- 1Biomedical Engineering, University of Calgary, Calgary, AB, Canada
- 2Libin Cardiovascular Institute, University of Calgary, Calgary, AB, Canada
- 3Biological Sciences, University of Calgary, Calgary, AB, Canada
- 4Department of Physiology and Pharmacology, Cumming School of Medicine, University of Calgary, Calgary, AB, Canada
- 5Department of Radiology and Bioengineering, University of Colorado, Anschutz Medical Campus, Aurora, CO, United States
- 6Division of Surgery—Cardiac Surgery, Bluhm Cardiovascular Institute, Northwestern University, Chicago, IL, United States
- 7Department of Radiology and Bioengineering, Northwestern University, Chicago, IL, United States
- 8Department of Cardiac Sciences, University of Calgary, Calgary, AB, Canada
Collagen has an essential role in aortic biomechanics, and collagen remodeling has been associated with the development and progression of aortic aneurysm. However, the exact mechanisms behind collagen remodeling and the biomechanical implications are not well understood. This study presents an investigation of the relationship between collagen remodeling in the aortic wall and biomechanics, by means of collagen assays, smooth muscle cell gene expression, and mechanical testing on human aortic specimens collected from patients with bicuspid aortic valve. Collagen assay analysis was employed to determine collagen-I and total collagen content; quantitative real-time PCR was used to determine amounts COL1A1 and COL3A1 expression in the tissue. These parameters were compared with the local biomechanical properties determined from biaxial and uniaxial tensile testing. Collagen-I content was found to relate to improved mechanical properties, while total collagen content did not exhibit a relationship with biomechanics. COL1A1 and COL3A1 expression were found to relate to the collagen-I content of the tissue, but not the total collagen content or biomechanical performance. Relationships between variables appeared to be dependent on the collagen content in specific layers of the aortic wall. The effect of age is also noted, as total collagen content and biomechanics were found to have significant associations with increasing age, while collagen-I content and collagen gene expression did not exhibit any correlation. Varying relationships were observed when looking at younger versus older patients. Findings highlight the importance of type and location in determining the influence of collagen on aortic biomechanics, as well as the role of gene expression in the onset and progression of collagen remodeling in aortic aneurysm.
1 Introduction
Bicuspid aortic valve (BAV) patients make up 1%–2% of the population and have a higher likelihood of developing ascending aortic aneurysm (AsAA) than the general population, a serious disease characterized by local weakening and dilation of the wall of the ascending aorta (Fedak et al., 2002). The aortic wall is composed of three layers: the intima, media, and adventitia. With the intima being only a few microns thick, it is thought that aortic biomechanics are primarily determined by the microarchitecture and content of the extracellular matrix (ECM) of the media with respect to daily cardiovascular functioning, with the adventitia providing support for high impact loading (Wolinsky and Glagov, 1967; Robertson et al., 2013). The media is composed of equally spaced elastic lamellae with smooth muscle cells and collagen interspaced between the layers. The adventitia is made of thick bundles of collagen (Rhodin, 2014). In healthy tissue, elastin is thought to dominate aortic tissue behaviour in normal pressure conditions, working in conjunction with contractile phenotype vascular smooth muscle cells (VSMCs) to allow the aorta to undergo the large deformations necessary for cardiovascular functioning. The collagen remains crimped or wavy, not contributing to the biomechanics until a sufficient deformation is reached, often assumed to be outside the physiological level of daily functioning.
The pathogenesis of AsAA is widely associated with ECM remodeling. As such, disruptions of the ECM in relation to AsAA have been of interest. Studies have outlined abnormal ECM remodeling in BAV AsAA, citing impaired collagen turnover (Wågsäter et al., 2013), elastin fragmentation and medial degeneration (Guzzardi et al., 2015; Halushka et al., 2016; Cotrufo et al., 2005), and VSMC phenotypic switching and death (Lu et al., 2021; Cotrufo et al., 2005) as possible factors in aneurysm progression.
Collagen is of particular interest as it is known to be heavily involved in ECM remodeling related to both aging and disease. Unlike elastin, which cannot be produced by the body after developmental years, collagen repair and regeneration in the aortic ECM occurs throughout a person’s life (Wang et al., 2021), and these processes may occur at an accelerated rate to compensate for elastin fragmentation and reduction. As collagen is intimately related to the structural integrity and biomechanical performance of the aorta, there have been several studies on its role in relation to AsAA development and progression. Results show that changes in collagen content, crosslinking, and type are found to occur in AsAA tissue (Tsamis et al., 2013), and the extent of these changes may relate to the probability of serious outcomes such as aortic dissection or rupture (Jana et al., 2019). Increased collagen in the media has been established as a sign of disease progression alongside fragmented elastin (Rizzo et al., 1989; Halushka et al., 2016) and previous studies from our group have shown that the presence of increased collagen in the media may correlate with a decrease in tissue strength (Martufi et al., 2018).
While it is evident that collagen plays an important role in tissue remodeling and disease progression, the nature of this role and the influence from various types of collagen remains unclear. Many different types of collagen structure exist in soft tissue, the two predominant types in aortic tissue being collagen-I and collagen-III (Jana et al., 2019). Collagen-I is thought to be associated with tensile stiffness and strength, while collagen-III is thought to be associated with extensibility. AsAAs are also often characterized by the loss of VSMCs or changes in VSMC phenotype from contractile to synthetic (Owens et al., 2004). The synthetic VSMC phenotype is associated with tissue remodeling processes, including collagen production (Owens et al., 2004). Specifically, synthetic VSMCs are known to secrete increased RNA gene expressions COL1A1 and COL3A1, which are thought to be associated with the production of collagen-I and III, respectively. Previous studies have shown that a decrease in COL1A1 expression led to a reduction in collagen-I content, which was associated with aortic dissection and rupture in mouse models (Rahkonen et al., 2004).
Multiple studies have sought to understand the process and effects of collagen remodeling and changes in the ECM observed using histology (Smoljkić et al., 2017; Deveja et al., 2018), microscopy (Pasta et al., 2016), and biochemical assays (Pichamuthu et al., 2013; Chim et al., 2020). Though it is likely that the microstructural changes observed in the ECM histopathologically would have biomechanical implications, there are relatively few studies that have investigated the relationship between biomechanical properties and collagen changes in the ECM of aortic aneurysm (Rizzo et al., 1989; Tanios et al., 2015; Forneris et al., 2021), and even fewer that are specific to the ascending aorta and BAV (Pichamuthu et al., 2013; Pasta et al., 2016). Further investigation into the relationship between microstructural and mechanical characteristics of AsAA may provide insight into the complex, multifactorial mechanisms of AsAA development and progression. In this study, we present a preliminary exploration into the relationship between collagen remodeling in BAV AsAA and biomechanics, using a combination of enzyme-linked immunosorbent assay (ELISA) analysis for collagen-I and total collagen content, quantitative reverse transcription PCR (RT-qPCR) analysis for synthetic VSMC gene expression, and biaxial and uniaxial tensile testing for biomechanical characterization of BAV AsAA tissue.
2 Materials and Methods
2.1 Patient Population and Tissue Samples
Tissue specimens were obtained from a population of fifteen BAV patients undergoing elective ascending aortic resection between 2016 and 2020 at the Foothills Medical Centre (Calgary, Alberta, Canada) under the approval of the Conjoint Faculties Research Ethics Board (REB14-0084) and Northwestern Memorial Hospital (Chicago, Illinois, United States) under the approval of the Northwestern University Institutional review board (STU00204434). Inclusion criteria: above 18 years old, had a BAV, and no previous history of aortic surgery, aortic dissection, rupture, or other connective tissue disorders. Demographic data such as age, sex, and hypertension were also collected.
Resected whole aortic aneurysm tissue was cut into regions (greater curvature, lesser curvature, posterior, and anterior), and then further divided into specimens based on region size (Figure 1). Specimens were marked to specify orientation, and then flash frozen at −80°C in VWR tissue freezing compound. Previous studies have indicated that freezing has minimal effects on the biomechanical properties of tissue (Macrae et al., 2016).
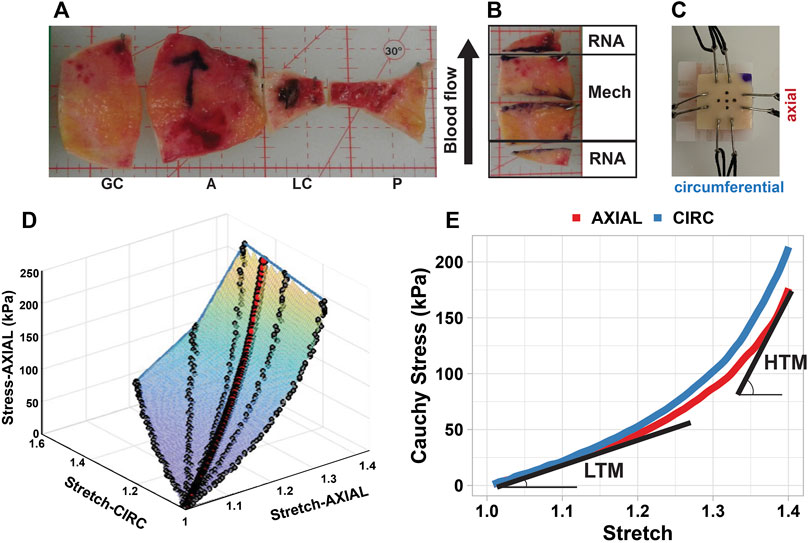
FIGURE 1. Aneurysm specimen processing and biaxial mechanical testing methodology. (A) whole aneurysms were divided into circumferential regions: greater curvature (GC), anterior (A), lesser curvature (LC), and posterior (P); (B) tissue was divided into specimens based on the size of the region, with large pieces used for mechanical testing, and adjacent pieces used for RT-qPCR analysis; (C) hook placement on a square biaxial specimen, showing dot placement used for camera tracking; (D) stress-strain surface obtained from fitting all experimental protocols, with the red dots representing the true equi-stretch curve in the axial direction; (E) stress-stretch curves obtained in axial (red) and circumferential (blue) directions were characterized by the low-strain tangential modulus (LTM) and high-strain tangential modulus (HTM). CIRC, circumferential direction; AXIAL, axial direction.
2.2 Mechanical Testing and Analysis
A combination of biaxial tensile and uniaxial tensile tests were performed on specimens to characterize the tissue response under varied mechanical conditions. For all tests, loading was induced in one or both of the defined principal directions: circumferential (Tsamis et al., 2013), referring to the direction along the circumference of the aorta; and axial (Forneris et al., 2021), referring to the direction along the length of the aorta. From each specimen, one circumferential and one axial test strip was removed for uniaxial testing, with the remainder designated for biaxial tensile testing (Figure 2B).
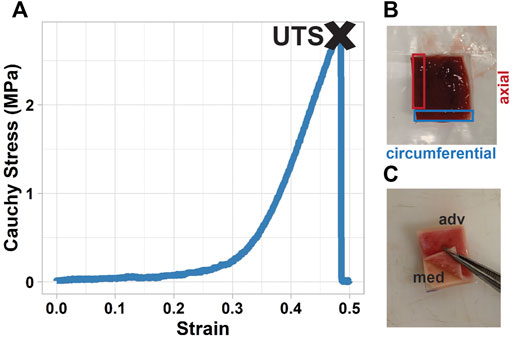
FIGURE 2. Uniaxial analysis and layer-separation of tissue. (A) stress-strain curve of a uniaxial test, with ultimate tensile strength (UTS) found as the stress of the tissue at failure; (B) uniaxial specimens were directly adjacent to the biaxial specimen, cut from the tissue in both the circumferential (blue) and axial (red) directions; (C) manual layer-separation of the tissue was done after biaxial testing, before the tissue was analyzed for collagen content.
2.1.1 Biaxial Tensile Testing
Biaxial tensile tests were conducted using a four-motor biaxial testing system (ElectroForce Systems, TA Instruments, Springfield, MO) equipped with two 22N load cells. Experimental protocol and subsequent analysis has been previously reported by our group (Forneris et al., 2021). Specimens were thawed at 4°C prior to testing, then cut into squares of approximately 12 mm × 12 mm and attached to four linear motors with sutures and hooks. The circumferential and axial specimen directions were oriented with the motor directions and orientation was maintained throughout testing and across specimens. Specimen thickness and hook-to-hook distance (i.e., effective specimen testing length) were measured using digital calipers. Five dots were placed in the center of the specimen to allow for local deformation tracking by an overhead high-resolution digital video extensometer (DVE) camera. To replicate in vivo conditions, the specimen was submerged in a PBS bath warmed to 37°C for the duration of the test (Macrae et al., 2016). A pre-load of 0.05 N in each axis was applied to the specimens before testing.
Each specimen was subjected to a series of displacement-controlled test protocols (1:1, 1:0.75, 1:0.5, 1:0.2, 0.2:1, 0.5:1, 0.75:1), where 1 indicates the maximum displacement in the circumferential or axial direction based on the original hook-to-hook specimen dimensions. All specimens were subjected to a maximum displacement of 60% with a subset of specimens also subjected to an additional 80% maximum displacement set of protocols. Each protocol consisted of five pre-conditioning cycles with the data from the last or second last cycle being used to characterize the passive mechanical behaviour of the specimen.
Cauchy stress and stretch were determined from the average local deformation gradient found via optical dot tracking, the global force readings in each direction, and the initial specimen dimensions, with the assumption of tissue homogeneity and incompressibility (Forneris et al., 2021; Nightingale et al., 2021). If high shear was present, the deformation gradient was calculated excluding the dot corresponding to the region of high shear. As the applied displacements do not always produce equivalent local stretch, a 3-dimensional surface was fitted to the stress-stretch data to find the true equi-stretch biaxial mechanical response for each specimen, allowing for better comparison across the specimens (Figure 1D) (Forneris et al., 2021). This data was further processed for biomechanical parameters to compare behaviour across specimens. Specifically, the equi-biaxial mechanical stress-stretch response was plotted and analyzed in the circumferential and axial directions to determine the low-strain tangential modulus (LTM) and the high-strain tangential modulus (HTM) (Figure 1E).
2.1.2 Uniaxial Tensile Testing
Uniaxial tensile testing was conducted using a linear motor uniaxial testing system (ElectroForce System 3200, TA Instruments, Eden Prairie, MN) equipped with a 200N load cell. Specimens tested uniaxially were cut in axial and circumferential directions (Figure 2B). An initial pre-conditioning protocol was applied in which the specimen was subjected to a set of 10 cycles to 15% strain—based on the initial grip-to-grip measurement—at a frequency of 1 Hz. The specimen was then pulled at a rate of 10 mm/min to failure. Displacement and force values were recorded, which were then processed into Cauchy stress and strain using the initial specimen dimensions. The stress-strain curve for each specimen was generated, and the ultimate tensile strength (UTS) of the specimen was found as the first local maximum stress (Figure 2).
2.3 Collagen Assays
Collagen samples were cut from the bottom 1/3 of the biaxial squares after they were tested. Specimens were separated into media and adventitia by gently rubbing a scalpel on the intimal side of the sample until separation was initiated, and then peeled manually (Figure 2C) (Angouras et al., 2019). The media (including intima) and adventitia layers were then processed independently using the methods described below.
2.3.1 Total Collagen
Total Collagen data was collected using the QuickZyme Total Collagen Assay from QuickZyme Biosciences (QuickZyme, 2022). To homogenize the sample specimens, approximately 10 mg of tissue was taken from each sample and added to a pressure-tight, screw-capped polypropylene vial containing 50 µL of 10N sodium hydroxide (NaOH). The vial was then hydrolyzed at 120 °C for 1 h and cooled on ice. Once the solution reached room temperature it was neutralized by adding 50 µL of 10N hydrochloric acid (HCl) and vortexed. To ensure the solution was homogeneous and pellet insoluble debris that may have been present, the polypropylene vial was centrifuged at 10,000 x g for 5 min 2 µl of the homogenized collagen sample was then diluted in 20 µl of ddH2O to fit within the standard curve. Preparation of collagen standard and subsequent assay procedure was done according to the QuickZyme Total Collagen Assay manual (QuickZyme, 2022).
Sample calculations were done according to the QuickZyme Total Collagen Assay manual after accounting for the 2 µl collagen: 20 µl ddH2O dilution. The calculated concentrations were then converted from µg to µg-collagen/µg-tissue.
2.3.2 Collagen-I
Collagen I data was collected using the Human Pro-Collagen I alpha ELISA Kit (ab210966) from Abcam (Abcam, 2022). To homogenize the sample specimens, approximately 10 mg of tissue was taken from each sample and added to a pressure-tight, screw-capped polypropylene vial containing 1 ml of Phosphate Buffered Saline (PBS). An electric homogenizer was then used at speed 6 for 3 × 30 s intervals to homogenize sample specimens until the solution was cloudy and no distinct particles were seen. Care was taken to avoid homogenizing for more than 90 s. 10 µl of the homogenized collagen sample was then diluted in 90 µl of the 1X cell extraction buffer, prepared according to the ELISA Kit procedure (Abcam, 2022). Preparation of collagen standard and subsequent assay procedure was done according to the Human Pro-Collagen I alpha ELISA Kit (Abcam, 2022).
Sample calculations were done using the concentrations generated respective to the Collagen I standard curve. To determine the mass of collagen (g) in the wells, concentrations were divided by 0.05 ml, the amount of dilute collagen solution added to the wells. This number was then divided by the mass of tissue (g) in well, after accounting for all dilutions, to give a final amount in g collagen/g tissue.
2.4 Quantitative Real-Time PCR
Total RNA was extracted from aortic tissues that had been fast frozen immediately after being obtained from patients using the TRIzol reagent (#15–596–018, Thermo Fisher Scientific) according to the manufacturer’s instructions. The extracted RNA was dissolved in RNase-free water, and the concentration of the total RNA was determined using SpectraMax iD3 (Molecular Devices, CA, United States). The RT-qPCR was performed as described previously (Ballasy et al., 2021). Briefly, cDNA was then prepared using the cDNA Reverse Transcription Kit (#4374966, Thermo Fisher Scientific). TaqMan probes for COL1A1 (Hs.PT.58.15517795), COL3A1 (Hs.PT.58.4249241), and 18s rRNA (Hs.PT.39a.22214856.g) were used to measure the gene expression. For each gene, a standard curve was generated using known concentrations of cDNA (0.1, 1, 10, 100, and 1,000 ng) as a function of cycle threshold (CT). Expression analysis of the reported genes was performed by TaqMan Real-time PCR using QuantStudio™ system (Thermo Fisher Scientific, MA, United States). Data were analyzed using QuantStudio™ design and analysis software version 1.4.3. All samples were run in triplicates in 384 well plates. 18S rRNA was used as an endogenous control.
2.5 Statistical Analysis
Statistical analysis was performed in R Studio (Version 4.04, 2021) (R Core Team, 2021) using the lme4 (Bates et al., 2015) and moments (Komsta and Novomestky, 2015) packages. Statistical significance was set at p < 0.05. A Shapiro-Wilk test was performed to assess the normality of each continuous variable, and in the case of non-normally distributed data, a logarithmic transformation was applied to achieve normality. To determine relationships between mechanical properties and collagen content in the full data set, a linear mixed effect (LME) model was used with random intercepts for patients, used to assess inter-patient variability. LME results are reported with the Marginal R2 (R2m-values), a ratio of the variance explained by the fixed variable as compared to the total variance in the data, and the Conditional R2 (R2c-values), which accounts for the variance explained by the fixed effects and the random effects. In the case of the age-grouped data sets, variables were again assessed for normality, followed by Pearson’s correlation test for normal data and Spearman’s rank correlation test for non-normal data to examine the strength and direction of relationships between variables. Two-tailed p-values are presented with correlation coefficients (R-values for Pearson’s correlation, ρ-values for Spearman’s rank correlation). Variation between groups was assessed using an ANOVA test for normal distributions, and Kruskal-Wallis test for non-normal distributions.
3 Results
3.1 Patient Characteristics
A total of 15 BAV patients were included in this study, with ages ranging from 40 to 68 years, patient demographics presented in Table 1. From these patients, 54 aortic tissue samples were collected and subjected to mechanical testing. Of the mechanical tests, 49 biaxial tensile tests and 63 (35 circumferential, 28 axial) uniaxial tensile tests were performed successfully. Collagen-I analysis was performed on 53 specimens, while total collagen analysis was done on a subset of 36 specimens, dependent on the amount of tissue remaining after collagen-I analysis.
RNA gene expression was conducted on 36 samples from 10 patients, samples were located adjacent to the mechanical and collagen specimens (Figure 1B). It was necessary to use adjacent specimens as the smooth muscle cells do not generally survive the mechanical testing.
3.2 Regional Differences
There was no significant difference in collagen-I or total collagen content between the anterior, posterior, greater curvature, and lesser curvature regions. Axial LTM and HTM were the only mechanical parameters to show regional variation, with the axial LTM of the anterior region significantly lower than that of the posterior (p = 0.028) and greater curvature regions (p = 0.028), and the axial HTM of the anterior region significantly lower than that of the greater curvature region (p = 0.0044).
3.3 Effect of Age
As previous findings from our group suggest a relationship between ECM remodeling effects and age (Nightingale et al., 2022), collagen content, RNA expression, and biomechanical parameters were assessed for age-related changes. It was found that while total collagen content had a significant positive correlation with age in both the media (p = 0.011, R = 0.50) and adventitia (p = 0.017, R = 0.47), collagen-I content did not show any relationship with patient age (Figure 3). The ratio of collagen-I to total collagen, however, did have a significant negative association with age in the adventitia (p < 0.001, ρ = −0.58) and not the media.
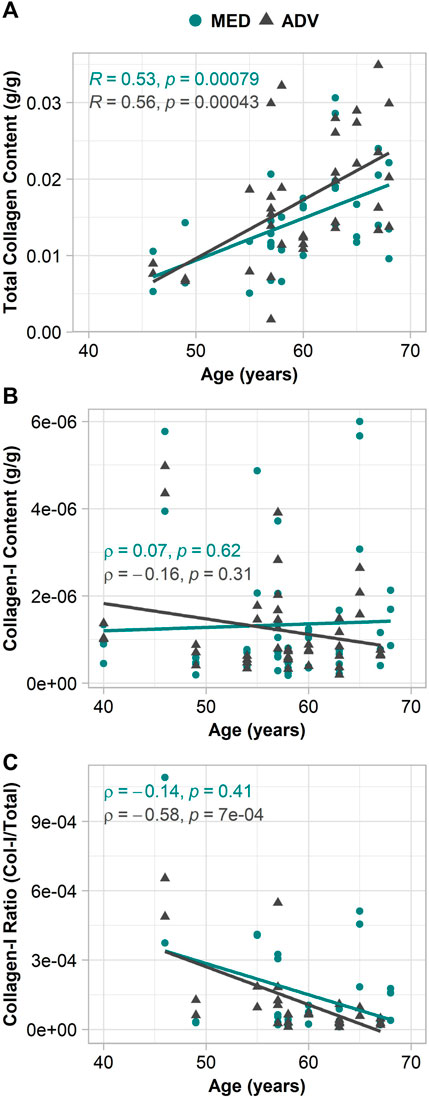
FIGURE 3. Relationship of collagen in the adventitia and media with patient age. (A) total collagen content; (B) collagen-I content; (C) collagen-I ratio.
RNA expression of COL1A1 and COL3A1 did not have any significant relationship with age, though the oldest patients did seem to have the highest levels of expression for both.
For biomechanics, increasing age was also found to correlate with the circumferential properties. Specifically, an increase in the circumferential LTM (p = 0.034, ρ = 0.30) and HTM (p = 0.032, ρ = 0.34), as well as a decrease in the circumferential UTS (p < 0.001, ρ = -0.55). Axial LTM, HTM, and UTS were not found to have any relationship with age.
Given the association of total collagen content and mechanical properties with age, a second set of analysis was completed with patients categorized into younger (age <60 years at time of aortic resection, N = 9) and older (age ≥60 years at time of aortic resection, N = 6) patient groups, to observe any age-specific remodeling characteristics. The age division was chosen based on previous work by Haskett et al. (2010) and Martin et al. (2013) who suggest that 60 years marks a significant transition in the in vivo and ex vivo mechanical properties of the ascending aorta. 60 years is also the approximate onset age for thoracic aortic aneurysm in the general population (Davies et al., 2006).
Descriptive statistics for collagen content and biomechanics are presented for the young (<60 years) and old (≥60 years) age group in Table 2, with comparative p-values. Comparison between the mechanical properties of the younger and older age groups revealed that older patients demonstrated stiffer behaviour at both low- and high-strain conditions in the circumferential direction, as well as significantly lower circumferential strength. No differences were realized between age groups in the axial mechanics. Like the findings above, older patients were found to have a higher total collagen content in the media, though no difference was found in the collagen-I content between the age groups. Despite the differences in total collagen content, there did not seem to be any significant difference in the level of COL1A1 or COL3A1 expression between the older and younger groups.
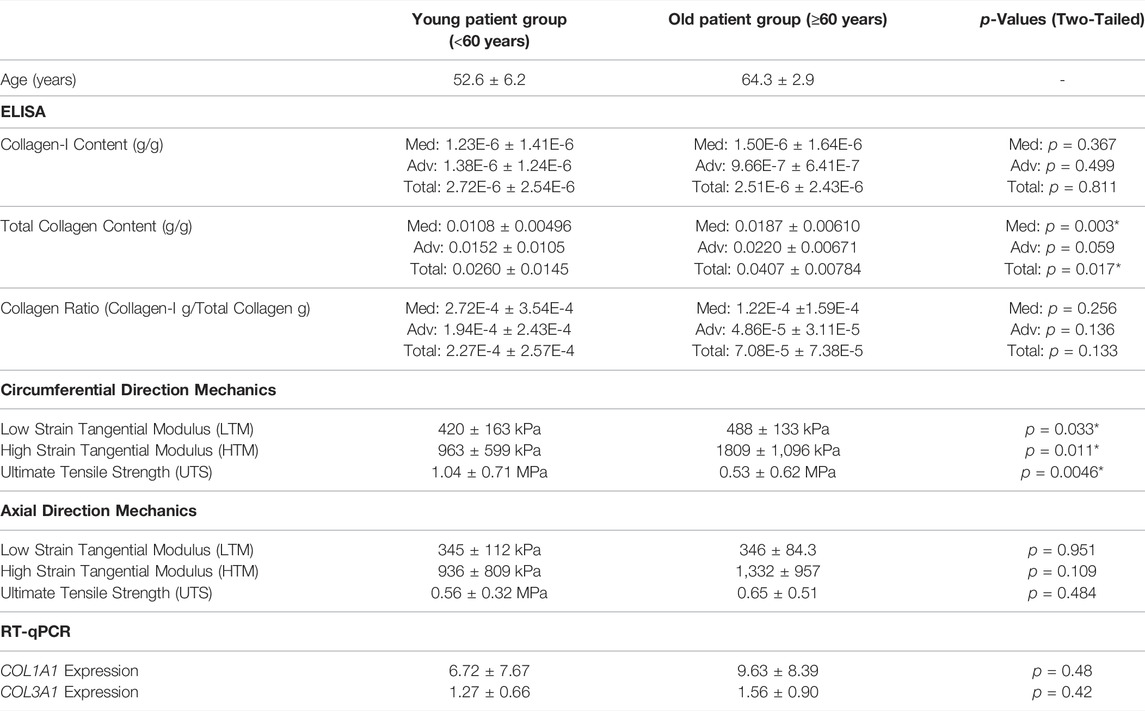
TABLE 2. Comparison of the collagen-I content, total collagen content, and mechanical properties between young (<60) and old (≥60) patient groups.
3.4 Collagen Content and Biomechanics
In the full patient group, the tissue biomechanics were not found to have any association with total collagen content in the media or adventitia. On the other hand, Collagen-I content, did appear to relate to the biomechanics. Specifically, in the full patient group, circumferential UTS was found to have a positive relationship with medial collagen-I content (p = 0.039, R2m = 0.13, R2c = 0.51) and medial collagen-I ratio (p = 0.0079, R2m = 0.23, R2c = 0.68). No significant relationships were found between collagen-I content or ratio in the adventitia and the mechanical properties of the full group.
When divided into groups by age, older patients seemed to have an inverse relationship between collagen-I ratio in the adventitia and LTM in both the circumferential (Figure 4) (p = 0.0055, ρ = -0.69) and axial (p = 0.031, ρ = -0.56) directions. This relationship was also present when considering collagen-I content in the adventitia, however the correlation was less strong (circ: p = 0.02, ρ = -0.58; axial: p = 0.062, ρ = -0.48). No association was found between biomechanics of the older patients and total collagen in the media and adventitia, or with collagen-I in the media.
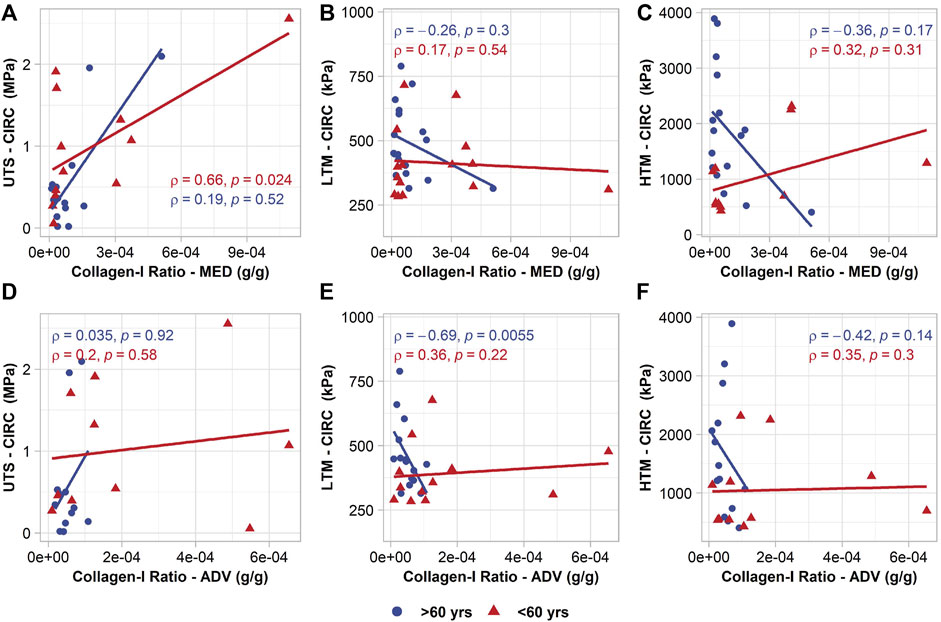
FIGURE 4. Age-group specific relationships between circumferential mechanical properties and collagen-I ratio in the media (top row) and collagen-I ratio in the adventitia (bottom row). (A,D) circumferential UTS; (B,E) circumferential LTM; (C,F) circumferential HTM.
In the younger patients, circumferential UTS was found to have a positive relationship with collagen-I ratio in the media (p = 0.024, ρ = 0.66) (Figure 4), but not with collagen-I content alone. It should be noted that UTS was defined as the maximum stress before the failure of any part or layer of the tissue, versus the entire separation of the tissue. The media layer was often observed to fail first, therefore it is reasonable that the UTS would be related to variations in collagen within that layer. No other significant associations were realized with collagen-I content or ratio. As in the older patients, total collagen content in the media and adventitia did not seem to correlate with biomechanics.
3.5 RT-qPCR Analysis
RT-qPCR analysis was performed on a subset of ten patients (N = 7 < 60 years, N = 3 ≥ 60 years) based on availability of tissue adjacent to mechanically tested specimens.
No relationships were realized between the biomechanical properties and COL1A1 expression. In fact, the only statistically significant relationship between RNA gene expression and biomechanical properties was found in the older patient group, where circumferential HTM had a negative association with COL3A1 expression (p = 0.042, R = -0.83).
COL1A1 and COL3A1 expression did, however, appear to relate to the collagen-I content and total collagen content in the tissue. Specifically, older patients displayed a positive association of collagen-I content in the media with COL1A1 (p = 0.044, ρ = 0.66) and COL3A1 (p = 0.031, ρ = 0.70) (Figure 5). In the adventitia, collagen-I content did not relate to gene expression, but total collagen content was found to relate to both gene expressions (COL1A1: p = 0.027, ρ = 0.73; COL3A1: p = 0.0021, R = 0.87).
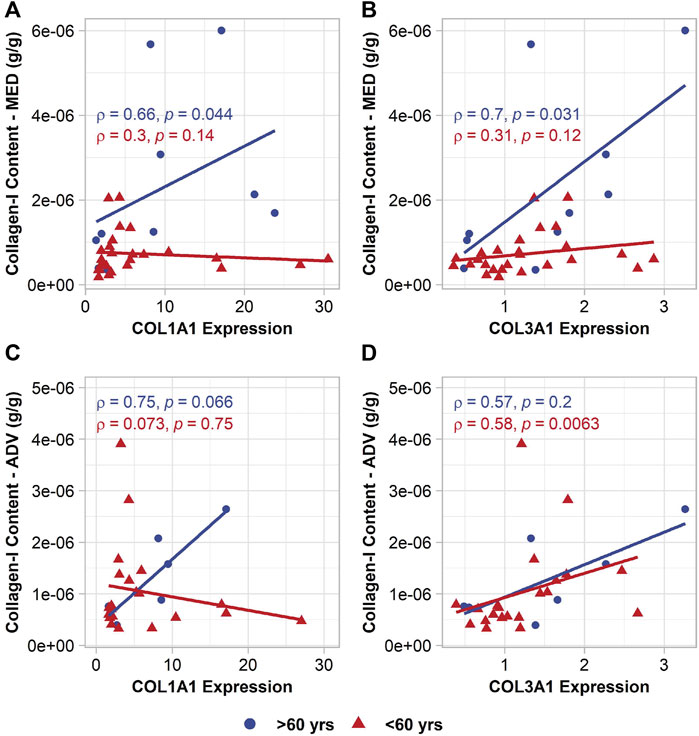
FIGURE 5. Age-group specific relationships between VSMC collagen gene expression and collagen-I content in the media (top row) and collagen-I content in the adventitia (bottom row). (A,C) COL1A1 expression; (B,D) COL3A1 expression.
In the young patients and full group, no relationship was observed between COL1A1 and collagen content. COL3A1, on the other hand, did seem to relate to the collagen-I content in the younger patients in the adventitia (p = 0.0063, ρ = 0.58) (Figure 5).
4 Discussion
ECM remodeling is associated with the onset and progression of aortic aneurysm, including AsAA (Jana et al., 2019; Mimler et al., 2019; Adams et al., 2021). Collagen is a principal component of the ECM in the aortic wall, and it interacts with other ECM components such as elastin or fibrillin. The appropriate amounts of each of these ECM proteins ensure arterial integrity necessary to support the heavy hemodynamic loading conditions in the aorta (Wang et al., 2006). Although elastin fragmentation and degradation have been implicated in AsAA (Nienaber and Eagle, 2003; Tsamis et al., 2013), the role of collagen is less clear. Collagen content in the aortic wall has been extensively studied, with numerous conflicting reports (Jana et al., 2019; Mimler et al., 2019). Increased (Rizzo et al., 1989; Bode et al., 2000) or decreased (Rahkonen et al., 2004) collagen levels have been associated with aortic wall weakening and aneurysm formation, and in AsAA specifically, changes in the ratio of collagen types present in the tissue have been reported (Jana et al., 2019; Cotrufo et al., 2005).
Collagen in the aorta is generally associated with structural function, responsible for mechanical performance at high deformations and stress due to its high tensile strength and stiffness (Holzapfel and Fratzl, 2008). However, our results agree with previous findings (Pichamuthu et al., 2013) that show no significant relationship between the total collagen content of the tissue and the biomechanical behaviour, suggesting that the role of collagen in AsAA tissue may vary considerably based on collagen type and microstructure. Collagen location within the tissue may also be a significant factor, as most of the relationships observed in collagen content were layer specific. Circumferential UTS appeared to be related to the collagen-I content of the media, but not the adventitia, while circumferential LTM seemed to be related to the collagen-I content of the adventitia, but not the media.
The study of collagen remodeling in aortic aneurysm also seems to be complicated by changes in the ECM that occur naturally with age. BAV patients are known to present with AsAA earlier than the general population, which makes it challenging to separate age-dependent ECM remodeling from disease-driven remodeling. In this study, the only parameters that appeared independent of age were collagen-I content and COL1A1 and COL3A1 gene expressions. Aortic aneurysm onset and progression are known to be accompanied by VSMC phenotypic switching, VSMC apoptosis, and medial degeneration (López-Candales et al., 1997; Zhong et al., 2019). The presence of synthetic VSMCs is characterized by increased production of ECM components, such as collagen-I and collagen-III (Petsophonsakul et al., 2019), and increased collagen-I and III content and expression have been previously reported in aortic aneurysms (Phillippi et al., 2010; Jana et al., 2019). Because collagen-I content and gene expression appear to have no association with age, it is possible they are related to disease-specific remodeling in the aortic wall. Interestingly, total collagen seems to significantly increase with age (as previously reported (Andreotti et al., 1985; Halme et al., 1985; Tsamis et al., 2013)) while collagen-I ratio decreases, suggesting other types of collagens are being produced. The biomechanical properties also show potential aging effects, with increased tissue stiffness at low- and high-strains, and decreased tissue strength in older patients.
When separated into age groups, relationships between the biomechanics and collagen content were found to differ between the old and young patients, with LTM and UTS relating to the content and ratio of collagen-I in the tissue. Given that there was no significant difference between the collagen-I content of the age groups, it stands to reason that these age-specific relationships may arise from changes in other constituents present in the ECM. Specifically, the increased influence of collagen-I on low-strain stiffness in the older age group may be related to the degradation of elastin with age. The aortic wall does not deform to the same level in vivo as is achieved during typical ex vivo mechanical testing protocols (60% or 80% sample strain). In healthy tissue, low-strain measurements, such as LTM, are generally more representative of physiological conditions, with elastin thought to be the primary load-bearing component. As elastin content decreases due to aging or disease progression, if the collagen remains crimped, the low-strain stiffness should decrease. In the case of the low-strain stiffness in the older patients, there is a significant inverse relationship between the LTM and collagen-I ratio, and it is possible that this result indicates a loss of elastin in the tissue and a switch to more collagen-dependent behaviour. With the older patients having significantly greater low-strain stiffness than the young, the inverse relationship between LTM and collagen-I in the old group suggests that the stiffening behaviour may be related to the contributions of other collagen types. This switch to collagen-dominated behaviour may also be the reason why older patients appear to have a stronger relationship between VSMC synthetic phenotype COL1A1 and COL3A1 expressions, which signal for increased collagen deposition. Whether associated with aging, disease, or both, degradation of elastin in the aortic wall has direct implications for the wall integrity and behaviour (Choudhury et al., 2009; Chow et al., 2014; Nightingale et al., 2022), and increased collagen deposition may mitigate some of the undesirable biomechanical changes. While the exact cause and mechanism behind VSMC phenotype switching is currently unknown, we hypothesize that the increased expression of COL1A1 and COL3A1 may be a consequence of compensatory repair mechanisms mediated by synthetic VSMCs in the aortic wall.
While the low-strain stiffness may be indicative of tissue behaviour in vivo, it is also interesting to note the relationship of collagen content and UTS. UTS is often used in biomechanical studies as a pseudo-measure of rupture potential, as it offers a means of comparing tissue strength at failure. The results show significant associations between circumferential UTS and medial collagen-I, as well as circumferential UTS and the medial collagen-I ratio in the full group and young patients. These results imply that the presence of collagen-I in the media may be a significant factor in aortic wall failure mechanics, more so than in the adventitia or composite tissue. As results show greatest significance in the relationship between circumferential UTS and the ratio of collagen-I content to total collagen, it highlights the question of what contribution other collagen types may have to AsAA biomechanics. In the human ascending aorta, collagen-I, III, and IV account for the majority of the total collagen present in the tissue (Berillis, 2013). A reduction in the collagen-I ratio in the tissue implies an increase in other types of collagens present. In BAV patients specifically, an increase in collagen-IV and decrease in collagen-I and III has been reported in AsAA tissue (Tsamis et al., 2013), though the relationship to biomechanics was not evaluated. Considering the positive relationship of collagen-I ratio and UTS, it may be that other collagen types are not as beneficial to the mechanical properties.
In the context of aortic aneurysms and disease progression, this is an important finding. It is generally accepted that collagen is a primary load-bearing constituent of the aortic wall. However, as detailed above, several studies investigating the relationship between collagen content and biomechanics in the aorta have found that variation in total collagen content does not seem to relate to the mechanical behaviour. As such, subsequent studies concerning collagen remodeling in the aortic wall have generally focused on the orientation and microarchitecture of the fibres to explain variation in biomechanical properties (Gasser et al., 2006; Pasta et al., 2016; Tsamis et al., 2016). Several groups have proposed that the reduced biomechanical properties of BAV AsAA tissue may be explained by differences in the collagen fibre alignment and distribution (Pichamuthu et al., 2013; Pasta et al., 2016). While these conclusions may be true, it is also accepted that different collagen types do not have the same mechanical response under loading (Silver et al., 2002). To have varying ratios of collagen types in the tissue would likely have direct implications for biomechanical properties, as was found in this study with the ratio of collagen-I. We suggest that the biomechanical contributions of collagen and the significance of collagen remodeling in AsAA cannot be reduced to simply the collagen content or orientation, but rather are a complex product of multiple factors, including collagen architecture, type, and location in the tissue.
As an exploratory study, the data presented may be better used to guide future research than to draw any concrete conclusions. This study has limitations, such as the lack of collagen-III content to be associated with COL3A1 gene expression and biomechanical parameters, making it challenging to interpret some of the relationships found without making assumptions. Additionally, the patient cohort contains only one female subject, and so differences in composition and biomechanics that may be sex-specific cannot be confidently evaluated. Ideally, this work should be extended to a larger group, including greater patient representations from both sexes across a range of ages, if possible. Further, methodology should be extended to include more collagen types, such as collagen-III, IV, and collagen crosslinking. Additionally, it would be beneficial to consider the relationship between collagen remodeling and changes in other constituents in the ECM that may occur simultaneously, such as elastin fragmentation and presence of matrix metalloproteinases, for a more comprehensive understanding of ECM remodeling in BAV AsAA.
The present findings are the first to show a potential relationship between collagen-I content, collagen gene expression, and biomechanics in BAV AsAA. The data shows an influence of collagen-I on mechanical behaviour and it seems that increased amounts of collagen-I relative to other collagens in the aortic wall may have a beneficial effect on the wall biomechanics in all ages. Presence of synthetic VSMCs and the increased expression of COL1A1 and COL3A1 genes were found to relate to collagen-I content in older patients and may indicate the onset of favorable collagen repair and regeneration in the ECM as a response to degrading mechanical properties or loss of other ECM components. Consequently, the results emphasize the importance of compensatory collagens in maintaining the structural integrity and stability of the aortic wall by intravascular collagen deposition. The future intent will be to investigate layer-specific collagen-I and III content and expression, and its correlation with layer-specific biomechanical properties.
Data Availability Statement
The original contributions presented in the study are included in the article/Supplementary Materials, further inquiries can be directed to the corresponding author.
Ethics Statement
The studies involving human participants were reviewed and approved by the Conjoint Faculties Research Ethics Board of the University of Calgary (Alberta, Canada) and the Northwestern University Institutional review board (Chicago, Illinois, United States). The patients/participants provided their written informed consent to participate in this study.
Author Contributions
Study conception and design: ED. Patient enrolment: SM, PM, PF, MM. Tissue samples collection: AB, SM, PM, and PF. Mechanical testing: LN and MN. Collagen assay analysis: MT. RNA analysis: AJ and VP. Statistical analysis and interpretation: LN and MN. Manuscript writing and preparation: LN, MN, AJ, and MT. Manuscript critical revision and approval: LN, MN, MT, AJ, VP, ED, AB, SM, PM, MM, and PF.
Funding
This work was supported by National Institutes of Health grant (NIH HL133504 AJB, ESDM, PWMF) and NSERC Discovery Programme (ESDM).
Conflict of Interest
The authors declare that the research was conducted in the absence of any commercial or financial relationships that could be construed as a potential conflict of interest.
Publisher’s Note
All claims expressed in this article are solely those of the authors and do not necessarily represent those of their affiliated organizations, or those of the publisher, the editors and the reviewers. Any product that may be evaluated in this article, or claim that may be made by its manufacturer, is not guaranteed or endorsed by the publisher.
Acknowledgments
The Authors gratefully acknowledge the contribution of Dr. Taisiya Sigaeva to the surface analysis of biaxial data.
References
Abcam (2022). Human Pro-collagen I Alpha 1 ELISA Kit (Ab210966). Available at: https://www.abcam.com/human-pro-collagen-i-alpha-1-elisa-kit-ab210966.html (Accessed: February 09, 2022).
Adams, L., Brangsch, J., Hamm, B., Makowski, M. R., and Keller, S. (2021). Targeting the Extracellular Matrix in Abdominal Aortic Aneurysms Using Molecular Imaging Insights. Int. J. Mol. Sci. 22 (5), 2685. doi:10.3390/ijms22052685
Andreotti, L., Bussotti, A., Cammelli, D., di Giovine, F., Sampognaro, S., Sterrantino, G., et al. (1985). Aortic Connective Tissue in Ageing-A Biochemical Study. Angiology 36 (12), 872–879. doi:10.1177/000331978503601206
Angouras, D. C., Kritharis, E. P., and Sokolis, D. P. (2019). Regional Distribution of Delamination Strength in Ascending Thoracic Aortic Aneurysms. J. Mech. Behav. Biomed. Mater. 98, 58–70. doi:10.1016/j.jmbbm.2019.06.001
Ballasy, N. N., Jadli, A. S., Edalat, P., Kang, S., Fatehi Hassanabad, A., Gomes, K. P., et al. (2021). Potential Role of Epicardial Adipose Tissue in Coronary Artery Endothelial Cell Dysfunction in Type 2 Diabetes. FASEB J. 35 (10), e21878. doi:10.1096/fj.202100684rr
Bates, D., Mächler, M., Bolker, B., and Walker, S. (2015). Fitting Linear Mixed-Effects Models Using Lme4. J. Stat. Softw. 67, 1–48. doi:10.18637/jss.v067.i01
Berillis, P. (2013). The Role of Collagen in the Aorta's Structure. Open Circ. Vasc. J. 6 (1), 1–8. doi:10.2174/1877382601306010001
Bode, M. K., Soini, Y., Melkko, J., Satta, J., Risteli, L., and Risteli, J. (2000). Increased Amount of Type III pN-Collagen in Human Abdominal Aortic Aneurysms: Evidence for Impaired Type III Collagen Fibrillogenesis. J. Vasc. Surg. 32 (6), 1201–1207. doi:10.1067/mva.2000.109743
Chim, Y. H., Davies, H. A., Mason, D., Nawaytou, O., Field, M., Madine, J., et al. (2020). Bicuspid Valve Aortopathy is Associated with Distinct Patterns of Matrix Degradation. J. Thorac. Cardiovasc. Surg. 160 (6), e239–e257. doi:10.1016/j.jtcvs.2019.08.094
Choudhury, N., Bouchot, O., Rouleau, L., Tremblay, D., Cartier, R., Butany, J., et al. (2009). Local Mechanical and Structural Properties of Healthy and Diseased Human Ascending Aorta Tissue. Cardiovasc. Pathol. 18 (2), 83–91. doi:10.1016/j.carpath.2008.01.001
Chow, M.-J., Turcotte, R., Lin, C. P., and Zhang, Y. (2014). Arterial Extracellular Matrix: A Mechanobiological Study of the Contributions and Interactions of Elastin and Collagen. Biophys. J. 106 (12), 2684–2692. doi:10.1016/j.bpj.2014.05.014
Cotrufo, M., Della Corte, A., De Santo, L. S, Quarto, C., De Feo, M., Romano, G., et al. (2005). Different Patterns of Extracellular Matrix Protein Expression in the Convexity and the Concavity of the Dilated Aorta with Bicuspid Aortic Valve: Preliminary Results. J. Thorac. Cardiovasc. Surg. 130 (2), 504–11.
Davies, R. R., Gallo, A., Coady, M. A., Tellides, G., Botta, D. M., Burke, B., et al. (2006). Novel Measurement of Relative Aortic Size Predicts Rupture of Thoracic Aortic Aneurysms. Ann. Thorac. Surg. 81 (1), 169–177. doi:10.1016/j.athoracsur.2005.06.026
Deveja, R. P., Iliopoulos, D. C., Kritharis, E. P., Angouras, D. C., Sfyris, D., Papadodima, S. A., et al. (2018). Effect of Aneurysm and Bicuspid Aortic Valve on Layer-specific Ascending Aorta Mechanics. Ann. Thorac. Surg. 106 (6), 1692–1701. doi:10.1016/j.athoracsur.2018.05.071
Fedak, P. W. M., Verma, S., David, T. E., Leask, R. L., Weisel, R. D., and Butany, J. (2002). Clinical and Pathophysiological Implications of a Bicuspid Aortic Valve. Circulation 106 (8), 900–904. doi:10.1161/01.cir.0000027905.26586.e8
Forneris, A., Nightingale, M., Ismaguilova, A., Sigaeva, T., Neave, L., Bromley, A., et al. (2021). Heterogeneity of Ex Vivo and In Vivo Properties along the Length of the Abdominal Aortic Aneurysm. Appl. Sci. 11 (8), 3485. doi:10.3390/app11083485
Gasser, T. C., Ogden, R. W., and Holzapfel, G. A. (2006). Hyperelastic Modelling of Arterial Layers with Distributed Collagen Fibre Orientations. J. R. Soc. Interface. 3 (6), 15–35. doi:10.1098/rsif.2005.0073
Guzzardi, D. G., Barker, A. J., van Ooij, P., Malaisrie, S. C., Puthumana, J. J., Belke, D. D., et al. (2015). Valve-Related Hemodynamics Mediate Human Bicuspid Aortopathy: Insights from Wall Shear Stress Mapping. J. Am. Coll. Cardiol. 66 (8), 892–900. doi:10.1016/j.jacc.2015.06.1310
Halme, T., Savunen, T., Aho, H., Vihersaari, T., and Penttinen, R. (1985). Elastin and Collagen in the Aortic Wall: Changes in the Marfan Syndrome and Annuloaortic Ectasia. Exp. Mol. Pathol. 43 (1), 1–12. doi:10.1016/0014-4800(85)90050-4
Halushka, M. K., Angelini, A., Bartoloni, G., Basso, C., Batoroeva, L., Bruneval, P., et al. (2016). Consensus Statement on Surgical Pathology of the Aorta from the Society for Cardiovascular Pathology and the Association for European Cardiovascular Pathology: II. Noninflammatory Degenerative Diseases - Nomenclature and Diagnostic Criteria. Cardiovasc. Pathol. 25 (3), 247–257. doi:10.1016/j.carpath.2016.03.002
Haskett, D., Johnson, G., Zhou, A., Utzinger, U., and Vande Geest, J. (2010). Microstructural and Biomechanical Alterations of the Human Aorta as a Function of Age and Location. Biomech. Model Mechanobiol. 9 (6), 725–736. doi:10.1007/s10237-010-0209-7
Holzapfel, G. A. (2008). “Collagen in Arterial Walls: Biomechanical Aspects,” in Collagen Structure and Mechanics. Editor P. Fratzl (New York, NY: Springer), 285–319.
Jana, S., Hu, M., Shen, M., and Kassiri, Z. (2019). Extracellular Matrix, Regional Heterogeneity of the Aorta, and Aortic Aneurysm. Exp. Mol. Med. 51 (12), 1–15. doi:10.1038/s12276-019-0286-3
Komsta, L., and Novomestky, F. (2015). Moments: Moments, Cumulants, Skewness, Kurtosis and Related Tests. Available at: https://CRAN.R-project.org/package=moments (Accessed: February 06, 2022).
López-Candales, A., Holmes, D. R., Liao, S., Scott, M. J., Wickline, S. A., and Thompson, R. W. (1997). Decreased Vascular Smooth Muscle Cell Density in Medial Degeneration of Human Abdominal Aortic Aneurysms. Am. J. Pathol. 150 (3), 993–1007.
Lu, H., Du, W., Ren, L., Hamblin, M. H., Becker, R. C., Chen, Y. E., et al. (2021). Vascular Smooth Muscle Cells in Aortic Aneurysm: From Genetics to Mechanisms. J. Am. Heart Assoc. 10 (24), e023601. doi:10.1161/JAHA.121.023601
Macrae, R. A., Miller, K., and Doyle, B. J. (2016). Methods in Mechanical Testing of Arterial Tissue: A Review. Strain 52 (5), 380–399. doi:10.1111/str.12183
Martin, C., Sun, W., Primiano, C., McKay, R., and Elefteriades, J. (2013). Age-Dependent Ascending Aorta Mechanics Assessed through Multiphase CT. Ann. Biomed. Eng. 41 (12), 2565–2574. doi:10.1007/s10439-013-0856-9
Martufi, G., Forneris, A., Nobakht, S., Rinker, K. D., Moore, R. D., and Di Martino, E. S. (2018). Case Study: Intra-patient Heterogeneity of Aneurysmal Tissue Properties. Front. Cardiovasc Med. 5, 82. doi:10.3389/fcvm.2018.00082
Mimler, T., Nebert, C., Eichmair, E., Winter, B., Aschacher, T., Stelzmueller, M.-E., et al. (2019). Extracellular Matrix in Ascending Aortic Aneurysms and Dissections - What We Learn from Decellularization and Scanning Electron Microscopy. PLOS ONE 14 (3), e0213794. doi:10.1371/journal.pone.0213794
Nienaber, C. A., and Eagle, K. A. (2003). Aortic Dissection: New Frontiers in Diagnosis and Management. Circulation 108 (5), 628–635. doi:10.1161/01.cir.0000087009.16755.e4
Nightingale, M., Gregory, A., Sigaeva, T., Dobson, G. M., Fedak, P. W. M., Appoo, J. J., et al. (2021). Biomechanics in Ascending Aortic Aneurysms Correlate with Tissue Composition and Strength. JTCVS Open 9, 1–10. doi:10.1016/j.xjon.2021.12.001
Nightingale, M., Guzzardi, D. G., Barker, A. J., Malaisrie, S. C., McCarthy, P. M., Markl, M., et al. (2022). Elastin Integrity in the Bicuspid Aortic Wall Is Associated with Mechanical Properties and Influenced by Age. Annals of Cardiothoracic Surgery. [IN PRESS].
Owens, G. K., Kumar, M. S., and Wamhoff, B. R. (2004). Molecular Regulation of Vascular Smooth Muscle Cell Differentiation in Development and Disease. Physiol. Rev. 84 (3), 767–801. doi:10.1152/physrev.00041.2003
Pasta, S., Phillippi, J. A., Tsamis, A., D'Amore, A., Raffa, G. M., Pilato, M., et al. (2016). Constitutive Modeling of Ascending Thoracic Aortic Aneurysms Using Microstructural Parameters. Med. Eng. Phys. 38 (2), 121–130. doi:10.1016/j.medengphy.2015.11.001
Petsophonsakul, P., Furmanik, M., Forsythe, R., Dweck, M., Schurink, G. W., Natour, E., et al. (2019). Role of Vascular Smooth Muscle Cell Phenotypic Switching and Calcification in Aortic Aneurysm Formation: Involvement of Vitamin K-dependent Processes. Arterioscler. Thromb. Vasc. Biol. 39 (7), 1351–1368. doi:10.1161/atvbaha.119.312787
Phillippi, J. A., Eskay, M. A., Kubala, A. A., Pitt, B. R., and Gleason, T. G. (2010). Altered Oxidative Stress Responses and Increased Type I Collagen Expression in Bicuspid Aortic Valve Patients. Ann. Thorac. Surg. 90 (6), 1893–1898. doi:10.1016/j.athoracsur.2010.07.069
Pichamuthu, J. E., Phillippi, J. A., Cleary, D. A., Chew, D. W., Hempel, J., Vorp, D. A., et al. (2013). Differential Tensile Strength and Collagen Composition in Ascending Aortic Aneurysms by Aortic Valve Phenotype. Ann. Thorac. Surg. 96 (6), 2147–2154. doi:10.1016/j.athoracsur.2013.07.001
QuickZyme (2022). Total Collagen Assay Kit for Collagen Quantification in Tissues. Available at: https://www.quickzyme.com/product-list-assay-kits/total-collagen-assay/ (Accessed: February 09, 2022).
R Core Team (2021). R: A Language and Environment for Statistical Computing. Vienna, Austria: R Foundation for Statistical Computing. Available at: https://www.R-project.org/.
Rahkonen, O., Su, M., Hakovirta, H., Koskivirta, I., Hormuzdi, S. G., Vuorio, E., et al. (2004). Mice with a Deletion in the First Intron of the Col1a1 Gene Develop Age-dependent Aortic Dissection and Rupture. Circulation Res. 94 (1), 83–90. doi:10.1161/01.res.0000108263.74520.15
Rhodin, J. A. G. (2014). “Architecture of the Vessel Wall,” in Comprehensive Physiology (John Wiley & Sons), 1–31. doi:10.1002/cphy.cp020201
Rizzo, R. J., McCarthy, W. J., Dixit, S. N., Lilly, M. P., Shively, V. P., Flinn, W. R., et al. (1989). Collagen Types and Matrix Protein Content in Human Abdominal Aortic Aneurysms. J. Vasc. Surg. 10 (4), 365–373. doi:10.1016/0741-5214(89)90409-6
Robertson, A. M., and Watton, P. N. (2013). “Chapter 8 - Mechanobiology of the Arterial Wall,” in Transport in Biological Media. Editors S. M. Becker, and A. V. Kuznetsov (Boston: Elsevier), 275–347.
Silver, F. H., Horvath, I., and Foran, D. J. (2002). Mechanical Implications of the Domain Structure of Fiber-Forming Collagens: Comparison of the Molecular and Fibrillar Flexibilities of the α1-Chains Found in Types I-III Collagen. J. Theor. Biol. 216 (2), 243–254. doi:10.1006/jtbi.2002.2542
Smoljkić, M., Fehervary, H., Van den Bergh, P., Jorge-Peñas, A., Kluyskens, L., Dymarkowski, S., et al. (2017). Biomechanical Characterization of Ascending Aortic Aneurysms. Biomech. Model Mechanobiol. 16 (2), 705–720. doi:10.1007/s10237-016-0848-4
Tanios, F., Gee, M. W., Pelisek, J., Kehl, S., Biehler, J., Grabher-Meier, V., et al. (2015). Interaction of Biomechanics with Extracellular Matrix Components in Abdominal Aortic Aneurysm Wall. Eur. J. Vasc. Endovasc. Surg. 50 (2), 167–174. doi:10.1016/j.ejvs.2015.03.021
Tsamis, A., Krawiec, J. T., and Vorp, D. A. (2013). Elastin and Collagen Fibre Microstructure of the Human Aorta in Ageing and Disease: A Review. J. R. Soc. Interface 10 (83), 20121004. doi:10.1098/rsif.2012.1004
Tsamis, A., Phillippi, J. A., Koch, R. G., Chan, P. G., Krawiec, J. T., D'Amore, A., et al. (2016). Extracellular Matrix Fiber Microarchitecture is Region-specific in Bicuspid Aortic Valve-Associated Ascending Aortopathy. J. Thorac. Cardiovasc. Surg. 151 (6), 1718–1728. doi:10.1016/j.jtcvs.2016.02.019
Wågsäter, D., Paloschi, V., Hanemaaijer, R., Hultenby, K., Bank, R. A., Franco-Cereceda, A., et al. (2013). Impaired Collagen Biosynthesis and Cross-Linking in Aorta of Patients with Bicuspid Aortic Valve. J. Am. Heart Assoc. 2 (1), e000034. doi:10.1161/JAHA.112.000034
Wang, X., LeMaire, S. A., Chen, L., Shen, Y. H., Gan, Y., Bartsch, H., et al. (2006). Increased Collagen Deposition and Elevated Expression of Connective Tissue Growth Factor in Human Thoracic Aortic Dissection. Circulation 114 (1_Suppl. ment), I200–I205. doi:10.1161/CIRCULATIONAHA.105.000240
Wang, K., Meng, X., and Guo, Z. (2021). Elastin Structure, Synthesis, Regulatory Mechanism and Relationship with Cardiovascular Diseases. Front. Cell Dev. Biol. 9, 596702. doi:10.3389/fcell.2021.596702
Wolinsky, H., and Glagov, S. (1967). A Lamellar Unit of Aortic Medial Structure and Function in Mammals. Circulation Res. 20 (1), 99–111. doi:10.1161/01.res.20.1.99
Zhong, L., He, X., Si, X., Wang, H., Li, B., Hu, Y., et al. (2019). SM22α (Smooth Muscle 22α) Prevents Aortic Aneurysm Formation by Inhibiting Smooth Muscle Cell Phenotypic Switching through Suppressing Reactive Oxygen Species/NF-κB (Nuclear Factor-κB). Arterioscler. Thromb. Vasc. Biol. 39 (1), e10–25. doi:10.1161/ATVBAHA.118.311917
Keywords: collagen remodeling, aortic aneurysm, bicuspid aortic valve, tissue biomechanics, extracellular matrix
Citation: Neave L, Tahir M, Nightingale M, Jadli AS, Patel VB, Barker AJ, Malaisrie SC, McCarthy PM, Markl M, Fedak PWM and Di Martino ES (2022) Medial Collagen Type and Quantity Influence Mechanical Properties of Aneurysm Wall in Bicuspid Aortic Valve Patients. Front. Mech. Eng 8:874243. doi: 10.3389/fmech.2022.874243
Received: 11 February 2022; Accepted: 02 June 2022;
Published: 29 June 2022.
Edited by:
Lucas Robert Smith, University of California, Davis, United StatesReviewed by:
Paolo Zunino, Politecnico di Milano, ItalyKaranvir Saini, University of Pennsylvania, United States
Copyright © 2022 Neave, Tahir, Nightingale, Jadli, Patel, Barker, Malaisrie, McCarthy, Markl, Fedak and Di Martino. This is an open-access article distributed under the terms of the Creative Commons Attribution License (CC BY). The use, distribution or reproduction in other forums is permitted, provided the original author(s) and the copyright owner(s) are credited and that the original publication in this journal is cited, in accordance with accepted academic practice. No use, distribution or reproduction is permitted which does not comply with these terms.
*Correspondence: Elena S. Di Martino, ZWRpbWFydGlAdWxjYWxnYXJ5LmNh