- 1LaserLab Amsterdam, Department of Physics and Astronomy, Faculty of Science Vrije Universiteit, Amsterdam, Netherlands
- 2Department of Plastic, Reconstructive and Hand Surgery, Amsterdam Movement Sciences, Amsterdam UMC, Vrije Universiteit, Amsterdam, Netherlands
- 3Burn Center, Red Cross Hospital, Beverwijk, Netherlands
- 4Department of Experimental Surgery, McGill University, Montreal, QC, Canada
- 5Shriners Hospital for Children, Montreal, QC, Canada
- 6Department of Molecular Cell Biology and Immunology, Amsterdam UMC, Location VUmc, Amsterdam, Netherlands
- 7Medical Library, Vrije Universiteit Amsterdam, Amsterdam, Netherlands
- 8Department of Plastic and Reconstructive Surgery, Red Cross Hospital, Beverwijk, Netherlands
- 9Paediatric Surgical Centre, Emma Children’s Hospital, Amsterdam UMC, Location AMC, Amsterdam, Netherlands
- 10Faculty of Dental Medicine and Oral Health Sciences, McGill University, Montreal, QC, Canada
In vitro research in the field of mechanotransducive regulation of dermal fibroblasts is characterized by highly variable methodology and contradictory results. The primary objective of this systematic review was to establish how in vitro mechanical stretch affects human dermal fibroblast function, by means of a quantitative synthesis of all available evidence. The secondary objectives were to examine the effects of covariates related to donor age, fibroblast origin, experimental treatments, and mechanical stimulation parameters on dermal fibroblast responsiveness to mechanical strain. Summary outcomes for fibroblast proliferation and collagen production were combined using a fixed-effects meta-analytical model. Subgroup analysis and meta-regression were used to investigate the effects of different conditions on the summary outcomes. Mechanical strain was found to not affect fibroblast proliferation in neonatal fibroblasts, while adult fibroblasts proliferation was significantly increased. Collagen production was significantly increased in response to mechanical stimulation, with Vitamin C stimulation as the most important covariate. Stretching frequency emerged as positively associated with fibroblast proliferation and negatively associated with collagen production. We conclude from this study that distinct differences exist in the effects of mechanical stretching between dermal fibroblasts from neonatal and adult donors, which will help to further elucidate the pathophysiological mechanism behind tension-induced scarring.
1 Introduction
Extensive cutaneous wound healing unavoidably leads to scarring. Excessive scarring in the form of a hypertrophic scar or keloid can occur if the process of wound healing is disordered (Ogawa, 2017). These raised and thickened scars involve a pronounced and prolonged activation of myofibroblasts during the remodeling stage of wound healing (Hinz, 2016), resulting in excessive production and reduced degradation of extracellular matrix (ECM); thus, contributing to a build-up of ECM (Hinz, 2016).
Excessive scarring seems to occur disproportionately at anatomical sites with higher resting tension or high cyclical tension (Barnes et al., 2018). In-plane mechanical tension in general is considered to have harmful effects on cutaneous wound healing, and on the quality of the subsequent scar. A myriad of therapies exist aimed at mitigating the negative effects of tension by diminishing in plane stresses, such as optimized suturing techniques (Marsidi et al., 2020) or devices that disperse tension to the surrounding tissue (Al-Mubarak and Al-Haddab, 2013).
The concept of mechanotransduction involves reciprocal interactions between cells and their mechanical environment (Hinz, 2016), and the subsequent translation into biochemical signals (Martino et al., 2018). The discovery of mechanically sensitive ion channels was the first step to unravelling the potential molecular basis behind scar exacerbation caused by tension (Guharay and Sachs, 1984). This field covers clinical research (Krishnan et al., 2016), animal models (Son and Hinz, 2021), in vitro studies (Shirakami et al., 2020), and in silico studies (Tsuge et al., 2020). In vitro studies play an important role in elucidating the role of mechanobiology in scar formation on a cellular and biomolecular level, however effects described in these articles vary widely and are often contradictory (Wong et al., 2011) These studies involve the seeding of primary cells on extensible membranes, and the subsequent analysis of outcomes while controlling for relevant deformation parameters. Increased attention has been paid recently to improving the translational value of animal studies by means of systematic reviews and meta-analyses (Hooijmans et al., 2014), given the abundance of narrative reviews on this topic of which none evaluate the methodological quality of individual studies. Although the framework for systematic reviewing of clinical trials does not easily lend itself to application in in vitro research (Hooijmans et al., 2014), sparse examples of its successful adaptation exist (Mikolajewicz et al., 2018; McKee et al., 2019).
In this work, we aimed to critically assess the effect of in vitro mechanical stretching on human dermal fibroblasts using a systematic review approach. The primary objective of this systematic review and meta-analysis was to characterize the effect of in vitro mechanical stretch on the functional parameters of human dermal fibroblasts, namely proliferation and collagen production. The secondary objective was to examine the effects of covariates related to donor age, fibroblast origin, experimental treatments, and parameters of mechanical stimulation on the dermal fibroblast responsiveness to mechanical strain. Finally, we evaluated the consistency of reporting of in vitro studies in order to improve their translational potential.
2 Results
2.1 Study characteristics
The systematic literature search (Figure 1) resulted in a total of 11,230 articles. Two additional articles were found by screening references of relevant articles. Title and abstract screening of the remaining articles resulted in the inclusion of 48 relevant full-text papers. Most of these articles were written in the context of cutaneous scar research. Two papers on bioengineered tendon tissue, and four papers on cardiovascular research were included as they involved experiments on dermal fibroblasts. All individual experiments that met the inclusion criteria were included, resulting in more datapoints than papers.
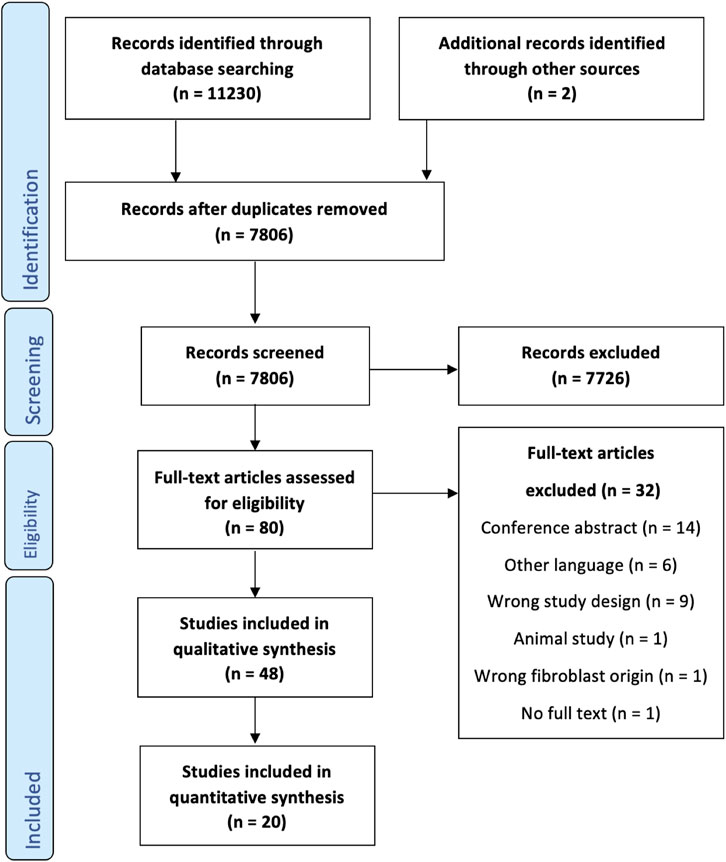
FIGURE 1. PRISMA flowchart detailing the search strategy, screening, and inclusion steps of the systematic review process.
2.2 Methodological quality
The research described in the 48 papers was of heterogeneous design. Donor location varied widely, foreskin being the most commonly used donor tissue being (n = 16) (Figure 2C) and nineteen articles not specifying donor location. The stretching device used in the experiments was described in all articles; 17 papers reported the use of a commercial device from Flexcell, others (n = 20) used custom-built devices (Figure 2D). Methodological quality was scored using a predefined set of items assessing methodological quality (Figure 2A, B, Supplementary Table S5). Items related to the materials used were generally well reported, with only two articles omitting culturing medium and conditioning altogether, and six articles missing a detailed description of the stretching device. Demographical information on cell donors was absent in the vast majority of articles (n = 35). Similarly, the number of cell donors was severely underreported (n = 31). Details on the extensible membrane and its coating or treatment were provided by all authors. Eight papers were missing one or two details on either the load duration, frequency, and magnitude. Conversely, the number of repetitions of experiments was poorly reported in nineteen articles. Four articles made no mention of sample size at all, and a further fifteen articles were vague or included a range of repetitions per analysis used. Most papers reported the use of an unstretched control sample, with only seven articles using an inappropriate control group or making no mention of an unstretched control. Further scrutiny resulted in a number of methodological flaws in articles regarding unstretched control groups, including the use of t = 0 measurements as a control group, and selective omission of control group data. Nineteen articles included an explicit statement on the identical exposure of both the experimental and control group. In nine cases the exposure was demonstrably different from the intervention group.
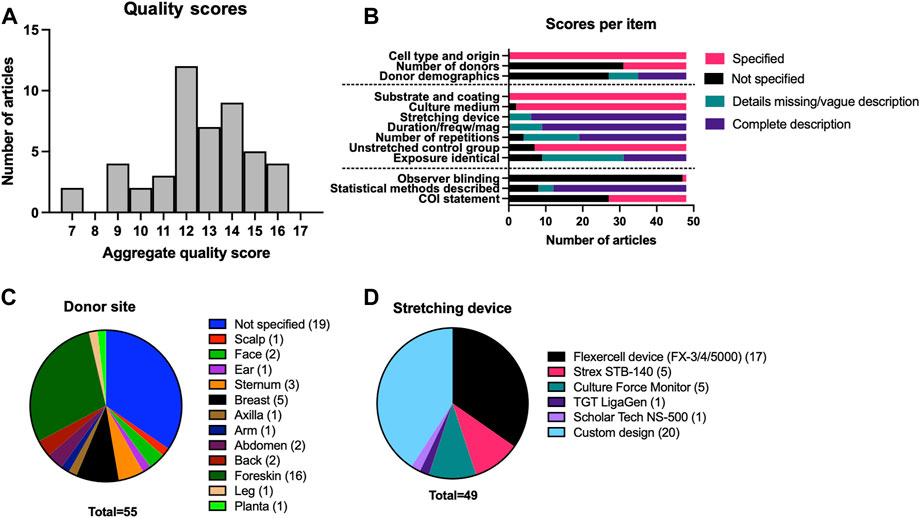
FIGURE 2. Overview of the quality assessment and a detailed description of methodology for included papers. (A) Distribution of quality assessment scores among the articles found through systematic review. (B) Reporting of specific categories in included papers. Cell origin refers to the type of skin i.e., healthy skin or scar. Demographics include age and sex. (C) Frequency of reporting of specific donor-site locations. (D) Frequency of use for different stretching devices in selected papers.
2.3 Data extraction and processing
Two outcome measures were chosen based on reporting frequency and relevance to the molecular mechanism of mechanotransduction: fibroblast proliferation and collagen production. This resulted in the inclusion of 20 studies for quantitative synthesis, 19 for fibroblast proliferation (Supplementary Table S1) and 10 for collagen synthesis (Supplementary Table S2). Two frequently reported outcomes measures, matrix reorganization or reorientation and sample contraction, were reported too heterogeneously and no scale-free overall effect size could be determined. Reporting of cell proliferation varied, with some giving detailed growth curves and some only differences between the cell counts at a single time point. Therefore, to combine the data from cell proliferation datasets we estimated the difference between the growth constants in experimental (ks) and control (kc) conditions under the assumption of exponential growth as ks-kc =
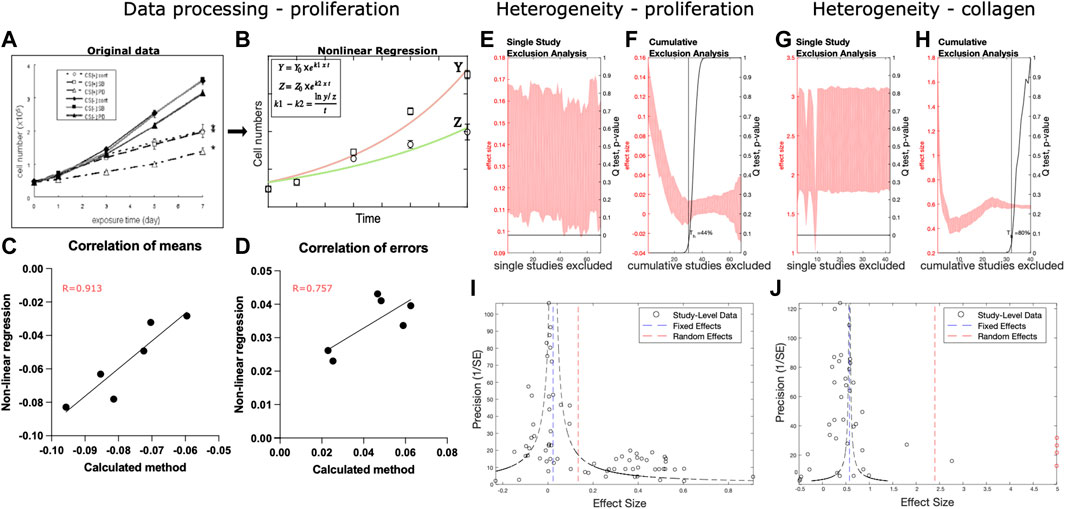
FIGURE 3. Data processing and heterogeneity analysis. (A–D) Overview of processing and validation of cell proliferation data that starts from original graph (A), reproduced with permission from Nishimura et al. (Nishimura et al., 2007)), which was fited with exponential growth curves for control Y and experimental group Z (B). The difference between growth constants obtained from fitting was compared to the ks - kc calculated from a single time point as correlation for means (C) and standard deviation (D). (E–H) Heterogeneity analysis was performed for the cell proliferation (E, F) and collagen production (G, H) using single (E, G) and cumulative (F, H) study exclusion. (I, J) Publication bias and effect size distributions were assessed using funnel plots for cell proliferation (I) and collagen production (J) datasets. Study level data is indicated by black circles. The outliers in the collagen dataset are indicated with red circles.
2.4 Analysis of heterogeneity
Heterogeneity was analyzed in both datasets. Single-study exclusion analysis of the fibroblast proliferation dataset did not affect the overall effect size of the outcome (Figure 3E). Cumulative study exclusion analysis determined that 44% of studies needed to be excluded to obtain a homogenous dataset and their removal would result in a decreased effect size (Figure 3F). In the collagen production dataset, single study exclusion analysis identified three studies that contributed to heterogeneity, and their exclusion affected the overall effect size of the outcome (Figure 3G). Cumulative study exclusion analysis demonstrated that 80% of the studies needed to be excluded to attain heterogeneity (Figure 3H). The removal of these studies resulted in a decreased effect size. Funnel plot analysis of the fibroblast proliferation dataset (Figure 3I) demonstrated the bi-modal distribution of the study level effect data points with one peak around the fixed-effects estimate and another one at the higher values. Funnel plot of the collagen production dataset (Figure 3J) demonstrated that the study level effects were distributed around the fixed-effects estimate.
2.5 Meta-analysis
2.5.1 Fibroblast proliferation
Since the distribution of study level effects in fibroblast proliferation showed an apparent division between two groups (Figure 3), we examined if two separate populations in this dataset corresponded to fibroblast donor age. The reporting of donor age allowed to separate data from neonatal and adult donors, while the studies in which donor age was not properly reported were combined together (Figure 4). Neonatal fibroblasts showed an apparent insensitivity to stretch-induced proliferation (Ks-Kc: 0.00[CI:−0.01:0.01]) while adult fibroblasts exhibited a clear increase in proliferation after mechanical stretch (Ks-Kc: 0.14[CI:0.12:0.16]). In the group of non-reported age, fibroblast proliferation showed the same insensitivity to stretch-induced proliferation as neonatal fibroblasts (Ks-Kc: 0.01[CI:−0.01:0.03]). While neonatal cell responded more to uniaxial than to biaxial stretch, adult cells showed the opposite response, with an extensive response to biaxial stretch (Figures 4A, B, D). Proliferation of neonatal cells was not affected by Vitamin C, however a very small subset of cells of non-reported age showed a positive effect of Vitamin C on stretch-induced proliferation. Unfortunately, none of the articles on adult cells described adding Vitamin C to the culture medium. While all the neonatal fibroblast originated from foreskin, adult fibroblasts were obtained from different locations including foreskin (Figure 4C). Although adult fibroblasts from different locations responded similarly to the stretch, notable outliers were breast skin and foreskin (however this was only one dataset), which demonstrated a remarkable low stretch-induced proliferation compared to other donor site locations.
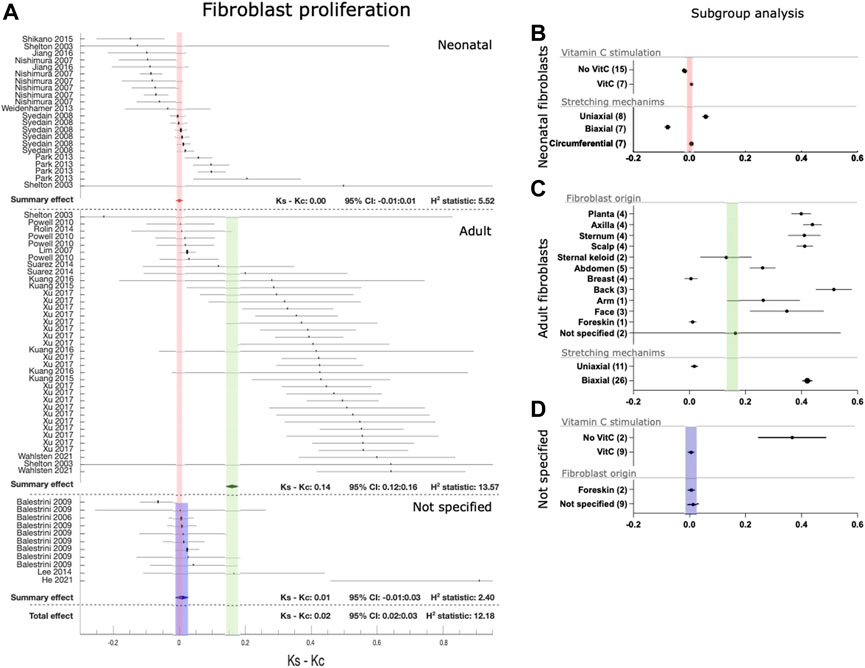
FIGURE 4. Effect of mechanical stretching on fibroblast proliferation. (A) The forest plot of the differences (ks - kc) between cell proliferation of cultured human dermal fibroblasts in mechanically stretched samples (ks) and unstretched controls (kc). Studies are separated by donor’s age as neonates, adults and not specified. Black circles/lines indicate study-level effect sizes with 95% CI. Diamonds/shaded bands indicate overall effect sizes with 95% CI for neonates (red), adults (green) and not specified studies (blue). In case a research article is mentioned multiple times, these constitute discrete exeperiments. (B–D) Subgroup analysis for fibroblasts of neonatal (B), adult (C), and non-specified (D) age groups for the use of vitamin C stimulation during culture (neonatal and non-specified), skin origin of fibroblasts (adult and non-specified) and different stretching mechanisms (neonatal and adult). Black circles/lines indicate effect size and 95% CI within subgroups, with the number of included datasets indicated in the parentheses, shaded bands indicate the 95% CI for the overal effect for neonates (red), adults (green) and studies with unspecified donor age (blue).
2.5.2 Collagen production
The collagen production dataset contained a small number of studies that demonstrated effect sizes that were orders of magnitude larger than the rest of the dataset (Figure 5). The ROUT method was thus used to identify five outliers in the collagen dataset that were subsequently omitted from further analysis. Overall, mechanical stretching increased collagen production (NMR: 0.44[CI:0.44:0.45]). Consistent with its known function, addition of Vitamin C almost doubled the stimulatory effect of mechanical stretching. Foreskin fibroblast derived from neonatal donors also produced more collagen in response to mechanical stretching. Collagen production was evenly stimulated by uniaxial and biaxial stretching, and an even greater effect was seen in circumferentially stretched fibroblasts.
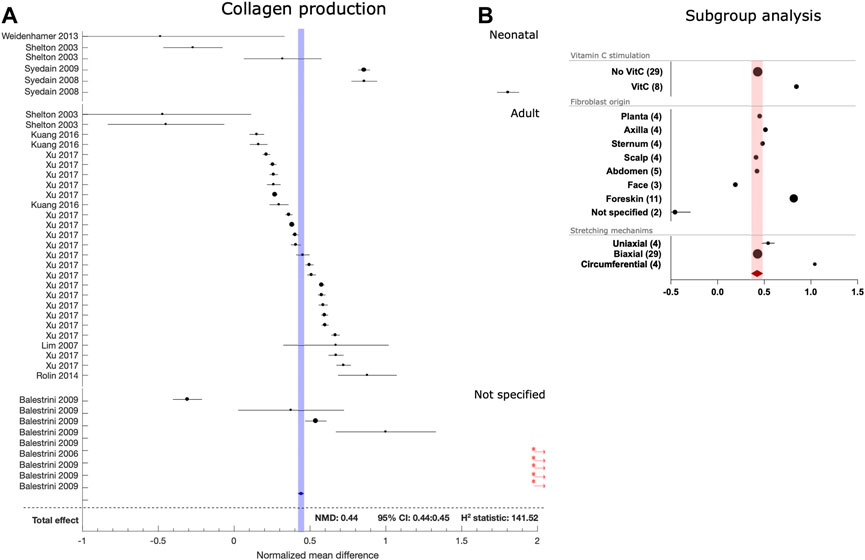
FIGURE 5. Effect of mechanical stretching on collagen prodution by fibroblasts. (A) The forest plot for the normalized mean differences between collagen production by mechanically stretched and control human dermal fibroblasts. Studies are stratified according to age of fibroblast donors. Black circles/lines indicate study level effect sizes with 95% CI. Diamonds/shaded bands indicate overall effect sizes with 95% CI. Red asterisks and arrows indicate outliers that were not included in the overall effect size calculation. (B) Subgroup analysis for the use of vitamin C stimulation during culture, skin origin of fibroblasts and different stretching mechanisms. Black circles/lines indicate effect size and 95% CI within subgroups, with the number of included datasets indicated in the parentheses, shaded bands indicate the 95% CI for the overall effect.
2.6 Meta-regression
The effects of the continuous covariates strain magnitude and frequency on stretch-induced fibroblast proliferation and collagen production was investigated using a fixed-effects meta-regression model. Stretch-induced proliferation (Figures 6A, B, D) and stretch-induced collagen production (Figures 6C, E) positively correlated with strain magnitude in both neonatal and adult cells. However, in all three cases, the slope of the association was minimal, and goodness of fit was poor. Inspection of the plots suggests a non-linear association between increasing magnitude and proliferation, with an initial increase and subsequent downward slope toward 20% strain. An increased strain frequency was strongly and positively associated with an increase in stretch-induced proliferation in neonatal cells (Figure 6B), and strongly and negatively associated with stretch induced collagen production (Figure 6E).
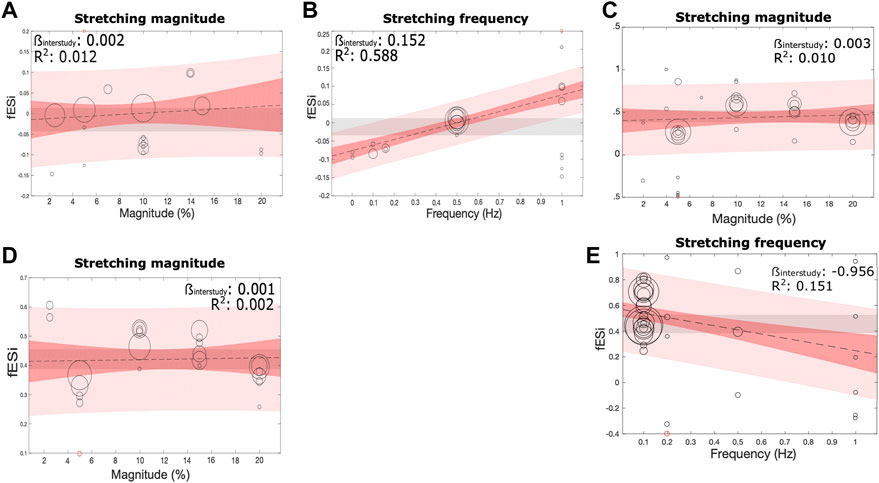
FIGURE 6. Effect of the parameters of mechanical stretching on the fibroblast proliferation and collagen production. The relationship between (A, C, D) stretching magnitude (%) and (B, E) frequency (Hz), and fibroblast proliferation in cultures of neonatal (A, B) and adult (D) origin, or collagen production (C, E) were assessed using a fixed-effects linear meta-regression model. Meta-regression is shown as a black line, with the slope (βinter) and goodness of fit (R2) indicated. 95% CI and prediction intervals are indicated by dark and light shaded red bands, respectively.
3 Discussion
This study constitutes the first systematic review and meta-analysis of in vitro studies assessing mechanobiology of human fibroblasts. The primary goal was to characterize the effect of in vitro mechanical stretch on human dermal fibroblasts. Two parameters, fibroblast proliferation and collagen deposition, were studied in a sufficient number of studies to perform a meta-analysis.
In total, 71 and 42 discrete experimental estimates on cell proliferation and collagen production were extracted, respectively. We found, for the first time, that only fibroblasts from adult donors increased both their proliferation and collagen production in response to mechanical stretch, while neonatal fibroblasts mainly responded by increasing their collagen production.
Meta-analysis on this data demonstrated the absence of stretch-induced proliferation in neonatal fibroblasts, while this effect was certainly present for adult fibroblasts. Scarless wound healing in the human fetus is an established fact (Larson et al., 2010). Our results seem to suggest that age-related difference in dermal fibroblast’s reactivity to mechanical tension may exist for a longer duration than previously thought.
In contrast, dermal fibroblasts from donors of all ages showed an increase in production of collagen type I in response to mechanical stimulation, although the degree of increase was variable, partially due to the differences in the use of vitamin C during culture. Unfortunately, other types of collagens were not reported abundantly enough to perform a quantitative synthesis, making an analysis of other types of collagen relevant to scar formation impossible (Oliveira et al., 2009).
We have found that stretch magnitude and the frequency of stretching differentially affected fibroblast proliferation and collagen secretion. Although there was no significant correlation between the stretch magnitude and fibroblast function, detailed data examination suggested that the relationship may be non-linear. In contrast, stretch frequency significantly correlated with both fibroblast proliferation (positive correlation) and collagen production (negative correlation). These data may explain the dichotomous effects of observed in clinical studies (Schouten et al., 2011; Wong et al., 2011). Thus, our studies propose a new direction of research in identifying an optimal mechanical stretching protocol to reduce scarring.
This study has several limitations. First, the quality of the 48 identified articles was critically appraised using a modified scoring system based on previous work by Golbach et al. (2016), and several important flaws were identified. Reporting of the demographic characteristics of fibroblast donors was particularly poor and seldom contained information on donor sex, age, and fibroblast site location. Moreover, the number of donors used per experiment were reported only in a minority of articles. Improved reporting on the number of donors will increase the generalizability of results. Similarly, increased reporting on donor characteristics will allow for more in-depth assessment of their role in scar formation. Second, we have identified deficiencies in reporting of experimental conditions. A minority of articles unequivocally stated that the conditions in the control experiments were identical to the experimental group. Cell passage numbers were not consistently reported, despite their known impact on proliferation. Moreover, although the majority of articles described clear samples sizes, a vague description or range of sample sizes was still a problem in many papers. Finally, 27 out of 48 articles did not include a conflict-of-interest statement. These findings emphasize the need for better reporting of basic science studies and the requirements of a specific quality assessment tools for the synthesis studies. Even though the articles included for quantitative syntheses had relatively high scores for reporting quality (Supplementary Tables S1, S2), reported conclusions are subject to the deficiencies in reporting identified above.
Furthermore, to control the scope, this study solely investigated the effect of mechanical strain given its predominant role, even though other types of mechanical deformation such as shear and compression may play important roles. We were also limited in the number of analyzed outcomes. More specifically, we were not able to include matrix reorganization and fibroblast differentiation due to lack of consistent reporting of the outcomes. Similarly, even though fibroblast subpopulations (e.g. reticular versus papillary fibroblasts) are thought to behave differentially in scar formation (Woodley, 2017), we could not assess a potential difference due to a lack of data.
To conclude, this study decisively demonstrated the stimulatory effect of mechanical stretching on human dermal fibroblast proliferation and collagen production and identified donor age and the frequency of mechanical stretching as important determinants of the outcome. Our study uniquely suggests that reducing proliferation while simultaneously increasing collagen production by adult cells may be beneficial for mimicking the mechanical stretching response in neonatal cells. Our analysis also suggests that potential strategies to achieve this may include treatment with ascorbic acid. These results provide concrete recommendations for future experimental research to further elucidate the physiological mechanism behind stretch-induced scarring, for the development of novel treatment strategies, and in optimization of tissue engineering protocols.
4 Methods
4.1 Software
Articles retrieved through database search were imported into EndNote X9 (Clarivate Analytics, Philadelphia, United States) for duplicate removal. The web application Rayyan (Ouzzani et al., 2016) was used for title and abstract screening. META-LAB (Mikolajewicz and Komarova, 2019), a plug-in developed in MATLAB R2016b was used for data extraction, meta-analysis, and meta-regression. Data fitting and statistics were carried out in GraphPad Prism9 (SanDiego, CA, United States) and Microsoft Excel was used for further data processing. The open-source graphics editing software Inkscape was used to edit plots and figures.
4.2 Search strategy
A systematic search of available literature was performed in the following databases: PubMed, Embase.com, Clarivate Analytics/Web of Science Core Collection on 1 June 2020. The systematic search was updated on 26 May 2021. The full search strategy can be found in Supplementary Table S3. Additional articles were identified by checking references. The PRISMA statement was followed for reporting in this systematic review (Moher et al., 2009), with the exception of protocol registration as basic science reviews are not accepted in protocol registries.
4.3 Selection of literature
Title and abstract screening were performed individually by two authors, LvH and YM. Disagreement on individual cases was resolved through discussion by the two authors. A third author (MLG) was available in case of unresolvable differences. Studies were included if the experimental protocol involved exposing human dermal or scar fibroblasts to mechanical stretch. Exclusion criteria were as follows: language other than English, animal studies, clinical studies, and mechanical stimulation that was based on other mechanisms (e.g., pressure). Reviews, letters, editorials, and conference abstracts were also excluded.
4.4 Assessment of methodological quality
Studies were ranked for quality using an assessment tool based on a tool previously described by (Golbach et al., 2016). The assessment included twelve items on the experimental setup, intervention, and reporting characteristics (Supplementary Table S4). Items were scored numerically based on the “completeness” of reporting in the relevant studies, in which only the quality of the experiments that fall within the scope of this review were assessed. Total aggregate quality scores were calculated at the study level. Assessment of methodological quality was done separately by two authors (LvH and YdJ), and mutual agreement was sought on divergent scores by discussion between these two authors.
4.5 Data extraction
Studies were included for quantitative synthesis when data on cell proliferation or collagen deposition were provided for both intervention and control groups. Data was extracted concerning experimental design, methodology, outcome measures, and results. The following results were exported into an Excel spreadsheet: sample size, means, and standard errors/deviations. When a range of sample sizes was provided, the lowest value was used. When sample size was not explicitly stated, n = 3 was assumed. In the case of unreported measure of variance, it was assumed to be a standard error. Graphical data was extracted using the META-LAB toolbox for basic research (Mikolajewicz and Komarova, 2019). When data was insufficient or unclear, the authors were contacted to elucidate the data.
4.6 Outcome measures and effect sizes
Two outcome measures were sufficiently reported for quantitative analysis: 1) cell proliferation and 2) collagen type I production. When collagen subtypes were reported, type I collagen data was extracted. When subtypes were not specified, overall collagen volumes were extracted.
4.6.1 Study-level outcome for cell proliferation
To account for differences in the duration of intervention and methodology, cell proliferation datasets were treated as follows. We assumed that cell growth occurs exponentially as
4.6.2 Study level outcome for collagen production
Normalized mean differences θi were calculated as θi =
4.7 Heterogeneity analysis
Heterogeneity was calculated using H2 and I2 statistic. The H2 statistic is independent of the number of datasets and is calculated as
4.8 Meta-analysis
Random effects models are generally preferred in basic research (Mikolajewicz and Komarova, 2019) because of high amounts of experimental and biological variability. However, given the clear distribution of study level effects around the fixed effects estimate for both outcomes, a fixed effects model was used to plot subsequent forest plots.
The study-level outcomes were used to generate a forest plot to achieve a summary outcome,
4.9 Subgroup analysis and meta-regression
Subgroup analysis and meta-regression (Mikolajewicz and Komarova, 2019) were carried out to identify factors related to cell donor, donor-site, experimental conditions, and stretching parameters that account for heterogeneity and influence the overall outcomes. Subgroup analysis was used for categorical variables. A fixed-effects meta-regression model was used when sufficient data for continuous variables was present was employed:
Author contributions
Conceptualization: LvH, CD, YdJ, PvZ MG, SK Data Curation: LvH, CD, YM, YdJ, JK, SK Formal Analysis: LvH, CD, YM, YdJ, JK, MG, SK Funding Acquisition: PvZ, MG, SK Investigation: LvH, CD, SK Methodology: LvH, CD, SK Project Administration: LvH, SK Resources: LvH, CD, JK, SK Software: LvH, CD, JK, SK Supervision: PvZ, MG, SK Validation: LvH, CD, IK, SK Visualization: LvH, CD, SK Writing - Original Draft Preparation: LvH, CD, YM, IK, YdJ, PvZ, MG, and SK Writing—Review and Editing: LvH, CD, YM, IK, YdJ, JK, PvZ, MG, and SK.
Funding
The collaboration project is co-funded by the PPP Allowance made available by Health∼Holland, Top Sector Life Sciences and Health, to stimulate public-private partnerships as well as by a contribution from the Dutch Burn Foundation (Grant number: TKI 16.01). We also acknowledge funding by Natural Sciences and Engineering Research Council (NSERC, RGPIN-288253) to SK, YM is supported by the China Scholarship Council (CSC).
Conflict of interest
The authors declare that the research was conducted in the absence of any commercial or financial relationships that could be construed as a potential conflict of interest.
Publisher’s note
All claims expressed in this article are solely those of the authors and do not necessarily represent those of their affiliated organizations, or those of the publisher, the editors and the reviewers. Any product that may be evaluated in this article, or claim that may be made by its manufacturer, is not guaranteed or endorsed by the publisher.
Supplementary material
The Supplementary Material for this article can be found online at: https://www.frontiersin.org/articles/10.3389/fmech.2023.1049659/full#supplementary-material
Abbreviation
ECM, extracellular matrix; ROUT, robust regression and outlier removal.
References
Al-Mubarak, L., and Al-Haddab, M. (2013). Cutaneous wound closure materials: An overview and update. J. Cutan. Aesthet. Surg. 6 (4), 178. doi:10.4103/0974-2077.123395
Balestrini, J. L., and Billiar, K. L. (2006). Equibiaxial cyclic stretch stimulates fibroblasts to rapidly remodel fibrin. J. Biomech. 39 (16), 2983–2990. doi:10.1016/j.jbiomech.2005.10.025
Barnes, L. A., Marshall, C. D., Leavitt, T., Hu, M. S., Moore, A. L., Gonzalez, J. G., et al. (2018). Mechanical forces in cutaneous wound healing: Emerging therapies to minimize scar formation. Adv. Wound Care 7 (2), 47–56. doi:10.1089/wound.2016.0709
Golbach, L. A., Portelli, L. A., Savelkoul, H. F. J., Terwel, S. R., Kuster, N., de Vries, R. B. M., et al. (2016). Calcium homeostasis and low-frequency magnetic and electric field exposure: A systematic review and meta-analysis of in vitro studies. Environ. Int. 92–93, 695–706. doi:10.1016/j.envint.2016.01.014
Guharay, F., and Sachs, F. (1984). Stretch-activated single ion channel currents in tissue-cultured embryonic chick skeletal muscle. J. Physiol. 352 (1), 685–701. doi:10.1113/jphysiol.1984.sp015317
He, J., Fang, B., Shan, S., Xie, Y., Wang, C., Zhang, Y., et al. (2021). Mechanical stretch promotes hypertrophic scar formation through mechanically activated cation channel Piezo1. Cell Death Dis. 12 (3), 226. doi:10.1038/s41419-021-03481-6
Hinz, B. (2016). The role of myofibroblasts in wound healing. Curr. Res. Transl. Med. 64 (4), 171–177. doi:10.1016/j.retram.2016.09.003
Hooijmans, C. R., IntHout, J., Ritskes-Hoitinga, M., and Rovers, M. M. (2014). Meta-analyses of animal studies: An introduction of a valuable instrument to further improve healthcare. ILAR J. 55 (3), 418–426. doi:10.1093/ilar/ilu042
Jiang, M., Qiu, J., Zhang, L., Lu, D., Long, M., Chen, L., et al. (2016). Changes in tension regulates proliferation and migration of fibroblasts by remodeling expression of ECM proteins. Exp. Ther. Med. 12 (3), 1542–1550. doi:10.3892/etm.2016.3497
Krishnan, N. M., Brown, B. J., Davison, S. P., Mauskar, N., Mino, M., Jordan, M. H., et al. (2016). Reducing wound tension with undermining or imbrication—Do they work? Plast. Reconstr. Surg. - Glob. Open. 4 (7), 1–6. doi:10.1097/gox.0000000000000799
Kuang, R., Wang, Z., Xu, Q., Cai, X., and Liu, T. (2016). Exposure to varying strain magnitudes influences the conversion of normal skin fibroblasts into hypertrophic scar cells. Ann. Plast. Surg. 76 (4), 388–393. doi:10.1097/sap.0000000000000654
Kuang, R., Wang, Z., Xu, Q., Liu, S., and Zhang, W. (2015). Influence of mechanical stimulation on human dermal fibroblasts derived from different body sites. Int. J. Clin. Exp. Med. 8 (5), 7641–7647.
Larson, B. J., Longaker, M. T., and Lorenz, H. (2010). Scarless fetal wound healing: A basic science review. Plast. Reconstr. Surg. [Internet 126 (3), 1172–1180. Available from. doi:10.1097/prs.0b013e3181eae781https://www.ncbi.nlm.nih.gov/pmc/articles/PMC3624763/pdf/nihms412728.pdf
Lee, E. J., Holmes, J. W., and Costa, K. D. (2008). Remodeling of engineered tissue anisotropy in response to altered loading conditions. Ann. Biomed. Eng. 36 (8), 1322–1334. doi:10.1007/s10439-008-9509-9
Lim, S. W., Son, Y., Kim, C. H., Shin, H., Kim, J. I., Shin, H., et al. (2007). The effect of a long-term cyclic strain on human dermal fibroblasts cultured in a bioreactor on chitosan-based scaffolds for the development of tissue engineered artificial dermis. Macromol. Res. 15 (4), 370–378. doi:10.1007/bf03218801
Marsidi, N., Vermeulen, S. A. M., Horeman, T., and Genders, R. E. (2020). Measuring forces in suture techniques for wound closure. J. Surg. Res. 255, 135–143. doi:10.1016/j.jss.2020.05.033
Martino, F., Perestrelo, A. R., Vinarský, V., Pagliari, S., and Forte, G. (2018). Cellular mechanotransduction: From tension to function. Front. Physiol. 9, 824–921. doi:10.3389/fphys.2018.00824
McKee, T. J., Perlman, G., Morris, M., and Komarova, S. V. (2019). Extracellular matrix composition of connective tissues: A systematic review and meta-analysis. Sci. Rep. [Internet] 9 (1), 10542–10615. Available from:. doi:10.1038/s41598-019-46896-0
Mikolajewicz, N., and Komarova, S. V. (2019). Meta-analytic methodology for basic research: A practical guide. Front. Physiol. 10, 203. doi:10.3389/fphys.2019.00203
Mikolajewicz, N., Mohammed, A., Morris, M., and Komarova, S. V. (2018). Mechanically stimulated ATP release from mammalian cells: Systematic review and meta-analysis. J. Cell Sci. 131 (22), jcs223354. doi:10.1242/jcs.223354
Moher, D., Liberati, A., Tetzlaff, J., and Altman, D. G. (2009). Preferred reporting items for systematic reviews and meta-analyses: The PRISMA statement. PLoS Med. 6, e1000097. doi:10.1371/journal.pmed.1000097
Nishimura, K., Blume, P., Ohgi, S., and Sumpio, B. E. (2007). Effect of different frequencies of tensile strain on human dermal fibroblast proliferation and survival. Wound Repair Regen. 15 (5), 646–656. doi:10.1111/j.1524-475x.2007.00295.x
Ogawa, R. (2017). Keloid and hypertrophic scars are the result of chronic inflammation in the reticular dermis. Int. J. Mol. Sci. 18 (3), 606. doi:10.3390/ijms18030606
Oliveira, G. V., Hawkins, H. K., Chinkes, D., Burke, A., Tavares, A. L. P., Ramos-E-Silva, M., et al. (2009). Hypertrophic versus non hypertrophic scars compared by immunohistochemistry and laser confocal microscopy: Type i and III collagens. Int. Wound J. 6 (6), 445–452. doi:10.1111/j.1742-481x.2009.00638.x
Ouzzani, M., Hammady, H., Fedorowicz, Z., and Elmagarmid, A. (2016). Rayyan-a web and mobile app for systematic reviews. Syst. Rev. 5 (1), 210–10. doi:10.1186/s13643-016-0384-4
Park, K. S., Lee, E. G., and Son, Y. (2014). Uniaxial cyclic strain stimulates cell proliferation and secretion of interleukin-6 and vascular endothelial growth factor of human dermal fibroblasts seeded on chitosan scaffolds. J. Biomed. Mater Res. - Part A 102 (7), 2268–2276. doi:10.1002/jbm.a.34881
Powell, H. M., McFarland, K. L., Butler, D. L., Supp, D. M., and Boyce, S. T. (2010). Uniaxial strain regulates morphogenesis, gene expression, and tissue strength in engineered skin. Tissue Eng. Part A 16 (3), 1083–1092. doi:10.1089/ten.tea.2009.0542
Rolin, G., Binda, D., Tissot, M., Viennet, C., Saas, P., Muret, P., et al. (2014). In vitro study of the impact of mechanical tension on the dermal fibroblast phenotype in the context of skin wound healing. J. Biomech. 47 (14), 3555–3561. doi:10.1016/j.jbiomech.2014.07.015
Schouten, H. J., Van Zuijlen, P. P., and Nieuwenhuis, M. K. (2011). A review on static splinting therapy to prevent burn scar contracture: Do clinical and experimental data warrant its clinical application? Burns 37, S7. doi:10.1016/s0305-4179(11)70025-0
Shelton, J. C., Bader, D. L., and Lee, D. A. (2003). Mechanical conditioning influences the metabolic response of cell-seeded constructs. Cells Tissues Organs 175 (3), 140–150. doi:10.1159/000074630
Shikano, K., Chiba, K., and Miyata, S. (2015). Response of human skin fibroblasts to mechanical stretch in wound healing process analyzed using a three-dimensional culture model. Adv. Biomed. Eng. 4, 170–178. doi:10.14326/abe.4.170
Shirakami, E., Yamakawa, S., and Hayashida, K. (2020). Strategies to prevent hypertrophic scar formation: A review of therapeutic interventions based on molecular evidence. Burn Trauma 8, tkz003. doi:10.1093/burnst/tkz003
Son, D. O., and Hinz, B. (2021). A rodent model of hypertrophic scarring: Splinting of rat wounds. Methods Mol. Biol. 2299, 405–417. doi:10.1007/978-1-0716-1382-5_27
Suarez, E., Syed, F., Rasgado, T. A., Walmsley, A., Mandal, P., and Bayat, A. (2014). Skin equivalent tensional force alters keloid fibroblast behavior and phenotype. Wound Repair Regen. 22 (5), 557–568. doi:10.1111/wrr.12215
Syedain, Z. H., and Tranquillo, R. T. (2009). Controlled cyclic stretch bioreactor for tissue-engineered heart valves. Biomaterials 30 (25), 4078–4084. doi:10.1016/j.biomaterials.2009.04.027
Syedain, Z. H., Weinberg, J. S., and Tranquillo, R. T. (2008). Cyclic distension of fibrin-based tissue constructs: Evidence of adaptation during growth of engineered connective tissue. Proc. Natl. Acad. Sci. U. S. A. 105 (18), 6537–6542. doi:10.1073/pnas.0711217105
Tsuge, T., Aoki, M., Akaishi, S., Dohi, T., Yamamoto, H., and Ogawa, R. (2020). Geometric modeling and a retrospective cohort study on the usefulness of fascial tensile reductions in severe keloid surgery. Surg. (United States) 167 (2), 504–509. doi:10.1016/j.surg.2019.07.028
Wahlsten, A., Rütsche, D., Nanni, M., Giampietro, C., Biedermann, T., Reichmann, E., et al. (2021). Mechanical stimulation induces rapid fibroblast proliferation and accelerates the early maturation of human skin substitutes. Biomaterials 273, 120779. doi:10.1016/j.biomaterials.2021.120779
Weidenhamer, N. K., and Tranquillo, R. T. (2013). Influence of cyclic mechanical stretch and tissue constraints on cellular and collagen alignment in fibroblast-derived cell sheets. Tissue Eng. Part C Methods 19 (5), 386–395. doi:10.1089/ten.tec.2012.0423
Wong, V. W., Akaishi, S., Longaker, M. T., and Gurtner, G. C. (2011). Pushing back: Wound mechanotransduction in repair and regeneration. J. Invest. Dermatol 131 (11), 2186–2196. doi:10.1038/jid.2011.212
Woodley, D. T. (2017). Distinct fibroblasts in the papillary and reticular dermis: Implications for wound healing. Dermatol Clin. 35 (1), 95–100. doi:10.1016/j.det.2016.07.004
Keywords: skin, biomechanics, keloid, hypertrophic scar, mechanotransduction
Citation: van Haasterecht L, Dsouza C, Ma Y, Korkmaz HI, de Jong Y, Ket JCF, van Zuijlen PPM, Groot ML and Komarova SV (2023) In vitro responses of human dermal fibroblasts to mechanical strain: A systematic review and meta-analysis. Front. Mech. Eng 9:1049659. doi: 10.3389/fmech.2023.1049659
Received: 20 September 2022; Accepted: 23 January 2023;
Published: 06 February 2023.
Edited by:
Rebecca G. Wells, University of Pennsylvania, United StatesReviewed by:
José Sanz-Herrera, Universidad de Sevilla, SpainFeihu Zhao, Swansea University, United Kingdom
Copyright © 2023 van Haasterecht, Dsouza, Ma, Korkmaz, de Jong, Ket, van Zuijlen, Groot and Komarova. This is an open-access article distributed under the terms of the Creative Commons Attribution License (CC BY). The use, distribution or reproduction in other forums is permitted, provided the original author(s) and the copyright owner(s) are credited and that the original publication in this journal is cited, in accordance with accepted academic practice. No use, distribution or reproduction is permitted which does not comply with these terms.
*Correspondence: L. van Haasterecht, bC52YW5oYWFzdGVyZWNodEBhbXN0ZXJkYW11bWMubmw=
†ORCID: L. Van Haasterecht, orcid.org/0000-0003-3785-9217; C. Dsouza, orcid.org/0000-0001-9996-6494; H. I. Korkmaz, orcid.org/0000-0001-7356-0701; J. C. F. Ket, orcid.org/0000-0002-1909-3150; P. P. M. Van Zuijlen, orcid.org/0000-0003-3461-8848