- 1State Key Laboratory of Engines, Tianjin University, Tianjin, China
- 2Automotive Technology and Research Center Co Ltd., Tianjin, China
Fuel additives are considered to be a cost-effective and simple approach to improve combustion and reduce the harmful emissions of internal combustion engines. In addition to the use of conventional fuel additives, some unconventional fuel additives also have potential to improve fuel properties. Exploring the effects of different unconventional additives can provide a valuable reference to improve vehicle performance by fuel optimization. In this study, five unconventional gasoline additives (i.e., isopropyl ether, aniline, diethylamine, dimethyl malonate and p-tert-butylphenol) were blended with the baseline gasoline (G92). The five blended fuels are referred to as G92-1, G92-2, G92-3, G92-4, and G92-5, respectively. Fuels with different additives were tested on a compact passenger vehicle with a 1.4-L gasoline direct injection engine to assess the effects of these additives on performance and emission characteristics, and G92 gasoline was compared as a baseline. The new European drive cycle (NEDC), which is representative for passenger car and light duty vehicles, was chosen in the tests. The experimental results show little or slight improvement in fuel consumption for fuels blended with additives. With respect to gaseous emissions, the vehicle obtains the lowest and highest NOx emissions by fueling G92-5 (blended with p-tert-butylphenol) and G92-3 (blended with diethylamine), respectively; the lowest and highest CO emission is acquired using G92-2 (blended with aniline) and G92-4 (blended with dimethyl malonate), respectively; the vehicle reaches the lowest and highest THC emissions when fueling G92-3 (blended with diethylamine) and G92-4 (blended with dimethyl malonate), respectively; and the lowest and highest CO2 emission using G92-3 (blended with diethylamine) and G92-2 (blended with aniline), respectively. Compared with the baseline gasoline fuel, all of the fuels with additives show improved engine-out PM emissions. Furthermore, all five additives can improve the acceleration performance slightly. In brief, diethylamine is potential gasoline additive to reduce carbon emissions, improve fuel consumption, and enhance performance.
Introduction
Internal combustion (IC) engines have been undergoing continuous improvement and development in terms of thermal efficiency, power density, and reliability for over one hundred years, and they are widely used in power generation, engineering machinery, transportation, and other fields. Although the number of electric vehicles is increasing, most vehicles are still equipped with IC engines as power devices. There is also a consensus that IC engines will continue to dominate in the foreseeable future. Meanwhile, it is reported that 11 billion tons of fossil fuels are being consumed by annually on a global scale, which accounts for 82.67% of the total energy consumption (Nanthagopal et al., 2019). Due to the large holding number and consequent massive consumption of fuel, vehicles with IC engines have caused serious problems, among which energy shortage and environmental pollution are the most significant issues (Gauderman et al., 2007; Perera, 2017). The main pollutant emissions from IC engine powered vehicles are carbon monoxide (CO), hydrocarbons (HC), nitrogen oxides (NOx), and particulate matter (PM). These pollutants can cause environmental issues that impact human health (Mannina et al., 2016). Furthermore, carbon dioxide (CO2) is the main product of fossil-fuel combustion and is considered to be a major greenhouse gas that contributes to global warming. Gasoline engines continue to be a major power source for light duty vehicles and they occupy a large share of the market, especially for passenger cars. Therefore, increasing the thermal efficiency and reducing the emissions of gasoline engines are of great significance.
The efficiency of IC engines can be increased by optimizing engine design, and improving engine management systems and fuel design (Pandey et al., 2018; Puškár and Kopas, 2018; Liu et al., 2020; Liu H. et al., 2021). The application of fuel additives is considered to be a cost-effective and simple approach to improve fuel properties, which can achieve higher efficiency and reduce harmful emissions of IC engines without adding devices or changing the structure of IC engines.
Gasoline additives can be roughly divided into three categories according to their functions: gasoline detergent additives, gasoline additives that increase the octane number, and compound functional gasoline additives. Gasoline additives that increase octane number are also considered as antiknock additives because their major role is to increase the fuel’s antiknock capability. Gasoline antiknock additives mainly include ethers (methyl tert-butyl ether, tert-amyl methyl ether, diisopropyl ether, and ethyl tert-butyl ether) (Arteconi et al., 2011; Magnusson and Nilsson, 2011; Yee et al., 2013; Dalli et al., 2014; Elfasakhany, 2015; Dhamodaran et al., 2016; Pereira et al., 2018; Sassykova et al., 2018), alcohols (methanol and ethanol) (Costa and Sodré, 2010; Çelik et al., 2011; Yang et al., 2012; Zhen et al., 2013; Gong et al., 2016; Iodice et al., 2017; Wang et al., 2019), esters (dimethyl carbonate and dimethyl malonate) (Wen et al., 2010; Schifter et al., 2016; Abdalla and Liu, 2018), organometallic compounds, and so on. Tetraethyl lead was a common and effective means to improve the antiknock capability of gasoline. However, the exhaust of leaded gasoline contains lead, which is a toxic and harmful heavy metal that can enter the human body through the respiratory tract when it diffuses into the atmosphere. Leaded gasoline can affect the intellectual development of children and can also cause poisoning of catalytic converters. Therefore, tetraethyl lead was soon forbidden internationally as a gasoline additive.
As a substitute to lead, methyl tert-butyl ether (MTBE) was considered promising due to high octane number and purification of the exhaust gasses (Awad et al., 2018; Yang et al., 2020). However, MTBE is a type of ether that is very soluble in water, and even very low concentrations can cause water odor. Inhaling a small amount of MTBE can cause inflammation of the nose and throat, and MTBE is difficult to decompose. Therefore, various states in the United States also prohibited the use of MTBE in gasoline in the early-twenty-first century. As a renewable fuel, ethanol can be made from bagasse, corn, some wild plants, and so on, which is conducive to maintaining sustainable development and alleviating the energy crisis. Brazil and the United States began research into the use of ethanol gasoline in the 1970s (Wheals et al., 1999). Ethanol gasoline has also been proposed in China and promoted in many regions (Yang and Chen, 2013).
Researchers from many countries have studied the application of fuel additives in engines. For example, Poulopoulos and Philippopoulos (2000) investigated the effect of adding 0%–11% MTBE in gasoline on the emissions of a spark ignition (SI) engine. Their experimental results indicate that blending MTBE in gasoline only reduced CO and HC emissions at high loads. Meanwhile, during engine cold start, the emissions of CO and HC increased with increasing MTBE blending ratio. Franklin et al. (2001) found that gasoline blending MTBE reduced CO and total hydrocarbon (THC) emissions to some extent, but the MTBE content in unburned hydrocarbon (UHC) increased significantly under engine cold start or fuel-rich conditions (i.e., oxygen lacking). Dimethyl carbonate (DMC) is a colorless liquid that is used as a green solvent or methylating additive. It can also be used as a gasoline additive due to its high antiknock properties (Liu X. et al., 2021). Wen et al. (2010) reported that blending DMC in gasoline reduced both volatile organic compounds and CO emissions, but had little effect on NOx emissions. Meanwhile, Abdullah et al. (2015) conducted an experiment on single-cylinder SI engine fueling ethanol-gasoline blends with 10%–30% of ethanol addition, which were referred to as E10, E20, and E30, respectively, at speeds of 2000, 2,500, and 3,000 rpm. The results demonstrate that the lowest brake specific fuel consumption is acquired by fueling E20, by which an improvement of about 41% can be achieved compared to baseline gasoline. These results were attributed to the better thermal efficiency of ethanol-gasoline blends compared with baseline gasoline (Al-Hasan, 2003; Najafi et al., 2009; Liu et al., 2015). Su et al. (2022) explored the effects of ethanol-gasoline mixtures on PM emissions in a direct injection SI engine. The results highlighted that the ethanol-gasoline blends reduced the total and accumulation mode PM compared to the neat gasoline. Yücesu et al. (2006) explored that the effects of ethanol-gasoline mixtures (10%, 20%, 40%, and 60% of ethanol blending ratio) on the combustion and emission characteristics of a SI engine. The HC emissions of ethanol-gasoline blends were lower than those of baseline fuel, and the maximum reductions of E40 (40% ethanol concentration) and E60 (60% ethanol concentration) were 9.9% and 16.45%, respectively, at 5,000 rpm engine speed. Several reasons contributed to these results, for instance ethanol has a higher latent heat of vaporization compared with the baseline fuel and the higher oxygen content was instrumental in the improvement of the combustion process (Feng et al., 2013; Sarathy et al., 2014).
In summary, these focused on the effects of conventional gasoline additives (e.g., ethanol, MTEB, DMC, etc.) on the combustion and emission characteristics of SI engines. However, there are relatively fewer studies concerning the effects of gasoline unconventional additives on vehicle performance. Although some of their chemical components are not clearly specified in the relevant national standards at present, some unconventional additives also have potential to improve the quality of gasoline to meet the standards of a relevant vehicle fuel. However, they may also have a negative influence on the vehicle’s performance. Therefore, it is particularly significant to systematically evaluate the impact of these unconventional gasoline additives in vehicles.
Based on this discussion, to enrich the types of gasoline additives for a reference of the diversification of gasoline additives, five unconventional additives, i.e., isopropyl ether, aniline, diethylamine, dimethyl malonate and p-tert-butylphenol were selected. The effects of different additives on fuel economy, power performance, and emission characteristics were investigated on a product passenger vehicle equipped with gasoline direct injection (GDI) engine via rotating drum experiment which simulates real road driving condition.
Experimental Setup and Methodology
Experimental Setup and Test Fuel
A commercial vehicle equipped with GDI engine was employed in the experiments, which was produced in China and has relatively higher market share on a global scale. The main engine and vehicle specifications are illustrated in Table 1. Before the experiments, the accumulated mileage of the GDI vehicle was approximately 500 km. Figure 1 demonstrates the schematic of the drum test bench that was used to simulate the actual driving conditions. During the experiments, air was introduced through the dilution channel to dilute the exhaust gasses of the vehicle to mimic the process of the exhaust entering the atmospheric environment. The diluted exhaust was discharged into the atmosphere at a constant flow rate and part of the diluted exhaust was gathered in an air bag. The pollutant concentrations of diluted exhaust were consecutively measured by means of an emission analyzer throughout the test process. The pollutant concentrations in the airbag were also analyzed after the experiment cycle. The results of the gaseous emissions and fuel consumption were based on the analysis of the airbag’s components. The types and manufacturers of the major instruments that are used in this study are shown in Table 2 and Table 3 shows the measurement errors.
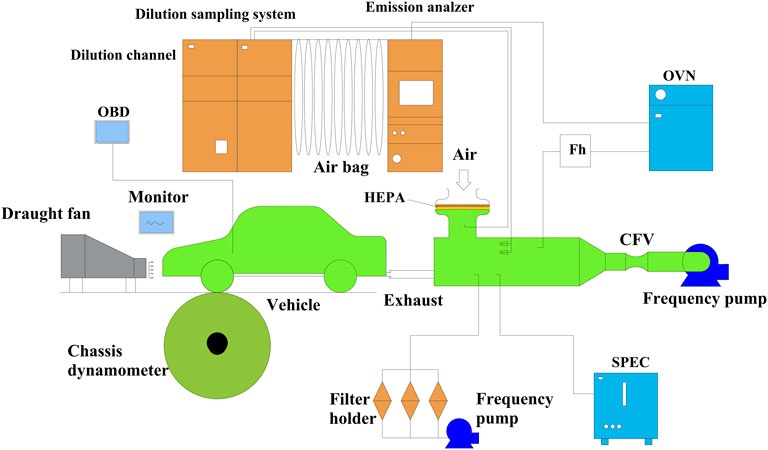
FIGURE 1. Schematic of the rotating drum test bench (Liu et al., 2019). OVN: oven type heated analyzer; HEPA: high efficiency particulate air filter; CFV: critical flow venturi.
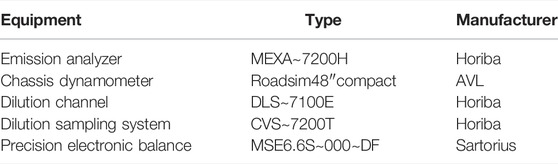
TABLE 2. Manufacturers and types of instruments (Liu, et al., 2019).
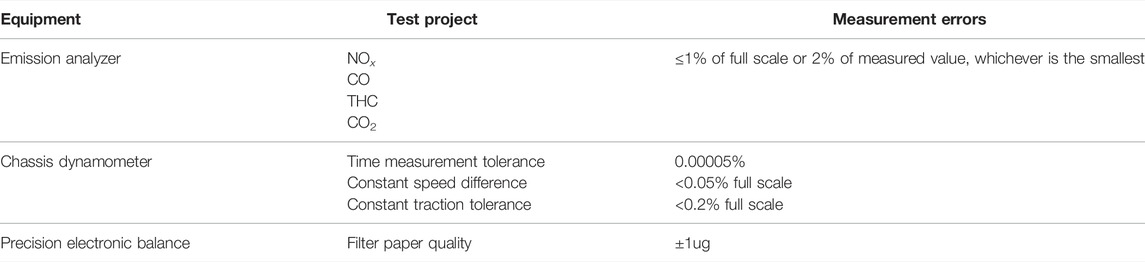
TABLE 3. Measurement errors of the instruments (Liu, et al., 2019).
The five unconventional additives that were selected in this study were isopropyl ether, aniline, diethylamine, dimethyl malonate, and p-tert-butylphenol. The experiment fuels in the current study were baseline gasoline (G92), gasoline blended with 15% (volume fraction, the same for other fuels) isopropyl ether additive (named as G92-1), gasoline blended with 1.5% aniline additive (G92-2), gasoline blended with 1.5% diethylamine additive (G92-3), gasoline blended with 3% dimethyl malonate additive (G92-4), and gasoline blended with 3% p-tert-butylphenol additive (G92-5). In general, all of the additives have an excellent ability to improve gasoline’s antiknock performance due to higher octane number, which further affects the combustion and emission performance of the vehicle. Therefore, it is necessary to investigate the effect of the selected additives on the vehicle’s combustion and emission characteristics. The fractions of different additives blending in gasoline are not the same because the effect of these additives on vehicle performance varies, and this study focuses on a comparison between gasoline blended with different additives and baseline gasoline. Hence, the fraction of additive with smaller effects is larger. The measured fuel properties are listed in Table 4. As the base fuel, G92 has the highest content of olefins, aromatics and C9+ aromatics. G92-2 has the highest research octane number (RON), G92-4 has the highest density and T50, and G92-5 has the highest T90 and heating value.
Experimental Approach
In the current study, the test cycle adopted new European driving cycle (NEDC) is divided further into two segments: extra urban driving cycle (EUDC) and urban driving cycle (UDC). The specific vehicle speed was shown in our previous study (Liu et al., 2019). The vehicle speed of the EUDC condition is higher than that of the UDC condition. In the current study, at least one complete NEDC cycle should be taken before the test to warm up the engine and enable the subsequent test to be started in a hot state, which is relevant to the widely adopted start-stop technology. For each fuel, at least two NEDC cycle tests were conducted repeatedly to ensure the accuracy of the test data.
The impacts of different additives on the vehicle power performance were estimated by means of an acceleration experiment. A method that took measurement separately for each gear was applied because the vehicle was equipped with a manual transmission. Specifically, the acceleration experiment velocity scale was 30–60, 40 to 80, and 60–110 km/h for the third, fourth, and fifth gear, respectively, which was determined by the minimum speed required for stable engine operation and the maximum speed limitation for each gear.
During the experiments, the filters of the intake and oil system were replaced regularly and the vehicle’s tire pressure was kept at 2.2–2.3 bar. The fuel filter was also displaced, and the previous fuel in the external gasoline pipeline and fuel tank was completely drained when changing the test fuel. To eliminate the interference of the previous test fuel on the new test fuel, the vehicle needed to operate with the new test fuel at a speed of 60–90 km/h for more than 30 min before experiment. It should be noted that to stress the impact of different fuels on the engine-out emission characteristics and to eliminate the effects of after-treatment system, especially considering that partial or complete failures in the after-treatment may occur during use, the three-way catalyst (TWC) was disabled before the experiment.
Results and Discussions
Effects of Different Additives on Fuel Consumption and Acceleration Performance
The vehicle volume fuel consumption for each 100 km fueled with different test fuels is shown in Figure 2. The fuel consumption values of the different fuels are labeled above the bar and error bars have been added. As illustrated in Figure 2, the minimum and maximum volume fuel consumption values are acquired by fueling G92-3 and G92-2 for the entire NEDC, respectively, which suggests that the addition of diethylamine decreases fuel consumption (0.17%), while blending aniline in gasoline increases fuel consumption (2.4%) compared to G92 fuel. This may be related to several factors. The volume fuel consumption has a strong negative correlation with volume heating value. Generally speaking, the higher the volume heating value, the lower the volume fuel consumption given that the heat released is the same. However, the details in the fuel composition also play a significant role in the fuel consumption. For instance, a fuel with higher aromatics content usually indicates poorer fuel quality, leading to higher volume fuel consumption (Jin et al., 2017). Furthermore, C9+ aromatics are a kind of polycyclic aromatic hydrocarbons with greater than nine carbon atoms, which are hard to react and decompose completely because of the benzene ring structure. This causes a relatively low vaporization rate, which results in a decrease of efficiency (Wen et al., 2020). Therefore, the engine fueled with a fuel with higher C9+ aromatics content may exhibit increased fuel consumption. The G92-2 fuel reveals a higher volume heating value, which may lead to a decrease in fuel consumption. However, G92-2 also shows a relatively high content of aromatic and C9+ aromatics. More importantly, it demonstrates the highest RON brings about a longer ignition delay and the main combustion process is extended to the expansion stroke stage, which causes a decrease in the maximum pressure and the engine’s output power. Hence, fuel consumption per output power increases, which plays a more dominant role and in turn leads to the highest volume fuel consumption. The G92-3 fuel displays a higher aromatics content, which tends to increase fuel consumption. However, the volume heating value is higher and the lowest volume fuel consumption was achieved as a result of combined effects. When compared with the baseline gasoline, the blended fuels generously increase fuel consumption, except for the G92-3 fuel. This result demonstrates that diethylamine can reduce carbon emission compared with baseline gasoline, although the reduction is not obvious, which is related to the lower blending ratio (only 1.5% volume fraction in the current study).
Figure 3 shows the acceleration time of the vehicle fueled with various test fuels at each gear. The acceleration speed scale was marked above all of the bars. All of the additives improve the acceleration performance slightly, by which the shortest acceleration time is achieved by fueling G92-5, except for third gear. However, the influence of the five unconventional fuel additives selected on the acceleration performance of this vehicle was only marginal—the change of acceleration time for the different fuels is less than 3%.
Effects of Different Additives on Gaseous and Particulate Matter Emissions
To emphasize the effects of different additives on engine-out pollutant emissions in the test, the TWC of vehicle was removed while maintaining the exhaust back pressure invariant. Consequently, the results that are discussed in this section are engine-out emissions and error bars have been added.
The impacts of the different additives on NOx emissions are illustrated in Figure 4. The results indicate that the engine shows the lowest and the highest NOx emissions when fueling G92-5 (blending with p-tert-butylphenol) and G92-3 (blending with diethylamine) for the entire NEDC cycle, respectively, which demonstrates that the addition of p-tert-butylphenol decreases NOx emissions (13%), while the addition of diethylamine increases NOx emissions (16%) to the maximum extent, respectively. This may because the molecular formula of diethylamine is C4H11N, which contains a nitrogen atom. During the engine combustion process, nitrogen atoms can be converted into NOx through a series of chemical reactions, which in turn leads to the increase in NOx generation of G92-3 fuel. The molecular formula of aniline is C6H7N and the molecule also contains N, but the content of nitrogen is lower than that of diethylamine. Therefore, when compared with the baseline gasoline, the G92-2 fuel has a slight increase in NOx emissions but the total NOx emissions are lower than those of the G92-3 fuel. Therefore, the fuel containing nitrogen is not conducive to reducing NOx emissions. Meanwhile, T90, which is an important fuel property parameter, reflects the proportion of the heavy components of fuel. Higher T90 usually indicates more heavy components and worse volatility. Therefore, the higher T90 of fuel, the more difficult for the fuel to form a homogeneous mixture with air. This is likely to cause a fuel-rich region in the cylinder to deviate from the conditions conducive to NOx formation and reduce NOx generation. T50 reflects the proportion of intermediate components in the fuel. A higher T50 suggests worse the fuel volatility. Engine fueling with the fuel of higher T50 tends to cause a fuel-rich region and leads to difficulty in NOx generation. Therefore, the lowest NOx emission was obtained when the engine was fueled with the G92-5 with the higher T50 and highest T90. It can also be seen from Figure 4 that NOx emissions are reduced by fueling gasoline blended with isopropyl ether and dimethyl malonate. Although the volume percentage of isopropyl ether is the highest, the degree of decreasing NOx emissions is the lowest compared with dimethyl malonate (G92-4) and p-tert-butylphenol (G92-5), which suggests that isopropyl ether has a smaller role in reducing NOx.
The effects of different fuels on CO emission of vehicle are illustrated in Figure 5. The results highlight that the minimum and maximum CO emission values are obtained using G92-2 (blending with aniline) and G92-4 (blending with dimethyl malonate) as fuel in the entire NEDC, respectively. This indicates that gasoline mixing with aniline decreases CO emission (3.1%); while gasoline mixing with dimethyl malonate increases CO emission (5%) to the maximum extent, respectively. Generally speaking, a higher T50 suggests worse fuel volatility. When the engine is fueled with a poor volatile fuel, it is difficult to form homogeneous mixture and more fuel-rich region appears in the cylinder. This will increase CO generation. The olefins are active in chemical properties and the flame propagation speed of olefins is higher than that of alkanes (Shen et al., 2008). Under the high load conditions of EUDC, the engine fueled with the lower olefin content fuel may cause poor CO oxidation and long combustion duration. Meanwhile, the aromatics are unsaturated hydrocarbons with lower H/C ratio. Higher carbon content usually brings about incomplete combustion due to insufficient air, which results in the formation of CO. These factors comprehensively affect CO emissions, while T50 may have a greater impact. Therefore, CO emission is highest when the engine is fueled with the G92-4 fuel with the highest T50. The lowest CO emission of the G92-2 fuel may be attributed to its highest RON, which leads to advanced ignition timing, increased combustion constant volume degree and combustion efficiency, and consequently results in the lowest CO emission.
The THC emissions of the vehicle fueled with different test fuels are illustrated in Figure 6. This suggests that the engine achieved the lowest and highest THC emissions when fueling G92-3 (blending with diethylamine) and G92-4 (blending with dimethyl malonate) for the entire NEDC cycle, respectively. This shows that the addition of diethylamine decreases THC emissions (8.1%), but the addition of dimethyl malonate increases THC emissions (2.5%) to the maximum extent, respectively. As discussed earlier, C9+ aromatics are difficult to react completely, and the higher C9+ aromatic content is prone to higher THC emissions. In addition, the higher T50 leads to lower volatility, which leads to a slow fuel-air mixing process and leaves some fuel-rich pockets that contribute to THC formation. The lower T90 indicates the decrease of fuel heavy components, leading to the decrease of fuel adsorbed by lubricant oil film on liner, which is desorbed during the exhaust process and forms unburned HC. Furthermore, an increase of oxygen content can promote the oxidation process of HC, which improves THC emissions. These four factors play a combined role on the THC emissions, which brings about the highest THC emissions of the G92-4 fuel.
The CO2 emissions of the vehicle fueled with different test fuels are illustrated in Figure 7. The lowest and the highest CO2 emissions were obtained when fueling G92-3 (blending with diethylamine) and G92-2 (blending with aniline) for the entire NEDC, respectively. This implies that gasoline mixing with diethylamine decreases CO2 emission (0.12%), while gasoline mixing with aniline increases CO2 emission (2.8%) to the maximum extent, respectively. It should be noted that the effects of adding different additives on CO2 emissions are basically the same as that of fuel consumption, which is the main factor affecting CO2 emissions. In terms of fuel properties, the chemical property of olefins is active, meanwhile the flame propagation speed of olefins is higher than that of alkanes (Shen, et al., 2008). The flame surface temperature of alkanes is lower than that of aromatics (Wedekind et al., 1995; Diana et al., 1998). Furthermore, the C/H ratios of aromatics and olefins are higher than those of alkanes. These elements are instrumental in the increase of the CO2 emission.
What interests us in this study is that the gas emissions vary in the UDC and EUDC stages. The vehicle speed of UDC is lower than that of EUDC, and there will be frequent acceleration and deceleration. This easily causes a fuel-rich region in the cylinder to increase the emissions of THC and CO. Hence, the emissions of CO and THC of UDC are higher than those of EUDC. The in-cylinder average temperature of EUDC is relatively higher due to the higher vehicle speed, which is conducive to the generation of NOx. Therefore, the NOx emissions of EUDC are higher than those of UDC. The CO2 emission is mainly related to fuel consumption. Furthermore, due to the lower in-cylinder temperature of UDC, the emissions are influenced by the test fuel properties and the discrepancies are relatively higher. Compared with baseline gasoline, the maximum differences of the emissions of NOx, CO, THC, and CO2 in the UDC are 30%, 3.5%, 9.6%, and 4.4%, respectively; while the maximum discrepancy of emissions of NOx, THC, CO, and CO2 in the EUDC are 12%, 6.0%, 7.4%, and 1.6%, respectively. Furthermore, the UDC is more intricate when compared to the EUDC. Therefore, the UDC condition should generally be of more concern. In detail, referring to the effects of different additives on gaseous emissions, it can be seen that although the blending volume ratio of isopropyl ether is the highest, the impacts on gaseous emissions are not obvious. It is also observed that the effects of different additives vary in different operation conditions, as seen from the comparison results between EUDC and UDC.
The impacts of different additives on PM emissions of the vehicle are illustrated in Figure 8. Compared with baseline gasoline, the engine can obtain lower PM emissions when fueling the five fuels with the selected additives. The reason for this may be that the chemical properties of olefins are more active than those of alkanes. The increased olefins content promotes combustion and reduces the PM formation. This aromatic is a kind of hydrocarbon with poor volatility and large molecular weight. It is likely to cause poor fuel-air mixing process when the engine is fueled with a relatively higher aromatics content fuel. Meanwhile, it should be noted that aromatics are precursors for the formation of soot particles (Wen, et al., 2020). This point has been discussed in previous research (Jain et al., 2007; Kim et al., 2013). Furthermore, the higher T50 implies incomplete combustion and slower fuel vaporization, which leads to increase PM formation. The lower T90 indicates less-heavy components in the fuels, which promotes the fuel-air mixing process and leads to decreased PM emissions. Finally, the oxygen content in fuel molecules is also beneficial for reducing PM formation. The combination effects of these five factors affect PM emissions. The difference between the maximum and minimum PM emissions values is 54.6% for the entire NEDC, which is acquired by the contrast of G92 and G92-3. It should be noted that although the blending ratio of diethylamine is the lowest (1.5% vol), the effects of the decreasing PM emissions are remarkable.
Conclusion
In this study, the impacts of different unconventional additives on the consumption, emission characteristics, and acceleration performance were investigated in a vehicle equipped with the GDI engine. Baseline gasoline (G92), gasoline blended with 15% (the percentage here is volume fraction, the same for other fuels) isopropyl ether (G92-1), gasoline blended with 1.5% aniline (G92-2), gasoline blended with 1.5% diethylamine (G92-3), gasoline blended with 3% dimethyl malonate (G92-4), and gasoline blended with 3% p-tert-butylphenol (G92-5) were selected as the test fuels. The experiments were conducted on a drum test bench that simulates real driving conditions and the NEDC was adopted. Moreover, the vehicle acceleration performance was analyzed. The detailed conclusions are as follows.
For the volume fuel consumption in each 100 km, the minimum fuel consumption value is acquired by fueling with G92-3, which shows that blending diethylamine in gasoline decreases fuel consumption (0.17%). The maximum fuel consumption value is acquired by fueling G92-2, which demonstrates that gasoline blended with aniline increases fuel consumption (2.4%). In terms of acceleration performance, all of the additives can shorten the acceleration time. However, the impacts of the five unconventional fuel additives on the acceleration performance are minor—the difference is less than 3%.
With respect to gaseous emissions, the engine obtains the lowest NOx and CO emissions when fueling G92-5 and G92-2, respectively. This demonstrates that the addition of p-tert-butylphenol and aniline decreases NOx (13%) and CO (3.1%), respectively. The engine obtains the lowest THC and CO2 emissions when fueling G92-3, which demonstrates that the addition of diethylamine decreases THC (8.1%) and CO2 (0.12%) emissions. The engine shows the highest NOx and CO2 emissions by fueling G92-3 and G92-2 respectively, which suggests that the addition of diethylamine and aniline increases NOx (13%) and CO2 (0.12%) emissions, respectively. The engine shows the highest CO and THC emissions by fueling G92-4, which indicates that the addition of dimethyl malonate increases CO (5%) and THC (2.5%) emissions. With regard to PM emissions, all of the fuels with additives improve PM emissions when compared with the baseline gasoline fuel. The difference between the maximum and minimum PM emissions values is 54.6%, which is obtained by comparing G92 (baseline gasoline) and G92-3 (the addition of diethylamine), respectively.
In conclusion, the lowest fuel consumption and emissions of THC, CO2, and PM are achieved by fueling with G92-3. Although NOx emissions are higher than those of other test fuels due to the nitrogen content of diethylamine, gaseous emissions can be addressed by TWC. Therefore, among all selected additives, diethylamine is a promising unconventional gasoline additive.
Data Availability Statement
The original contributions presented in the study are included in the article/supplementary material, further inquiries can be directed to the corresponding author.
Author Contributions
All authors listed have made a substantial, direct, and intellectual contribution to the work and approved it for publication.
Funding
This work was supported by National Natural Science Foundation of China (NSFC) and Science Fund for Distinguished Young Scholars of Tianjin through the Project of 91941102 and 20JCJQJC00160 respectively.
Conflict of Interest
Author ZY was employed by the company Automotive Technology and Research Center Co Ltd.
The remaining authors declare that the research was conducted in the absence of any commercial or financial relationships that could be construed as a potential conflict of interest.
Publisher’s Note
All claims expressed in this article are solely those of the authors and do not necessarily represent those of their affiliated organizations, or those of the publisher, the editors, and the reviewers. Any product that may be evaluated in this article, or claim that may be made by its manufacturer, is not guaranteed or endorsed by the publisher.
References
Abdalla, A., and Liu, D. (2018). Dimethyl Carbonate as a Promising Oxygenated Fuel for Combustion: A Review. Energies 11, 1552. doi:10.3390/en11061552
Abdullah, N. R., Zaharin, M. S. M., Mamat, A. M. I., Nawi, M. R. M., and Sharudin, H. (2015). Effects of Ethanol Blends on Gasoline Engine Performance and Exhaust Emissions. J. Teknol. 76, 107–112. doi:10.11113/jt.v76.5920
Al-Hasan, M. (2003). Effect of Ethanol-Unleaded Gasoline Blends on Engine Performance and Exhaust Emission. Energy Convers. Manag. 44, 1547–1561. doi:10.1016/s0196-8904(02)00166-8
Arteconi, A., Mazzarini, A., and Di Nicola, G. (2011). Emissions from Ethers and Organic Carbonate Fuel Additives: A Review. Water Air Soil Pollut. 221, 405–423. doi:10.1007/s11270-011-0804-y
Awad, O. I., Mamat, R., Ali, O. M., Sidik, N. A. C., Yusaf, T., Kadirgama, K., et al. (2018). Alcohol and Ether as Alternative Fuels in Spark Ignition Engine: A Review. Renew. Sustain. Energy Rev. 82, 2586–2605. doi:10.1016/j.rser.2017.09.074
Çelik, M. B., Özdalyan, B., and Alkan, F. (2011). The Use of Pure Methanol as Fuel at High Compression Ratio in a Single Cylinder Gasoline Engine. Fuel 90, 1591–1598. doi:10.1016/j.fuel.2010.10.035
Costa, R. C., and Sodré, J. R. (2010). Hydrous Ethanol vs. Gasoline-Ethanol Blend: Engine Performance and Emissions. Fuel 89, 287–293. doi:10.1016/j.fuel.2009.06.017
Dalli, D., Lois, E., and Karonis, D. (2014). Vapor Pressure and Octane Numbers of Ternary Gasoline–Ethanol–ETBE Blends. J. Energy Eng. 140, A4014002. doi:10.1061/(asce)ey.1943-7897.0000153
Dhamodaran, G., Esakkimuthu, G. S., and Pochareddy, Y. K. (2016). Experimental Study on Performance, Combustion, and Emission Behaviour of Diisopropyl Ether Blends in MPFI SI Engine. Fuel 173, 37–44. doi:10.1016/j.fuel.2016.01.014
Diana, S., Giglio, V., Iorio, B., and Police, G. (1998). The Influence of Fuel Composition on Pollutant Emission of Premixed Spark Ignition Engines in Presence of EGR. SAE Technical Paper 982621. Warrendale, PA: SAE International.
Elfasakhany, A. (2015). Investigations on the Effects of Ethanol-Methanol-Gasoline Blends in a Spark-Ignition Engine: Performance and Emissions Analysis. Eng. Sci. Technol. Int. J. 18, 713–719. doi:10.1016/j.jestch.2015.05.003
Feng, R., Yang, J., Zhang, D., Deng, B., Fu, J., Liu, J., et al. (2013). Experimental Study on SI Engine Fuelled with Butanol-Gasoline Blend and H2O Addition. Energy Convers. Manag. 74, 192–200. doi:10.1016/j.enconman.2013.05.021
Franklin, P. M., Koshland, C. P., Lucas, D., and Sawyer, R. F. (2001). Evaluation of Combustion By-Products of MTBE as a Component of Reformulated Gasoline. Chemosphere 42, 861–872. doi:10.1016/s0045-6535(00)00261-7
Gauderman, W. J., Vora, H., McConnell, R., Berhane, K., Gilliland, F., Thomas, D., et al. (2007). Effect of Exposure to Traffic on Lung Development from 10 to 18 Years of Age: a Cohort Study. Lancet 369, 571–577. doi:10.1016/s0140-6736(07)60037-3
Gong, C., Liu, F., Sun, J., and Wang, K. (2016). Effect of Compression Ratio on Performance and Emissions of a Stratified-Charge DISI (Direct Injection Spark Ignition) Methanol Engine. Energy 96, 166–175. doi:10.1016/j.energy.2015.12.062
Iodice, P., Senatore, A., Langella, G., and Amoresano, A. (2017). Advantages of Ethanol-Gasoline Blends as Fuel Substitute for Last Generation Si Engines. Environ. Prog. Sustain. Energy 36, 1173–1179. doi:10.1002/ep.12545
Jain, A. K., Pathak, S., Singh, Y., Singh, S., Saxena, M., Subramanian, M., et al. (2007). Effect of Gasoline Composition (Olefins, Aromatics and Benzene) on Exhaust Mass Emissions from Two-Wheelers - an Experimental Study. SAE Technical Paper 2007-26-014. Warrendale, PA: SAE International.
Jin, D., Choi, K., Myung, C.-L., Lim, Y., Lee, J., and Park, S. (2017). The Impact of Various Ethanol-Gasoline Blends on Particulates and Unregulated Gaseous Emissions Characteristics from a Spark Ignition Direct Injection (SIDI) Passenger Vehicle. Fuel 209, 702–712. doi:10.1016/j.fuel.2017.08.063
Kim, Y., Kim, Y., Kang, J., Jun, S., Rew, S., Lee, D., et al. (2013). Fuel Effect on Particle Emissions of a Direct Injection Engine. SAE Technical Paper 2013-01-1559. Warrendale, PA: SAE International.
Liu, H., Wang, X., Zhang, D., Dong, F., Liu, X., Yang, Y., et al. (2019). Investigation on Blending Effects of Gasoline Fuel with N-Butanol, DMF, and Ethanol on the Fuel Consumption and Harmful Emissions in a GDI Vehicle. Energies 12, 1845. doi:10.3390/en12101845
Liu, H., Wang, Z., Long, Y., Xiang, S., Wang, J., and Wagnon, S. W. (2015). Methanol-gasoline Dual-Fuel Spark Ignition (DFSI) Combustion with Dual-Injection for Engine Particle Number (PN) Reduction and Fuel Economy Improvement. Energy 89, 1010–1017. doi:10.1016/j.energy.2015.06.051
Liu, H., Wen, M., Cui, Y., Zhang, C., Zheng, Z., and Yao, M. (2020). Effect of Blending N-Butanol in Diesel on Flame Development and Spectrum. Spectrosc. Spectr. Analysis 40, 1998–2004.
Liu, H., Wen, M., Yang, H., Yue, Z., and Yao, M. (2021a). A Review of Thermal Management System and Control Strategy for Automotive Engines. J. Energy Eng. 147, 03121001. doi:10.1061/(asce)ey.1943-7897.0000743
Liu, X., Xue, S., Ikram, R., Zhu, C., Shi, Y., and He, M. (2021b). Improving the Viscosity and Density of N-Butanol as Alternative to Gasoline by Blending with Dimethyl Carbonate. Fuel 286, 119360. doi:10.1016/j.fuel.2020.119360
Magnusson, R., and Nilsson, C. (2011). The Influence of Oxygenated Fuels on Emissions of Aldehydes and Ketones from a Two-Stroke Spark Ignition Engine. Fuel 90, 1145–1154. doi:10.1016/j.fuel.2010.10.026
Mannina, G., Ekama, G., Caniani, D., Cosenza, A., Esposito, G., Gori, R., et al. (2016). Greenhouse Gases from Wastewater Treatment - A Review of Modelling Tools. Sci. Total Environ. 551-552, 254–270. doi:10.1016/j.scitotenv.2016.01.163
Najafi, G., Ghobadian, B., Tavakoli, T., Buttsworth, D. R., Yusaf, T. F., and Faizollahnejad, M. (2009). Performance and Exhaust Emissions of a Gasoline Engine with Ethanol Blended Gasoline Fuels Using Artificial Neural Network. Appl. Energy 86, 630–639. doi:10.1016/j.apenergy.2008.09.017
Nanthagopal, K., Ashok, B., Garnepudi, R. S., Tarun, K. R., and Dhinesh, B. (2019). Investigation on Diethyl Ether as an Additive with Calophyllum Inophyllum Biodiesel for CI Engine Application. Energy Convers. Manag. 179, 104–113. doi:10.1016/j.enconman.2018.10.064
Pandey, S., Diwan, P., Sahoo, P. K., and Thipse, S. S. (2018). A Review of Combustion Control Strategies in Diesel HCCI Engines. Biofuels 9, 61–74. doi:10.1080/17597269.2016.1257315
Pereira, I. L. G., Neto, A. F. G., Moraes, E. S., Sousa, B. S. M., Chen, J., Costa, J. F. S., et al. (2018). DFT and Canonical Ensemble Investigations of Gasoline Additives at the Gas Phase: ETBE, MTBE, DIPE, Ethanol and Methanol. Theor. Chem. Acc. 137, 127. doi:10.1007/s00214-018-2319-8
Perera, F. P. (2017). Multiple Threats to Child Health from Fossil Fuel Combustion: Impacts of Air Pollution and Climate Change. Environ. Health Perspect. 125, 141–148. doi:10.1289/ehp299
Poulopoulos, S., and Philippopoulos, C. (2000). Influence of MTBE Addition into Gasoline on Automotive Exhaust Emissions. Atmos. Environ. 34, 4781–4786. doi:10.1016/s1352-2310(00)00257-0
Puškár, M., and Kopas, M. (2018). System Based on Thermal Control of the HCCI Technology Developed for Reduction of the Vehicle NOx Emissions in Order to Fulfil the Future Standard Euro 7. Sci. Total Environ. 643, 674–680. doi:10.1016/j.scitotenv.2018.06.082
Sarathy, S. M., Oßwald, P., Hansen, N., and Kohse-Höinghaus, K. (2014). Alcohol Combustion Chemistry. Prog. Energy Combust. Sci. 44, 40–102. doi:10.1016/j.pecs.2014.04.003
Sassykova, L. R., Basheva, Z. T., Kalykberdyev, M. K., Nurakhmetova, M., Massenova, A. T., and Rakhmetova, K. S. (2018). The Selective Catalytic Reactions for Improvement of Characteristics of Gasolines. Bulg. Chem. Commun. 50, 82–88.
Schifter, I., González, U., and González-Macías, C. (2016). Effects of Ethanol, Ethyl-Tert-Butyl Ether and Dimethyl-Carbonate Blends with Gasoline on SI Engine. Fuel 183, 253–261. doi:10.1016/j.fuel.2016.06.051
Shen, Y., Shuai, S., and Wang, J. (2008). A Study on the Effect of Olefin on the Emissions and Combustion Characteristics of Engines. Automot. Eng. 8, 644–647.
Su, Y., Zhang, Y., Xie, F., Duan, J., Li, X., and Liu, Y. (2022). Influence of Ethanol Blending Ratios on In-Cylinder Soot Processes and Particulate Matter Emissions in an Optical Direct Injection Spark Ignition Engine. Fuel 308, 121944. doi:10.1016/j.fuel.2021.121944
Wang, C., Li, Y., Xu, C., Badawy, T., Sahu, A., and Jiang, C. (2019). Methanol as an Octane Booster for Gasoline Fuels. Fuel 248, 76–84. doi:10.1016/j.fuel.2019.02.128
Wedekind, B., Bennett, P. J., Goodfellow, C. L., Jeffrey, J. G., Marchesi, G. F., and McDonald, C. R. (1995). The Independent Effect of Mid-range, Back-End Volatility and Aromatics on Emissions from Two European Gasoline Engines. SAE Technical Paper 952522. Warrendale, PA: SAE International.
Wen, L.-b., Xin, C.-Y., and Yang, S.-C. (2010). The Effect of Adding Dimethyl Carbonate (DMC) and Ethanol to Unleaded Gasoline on Exhaust Emission. Appl. Energy 87, 115–121. doi:10.1016/j.apenergy.2009.06.005
Wen, M., Zhang, C., Yue, Z., Liu, X., Yang, Y., Dong, F., et al. (2020). Effects of Gasoline Octane Number on Fuel Consumption and Emissions in Two Vehicles Equipped with GDI and PFI Spark-Ignition Engine. J. Energy Eng. 146, 04020069. doi:10.1061/(asce)ey.1943-7897.0000722
Wheals, A., Basso, L. C., Alves, D. M. G., and Amorim, H. V. (1999). Fuel Ethanol after 25 Years. Trends Biotechnol. 17, 482–487. doi:10.1016/s0167-7799(99)01384-0
Yang, H.-H., Liu, T.-C., Chang, C.-F., and Lee, E. (2012). Effects of Ethanol-Blended Gasoline on Emissions of Regulated Air Pollutants and Carbonyls from Motorcycles. Appl. Energy 89, 281–286. doi:10.1016/j.apenergy.2011.07.035
Yang, Q., and Chen, G. Q. (2013). Greenhouse Gas Emissions of Corn-Ethanol Production in China. Ecol. Model. 252, 176–184. doi:10.1016/j.ecolmodel.2012.07.011
Yang, Q., Shao, S., Zhang, Y., Hou, H., Qin, C., Sun, D., et al. (2020). Comparative Study on Life Cycle Assessment of Gasoline with Methyl Tertiary-Butyl Ether and Ethanol as Additives. Sci. Total Environ. 724, 138130. doi:10.1016/j.scitotenv.2020.138130
Yee, K. F., Mohamed, A. R., and Tan, S. H. (2013). A Review on the Evolution of Ethyl Tert-Butyl Ether (ETBE) and its Future Prospects. Renew. Sustain. Energy Rev. 22, 604–620. doi:10.1016/j.rser.2013.02.016
Yücesu, H. S., Topgül, T., Çinar, C., and Okur, M. (2006). Effect of Ethanol–Gasoline Blends on Engine Performance and Exhaust Emissions in Different Compression Ratios. Appl. Therm. Eng. 26, 2272–2278. doi:10.1016/j.applthermaleng.2006.03.006
Keywords: fuel additives, vehicle, gasoline direct injection engine, fuel consumption, emissions
Citation: Wen M, Yin Z, Zheng Z, Liu H, Zhang C, Cui Y, Ming Z, Feng L, Yue Z and Yao M (2022) Effects of Different Gasoline Additives on Fuel Consumption and Emissions in a Vehicle Equipped With the GDI Engine. Front. Mech. Eng 8:924505. doi: 10.3389/fmech.2022.924505
Received: 20 April 2022; Accepted: 24 May 2022;
Published: 22 July 2022.
Edited by:
Jose Ramon Serrano, Universitat Politècnica de València, SpainReviewed by:
Yong Qian, Shanghai Jiao Tong University, ChinaMohammed Ojapah, University of Port Harcourt, Nigeria
Silvana Di Iorio, CNR—Istituto di Scienze e Tecnologie per l’Energia e la Mobilità Sostenibili (STEMS), Italy
Copyright © 2022 Wen, Yin, Zheng, Liu, Zhang, Cui, Ming, Feng, Yue and Yao. This is an open-access article distributed under the terms of the Creative Commons Attribution License (CC BY). The use, distribution or reproduction in other forums is permitted, provided the original author(s) and the copyright owner(s) are credited and that the original publication in this journal is cited, in accordance with accepted academic practice. No use, distribution or reproduction is permitted which does not comply with these terms.
*Correspondence: Haifeng Liu, aGFpZmVuZ2xpdUB0anUuZWR1LmNu