- 1Florida Medical Entomology Laboratory, Department of Entomology and Nematology, University of Florida, Vero Beach, FL, United States
- 2Hawai’i Department of Health, Hilo, HI, United States
- 3School of Biological Sciences, Department of Cell and Development Biology, University of California, San Diego, San Diego, CA, United States
- 4U. S. Fish and Wildlife Service, Pacific Islands Fish and Wildlife Office, Honolulu, HI, United States
- 5Department of Entomology and Nematology, University of California, Davis, Davis, CA, United States
Recurring outbreaks of mosquito-borne diseases, like dengue, in the Pacific region represent a major biosecurity risk to neighboring continents through potential introductions of disease-causing pathogens. Aedes mosquitoes, highly prevalent in this region, are extremely invasive and the predominant vectors of multiple viruses including causing dengue, chikungunya, and Zika. Due to the absence of vaccines for most of these diseases, Aedes control remains a high priority for public health. Currently, international organizations put their efforts into improving mosquito surveillance programs in the Pacific region. Also, a novel biocontrol method using Wolbachia has been tried in the Pacific region to control Aedes mosquito populations. A comprehensive understanding of mosquito biology is needed to assess the risk that mosquitoes might be introduced to neighboring islands in the region and how this might impact arboviral virus transmission. As such, we present a comprehensive review of arboviral disease outbreak records as well as Aedes mosquito biology research findings relevant to the Pacific region collected from both non-scientific and scientific sources.
Introduction
Arthropod-borne viral (arboviral henceforth) diseases cause significant burden to global health. Around 400 million people have been infected dengue virus (DENV) per year, and of which 96 million people have undergone dengue fever (1). Chikungunya virus (CHIKV) and Zika virus (ZIKV) spread worldwide over the last decade and currently 77.9% and 48.6% of global human populations are living in the countries affected by CHIKV and ZIKV, respectively (2).
Both Aedes aegypti and Aedes albopictus are known major vectors of these arboviral diseases. Aedes aegypti uses artificial habitats to survive even outside of its temperature limit for development and Ae. albopictus can survive in much colder regions than Ae. aegypti, allowing them to be globally distributed (3). Although both species are similar in that they are highly adaptable, it is important to use different mosquito control strategies to each species since they have different habits and behaviors (3, 4). For example, the WHO recommended targeted indoor residual spraying to control Ae. aegypti, while recommending targeted outdoor residual spraying against Ae. albopictus (4).
Tropical islands, including the Pacific islands, typically have socio-economic, climatic, and human activity-related factors favorable for arbovirus outbreaks (5). Suboptimal healthcare infrastructure driven by factors including poverty can slow the timely detection of pathogens before outbreaks begin (5). The lack of water management infrastructure also serves to increase potential mosquito-breeding sites (5), and climate change has facilitated the spread of arboviruses in the Pacific (6). It has been suggested that dengue became an endemic disease in New Caledonia due to climate change (7).
The Pacific region experiences recurring outbreaks of arboviral diseases (1). The warm temperatures of the Pacific region make them a suitable environment for the transmission of arboviruses (8), including CHIKV, DENV, and ZIKV. No outbreaks of yellow fever virus (YFV) have been recorded in the region. These three viruses are transmitted by Aedes mosquitoes of the subgenus Stegomyia, mainly Aedes aegypti and Ae. albopictus, although additional species in the scutellaris group of this subgenus may be important local vectors in the Pacific (e.g., Ae. hensilli, Ae. polynesiensis, Ae. scutellaris, etc.) (9–13). Nonetheless, this review focuses on Ae. aegypti and Ae. albopictus mosquitoes.
Outbreaks
We collected records from scientific literature, news, reports, and online databases to compile reports of historical outbreaks in the Pacific region (Supplemental Table S1). Outbreak records in the Pacific region appeared in various sources, with gray literatures accounting for 12% of the total sources (Supplemental Table S2) (1). Based on our data, a total of 412 arboviral outbreaks were reported from 25 Pacific nations or territories (Figure 1) through December 2020. From 1971 to 2000, the incidence of arboviral disease in the Pacific remained relatively constant, with no more than thirty outbreaks documented within each ten-year interval (Figure 1). However, during the period between 2001 and 2010, the scale of arbovirus disease increased drastically, with outbreaks documented in 70 locations. From 2011 to 2020, a total of 153 outbreaks were reported, more than two times higher than the number of outbreaks from the previous period. The reason for the recent increase in arbovirus transmission in this region is yet to be determined.
Across the Pacific region, DENV was first reported in Hawaii in the late 1840s (14), and was subsequently reported in French Polynesia in the early 1850s (15). Outbreaks occurred sporadically until the mid-1920s, thereafter they increased until the 1940s when mosquito control was widely implemented, and a slight decrease in the occurrence of outbreaks was observed between 1941 to 1945. As this time frame overlaps with the height of the Pacific campaign of World War II, it is likely that such a major event impacted typical reporting of arboviral illnesses, as well as arbovirus transmission. Reports of dengue-like illness in the Western Pacific Region in the 1950s correlated with troop movements among dengue-endemic and non-endemic areas in the early to mid-1940s (16, 17). Nevertheless, there are no reports of dengue outbreaks following WWII, between 1945 and 1964, potentially due to the commencement of mosquito control activities using DDT, but cases reappeared in the mid-1960s (Figure 1). This gap in dengue outbreak records does not necessarily reflect a lack of DENV transmission during this period and highlights the difficulty to disentangle the issue of underreporting from low occurrence outbreak events. Despite this difficulty, a graph of the number of outbreaks in the Pacific region revealed the overall trend of increasing arboviral disease outbreaks over the entire period (Figure 1).
The first identification of a DENV infection in the Pacific region to the serotype level occurred in 1944, with DENV-1 detected in Tahiti, French Polynesia (15). Subsequently, DENV-3 was reported in the Windward Islands, French Polynesia in 1964 (15). The first reported DENV-2 cases occurred for individuals in American Samoa, Fiji, French Polynesia, Kiribati, New Caledonia, Papua New Guinea (PNG), Tonga, Tuvalu, and Wallis and Futuna in 1971, while the first report of DENV-4 in Pacific was from French Polynesia, New Caledonia, Samoa, and Wallis and Futuna in 1979 (18).
In contrast to DENV, other Aedes-transmitted arboviruses have a much shorter history in the region. CHIKV was first detected in the region in 2011 in New Caledonia, with subsequent outbreaks occurring in 2012 in PNG, in 2013 in the Federated State of Micronesia (FSM), in 2014 in the Cook Islands, French Polynesia, Tonga, American Samoa, Samoa, and Tokelau, and in 2015 in Fiji, and RMI, Kiribati, and Tuvalu (19–24). There were 17 total CHIKV outbreaks between 2011 and 2015, and three outbreaks between 2016 and 2020 (Figure 1).
The first ZIKV outbreaks in the Pacific region occurred in 2007 in the FSM, and subsequent cases emerged in 2013 in French Polynesia. The virus then spread to the Cook Islands, Easter Island, and New Caledonia in 2014, followed by Fiji, PNG, RMI, Samoa, Solomon Islands, Vanuatu in 2015, and then American Samoa, Kiribati, Palau, and Tonga in 2016 (22, 23, 25–29). Between 2006 and 2010, there was one outbreak of ZIKV. There were 12 outbreaks between 2011 and 2015, and 12 outbreaks between 2016 and 2020 (Figure 1).
Distribution of Aedes aegypti and Aedes albopictus
The Aedes aegypti group originated in Madagascar and/or islands in the Southwestern Indian Ocean and then spread to mainland Africa (30). The domesticated subspecies Ae. aegypti likely originated in West Africa where the species was initially forest-dwelling and zoophilic (31). It likely shifted its feeding preference from animals to humans and began using artificial containers as breeding sites when human settlements developed adjacent to forests in West Africa (31). A global genetic analysis reported that Ae. aegypti populations in the New World were established in the 15th and 16th centuries (32). It is possible that slave traders carried Ae. aegypti to European countries on their return from the New World, enabling persistence in the Mediterranean region until around 1950 (31). Their establishment in Asia was estimated to have occurred in the 19th century, and it is hypothesized to have occurred via the Mediterranean following the opening of the Suez Canal in 1869 (32).
It is believed that human activities introduced Ae. aegypti from Asia and/or the Americas to the Pacific region during the 19th century (33). The whaling industry and migration of Asian people to New Caledonia and French Polynesia may have contributed to the introduction of the Asian lineage of this species (33). Similarly, the whaling industry, international trade, and World War II are thought to have enabled the westward expansion of the American lineage into the Pacific region (33). Within the region, the earliest published record of Ae. aegypti was from Brisbane, Australia, in 1887 where the species was described by Skuse as Culex bancroftii (34). However, historical records of dengue-like illness and museum specimens that predate Skuse’s report suggest that Ae. aegypti had a wide distribution in eastern Australia by the latter part of the nineteenth century (35). Regional maritime trade within the Pacific region promoted the movement of Ae. aegypti larvae and eggs in water-storage containers from port to port (35), but because of its behavior and reliance on artificial containers for breeding sites, Ae. aegypti often stayed localized to port areas and typically only moved inland on larger populated islands when enabled by human activity (35). Consequently, Ae. aegypti has been broadly distributed throughout the Pacific for well over a century, but its local distribution within archipelagos has varied substantially between Pacific nations and territories (35–37).
Aedes albopictus is native to the forests of Southeast Asia (38). Like Ae. aegypti, it is an invasive species in the Pacific region. Within this region, Ae. albopictus has the longest history in Hawaii and was first documented as Ae. scutellaris in the early 1900s (39). Aedes albopictus was first reported in PNG in 1932 (40). Although some have discredited that record (36), collections from PNG in the 1960s and early 1970s indicate that it was present but relatively sparse compared to Ae. aegypti and Ae. scutellaris (41, 42). It likely spread to other Pacific region from there in the 1970s, as it was reported in the Solomon and Santa Cruz Islands, east of PNG, from collections made in 1978 (43). Similar to Ae. aegypti, Ae. albopictus was reported to use a wide variety of artificial containers in contrast to native Stegomyia species in the scutellaris group (43). Worldwide, the invasion of this species was mediated primarily through the international trade of used tires over the last 40 years (38), a mechanism that may have played a more recent role in the spread of Ae. albopictus between neighboring islands (44).
Currently, both species can be found in the Pacific region, but Ae. aegypti has colonized more islands than Ae. albopictus (Figure 2 and Supplementary Table S2). Aedes aegypti has been reported in American Samoa, Australia, the Cook Islands, Easter Island, FSM, Fiji, French Polynesia, Galápagos, Hawaii, Kiribati, Nauru, New Caledonia, Niue, Palau, PNG, Pitcairn Islands, RMI, Samoa, the Solomon Islands, Tokelau, Tonga, Tuvalu, Vanuatu, and Wallis and Futuna (45–51). In contrast, Ae. albopictus has been found in Australia, FSM, Fiji, Guam, Hawaii, Kiribati, Nauru, Northern Mariana Islands, Palau, PNG, RMI, Samoa, Solomon Islands, Tonga, and Vanuatu (4, 43–46, 48, 50, 52). Although the two species are not established in New Zealand, interceptions have been recorded from ports in Auckland, New Zealand (53).
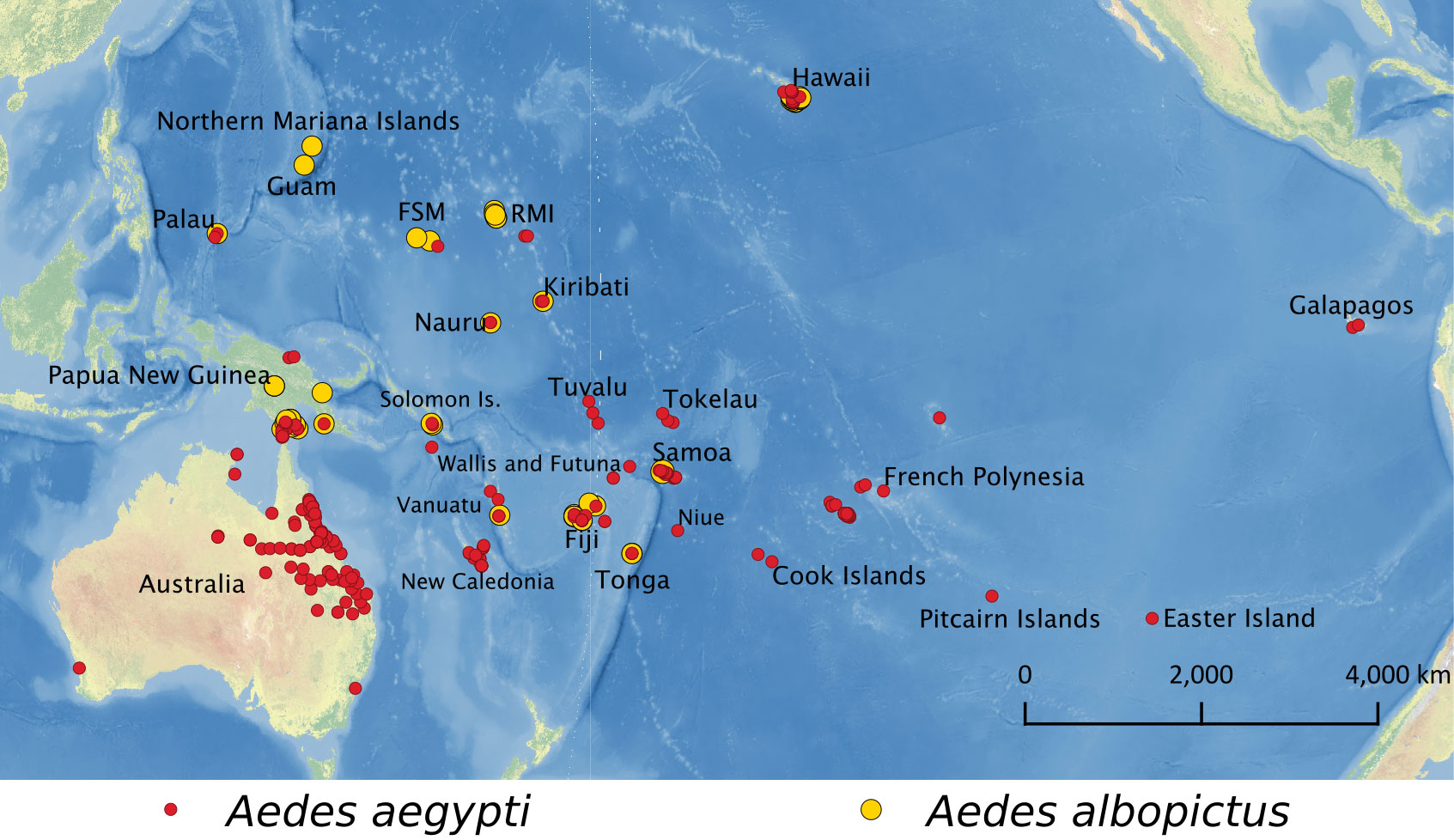
Figure 2 Locations where Ae. aegypti (red) and Ae. albopictus (yellow) have been reported in the Pacific region.
Transportation of goods and human travel can contribute to the dispersal of vectors and pathogens (54–57). Also, military activities during WWII and the Vietnam War provided opportunities for mosquitoes and the pathogens they transmit to migrate to the Pacific region (16, 17, 55). The introduction of arbovirus by airplane appears to be through infected humans rather than infected mosquitoes (58). Aedes aegypti and Ae. albopictus exhibit an extraordinary ability to move between and adapt to new environments (59). Both species have spread worldwide through cargo containers and tires (59–63). The frequency at which these new introductions occur is unknown. Genetic or genomic studies revealing the population origin and demographic history of various invasive Aedes populations could provide vital insight into this issue in the future.
Vector competence of Aedes aegypti and Aedes albopictus
Aedes aegypti is competent for several arboviruses such as CHIKV, DENV, YFV, and ZIKV. Its competence differs depending on the lineage of each virus and the population of the vector (64). Aedes albopictus is also competent for these same arboviruses, but based on studies utilizing mosquito populations from Africa, Europe, and the United States (Connecticut and New York), it has a greater vector competence for CHIKV than for DENV, ZIKV, or YFV based on (65–68). Both mosquito species can vary in their vector competence for different serotypes or strains of viruses (69–73), which might complicate the risk of disease transmission if multiple competent mosquito species and multiple virus lineages are circulating within a region.
Limited vector competence data are available for the Pacific region. Thus far, only Australia, French Polynesia, New Caledonia, and Samoa have reported the competence of Aedes mosquitoes for virus transmissions. Virus transmission efficiencies data from past studies are summarized in Table 1, where transmission efficiency was calculated as the number of mosquitoes with infected saliva divided by the total number of mosquitoes tested (79).
Overall, Ae. aegypti populations from the Pacific region appear to be efficient (>20%) at transmitting each DENV, ZIKV, and CHIKV (Table 1). However, across viral lineages and strains, CHIKV seemed to be consistently more infective to Ae. aegypti than DENV and ZIKV, with the highest transmission efficiencies observed between 53%-90% (5, 15, 69). Cases of extremely low (0%) and high (100%) efficiency of transmission have only been found for ZIKV (67, 73). Since the vector competent results in Table 1 were derived from different research settings, caution is required for the comparison and interpretation of results.
Specifically, Australian Ae. aegypti was particularly efficient in transmitting CHIKV (64% transmission efficiency at 14-15 days post-infection [dpi]) and ZIKV (Table 1). ZIKV strains from Cambodia (87% at 14 dpi) and Brazil (50-60% at 14 dpi) tended to be more efficiently transmitted than those from Tonga and Uganda (>20% at 14 dpi). Comparing with Australian populations, French Polynesian Ae. aegypti seemed to be a more efficient dengue vector (35% for DENV-1 at 21 dpi). French Polynesian Ae. aegypti was also highly competent in transmitting CHIKV (49% at 14 dpi) and had variable transmission efficiencies for different ZIKV strains. For ZIKV from French Polynesia, however, French Polynesian Ae. aegypti had significantly higher transmission efficiencies (36% at 14 dpi) than the Polynesian islands-endemic species Ae. polynesiensis (0% at 14 dpi) (81). New Caledonian Ae. aegypti was also efficient in CHIKV (20-90% at 14 dpi) and ZIKV (>20%) transmission, particularly for the ZIKV African lineage (max 100% at 14 dpi). Moreover, this mosquito population was more susceptible to DENV-1 than the French Polynesian population. Although its rates of infection and dissemination varied between DENV serotypes and genotypes, transmission efficiencies of New Caledonian Ae. aegypti are similar (ranging from 3-16% at 14 dpi) with the exception of a DENV-1 isolated from genotype I (21% at 14 dpi) (82). As for Samoan Ae. aegypti, a single study investigated vector competence for ZIKV and reported a low transmission efficiency (2% at 14 dpi), which contrasts with other Ae. aegypti populations from Australia, French Polynesia, and New Caledonia (69). In combination, these results highlight the potential importance of local variation in vector competence for arbovirus epidemiology. Vector competencies of Ae. albopictus populations among the Pacific region were only tested in the Australian population.
The co-circulation of multiple viruses in a region may increase the occurrence of co-infection and therefore influence arboviral transmissions (71). Studies have implied that Aedes mosquitoes could cause concurrent outbreaks when coinfected with arboviruses; however, co-infection led to varying outcomes for transmission depending on the viruses involved in experimental infection. Although Aedes mosquitoes from the Pacific regions have not been investigated for co-infection under experimental conditions, co-infection of DENV and ZIKV and even co-infection with three viruses has been observed in Mexican Ae. aegypti (84). Besides, Aedes aegypti was shown to be capable of transmitting CHIKV and ZIKV simultaneously without a significant reduction in transmission rates (84, 85). Aedes albopictus from Reunion Island was able to co-transmit both CHIKV and DENV-1 (86). In nature, little is still known about co-infection and co-transmission of arboviruses, but Ae. aegypti and Ae. albopictus appear to be capable of transmitting multiple arboviruses in a single bite (87). To date, there have been no studies about simultaneous transmission by the one single mosquito in the Pacific, but two patients co-infected with DENV and ZIKV have been reported in New Caledonia in 2014 (88). Since each patient was infected with a different serotype of DENV and had a different travel history, it is assumed that there were at least two co-infections (88). In the Pacific region where CHIKV, ZIKV, and all of serotypes of DENV are circulating, co-infection is a subject we need to pay close attention to.
Insecticide resistance
Insecticide resistance refers to the ability of insects to survive the exposure to a standard dose of insecticide, with this ability mediated through physiological or behavioral adaptation (4). There are three major mechanisms driving insecticide resistance in mosquitoes: (a) Target-site resistance, which decreases the affinity of insecticides to the target protein by mutation(s) in the protein’s gene, (b) metabolic resistance, which is caused by overexpression or conformational change of enzymes involved in detoxification and xenobiotic metabolism, and (c) penetration resistance, which refers to cuticular modifications that interrupt penetration of insecticide into insects’ body (89, 90). Insecticide resistance against all four major insecticides has evolved in Ae. aegypti and Ae. albopictus via both the target-site and metabolic resistance mechanisms while penetration resistance has been largely uncharacterized in Aedes mosquitoes (89).
Insecticide resistance management should be included in vector control strategies because there is a limited number of insecticide classes, with many of these used readily in pest management programs on a global scale (91). There are only four insecticide classes approved for adult mosquito control by the World Health Organization (WHO) (pyrethroids, organophosphates, organochlorines, and carbamates), and the threat of insecticide resistance has been increasing due to this limitation (92). Of these four insecticide classes, only pyrethroids and organophosphates were used in the Western Pacific Region from 2000 to 2009, with known usage in the Cook Islands, Fiji, Kiribati, FSM, Nauru, Palau, the Solomon Islands, Tonga, Tuvalu, and Vanuatu (93).
The determination of insecticide resistance of Aedes mosquitoes in the Pacific region is performed using the CDC bottle bioassay and/or the WHO insecticide resistance test, two of the most utilized biological assays. Both methods determine mosquito mortality after a specific insecticide exposure time (diagnostic time) with minor differences in the guidelines for interpreting results (Table 2) (94, 95). Resistance ratios (RR) are often used in the literature when reporting insecticide resistance of mosquito populations (95). Resistance ratios are defined as the ratio of lethal concentrations of insecticide which results in 50% mortality (LC50) of the test population to the LC50 of a susceptible strain. An RR < 5 indicates that the mosquito population is susceptible to the applied insecticide, an RR between 5-10 indicates moderate resistance, and an RR >10 indicates a highly resistant population (95).
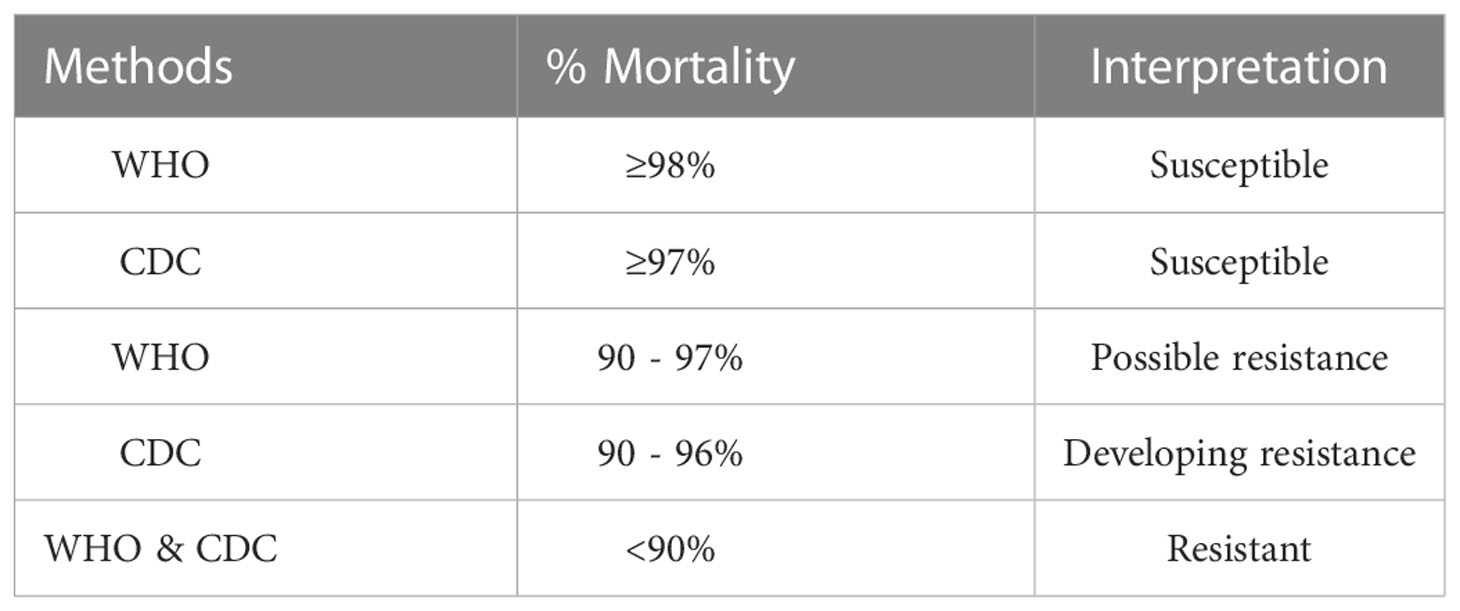
Table 2 Guidelines for interpreting results using the CDC bottle bioassay and the WHO insecticide resistance test.
Pyrethroid resistance in Ae. aegypti and Ae. albopictus has been reported worldwide, with a higher level of resistance observed in Ae. aegypti (96). Mutations in domains II and III of Voltage-gated sodium channel (VGSC) genes have been observed in Ae. aegypti from several populations in the Pacific region (V1016G + S989P in Vanuatu and Kiribati, and F1534C in New Caledonia, Fiji, and Kiribati) (97). Additionally, upregulations of detoxifying cytochrome P450 genes were identified in Ae. aegypti from New Caledonia (98). Interestingly, resistance against pyrethroids was not observed in Ae. aegypti from Queensland, Australia (97). The overall pyrethroids resistance profile of Ae. aegypti populations in the Pacific region is shown in Figure 3. Organophosphates ranked first for usage in the Western Pacific Region although the usage of organophosphates worldwide for the control of vector-borne diseases was less than 10% from 2001 to 2009 (93). The majority of Aedes aegypti populations in the Pacific region were susceptible to organophosphates. Aedes aegypti populations in French Polynesia and PNG were susceptible to organophosphates (malathion and/or temephos) (99, 100). Developments of resistance against organophosphate have been found in two Aedes mosquitoes in Fiji, New Caledonia, and Palau since 2010 (Table 3).
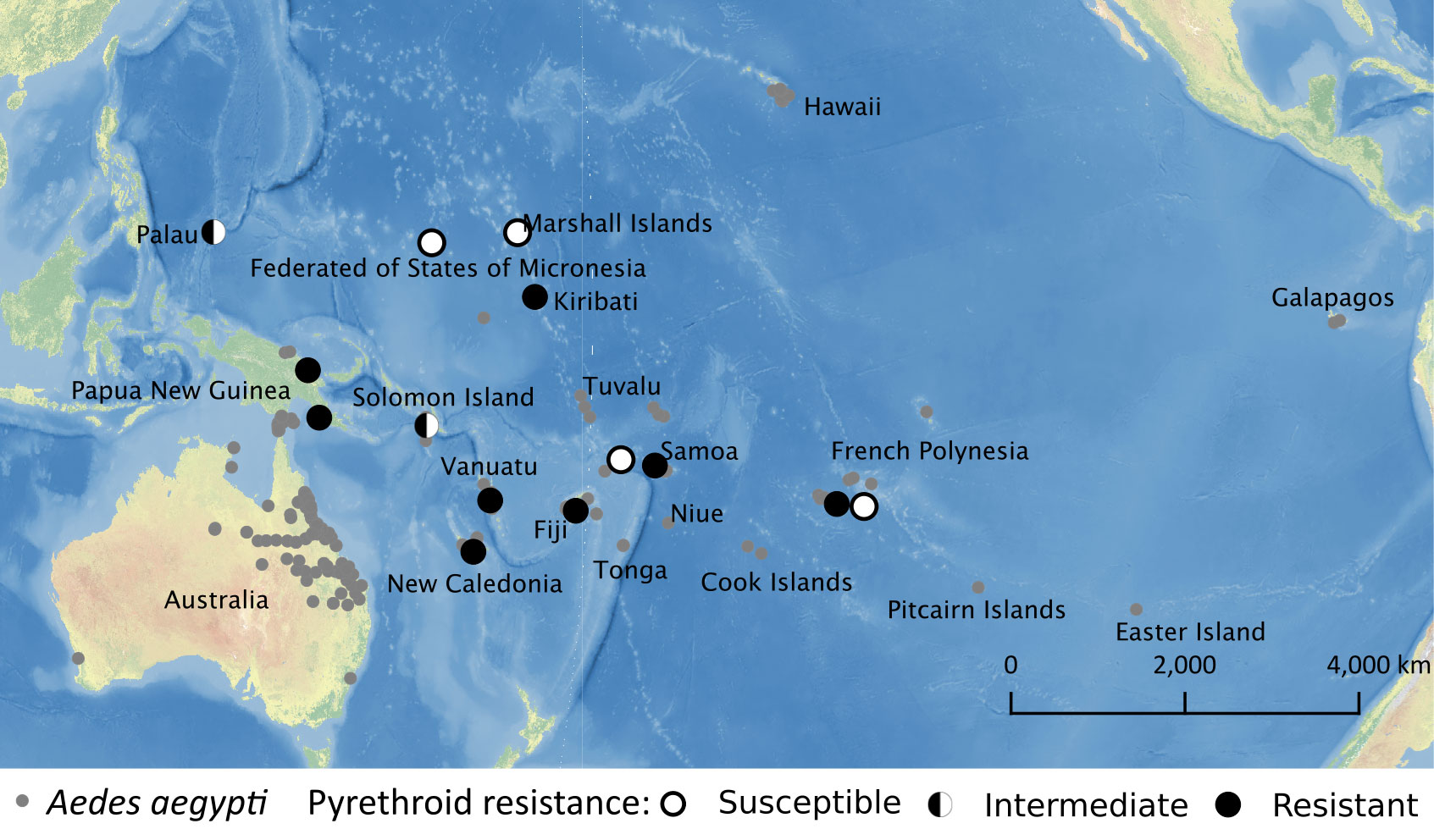
Figure 3 Pyrethroid resistance profile of Ae. aegypti available as of March 2022 overlaid with historical Ae. aegypti occurrence data.
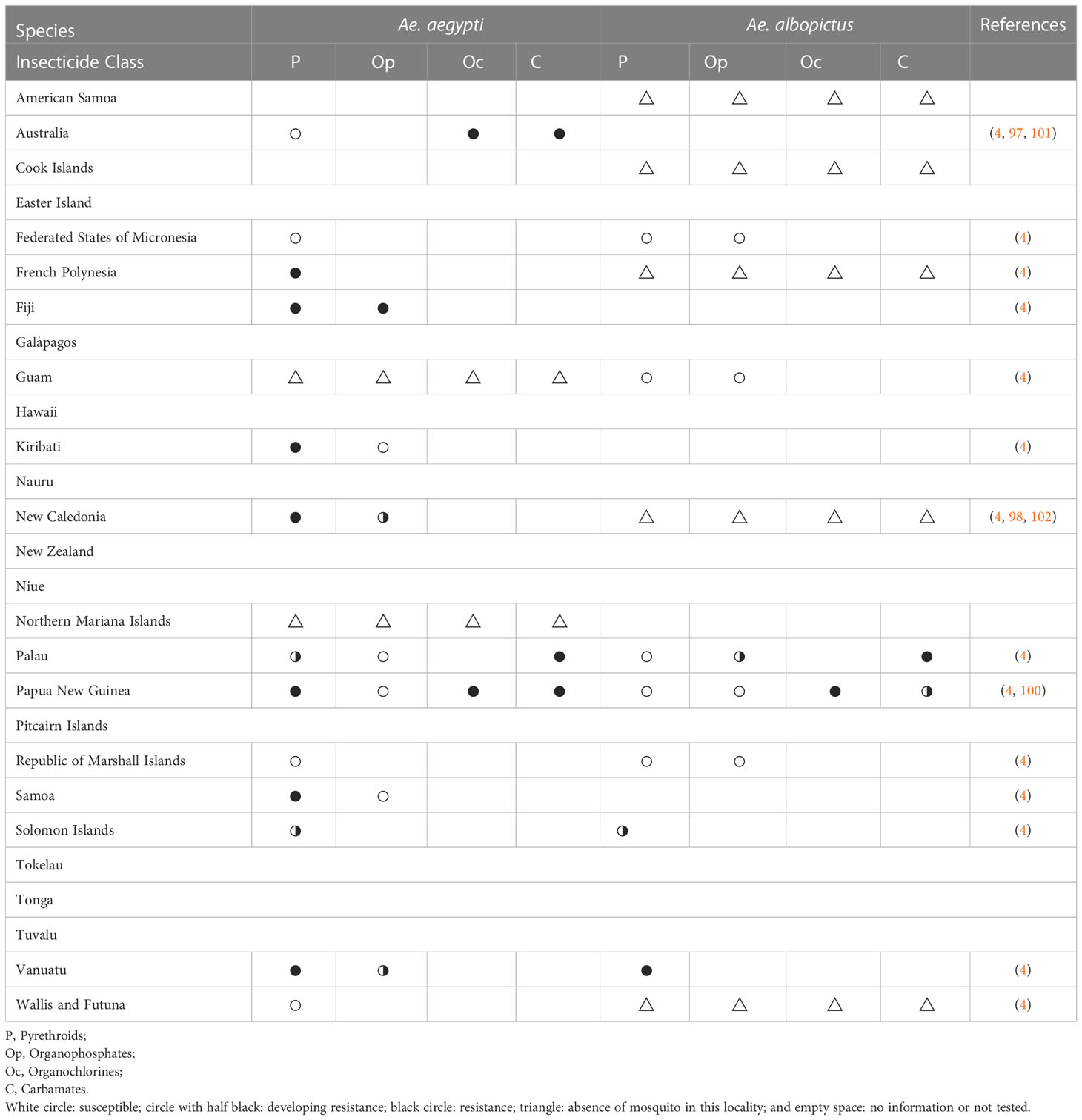
Table 3 Outcomes of insecticide resistance testing for Ae. aegypti and Ae. albopictus from the Pacific region since 2010.
Although several populations in the Pacific region are testing for mosquito insecticide resistance, there are still Aedes populations where this practice has not been implemented since 2010 or which no information was available (Table 3). Given that insecticide resistance was detected in Ae. aegypti populations which inhabit the same island with untested Ae. albopictus, it is possible that there are more insecticide resistant Ae. albopictus populations. All tested Ae. aegypti and Ae. albopictus populations showed resistance to organochlorines and carbamates insecticides despite low usages (93). It may be due to the high concentrations of those insecticides remaining in the Pacific region (103). For a better mosquito control, it is important that all countries in the region determine the insecticide resistance status of their mosquito populations, share it with the entire region and work together to find strategies to control and prevent mosquito insecticide resistance which ultimately will reduce the prevalence of mosquito-borne disease in the Pacific region.
Population genetics
Multiple population genetics studies have been conducted for Ae. aegypti populations from the Pacific region (32, 33, 46, 104). A recent study measured genetic differentiation using 12 microsatellite loci in 79 populations of Ae. aegypti from 30 countries across six continents, including three Pacific regions – Australia, Hawaii, and Tahiti (32). The Pacific group, including two Australian populations, was genetically closer to the Asian Ae. aegypti populations than to those from other continents (32). Another study analyzed nine microsatellites and two mitochondrial DNA loci (COI and ND4) from 270 Ae. aegypti individuals collected from Fiji, French Polynesia, New Caledonia, and Tonga, and identified both Asian and American genetic lineages (33). A further study using a double-digest restriction site-associated (ddRAD) sequencing protocol for 224 individuals examined the genetic structure of Ae. aegypti populations from the Indo-Pacific regions, including Australia, Kiribati, Fiji, New Caledonia, and Vanuatu (46). Single nucleotide polymorphism (SNP) derived genotypes from ddRAD sequencing indicated that Ae. aegypti from the Pacific region were distinct from Asian or Australian Ae. aegypti lineages as shown in Figure 4 (46). This suggests that populations from Australia and other Pacific regions may have different invasion histories (46, 104). The genetic relatedness of Ae. aegypti within and between other Pacific nations and territories is yet to be investigated.
Aedes aegypti has a longer history in the Pacific region compared to Ae. albopictus, which spread more rapidly around the world through trade in a shorter time frame. This difference in time scale has likely influenced the relatedness of populations of the two species across the Pacific region. Aedes albopictus populations in Fiji and Nauru may be genetically similar to those from mainland Southeast Asia, but additional specimens must be sequenced to definitively conclude this (52). Population genomics using ddRAD data also demonstrated that Fiji Ae. albopictus populations were closely related to those from Southeast Asia, but Vanuatu populations were distinct as shown in Figure 5 (46). Results of analyses from COI and 13 microsatellite loci revealed that Ae. albopictus populations from the Southern Fly River in PNG and the Torres Strait Islands in Australia were introduced from the Indonesian region (52, 105). However, Ae. albopictus populations from other locations in PNG showed distinct genetic structures when compared with those from the Southern Fly River (105). A study comparing possible historical routes of Ae. albopictus invasions indicated that PNG populations, except for the Southern Fly River population, likely came from the mainland of southeast Asia and then became the source of Ae. albopictus that was established in the Solomon Islands (52). In contrast, the Hawaii population appeared more closely related to the East Asian population than to the Southeast Asian population (52, 106). It is plausible that Ae. albopictus from other Pacific nations and territories would demonstrate close relatedness to either Southeast Asian or East Asian Ae. albopictus, but more data from more Pacific nations and territories needs to be collected to test that hypothesis. This is because their genetic structure was not associated with distance, but with human transportation routes, suggesting Ae. albopictus enables to disperse even over long distances (46).
Surveillance and novel mosquito control strategies
The Pacific region continues to experience outbreaks of mosquito-borne diseases representing a major biosecurity threat to the neighboring continents (Figure 1). Due to the high prevalence and invasive characteristics of Ae. aegypti and Ae. albopictus mosquitoes and the lack of vaccines and treatments for many mosquito-transmitted diseases, it is of utmost importance to conduct continual surveillance to assess outbreak risk and/or assess the response to an active outbreak and enable mosquito control programs to reduce the incidence of these diseases. Figure 6 shows the timeline for an overview of major events related to mosquito-borne diseases in the Pacific region, which are discussed in more detail below.
The Pacific Community (SPC), which was founded in 1947, is a scientific and technical organization with members of 27 Pacific nations and territories. SPC and WHO established the Pacific Public Health Surveillance Network (PPHSN) in 1996 (https://www.pphsn.net). PPHSN prioritizes surveillance of infectious diseases including dengue fever in the Pacific (107). The SPC’s Public Health Division, which established in 2009, provides timely alerts of epidemics and emerging diseases in the Pacific region (https://www.spc.int/phd/epidemics/). This system is based on integrated data collected by routine surveillance systems and informal sources such as media and personal communications.
To improve the capacity for vector surveillance and control across the Pacific region, SPC and WHO have been providing a standardized methodology to mosquito control program staff. SPC and WHO Division of Pacific Technical Support distributed a manual for the surveillance and control of Aedes vectors in the Pacific region in 2020 (4). Additionally, the Pacific Mosquito Surveillance Strengthening for Impact (PacMOSSI) was launched by James Cook University, SPC, and WHO in 2021. It focuses on providing systematic surveillance, control of Aedes mosquitoes, and training control program staff. This project utilizes an online platform that provides (a) assessments to determine vector surveillance and control strengths and needs for the Pacific region; (b) training to use web-based data management to support the country and regional mosquito surveillance; (c) training on vector surveillance and control best practices, mosquito species identification, and insecticide resistance testing; (d) grants to support country-specific research to generate data for the improvement of mosquito control and surveillance; and (e) support for countries developing Aedes surveillance and control plans aligned with best practices (108). Information on Aedes vectors generated by these standardized methods will help us understand the current state of the Pacific region consisting of dozens of different countries and territories.
The Incompatible insect technique (IIT) is a control strategy using Wolbachia, an endosymbiotic bacterium found in many insect species. Uninfected female mosquitoes are not able to produce viable eggs when they mate with males infected with Wolbachia (109). Wolbachia-based mosquito suppression strategies have been tried in Australia for Ae. aegypti control (110), and French Polynesia for Ae. polynesiensis control (111). Aedes aegypti carrying wAlbB2-F4 were released to the Northern Cassowary Coast regions of Australia where wild-type Ae. aegypti and wMel-Wolbachia infected Ae. aegypti coexisted. Over the 20-week period of release in 2018, over 80% of suppression in Ae. aegypti populations was achieved in the release sites, and one of the three sites showed over 97% suppression 11 months later (110). Aedes polynesiensis carrying Wolbachia were released at a hotel operating on the private atoll of Tetiaroa, north of Tahiti occupying approximately 1 km2 area for a 12-month period between 2015 and 2016. This pilot study achieved successful suppression of the local Ae. polynesiensis population and the 2nd trial with an 18-month period was conducted between 2018-2020. The study reported a noticeable drop in mosquito bites perceived by hotel visitors and hotel workers (112).
Another approach involving Wolbachia relies on cytoplasmic incompatibility to replace the natural mosquito population with Wolbachia-infected mosquitoes (46, 113). Aedes aegypti carrying Wolbachia have a lower susceptibility to infection with viruses including dengue, Zika, chikungunya, yellow fever, and Mayaro viruses, and also display reduced transmission potential (113–118). Aedes aegypti infected with the wMel strain of Wolbachia (originally found in Drosophila melanogaster) was successfully introduced into two natural Ae. aegypti populations in Yorkeys Knob and Gordonvale, Australia (119). Continuous monitoring for two years showed that Wolbachia infection frequencies persisted at high levels in both populations (120). In 2013, Ae. aegypti carrying wMel Wolbachia were released in three areas around Cairns, Australia, and this release suggested the possibility that wMel Wolbachia-infected Ae. aegypti can become established in urban areas (121). For 28 months from October 2014, 4 million wMel Wolbachia-infected Ae. aegypti were released across a total area of 66km2 in Townsville, Australia (122). During this period, although imported DENV cases from overseas were continuously reported, only one case of locally acquired DENV occurred in this region (122).
A similar result in controlling local infection of DENV was also observed during the period of release of Ae. aegypti with wMel Wolbachia conducted from 2011 to 2017 in Cairns, Australia (123). The same method has been implemented and monitored in Fiji since 2017, Vanuatu since 2018, and Kiribati and New Caledonia since 2019 by the World Mosquito Program (124). Based on these successes, Hawaii departments are considering using Wolbachia to control mosquitoes to improve their public health as well as for avian population conservation given high case rates of avian malaria (125). In Australia, experimental releases of another, more pathogenic Wolbachia strain (wMelPop) were also conducted, but the frequency of the infection steadily decreased within the local Ae. aegypti populations after releases were halted (126). Overall, replacement strategies with the wMel strain of Wolbachia significantly reduced the possibility of being infected by DENV in the field application.
To overcome the limitation of current insecticide- and environmental maintenance-based current control methods, various novel transgenic-based approaches have been developed. The transgenic approaches for suppressing mosquito populations include producing sterile males or lethal toxic products to offspring and inducing a sex bias ratio. However, careful consideration before implementation is required (109). To date, no transgene control approaches have been applied for mosquito control in the Pacific region. However, weekly releases of Ae. aegypti eggs generated by the precision-guided sterile insect technique (pgSIT) were simulated on Onetahi, Teti’aroa, French Polynesia to explore the potential of pgSIT to suppress the wild Ae. aegypti population (127). The mathematical simulation demonstrated that population elimination was the common result for large release schemes such as 18 weekly releases of 200 pgSIT eggs per wild-type adult.
Future studies needed
There is a paucity of scientific literature on Aedes mosquitoes in the Pacific region and scientific understanding of arboviral disease in this region has been complicated because many arboviral disease outbreaks records and Aedes surveillance data have not been published in standard scientific journals. This represents vital information for students, scientists, government regulators, mosquito control programs, and public health officials. Obtaining current information about Aedes mosquitoes and arbovirus disease outbreak records is critical to improving decision support capacity and will spur future research in this region.
Although Aedes albopictus is also a major vector of CHIKV, DENV, and ZIKV in the Pacific regions, it has been less studied than Ae. aegypti. For example, its vector competence has only been studied for one Australian population. Due to the lack of information about its vector competence, it is challenging to properly evaluate the risk of Ae. albopictus represents as a vector in the Pacific region. Additionally, since insecticide resistance tests have only been conducted on a small number of islands, it is likely that many control programs are proceeding without any consideration of chemical efficacy. Further studies in these areas are essential for the long-term, persistent control of Ae. albopictus in this region.
Over the last two decades, there has been rapid development of novel Aedes mosquito control strategies (128). In addition to the Wolbachia biocontrol strategies, active laboratory studies are being conducted to develop improved sterile insect techniques using a genetic engineering approach (127). All these novel control strategies depend on the ability of mosquitoes to mate and disperse in a natural setting. A comprehensive understanding of mosquito biology is needed to assess the risk of novel mosquito control strategies about introduction to neighboring islands in the region and their potential impact on arboviral disease transmission. In this review, we have outlined several major knowledge gaps that must be addressed to facilitate the development of a road map for future mosquito research and mosquito control activities in the Pacific region.
Author contributions
SS, AV, and YL conceived the study. SS, CR, JM, AM, ME, AR-W, and BG conducted literature search and data collection. SS, CJ, and YL conducted field collection of Aedes mosquitoes in Hawai’i Island. AV conducted field collection of Aedes mosquitoes in the Republic of Marshall Islands. AV, YL, and JC contributed to Aedes albopictus data collection. XW, OA, RR, DM, EC, AV, JC contributed to data analysis and manuscript editing. All authors contributed to the article and approved the submitted version.
Funding
We acknowledge funding support from the Pacific Southwest Regional Center of Excellence for Vector-Borne Diseases funded by the U.S. Centers for Disease Control and Prevention (Cooperative Agreement 1U01CK000516), the USDA National Institute of Food and Agriculture (Multistate Hatch project 1025565), U.S. Environmental Protection Agency Science To Achieve Results (STAR) Award (# 84020401), NIH award (R01AI151004), University of Florida Institute of Food and Agricultural Sciences (UF/IFAS) Entomology and Nematology Department Matching Assistantship support to SS, UF College of Agricultural and Life Sciences William C. and Bertha M. Cornett Fellowship to SS, and ad hoc funds from the Hawai’i Department of Health to purchase some supplies.
Acknowledgments
We thank Dr. Limb Hapairai from Pacific Islands Health Officers’ Association (PIHOA) for sharing up-to-date information on Aedes species distribution in Guam and Northern Mariana Islands. We thank Dr. Dennis A. LaPointe from Pacific Island Ecosystem Research Center, USGS, Hawaii National Park for sharing his past Aedes collection experience with our team. The findings and conclusions in this article are those of the author(s) and do not necessarily represent the views of the funding agencies or the U.S. Fish and Wildlife Service. This research has been supported by a grant from the U.S. Environmental Protection Agency’s Science to Achieve Results (STAR) program. This publication was developed under Assistance Agreement No. 84020401 awarded by the U.S. Environmental Protection Agency to OSA. It has not been formally reviewed by EPA. The views expressed in this document are solely those of authors and do not necessarily reflect those of the Agency. EPA does not endorse any products or commercial services mentioned in this publication.
Conflict of interest
OSA is a founder of both Agragene, Inc. and Synvect, Inc. with equity interest. The terms of this arrangement have been reviewed and approved by the University of California, San Diego in accordance with its conflict of interest policies.
The remaining authors declare that the research was conducted in the absence of any commercial or financial relationships that could be construed as a potential conflict of interest.
Publisher’s note
All claims expressed in this article are solely those of the authors and do not necessarily represent those of their affiliated organizations, or those of the publisher, the editors and the reviewers. Any product that may be evaluated in this article, or claim that may be made by its manufacturer, is not guaranteed or endorsed by the publisher.
Supplementary material
The Supplementary Material for this article can be found online at: https://www.frontiersin.org/articles/10.3389/fitd.2023.1035273/full#supplementary-material
Supplementary Table 1 | Arboviral outbreak records in the Pacific Islands.
Supplementary Table 2 | The number of arbovirus disease outbreaks in the Pacific region at 10-year intervals.
Supplementary Data Sheet 1 | Aedes albopictus and Aedes aegypti distribution records in the Pacific Islands.
References
1. Bhatt S, Gething PW, Brady OJ, Messina JP, Farlow AW, Moyes CL, et al. The global distribution and burden of dengue. Nature (2013) 496:504–7. doi: 10.1038/nature12060
2. Puntasecca CJ, King CH, Labeaud AD. Measuring the global burden of chikungunya and zika viruses: A systematic review. PLoS Negl Trop Dis (2021) 15(3):e0009055. doi: 10.1371/journal.pntd.0009055
3. Reinhold J, Lazzari C, Lahondère C. Effects of the environmental temperature on Aedes aegypti and Aedes albopictus mosquitoes: A review. Insects (2018) 9(4):158. doi: 10.3390/insects9040158
4. Pacific Community, World Health Organization. Manual for surveillance and control of aedes vectors in the pacific. Suva, Fiji: Pacific Community and World Health Organization (2020).
5. Mavian C, Dulcey M, Munoz O, Salemi M, Vittor AY, Capua I. Islands as hotspots for emerging mosquito-borne viruses: A one-health perspective. Viruses (2018) 11(1):11. doi: 10.3390/v11010011
6. Filho WL, Scheday S, Boenecke J, Gogoi A, Maharaj A, Korovou S. Climate change, health and mosquito-borne diseases: Trends and implications to the pacific region. Int J Environ Res Public Health (2019) 16(24):5114. doi: 10.3390/ijerph16245114
7. Ochida N, Mangeas M, Dupont-Rouzeyrol M, Dutheil C, Forfait C, Peltier A, et al. Modeling present and future climate risk of dengue outbreak, a case study in new Caledonia. Environ Health (2022) 21:20. doi: 10.1186/s12940-022-00829-z
8. Ciota AT, Keyel AC. The role of temperature in transmission of zoonotic arboviruses. Viruses (2019) 11(11):1013. doi: 10.3390/v11111013
9. Rosen L, Rozeboom LE, Sweet BH, Sabin AB. The transmission of dengue by aedes polynesiensis marks. Am J Trop Med Hyg (1954) 3(5):878–82.
10. Savage HM, Fritz CL, Rutstein D, Yolwa A, Vorndam V, Gubler DJ. Epidemic of dengue-4 virus in yap state, federated states of Micronesia, and implication of aedes hensilli as an epidemic vector. Am J Trop Med Hyg (1998) 58(4):519–24.
11. Richard V, Paoaafaite T, Cao-Lormeau VM. Vector competence of Aedes aegypti and Aedes polynesiensis populations from French Polynesia of chikungunya virus. PLoS Negl Trop Dis (2016) 10(5):e0004694. doi: 10.1371/journal.pntd.0004694
12. Moore PR, Johnson PH, Smith GA, Ritchie SA, van den Hurk AF. Infection and dissemination of dengue virus type 2 in Aedes aegypti, aedes albopictus, and Aedes scutellaris from the Torres strait, Australia. J Am Mosq Control Assoc (2007) 23(4):383–8. doi: 10.2987/5598.1
13. Guillaumot L. Arboviruses and their vectors in the pacific – status report. Pac Health Surveill Response (2005) 12(2):45–52.
14. Effler PV, Pang L, Kitsutani P, Vorndam V, Nakata M, Ayers T, et al. Dengue fever, Hawaii, 2001-2002. Emerg Infect Dis (2005) 11(5):742–9. doi: 10.3201/eid1105.041063
15. Chungue E, Deparis X, Murgue B. Dengue in French Polynesia: Major features, surveillance, molecular epidemiology and current situation. Dengue Bull (1998) 12, 74–93.
16. Kuno G. Research on dengue and dengue-like illness in East Asia and the Western pacific during the first half of the 20th century. Rev Med Virol (2007) 17(5):327–41. doi: 10.1002/rmv.545
17. Gibbons RV, Streitz M, Babina T, Fried JR. Dengue and US military operations from the Spanish-American war through today. Emerg Infect Dis (2012) 18(4):623–30. doi: 10.3201/eid1804.110134
18. Singh N, Kiedrzynski T, Lepers C, Benyon EK. Dengue in the pacific – and update of the current situation. Pac Health Surveill Response (2005) 12(2):111–9.
19. Cao-Lormeau VM, Musso D. Emerging arboviruses in the pacific. Lancet (2014) 384:1571–2. doi: 10.1016/S0140-6736(14)61977-2
20. Dupont-Rouzeyrol M, Caro V, Guillaumot L, Vazeille M, D’Ortenzio E, Thiberge JM, et al. Chikungunya virus and mosquito vector Aedes aegypti in new Caledonia (South Pacific region). Vector-Borne Zoonotic Dis (2012) 12(12):1036–41. doi: 10.1089/vbz.2011.0937
21. Aubry M, Teissier A, Roche C, Richard V, Yan AS, Zisou K, et al. Chikungunya outbreak, French Polynesia, 2014. Emerg Infect Dis (2015) 21(4):724. doi: 10.3201/eid2104.141741
22. Matthews RJ, Kaluthotage I, Russell TL, Knox TB, Horwood PF, Craig AT. Arboviral disease outbreaks in the pacific islands countries and areas, 2014 to 2020: a systematic literature and document review. Pathog (2022) 11(1):74. doi: 10.3390/pathogens11010074
23. Gideon. (2021). Available at: https://app.gideononline.com/explore (Accessed October 1, 2021).
24. Roth A, Mercier A, Lepers C, Hoy D, Duituturaga S, Benyon E, et al. Concurrent outbreaks of dengue, chikungunya and zika virus infections – an unprecedented epidemic wave of mosquito-borne viruses in the Pacific 2012-2014. Euro surveill (2014) 19(41):20929. doi: 10.2807/1560-7917.ES2014.19.41.20929
25. Cao-Lormeau VM, Roche C, Teissier A, Robin E, Berry AL, Mallet HP, et al. Zika virus, French Polynesia, South Pacific, 2013. Emerg Infect Dis (2014) 20(6):1085. doi: 10.3201/eid2006.140138
26. Healy JM, Burgess MC, Chen TH, Hancock WT, Toews KAE, Anesi MS, et al. Notes from the field: Outbreak of zika virus disease – American Samoa, 2016. Morb Mortal Wkly Rep (2016) 65:1146–7. doi: 10.15585/mmwr.mm6541a4
27. Simon O, Acket B, Forfait C, Girault D, Gourinat AC, Millon P, et al. Zika virus outbreak in new Caledonia and Guillain-Barré syndrome: A case-control study. J Neurovirol (2018) 24(3):362–8. doi: 10.1007/s13365-018-0621-9
28. Duffy MR, Chen TH, Hancock WT, Powers AM, Kool JL, Lanciotti RS, et al. Zika virus outbreak on yap island, federated states of Micronesia. N Engl J Med (2009) 360(24):2536–43. doi: 10.1056/NEJMoa0805715
29. Tognarelli J, Ulloa S, Villagra E, Lagos J, Aguayo C, Fasce R, et al. A report on the outbreak of Zika virus on Easter island, South Pacific, 2014. Arch Virol (2016) 161(3):665–8. doi: 10.1007/s00705-015-2695-5
30. Soghigian J, Gloria-Soria A, Robert V, le Goff G, Failloux AB, Powell JR. Genetic evidence for the origin of Aedes aegypti, the yellow fever mosquito, in the southwestern Indian ocean. Mol Ecol (2020) 29(19):3593–606. doi: 10.1111/mec.15590
31. Powell JR, Gloria-Soria A, Kotsakiozi P. Recent history of Aedes aegypti: Vector genomics and epidemiology records. Biosci (2018) 68(11):854–60. doi: 10.1093/biosci/biy119
32. Gloria-Soria A, Ayala D, Bheecarry A, Calderon-Arguedas O, Chadee DD, Chiappero M, et al. Global genetic diversity of. Aedes aegypti Mol Ecol (2016) 25(21):5377–95. doi: 10.1111/mec.13866
33. Calvez E, Guillaumot L, Millet L, Marie J, Bossin H, Rama V, et al. Genetic diversity and phylogeny of Aedes aegypti, the main arbovirus vector in the pacific. PLoS Negl Trop Dis (2016) 10(1):e0004374. doi: 10.1371/journal.pntd.0004374
35. Lee DJ, Hicks MM, Griffiths M, Debenham L, Bryan JH, Russell RC. The culicidae of the Australasian region Vol. Vol 4. Canberra: Australian Government Publishing Service (1987).
36. Belkin JN. The mosquitoes of the south pacific (Diptera, culicidae) Vol. 2. London: Cambridge University Press (1962).
37. Chow CY. Aedes aegypti in the Western pacific region. Bull World Health Organ (1967) 36(4):544–6.
38. Paupy C, Delatte H, Bagny L, Corbel V, Fontenille D. Aedes albopictus, an arbovirus vector: From the darkness to the light. Microbes Infect (2009) 11(14–15):1177–85. doi: 10.1016/j.micinf.2009.05.005
40. Bonne-Wepster J, Brug SL. The subgenus Stegomyia in netherland India. Geneesk Tijdschr Ned-Ind (1932) 2:35–119.
42. Schoenig E. Distribution of three species of Aedes (Stegomyia) carriers of virus diseases on the main island of Papua new Guinea. Philipp Sci (1972) 9:61–82.
43. Elliott SA. Aedes albopictus in the Solomon and Santa Cruz islands, south pacific. Trans R Soc Trop Med Hyg (1980) 74(6):747–8. doi: 10.1016/0035-9203(80)90192-3
44. Guillaumot L, Ofanoa R, Swillen L, Singh N, Bossin HC, Schaffner F. Distribution of Aedes albopictus (Diptera, culicidae) in southwestern Pacific countries, with a first report from the kingdom of Tonga. Parasites Vectors (2012) 5:247. doi: 10.1186/1756-3305-5-247
45. Kraemer MUG, Sinka ME, Duda KA, Mylne A, Shearer FM, Brady OJ, et al. The global compendium of Aedes aegypti and ae. albopictus occurrence. Sci Data (2015) 2:150035. doi: 10.1038/sdata.2015.35
46. Schmidt TL, Chung J, Honnen AC, Weeks AR, Hoffmann AA. Population genomics of two invasive mosquitoes (Aedes aegypti and Aedes albopictus) from the indo-pacific. PLoS Negl Trop Dis (2020) 14(7):30008463. doi: 10.1371/journal.pntd.0008463
47. Perret C, Abarca K, Ovalle J, Ferrer P, Godoy P, Olea A, et al. Dengue-1 virus isolation during first dengue fever outbreak on Easter island, Chile. Emerg Infect Dis (2003) 9(11):1465–7. doi: 10.3201/eid0911.020788
48. Noda S, Yamamoto S, Toma T. Mosquitoes collected on pohnpei island, mokil atoll and pingelap atoll, pohnpei state, the federated states of Micronesia (Diptera: Culicidae). Med Entomol Zool (2013) 64(4):197–201. doi: 10.7601/mez.64.197
49. Asigau S, Hartman DA, Higashiguchi JM, Parker PG. The distribution of mosquitoes across an altitudinal gradient in the Galapagos islands. J Vector Ecol (2017) 42(2):243–53. doi: 10.1111/jvec.12264
50. Ashford DA, Savage HM, Hajjeh RA, McReady J, Bartholomew DM, Spiegel RA, et al. Outbreak of dengue fever in Palau, Western pacific: risk factors for infection. Am J Trop Med Hyg (2003) 69(2):135–40.
51. Calvez E, Pocquet N, Malau A, Kilama S, Taugamoa A, Labrousse D, et al. Assessing entomological risk factors for arboviral disease transmission in the French territory of the Wallis and Futuna islands. PLoS Negl Trop Dis (2020) 14(5):e0008250. doi: 10.1371/journal.pntd.0008250
52. Maynard AJ, Ambrose L, Cooper RD, Chow WK, Davis JB, Muzari MO, et al. Tiger on the prowl: Invasion history and spatio-temporal genetic structure of the Asian tiger mosquito Aedes albopictus (Skuse 1894) in the indo-pacific. PLoS Negl Trop Dis (2017) 11(4):e0005546. doi: 10.1371/journal.pntd.0005546
53. Ammar SE, Mclntyre M, Swan T, Kasper J, Derraik JGB, Baker MG, et al. Intercepted mosquitoes at new zealand’s ports of entry, 2001 to 2018: Current status and future concerns. Trop Medi Infect Dis (2019) 4(3):101. doi: 10.3390/tropicalmed4030101
54. Nunes MRT, Palacios G, Faria NR, Sousa EC Jr., Pantoja JA, Rodrigues SG, et al. Air travel is associated with intracontinental spread of dengue virus serotypes 1-3 in Brazil. PLoS Negl Trop Dis (2014) 8(4):e2769. doi: 10.1371/journal.pntd.0002769
55. LaPointe DA. Current and potential impacts of mosquitoes and the pathogens they vector in the pacific region. Proc Hawaiian Entomol Soc (2007) 39:75–81.
56. Guagliardo SAJ, Lee Y, Pierce AA, Wong J, Chu YY, Morrison AC, et al. The genetic structure of Aedes aegypti populations is driven by boat traffic in the Peruvian Amazon. PLoS Negl Trop Dis (2019) 16(6):e0010552. doi: 10.1371/journal.pntd.0007552
57. Tatem AJ, Rogers DJ, Hay SI. Estimating the malaria risk of African mosquito movement by air travel. Malar J (2006) 5:57. doi: 10.1186/1475-2875-5-57
58. Mier-y-Teran-Romero L, Tatem AJ, Johansson MA. Mosquitoes on a plane: Disinsection will not stop the spread of vector-borne pathogens, a simulation study. PLoS Negl Trop Dis (2017) 11(7):e0005683. doi: 10.1371/journal.ptnd.0005683
59. Metzger ME, Yoshimizu MH, Padgett KA, Hu R, Kramer VL. Detection and establishment of Aedes aegypti and Aedes albopictus (Diptera: Culicidae) mosquitoes in California, 2011-2015. J Med Entomol (2017) 54(3):533–43. doi: 10.1093/jme/tjw237
60. Laird M, Calder L, Thornton RC, Syme R, Holder PW, Mogi M. Japanese Aedes albopictus among four mosquito species reaching new Zealand in used tires. J Am Mosq Control Assoc (1994) 10(1):14–23.
61. Scholte EJ, den Hartog W, Dik M, Schoelitsz B, Brooks M, Schaffner F, et al. Introduction and control of three invasive mosquito species in the Netherlands, July-October 2010. Euro Surveill (2010) 15(45):19710. doi: 10.2807/ese.15.45.19710-en
62. Sukehiro N, Kida N, Umezawa M, Murakami T, Arai N, Jinnai T, et al. First report on invasion of yellow fever mosquito, Aedes aegypti, at narita international airport, Japan in august 2012. Jpn J Infect Dis (2013) 66(3):189–94. doi: 10.7883/yoken.66.189
63. Yang C, Sunahara T, Hu J, Futami K, Kawada H, Minakawa N. Searching for a sign of exotic Aedes albopictus (Culicidae) introduction in major international seaports on Kyushu Island, Japan. PLoS Negl Trop Dis (2021) 15(10):e0009827. doi: 10.1371/journal.pntd.0009827
64. Souza-Neto JA, Powell JR, Bonizzoni M. Aedes aegypti vector competence studies: A review. Infect Genet Evol (2019) 67:191–209. doi: 10.1016/j.meegid.2018.11.009
65. Amraoui F, Ben Ayed W, Madec Y, Faraj C, Himmi O, Btissam A, et al. Potential of Aedes albopictus to cause the emergence of arboviruses in Morocco. PLoS Negl Trop Dis (2019) 13(2):e0006997. doi: 10.1371/journal.pntd.0006997
66. Mariconti M, Obadia T, Mousson L, Malacrida A, Gasperi G, Failloux AB, et al. Estimating the risk of arbovirus transmission in southern Europe using vector competence data. Sci Rep (2019) 9:17852. doi: 10.1038/s41598-019-54395-5
67. Bohers C, Mousson L, Madec Y, Vazeille M, Rhim A, M’ghirbi Y, et al. The recently introduced Aedes albopictus in Tunisia has the potential to transmit chikungunya, dengue and zika viruses. PLoS Negl Trop Dis (2020) 14(10):e0008475. doi: 10.1371/journal.pntd.0008475
68. Gloria-Soria A, Payne AF, Bialosuknia SM, Stout J, Mathias N, Eastwood G, et al. Vector competence of Aedes albopictus populations from the northeastern united states for chikungunya, dengue, and zika viruses. Am J Trop Med Hyg (2021) 104(3):1123–30. doi: 10.4269/ajtmh.20-0874
69. Roundy CM, Azar SR, Rossi S, Huang JH, Leal G, Yun R, et al. Variation in Aedes aegypti mosquito competence for zika virus transmission. Emerg Infect Dis (2017) 23(4):625–32. doi: 10.3201/eid2304/161484
70. Azar SR, Roundy CM, Rossi S, Huang JH, Leal G, Yun R, et al. Differential vector competency of Aedes albopictus populations from the americas for zika virus. Am J Trop Med Hyg (2017) 97(2):330–9. doi: 10.4269/ajtmh.16-0969
71. Vega-Rúa A, Zouache K, Girod R, Failloux AB, Lourenço-de-Oliveira R. High level of vector competence of Aedes aegypti and Aedes albopictus from ten American countries as a crucial factor in the spread of chikungunya virus. J Virol (2014) 88(11):6294–306. doi: 10.1128/JVI.00370-14
72. Stephenson CJ, Coatsworth H, Waits CM, Nazario-Maldonado NM, Mathias DK, Dinglasan R, et al. Geographic partitioning of dengue virus transmission risk in Florida. Viruses (2021) 13(11):2232. doi: 10.3390/v13112232
73. Knox TB, Kay BH, Hall RA, Ryan PA. Enhanced vector competence of Aedes aegypti (Diptera: Culicidae) from the Torres strait compared with mainland Australia for dengue 2 and 4 viruses. J Med Entomol (2003) 40(6):950–6. doi: 10.1603/0022-2585-40.6.950
74. Hugo LE, Stassen L, La J, Gosden E, Ekwudu O, Winterford C, et al. Vector competence of Australian Aedes aegypti and Aedes albopictus for an epidemic strain of zika virus. PLoS Negl Trop Dis (2019) 13(4):e0007281. doi: 10.1371/journal.pntd.0007281
75. Duchemin JB, Mee PT, Lynch SE, Vedururu R, Trinidad L, Paradkar P. Zika vector transmission risk in temperate Australia: A vector competence study. Virol J (2017) 14(1):108. doi: 10.1186/s12985-017-0772-y
76. Hall-Mendelin S, Pyke AT, Moore PR, Ritchie SA, Moore FAJ, van den Hurk AF. Characterization of a Western Pacific zika virus strain in Australian. Aedes aegypti Vector-Borne Zoonotic Dis (2018) 18(6):317–22. doi: 10.1089/vbz.2017.2232
77. Hall-Mendelin S, Pyke AT, Moore PR, Mackay IM, McMahon JL, Ritchie SA, et al. Assessment of local mosquito species incriminates Aedes aegypti as the potential vector of zika virus in Australia. PLoS Negl Trop Dis (2016) 10(9):e0004959. doi: 10.1371/journal.pntd.0004959
78. van den Hurk AF, Hall-Mendelin S, Pyke AT, Frentiu FD, McElroy KM, Day A, et al. Impact of Wolbachia on infection with chikungunya and yellow fever viruses in the mosquito vector Aedes aegypti. PLos Negl Trop Dis (2012) 6(11):e1892. doi: 10.1371/journal.pntd.0001892
79. Calvez E, Guillaumot L, Girault D, Richard V, O’Connor O, Paoaafaite T, et al. Dengue-1 virus and vector competence of Aedes aegypti (Diptera: Culicidae) populations from new Caledonia. Parasites Vectors (2017) 10:381. doi: 10.1186/s13071-017-2319-x
80. Calvez E, Mousson L, Vazeille M, O’Connor O, Cao-Lormeau VM, Mathieu-Daudé F, et al. Zika virus outbreak in the pacific: Vector competence of regional vectors. PLoS Negl Trop Dis (2018) 12(7):e0006637. doi: 10.1371/journal.pntd.0006637
81. Richard V, Paoaafaite T, Cao-Lormeau VM. Vector competence of French polynesian Aedes aegypti and Aedes polynesiensis for zika virus. PLoS Negl Trop Dis (2016) 10(9):e0005024. doi: 10.1371/journal.pntd.0005024
82. O’connor O, Calvez E, Inizan C, Pocquet N, Richard V, Dupont-Rouzeyrol M. Vector competence of Aedes aegypti from new Caledonia for the four recent circulating dengue virus serotypes. PLoS Negl Trop Dis (2020) 14(5):e0008303. doi: 10.1371/journal.pntd.0008303
83. Fernandes RS, O’connor O, Bersot MIL, Girault D, Dokunengo MR, Pocquet N, et al. Vector competence of Aedes aegypti, Aedes albopictus and Culex quinquefasciatus from Brazil and new Caledonia for three zika virus lineages. Pathogens (2020) 9(7):575. doi: 10.3390/pathogens9070575
84. Rückert C, Weger-Lucarelli J, Garcia-Luna SM, Young MC, Byas AD, Murrieta RA, et al. Impact of simultaneous exposure to arboviruses on infection and transmission by Aedes aegypti mosquitoes. Nat Commun (2017) 8:15412. doi: 10.1038/ncomms15412
85. Göertz GP, Vogels CBF, Geertsema C, Koenraadt CJM, Pijlman GP. Mosquito co-infection with zika and chikungunya virus allows simultaneous transmission without affecting vector competence of Aedes aegypti. PLoS Negl Trop Dis (2017) 11(6):e0005654. doi: 10.1371/journal.pntd.0005654
86. Vazeille M, Mousson L, Martin E, Failloux AB. Orally co-infected Aedes albopictus from la reunion island, Indian ocean, can deliver both dengue and chikungunya infectious viral particles in their saliva. PLoS Negl Trop Dis (2010) 4(6):e706. doi: 10.1371/journal.pntd.0000706
87. Vogels CBF, Rückert C, Cavany SM, Perkins TA, Ebel GD, Grubaugh ND. Arbovirus coinfection and co-transmission: A neglected public health concern? PLoS Biol (2019) 17(1):e3000130. doi: 10.1371/journal.pbio.3000130
88. Dupont-Rouzeyrol M, O’Connor O, Calvez E, Daurès M, John M, Grangeon JP, et al. Co-Infection with zika and dengue viruses in 2 patients, new Caledonia, 2014. Emerg Infect Dis (2015) 21(2):381–2. doi: 10.3201/eid2102.141553
89. Moyes CL, Vontas J, Martins AJ, Ng LC, Koou SY, Dusfour I, et al. Contemporary status of insecticide resistance in the major Aedes vectors of arboviruses infecting humans. PLos Negl Trop Dis (2017) 11(7):e0005625. doi: 10.1371/journal.pntd.0005625
90. Balabanidou V, Grigoraki L, Vontas J. Insect cuticle: a critical determinant of insecticide resistance. Curr Opin Insect Sci (2018) 27:68–74. doi: 10.1016/j.cois.2018.03.001
91. Dusfour I, Vontas J, David J, Weetman D, Fonseca DM, Corbel V, et al. Management of insecticide resistance in the major Aedes vectors of arboviruses: advances and challenges. PLoS Negl Trop Dis (2019) 13(10):e0007615. doi: 10.1371/journal.pntd.0007615
92. David JP, Ismail HM, Chandor-Proust A, Paine MJI. Role of cytochrome P450s in insecticide resistance: impact on the control of mosquito-borne diseases and use of insecticides on earth. Philos Trans R Soc B (2013) 368(1612):20120429. doi: 10.1098/rstb.2012.0429
93. van den Berg H, Zaim M, Yadav RS, Soares A, Ameneshewa B, Mnzava A, et al. Global trends in the use of insecticides to control vector-borne diseases. Environ Health Perspect (2012) 120(4):577–82. doi: 10.1289/ehp.1104340
94. McAllister JC, Scott M. CONUS manual for evaluating insecticide resistance in mosquitoes using CDC bottle bioassay kit. Atlanta, GA: The Centers for Disease Control and Prevention (CDC (2020).
95. World Health Organization. Monitoring and managing insecticide resistance in aedes mosquito populations interim guidance for entomologists. Geneva, Switzerland: World Health Organization (2016).
96. Smith LB, Kasai S, Scott JG. Pyrethroid resistance in Aedes aegypti and Aedes albopictus: important mosquito vectors of human diseases. Pestic Biochem Physiol (2016) 133:1–12. doi: 10.1016/j.pestbp.2016.03.005
97. Endersby-Harshman NM, Wuliandari JR, Harshman LG, Frohn V, Johnson BJ, Ritchie SA, et al. Pyrethroid susceptibility has been maintained in the dengue vector, Aedes aegypti (Diptera: Culicidae), in Queensland, Australia. J Med Entomol (2017) 54(6):1649–58. doi: 10.1093/jme/tjx145
98. Dusfour I, Zorrilla P, Guidez A, Issaly J, Girod R, Guillaumot L, et al. Deltamethrin resistance mechanisms in Aedes aegypti populations from three French overseas territories worldwide. PLoS Negl Trop Dis (2015) 9(11):e0004226. doi: 10.1371/journal.pntd.0004226
99. Failloux AB, Ung A, Raymond M, Pasteur N. Insecticide susceptibility in mosquitoes (Diptera: Culicidae) from French Polynesia. J Med Entomol (1994) 31(5):639–44. doi: 10.1093/jmedent/31.5.639
100. Demok S, Endersby-Harshman N, Vinit R, Timinao L, Robinson LJ, Susapu M, et al. Insecticide resistance status of Aedes aegypti and Aedes albopictus mosquitoes in Papua new Guinea. Parasites Vectors (2019) 12:333. doi: 10.1186/s13071-019-3585-6
101. Canyon D, Hii J. Insecticide susceptibility status of Aedes aegypti (Diptera: Culicidae) from townsville. Aust J Entomol (1999) 38(1):40–3. doi: 10.1046/j.1440-6055.1999.00071.x
102. World Health Organization Regional Office for the Western Pacific. Meeting on the global vector control response. Manila, Philippines: World Health Organization Regional Office for the Western Pacific (2019).
103. Bogdal C, Scheringer M, Abad E, Abalos M, van Bavel B, Hagberg J, et al. Worldwide distribution of persistent organic pollutants in air, including results of air monitoring by passive air sampling in five continents. TrAC Trends Anal Chem (2013) 46:150–61. doi: 10.1016/j.trac.2012.05.011
104. Endersby NM, Hoffmann AA, White VL, Ritchie SA, Johnson PH, Weeks AR. Changes in the genetic structure of Aedes aegypti (Diptera: Culicidae) populations in Queensland, Australia, across two seasons: implications for potential mosquito releases. J Med Entomol (2011) 48(5):999–1007. doi: 10.1603/ME10264
105. Beebe NW, Ambrose L, Hill LA, Davis JB, Hapgood G, Cooper RD, et al. Tracing the tiger: population genetics provides valuable insights into the Aedes (Stegomyia) albopictus invasion of the Australasian region. PLoS Negl Trop Dis (2013) 7(8):e2361. doi: 10.1371/journal.pntd.0002361
106. Zhong D, Lo E, Hu R, Metzger ME, Cummings R, Bonizzoni M, et al. Genetic analysis of invasive Aedes albopictus populations in Los Angeles county, California and its potential public health impact. PLoS One (2013) 8(7):e68586. doi: 10.1371/journal.pone.0068586
107. PPHSN. Pacific public health surveillance network (2022). Available at: https://www.pphsn.net (Accessed August 10, 2022).
108. PacMOSSI. Pacific mosquito surveillance strengthening for impact (2022). Available at: https://www.pacmossi.org (Accessed August 10, 2022).
109. Wang GH, Gamez S, Raban RR, Marshall JM, Alphey L, Li M, et al. Combating mosquito-borne disease using genetic control technologies. Nat Commun (2021) 12:4388. doi: 10.1038/s41467-021-24654-z
110. Beebe NW, Pagendam D, Trewin BJ, Boomer A, Bradford M, Ford A, et al. Releasing incompatible males drives strong suppression across populations of wild and Wolbachia-carrying Aedes aegypti in austraila. Proc Natl Acad Sci USA (2021) 118(41):e2106828118. doi: 10.1073/pnas.2106828118
111. Marris E. Bacteria could be key to freeing South Pacific of mosquitoes. Nat (2017) 548(7665):17–8. doi: 10.1038/548017a
112. Institut Louis Malardé. ILM Wolbachia technology a sustainable approach to mosquito suppression and elimination. Institut Louis Malardé (2017) :1–5.
113. Pereira TN, Rocha MN, Sucupira PHF, Carvalho FD, Moreira LA. Wolbachia significantly impacts the vector competence of Aedes aegypti for mayaro virus. Sci Rep (2018) 8:6889. doi: 10.1038/s41598-018-25236-8
114. van den Hurk AF, Hall-Mendelin S, Pyke AT, Smith GA, Mackenzie JS. Vector competence of Australian mosquitoes for chikungunya virus. Vector-borne Zoonotic Dis (2010) 10(5):489–95. doi: 10.1089/vbz.2009.0106
115. Caragata EP, Dutra HLC, Moreira LA. Inhibition of zika virus by Wolbachia in Aedes aegypti. Microb Cell (2016) 3(7):293–95. doi: 10.15698/mic2016.07.513
116. Aliota MT, Walker EC, Yepes AU, Velez ID, Christensen BM, Osorio JE. The wMel strain of Wolbachia reduces transmission of chikungunya virus in Aedes aegypti. PLoS Negl Trop Dis (2016) 10(4):e0004677. doi: 10.1371/journal.pntd.0004677
117. Aliota MT, Peinado SA, Velez ID, Osorio JE. The wMel strain of Wolbachia reduces transmission of zika virus by aedes aegypti. Sci Rep (2016) 6:28792. doi: 10.1038/srep28792
118. Carrington LB, Tran BCN, Le NTH, Luong TTH, Nguyen TT, Nguyen PT, et al. Field- and clinically derived estimates of Wolbachia-mediated blocking of dengue virus transmission potential in Aedes aegypti mosquitoes. Proc Natl Acad Sci USA (2017) 115(2):361–6. doi: 10.1073/pnas.1715788115
119. Hoffmann AA, Montgomery BL, Popovici J, Iturbe-Ormaetxe I, Johnson PH, Muzzi F, et al. Successful establishment of Wolbachia in Aedes populations to suppress dengue transmission. Nat (2011) 476:454–7. doi: 10.1038/nature10356
120. Hoffmann AA, Iturbe-Ormaetxe I, Callahan AG, Phillips BL, Billington K, Axford JK. Stability of the wMel Wolbachia infection following invasion into Aedes aegypti populations. PLoS Negl Trop Dis (2014) 8(9):e3115. doi: 10.1371/journal.pntd.0003115
121. Schmidt TL, Barton NH, Rašić G, Turley AP, Montgomery BL, Iturbe-Ormaetxe I, et al. Local introduction and heterogeneous spatial spread of dengue-suppressing Wolbachia through an urban population of Aedes aegypti. PLoS Biol (2017) 15(5):e2001895. doi: 10.1371/journal.pbio.2001894
122. O’Neill S, Ryan P, Turley AP, Wilson G, Retzki K, Iturbe-Ormaetxe I, et al. Scaled deployment of Wolbachia to protect the community from dengue and other Aedes transmitted arboviruses. Gates Open Res (2018) 2:36. doi: 10.12688/gatesopenres.12844.3
123. Ryan PA, Turley AP, Wilson G, Hurst TP, Retzki K, Brown-Kenyon J, et al. Establishment of wMel Wolbachia in Aedes aegypti mosquitoes and reduction of local dengue transmission in cairns and surrounding locations in northern Queensland, Australia. Gates Open Res (2019) 3:1547. doi: 10.12688/gatesopenres.13061.2
124. World Mosquito Program. (2022). Available at: https://www.worldmosquitoprogram.org (Accessed April 17, 2022).
125. Hawaii Department of Agriculture, Hawaii Department of Health, Hawaii Department of Land and Natural Resources. Report on the importation and use of Aedes aegypti with Wolbachia bacteria for landscape scale control of mosquitos in a vector control program ACT 106, SLH 2019. (2019).
126. Nguyen TH, Nguyen HL, Nguyen TY, Vu SN, Tran ND, Le TN, et al. Field evaluation of the establishment potential of wmelpop Wolbachia in Australia and Vietnam of dengue control. Parasites Vectors (2015) 8:563. doi: 10.1186/s13071-015-1174-x
127. Li M, Yang T, Bui M, Gamez S, Wise T, Kandul NP, et al. Suppressing mosquito populations with precision guided sterile males. Nat Commun (2021) 12:5374. doi: 10.1038/s41467-021-25421-w
Keywords: Aedes, Pacific region, arbovirus, mosquito-borne diseases, mosquitoes, insecticide resistance, vector competence, novel biocontrol
Citation: Seok S, Raz CD, Miller JH, Malcolm AN, Eason MD, Romero-Weaver AL, Giordano BV, Jacobsen CM, Wang X, Akbari OS, Raban R, Mathias DK, Caragata EP, Vorsino AE, Chiu JC and Lee Y (2023) Arboviral disease outbreaks, Aedes mosquitoes, and vector control efforts in the Pacific. Front. Trop. Dis 4:1035273. doi: 10.3389/fitd.2023.1035273
Received: 02 September 2022; Accepted: 03 January 2023;
Published: 25 January 2023.
Edited by:
Diawo Diallo, Institut Pasteur de Dakar, SenegalReviewed by:
Rafael Piovezan, University of São Paulo, BrazilJose G. Juarez, Universidad del Valle de Guatemala, Guatemala
Copyright © 2023 Seok, Raz, Miller, Malcolm, Eason, Romero-Weaver, Giordano, Jacobsen, Wang, Akbari, Raban, Mathias, Caragata, Vorsino, Chiu and Lee. This is an open-access article distributed under the terms of the Creative Commons Attribution License (CC BY). The use, distribution or reproduction in other forums is permitted, provided the original author(s) and the copyright owner(s) are credited and that the original publication in this journal is cited, in accordance with accepted academic practice. No use, distribution or reproduction is permitted which does not comply with these terms.
*Correspondence: Yoosook Lee, yoosook.lee@ufl.edu