- 1Department of Mechanical Engineering, College of Engineering, Prince Mohammad Bin Fahd University, Al Khobar, Saudi Arabia
- 2Faculty of Applied Sciences, Ton Duc Thang University, Ho Chi Minh City, Vietnam
The pros and the cons of lean-burn, compression ignition (CI), direct injection (DI) internal combustion engines (ICE) are reviewed for transport applications. Fueling options considered include diesel only and dual-fuel applications with diesel and a gaseous fuel (CNG, LNG, and LPG). CIDI ICEs have higher fuel conversion efficiencies than stoichiometric, spark ignition (SI) ICEs, whether DI or port fuel injected (PFI). However, diesel-fueled CIDI ICEs have higher particulate matter (PM) and NOx engine-out emissions. The tail-pipe NOx emissions in real-world driving of diesel-powered vehicles have been, in the past, above the limits requested over the simplified cold start driving cycles used for certification. This issue has recently been resolved. The newest diesel-powered vehicles are now compliant with new laboratory test cycles and real-world-driving schedules and have no disadvantages in terms of criteria air pollutants compared to older diesel vehicles, while delivering improvements in fuel economy and CO2 emissions. Dual-fuel CIDI ICEs offer the opportunity for enhanced environmental friendliness. Dual-fuel CIDI ICEs have lower engine-out NOx and PM emissions compared to diesel-only CIDI ICEs. The latest diesel-only vehicles and vehicles with dual-fuel ICEs deliver dramatic reductions in tail-pipe PM emissions compared to older diesel-only vehicles. Moreover, they deliver tail-pipe PM emissions well below the ambient conditions in most city areas that are highly polluted, thereby helping to clean the air. The diesel-fueled CIDI ICEs may be further improved to deliver better fuel economy and further reduced tail-pipe emissions. The dual-fuel CIDI ICE has more room for improvement to produce similar or better steady state and transient performance in terms of torque, power output and fuel conversion efficiency compared to diesel-fueled CIDI ICEs, while drastically reducing CO2 and PM tail-pipe emissions, and improving NOx tail-pipe emissions. This is due to the ability to modulate the premixed and diffusion phases of combustion with a second fuel that is much easier to vaporize and is less prone to auto-ignition. Further development of the fuel injection system for the second fuel will lead to novel dual-fuel CIDI ICE designs with better performance.
Introduction
The lean-burn, compression ignition (CI), direct injection (DI), is the most efficient internal combustion engine (ICE) (Zhao, 2009; Mollenhauer and Tschöke, 2010). It produces engine-out emissions of nitrous oxides and particulate matter (PM) that need after treatment to match the extremely low limits set up for transport applications (Lloyd and Cackette, 2001; Burtscher, 2005; Maricq, 2007), despite air quality is not only influenced by the transport emissions but by many other sources. Combustion strategies alone (Khair and Majewski, 2006) were not enough to meet the emission threshold, and specific lean burn catalysts were needed especially for NOx additional to particulate filters in the exhaust. Despite their economic success, diesel engines were facing ever more stringent emission legislation worldwide (Knecht, 2008; Zhao, 2009) at the price of phasing out the technology targeting unrealistic minimal incremental improvements.
The diesel engine has pros and cons as everything. It has full and part load fuel conversion efficiencies exceeding those of stoichiometric, spark ignition (SI) ICEs, both DI and port fuel injected (PFI). CIDI ICEs have peak efficiencies about 50% and efficiencies above 40% over most of the speeds and loads. Opposite, SI ICEs have peak efficiencies in the mid 30%, and these efficiencies are dramatically reduced by reducing the load. CI ICEs deliver mechanical energy on demand at fuel conversion efficiencies that are also higher than the efficiencies of combustion fuel power plants producing electricity. According to EIA (2018), in 2017, across the United States, coal steam generators run with an average efficiency of 33.98%. Petroleum and natural gas steam generators run at about the same efficiency, 33.45 and 32.96%. Gas turbine generators run at a reduced efficiency of 25.29% with petroleum, and 30.53% with natural gas. The efficiency of internal combustion engine generators is larger than gas turbine and steam generators, at 33.12% with petroleum and 37.41% with natural gas. Only combined-cycle generators, not with petroleum, that have an efficiency of 34.78%, but with natural gas, that have an efficiency of 44.61%, surpass the internal combustion generators.
In the comparison of the electric mobility, CIDI ICEs still have advantages for transport applications is unchallenged (Boretti, 2018). However, the CIDI ICE is suffering from a bad reputation jeopardizing its potentials. Diesel CIDI ICEs have failed in the recent past to deliver the specific NOx emissions of cold start certification cycles during warmed-up real-world driving schedules that were strongly differing from the certification cycles (Boretti, 2017; Boretti and Lappas, 2019). This unfortunate occurrence has been played against the CIDI ICE to give the impression this engine is environmentally unfriendly for pollutant emissions where it is not.
The large NOx emissions of CIDI ICEs are the result of large in-cylinder production of NOx working in excess air lean of stoichiometry, coupled to the improper operation of the after treatment. The lean-burn catalyst of CIDI ICEs is less evolved than the three-way catalytic (TWC) converter of stoichiometric, SI ICEs (Heywood, 1988; Zhao, 2009; Mollenhauer and Tschöke, 2010; Reşitoglu et al., 2015). Additionally, the prolonged operation warmed-up was not considered (Boretti and Lappas, 2019). Also, some manufacturers adopting urea injection in the after-treatment decided to inject less urea that the necessary when not strictly requested by the emission certification. Similarly, some manufacturers also focused on drivability and fuel economy issues rather than emissions when not strictly asked, far from the operating conditions of concern for the emission certification. Thus, the failure to meet the NOx emissions over randomly selected conditions was not a fundamental flaw of the CIDI ICEs in general, but only of the specific products developed for what were the emission regulations and the market requirements of the specific time. Not considered by the detractors of the CIDI ICEs, as these engines are equipped with particle traps of almost perfect efficiency, circulation of vehicles fitted with these engines, in high polluted areas, is resulting in better tailpipe than intake conditions, for particulate, thus contributing to cleaning the air.
The present contribution supplies a fair review of the pros and cons of the lean burn, CIDI ICEs, that are much better than what is thought. As the ICE is certainly needed for more decades to come, further improvements of the lean burn, CIDI ICE will be beneficial to the economy and the environment. In addition to diesel CIDI ICEs, this work also considers dual-fuel engines diesel-LNG (Goudie et al., 2004; Osorio-Tejada et al., 2015; Laughlin and Burnham, 2016), diesel-CNG (Maji et al., 2008; Shah et al., 2011; Ryu, 2013) or diesel-LPG (Jian et al., 2001; Ashok et al., 2015). The operation with a small amount of diesel and a much larger (in energy term) amount of a much lighter hydrocarbon fuel, with a reduced carbon to hydrogen content, permits to further reduce the PM engine-out emissions, as well as the CO2 emissions, and being liberated from the PM-NOx trade-off that afflict the diesel-only injection strategies, also reduce the engine-out emissions of NOx. Development tendencies for dual fuel CIDI ICEs are also reviewed.
The utilization of biodiesel to produce low carbon diesel fuels using a single-fuel approach is certainly another option to reduce CO2 emissions. While this opportunity does not impact on the pollutant emission, production of biofuels, in general, is increasing, but not at the expected rate (IEA, 2019), and the food vs. fuel issue (Ayre, 2007; Kingsbury, 2007; Inderwildi and King, 2009) may also weight negatively in a world with a projected imminent water and food crisis (United Nations, 2019). Additionally, the advantages of biofuels over LCA are a long-standing, controversial debate in the literature (McKone et al., 2011).
There is the opportunity of methane emissions from dual-fuel natural gas–diesel engines (Camuzeaux et al., 2015). As methane is a potent GHG, this aspect should be taken into due consideration when targeting GHG emissions reduction. There is not only the potential for methane leaks from the vehicles incorporating dual-fuel diesel-LNG engines. There are also methane emissions from oil and gas production. Additional to the methane emissions from natural gas production, there are the electricity emissions associated with the operation of the LNG facility. While LNG (and CNG) will certainly still give advantages vs. diesel, this advantage may be less than what could be inferred from the C-H ratio of the fuel. There is certainly an issue to reduce the methane emissions associated with the production, transportation, and liquefaction of natural gas (Ravikumar, 2018).
Finally, while natural gas fumigation for dual-fuel diesel engines has been used widely, as it is much simpler and it can be accomplished through low tech conversions, and thus most vehicles utilize this approach, diesel engines converted to diesel and fumigated natural gas suffer from significant fuel conversion efficiency degradation vs. the original diesel, both full load and part load, and reduced power and torque density. If the natural gas is mixed (fumigated) with the intake air before induction to the cylinder, and diesel fuel is used as an ignition source, the amount of natural gas introduced is limited by the opportunity of knock of the premixed mixture. Furthermore, the load is typically controlled by throttling the intake as in conventional gasoline engines rather than by the quantity of fuel injected as in the diesel engine. As the goal is to provide equal or better performances (power, torque, transient operation) and emissions of the latest diesel with a dual fuel design, this dual-fuel design must adopt the direct injection of the diesel and the gaseous fuel.
The Genesis of the Bad Reputation of the Diesel
The bad reputation of the diesel, and more in general of the internal combustion engine (ICE), is the result of actions by the California Air Resources Board (CARB), as well as of the US Environmental Protection Agency (EPA) (Parker, 2019), with “Diesel-gate” only one step of a ploy.
Back in the days, the hydrogen economy was the more likely future model for transportation, better than every other alternative considering the intermittency of wind and solar energy production (Crabtree et al., 2004; Muradov and Veziroglu, 2005; Marbán and Valdés-Solís, 2007). Vehicles were supposed to use ICEs powered by renewable hydrogen (H2-ICEs), with everything but dramatic changes needed in the engine technology, but efforts mostly devoted to storage and distribution. About the same days, it was also popular the idea of a methanol economy, where the methanol produced by using the renewable hydrogen and the CO2 captured in coal-fired power plants was a direct replacement of traditional gasoline fuel (Olah, 2004, 2005). The H2-ICE become history after the CARB deliberated the BMW Hydrogen 7, the first vehicle powered by an ICE was delivered to the market, did not qualify as a zero (CO2) emission vehicle. In 2005 BMW proposed the Hydrogen 7 car as a zero-emissions vehicle. Burning hydrogen, at the tailpipe there was mostly water vapor and absolutely no CO2 emission, but the US EPA did not agree with the zero-emission of CO2 (Nica, 2016). The US EPA said that the vehicle still had an ICE, with the opportunity that oil used for lubrication could have ended up within the cylinder, thus producing CO2. The fact that the total oil consumption was a negligible 0.04 L of oil every 1,000 km was not considered. Because of the unofficial deliberation, BMW dropped the hydrogen ICE research. All the other Original Equipment Manufacturers stopped their R&D afterward.
Regarding the negative attitude of CARB and US EPA toward the ICE in general, in 2011 BMW proposed as a concept car the battery-electric i3 with the opportunity of a range extender (Ramsbrock et al., 2013; Scott and Burton, 2013). The range extender was a small gasoline ICE powering a generator to recharge the battery. By introducing the range extender, it was possible to increase the range of the car and reduce the cost, weight, and volume of the battery pack, which is a major issue for the economy and the environment. With production only planned to start in 2013, the CARB at once rushed to set up rules to prevent the optimization of this concept, issuing in 2012 (CARB, 2012) an overlong regulation prescribing a range extender must only be used to reach the nearest recharging point. In between the other requirements, the CARB requested, from a range extender vehicle, a rated all-electric range of at least 75 miles, a range less than, or equal to, battery range from the auxiliary power unit, and finally, that the auxiliary power unit must not be capable of switching on until the battery charge was depleted. As a result, of all these limitations, BMW struggled to make the range extender competitive, and ultimately, they recently dropped the production of the i3 with range extender (Autocar, 2018).
These two events help to explain the 2015 “diesel-gate” and the subsequent “diesel-phobia.” The diesel engine was popular (for passenger cars) mostly in Europe, and the EU was promoting diesel vehicles to tackle the issues of climate change. At the time, it was clear that a premature move toward electric mobility could have translated into an economic and environmental disaster. Thus, the Volkswagen group was targeted by the “diesel gate” scandal. Diesel ICEs were providing low CO2 emissions, competitive with battery electric vehicles in life cycle analyses while emitting less than the prescribed pollutants over the tests prescribed at the time. Passenger cars were tested for compliance with emission rules over a prescribed cycle, in a laboratory, under repeatable conditions with the correct equipment. The International Council on Clean Transportation (ICCT), organized some casual driving on the road of different diesel vehicles, and measurements of pollutants with PEMs. They found that vehicles optimized to produce low specific (per km) CO2 and pollutant emissions in certain conditions, were not able to ensure the same specific emissions under every other condition, as it was logic to expect. The EPA issued a notice of violation against Volkswagen then resulting in a huge fine in the following court actions. “Diesel-gate” has cost so far to VW more than 29 billion $, in fines, compensation, and buybacks, mainly in the United States (phys.org, 2018). Part of the Volkswagen billion $ ended up supporting the battery electric vehicle mobility, funding the electric vehicle recharging infrastructure in the United States by selected suppliers (O'Boyle, 2018). “Diesel-gate” has then been used to determine the end of the ICE-based mobility (Raftery, 2018; Taylor, 2018).
The alleged excess emission of NOx by vehicles equipped with CIDI diesel ICEs that started with “diesel gate,” is still popular, despite untrue (Chossière et al., 2018) claims that diesel vehicles caused 2,700 premature mortalities in 2015 only across Europe, because of their “excess” NOx emissions. This work is not objective in analyzing the emissions of the diesel engine. It is incorrect to claim that diesel vehicles in the EU emit much more NOx on the road than the regulatory limits. As previously written, the emission rules regulated the pollutant emissions in the specific laboratory test conditions, and not in every other possible condition. It is unreasonable to expect a specific fuel economy and emissions of regulated pollutants and carbon dioxide that are independent of the specific test. To have an “excess” emission, first there is a need for a limit for the specific application, and then the measure of the “excess” under the specific condition. The claim of premature mortalities caused by excess NOx emissions from diesel vehicles is based on an overrated differential emission of NOx, assuming much worse than actual emissions, and comparing this emission to an improbable near-zero-emission reference situation. The claim is also based on an overrated attribution of the number of mortalities to this differential emission. These two assumptions are not supported by proven data.
As the more recent diesel vehicles have replaced even more polluting vehicles, the only possible objective statement that can be made about the emissions of old and new diesel vehicles in Europe, based on unquestionable evidence, is only based on the emission complaint rules of the time of their registrations. As the emission rules have been made increasingly restrictive, albeit, as only verified over the laboratory certification test, as shown in Table 1, it is incorrect to assume CIDI diesel ICEs emit more NOx than before. While Euro 6 compliant diesel passenger vehicles were required to emit less than 0.08 g/km of NOx when covering the NEDC in the laboratory test, Euro 5 to 3 compliant diesel vehicles were otherwise permitted to emit 0.18, 0.25 and 0.50 g/km on the same test, and Euro 1 and 2 compliant diesel vehicles had to verify only an emission threshold of 0.7-0.9 and 0.97 g/km on the same test. There are no measurements that prove old diesel vehicles, that were compliant with prior Euro rules, were more environmentally friendly, under every pollutant criterion, including NOx, during real-world driving than the latest diesel vehicles. Furthermore, the emission performances usually deteriorate with age, and lack of maintenance can make things even worse. This makes the claim of Chossière et al. (2018) inconsistent.
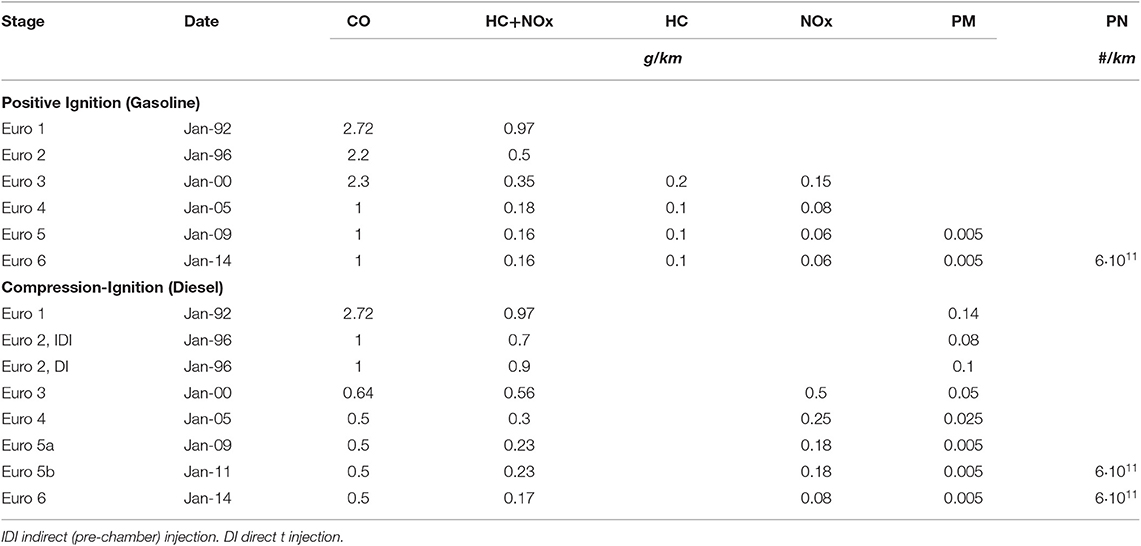
Table 1. European Union emission standards for passenger vehicles (category M) of positive (gasoline) and compression (diesel) design.
Advantages and Disadvantages of the Lean-Burn CIDI Engine
The major advantage of the lean burn, CIDI ICE is the fuel conversion efficiency, which is much higher than in stoichiometric, SI ICEs, both full loads, and more than that, part load (Heywood, 1988; Zhao, 2009; Mollenhauer and Tschöke, 2010). While passenger vehicles with a lean burn, CIDI ICE fueled with diesel have fuel peak fuel conversion efficiencies of about 45%, the peak efficiency of passenger vehicles with stoichiometric, SI ICEs fueled with gasoline is only about 35%. Reducing the load by the quantity of fuel injected, the fuel conversion efficiency of the lean burn, CIDI ICE is high over most of the load range. Conversely, by reducing the load throttling the intake, the fuel conversion efficiency of the stoichiometric, SI ICE dramatically deteriorates when reducing the load. This gives the opportunity to passenger vehicles equipped with a lean burn, CIDI ICE to consume much less fuel, and therefore emit much less CO2, during driving cycles (Schipper et al., 2002; Zervas et al., 2006; Johnson, 2009; Zhao, 2009; Mollenhauer and Tschöke, 2010; Boretti, 2017, 2018; Boretti and Lappas, 2019).
The lean-burn after treatment in general (CIDI diesel ICEs innately run lean, apart from cases of extreme uses of exhaust gas recirculation, EGR) is however much less efficient than the stoichiometric after treatment by TWC converters of SI gasoline ICEs (Lloyd and Cackette, 2001; Burtscher, 2005; Maricq, 2007). Hence, emissions of regulated pollutants, in particular, NOx, over duty cycles that largely deviate from the certification cycles, being much longer and needing the engine to run to a significant extent fully warmed up, are much larger in lean-burn ICEs rather than stoichiometric ICEs. Additionally, lean-burn, CIDI ICEs have particulate, a common downfall, even to a reduced extent, of directly injected engines, including SI DI ICEs. PM originates when the injected fluid still liquid interacts with the flame thus producing soot. Soot is formed in the fuel-rich regions of the combustion chamber (Hiroyasu and Kadota, 1976; Smith, 1981; Neeft et al., 1997). Lean burn, CIDI ICEs thus need particle traps (Neeft et al., 1996; Saracco et al., 2000; Ambrogio et al., 2001; Mohr et al., 2006). This is, however, also an opportunity, as circulation in areas with background particulate may produce better air quality at the tailpipe than the intake. An added downfall of lean-burn, CIDI ICEs, these engines, typically turbocharged, are more expensive. The dual-fuel operation with LPG, CNG, or LNG, does not introduce any disadvantage in terms of regulated pollutants or CO2, but only advantages.
Fuel Conversion Efficiency
Without targeting the waste heat recovery (WHR), diesel CIDI ICEs have proven their ability to achieve peak fuel conversion efficiencies about 50% while supplying extremely high brake mean effective pressures in endurance racing (Boretti and Ordys, 2018). Thanks to high pressure, high atomization, high flow rate, and fast-actuating injectors, multiple injection strategies allow controlled combustion processes occurring in the bulk of the combustion chamber, for the best trade-off between pressure work, pressure build-up, and peak pressure.
While waste heat recovery systems (WHR) may certainly improve the steady-state fuel conversion efficiency of diesel engines (Teng et al., 2007, 2011; Teng and Regner, 2009; Park et al., 2011; Wang et al., 2014; Yu et al., 2016; Shi et al., 2018), cold start transients are the Achille's heel of traditional WHRs. Additionally, WHRs add weight, thermal inertia, packaging issues, and complexity. Innovative concepts for WHR, utilizing the coolant circuit as the pre-heater of a modified “turbo steamer” (Freymann et al., 2008, 2012) without any need of a double circuit, require significant research and development efforts.
The results achieved in endurance racing by Audi (Audi, 2014), in less than a decade of developments, are important. 2006 to 2008, Audi adopted the V12 TDI in the Audi R10 TDI. The 5.5 L engine delivered 1,100 Nm of torque. At rated speed, the very quiet-running twin-turbo produced roughly 480 kW. In 2009 and 2010, Audi moved to the V10 TDI in the Audi R15 TDI. It was shorter and lighter than the twelve-cylinder. The 5.5 L of displacement were spread across two fewer cylinders. The engine had roughly 440 kW and more than 1,050 Nm of torque. Top BMEP were exceeding 24 bars. Then, 2011 to 2013, Audi moved to the V6 TDI in the Audi R18 TDI, R18 ultra and R18 e-Tron Quattro. Downsizing of the engine brought to a displacement of 3.7 L. The lightweight and compact V6 TDI produced over 397 kW and more than 900 Nm of torque. The common-rail system generated up to 2,600 bars of pressure. Top BMEP were exceeding 30 bars.
When the focus was placed more on fuel economy, in 2014, the V6 TDI in the Audi R18 e-Tron Quattro was powered by a redesigned V6 TDI with displacement increased to 4.0 L. Maximum power was 395 kW and maximum torque was more than 800 Nm. The injection pressure was more than 2,800 bars. Fuel consumption decreased by more than 25% compared with the 3.7-liter engine. Latest (2016) power outputs from the 4-liter engine were 410 kW, corresponding to 870 Nm of torque at the maximum speed of 4,500 rpm. This translated into 27.3 bar BMEP in the maximum speed / maximum power operating point. The latest engines had a limited fuel flow rate, that for a 6 MJ energy recovery system (ERS) for braking, the maximum fuel flow rate was 71.4 kg/h. For a diesel fuel of 43.4 MJ/kg Lower Heating Value (LHV), the fuel flow power was 860.8 kW. The maximum power was thus obtained with a peak power brake efficiency η = 0.475, which is much larger than the peak efficiency of many production high-speed diesel engines, that may run, up to a peak efficiency η = 0.45 at lower engine speeds.
From computations, the maximum torque, as well as the maximum brake efficiency, were obtained at speeds <4,500 rpm, which is a technological limit of the diffusion combustion (Boretti and Ordys, 2018). Due to the constant time needed for the fuel to vaporize and mix with air, the diffusion combustion phase has a duration in terms of crank angle degrees that increases with the engine speed. Thus, at speeds above 4,500 rpm, the length of the combustion phase usually becomes excessive and much better power is obtained at lower speeds. Peak torque was highly likely more than 916 Nm, corresponding to 29 bar BMEP. Peak fuel conversion efficiency was highly likely approaching η = 0.50. Further developments for racing were within easy reach, at the time the activity was stopped in the aftermath of “diesel-gate.” Higher injection pressures, and more advanced turbocharging such as the contemporary F1 e-turbo, or super turbocharging (Boretti and Castelletto, 2018; Boretti and Ordys, 2018), could have been beneficial to normal production diesel engines for passenger cars.
Laboratory Test Emissions
The past emission certification, that was carried out by the original equipment manufacturers (OEM) and not independently tested, was flawed by inaccuracies in the tests, and inadequacy of the certification cycle (Boretti, 2017; Boretti and Lappas, 2019). The short, highly stylized new European driving cycle (NEDC), was extremely far from the real-world-driving conditions experienced by European commuters. As the OEM were forced for more than two decades to focus their RandD to produce engines compliant and fuel-efficient during this cycle, having the aggravation of the cold start, other possible uses were not regulated and left of the discretion of the OEM. Inaccuracies (and discretion) in the way the tests were handled produced many inconsistencies, starting from large spreading of carbon dioxide (CO2) emissions for the consumption of theoretically the same liter of fuel (Boretti and Lappas, 2019). The new Worldwide harmonized Light Vehicles' Test Cycle (WLTC), that has recently replaced the NEDC, because of “diesel gate” (Chossière et al., 2018), is better, being a little bit longer. However, it still involves driving conditions different from those experienced during peak hours in congested areas (Boretti and Lappas, 2019).
For a historical perspective, emission rules have been made tougher and tougher year after year, but only declared to be measured during the prescribed laboratory tests. Table 1 presents the European Union (EU) emission standards for passenger vehicles (category M) of positive (gasoline) and compression (diesel) ignition design. Unburned hydrocarbons (HC)+NOx was prescribed for gasoline and diesel only in Euro 1 and 2 standards. Emissions were tested over the NEDC by using a chassis dynamometer laboratory procedure. What was requested to the OEM over the years, was to produce vehicles emitting less than the regulated pollutant over the specific certification cycle during the laboratory tests. Real-world driving was an immaterial concept, not translated in any specific legislative requirement. The reduction of the emission limits for NOx and PM in Euro 5 and 6 resulted in dramatically increased costs of the after treatment, and in an increased, rather than reduced, fuel consumption, with sometimes also issues of drivability. Once more it is important to understand the trade-off between fuel economy and pollutant emissions and realize that excessive requests under one criterion may translate in the impossibility to meet the other criteria.
Real World Driving Emissions
Only recently, the European Union (EU) has introduced Real Driving Emissions (RDE) tests. On-the-road vehicle emissions are now measured by using portable emission analyzers (PEMs). The RDE test must last 90–120 min and include one urban (<60 km/h), one rural (60–90 km/h) and one motorway (>90 km/h) segment, of equal weight, covering a distance of at least 16 km. RDE emission limits then use conformity factors to relate to the chassis dynamometer laboratory test. For what concerns NOx, the conformity factor is 2.1 since September 2017 for new models and since September 2019 for all new vehicles. Other conformity factors are still to be defined. While the RDE test is still not representative of real-world driving in congested areas, it is non-accurate, subjective, non-reproducible, and not yet determinant (Boretti and Lappas, 2019), it is certainly a step forward.
Australian real-world driving emissions data of pre-new rules vehicles are proposed by ABMARC (ABMARC, 2017). The report prepared for the Australian Automobile Association presents the emissions and fuel consumption test results from 30 different passenger and light commercial vehicles, measured with PEMS on Australian roads. Most of the vehicles were compliant with Euro 4, 5, and 6 standards, while 1 of them was compliant with Euro 2 standards. The real-world fuel consumption of the tested vehicles, compared to the certification cycle results, was on average 23% higher, 21% higher for diesel vehicles, from 4% below to 59% above, and 24% higher for petrol vehicles, from 3% below to 55% above. One LPG vehicle had a real-world fuel consumption 27% higher than the certification cycle result. One plug-in hybrid vehicle had a real-world fuel consumption 166% higher than the certification cycle result with a full state of charge, and 337% higher when tested with a low state of charge. Fuel consumption figures for vehicles with diesel particulate filters include the application of a correction factor to account for filter regeneration.
The discrepancies between the laboratory tests and real-world driving were thus different not only for vehicles fitted with CIDI diesel ICEs, but also for vehicles with SI gasoline ICEs, and traditional as well as hybrid powertrains. However, the major difference was the NOx emissions of the CIDI diesel ICEs. In the latest EURO rules, vehicles were requested to meet increasingly stringent emission standards of regulated pollutants, while also reducing CO2 emissions. As these requirements were conflicting and difficult to meet, the discrepancy between the real-world fuel consumption and the certification cycle results increases with the standard. The Euro 6 compliant vehicles had the highest discrepancy between the real world and certification cycle results.
Regarding emissions, 13 vehicles exceeded the NOx specific emission prescribed for the certification cycle. Of these 13 vehicles, 11 were diesel vehicles. Only 1 of 12 diesel vehicles delivered a NOx specific emission within the limit of the certification cycle. Five petrol vehicles exceeded the CO limit of the certification cycle. Only 1 diesel vehicle exceeded the PM limit of the certification cycle. On average, diesel vehicles' NOx and PM emissions were 24 and 26 times higher than that of petrol vehicles, while diesel vehicles' CO emissions were 10 times lower than that of petrol vehicles. The diesel vehicles exceeded the NOx limit of the certification cycle by 370%, while the petrol vehicles emitted 43% of the NOx limit of the certification cycle. The petrol vehicles emitted 95% of the CO limit of the certification cycle. The diesel vehicles emitted 20% of the CO limit of the certification cycle. For what concerns PM, the diesel vehicles emitted 43% of the PM limit of the certification cycle, and the 2-petrol gasoline direct injection (GDI) vehicles emitted 26% of the PM limit of the certification cycle. For what concerns the NOx emissions of lean-burn CI engines, the measured results were better than what was claimed during “diesel gate,” or it is claimed in works such as (Chossière et al., 2018).
New rules have been introduced since “diesel gate,” and CIDI diesel engines have been improved. European real-world driving emissions data of post-new rules vehicles are proposed by ACEA (2018a). In a properly conducted experimental campaign, under repeatable conditions, with adequate equipment, and applying the scientific method, the European Automobile Manufacturers' Association (ACEA), has recently shown that all the 270 diesel vehicles tested were below the emission limits of the newly defined real-world driving (RDE) tests, both total and urban. None of the vehicles was above the NOx specific emission of 165 mg/km that is now prescribed (ACEA, 2018a), Figure 1. The detailed type-approval results for the 270 RDE-compliant diesel vehicle types are available in ACEA (2018b). The RDE results for individual vehicles can be found at (ACEA, 2018c).
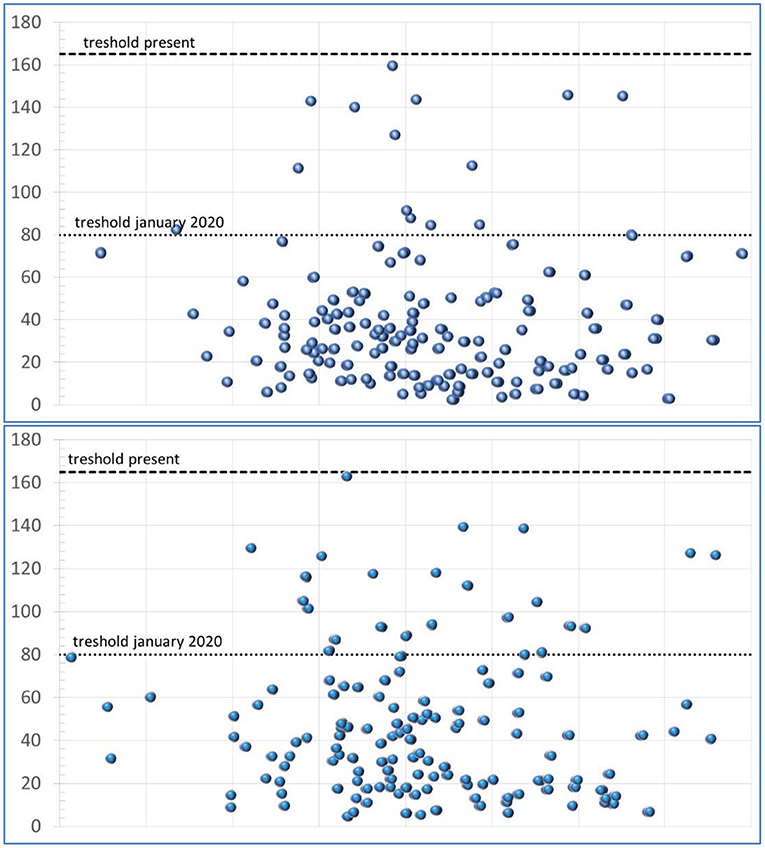
Figure 1. Real-world driving emissions of NOx of diesel vehicles. NOx total (mg/km) vs. PM total (#/km). Top total RDE. Bottom urban RDE. Data digitized from www.acea.be/uploads/press_releases_files/RDE-compliant_diesels_November_2018.pdf.
The new data released by the ACEA supply unambiguous evidence that the latest-generation diesel vehicles emit low pollutant emissions on the road and are fuel-efficient. The tests were performed in real-driving conditions by the drivers of the various national type-approval authorities. The 270 new types of diesel cars certified against the latest Euro 6d-TEMP standard were introduced on the European market over the previous year. All these diesel cars performed very well below the NOx threshold of the RDE test, which now applies to all new car types since September 2017. Most of these vehicles have NOx emissions well below the stricter threshold that will be mandatory from January 2020. The RDE test ensures that pollutant emission levels measured during the new WLTP laboratory test are confirmed on the road. Every car tested stands for a “family” of similar cars of differing variants. This activity proves that the diesel vehicles now available on the market are low-emitting in any reasonable condition. The German automobile club (ADAC) recently estimated that there were 1,206 different RDE-compliant cars available on 30 October 2018, both gasoline and diesel (ADAC, 2018a). Hence, CIDI diesel ICEs do not deserve the bad reputation they got because of the “diesel gate,” that is a political more than a technological issue.
Modern diesel vehicles, supported by fleet renewal policies and combined with alternative powertrains, may play a strong role in helping cities to move toward compliance with air quality targets while improving fuel efficiency and reducing CO2 emissions in the short and medium-term. Recent on-road testing by the ADAC (2018b) found that the latest diesel vehicles emit 85% less NOx on average than Euro 5 cars, with the best-performing RDE-compliant Euro 6 diesel vehicles emitting as much as 95–99% less NOx than Euro 5 vehicles. Every car tested emit less than the limits for every regulated pollutant. These cars also supply an exceptional fuel economy. Additionally, there is the opportunity to produce even less CO2, and less regulated pollutant, moving to dual-fuel diesel-LNG, CNG, or LPG.
PM Benefits of Diesel Vehicles
Diesel engines are not targeted because of their PM contribution by the transportation sector to the overall air quality. However, as the air quality is poor in many parts of the world, and diesel particulate filters can help to improve the air quality, the PM argument may actually be played in favor of the diesel-based mobility, also against alternatives such as the electric mobility. While it is incorrect to claim that the more recent diesel vehicles emit “excess” NOx and make worse the air quality, the more recent diesel vehicles contribute to cleaning the air of polluted areas, for example of PM. From Table 1, old diesel vehicles were produced compliant with much less restrictive PM rules. Air pollutants are emitted from many natural and anthropogenic sources, the latter including the burning of fossil fuels in power generation, industry, households, transport, industrial processes, solvent uses, agriculture, and waste treatment. Hence, to have vehicles with tailpipe PM emissions potentially lower than in the intake is an opportunity to clean up the air.
Environmental tobacco smoke (ETS) causes fine PM indoor pollution exceeding the outdoor limits of vehicles. Data comparing PM emission from ETS and a Euro 3 diesel vehicle show indoor PM concentrations up to 10-fold those emitted from the idling Euro 3 diesel vehicle (Invernizzi et al., 2004). PM limits have been drastically improved in Euro 4, 5, and 6, 10 times to be precise. The World Health Organization (WHO) study (Martuzzi et al., 2006) shows a significant health impact of PM10 on urban populations of 13 large Italian cities, assessed in 8,220 deaths a year, attributable to PM10 concentrations above 20 μg/m. This is 9% of the mortality for all causes (excluding accidents) in the population over 30 years of age. These levels of PM10 are not the result of the circulation of the latest, clean diesel vehicles.
The performances of diesel particulate filters (DPF) are relatively complex (Fiebig et al., 2014). The latest DPF technologies are more effective on larger sizes while less effective, or even negative, on the smaller nanometric sizes. Monitoring is often limited to PM10–particles of 10-micrometer diameter—or PM2.5–particles of 2.5-micrometer diameter. DPF can capture from 30% to more than 95% of the micrometric PM (Barone et al., 2010). With optimal DPF, PM emissions may be decreased to 0.001 g/km or less (Fiebig et al., 2014), down 5 times from the present 0.005 of Euro 6. While this mass measure does not pay justice to pollution by sub-micrometric and nanometric particles, there is no present control of this type of pollutant from any source.
If new diesel vehicles do not emit more NOx than older diesel vehicles, they certainly emit much less PM, with possibly in some circumstances, the ability to clean the air of the PM produced by other sources, that are not adequately targeted by the policymakers. The case of Hong Kong, that is not the worse on Earth, is reported in Haas (2017). In addition to local emissions from various sources, including passenger vehicles, there is Hong Kong a significant amount of pollutants brought from mainland China. While data of pollutants in China is limited, it is well-known that Hong Kong is facing serious health issues linked to air pollution mostly imported from the mainland. Hong Kong's air pollution is not as bad as it is in China, or India, where the toxic cloud dubbed “airpocalypse” often covers substantial portions of these countries, but it is still one good example of more latest diesel vehicles replacing on the road older vehicles making a positive impact.
Of the many types of aerosol particles circulated in the atmosphere, one of the most damaging is PM2.5. Many areas of China and India have levels of PM2.5 and PM10 much larger than the WHO guidelines, Figure 2. The WHO guidelines (annual mean) are PM2.5 of 10 μg/m3 and PM10 of 20 μg/m3. Across the world, the mean ambient air pollution ranges from <10 to over 100 μg/m3 for PM2.5, and from <10, to over 200 μg/m3, for PM10. There are widespread cases of poor air quality not limited to China and India. However, China's south coast industrial heartland is one of the areas with the worse pollution, like Beijing and Delhi. While Beijing's “airpocalypse” is being clamped down on by drastic measures, mostly targeting the use of coal, but also limiting the circulation of any vehicle (South China Morning Post, 2018), Delhi's “airpocalypse” is reaching a new dramatic high, also thanks to “stubble burning” from neighborhoods (Indiatimes, 2018).
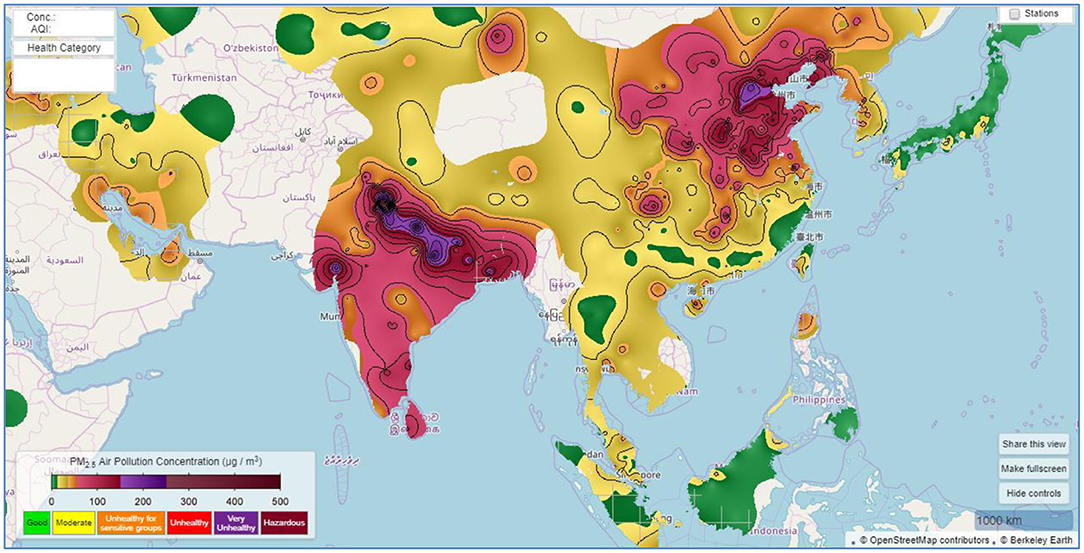
Figure 2. Nearly real-time PM2.5 map for Asia during Autumn 2018. Only the areas covered by stations are shown. Image from Berkeley Earth, www.berkeleyearth.org.
The air quality in Hong Kong is everything but excellent (Haas, 2017). Levels of pollutants have exceeded the WHO standards for over 15 years. At peaks, they have reached more than five times the acceptable levels. Emissions from vehicles and ships are some of the largest local contributors to pollution. Power plants also have their role, being reliant almost entirely on fossil fuels, mostly coal. However, about 60-70% of the PM comes from mainland China. This flux is extremely relevant especially in winter when the imported PM is about 77% of the total. Asthma and bronchial infections have rocketed in recent years. In Hong Kong alone, there were more than 1,600 actuals, not hypothetical as those of Chossière et al. (2018), premature deaths in 2016 only because of air pollution (Haas, 2017).
In addition to improved fuel standards and expansion of the use of electric vehicles, significant uptake of recent diesel vehicles equipped with particle traps may further contribute to the improved air quality of a city that still falls short of any WHO guideline. Regarding the opportunity to use electric vehicles, recharged by combustible fuel power plants, the EV may actually contribute to PM pollution. According to Hodan and Barnard (2004), the largest source of PM2.5 from anthropogenic sources are from tire and road surface wear. As the electric vehicles are heavier and have more instant torque than ICE based cars, they produce a lot more PM2.5. Hence, more EV will make Hong Kong even dirtier of PM, as they produce PM2.5 and they cannot burn the PM produced by other sources, like a CIDI diesel ICE fitted with a particle trap.
As shown in Figure 1 and Table 1, vehicles fitted with the latest CI engines do not produce excess NOx, and from Figures 2, 3, there are many areas of the world with PM concentrations in the air much larger than what can be found at the tailpipe of vehicles fitted with the latest CIDI diesel engines, Table 1, and NO2 concentrations also quite large. The dual-fuel operation with LNG, CNG or LPG, with an otherwise unchanged vehicle that keeps the particulate filter, may contribute even more to the cleaning of the ambient air from particulate.
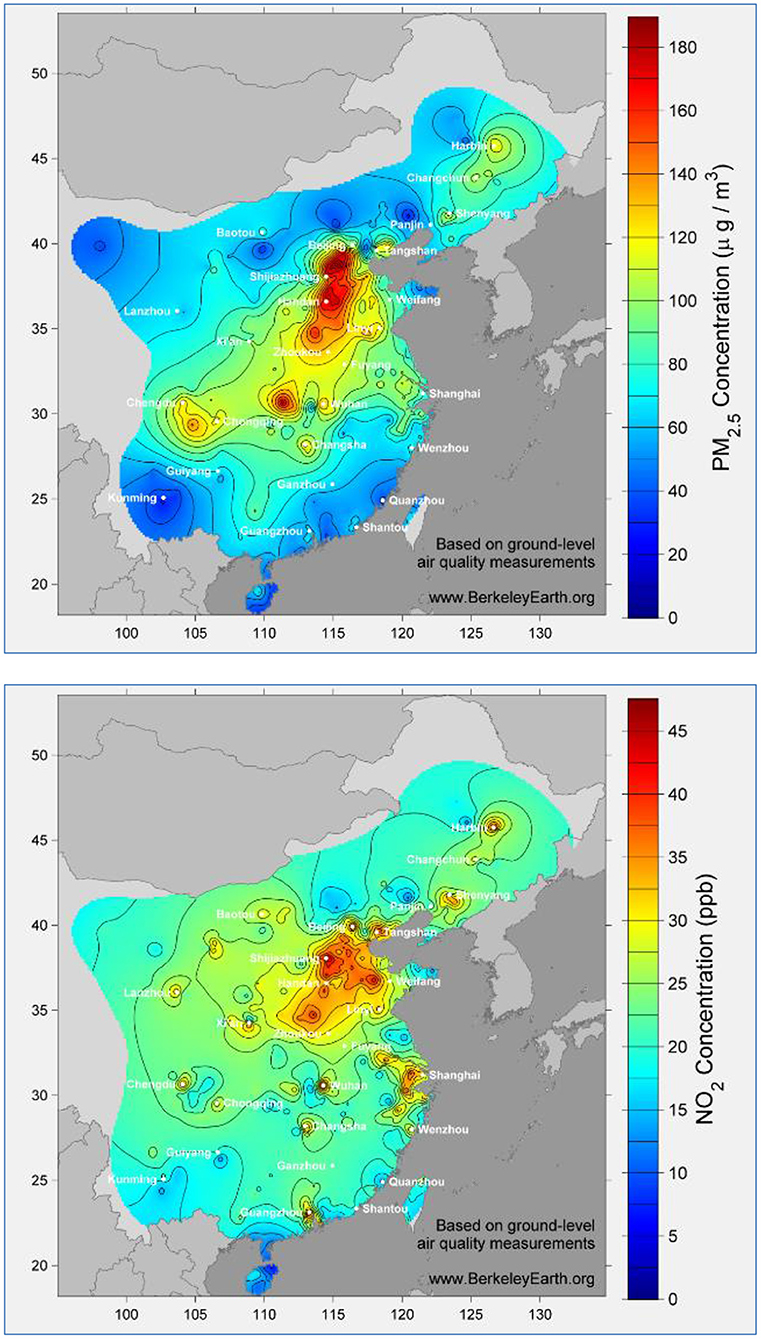
Figure 3. Average monthly concentrations for China during January 2015 of PM2.5, top, and NO2, bottom. Images from Berkeley Earth, www.berkeleyearth.org.
Advantages of Dual-Fuel Diesel-LNG/LPG/CNG
Current Technology
Diesel-LNG (Goudie et al., 2004; Osorio-Tejada et al., 2015; Laughlin and Burnham, 2016), diesel-CNG (Maji et al., 2008; Shah et al., 2011; Ryu, 2013) or diesel-LPG (Jian et al., 2001; Ashok et al., 2015) engines supply like diesel fuel conversion efficiency and power density, while improving the emissions, for both regulated pollutants (PM, NOx) and CO2. LNG can be used for heavy-duty trucks, due to the cryogenic storage. LPG (and CNG) may be preferred in passenger cars and light-duty vehicle applications.
Diesel engines still generate significant amounts of carbon dioxide (CO2) and engine-out emissions of particulate matter (PM), because of the diffusion combustion of the heavy hydrocarbon, high C/H ratio, liquid diesel fuel. Nitrogen oxides (NOx) engine-out emissions are also intrinsic to the lean-burn operation in excess air (Heywood, 1988). Both PM and NOx can be reduced through the after-treatment, albeit diesel combustion strategies are often determined for the best NOx-PM trade-off.
The use of a reduced carbon content gaseous fuel such as natural gas, that is mostly methane CH4, in liquid form as LNG, or gas form as CNG, or liquified petroleum gas (LPG), that is mostly propane C3H8, has intuitive major advantages in CO2 emissions vs. the diesel, of variable composition but roughly C13.5H23.6. Being vaporization much easier, there are also advantages for engine-out PM emissions, and thus indirectly also of engine-out NOx emissions, vs. the diesel (Kathuria, 2004; Chelani and Devotta, 2007; Yeh, 2007; Engerer and Horn, 2010; Lin et al., 2010; Kumar et al., 2011).
LNG, CNG, and LPG have smaller carbon-to-hydrogen ratios. Hence, much less CO2 is emitted to produce the same output with about the same fuel conversion efficiency. CNG is injected gas. LNG is also gas in normal conditions. LPG is liquid in normal conditions, but it vaporizes much quicker than the diesel. This practically reduces to zero the PM emissions (apart from those originating from the pilot diesel). As LNG, CNG and LPG are high octane low cetane fuels, they are difficult to use alone in a compression ignition engine. The issue is solved with the dual fuel operation (westport.com, 2019a,b). A small amount of diesel fuel produces the ignition. The LNG, CNG or LPG injected prior or after the diesel injection ignition may then burn premixed or diffusion. The first combustion phase produces a rapid pressure build-up. The rate of combustion of the second phase is determined by the injection rate of the LNG, CNG, or LPG, is targeting pressure maintenance during the first part of the expansion stroke.
One major issue with the use of LNG or CNG is the specific volume of the fuel, as the density of the gas is low at normal conditions. This creates problems for the injection system, that needs injectors with much larger cross-sectional areas of the diesel, and makes much more difficult the fast actuation, multiple injection capabilities, proper of the latest diesel injectors. This is also a problem for the storage, as the volume of fuel needed for a given amount of energy onboard the vehicle is much larger than the diesel. LNG has a better volumetric density, but it needs a cryogenic system to keep the temperature low. CNG has a smaller volumetric density and additionally needs pressurized tanks.
The Westport HPDI system for diesel and CNG/LNG is a technology very well-proven by decades (Li et al., 1999; westport.com, 2015). In the beginning, HPDI was a simple main injection of natural gas following the pilot/pre-diesel injection. Recently, HPDI is evolving toward more complex strategies, modulating premixed and diffusion combustion of the natural gas, as suggested by Boretti (2013).
Traditional HPDI in heavy-duty ICEs allows a natural gas ICE to keep diesel-like performance while deriving most of its power from natural gas. A small diesel pilot injection (5–10% of the fuel energy) is used to ignite the directly injected gas jet. The natural gas burns in a mixing-controlled, diffusion, combustion mode (Li et al., 1999; westport.com, 2015).
Future Technology
Several works describe the development trends in HPDI technology. McTaggart-Cowan et al. (2015) report on 600 bar dual fuel injectors for the LNG. The LNG combustion event is limited by the injection pressure, which dictates the rate of mixing and combustion. Significant efficiency improvements and PM reductions are achieved at high loads, and especially at higher speeds, by increasing the injection pressure from the traditional 300 bar to the latest 600 bar. Combustion is an injection rate limited. McTaggart-Cowan et al. (2015) report efficiency benefits of higher pressures about 3%, added to PM reductions of 40–60%.
Different nozzle shapes were considered by Mabson et al. (2016). A “paired-hole nozzles” injector was designed to reduce PM formation by increasing air entrainment due to jet interaction. CO and PM emissions were opposite 3–10 times higher with the paired hole nozzles. The paired hole nozzle produced larger soot aggregates and larger numbers of particles.
Mumford et al. report improvements of Westport HPDI 2.0, (Mumford et al., 2017). HPDI 2.0 delivers better performances and emissions vs. the first generation HPDI as well as the baseline diesel only. Mumford et al. (2017) also discuss the potential and challenges of higher injection pressures.
Diffusion-controlled and partially premixed combustion strategies are considered by Florea et al. (2016) by using the Westport HPDI. The partially premixed combustion, called DI2 shows promise, improving engine efficiency of more than 2 efficiency points, compared to the diffusion-controlled combustion strategy. Modulation of the two combustion phases, potentially more rewarding, is not investigated in the work.
The DI2 combustion mode is also studied in Neely et al. (2017). The natural gas is injected during the compression stroke prior to the diesel injection ignition. This partially premixed combustion of the natural gas is shown to improve both the thermal and the combustion efficiencies over traditional fumigated dual-fuel combustion mode. The partially premixed combustion of the natural gas also supplies thermal efficiency improvement over the baseline diffusion-controlled combustion where the natural gas injection occurs after the diesel ignition injection.
The effect of injection strategies on the emissions and engine performances from an HPDI engine is studied by Faghani et al. (2017a,b). They investigate the effect of late post-injection (LPI), as well as slightly premixed combustion (SPC) on emission and engine performance. With SPC, the diesel injection is delayed. High load SPC operation reduces over 90% the PM with a 2% improvement in fuel efficiency with the almost same level of NOx. SPC has however large cycle-to-cycle variation and excessive rate of pressure build-up. PM does not increase for SPC with higher EGR level, higher global oxygen-based equivalence ratio (EQR) or higher pilot mass, which normally increases PM in mixing-controlled HPDI combustion. LPI, a post-injection of 10–25% of the total fuel occurring after the main combustion event, results in significant PM reductions with only small effects on other emissions and engine performance. The main PM reduction from LPI is due to the reduced amount of fuel in the first injection. The second injection makes an insignificant net contribution to the total PM.
The Westport HPDI dual fuel diesel-LNG injector is producing excellent results. However, there is a fundamental downfall of this approach. It does not offer the same performance of a latest-generation diesel-only injector, in both flow rate, speed of actuation, and atomization of the diesel. Thus, it may be preferable to couple to one latest generation diesel-only injector, with a dedicated injector for the second fuel, to deliver better injection performances for the diesel as well as the second fuel. Higher injection pressures and faster actuation are drivers of improved combustion patterns.
Dual fuel diesel-hydrogen CIDI ICEs featuring this possibility of two direct injectors per cylinder were studied for example in (Boretti, 2011b,c). One injector was used for the diesel, and one for the hydrogen. A modeled diesel engine converted to dual fuel diesel-hydrogen following this approach was shown to deliver full load efficiencies up to 40–45%, and reduced penalties in efficiencies reducing the load working slightly better than the baseline diesel in every operating point. While the use of two injectors per cylinder does not pose an issue for new engines, it is difficult to introduce two injectors when retrofitting existing diesel engines. Dedicated direct injectors for LNG, LPG, or CNG, need to be further evolved for the specific application.
The use of two dedicated injectors, rather than one dual fuel injector, of higher injection pressure, faster actuation, and complete independence of the individual fuels' injection, allows much more flexibility in injection shaping. The dual-fuel operation is typically characterized by pilot/pre-diesel injections, followed by the main second fuel injection. Preferably, the second fuel has not to be all injected after the diesel injection ignition. It may be injected before, or at the same time as the diesel, or after the diesel, not only in a single injection but in multiple injections. Thus, the second fuel may burn partially premixed and partially diffusion.
Different combustion modes are possible. “Controlled” HCCI is one of these modes. In controlled HCCI, the second fuel is injected first, and the diesel injection ignition occurs prior to the expected start of the HCCI autoignition (Boretti, 2011a,b). HCCI has no advantage, in terms of fuel conversion efficiency, vs. the bulk combustion in the center of the chamber surrounded by a cushion of air. Homogeneous combustion always suffers from larger heat losses at the walls and incomplete combustion for the quenching of the flame. HCCI also does not produce a peak pressure during the expansion stroke, delivering peak pressure exactly at top dead center. However, HCCI may have advantages for engine-out emissions, as it is an extremely low-temperature process, and it is the combustion event much closer to the theoretically best isochoric combustion of pressure cycle analyses.
The most interesting modes are premixed, diffusion or modulated premixed and diffusion in the center of the chamber. In premixed, but stratified combustion, the second fuel is injected at the center of the chamber and burned by the diesel injection before filling homogeneously all the chamber. In diffusion combustion, the second fuel is injected at the center of the chamber, after the diesel injection ignition has created suitable conditions for the next combustion to progress diffusion-controlled, and there it burns. There is the scope for pre- injections of the second fuel, as well as contemporary, or post-injections of the second fuel, with reference to the diesel pilot/pre-injection ignition, to be carefully shaped for producing the best fuel conversion efficiency, within constraints of engine-out emissions, pressure build-up rate, and peak pressure.
The Electric Mobility Alternative Is Still Premature
The environmental friendliness and economy of the diesel-based mobility fact is not recognized by many countries, that have otherwise deliberated toward a premature move to the electric mobility, without having addressed first the many issue of electric vehicles, i.e., the high economic and environmental costs to build, run and dispose of the cars, the limited performances of these heavy vehicles due to the still inadequate battery technologies, the lack of a recharging infrastructure only feed by renewable energy.
Nominally to address global warming, more than air pollution, Britain, France, and China have deliberated the end of the ICE-based mobility by 2040. However, the IEA data (IEA, 2018), shows that the production of electricity geothermal, solar, wind, tide, wave, and the ocean was still about 1% of the total in 2015, with the total primary energy supply (TPES) that is largely exceeding the electricity production. With the solar and wind share of the TPES still small, it does not make any sense to propose only electric vehicles, even forgetting the other key issues embedded in the electric mobility quest.
Presently, the CO2 emission life cycle analyses (LCA), do not show a clear advantage of electric vs. ICE-based mobility (Boretti, 2018). The LCA case for electric mobility critically depends on how the electricity is generated, that without an enormous increment of the energy storage, more than the simple increase of the registered capacity of wind and solar, needs back up by fossil fuels. Since the 1990s, there have been advancements, but not yet the breakthroughs needed, in battery technologies. It is still too expensive, economically, and environmentally, to produce, use and dispose of electric vehicles, with the added challenge of the materials needed to produce the batteries that are at a greater risk of depletion than the fossil fuels (Boretti, 2018). Additionally, these materials are unethically mined in very few places.
Amnesty International (Onstad, 2019) recently pointed out as the electric vehicle (EV) industry is selling itself as environmentally friendly but is producing many of its batteries using fossil fuels and unethically sourced minerals tainted by human rights abuses. It is unlikely that there are enough raw materials to meet the dramatic demand expected for lithium-ion batteries of electric vehicles, and grid-connected battery systems for storing intermittent wind and solar renewable energy electricity (Jaffe, 2017). Furthermore, without any clear path considered for recycling, and the negative past (and present) examples of recycling by industrialized countries at the expense of environmental damage in developing countries (Minter, 2016), the electric mobility may translate in considerable damage to the economy and the environment.
While the electric mobility may certainly solve some of the issues of air pollution-related to transport, it is unlikely it may occur soon, it does not address the pollution from other sources, and it is not yet that friendly, in the big picture, where everything is included. Consumption of combustion fuels is still dramatically increasing, and there are very few examples of technological opportunities to convert the chemical energy of the fuels into mechanical or electrical power with a better fuel energy conversion efficiency, and reduced pollutant emissions, of the CIDI diesel ICEs. There will be enormous costs, also in terms of GHG emissions, to switch to electric mobility in the transportation sector.
Discussion and Conclusions
Although the ICCT, US EPA, and CARB depict diesel vehicles as being bad for the environment, the latest real-world driving tests conducted by ACEA show that this is incorrect. Modern diesel vehicles have relatively low CO2 and pollutant emissions, including NOx, and PM. As they are, the circulation of diesel vehicles in highly polluted areas may improve the quality of the air that is polluted by other sources, not only older diesel vehicles.
CIDI diesel ICEs can be made better, much more environmentally friendly, thanks to the further developments in the injection system as well as the after-treatment. CIDI ICEs may also be improved by simply adopting dual-fuel designs, with LPG, CNG, or LNG the second fuel. These alternative fuels allow the same, or better, performances of the diesel-only ICE, for what concerns steady-state torque, power, and fuel conversion efficiency, and transients, while dramatically improving the CO2 emissions, as well as the PM and NOx engine-out emissions.
In addition to the better C-H ratio for what concerns the CO2 emission, the advantages of the dual-fuel CIDI ICEs with LNG, CNG, or LPG also originate from the opportunity of modulating premixed and diffusion phases of combustion injecting the second fuel, that is much easier to vaporize, and less prone to auto-ignition, prior, contemporary or after the pre/pilot diesel. Also relevant especially with LNG is the cooling effect due to the cryogenic injection. Further developments in the injection system are the area of major concern for the development of these novels CIDI dual-fuel ICEs.
The advantages of CIDI ICEs, diesel or dual-fuel vs. every other alternative solution for transport applications, are presently not acknowledged by any policymaker. The European carmakers have already stopped their research and development plans for their ICEs to focus only on electric cars. Considering the unresolved issues embedded in electric mobility, this may prove shortly to be wrong for the economy and the environment. The use of more recent diesel vehicles, and dual fuel diesel-alternative fuels vehicles, may only save lives, not cause mortalities, improving air quality, while limiting the depletion of natural resources and the emission of CO2 without requiring unaffordable efforts and dramatic changes.
Author Contributions
The author confirms being the sole contributor of this work and has approved it for publication.
Conflict of Interest
The author declares that the research was conducted in the absence of any commercial or financial relationships that could be construed as a potential conflict of interest.
References
ABMARC (2017). Real-World Driving. Fuel Efficiency and Emissions Testing. Available online at: www.aaa.asn.au/storage/2017-abmarc-aaa-rde-report-executive-summary-first-17.pdf
ACEA (2018a). Diesel: New Data Proves That Modern Diesel Cars Emit Low Pollutant Emissions on the Road. Available online at: www.acea.be/press-releases/article/diesel-new-data-proves-that-modern-diesel-cars-emit-low-pollutant-emissions
ACEA (2018c). RDE Results for Individual Vehicles. Available online at: www.acea.be/publications/article/access-to-euro-6-rde-monitoring-data
ACEA (European Automobile Manufacturers' Association) (2018b). The Type-Approval Results for the 270 RDE-Compliant Diesel Vehicle Types. Available online at: www.acea.be/uploads/press_releases_files/RDE-compliant_diesels_November_2018.pdf
ADAC (2018a). Overview of RDE-Compliant Cars Available on the Market. Available online at: www.adac.de/infotestrat/umwelt-und-innovation/abgas/modelle_mit_euro_6d_temp/
ADAC (2018b) Ecotest, Moderne Diesel Sind Sehr Sauber. Available online at: www.presse.adac.de/meldungen/adac-ev/technik/euro6-d-temp-diesel-sind-sehr-sauber.html.
Ambrogio, M., Saracco, G., and Specchia, V. (2001). Combining filtration and catalytic combustion in particulate traps for diesel exhaust treatment. Chem. Eng. Sci. 56, 1613–1621. doi: 10.1016/S0009-2509(00)00389-4
Ashok, B., Ashok, S. D., and Kumar, C. R. (2015). LPG diesel dual fuel engine–A critical review. Alexand. Eng. J. 54, 105–126. doi: 10.1016/j.aej.2015.03.002
Audi (2014). The Racing Engines. Available online at: www.audi-mediacenter.com/en/the-audi-tdi-tech-workshop-2014-3039/the-racing-engines-3109
Autocar (2018). BMW to Cease Production of the i3 Range Extender. Available online at: www.autocar.co.uk/car-news/new-cars/bmw-cease-production-i3-range-extender
Ayre, M. (2007). Will Biofuel Leave the Poor Hungry?. Available online at: news.bbc.co.uk/1/hi/business/7026105.stm
Barone, T. L., Storey, J. M., and Domingo, N. (2010). An analysis of field-aged diesel particulate filter performance: particle emissions before, during, and after regeneration. J. Air Waste Manage. Assoc. 60, 968–976. doi: 10.3155/1047-3289.60.8.968
Boretti, A. (2011a). Diesel-like and HCCI-like operation of a truck engine converted to hydrogen. Int. J. Hydr. Energy 36, 15382–15391. doi: 10.1016/j.ijhydene.2011.09.005
Boretti, A. (2011b). Advances in hydrogen compression ignition internal combustion engines. Int. J. Hydr. Energy 36, 12601–12606. doi: 10.1016/j.ijhydene.2011.06.148
Boretti, A. (2011c). Advantages of the direct injection of both diesel and hydrogen in dual fuel H2ICE. Int. J. Hydr. Energy 36, 9312–9317. doi: 10.1016/j.ijhydene.2011.05.037
Boretti, A. (2013). Latest concepts for combustion and waste heat recovery systems being considered for hydrogen engines. Int. J. Hydr. Energy 38, 3802–3807. doi: 10.1016/j.ijhydene.2013.01.112
Boretti, A. (2017). The Future of the Internal Combustion Engine After “Diesel-Gate. Warrendale, PA: SAE Technical Paper 2017-28-1933. doi: 10.4271/2017-28-1933
Boretti, A. (2018). Life Cycle Analysis Comparison of Electric and Internal Combustion Engine Based Mobility. Warrendale, PA: SAE Technical Paper 2018-28-0037. doi: 10.4271/2018-28-0037
Boretti, A., and Castelletto, S. (2018). “Super-turbo-charged direct injection jet ignition gasoline engine,” in Proceedings of the FISITA World Automotive Conference, 2–5> OCTOBER 2018 (Chennai).
Boretti, A., and Lappas, P. (2019). Complex independent laboratory tests to signify fuel economy and emissions under real world driving. Adv. Technol. Innovat. 4, 59–72.
Boretti, A., and Ordys, A. (2018). Super-Turbocharging the Dual Fuel Diesel Injection Ignition Engine. SAE Technical Paper 2018-28-0036. doi: 10.4271/2018-28-0036
Burtscher, H. (2005). Physical characterization of particulate emissions from diesel engines: a review. J. Aerosol. Sci. 36, 896–932. doi: 10.1016/j.jaerosci.2004.12.001
Camuzeaux, J. R., Alvarez, R. A., Brooks, S. A., Browne, J. B., and Sterner, T. (2015). Influence of methane emissions and vehicle efficiency on the climate implications of heavy-duty natural gas trucks. Environ. Sci. Technol. 49, 6402–6410. doi: 10.1021/acs.est.5b00412
CARB (2012). Final Statement of Reasons For Rulemaking Including Summary of Comments and Agency Responses. 2012 Amendments to the Zero Emission Vehicle Regulations. Available online at: www.arb.ca.gov/regact/2012/zev2012/zevfsor.pdf
Chelani, A. B., and Devotta, S. (2007). Air quality assessment in Delhi: before and after CNG as fuel. Environ. Monit. Assess. 125, 257–263. doi: 10.1007/s10661-006-9517-x
Chossière, G. P., Malina, R., Allroggen, F., Eastham, S. D., Speth, R. L., and Barrett, S. R. (2018). Country-and manufacturer-level attribution of air quality impacts due to excess NOx emissions from diesel passenger vehicles in Europe. Atmos. Environ. 189, 89–97. doi: 10.1016/j.atmosenv.2018.06.047
Crabtree, G. W., Dresselhaus, M. S., and Buchanan, M. V. (2004). The hydrogen economy. Phys. Today 57, 39–44. doi: 10.1063/1.1878333
EIA (2018). Table 8.2. Average Tested Heat Rates by Prime Mover and Energy Source, 2007 – 2017. Available online at: www.eia.gov/electricity/annual/html/epa_08_02.html
Engerer, H., and Horn, M. (2010). Natural gas vehicles: an option for Europe. Energy Policy 38, 1017–1029. doi: 10.1016/j.enpol.2009.10.054
Faghani, E., Kheirkhah, P., Mabson, C., McTaggart-Cowan, G., et al. (2017a). Effect of Injection Strategies on Emissions from a Pilot-Ignited Direct-Injection Natural-Gas Engine- Part I: Late Post Injection. Warrendale, PA: SAE Paper 2017-01-0774. doi: 10.4271/2017-01-0774
Faghani, E., Kheirkhah, P., Mabson, C., McTaggart-Cowan, G., et al. (2017b). Effect of Injection Strategies on Emissions from a Pilot-Ignited Direct-Injection Natural-Gas Engine- Part II: Slightly Premixed Combustion. Warrendale, PA: SAE Technical Paper 2017-01-0763. doi: 10.4271/2017-01-0763
Fiebig, M., Wiartalla, A., Holderbaum, B., and Kiesow, S. (2014). Particulate emissions from diesel engines: correlation between engine technology and emissions. J. Occup. Med. Toxicol. 9:6. doi: 10.1186/1745-6673-9-6
Florea, R., Neely, G., Abidin, Z., and Miwa, J. (2016). Efficiency and Emissions Characteristics of Partially Premixed Dual-Fuel Combustion by Co-Direct Injection of NG and Diesel Fuel (DI2). Warrendale, PA: SAE Paper 2016-01-0779. doi: 10.4271/2016-01-0779
Freymann, R., Ringler, J., Seifert, M., and Horst, T. (2012). The second generation turbosteamer. MTZ Worldwide 73, 18–23. doi: 10.1365/s38313-012-0138-1
Freymann, R., Strobl, W., and Obieglo, A. (2008). The turbosteamer: a system introducing the principle of cogeneration in automotive applications. MTZ Worldwide 69, 20–27. doi: 10.1007/BF03226909
Goudie, D., Dunn, M., Munshi, S. R., Lyford-Pike, E., Wright, J., Duggal, V., et al. (2004). Development of a Compression Ignition Heavy Duty Pilot-Ignited Natural Gas Fuelled Engine for Low NOx Emissions (No. 2004-01-2954). Warrendale, PA: SAE Technical Paper. doi: 10.4271/2004-01-2954
Haas, B. (2017). Where the Wind Blows: How China's Dirty Air Becomes Hong Kong's Problem. Available online at: www.theguardian.com/cities/2017/feb/16/hong-kong-death-trap-dirty-air-pollution-china
Heywood, J. B. (1988). “Combustion in compression-ignition engines,” in Internal Combustion Engine Fundamentals (New York, NY:McGraw-Hill), 522–562.
Hiroyasu, H., and Kadota, T. (1976). Models for combustion and formation of nitric oxide and soot in direct injection diesel engines. SAE Trans. 85, 513–526. doi: 10.4271/760129
Hodan, W. M., and Barnard, W. R. (2004). Evaluating the Contribution of PM2. 5 Precursor Gases and Re-entrained Road Emissions to Mobile Source PM2. 5 Particulate Matter Emissions. Research Triangle Park, NC: MACTEC Federal Programs. Available online at: www3.epa.gov/ttnchie1/conference/ei13/mobile/hodan.pdf
IEA (2018). IEA World Energy Balances 2018. Available online at: webstore.iea.org/world-energy-balances-2018
IEA (2019). Transport Biofuels. Available online at: www.iea.org/tcep/transport/biofuels/
Inderwildi, O. R., and King, D. A. (2009). Quo vadis biofuels? Energy Environ. Sci. 2, 343–346. doi: 10.1039/b822951c
Indiatimes (2018). Construction Activities Banned Till November 12 To Save Delhi From ‘Airpocalypse’. But Is It Enough? Available online at: www.indiatimes.com/news/india/construction-activities-banned-till-november-12-to-save-delhi-from-airpocalypse-but-is-it-enough-356413.html
Invernizzi, G., Ruprecht, A., Mazza, R., Rossetti, E., Sasco, A., Nardini, S., et al. (2004). Particulate matter from tobacco versus diesel car exhaust: an educational perspective. Tobacco Control 13, 219–221. doi: 10.1136/tc.2003.005975
Jaffe, S. (2017). Vulnerable links in the lithium-ion battery supply chain. Joule 1, 225–228. doi: 10.1016/j.joule.2017.09.021
Jian, D., Xiaohong, G., Gesheng, L., and Xintang, Z. (2001). Study on Diesel-LPG Dual Fuel Engines (No. 2001-01-3679). Warrendale, PA: SAE Technical Paper. doi: 10.4271/2001-01-3679
Johnson, T. V. (2009). Review of diesel emissions and control. Int. J. Eng. Res. 10, 275–285. doi: 10.1243/14680874JER04009
Kathuria, V. (2004). Impact of CNG on vehicular pollution in Delhi: a note. Transport. Res. Part D. 9, 409–417. doi: 10.1016/j.trd.2004.05.003
Khair, M. K., and Majewski, W. A. (2006). Diesel Emissions and their Control (Vol. 303). Warrendale, PA: SAE Technical Paper. doi: 10.4271/R-303
Kingsbury, K. (2007). After the Oil Crisis, a Food Crisis? Available online at: www.time.com/time/business/article/0,8599,1684910,00.html?iid = sphere-inline-sidebar
Knecht, W. (2008). Diesel engine development in view of reduced emission standards. Energy 33, 264–271. doi: 10.1016/j.energy.2007.10.003
Kumar, S., Kwon, H. T., Choi, K. H., Lim, W., Cho, J. H., Tak, K., et al. (2011). LNG: an eco-friendly cryogenic fuel for sustainable development. Appl. Energy 88, 4264–4273. doi: 10.1016/j.apenergy.2011.06.035
Laughlin, M., and Burnham, A. (2016). Case Study: Natural Gas Regional Transport Trucks (No. DOE/CHO-AC02-06CH11357-1603). Argonne, IL; Columbia, MD: Energetics; Argonne National Laboratory.
Li, G., Ouellette, P., Dumitrescu, S., and Hill, P. G. (1999). Optimization Study of Pilot-Ignited Natural Gas Direct-Injection in Diesel Engines. Warrendale, PA: SAE Paper 1999-01-3556. doi: 10.4271/1999-01-3556
Lin, W., Zhang, N., and Gu, A. (2010). LNG (liquefied natural gas): a necessary part in China's future energy infrastructure. Energy 35, 4383–4391. doi: 10.1016/j.energy.2009.04.036
Lloyd, A. C., and Cackette, T. A. (2001). Diesel engines: environmental impact and control. J. Air Waste Manage. Assoc. 51, 809–847. doi: 10.1080/10473289.2001.10464315
Mabson, C., Faghani, E., Kheirkhah, P., Kirchen, P., et al. (2016). Combustion and Emissions of Paired-Nozzle Jets in a Pilot-Ignited Direct-Injection Natural Gas Engine. Warrendale, PA: SAE Paper 2016-01-0807. doi: 10.4271/2016-01-0807
Maji, S., Pal, A., and Arora, B. B. (2008). Use of CNG and Diesel in CI Engines in Dual Fuel Mode (No. 2008-28-0072). Warrendale, PA: SAE Technical Paper. doi: 10.4271/2008-28-0072
Marbán, G., and Valdés-Solís, T. (2007). Towards the hydrogen economy? Int. J. Hydr. Energy 32, 1625–1637. doi: 10.1016/j.ijhydene.2006.12.017
Maricq, M. M. (2007). Chemical characterization of particulate emissions from diesel engines: a review. J. Aerosol. Sci. 38, 1079–1118. doi: 10.1016/j.jaerosci.2007.08.001
Martuzzi, M., Mitis, F., Iavarone, I., and Serinelli, M. (2006). Health Impact of PM10 and Ozone in 13 Italian Cities. WHO Regional Office for Europe.
McKone, T. E., Nazaroff, W. W., Berck, P., Auffhammer, M., Lipman, T., Torn, M. S., et al. (2011). Grand challenges for life-cycle assessment of biofuels. Environ. Sci. Technol. 45, 1751–1756. doi: 10.1021/es103579c
McTaggart-Cowan, G., Mann, K., Huang, J., Singh, A., et al. (2015). Direct injection of natural gas at up to 600 bar in a pilot-ignited heavy-duty engine. SAE Int. J. Eng. 8, 981–996. doi: 10.4271/2015-01-0865
Minter, A. (2016). The Burning Truth Behind an E-Waste Dump in Africa. SMITHSONIAN.COM. Available online at: www.smithsonianmag.com/science-nature/burning-truth-behind-e-waste-dump-africa-180957597/
Mohr, M., Forss, A. M., and Lehmann, U. (2006). Particle emissions from diesel passenger cars equipped with a particle trap in comparison to other technologies. Environ. Sci. Technol. 40, 2375–2383. doi: 10.1021/es051440z
Mollenhauer, K., and Tschöke, H., (eds.). (2010). Handbook of Diesel Engines, Vol. 1. Berlin: Springer. doi: 10.1007/978-3-540-89083-6
Mumford, D., Goudie, D., and Saunders, J. (2017). Potential and Challenges of HPDI. Warrendale, PA: SAE Paper 2017-01-1928. doi: 10.4271/2017-01-1928
Muradov, N. Z., and Veziroglu, T. N. (2005). From hydrocarbon to hydrogen–carbon to hydrogen economy. Int. J. Hydr. Energy 30, 225–237. doi: 10.1016/j.ijhydene.2004.03.033
Neeft, J. P., Makkee, M., and Moulijn, J. A. (1996). Diesel particulate emission control. Fuel Process. Technol. 47, 1–69. doi: 10.1016/0378-3820(96)01002-8
Neeft, J. P., Nijhuis, T. X., Smakman, E., Makkee, M., and Moulijn, J. A. (1997). Kinetics of the oxidation of diesel soot. Fuel 76, 1129–1136. doi: 10.1016/S0016-2361(97)00119-1
Neely, G., Florea, R., Miwa, J., and Abidin, Z. (2017). Efficiency and Emissions Characteristics of Partially Premixed Dual-Fuel Combustion by Co-Direct Injection of NG and Diesel Fuel (DI2) - Part 2. Warrendale, PA: SAE Paper 2017-01-0766. doi: 10.4271/2017-01-0766
Nica, G. (2016). Why Did BMW Really Stop Making the Hydrogen 7 Model? Available online at: www.bmwblog.com/2016/08/17/bmw-stop-making-hydrogen-7-model/
O'Boyle, M. (2018). How Volkswagen's Dieselgate Billions Are Helping to Jump-Start EV Charging. Available online at: www.energypost.eu/are-electric-vehicle-charging-corridors-the-best-way-to-spend-volkswagens-dieselgate-billions/
Olah, G. A. (2004). After oil and gas: methanol economy. Catal. Lett. 93, 1–2. doi: 10.1023/B:CATL.0000017043.93210.9c
Olah, G. A. (2005). Beyond oil and gas: the methanol economy. Angew. Chem. Int. Edn. 44, 2636–2639. doi: 10.1002/anie.200462121
Onstad, E. (2019). Amnesty Faults Electric Vehicle Batteries as Carbon Intensive. Available online at: www.uk.mobile.reuters.com/article/amp/idUKKCN1R200B
Osorio-Tejada, J., Llera, E., and Scarpellini, S. (2015). LNG: an alternative fuel for road freight transport in Europe. WIT Trans. Built Environ. 168, 235–246. doi: 10.2495/SD150211
Park, T., Teng, H., Hunter, G. L., van der Velde, B., and Klaver, J. (2011). A Rankine Cycle System for Recovering Waste Heat from Hd Diesel Engines-Experimental Results (No. 2011-01-1337). Warrendale, PA: SAE Technical Paper. doi: 10.4271/2011-01-1337
Parker, A. (2019). Some Examples the War Against the Internal Combustion Engine by CARB and US EPA is not Based on Real Scientific Evidence. Available online at: www.wattsupwiththat.com/2019/03/22/the-war-against-the-internal-combustion-engine-by-carb-and-us-epa-is-not-based-on-real-scientific-evidence-examples/
phys.org (2018). Five things to know about VW's ‘dieselgate’ scandal. Available online at: www.phys.org/news/2018-06-vw-dieselgate-scandal.html#jCp
Raftery, T. (2018). Seven Reasons Why The Internal Combustion Engine Is A Dead Man Walking [Updated]. Available online at: www.forbes.com/sites/sap/2018/09/06/seven-reasons-why-the-internal-combustion-engine-is-a-dead-man-walking-updated/#1284409e603f
Ramsbrock, J., Vilimek, R., and Weber, J. (2013). “Exploring electric driving pleasure–the BMW EV pilot projects,” in International Conference on Human-Computer Interaction (Berlin; Heidelberg: Springer), 621–630. doi: 10.1007/978-3-642-39262-7_70
Ravikumar, A. P. (2018). How to Make the Liquefied Natural Gas Industry More Sustainable. Available online at: www.phys.org/news/2018-11-liquefied-natural-gas-industry-sustainable.html
Reşitoglu, I. A., Altinişik, K., and Keskin, A. (2015). The pollutant emissions from diesel-engine vehicles and exhaust aftertreatment systems. Clean Technol. Environm. Policy 17, 15–27. doi: 10.1007/s10098-014-0793-9
Ryu, K. (2013). Effects of pilot injection timing on the combustion and emissions characteristics in a diesel engine using biodiesel–CNG dual fuel. Appl. Energy 111, 721–730. doi: 10.1016/j.apenergy.2013.05.046
Saracco, G., Russo, N., Ambrogio, M., Badini, C., and Specchia, V. (2000). Diesel particulate abatement via catalytic traps. Catal. Today, 60, 33–41. doi: 10.1016/S0920-5861(00)00314-X
Schipper, L., Marie-Lilliu, C., and Fulton, L. (2002). Diesels in Europe: analysis of characteristics, usage patterns, energy savings and Co2 emission implications. J. Transp. Econ. Policy 36, 305–340.
Scott, P., and Burton, M. (2013). The New BMW i3. Hg. v. BMW AG. Available online at: www.asymcar.com/graphics/14/i3/bmwi3b.pdf
Shah, A., Thipse, S. S., Tyagi, A., Rairikar, S. D., Kavthekar, K. P., Marathe, N. V., et al. (2011). Literature Review and Simulation of Dual Fuel Diesel-CNG Engines (No. 2011-26-0001). Warrendale, PA: SAE Technical Paper. doi: 10.4271/2011-26-0001
Shi, L., Shu, G., Tian, H., and Deng, S. (2018). A review of modified Organic Rankine cycles (ORCs) for internal combustion engine waste heat recovery (ICE-WHR). Renew. Sustain. Energy Rev. 92, 95–110. doi: 10.1016/j.rser.2018.04.023
Smith, O. I. (1981). Fundamentals of soot formation in flames with application to diesel engine particulate emissions. Prog. Energy Combust. Sci. 7, 275–291. doi: 10.1016/0360-1285(81)90002-2
South China Morning Post (2018). ‘Airpocalypse’ Over? Beijing Breathes Easier as Clean Air Drive Pays Off, US Embassy Smog Readings Suggest. Available online at: www.scmp.com/news/china/policies-politics/article/2160444/beijings-clean-air-drive-paying-swift-recovery
Taylor, E. (2018). Volkswagen Says Last Generation of Combustion Engines to be Launched in 2026. Available online at: www.reuters.com/article/us-volkswagen-emissions-combustion/volkswagen-says-last-generation-of-combustion-engines-to-be-launched-in-2026-idUSKBN1O32O6
Teng, H., Klaver, J., Park, T., Hunter, G. L., and van der Velde, B. (2011). A Rankine Cycle System for Recovering Waste Heat from HD Diesel Engines-WHR System Development (No. 2011-01-0311). Warrendale, PA: SAE Technical Paper. doi: 10.4271/2011-01-0311
Teng, H., and Regner, G. (2009). Improving Fuel Economy for HD Diesel Engines with WHR Rankine Cycle Driven by EGR Cooler Heat Rejection (No. 2009-01-2913). Warrendale, PA: SAE Technical Paper. doi: 10.4271/2009-01-2913
Teng, H., Regner, G., and Cowland, C. (2007). Waste Heat Recovery of Heavy-Duty Diesel Engines by Organic Rankine Cycle Part I: Hybrid Energy System of Diesel and Rankine Engines (No. 2007-01-0537). Warrendale, PA: SAE Technical Paper. doi: 10.4271/2007-01-0537
United Nations (2019). World Water Development Report 2019. Available online at: www.unwater.org/publications/world-water-development-report-2019/
Wang, T., Zhang, Y., Zhang, J., Peng, Z., and Shu, G. (2014). Comparisons of system benefits and thermo-economics for exhaust energy recovery applied on a heavy-duty diesel engine and a light-duty vehicle gasoline engine. Energy Conv. Manage. 84, 97–107. doi: 10.1016/j.enconman.2014.04.022
westport.com (2015). Westport HPDI 2.0 on Track for Commercial Production. Available online at: www.westport.com/news/2015/westport-hpdi-2.0-on-track-for-commercial-production
westport.com (2019a). 1st Gen. Westport HPDI Technology. Available online at: www.westport.com/old-pages/combustion/hpdi/integration
westport.com (2019b). Westport™ HPDI 2.0. Available online at: www.westport.com/is/core-technologies/hpdi-2
Yeh, S. (2007). An empirical analysis on the adoption of alternative fuel vehicles: the case of natural gas vehicles. Energy Policy 35, 5865–5875. doi: 10.1016/j.enpol.2007.06.012
Yu, G., Shu, G., Tian, H., Huo, Y., and Zhu, W. (2016). Experimental investigations on a cascaded steam-/organic-Rankine-cycle (RC/ORC) system for waste heat recovery (WHR) from diesel engine. Energy Conv. Manage. 129, 43–51. doi: 10.1016/j.enconman.2016.10.010
Zervas, E., Poulopoulos, S., and Philippopoulos, C. (2006). CO2 emissions change from the introduction of diesel passenger cars: case of Greece. Energy 31, 2915–2925. doi: 10.1016/j.energy.2005.11.005
Keywords: compression ignition diesel engines, dual fuel engines, natural gas, greenhouse gas emissions, particulate matter
Citation: Boretti A (2019) Advantages and Disadvantages of Diesel Single and Dual-Fuel Engines. Front. Mech. Eng. 5:64. doi: 10.3389/fmech.2019.00064
Received: 27 August 2019; Accepted: 14 November 2019;
Published: 03 December 2019.
Edited by:
Hongsheng Guo, National Research Council Canada (NRC-CNRC), CanadaReviewed by:
Stuart Neill, National Research Council Canada (NRC-CNRC), CanadaLorenzo Bartolucci, University of Rome Tor Vergata, Italy
Copyright © 2019 Boretti. This is an open-access article distributed under the terms of the Creative Commons Attribution License (CC BY). The use, distribution or reproduction in other forums is permitted, provided the original author(s) and the copyright owner(s) are credited and that the original publication in this journal is cited, in accordance with accepted academic practice. No use, distribution or reproduction is permitted which does not comply with these terms.
*Correspondence: Alberto Boretti, YS5hLmJvcmV0dGlAZ21haWwuY29t