- Birck Nanotechnology Center, School of Mechanical Engineering, Purdue University, West Lafayette, IN, USA
Thermal and Mass Transport
The development of thermal and mass transport as a subject of scientific study holds a central place in the origin and advancement of the Industrial Revolution by enabling the steam engine, which in turn provided unprecedented levels of power and speed for transportation, material processing, and eventually the distribution of electricity. Even earlier, elementary understanding of heat and mass transfer were crucial elements in providing for human safety and comfort, as well as food preservation via cooking and drying. Given this rich and diverse history, a logical question is: how much more of this subject remains to be discovered and explored? One answer would point to nuances of how heat and mass flow occurs at different scales through various material solid and fluid hosts, new forms of which are constantly being discovered and developed (Bergman et al., 2008). However, such a perspective seems relatively narrow in comparison to the outsize history of impact in the field, yet at the same time global grand challenge problems, such as clean energy and water, food security, or eradication of disease are too general to have useful meaning for the present purpose. Instead, we consider here how improved understanding of the details of thermal and mass transport might rekindle a new set of discoveries and technologies with broad global impact.
Transient and Non-Equilibrium Processes
A useful starting point for imagining the possibilities of transformative discoveries in the area of transport processes is the semiconductor industry. Borne of a seemingly simple yet profound combination of materials built into a unique device, the solid-state transistor has served as the genesis of multiple industries, ranging from personal computing to telecommunications (Brinkman et al., 1997). One of the crucial insights to the development of integrated circuits (ICs) was that individual devices could be operated away from thermodynamic equilibrium in a repeated and coordinated manner to produce a greater overall effect than the “sum of their parts.” With such developments arose other specialized circuit elements such as micro-capacitors and dynamic memory devices that, along with a set of community-driven and shared design tools (Dutton and Yu, 2012), have enabled the simple transistor to form the basis of ICs that now touch nearly every technology-based device and product.
In comparison, the study of transport phenomena has focused strongly on near-equilibrium and steady- or quasi-steady-state processes. This emphasis is apparent in virtually any textbook on heat, mass, and/or fluid transport, largely because of the myriad complexities produced by non-equilibrium thermodynamics and transient dynamics (Groot and Mazur, 1962). One important example of non-equilibrium behavior involves the fast collection of “hot carriers” as proposed in the 1980s (Ross and Nozik, 1982) for solar photovoltaics. However, actually harnessing such effects, for example, with energy conversion devices that exploit the highly energetic but ephemeral non-equilibrium states, has presented an ongoing challenge that remains largely unsolved. Moreover, any prospective thermal system that exploits transient behavior and non-equilibrium effects will almost surely require much-improved thermal “capacitors,” or storage materials and related devices (Doty et al., in press). Following the “Ragone plot” format for electrochemical storage systems that compares energy and power densities (Simon et al., 2014), Figure 1 provides a notional map of various thermal storage materials and approaches. The quantitative metrics for thermal energy (1 MJ/kg) and power (1 kW/kg) are surely debatable, but they provide reasonable order-of-magnitude goals. Clearly, power (i.e., speed) is a serious shortcoming of thermal storage. Few existing approaches if any hold the promise of both high energy and power simultaneously, and even “volumetric boiling” with relatively high levels of both has received relatively little attention for thermal storage, likely because of its need for active control and its highly non-equilibrium nature (Kim and Lior, 1997). Nevertheless, many emerging phase-change materials (and some old ones) offer promise of managing both high power and energy (Shamberger, 2016).
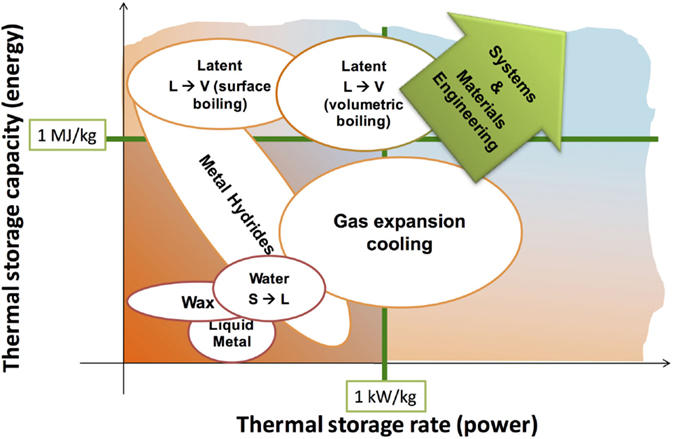
Figure 1. Notional map of energy and power densities of thermal storage approaches (L, liquid; S, solid; V, vapor).
Small-Scale Materials and Devices
The unique transport characteristics caused by small-scale dimensions of materials have captured much attention from the research community over the past several decades (Cahill et al., 2003). Most such studies have focused on the properties of individual nanoscale elements or features (e.g., interfaces), often with intricate experimental constructs (Shi et al., 2003). Relatively less attention has been paid to the collective behavior of such objects, yet from an engineering perspective, ensemble characteristics would certainly seem to be paramount. Another important advantage to study collective behavior is the natural statistical averaging that is needed to analyze the exceptionally high variability in properties and characteristics of individual nanoscale objects (Feser et al., 2012) caused by fabrication processes with high intrinsic variability. A useful example of the value of community-based efforts in this regard is the global round-robin testing of a variety of the so-called nanofluids (i.e., suspensions of nanoparticles in liquids), which found that (Buongiorno et al., 2009) “no anomalous enhancement of thermal conductivity was achieved in the nanofluids tested in this exercise.” Nevertheless, the development of new nanomaterial ensembles that offer unique advantages at the human scale remains an important challenge and objective for the field. In this sense, a greater emphasis on self-organized fabrication and manufacturing of such assemblies (Srivastava et al., 2013), models of complex media through rigorous volume-averaging techniques (Sbutega et al., 2015) and thorough uncertainty quantification (Murthy and Mathur, 2012) will be crucial.
Adaptable Thermofluidic Transport
Intimately connected to solid-state nanoscale and composite materials are fluid-based thermal and mass transport systems, including both man-made and natural architectures. Interestingly, few examples of high-transport solid thermal materials exist in nature, in contrast to the opposite extreme of highly insulating structures, such as animal fur (Simonis et al., 2014). This state of affairs is likely the result of the peculiarly good thermal transport properties of water – by far the most abundant natural liquid. Nature has developed various means of managing thermal and mass balances in plants and animals through exquisitely complex aqueous networks that adapt to their environments autonomously. In comparison, man-made thermal systems, such as power plants, also use water extensively, but they tend to employ brute-force thermal management approaches that exploit large bodies of water, causing significant thermal pollution and other adverse side-effects (Roy et al., 2005). While biologically inspired but coarse facsimiles of multi-phase, vascular networks have been studied recently (Chen et al., 2013), the opportunity to integrate adaptable materials that autonomously respond to dynamic fluidic and thermodynamic conditions remains largely unaddressed, and the likely gains in engineering efficiency, ecological health, and water conservation make such approaches particularly appealing. At the same time, the question of adaptation in thermo-fluid systems has reached the full global scale with the contemporary emergence of climate change. More than ever, policymakers require the objective expertise of scientists and engineers to understand the many complexities of solar forcing, greenhouse gas emissions and accumulation, and the roles of evaporation and ocean currents, among many other highly technical issues that are not well understood (Incropera, 2015).
Mass Transport in Medicine
The full titles of undergraduate courses and books in this field commonly use some variant of “Heat and Mass Transfer,” which is often colloquially abbreviated to “Heat Transfer.” The truncation is not without reason or consequence, as the topics involving transport of mass are typically delivered secondarily through analogies to thermal transport. Yet mass transfer technology when viewed from a societal perspective would seem to deserve equal if not greater billing, as it is central to global grand challenges associated with clean water and providing quality health care to an aging population. The latter topic is particularly compelling as progress in the so-called “personalized medicine” continues to accelerate (Chan and Ginsburg, 2011). However, broad adoption of personalized medical treatments – i.e., beyond clinical trials – will require major new advances in many areas, none more important than controlled drug delivery. This area has attracted much attention in mass transport research, but with generally underwhelming results. For example, concerning nanoparticle-based drug delivery to tumors, a recent review (Il Keun et al., 2012) states, “Almost the whole decade of [the] 2000s has been consumed by developing various nanoparticles for targeted drug delivery to tumors, and the results are not, on the whole, encouraging.” The reasons for this disappointment are numerous and complex, but many relate to “oversimplification of biotransport phenomena.” Clearly, this area of controlled and targeted drug release therapies represents a great challenge to our community, with immense potential for impact on the world.
Outlook for This Specialty Section
Given the foregoing challenges, the study of thermal and mass transport is as rich and interesting as ever, while also holding a unique legacy of translating fundamental scientific discoveries into technologies that change the world (Viskanta, 2014). This specialty section of Frontiers in Mechanical Engineering seeks to draw from the broader interdisciplinary ethos that already exists within the transport research community, as evident from the foregoing discussion, and that also has come to characterize the “Frontiers In” project and family of journals. The section will broaden the reach of the field through a successful and growing open-access model of scholarly publication, along with a refreshing and technically rigorous style of collaborative peer review that has been pioneered by the “Frontiers In” family. We hope that you will consider engaging in this venue for world-changing scholarship in this exciting field.
Author Contributions
The author confirms being the sole contributor of this work and approved it for publication.
Conflict of Interest Statement
The author declares that the research was conducted in the absence of any commercial or financial relationships that could be construed as a potential conflict of interest.
References
Bergman, T. L., Faghri, A., and Viskanta, R. (2008). Frontiers in transport phenomena research and education: energy systems, biological systems, security, information technology and nanotechnology. Int. J. Heat Mass Transf. 51, 4599–4613. doi: 10.1016/j.ijheatmasstransfer.2008.01.024
Brinkman, W. F., Haggan, D. E., and Troutman, W. W. (1997). A history of the invention of the transistor and where it will lead us. IEEE J. Solid-State Circuits 32, 1858–1865. doi:10.1109/4.643644
Buongiorno, J., Venerus, D. C., Prabhat, N., Mckrell, T., Townsend, J., Christianson, R., et al. (2009). A benchmark study on the thermal conductivity of nanofluids. J. Appl. Phys. 106, 14. doi:10.1063/1.3245330
Cahill, D. G., Ford, W. K., Goodson, K. E., Mahan, G. D., Majumdar, A., Maris, H. J., et al. (2003). Nanoscale thermal transport. J. Appl. Phys. 93, 793. doi:10.1063/1.1524305
Chan, I. S., and Ginsburg, G. S. (2011). Personalized medicine: progress and promise. Annu. Rev. Genomics Hum. Genet. 12, 217–244. doi:10.1146/annurev-genom-082410-101446
Chen, I. T., Pharkya, A., and Stroock, A. D. (2013). Analysis of superheated loop heat pipes exploiting nanoporous wick membranes. AIChE J. 60, 762–777. doi:10.1002/aic.14303
Doty, J., Yerkes, K., Byrd, L., Murthy, J., Alleyne, A., Wolff, M., et al. (in press). Dynamic thermal management for aerospace technology: review and outlook. J. Thermophys. Heat Transf. 1–13. doi:10.2514/1.T4701
Dutton, R. W., and Yu, Z. (2012). Technology CAD – Computer Simulation of IC Processes and Devices. New York: Springer Science & Business Media.
Feser, J. P., Sadhu, J. S., Azeredo, B. P., Hsu, K. H., Ma, J., Kim, J., et al. (2012). Thermal conductivity of silicon nanowire arrays with controlled roughness. J. Appl. Phys. 112, 114306–114308. doi:10.1063/1.4767456
Groot, S. R. D., and Mazur, P. (1962). Non-Equilibrium Thermodynamics. Amsterdam, New York: North-Holland Publishing Company; Interscience Publishers.
Il Keun, K., Lee, S. C., Han, B., and Park, K. (2012). Analysis on the current status of targeted drug delivery to tumors. J. Control. Release 164, 108–114. doi:10.1016/j.jconrel.2012.07.010
Kim, J. I., and Lior, N. (1997). Some critical transitions in pool flash evaporation. Int. J. Heat Mass Transf. 40, 2363–2372. doi:10.1016/S0017-9310(96)00296-7
Murthy, J. Y., and Mathur, S. R. (2012). Computational heat transfer in complex systems: a review of needs and opportunities. J. Heat Transfer 134, 031016. doi:10.1115/1.4005153
Ross, R. T., and Nozik, A. J. (1982). Efficiency of hot-carrier solar energy converters. J. Appl. Phys. 53, 3813–3817. doi:10.1063/1.331124
Roy, S. B., Ricci, P. F., Summers, K. V., Chung, C.-F., and Goldstein, R. A. (2005). Evaluation of the sustainability of water withdrawals in the United States, 1995 to 2025. J. Am. Water Resour. Assoc. 41, 1091–1108. doi:10.1111/j.1752-1688.2005.tb03787.x
Sbutega, K., Geb, D., and Catton, I. (2015). “Modeling of multiscale heat transfer systems using volume averaging theory,” in Advances in Heat Transfer, Vol. 47, eds E. M. Sparrow, J. P. Abraham, and J. M. Gorman (Waltham, MA: Elsevier), 41–165.
Shamberger, P. J. (2016). Cooling capacity figure of merit for phase change materials. J. Heat Transfer 138, 024502–024508. doi:10.1115/1.4031252
Shi, L., Li, D., Yu, C., Jang, W., Kim, D., Yao, Z., et al. (2003). Measuring thermal and thermoelectric properties of one-dimensional nanostructures using a microfabricated device. J. Heat Transfer 125, 881–888. doi:10.1115/1.1597619
Simon, P., Gogotsi, Y., and Dunn, B. (2014). Where do batteries end and supercapacitors begin? Science 343, 1210–1211. doi:10.1126/science.1249625
Simonis, P., Rattal, M., Oualim, E. M., Mouhse, A., and Vigneron, J.-P. (2014). Radiative contribution to thermal conductance in animal furs and other woolly insulators. Opt. Express 22, 1940–1951. doi:10.1364/OE.22.001940
Srivastava, I., Sadasivam, S., Smith, K. C., and Fisher, T. S. (2013). Combined microstructure and heat conduction modeling of heterogeneous interfaces and materials. J. Heat Transfer 135, 061603. doi:10.1115/1.4023583
Keywords: heat transfer, mass transfer, non-equilibrium thermodynamics, nanostructures, fluid dynamics
Citation: Fisher TS (2016) Contemporary Challenges for Thermal and Mass Transport Technologies: A Perspective on Twenty-First Century Opportunities for the Field. Front. Mech. Eng. 2:3. doi: 10.3389/fmech.2016.00003
Received: 18 February 2016; Accepted: 30 March 2016;
Published: 07 April 2016
Edited and Reviewed by: Nesrin Ozalp, KU Leuven, Belgium
Copyright: © 2016 Fisher. This is an open-access article distributed under the terms of the Creative Commons Attribution License (CC BY). The use, distribution or reproduction in other forums is permitted, provided the original author(s) or licensor are credited and that the original publication in this journal is cited, in accordance with accepted academic practice. No use, distribution or reproduction is permitted which does not comply with these terms.
*Correspondence: Timothy S. Fisher, dHNmaXNoZXJAcHVyZHVlLmVkdQ==