and activate phagocytes (26, 71–73). Additionally, γδ T cells secrete a range of cytokines to regulate and shape the immune response (37, 74). Secondly, γδ T cells migrate from the thymus to the intestine during the embryonic development, starting at embryonic day 15. This is even significantly earlier than αβ T cell migration (75). Thus, γδ T cells seem to fill the immunological gap shortly after hatching, when the immune functions of αβ T cells are not yet available. Thirdly, the TCR Cγ−/− chickens in this study may have had an impaired epithelial barrier integrity due to the absence of γδ T cells. Research on intraepithelial lymphocytes in mouse, human and chickens intestinal tissue showed that γδ T cells represent an essential intraepithelial population, contributing to immune surveillance and maintenance of the intestinal integrity (76–80). In Salmonella-infected mice, γδ T cells maintain the integrity of the epithelial tight junctions, thereby limiting bacterial invasion into the gut mucosa (54, 70, 81, 82). In chickens, Majeed et al. (79, 80) demonstrated that the number of IEL subsets, including CD8αα+hi γδ T cells, increased significantly during early S. Typhimurium and Clostridium Perfringens infection, suggesting a potential role in the immune response. However, whether and to what extent avian γδ T cells actually contribute to the maintenance of the intestinal barrier remains to be elucidated. In the absence of infection, Heyl et al. (43) reported no notable differences in intestinal morphology between TCR Cγ−/− chickens and their wild-type counterparts.
In mice, other lymphocyte subsets can partially compensate for the absence of γδ T cells (52, 83, 84). For non-infected TCR Cγ−/− chickens, a significant increase in the CD8αα+ αβ T cell population has been reported (43). In the present study, no significant differences were found in the number of CD8αα+CD4- αβ T cells in the blood and spleen of healthy TCR Cγ-/- and wild-type chickens. However, shortly after the increase of γδ T cells in wild-type chickens post Salmonella infection, a significantly enhanced number of previously undescribed TCR1- cells was detected in blood and spleen of infected TCR Cγ−/− chickens. These TCR1- cells of TCR Cy-/- chickens had the same CD8αα+hi sub-phenotype, together with an increased activation level (CD25+), as the elevated γδ T cells of wild-type birds. Based on these findings, we suggest that the TCR1- cells may potentially substitute the functions of γδ T cells in the TCR Cγ−/− chickens after Salmonella infection. This possibility is further supported by the timing of the increase in TCR1- cells, which coincided with a substantial reduction in Salmonella counts in the liver. However, the direct involvement of these cells in bacterial control in the liver requires further investigation.
Previous research has reported that the γδ T cell population includes lymphocytes that share numerous phenotypic, functional, and homeostatic characteristics with their αβ T cell counterparts (85). To investigate whether αβ T cells potentially compensate for the absence of γδ T cells and their functions in the knockout animals after Salmonella infection, the emergence of TCR1- and αβ T cell (TCR2/TCR3+) subsets was compared. Our results indicate that at least parts of the CD8αα+hiCD4-TCR1- cells rising after infection may indeed be αβ T cells (CD4-) in Salmonella-infected TCR Cγ−/− chickens. This suggests a compensatory role for CD8αα+hiCD4- αβ T cells in the immune response against Salmonella in chickens lacking γδ T cells. However, the number of CD8αα+hi αβ T cells detected did not completely reach the levels of CD8αα+hiCD4-TCR1- cells found in infected knockout animals.
The increased CD8αα+hiCD4-TCR1- cell population in Salmonella-infected TCR Cγ−/− chickens may include additional, yet unidentified cell populations beyond Vβ1 and Vβ2 αβ T cells. Of relevance are natural killer cells, which express CD8 but lack T or B cell lineage-specific antigens (65, 86). NK cells comprise approximately 30% of intestinal intraepithelial lymphocytes and show enhanced activation following Salmonella infection (65, 87, 88). However, despite their prominence during embryonic development, NK cell abundance in blood and spleen of adult birds remains relatively low, representing only 0.5% to 1% of the lymphocyte population (65, 86). Our study identified a low abundance population of CD8α+ lymphocytes lacking TCR1, TCR2, TCR3, CD4, Bu1 and K1 expression (termed NK-like cells herein) in blood and spleen. Consistent with existing literature (65, 86), a higher percentage of these cells was found in the cecum. While the overall NK-like cell population remained comparable between chicken lines, we observed significantly more CD8+ NK-like cells expressing the CD25 antigen in the blood of infected knockout animals compared to wild-type animals, with a similar trend in the cecum and spleen. The expression of the CD25 antigen (IL-2Rα) by human NK cells has been correlated with induced cytotoxic activity and cytokine secretion (89). Thus, NK-like cells appear to contribute to the functional compensation of γδ T cells in knockout chicken post infection. Indeed, Göbel et al. (65) previously postulated that γδ T cells and NK cells have redundant functions. Notably, in pigs, a CD8αα+ NK cell phenotype has been described, characterized with a cytotoxic function against target antigens (90, 91). This hints that the detected NK-like cells in our experiments may belong to the described CD8αα+hiCD4-TCR1- population, representing an additional immune cell subset contributing to the compensation of missing γδ T cells. However, these findings need to be clarified in further studies. Notably, NK cells do not consistently express the CD8 antigen on their surface, but also other markers, including CD56, CD16, and 20E5 (88, 92, 93). Further research is required to conclusively determine the compensatory capacity of NK cells in Salmonella-infected TCR Cγ−/− chickens.
Furthermore, our findings suggest that CD4+ T cells may contribute to immune compensation in TCR Cγ−/− chickens during Salmonella infection. We observed an increase in CD25-expressing CD8α+CD4+TCR1- cells in infected TCR Cγ−/− birds compared to both non-infected knockout and infected wild-type animals. This expansion suggests an adaptive response to the absence of γδ T cells. CD4+ T cells are well known mediators of Salmonella immunity in both mammals and birds, facilitating pathogen clearance and coordinating humoral and cellular immune responses via cytokines such as IL-2 and IFN-γ (9, 94, 95). They may also act as CD4+CD25+FoxP3+ regulatory T cells, suppressing host effector functions (17, 96). Recent evidence of Dai et al. (19) suggests that CD4+CD8+ T cells in chicken exhibit immunosuppressive properties, functioning more similarly to CD4+ T cells than cytotoxic CD8+ T cells, likely contributing to negative regulation of T cell responses. The increased prevalence of this subset in TCR Cγ−/− chickens indicates a possible role in immune regulation, potentially preventing excessive inflammation during Salmonella infection in the absence of γδ T cells.
Results from this study further indicate that γδ T cell compensation in knockout chickens is not restricted to T or NK-like cells. There was a trend towards increased numbers of blood monocytes in TCR Cγ−/− chickens compared to wild-type chickens after infection. This observation suggests enhanced cellular migration to sites of inflammation in infected knockout chickens. In tissues, monocytes differentiate into macrophages, which can typically be activated by γδ T cells during immune responses to infections (71). The elevated number of circulating monocytes may thus contribute to the attempt to compensate for the absence of γδ T cells and the resulting deficit of activated resident macrophages in tissues of knockout birds.
Regulation or dysregulation of specific immune mediators are also indicators of compensatory measures taken by an immunologically impaired and infected host. Analysis of immune gene transcription in the cecum showed elevated expression of IFN-γ and iNOS in TCR Cγ−/− chickens compared to wild-type chickens post infection. Previous studies have demonstrated that the transcription levels of proinflammatory cytokines, including IFN-γ and iNOS, depend on the invasiveness of the Salmonella strain into the tissues of infected chickens (9, 66). The findings of our study support this correlation, as higher Salmonella counts were also found in the tissues of TCR Cγ−/− chickens compared to wild-type animals. Notably, the transcription rates of other proinflammatory cytokines, such as LITAF and IL-1β, did not differ significantly between the infected TCR Cγ−/− chickens and wild-type animals. This indicates that the knockout animals can partially compensate for the loss of γδ T cells, with certain regulatory and inflammatory pathways being upregulated while others remain unchanged after infection.
To determine whether the newly described CD8αα+hiCD4-TCR1- cells can replace the functions typically performed by γδ T cells, distinct T cell populations were sorted from PBMCs and the transcription of key immune genes was analyzed. The results suggest that CD8αα+hiCD4-TCR1- cells are not only functionally equivalent to their CD8αα+hi γδ T cell counterparts, but may surpass them in responsiveness following infection. Specifically, CD8αα+hiCD4-TCR1- cells of TCR Cγ−/− chickens showed higher transcription rates of IL-17A, IL-2Rα and IFN-γ compared to CD8αα+hi γδ T cells of wild-type chickens during the early stages of infection. Over time, CD8αα+hi γδ T cells increased their transcription levels, eventually reaching rates comparable to their TCR1- cell counterparts. This response dynamic of avian CD8αα+hi γδ T cells aligns with previous studies showing an upregulation of IFN-γ in response to Salmonella infection (37, 41).
IL-17A is essential for the recruitment and activation of macrophages and neutrophils as first line of defense against salmonellosis (97). Additionally, IL-17A promotes pro-inflammatory cytokines at mucosal barriers, thereby enhancing pathogen control (98, 99). Notably, γδ T cells are a major source of IL-17A in the early stages of inflammatory and infectious responses. During Listeria monocytogenes infection in mice, Hamada et al. (45) showed an elevated absolute number of IL-17A producing γδ T cells at 5 dpi. In chickens, Walliser and Göbel (39, 100) first described IL-17 producing CD4+ αβ T helper17 and γδ T cells. Our findings confirmed that avian CD8αα+hi γδ T cells not only increase in number but also upregulate IL-17A transcription, highlighting an essential regulatory role during infection. Interestingly, our study revealed an increased IL-17A transcription by CD8αα+hi γδ T cells particularly at later time points post infection, when innate immune defenses to control bacterial invasion were already advanced. These observations show a contribution of γδ T cells to IL-17A production beyond early innate immunity, but also during later adaptive immune responses to S. Enteritidis infection (101).
CD8αα+hiCD4-TCR1- cells in chickens not only produce IL-17A but also respond more rapidly than γδ T cells during Salmonella infection. Previous studies have reported on the role of CD4-TCR1- cells as additional IL-17A-producers during Salmonella infection (101). While CD8αα+hiCD4-TCR1- cells are present in low numbers in wild-type chickens, in our study they may compensate for the loss of γδ T cell-derived IL-17A in TCR Cγ−/− chickens through both numerical expansion and early activation post infection. The high transcription rate of IL-2Rα in CD8αα+hiCD4-TCR1- cells, indicating enhanced activation and proliferation, further supports the crucial role of these cells for immune regulation in the absence of γδ T cells (102, 103).
In conclusion, the present study emphasizes the pivotal role of γδ T cells in mediating the early immune response of chickens to Salmonella Enteritidis infection. Other cell populations, particularly CD8αα+hiCD4-TCR1- cells, but also CD4+CD8+ T cells and NK-like cells, appear to be utilized by the host to compensate for the absence of γδ T cells. The CD8αα+hiCD4-TCR1- cells, consisting at least in part of αβ T cells, exhibit pre-activated characteristics suggesting a potential role in the immune response of Salmonella-infected TCR Cγ−/− chickens. Beyond numerical replacement of the missing γδ T cells, compensatory efforts by the host also make an impact on the functional and regulatory level of the immune response. Nevertheless, TCR Cy-/- chickens exhibit impaired early immune protection, resulting in comparatively stronger Salmonella dissemination throughout the body. Further research is needed to assess whether the identified compensatory elements are only indicative of a dysregulated, functionally impaired immune response, or whether they can effectively replace the γδ T cells in conferring a protective host response against Salmonella.
Data availability statement
The raw data supporting the conclusions of this article will be made available by the authors, without undue reservation.
Ethics statement
The animal study was approved by Ethics Committee for Animal Experiments and Animal Welfare of the State of Thuringia, Germany, identification code: BFI-20-001. The study was conducted in accordance with the local legislation and institutional requirements.
Author contributions
FT: Conceptualization, Data curation, Formal analysis, Investigation, Visualization, Writing – original draft, Writing – review & editing. UM: Investigation, Methodology, Writing – review & editing. TH: Resources, Writing – review & editing. CM: Conceptualization, Resources, Writing – review & editing. BS: Resources, Writing – review & editing. AB: Conceptualization, Funding acquisition, Investigation, Methodology, Project administration, Supervision, Writing – original draft, Writing – review & editing.
Funding
The author(s) declare that financial support was received for the research and/or publication of this article. This project was funded by the Deutsche Forschungsgemeinschaft (DFG, German Research Foundation) in the framework of the Research Unit ImmunoChick (FOR5130) project 434524639 (BE 3221/2-1). Benjamin Schusser and Theresa von Heyl were supported by the Deutsche Forschungsgemeinschaft in the framework of the Research Unit FOR5130 (DFG SCHU2446/6-1).
Acknowledgments
We thank Katrin Schlehahn for excellent technical assistance especially with the flow cytometry and RT-PCR experiments. Special thanks go to the staff of the animal production and experimentation unit at the ‘Friedrich-Loeffler-Institut’ Jena. Also, we thank Marie-Sophie Sädler, Norman Müller and Nadine Taupitz for their experimental assistance during this study.
Conflict of interest
The authors declare that the research was conducted in the absence of any commercial or financial relationships that could be construed as a potential conflict of interest.
The author(s) declared that they were an editorial board member of Frontiers, at the time of submission. This had no impact on the peer review process and the final decision.
Generative AI statement
The author(s) declare that no Generative AI was used in the creation of this manuscript.
Publisher’s note
All claims expressed in this article are solely those of the authors and do not necessarily represent those of their affiliated organizations, or those of the publisher, the editors and the reviewers. Any product that may be evaluated in this article, or claim that may be made by its manufacturer, is not guaranteed or endorsed by the publisher.
Supplementary material
The Supplementary Material for this article can be found online at: https://www.frontiersin.org/articles/10.3389/fimmu.2025.1576766/full#supplementary-material
References
1. EFSA EFSA, EFSA EFSA, ECDC ECDPC. The european union one health 2022 zoonoses report. Efsa J. (2023) 21:31–64. doi: 10.2903/j.efsa.2023.8442
2. Barrow PA, Huggins MB, Lovell MA, Simpson JM. Observations on the pathogenesis of experimental Salmonella typhimurium infection in chickens. Res Vet Sci. (1987) 42:194–9. doi: 10.1016/S0034-5288(18)30685-4
3. Beal RK, Powers C, Wigley P, Barrow PA, Kaiser P, Smith AL. A strong antigen-specific T-cell response is associated with age and genetically dependent resistance to avian enteric salmonellosis. Infect Immun. (2005) 73:7509–16. doi: 10.1128/IAI.73.11.7509-7516.2005
4. Beal RK, Wigley P, Powers C, Hulme SD, Barrow PA, Smith AL. Age at primary infection withserovar Typhimurium in the chicken influences persistence of infection and subsequent immunity to re-challenge. Vet Immunol Immunop. (. 2004) 100:151–64. doi: 10.1016/j.vetimm.2004.04.005
5. Tanikawa T, Shoji N, Sonohara N, Saito S, Shimura Y, Fukushima J, et al. Aging transition of the bacterial community structure in the chick ceca. Poult Sci. (2011) 90:1004–8. doi: 10.3382/ps.2010-01153
6. Beal RK, Powers C, Wigley P, Barrow PA, Smith AL. Temporal dynamics of the cellular, humoral and cytokine responses in chickens during primary and secondary infection with Salmonella enterica serovar Typhimurium. Avian Pathol. (2004) 33:25–33. doi: 10.1080/03079450310001636282
7. Berndt A, Methner U. Gamma/delta T cell response of chickens after oral administration of attenuated and non-attenuated Salmonella typhimurium strains. Vet Immunol Immunopathol. (2001) 78:143–61. doi: 10.1016/S0165-2427(00)00264-6
8. Kogut MH, Tellez GI, McGruder ED, Hargis BM, Williams JD, Corrier DE, et al. Heterophils are decisive components in the early responses of chickens to Salmonella enteritidis infections. Microb Pathog. (1994) 16:141–51. doi: 10.1006/mpat.1994.1015
9. Berndt A, Wilhelm A, Jugert C, Pieper J, Sachse K, Methner U. Chicken cecum immune response to Salmonella enterica serovars of different levels of invasiveness. Infect Immun. (2007) 75:5993–6007. doi: 10.1128/IAI.00695-07
10. Cheeseman JH, Levy NA, Kaiser P, Lillehoj HS, Lamont SJ. Salmonella Enteritidis-induced alteration of inflammatory CXCL chemokine messenger-RNA expression and histologic changes in the ceca of infected chicks. Avian Dis. (2008) 52:229–34. doi: 10.1637/8156-102307-Reg.1
11. Wigley P, Hulme S, Rothwell L, Bumstead N, Kaiser P, Barrow P. Macrophages isolated from chickens genetically resistant or susceptible to systemic salmonellosis show magnitudinal and temporal differential expression of cytokines and chemokines following Salmonella enterica challenge. Infect Immun. (2006) 74:1425–30. doi: 10.1128/IAI.74.2.1425-1430.2006
12. Cooper MD, Alder MN. The evolution of adaptive immune systems. Cell. (2006) 124:815–22. doi: 10.1016/j.cell.2006.02.001
13. Davis MM, Bjorkman PJ. T-cell antigen receptor genes and T-cell recognition. Nature. (1988) 334:395–402. doi: 10.1038/334395a0
14. Schat KA. Cell-mediated immune effector functions in chickens. Poult Sci. (1994) 73:1077–81. doi: 10.3382/ps.0731077
15. Luhtala M. Chicken CD4, CD8alphabeta, and CD8alphaalpha T cell co-receptor molecules. Poult Sci. (1998) 77:1858–73. doi: 10.1093/ps/77.12.1858
16. Fontenot JD, Gavin MA, Rudensky AY. Foxp3 programs the development and function of CD4+CD25+ regulatory T cells. Nat Immunol. (2003) 4:330–6. doi: 10.1038/ni904
17. Burkhardt NB, Elleder D, Schusser B, Krchlikova V, Gobel TW, Hartle S, et al. The discovery of chicken foxp3 demands redefinition of avian regulatory T cells. J Immunol. (2022) 208:1128–38. doi: 10.4049/jimmunol.2000301
18. Dai M, Li S, Shi K, Liao J, Sun H, Liao M. Systematic identification of host immune key factors influencing viral infection in PBL of ALV-J infected SPF chicken. Viruses. (2020) 12:1–15. doi: 10.3390/v12010114
19. Dai M, Zhao L, Li Z, Li X, You B, Zhu S, et al. The transcriptional differences of avian CD4(+)CD8(+) double-positive T cells and CD8(+) T cells from peripheral blood of ALV-J infected chickens revealed by smart-seq2. Front Cell Infect Microbiol. (2021) 11:747094. doi: 10.3389/fcimb.2021.747094
20. Sowder JT, Chen CL, Ager LL, Chan MM, Cooper MD. A large subpopulation of avian T cells express a homologue of the mammalian T gamma/delta receptor. J Exp Med. (1988) 167:315–22. doi: 10.1084/jem.167.2.315
21. Arstila TP, Lassila O. Androgen-induced expression of the peripheral blood gamma delta T cell population in the chicken. J Immunol. (1993) 151:6627–33. doi: 10.4049/jimmunol.151.12.6627
22. Holderness J, Hedges JF, Ramstead A, Jutila MA. Comparative biology of gammadelta T cell function in humans, mice, and domestic animals. Annu Rev Anim Biosci. (2013) 1:99–124. doi: 10.1146/annurev-animal-031412-103639
23. Pieper J, Methner U, Berndt A. Heterogeneity of avian gammadelta T cells. Vet Immunol Immunopathol. (2008) 124:241–52. doi: 10.1016/j.vetimm.2008.03.008
24. McCarthy NE, Bashir Z, Vossenkamper A, Hedin CR, Giles EM, Bhattacharjee S, et al. Proinflammatory Vdelta2+ T cells populate the human intestinal mucosa and enhance IFN-gamma production by colonic alphabeta T cells. J Immunol. (2013) 191:2752–63. doi: 10.4049/jimmunol.1202959
25. Guy-Grand D, Vassalli P, Eberl G, Pereira P, Burlen-Defranoux O, Lemaitre F, et al. Origin, trafficking, and intraepithelial fate of gut-tropic T cells. J Exp Med. (2013) 210:1839–54. doi: 10.1084/jem.20122588
26. Di Marco Barros R, Roberts NA, Dart RJ, Vantourout P, Jandke A, Nussbaumer O, et al. Epithelia use butyrophilin-like molecules to shape organ-specific gammadelta T cell compartments. Cell. (2016) 167:203–18.e17. doi: 10.1016/j.cell.2016.08.030
27. Morita CT, Jin C, Sarikonda G, Wang H. Nonpeptide antigens, presentation mechanisms, and immunological memory of human Vgamma2Vdelta2 T cells: discriminating friend from foe through the recognition of prenyl pyrophosphate antigens. Immunol Rev. (2007) 215:59–76. doi: 10.1111/j.1600-065X.2006.00479.x
28. Bedoui S, Gebhardt T, Gasteiger G, Kastenmuller W. Parallels and differences between innate and adaptive lymphocytes. Nat Immunol. (2016) 17:490–4. doi: 10.1038/ni.3432
29. Born WK, Jin N, Aydintug MK, Wands JM, French JD, Roark CL, et al. gammadelta T lymphocytes-selectabl e cells within the innate system? J Clin Immunol. (2007) 27:133–44. doi: 10.1007/s10875-007-9077-z
30. Bonneville M, O’Brien RL, Born WK. Gammadelta T cell effector functions: a blend of innate programming and acquired plasticity. Nat Rev Immunol. (2010) 10:467–78. doi: 10.1038/nri2781
31. Egan PJ, Carding SR. Downmodulation of the inflammatory response to bacterial infection by gammadelta T cells cytotoxic for activated macrophages. J Exp Med. (2000) 191:2145–58. doi: 10.1084/jem.191.12.2145
32. Skeen MJ, Rix EP, Freeman MM, Ziegler HK. Exaggerated proinflammatory and Th1 responses in the absence of gamma/delta T cells after infection with Listeria monocytogenes. Infect Immun. (2001) 69:7213–23. doi: 10.1128/IAI.69.12.7213-7223.2001
33. Born WK, Yin Z, Hahn YS, Sun D, O’Brien RL. Analysis of gamma delta T cell functions in the mouse. J Immunol. (2010) 184:4055–61. doi: 10.4049/jimmunol.0903679
34. Brandes M, Willimann K, Moser B. Professional antigen-presentation function by human gammadelta T Cells. Science. (2005) 309:264–8. doi: 10.1126/science.1110267
35. Scotet E, Nedellec S, Devilder MC, Allain S, Bonneville M. Bridging innate and adaptive immunity through gammadelta T-dendritic cell crosstalk. Front Biosci. (2008) 13:6872–85. doi: 10.2741/3195
36. Kano R, Konnai S, Onuma M, Ohashi K. Microarray analysis of host immune responses to Marek’s disease virus infection in vaccinated chickens. J Vet Med Sci. (2009) 71:603–10. doi: 10.1292/jvms.71.603
37. Pieper J, Methner U, Berndt A. Characterization of avian gammadelta T-cell subsets after Salmonella enterica serovar Typhimurium infection of chicks. Infect Immun. (2011) 79:822–9. doi: 10.1128/IAI.00788-10
38. Laursen AMS, Kulkarni RR, Taha-Abdelaziz K, Plattner BL, Read LR, Sharif S. Characterizaton of gamma delta T cells in Marek’s disease virus (Gallid herpesvirus 2) infection of chickens. Virology. (2018) 522:56–64. doi: 10.1016/j.virol.2018.06.014
39. Walliser I, Gobel TW. Chicken IL-17A is expressed in alphabeta and gammadelta T cell subsets and binds to a receptor present on macrophages, and T cells. Dev Comp Immunol. (2018) 81:44–53. doi: 10.1016/j.dci.2017.11.004
40. Fenzl L, Gobel TW, Neulen ML. gammadelta T cells represent a major spontaneously cytotoxic cell population in the chicken. Dev Comp Immunol. (2017) 73:175–83. doi: 10.1016/j.dci.2017.03.028
41. Berndt A, Pieper J, Methner U. Circulating gamma delta T cells in response to Salmonella enterica serovar enteritidis exposure in chickens. Infect Immun. (2006) 74:3967–78. doi: 10.1128/IAI.01128-05
42. Braukmann M, Methner U, Berndt A. Avian CD25(+) gamma/delta (gammadelta) T cells after Salmonella exposure. Vet Immunol Immunopathol. (2015) 168:14–8. doi: 10.1016/j.vetimm.2015.09.010
43. von Heyl T, Klinger R, Aumann D, Zenner C, Alhussien M, Schlickenrieder A, et al. Loss of alphabeta but not gammadelta T cells in chickens causes a severe phenotype. Eur J Immunol. (2023) 53:e2350503. doi: 10.1002/eji.202350503
44. Dejima T, Shibata K, Yamada H, Hara H, Iwakura Y, Naito S, et al. Protective role of naturally occurring interleukin-17A-producing gammadelta T cells in the lung at the early stage of systemic candidiasis in mice. Infect Immun. (2011) 79:4503–10. doi: 10.1128/IAI.05799-11
45. Hamada S, Umemura M, Shiono T, Tanaka K, Yahagi A, Begum MD, et al. IL-17A produced by gammadelta T cells plays a critical role in innate immunity against listeria monocytogenes infection in the liver. J Immunol. (2008) 181:3456–63. doi: 10.4049/jimmunol.181.5.3456
46. Ladel CH, Blum C, Kaufmann SH. Control of natural killer cell-mediated innate resistance against the intracellular pathogen Listeria monocytogenes by gamma/delta T lymphocytes. Infect Immun. (1996) 64:1744–9. doi: 10.1128/iai.64.5.1744-1749.1996
47. Monin L, Ushakov DS, Arnesen H, Bah N, Jandke A, Munoz-Ruiz M, et al. gammadelta T cells compose a developmentally regulated intrauterine population and protect against vaginal candidiasis. Mucosal Immunol. (2020) 13:969–81. doi: 10.1038/s41385-020-0305-7
48. Fu YX, Roark CE, Kelly K, Drevets D, Campbell P, O’Brien R, et al. Immune protection and control of inflammatory tissue necrosis by gamma delta T cells. J Immunol. (1994) 153:3101–15. doi: 10.4049/jimmunol.153.7.3101
49. Dalton JE, Howell G, Pearson J, Scott P, Carding SR. Fas-Fas ligand interactions are essential for the binding to and killing of activated macrophages by gamma delta T cells. J Immunol. (2004) 173:3660–7. doi: 10.4049/jimmunol.173.6.3660
50. Hsieh B, Schrenzel MD, Mulvania T, Lepper HD, DiMolfetto-Landon L, Ferrick DA. In vivo cytokine production in murine listeriosis. Evidence for immunoregulation by gamma delta+ T cells. J Immunol. (1996) 156:232–7. doi: 10.4049/jimmunol.156.1.232
51. Jameson JM, Cauvi G, Witherden DA, Havran WL. A keratinocyte-responsive gamma delta TCR is necessary for dendritic epidermal T cell activation by damaged keratinocytes and maintenance in the epidermis. J Immunol. (2004) 172:3573–9. doi: 10.4049/jimmunol.172.6.3573
52. Sandrock I, Reinhardt A, Ravens S, Binz C, Wilharm A, Martins J, et al. Genetic models reveal origin, persistence and non-redundant functions of IL-17-producing gammadelta T cells. J Exp Med. (2018) 215:3006–18. doi: 10.1084/jem.20181439
53. Weintraub BC, Eckmann L, Okamoto S, Hense M, Hedrick SM, Fierer J. Role of alphabeta and gammadelta T cells in the host response to Salmonella infection as demonstrated in T-cell-receptor-deficient mice of defined Ity genotypes. Infect Immun. (1997) 65:2306–12. doi: 10.1128/iai.65.6.2306-2312.1997
54. Edelblum KL, Singh G, Odenwald MA, Lingaraju A, El Bissati K, McLeod R, et al. gammadelta intraepithelial lymphocyte migration limits transepithelial pathogen invasion and systemic disease in mice. Gastroenterology. (2015) 148:1417–26. doi: 10.1053/j.gastro.2015.02.053
55. Methner U, al-Shabibi S, Meyer H. Experimental oral infection of specific pathogen-free laying hens and cocks with Salmonella enteritidis strains. . Zentralbl Veterinarmed B. (1995) 42:459–69. doi: 10.1111/j.1439-0450.1995.tb00737.x
56. Methner U, Berndt A, Locke M. Salmonella Enteritidis with double deletion in phoP fliC and a competitive exclusion culture elicit substantial additive protective effects against Salmonella exposure in newly hatched chicks. Vaccine. (2017) 35:6076–82. doi: 10.1016/j.vaccine.2017.09.071
57. Methner U. Immunisation of chickens with live Salmonella vaccines - Role of booster vaccination. Vaccine. (2018) 36:2973–7. doi: 10.1016/j.vaccine.2018.04.041
58. Methner U, Berndt A, Steinbach G. Combination of competitive exclusion and immunization with an attenuated live Salmonella vaccine strain in chickens. Avian Dis. (2001) 45:631–8. doi: 10.2307/1592904
59. Methner U, Barrow PA, Berndt A. Induction of a homologous and heterologous invasion-inhibition effect after administration of Salmonella strains to newly hatched chicks. Vaccine. (2010) 28:6958–63. doi: 10.1016/j.vaccine.2010.08.050
60. Sreekantapuram S, Lehnert T, Prausse MTE, Berndt A, Berens C, Figge MT, et al. Dynamic interplay of host and pathogens in an avian whole-blood model. Front Immunol. (2020) 11:500. doi: 10.3389/fimmu.2020.00500
61. Seliger C, Schaerer B, Kohn M, Pendl H, Weigend S, Kaspers B, et al. A rapid high-precision flow cytometry based technique for total white blood cell counting in chickens. Vet Immunol Immunopathol. (2012) 145:86–99. doi: 10.1016/j.vetimm.2011.10.010
62. Braukmann M, Methner U, Berndt A. Immune reaction and survivability of salmonella typhimurium and salmonella infantis after infection of primary avian macrophages. PloS One. (2015) 10:e0122540. doi: 10.1371/journal.pone.0122540
63. Sreekantapuram S, Berens C, Barth SA, Methner U, Berndt A. Interaction of Salmonella Gallinarum and Salmonella Enteritidis with peripheral leucocytes of hens with different laying performance. Vet Res. (2021) 52:123. doi: 10.1186/s13567-021-00994-y
64. Polasky C, Weigend S, Schrader L, Berndt A. Non-specific activation of CD8alpha-characterised gammadelta T cells in PBL cultures of different chicken lines. Vet Immunol Immunopathol. (2016) 179:1–7. doi: 10.1016/j.vetimm.2016.07.008
65. Gobel TW, Kaspers B, Stangassinger M. NK and T cells constitute two major, functionally distinct intestinal epithelial lymphocyte subsets in the chicken. Int Immunol. (2001) 13:757–62. doi: 10.1093/intimm/13.6.757
66. Carvajal BG, Methner U, Pieper J, Berndt A. Effects of Salmonella enterica serovar Enteritidis on cellular recruitment and cytokine gene expression in caecum of vaccinated chickens. Vaccine. (2008) 26:5423–33. doi: 10.1016/j.vaccine.2008.07.088
67. Braukmann M, Sachse K, Jacobsen ID, Westermann M, Menge C, Saluz HP, et al. Distinct intensity of host-pathogen interactions in Chlamydia psittaci- and Chlamydia abortus-infected chicken embryos. Infect Immun. (2012) 80:2976–88. doi: 10.1128/IAI.00437-12
68. Livak KJ, Schmittgen TD. Analysis of relative gene expression data using real-time quantitative PCR and the 2(-Delta Delta C(T)) Method. Methods. (2001) 25:402–8. doi: 10.1006/meth.2001.1262
69. Holt PS, Gast RK, Porter RE Jr., Stone HD. Hyporesponsiveness of the systemic and mucosal humoral immune systems in chickens infected with Salmonella enterica serovar enteritidis at one day of age. Poult Sci. (1999) 78:1510–7. doi: 10.1093/ps/78.11.1510
70. Ismail AS, Severson KM, Vaishnava S, Behrendt CL, Yu X, Benjamin JL, et al. Gammadelta intraepithelial lymphocytes are essential mediators of host-microbial homeostasis at the intestinal mucosal surface. Proc Natl Acad Sci U S A. (2011) 108:8743–8. doi: 10.1073/pnas.1019574108
71. Ribot JC, Lopes N, Silva-Santos B. gammadelta T cells in tissue physiology and surveillance. Nat Rev Immunol. (2021) 21:221–32. doi: 10.1038/s41577-020-00452-4
72. Hayday AC. gammadelta T cell update: adaptate orchestrators of immune surveillance. J Immunol. (2019) 203:311–20. doi: 10.4049/jimmunol.1800934
73. Vantourout P, Hayday A. Six-of-the-best: unique contributions of gammadelta T cells to immunology. Nat Rev Immunol. (2013) 13:88–100. doi: 10.1038/nri3384
74. Matulova M, Stepanova H, Sisak F, Havlickova H, Faldynova M, Kyrova K, et al. Cytokine signaling in splenic leukocytes from vaccinated and non-vaccinated chickens after intravenous infection with Salmonella enteritidis. PloS One. (2012) 7:e32346. doi: 10.1371/journal.pone.0032346
75. Dunon D, Imhof BA. T cell migration during ontogeny and T cell repertoire generation. Curr Top Microbiol Immunol. (1996) 212:79–93.
76. Chen Y, Chou K, Fuchs E, Havran WL, Boismenu R. Protection of the intestinal mucosa by intraepithelial gamma delta T cells. Proc Natl Acad Sci U S A. (2002) 99:14338–43. doi: 10.1073/pnas.212290499
77. Cheroutre H, Lambolez F, Mucida D. The light and dark sides of intestinal intraepithelial lymphocytes. Nat Rev Immunol. (2011) 11:445–56. doi: 10.1038/nri3007
78. Fischer MA, Golovchenko NB, Edelblum KL. gammadelta T cell migration: Separating trafficking from surveillance behaviors at barrier surfaces. Immunol Rev. (2020) 298:165–80. doi: 10.1111/imr.v298.1
79. Majeed S, Hamad SK, Shah BR, Bielke L, Nazmi A. Natural intraepithelial lymphocyte populations rise during necrotic enteritis in chickens. Front Immunol. (2024) 15:1354701. doi: 10.3389/fimmu.2024.1354701
80. Majeed S, Shah BR, Khalid N, Bielke L, Nazmi A. Dynamic changes in the intraepithelial lymphocyte numbers following salmonella typhimurium infection in broiler chickens. Anim (Basel). (2024) 14. doi: 10.3390/ani14233463
81. Dalton JE, Cruickshank SM, Egan CE, Mears R, Newton DJ, Andrew EM, et al. Intraepithelial gammadelta+ lymphocytes maintain the integrity of intestinal epithelial tight junctions in response to infection. Gastroenterology. (2006) 131:818–29. doi: 10.1053/j.gastro.2006.06.003
82. Li GQ, Xia J, Zeng W, Luo W, Liu L, Zeng X, et al. The intestinal gammadelta T cells: functions in the gut and in the distant organs. Front Immunol. (2023) 14:1206299. doi: 10.3389/fimmu.2023.1206299
83. Gladiator A, Wangler N, Trautwein-Weidner K, LeibundGut-Landmann S. Cutting edge: IL-17-secreting innate lymphoid cells are essential for host defense against fungal infection. J Immunol. (2013) 190:521–5. doi: 10.4049/jimmunol.1202924
84. Conti HR, Peterson AC, Brane L, Huppler AR, Hernandez-Santos N, Whibley N, et al. Oral-resident natural Th17 cells and gammadelta T cells control opportunistic Candida albicans infections. J Exp Med. (2014) 211:2075–84. doi: 10.1084/jem.20130877
85. Lombes A, Durand A, Charvet C, Riviere M, Bonilla N, Auffray C, et al. Adaptive Immune-like gamma/delta T Lymphocytes Share Many Common Features with Their alpha/beta T Cell Counterparts. J Immunol. (2015) 195:1449–58. doi: 10.4049/jimmunol.1500375
86. Jansen CA, van de Haar PM, van Haarlem D, van Kooten P, de Wit S, van Eden W, et al. Identification of new populations of chicken natural killer (NK) cells. Dev Comp Immunol. (2010) 34:759–67. doi: 10.1016/j.dci.2010.02.009
87. Schokker D, Smits MA, Hoekman AJ, Parmentier HK, Rebel JM. Effects of Salmonella on spatial-temporal processes of jejunal development in chickens. Dev Comp Immunol. (2010) 34:1090–100. doi: 10.1016/j.dci.2010.05.013
88. Meijerink N, van Haarlem DA, Velkers FC, Stegeman AJ, Rutten V, Jansen CA. Analysis of chicken intestinal natural killer cells, a major IEL subset during embryonic and early life. Dev Comp Immunol. (2021) 114:103857. doi: 10.1016/j.dci.2020.103857
89. Rudnicka K, Matusiak A, Chmiela M. CD25 (IL-2R) expression correlates with the target cell induced cytotoxic activity and cytokine secretion in human natural killer cells. Acta Biochim Pol. (2015) 62:885–94. doi: 10.18388/abp.2015_1152
90. Pescovitz MD, Lowman MA, Sachs DH. Expression of T-cell associated antigens by porcine natural killer cells. Immunology. (1988) 65:267–71.
91. Saalmuller A, Hirt W, Maurer S, Weiland E. Discrimination between two subsets of porcine CD8+ cytolytic T lymphocytes by the expression of CD5 antigen. Immunology. (1994) 81:578–83.
92. Freud AG, Mundy-Bosse BL, Yu J, Caligiuri MA. The broad spectrum of human natural killer cell diversity. Immunity. (2017) 47:820–33. doi: 10.1016/j.immuni.2017.10.008
93. Battella S, Cox MC, Santoni A, Palmieri G. Natural killer (NK) cells and anti-tumor therapeutic mAb: unexplored interactions. J Leukoc Biol. (2016) 99:87–96. doi: 10.1189/jlb.5VMR0415-141R
94. Srinivasan A, Foley J, McSorley SJ. Massive number of antigen-specific CD4 T cells during vaccination with live attenuated Salmonella causes interclonal competition. J Immunol. (2004) 172:6884–93. doi: 10.4049/jimmunol.172.11.6884
95. Withanage GS, Wigley P, Kaiser P, Mastroeni P, Brooks H, Powers C, et al. Cytokine and chemokine responses associated with clearance of a primary Salmonella enterica serovar Typhimurium infection in the chicken and in protective immunity to rechallenge. Infect Immun. (2005) 73:5173–82. doi: 10.1128/IAI.73.8.5173-5182.2005
96. Shanmugasundaram R, Acevedo K, Mortada M, Akerele G, Applegate TJ, Kogut MH, et al. Effects of Salmonella enterica ser. Enteritidis and Heidelberg on host CD4+CD25+ regulatory T cell suppressive immune responses in chickens. PloS One. (2021) 16:e0260280.
97. Johansson C, Ingman M, Jo Wick M. Elevated neutrophil, macrophage and dendritic cell numbers characterize immune cell populations in mice chronically infected with Salmonella. Microb Pathog. (2006) 41:49–58. doi: 10.1016/j.micpath.2006.03.004
98. Veldhoen M. Interleukin 17 is a chief orchestrator of immunity. Nat Immunol. (2017) 18:612–21. doi: 10.1038/ni.3742
99. Ishigame H, Kakuta S, Nagai T, Kadoki M, Nambu A, Komiyama Y, et al. Differential roles of interleukin-17A and -17F in host defense against mucoepithelial bacterial infection and allergic responses. Immunity. (2009) 30:108–19. doi: 10.1016/j.immuni.2008.11.009
100. Walliser I, Gobel TW. Generation of glycosylphosphatidylinositol linked chicken IL-17 to generate specific monoclonal antibodies applicable for intracellular cytokine staining. Dev Comp Immunol. (2017) 73:27–35. doi: 10.1016/j.dci.2017.03.006
101. Schulz SM, Kohler G, Holscher C, Iwakura Y, Alber G. IL-17A is produced by Th17, gammadelta T cells and other CD4- lymphocytes during infection with Salmonella enterica serovar Enteritidis and has a mild effect in bacterial clearance. Int Immunol. (2008) 20:1129–38. doi: 10.1093/intimm/dxn069
102. Shipkova M, Wieland E. Surface markers of lymphocyte activation and markers of cell proliferation. Clin Chim Acta. (2012) 413:1338–49. doi: 10.1016/j.cca.2011.11.006
Keywords: γδ T cells, knockout, chickens, Salmonella, compensation, CD8α T cells
Citation: Tetzlaff F, Methner U, von Heyl T, Menge C, Schusser B and Berndt A (2025) Compensatory mechanisms in γδ T cell-deficient chickens following Salmonella infection. Front. Immunol. 16:1576766. doi: 10.3389/fimmu.2025.1576766
Received: 14 February 2025; Accepted: 23 April 2025;
Published: 14 May 2025.
Edited by:
Angela Wahl, University of Alabama at Birmingham, United StatesReviewed by:
Wilhelm Gerner, The Pirbright Institute, United KingdomAli Nazmi, The Ohio State University, United States
Copyright © 2025 Tetzlaff, Methner, von Heyl, Menge, Schusser and Berndt. This is an open-access article distributed under the terms of the Creative Commons Attribution License (CC BY). The use, distribution or reproduction in other forums is permitted, provided the original author(s) and the copyright owner(s) are credited and that the original publication in this journal is cited, in accordance with accepted academic practice. No use, distribution or reproduction is permitted which does not comply with these terms.
*Correspondence: Angela Berndt, YW5nZWxhLmJlcm5kdEBmbGkuZGU=